- 1Department of Hematology, The Third Xiangya Hospital, Central South University, Changsha, China
- 2Department of Thoracic Surgery, Xiangya Hospital, Central South University, Changsha, China
- 3Hunan Engineering Research Center for Pulmonary Nodules Precise Diagnosis & Treatment, Changsha, China
- 4National Clinical Research Center for Geriatric Disorders, Changsha, China
- 5Department of Dermatology, The Third Xiangya Hospital, Central South University, Changsha, China
Sepsis, the most common life-threatening multi-organ dysfunction syndrome secondary to infection, lacks specific therapeutic strategy due to the limited understanding of underlying mechanisms. It is currently believed that inflammasomes play critical roles in the development of sepsis, among which NLRP3 inflammasome is involved to most extent. Recent studies have revealed that dramatic reprogramming of macrophage metabolism is commonly occurred in sepsis, and this dysregulation is closely related with the activation of NLRP3 inflammasome. In view of the fact that increasing evidence demonstrates the mechanism of metabolism reprogramming regulating NLRP3 activation in macrophages, the key enzymes and metabolites participated in this regulation should be clearer for better interpreting the relationship of NLRP3 inflammasome and sepsis. In this review, we thus summarized the detail mechanism of the metabolic reprogramming process and its important role in the NLRP3 inflammasome activation of macrophages in sepsis. This mechanism summarization will reveal the applicational potential of metabolic regulatory molecules in the treatment of sepsis.
Introduction
Sepsis is a complicated syndrome associated with physiological, pathological, and biochemical abnormalities induced by the infection that manifests as life-threatening multiorgan dysfunction (Singer et al., 2016). As a leading public health problem, sepsis is associated with more than $20 billion in total hospital costs in the United States (Torio and Andrews, 2006). The reported incidence of sepsis continues to increase due to the growing number of the aging population and greater awareness (Iwashyna et al., 2012; Gaieski et al., 2013). Although the understanding of the pathological mechanisms has been improved in recent years, targeted treatments are still scant for sepsis (Englert et al., 2019).
The pathogenesis of sepsis is complex, and innate immunity plays a key role. Macrophages are important innate immune cells that significantly affect the occurrence and development of sepsis due to their vital effects on immune homeostasis and the inflammatory response (Gordon and Taylor, 2005; Kumar, 2018; Chen et al., 2021). In sepsis, the metabolic status of macrophages is significantly different from their physiological status, and this abnormal metabolic status regulates their immune function. Pathogen-associated molecular patterns (PAMPs) or damage-associated molecular patterns (DAMPs) and other alarmins can activate macrophages. Activated macrophages suffer from alterations in metabolism and further aggravate or limit the inflammatory response (Chen et al., 2021). Therefore, changes in the metabolic status of macrophages play a complex role in defending against external pressures and stabilizing homeostasis.
Immunometabolism is an interdisciplinary subject combining classical immunology and metabolism and employs the experimental research advances and paradigms of the two fields. Immunometabolism includes two main aspects: cellular immunometabolism studies the changes in immune metabolism that determine the fate of immune cells under different conditions, and tissue immunometabolism studies the effects of immune cells on the metabolism of other tissues and the immune cell-induced regulation of individuals’ adaptation to the environment (Man et al., 2017; Kumar, 2018). Recent studies have shown that immunometabolism plays an important role in infection, tumorigenesis, and many autoimmune diseases (Gaber et al., 2017; Hotamisligil, 2017). Research on immunometabolism in sepsis has also received extensive attention. Metabolism-related enzymes and their intermediates play vital roles in the pathogenesis of sepsis by regulating nucleotide-binding domain, leucine-rich repeat, pyrin domain-containing protein 3 (NLRP3) inflammasome activation and other mechanisms. In this review, we summarized the metabolic reprogramming mechanisms of macrophages in sepsis, with the aim to shed light on novel targets and methods for the treatment of sepsis.
The Role of Macrophages in Sepsis
Monocytes and macrophages play important roles in host resistance to multiple pathogens and the inflammatory immune responses in sepsis (Lauvau et al., 2015; Kumar, 2018). During the early stage of sepsis, a variety of inflammatory signaling pathways in macrophages are activated, releasing a large number of inflammatory mediators and aggravating the progression of sepsis. During this process, M1 macrophages are dominant (Zubair et al., 2021). The late stage of sepsis, often accompanied by immunosuppression or paralysis, is mainly caused by the apoptosis of conventional T cells and the upregulation of regulatory T cells (Tregs), in which macrophages also play an important role (Zubair et al., 2021). This endotoxin tolerance exhibited by macrophages is the result of a phenotypic shift M1 to M2 macrophages or alternatively activated macrophages (AAMs), which contributes to the immunosuppression observed during the later stage of sepsis (Wang and Deng, 2008; Delano and Ward, 2016).
Studies have shown that in different stages of sepsis, especially in the early stage, the regulatory function of the metabolic pathway of macrophages is significantly changed (Artyomov et al., 2016; Arts et al., 2017). Macrophage glycolysis, which includes the pentose phosphate pathway (PPP), and the tricarboxylic acid (TCA) cycle are both altered during sepsis. Moreover, changes in cellular glucose metabolism pathways, such as in the key enzymes and intermediates, could impact the progression of sepsis by regulating NLRP3 inflammasome activation, multi-inflammatory factor release, and others (Kumar, 2018).
Macrophage Metabolic Reprogramming and Adaptation in Sepsis
Glucose metabolism is the core metabolic pathway that is mainly achieved by glycolysis, the PPP, and the TCA cycle. The following is a summary of changes in macrophage glycolysis and the TCA cycle in sepsis.
Glycolysis
Glycolysis is a key step in cellular glucose metabolism, enabling cells to obtain energy in the form of ATP. Glucose in the serum is transferred to cells by glucose transporters (GLUTs) and converted to pyruvate through a series of enzymatic reactions during anaerobic glycolysis (Freemerman et al., 2014). The resulting pyruvate can be converted to lactate by the anaerobic glycolysis pathway to produce 2 ATPs or converted to acetyl-CoA to enter the TCA cycle and ultimately produce 36 ATPs through oxidative phosphorylation (OxPhos). Although ATP production is consumed during glycolysis, it is could be quickly restored via glycolysis to fulfil the short-term energy. In contrast, the TCA cycle and OxPhos produce ATP at a relatively slow rate despite high production. The Warburg effect refers to the transformation of cell metabolism from OxPhos to glycolysis to produce ATP at a faster speed for cell energy requirements. This phenomenon was initially observed in tumor cells and has subsequently been demonstrated in activated immune cells, including macrophages (Hsu and Sabatini, 2008).
During sepsis, glycolysis in highly activated macrophages is significantly upregulated, and the molecular mechanisms are currently well-documented. Studies have shown that hypoxia-inducible factor (HIF-1α) plays a key role in promoting glycolysis, and the up-regulation of the Akt-mTOR signaling pathway targets HIF-1α to promote glycolysis in activated macrophages (Cheng et al., 2014). In addition, lipopolysaccharide (LPS), a key pathogenic factor of sepsis, could promote the expression of GLUT1 and GLUT3 and thus increase the glucose uptake of macrophages (Fukuzumi et al., 1996; Reddy et al., 2010). In addition, LPS has also been reported the expression of key enzymes in the glycolysis pathway, such as hexokinase (HK) and fructose 2,6-bisphosphate (F2,6BP) (Tavakoli et al., 2013). Likewise, zinc fingers and homeoboxes (Zhx2) have been confirmed to be involved in sepsis by promoting the expression of 6-phosphofructo-2-kinase/fructose-2,6-biphosphatase 3 (Pfkfb3), thereby increasing glycolysis (Wang et al., 2020). Lactate, the end product of glycolysis, is significantly accumulated in macrophages after LPS treatment (Yang et al., 2014). The increase of lactate caused by macrophage glycolysis is thought to partly contribute to the accumulation of lactate in tissues during sepsis. As a result, lactate is known as an independent risk factor for sepsis-inducing death (James et al., 1996; Gomez and Kellum, 2015). In addition, caspase-1 has been proven to be a key actuator of inflammation, pyroptosis, and sepsis. Studies have shown that caspase-1 can cleave several key enzymes in glycolysis, including aldolase, triose-phosphate isomerase, glyceraldehyde-3-phosphate dehydrogenase, alpha-enolase, and pyruvate kinase, which are further involved in the occurrence of sepsis and septic shock (Shao et al., 2007).
Based on the important role of macrophage glycolysis in the occurrence of sepsis as summarized above, the targeted regulation of glycolysis can impact the progression of the disease. Studies have shown that exosomes released from bone marrow mesenchymal stem cells (BMSCs) can reduce macrophage glycolysis by inhibiting HIF-1α, thereby attenuating sepsis-inducing lung injury (Deng et al., 2020). Likewise, glycolytic inhibitor 3PO could robustly improve the prognosis of acute lung injury (ALI)-induced by sepsis via reducing the lung inflammation and histopathological changes (Gong et al., 2017). 2-deoxy-D-glucose (2-DG), an inhibitor of aerobic glycolysis, has been found to alleviate sepsis-induced kidney injury by regulating autophagy (Tan et al., 2021). The molecular mechanism is related to the upregulated sirtuin 3 (SIRT3) and phosphorylation-AMP-activated protein kinase (p-AMPK). Xijiao Dihuang decoction (XJDHT) is a traditional Chinese medicine (TCM) formula. XJDHT is proven to restrain the activation of TLR4-HIF-1α signal pathway, reduce aerobic glycolysis and subsequently prompt the survival rate of sepsis (Lu et al., 2020).
In addition, PPP is also a vital metabolic pathway derived from glycolysis to achieve glucose oxygenolysis. PPP is divided into an oxidized branch and a non-oxidized branch, which can generate NADPH for fatty acid synthesis and ribose-5-phosphate for nucleoside synthesis. Moreover, the non-oxidized branch can transport metabolites back to glycolysis. Studies have shown that G6PD, a key enzyme in the PPP, was significantly upregulated in macrophages from a mouse sepsis model induced by intraperitoneal LPS injection (Arts et al., 2017).
Lipid Metabolism
Lipids included fatty acids, phospholipids, cholesterol and other different types of molecules (Meyers and Zhu, 2020). Besides of serving as important sources of energy when nutrients are limited or as a key component of different membranes, lipids, also are regarded as key regulators of macrophage function, especially in the presence of abnormal activation of inflammatory pathways, such as sepsis (Meyers and Zhu, 2020). The results of a metabolomics and proteomics study on 1000 sepsis patients’ plasma showed that, nine fatty acid transport proteins were reduced in non-survivors, indicated that fatty acid ß-oxidation is severely impaired in sepsis non-survivors (Langley et al., 2013). Thus, fatty acid metabolism could be one of the most promising metabolic biomarkers for predicting survival rate for sepsis patients (Langley et al., 2013). In rat sepsis model, several fatty acids were shown to be significantly increased in serum 2 h after cecal ligation (Wei et al., 2016).
Besides as a biomarker, the fatty acid has been explored as a potential treatment in sepsis. In two classic models of sepsis, murine cecal ligation (CLP) model and intraperitoneal injection with E. coli, adding exogenous stearoyl-GPC has been shown to decline the death rate in septic mice (Yan et al., 2004). Supplementation with ω-3-fatty acid supplements has been shown fewer secondary infections and shorter ICU time in sepsis patients than the patients without extra supplements (Galbán et al., 2000; Beale et al., 2008; Al-Biltagi et al., 2017).
Amino Acid Metabolism
Disorder of amino acid metabolism is also an important feature of sepsis. Different types of amino acids vary to different degrees in severe sepsis patients. the levels of 3-methyl-L-histidine, a-aminoadipic acid, a-amino-n-butyric acid, argininosuccinic acid, ß-aminoisobutyric acid, carnosine, cystathionine, glutamine, phenylalanine, and proline increased, while the levels of arginine, asparagine, aspartic acid, cystine, glutamic acid, leucine, serine, taurine, and tryptophan decreased in sepsis patients (Freund et al., 1979). A new study has found that level of branched-chain amino acids (BCAA) was significantly lower in sepsis non-survivors compared with survivors and may be a promising early biomarker for predicting outcome in sepsis patients (Reisinger et al., 2021). The prevailing interpretation of imbalance of amino acid levels in sepsis and the underlying mechanisms remain to be further investigated (Su et al., 2015; Reisinger et al., 2021).
TCA Cycle/OxPhos
The TCA cycle is an important part of glucose metabolism that connects different metabolic pathways. Pyruvate, the product of glycolysis, is the raw material of the TCA cycle. Pyruvate dehydrogenase catalyzes the conversion of pyruvate to acetyl-CoA, which subsequently enters the TCA cycle. The TCA cycle can produce a large amount of ATP to sustain normal body functions, and it is also a common pathway in glucose metabolism, lipid metabolism and amino acid metabolism. The metabolites of the TCA cycle can participate in the synthesis of various fats and amino acids.
As previously mentioned, LPS-activated macrophages change glucose metabolism, manifested by increased glycolysis and decreased TCA cycle/OxPhos. Although the TCA cycle was reduced, LPS-stimulated macrophages were found to be significantly enriched in various TCA cycle metabolites, including succinate, malate, fumarate, and others (Tannahill et al., 2013). Citrate and oxaloacetic acid were proven to be increased in the experimental rat model of Klebsiella pneumonia bacteremia (Dong et al., 2012). In addition, recent studies showed that itaconate, another intermediate of the TCA cycle, is significantly increased in LPS-activated macrophages (Bambouskova et al., 2018; Mills et al., 2018).
Key Enzymes and Metabolic Intermediates Emerging as Vital Players in Macrophage Functions in Sepsis
It has been summarized above that the metabolism of macrophages is changed in sepsis, and the altered metabolism will further affect their immune effect through key enzymes or intermediate metabolites. In the following section, we will summarize how the molecular mechanisms of the key enzymes and intermediate metabolites of glucose metabolism affect the immune effect of macrophages.
The Critical Processes of NLRP3 Inflammasome Activation
Inflammasomes are a class of pattern-recognition receptors (PPRs) located in the cytoplasm and were first detected in 2002 (Martinon et al., 2002). Inflammasomes are multi-protein complexes that can be classified as NLRP1, NLRP3, NLRC4, or AIM2 based on the different kinds of nucleotide-binding oligomerization domain (NOD)-like receptors (NLRs) (Xu et al., 2019). The NLRP3 inflammasome is composed of NLRP3 (sensor), ASC (adaptor), and caspase-1 (effector), which is the most intensely studied inflammasome currently. A previous study summarized the molecular mechanism of NLRP3 inflammasome activation and its research progress in inflammation-related diseases (Martinon et al., 2002).
It is believed that the progress of activation of the NLRP3 inflammasome requires two signals. The priming signal is composed of the upregulation of NLRP3 and proinflammatory cytokines such as IL-1β. The process is induced by the activation of NF-kB in the presence of microbial components or endogenous molecules. The robust activation of these molecules can elevate the expression of NLRP3. Recent studies have shown that the NLRP3 inflammasome is activated by a variety of stimuli, including PAMPs (LPS, nigericin, gramicidin, etc.) or DAMPs (ATP, cholesterol crystals, monosodium urate crystals, alum, silica, etc.), and many kinds of posttranscriptional modifications (PTMs) participate in the priming step. Among them, phosphorylation and ubiquitination were the most common (Fernandes-Alnemri et al., 2007; Lamkanfi, 2011).
The second signal, also known as the activating step, is currently obscured because of the diversity of NLRP3 activation stimuli. Many common cellular signals can trigger NLRP3 activation (Rathinam et al., 2012). Changes in intracellular and extracellular ion content, such as K+ or Ca2+, or mitochondrial dysfunction and lysosomal rupture, are all thought to be critical triggers of NLRP3 activation.
The activated NLRP3 inflammasome cleaved caspase-1 and promotes interleukin (IL)-1β maturation. Meanwhile, gasdermin D (GSDMD) is cleaved, which subsequently causes pore formation in the cell membrane and mediates cell pyroptosis. In addition to the canonical pathways, caspase-11 can also induce NLRP3 activation. Once LPS is delivered into the cytosol, Caspase-11, senses the cytosolic LPS and cleaves the substrate GSDMD and using the efflux of K+ to complete the activation of NLRP3 by inducing the opening of the pannexin-1 channel (Kayagaki et al., 2011). Recent studies revealed that these standard NLRP3 activation signals were also regulated by metabolic intermediates and enzymes, which we will discuss in the following section.
Key Enzymes and Metabolic Intermediates Regulating NLRP3 Inflammasome Activation
As mentioned above, the activated NLRP3 inflammasome mediates the release of inflammatory factors and the occurrence of pyroptosis, and therefore is thought to play an important role in the development and progression of sepsis.
Studies have shown that NLRP3 inflammasome expression and activation are significantly enhanced in the peripheral blood monocytes of septic patients (Esquerdo et al., 2017; Martínez-García et al., 2019). The knockout of NLRP3 or inhibition of its activation significantly reduces the release of inflammatory mediators, decreases multiple organ failure (MOF) in sepsis, and improves the survival rate of sepsis (Sutterwala et al., 2006; Mao et al., 2013; Danielski et al., 2020). Conversely, upregulation of NLRP3 inflammasome activation can aggravate MOF in sepsis and increase sepsis mortality (Shi et al., 2020). Likewise, our previous study also confirmed that regulation of NLRP3 inflammasome activation can affect the occurrence and development of sepsis (Yu et al., 2019a; Wang et al., 2021). Therefore, the NLRP3 inflammasome plays a key role in sepsis, and regulation of its activation can affect the progression of sepsis. Recently, an increasing number of studies have found that the key enzymes and metabolites of glucose metabolism in macrophages are involved in the pathogenesis of sepsis by regulating the activation of the NLRP3 inflammasome.
Studies have shown that HK1, an HK isozyme, upregulates NLRP3 inflammasome activation to augment the secretion of IL-1β and IL-18 in macrophages (Moon et al., 2015a). Based on previous studies showing that LPS treatment significantly promoted glycolysis in macrophages, researchers found that inhibition of glycolysis could reduce the production of the inflammatory factor IL-1β(Moon et al., 2015a). Jong-Seok Moon et al also found that the molecular mechanism of glycolysis impacting IL-1β production is attributed to the upregulation of NLRP3 inflammasome activation controlled by the mTORC1-dependent glycolysis metabolic pathway. mTORC1 promotes NLRP3 activation by promoting the expression of HK1, a key enzyme in the glycolysis pathway that converts glucose to glucose-6-phosphate, while suppression of HK1-dependent glycolysis restrains NLRP3 inflammasome activation (Moon et al., 2015a).
The M2 isoform of the pyruvate kinase muscle isozyme (PKM2), is a key rate-limiting enzyme in the glycolysis pathway, catalyzing the conversion of phosphoenolpyruvate and ADP to pyruvate and ATP. Studies have shown that the expression of PKM2 is significantly increased in LPS-stimulated macrophages (Palsson-McDermott et al., 2015a). Inhibiting PKM2 activation reduced IL-1β release and improved survival in septic mice while promoting PKM2 activation increased IL-1β levels and significantly reduced the host immune response in a sepsis model (Yang et al., 2014; Palsson-McDermott et al., 2015a). Subsequent studies have shown that PKM2 promotes IL-1β, IL-18, and HMGB1 release by upregulating the activation of the NLRP3 inflammasome (Xie et al., 2016). The molecular mechanism is related to the promotion of phosphorylation by PKM2. EIF2AK2 is closely related to NLRP3 inflammasome activation (Lu et al., 2012). In vivo, inhibition of PKM2-EIF2AK2 signaling protects mice from lethal endotoxemia and polymicrobial sepsis (Xie et al., 2016).
Lactate, a product of glycolysis, was shown to promote IL-1β and HMGB1 release in LPS-stimulated macrophages (Yang et al., 2014). Further studies revealed that the molecular mechanism was via the promotion of the activation of the NLRP3 inflammasome by lactate (Xie et al., 2016). Caspase-1 deficiency reduced the lactate-induced IL-1β release in macrophages under LPS stimulation, while both caspase-1 and caspase-11 deficiency completely blocked the IL-1β release induced by lactate after LPS treatment (Xie et al., 2016).
In addition, citrate is a metabolic intermediate of the TCA cycle and plays a critical role in cellular energy metabolism as a substrate. As mentioned above, citrate is enriched in LPS-treated macrophages. It has been recently found that enriched citrate can promote subsequent inflammatory responses (Duan et al., 2021). Citrate has been shown to increase the expression of NLRP3 and pro-IL-1β and subsequently augment NLRP3 inflammasome activation. Abnormal NLRP3 inflammasome activation was considered to then aggravate lung injury induced by LPS(Duan et al., 2021).
However, itaconate, a TCA cycle-related product, was found to reduce IL-1β and IL-18 release by inhibiting NLPR3 activation (Lampropoulou et al., 2016). Fumarate, an intermediate of the TCA cycle, accumulated in LPS-stimulated macrophages. Studies have shown that fumarate inhibits both canonical and noncanonical pathway activation by blocking the interaction of GSDMD with caspases, limiting the capacity to induce cell death (Humphries et al., 2020).
As indicated above, abnormally increased glycolysis-related enzymes and intermediate products of TCA cycle metabolism affect the inflammatory process of macrophages by regulating the activation of the NLRP3 inflammasome. Targeting the key signaling pathways is expected to lead to advances in sepsis treatment.
Besides glycolysis metabolism, key enzymes in lipid metabolism also play an invaluable role in regulating NLRP3 inflammasome activation. UCP2, which appears mainly in innate immune cells, has higher expression in sepsis patients compared with non-sepsis patients (Fleury et al., 1997; Moon et al., 2015b). In UCP2-deficient macrophages, lipid synthesis was suppressed due to the downregulation of fatty acid synthase (FASN) which is a key regulator of fatty acid synthesis (Moon et al., 2015b). UCP2-deficient or FASN inhibition suppressed NLRP3 inflammasomes activation by decreasing NLRP3 and pro-IL-1β gene expression in macrophages and has a protective effect on the endotoxin shock model and the CLP model (Moon et al., 2015b).
Other Mechanisms of Key Enzymes and Metabolic Intermediates Impacting Macrophage Functions in Sepsis
In addition to the NLRP3 inflammasome, key enzymes and metabolic intermediates of cellular glucose metabolism also affect macrophage function through a variety of molecular mechanisms, thus contributing to the occurrence and development of sepsis.
PKM2 not only regulates macrophage function by promoting NLRP3 inflammasome activation but also promotes macrophage activation through other signaling pathways. PKM2 was shown to interact with HIF-1α, and HIF-1α subsequently combined with the IL-1β promoter to induce IL-1β expression (Yang et al., 2014). PKM2 induces various proinflammatory factors and promotes the anti-inflammatory state of macrophages, also known as the M1 macrophage phenotype (Palsson-McDermott et al., 2015b).
Succinate, an intermediate of the TCA cycle, plays a key role in regulating macrophage activation. Studies have shown that succinate accumulation induced by LPS in macrophages contributes to the production of IL-1β. The molecular mechanism is that succinate stabilizes HIF-1α and further upregulates IL-1β transcription (Tannahill et al., 2013). In addition, Evanna L Mills et al. found that in LPS-stimulated macrophages, succinate dehydrogenase (SDH) induced the mitochondrial oxidation of succinate, which led to the production of mitochondrial reactive oxygen species (mtROS), an important proinflammatory mediator (Mills et al., 2016). Inhibition of succinate oxidation protected mice from endotoxin-induced lethalitye (Mills et al., 2016). However, succinate was shown to play a potential role in limiting inflammation. Succinate receptor 1 (SUNCR1) is an extracellular sensor of succinate. The myeloid-specific SUNCR1 knockout induced the expression of various pro-inflammatory factors, including IL-1β, while activated SUNCR1 led to an anti-inflammatory state and caused macrophage polarization (Keiran et al., 2019).
In addition, itaconate inhibits macrophage inflammation through a variety of molecular mechanisms, although it does not regulate NLRP3 inflammasome activation. Itaconate decreased the alkylation of Keap1 cysteine residues, which was related to the translocation and activation of Nrf2. Moreover, itaconate blocked the ATF3/eIF2a signaling pathway to restrain the production of proinflammatory mediators. In addition, accumulated IFN-β was shown to increase the accumulation of itaconate, while itaconate was shown to inhibit the expression of IFN-β to control the inflammatory response (Yu et al., 2019b).
Potential Anti-Sepsis Drugs Targeting Glucose Metabolic
It has been concluded that the glucose metabolism of macrophages is disturbed in sepsis, and glucose metabolism further influences the occurrence and development of sepsis by regulating NLRP3 inflammasome activation. Therefore, in recent years, more and more studies have found that a variety of drugs can play a therapeutic role in sepsis by interfering with glucose metabolism.
2‐deoxyglucose (2‐DG)
2-DG is a nonmetabolizable glucose analog that inhibits glycolysis by acting on a rate-limiting enzyme, hexokinase. The mechanism is that 2-DG is phosphorylated by hexokinase to 2-DG-P, which cannot be further metabolized to produce ATP. Based on the important role of glycolysis in sepsis, whether 2-DG can affect the development of sepsis is gradually being revealed. Studies showed that 2-DG treatment reduced pro-inflammatory factors expression, inflammatory cell accumulation, lung tissue pathological injury, and lethality in sepsis in vivo (Zhong et al., 2019). Further exploration found that 2-DG inhibited NLRP3 inflammasome activation in macrophages during sepsis (Zhong et al., 2019). Besides, 2-DG was proved to improve the survival of sepsis as well as reduce sepsis-related kidney, liver, and cardiac injury by suppressing IL-1β and lactate levels (Zheng et al., 2017). Chuyi Tan et al. found that sepsis-induced acute kidney injury could be alleviated by 2-DG via the lactate/SIRT3/AMPK pathway (Tan et al., 2021). To sum up, 2-DG plays an important role in sepsis and sepsis-related multi-organ dysfunction, which suggested that 2-DG has the potential to be a treatment for sepsis.
Terazosin
Terazosin, an alpha1-adrenergic receptor agonist, is mainly used for the treatment of benign prostatic hyperplasia in the elderly. Recently studies showed that terazosin increased sepsis survival rate and decreased organ injury in vivo. The molecular mechanism was that terazosin acted on the key glycolysis enzyme PGK1, which was a novel target (Chen et al., 2015). This study suggests that terazosin may be useful in the treatment of sepsis.
Insulin
Insulin is the only physiological glucose-lowering hormone and is often used in the treatment of diabetic patients. However, some research has revealed that insulin has anti-inflammatory effects. Pretreatment with insulin inhibited inflammatory response by suppressing the activation of NF-κB and expression of pro-inflammatory cytokines (Chen et al., 2014; Sun et al., 2014; Huang et al., 2020). A recent study also found that insulin inhibited NLRP3 inflammasomes activation by reducing the oligomerization of the ASC(Chang et al., 2020). Thus, insulin is a promising drug for sepsis.
Metformin
Metformin, as the first-line medication for the treatment of diabetes, inhibited NLRP3 inflammasome activation and IL-1β production in cultured and alveolar macrophages, thus attenuating LPS and SARS-CoV-2-induced acute respiratory distress syndrome (ARDS) (Xian et al., 2021). The result of phase II randomized controlled trial suggested that metformin dose not reduce the morbidity of severely burned patients who suffered sepsis, indicated further study is needed. Besides several infectious diseases, metformin has also been discovered to prevent NLRP3 inflammasome activity both in periodontitis (Li et al., 2016) and psoriasis (Tsuji et al., 2020).
Conclusion and Future Directions
This review summarizes changes in the immune metabolism of macrophages, which play a key role in sepsis (Figure 1). In this paper, two aspects are discussed: first, the changes of macrophage metabolism including glycolysis and TCA cycle in sepsis are summarized; next, we review the key enzymes and metabolites of altered cell metabolism that affect macrophage immune status by regulating NLRP3 inflammasome activation and other mechanisms; besides, the potential drugs targeted the immune metabolism to treat sepsis are summarized. This summary of the research progress on the immune metabolism of macrophages in sepsis is helpful to deeply understand the pathogenesis of sepsis and provide targets and a theoretical basis for the treatment of sepsis. However, the molecular mechanism of a large number of cellular metabolic enzymes and intermediates regulating macrophage immune status is still unclear. Further exploration of new molecular mechanisms is expected to allow for more specific and precise interventions to influence the immune response in macrophage metabolic disorders, improve the effectiveness of the interventions, and reduce side effects.
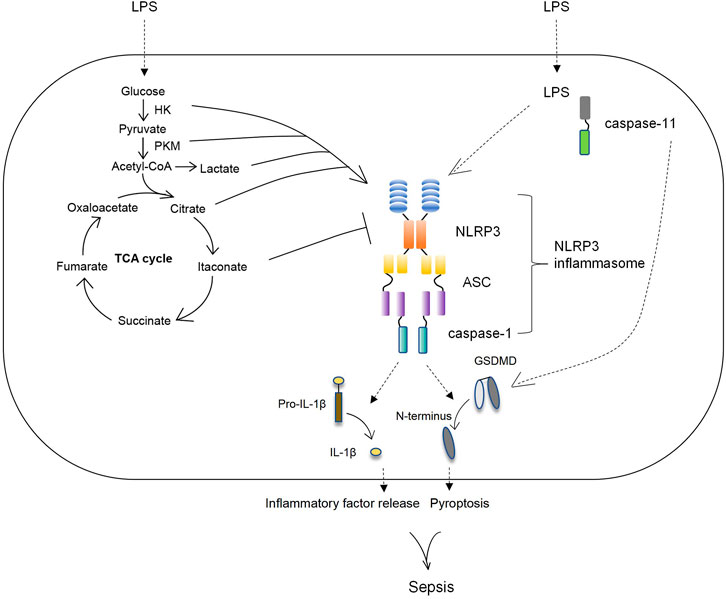
FIGURE 1. The key enzymes and metabolic intermediates participated in the activation of NLRP3 inflammasome. During sepsis the glucose metabolism of macrophage was changed, manifested by increased glycolysis and decreased TCA cycle/OxPhos as well as enriched various TCA cycle metabolites. Subsequently, the key enzymes and intermediates of glucose metabolism in macrophage regulated the activation of NLRP3 inflammasome to affect macrophage functions. HK and PKM, the key enzymes of glycolysis, positively upregulated NLRP3 inflammasome. Lactate, the metabolism of glycolysis, as well as citrate, the metabolism of TCA cycle, increased NLRP3 inflammasome activation. Itaconate, the intermediate of TCA cycle, downregulated NLRP3 inflammasome activation.
Author Contributions
RL researched the topic and wrote draft manuscripts. XL and DW contributed to discussions and drafting of the manuscript.
Funding
This work was supported by the Natural Science Foundation of Changsha (kq2014263), Hunan Science and Technology Department project (202204124082), Hunan Science and Technology Innovation Talent Program-Excellent Postdoctoral Innovation Talent Project (2021RC 2029), Outstanding Youth Foundation of Hunan Province (S2022JJYXQN0414).
Conflict of Interest
The authors declare that the research was conducted in the absence of any commercial or financial relationships that could be construed as a potential conflict of interest.
Publisher’s Note
All claims expressed in this article are solely those of the authors and do not necessarily represent those of their affiliated organizations, or those of the publisher, the editors and the reviewers. Any product that may be evaluated in this article, or claim that may be made by its manufacturer, is not guaranteed or endorsed by the publisher.
References
Al-Biltagi, M. A. M., Abo-Elezz, A. A. E., Abd-Elhafez, M. A., Mabrouk, M. M., and Suliman, G. A. (2017). Beneficial Effects of Omega-3 Supplement to the Enteral Feeding in Children with Mild to Moderate Sepsis. J. Intensive Care Med. 32 (3), 212–217. doi:10.1177/0885066615623927
Arts, R. J. W., Gresnigt, M. S., Joosten, L. A. B., and Netea, M. G. (2017). Cellular Metabolism of Myeloid Cells in Sepsis. J. Leukoc. Biol. 101 (1), 151–164. doi:10.1189/jlb.4mr0216-066r
Artyomov, M. N., Sergushichev, A., and Schilling, J. D. (2016). Integrating Immunometabolism and Macrophage Diversity. Seminars Immunol. 28 (5), 417–424. doi:10.1016/j.smim.2016.10.004
Bambouskova, M., Gorvel, L., Lampropoulou, V., Sergushichev, A., Loginicheva, E., Johnson, K., et al. (2018). Electrophilic Properties of Itaconate and Derivatives Regulate the IκBζ-ATF3 Inflammatory axis. Nature 556 (7702), 501–504. doi:10.1038/s41586-018-0052-z
Beale, R. J., Sherry, T., Lei, K., Campbell-Stephen, L., McCook, J., Smith, J., et al. (2008). Early Enteral Supplementation with Key Pharmaconutrients Improves Sequential Organ Failure Assessment Score in Critically Ill Patients with Sepsis: Outcome of a Randomized, Controlled, Double-Blind Trial*. Crit. Care Med. 36 (1), 131–144. doi:10.1097/01.ccm.0000297954.45251.a9
Chang, Y. W., Hung, L. C., Chen, Y. C., Wang, W. H., Lin, C. Y., Tzeng, H. H., et al. (2020). Insulin Reduces Inflammation by Regulating the Activation of the NLRP3 Inflammasome. Front. Immunol. 11, 587229. doi:10.3389/fimmu.2020.587229
Chen, Q., Yu, W., Shi, J., Shen, J., Gao, T., Zhang, J., et al. (2014). Insulin Alleviates the Inflammatory Response and Oxidative Stress Injury in Cerebral Tissues in Septic Rats. J. Inflamm. 11, 18. doi:10.1186/1476-9255-11-18
Chen, X., Liu, Y., Gao, Y., Shou, S., and Chai, Y. (2021). The Roles of Macrophage Polarization in the Host Immune Response to Sepsis. Int. Immunopharmacol. 96, 107791. doi:10.1016/j.intimp.2021.107791
Chen, X., Zhao, C., Li, X., Wang, T., Li, Y., Cao, C., et al. (2015). Terazosin Activates Pgk1 and Hsp90 to Promote Stress Resistance. Nat. Chem. Biol. 11 (1), 19–25. doi:10.1038/nchembio.1657
Cheng, S.-C., Quintin, J., Cramer, R. A., Shepardson, K. M., Saeed, S., Kumar, V., et al. (2014). mTOR- and HIF-1α-Mediated Aerobic Glycolysis as Metabolic Basis for Trained Immunity. Science 345 (6204), 1250684. doi:10.1126/science.1250684
Danielski, L. G., Giustina, A. D., Bonfante, S., Barichello, T., and Petronilho, F. (2020). The NLRP3 Inflammasome and its Role in Sepsis Development. Inflammation 43 (1), 24–31. doi:10.1007/s10753-019-01124-9
Delano, M. J., and Ward, P. A. (2016). Sepsis-induced Immune Dysfunction: Can Immune Therapies Reduce Mortality? J. Clin. Invest. 126 (1), 23–31. doi:10.1172/jci82224
Deng, H., Wu, L., Liu, M., Zhu, L., Chen, Y., Zhou, H., et al. (2020). Bone Marrow Mesenchymal Stem Cell-Derived Exosomes Attenuate LPS-Induced ARDS by Modulating Macrophage Polarization through Inhibiting Glycolysis in Macrophages. Shock 54 (6), 828–843. doi:10.1097/shk.0000000000001549
Dong, F., Wang, B., Zhang, L., Tang, H., Li, J., and Wang, Y. (2012). Metabolic Response to Klebsiella pneumoniae Infection in an Experimental Rat Model. PLoS One 7 (11), e51060. doi:10.1371/journal.pone.0051060
Duan, J.-X., Jiang, H.-L., Guan, X.-X., Zhang, C.-Y., Zhong, W.-J., Zu, C., et al. (2021). Extracellular Citrate Serves as a DAMP to Activate Macrophages and Promote LPS-Induced Lung Injury in Mice. Int. Immunopharmacol. 101 (Pt B), 108372. doi:10.1016/j.intimp.2021.108372
Englert, J. A., Bobba, C., and Baron, R. M. (2019). Integrating Molecular Pathogenesis and Clinical Translation in Sepsis-Induced Acute Respiratory Distress Syndrome. JCI Insight 4 (2). doi:10.1172/jci.insight.124061
Esquerdo, K. F., Sharma, N. K., Brunialti, M. K. C., Baggio-Zappia, G. L., Assunção, M., Azevedo, L. C. P., et al. (2017). Inflammasome Gene Profile Is Modulated in Septic Patients, with a Greater Magnitude in Non-survivors. Clin. Exp. Immunol. 189 (2), 232–240. doi:10.1111/cei.12971
Fernandes-Alnemri, T., Wu, J., Yu, J.-W., Datta, P., Miller, B., Jankowski, W., et al. (2007). The Pyroptosome: a Supramolecular Assembly of ASC Dimers Mediating Inflammatory Cell Death via Caspase-1 Activation. Cell Death Differ. 14 (9), 1590–1604. doi:10.1038/sj.cdd.4402194
Fleury, C., Neverova, M., Collins, S., Raimbault, S., Champigny, O., Levi-Meyrueis, C., et al. (1997). Uncoupling Protein-2: a Novel Gene Linked to Obesity and Hyperinsulinemia. Nat. Genet. 15 (3), 269–272. doi:10.1038/ng0397-269
Freemerman, A. J., Johnson, A. R., Sacks, G. N., Milner, J. J., Kirk, E. L., Troester, M. A., et al. (2014). Metabolic Reprogramming of Macrophages. J. Biol. Chem. 289 (11), 7884–7896. doi:10.1074/jbc.m113.522037
Freund, H., Atamian, S., Holroyde, J., and Fischer, J. E. (1979). Plasma Amino Acids as Predictors of the Severity and Outcome of Sepsis. Ann. Surg. 190 (5), 571–576. doi:10.1097/00000658-197911000-00003
Fukuzumi, M., Shinomiya, H., Shimizu, Y., Ohishi, K., and Utsumi, S. (1996). Endotoxin-induced Enhancement of Glucose Influx into Murine Peritoneal Macrophages via GLUT1. Infect. Immun. 64 (1), 108–112. doi:10.1128/iai.64.1.108-112.1996
Gaber, T., Strehl, C., and Buttgereit, F. (2017). Metabolic Regulation of Inflammation. Nat. Rev. Rheumatol. 13 (5), 267–279. doi:10.1038/nrrheum.2017.37
Gaieski, D. F., Edwards, J. M., Kallan, M. J., and Carr, B. G. (2013). Benchmarking the Incidence and Mortality of Severe Sepsis in the United States*. Crit. Care Med. 41 (5), 1167–1174. doi:10.1097/ccm.0b013e31827c09f8
Galbán, C., Montejo, J. C., Mesejo, A., Marco, P., Celaya, S., Sánchez-Segura, J. M., et al. (2000). An Immune-Enhancing Enteral Diet Reduces Mortality Rate and Episodes of Bacteremia in Septic Intensive Care Unit Patients. Crit. Care Med. 28 (3), 643–648. doi:10.1097/00003246-200003000-00007
Gomez, H., and Kellum, J. A. (2015). Lactate in Sepsis. JAMA 313 (2), 194–195. doi:10.1001/jama.2014.13811
Gong, Y., Lan, H., Yu, Z., Wang, M., Wang, S., Chen, Y., et al. (2017). Blockage of Glycolysis by Targeting PFKFB3 Alleviates Sepsis-Related Acute Lung Injury via Suppressing Inflammation and Apoptosis of Alveolar Epithelial Cells. Biochem. Biophysical Res. Commun. 491 (2), 522–529. doi:10.1016/j.bbrc.2017.05.173
Gordon, S., and Taylor, P. R. (2005). Monocyte and Macrophage Heterogeneity. Nat. Rev. Immunol. 5 (12), 953–964. doi:10.1038/nri1733
Hotamisligil, G. S. (2017). Inflammation, Metaflammation and Immunometabolic Disorders. Nature 542 (7640), 177–185. doi:10.1038/nature21363
Hsu, P. P., and Sabatini, D. M. (2008). Cancer Cell Metabolism: Warburg and beyond. Cell 134 (5), 703–707. doi:10.1016/j.cell.2008.08.021
Huang, C.-T., Lue, J.-H., Cheng, T.-H., and Tsai, Y.-J. (2020). Glycemic Control with Insulin Attenuates Sepsis-Associated Encephalopathy by Inhibiting Glial Activation via the Suppression of the Nuclear Factor Kappa B and Mitogen-Activated Protein Kinase Signaling Pathways in Septic Rats. Brain Res. 1738, 146822. doi:10.1016/j.brainres.2020.146822
Humphries, F., Shmuel-Galia, L., Ketelut-Carneiro, N., Li, S., Wang, B., Nemmara, V. V., et al. (2020). Succination Inactivates Gasdermin D and Blocks Pyroptosis. Science 369 (6511), 1633–1637. doi:10.1126/science.abb9818
Iwashyna, T. J., Cooke, C. R., Wunsch, H., and Kahn, J. M. (2012). Population Burden of Long-Term Survivorship after Severe Sepsis in Older Americans. J. Am. Geriatr. Soc. 60 (6), 1070–1077. doi:10.1111/j.1532-5415.2012.03989.x
James, J. H., Fang, C. H., Schrantz, S. J., Hasselgren, P. O., Paul, R. J., and Fischer, J. E. (1996). Linkage of Aerobic Glycolysis to Sodium-Potassium Transport in Rat Skeletal Muscle. Implications for Increased Muscle Lactate Production in Sepsis. J. Clin. Invest. 98 (10), 2388–2397. doi:10.1172/jci119052
Kayagaki, N., Warming, S., Lamkanfi, M., Walle, L. V., Louie, S., Dong, J., et al. (2011). Non-canonical Inflammasome Activation Targets Caspase-11. Nature 479 (7371), 117–121. doi:10.1038/nature10558
Keiran, N., Ceperuelo-Mallafré, V., Calvo, E., Hernández-Alvarez, M. I., Ejarque, M., Núñez-Roa, C., et al. (2019). SUCNR1 Controls an Anti-inflammatory Program in Macrophages to Regulate the Metabolic Response to Obesity. Nat. Immunol. 20 (5), 581–592. doi:10.1038/s41590-019-0372-7
Kumar, V. (2018). Targeting Macrophage Immunometabolism: Dawn in the Darkness of Sepsis. Int. Immunopharmacol. 58, 173–185. doi:10.1016/j.intimp.2018.03.005
Lamkanfi, M. (2011). Emerging Inflammasome Effector Mechanisms. Nat. Rev. Immunol. 11 (3), 213–220. doi:10.1038/nri2936
Lampropoulou, V., Sergushichev, A., Bambouskova, M., Nair, S., Vincent, E. E., Loginicheva, E., et al. (2016). Itaconate Links Inhibition of Succinate Dehydrogenase with Macrophage Metabolic Remodeling and Regulation of Inflammation. Cell Metab. 24 (1), 158–166. doi:10.1016/j.cmet.2016.06.004
Langley, R. J., Tsalik, E. L., van Velkinburgh, J. C., Glickman, S. W., Rice, B. J., Wang, C., et al. (2013). An Integrated Clinico-Metabolomic Model Improves Prediction of Death in Sepsis. Sci. Transl. Med. 5 (195), 195ra95. doi:10.1126/scitranslmed.3005893
Lauvau, G., Loke, P. n., and Hohl, T. M. (2015). Monocyte-mediated Defense against Bacteria, Fungi, and Parasites. Seminars Immunol. 27 (6), 397–409. doi:10.1016/j.smim.2016.03.014
Li, A., Zhang, S., Li, J., Liu, K., Huang, F., and Liu, B. (2016). Metformin and Resveratrol Inhibit Drp1-Mediated Mitochondrial Fission and Prevent ER Stress-Associated NLRP3 Inflammasome Activation in the Adipose Tissue of Diabetic Mice. Mol. Cell. Endocrinol. 434, 36–47. doi:10.1016/j.mce.2016.06.008
Lu, B., Nakamura, T., Inouye, K., Li, J., Tang, Y., Lundbäck, P., et al. (2012). Novel Role of PKR in Inflammasome Activation and HMGB1 Release. Nature 488 (7413), 670–674. doi:10.1038/nature11290
Lu, J., Zhang, L., Cheng, L., He, S., Zhang, Y., Yan, J., et al. (2020). Xijiao Dihuang Decoction Improves Prognosis of Sepsis via Inhibition of Aerobic Glycolysis. Biomed. Pharmacother. 129, 110501. doi:10.1016/j.biopha.2020.110501
Man, K., Kutyavin, V. I., and Chawla, A. (2017). Tissue Immunometabolism: Development, Physiology, and Pathobiology. Cell Metab. 25 (1), 11–26. doi:10.1016/j.cmet.2016.08.016
Mao, K., Chen, S., Chen, M., Ma, Y., Wang, Y., Huang, B., et al. (2013). Nitric Oxide Suppresses NLRP3 Inflammasome Activation and Protects against LPS-Induced Septic Shock. Cell Res. 23 (2), 201–212. doi:10.1038/cr.2013.6
Martínez-García, J. J., Martínez-Banaclocha, H., Angosto-Bazarra, D., de Torre-Minguela, C., Baroja-Mazo, A., Alarcón-Vila, C., et al. (2019). P2X7 Receptor Induces Mitochondrial Failure in Monocytes and Compromises NLRP3 Inflammasome Activation during Sepsis. Nat. Commun. 10 (1), 2711. doi:10.1038/s41467-019-10626-x
Martinon, F., Burns, K., and Tschopp, J. (2002). The Inflammasome. Mol. Cell 10 (2), 417–426. doi:10.1016/s1097-2765(02)00599-3
Meyers, A. K., and Zhu, X. (2020). The NLRP3 Inflammasome: Metabolic Regulation and Contribution to Inflammaging. Cells 9 (8), 1808. doi:10.3390/cells9081808
Mills, E. L., Kelly, B., Logan, A., Costa, A. S. H., Varma, M., Bryant, C. E., et al. (2016). Succinate Dehydrogenase Supports Metabolic Repurposing of Mitochondria to Drive Inflammatory Macrophages. Cell 167 (2), 457–470. doi:10.1016/j.cell.2016.08.064
Mills, E. L., Ryan, D. G., Prag, H. A., Dikovskaya, D., Menon, D., Zaslona, Z., et al. (2018). Itaconate Is an Anti-inflammatory Metabolite that Activates Nrf2 via Alkylation of KEAP1. Nature 556 (7699), 113–117. doi:10.1038/nature25986
Moon, J.-S., Hisata, S., Park, M.-A., DeNicola, G. M., Ryter, S. W., Nakahira, K., et al. (2015). mTORC1-Induced HK1-dependent Glycolysis Regulates NLRP3 Inflammasome Activation. Cell Rep. 12 (1), 102–115. doi:10.1016/j.celrep.2015.05.046
Moon, J.-S., Lee, S., Park, M.-A., Siempos, I. I., Haslip, M., Lee, P. J., et al. (2015). UCP2-induced Fatty Acid Synthase Promotes NLRP3 Inflammasome Activation during Sepsis. J. Clin. Invest. 125 (2), 665–680. doi:10.1172/jci78253
Palsson-McDermott, E. M., Curtis, A. M., Goel, G., Lauterbach, M. A. R., Sheedy, F. J., Gleeson, L. E., et al. (2015). Pyruvate Kinase M2 Regulates Hif-1α Activity and IL-1β Induction and Is a Critical Determinant of the Warburg Effect in LPS-Activated Macrophages. Cell Metab. 21 (1), 65–80. doi:10.1016/j.cmet.2014.12.005
Palsson-McDermott, E. M., Curtis, A. M., Goel, G., Lauterbach, M. A. R., Sheedy, F. J., Gleeson, L. E., et al. (2015). Pyruvate Kinase M2 Regulates Hif-1α Activity and IL-1β Induction and Is a Critical Determinant of the Warburg Effect in LPS-Activated Macrophages. Cell Metab. 21 (2), 347. doi:10.1016/j.cmet.2015.01.017
Rathinam, V. A. K., Vanaja, S. K., and Fitzgerald, K. A. (2012). Regulation of Inflammasome Signaling. Nat. Immunol. 13 (4), 333–342. doi:10.1038/ni.2237
Reddy, A. B. M., Srivastava, S. K., and Ramana, K. V. (2010). Aldose Reductase Inhibition Prevents Lipopolysaccharide-Induced Glucose Uptake and Glucose Transporter 3 Expression in RAW264.7 Macrophages. Int. J. Biochem. Cell Biol. 42 (6), 1039–1045. doi:10.1016/j.biocel.2010.03.014
Reisinger, A. C., Posch, F., Hackl, G., Marsche, G., Sourij, H., Bourgeois, B., et al. (2021). Branched-Chain Amino Acids Can Predict Mortality in ICU Sepsis Patients. Nutrients 13 (9), 3106. doi:10.3390/nu13093106
Shao, W., Yeretssian, G., Doiron, K., Hussain, S. N., and Saleh, M. (2007). The Caspase-1 Digestome Identifies the Glycolysis Pathway as a Target during Infection and Septic Shock. J. Biol. Chem. 282 (50), 36321–36329. doi:10.1074/jbc.m708182200
Shi, C.-x., Wang, Y., Chen, Q., Jiao, F.-z., Pei, M.-h., and Gong, Z.-j. (2020). Extracellular Histone H3 Induces Pyroptosis during Sepsis and May Act through NOD2 and VSIG4/NLRP3 Pathways. Front. Cell. Infect. Microbiol. 10, 196. doi:10.3389/fcimb.2020.00196
Singer, M., Deutschman, C. S., Seymour, C. W., Shankar-Hari, M., Annane, D., Bauer, M., et al. (2016). The Third International Consensus Definitions for Sepsis and Septic Shock (Sepsis-3). JAMA 315 (8), 801–810. doi:10.1001/jama.2016.0287
Su, L., Li, H., Xie, A., Liu, D., Rao, W., Lan, L., et al. (2015). Dynamic Changes in Amino Acid Concentration Profiles in Patients with Sepsis. PLoS One 10 (4), e0121933. doi:10.1371/journal.pone.0121933
Sun, Q., Li, J., and Gao, F. (2014). New Insights into Insulin: The Anti-inflammatory Effect and its Clinical Relevance. Wjd 5 (2), 89–96. doi:10.4239/wjd.v5.i2.89
Sutterwala, F. S., Ogura, Y., Szczepanik, M., Lara-Tejero, M., Lichtenberger, G. S., Grant, E. P., et al. (2006). Critical Role for NALP3/CIAS1/Cryopyrin in Innate and Adaptive Immunity through its Regulation of Caspase-1. Immunity 24 (3), 317–327. doi:10.1016/j.immuni.2006.02.004
Tan, C., Gu, J., Li, T., Chen, H., Liu, K., Liu, M., et al. (2021). Inhibition of Aerobic Glycolysis Alleviates Sepsis-induced A-cute K-idney I-njury by P-romoting lactate/Sirtuin 3/AMPK-regulated A-utophagy. Int. J. Mol. Med. 47 (3), 19. doi:10.3892/ijmm.2021.4852
Tannahill, G. M., Curtis, A. M., Adamik, J., Palsson-McDermott, E. M., McGettrick, A. F., Goel, G., et al. (2013). Succinate Is an Inflammatory Signal that Induces IL-1beta through HIF-1alpha. Nature 496 (7444), 238–242.
Tavakoli, S., Zamora, D., Ullevig, S., and Asmis, R. (2013). Bioenergetic Profiles Diverge during Macrophage Polarization: Implications for the Interpretation of 18F-FDG PET Imaging of Atherosclerosis. J. Nucl. Med. 54 (9), 1661–1667. doi:10.2967/jnumed.112.119099
Torio, C. M., and Andrews, R. M. (2006). “National Inpatient Hospital Costs: The Most Expensive Conditions by Payer, 2011: Statistical Brief #160,” in Healthcare Cost and Utilization Project (HCUP) Statistical Briefs (Rockville (MD): Agency for Healthcare Research and Quality).
Tsuji, G., Hashimoto-Hachiya, A., Yen, V. H., Takemura, M., Yumine, A., Furue, K., et al. (2020). Metformin Inhibits IL-1β Secretion via Impairment of NLRP3 Inflammasome in Keratinocytes: Implications for Preventing the Development of Psoriasis. Cell Death Discov. 6, 11. doi:10.1038/s41420-020-0245-8
Wang, D., Zhang, Y., Xu, X., Wu, J., Peng, Y., Li, J., et al. (2021). YAP Promotes the Activation of NLRP3 Inflammasome via Blocking K27-Linked Polyubiquitination of NLRP3. Nat. Commun. 12 (1), 2674. doi:10.1038/s41467-021-22987-3
Wang, T. S., and Deng, J. C. (2008). Molecular and Cellular Aspects of Sepsis-Induced Immunosuppression. J. Mol. Med. 86 (5), 495–506. doi:10.1007/s00109-007-0300-4
Wang, Z., Kong, L., Tan, S., Zhang, Y., Song, X., Wang, T., et al. (2020). Zhx2 Accelerates Sepsis by Promoting Macrophage Glycolysis via Pfkfb3. J. I. 204 (8), 2232–2241. doi:10.4049/jimmunol.1901246
Wei, Y., Gao, N., Zhang, Z., Zu, X., Hu, Z., Zhang, W., et al. (2016). Metabolic Changes at the Early Stage of Sepsis Induced by Cecal Ligation and Puncture in Rats and the Interventional Effects of Huang-Lian-Jie-Du-Tang. J. Chromatogr. B 1026, 176–182. doi:10.1016/j.jchromb.2015.08.009
Xian, H., Liu, Y., Rundberg Nilsson, A., Gatchalian, R., Crother, T. R., Tourtellotte, W. G., et al. (2021). Metformin Inhibition of Mitochondrial ATP and DNA Synthesis Abrogates NLRP3 Inflammasome Activation and Pulmonary Inflammation. Immunity 54 (7), 1463–1477. doi:10.1016/j.immuni.2021.05.004
Xie, M., Yu, Y., Kang, R., Zhu, S., Yang, L., Zeng, L., et al. (2016). PKM2-dependent Glycolysis Promotes NLRP3 and AIM2 Inflammasome Activation. Nat. Commun. 7, 13280. doi:10.1038/ncomms13280
Xu, S., Li, X., Liu, Y., Xia, Y., Chang, R., and Zhang, C. (2019). Inflammasome Inhibitors: Promising Therapeutic Approaches against Cancer. J. Hematol. Oncol. 12 (1), 64. doi:10.1186/s13045-019-0755-0
Yan, J.-J., Jung, J.-S., Lee, J.-E., Lee, J., Huh, S.-O., Kim, H.-S., et al. (2004). Therapeutic Effects of Lysophosphatidylcholine in Experimental Sepsis. Nat. Med. 10 (2), 161–167. doi:10.1038/nm989
Yang, L., Xie, M., Yang, M., Yu, Y., Zhu, S., Hou, W., et al. (2014). PKM2 Regulates the Warburg Effect and Promotes HMGB1 Release in Sepsis. Nat. Commun. 5, 4436. doi:10.1038/ncomms5436
Yu, S., Wang, D., Huang, L., Zhang, Y., Luo, R., Adah, D., et al. (2019). The Complement Receptor C5aR2 Promotes Protein Kinase R Expression and Contributes to NLRP3 Inflammasome Activation and HMGB1 Release from Macrophages. J. Biol. Chem. 294 (21), 8384–8394. doi:10.1074/jbc.ra118.006508
Yu, X. H., Zhang, D. W., Zheng, X. L., and Tang, C. K. (2019). Itaconate: an Emerging Determinant of Inflammation in Activated Macrophages. Immunol. Cell Biol. 97 (2), 134–141. doi:10.1111/imcb.12218
Zheng, Z., Ma, H., Zhang, X., Tu, F., Wang, X., Ha, T., et al. (2017). Enhanced Glycolytic Metabolism Contributes to Cardiac Dysfunction in Polymicrobial Sepsis. J. Infect. Dis. 215 (9), 1396–1406. doi:10.1093/infdis/jix138
Zhong, W. J., Yang, H. H., Guan, X. X., Xiong, J. B., Sun, C. C., Zhang, C. Y., et al. (2019). Inhibition of Glycolysis Alleviates Lipopolysaccharide‐induced Acute Lung Injury in a Mouse Model. J. Cell. Physiology 234 (4), 4641–4654. doi:10.1002/jcp.27261
Keywords: sepsis, NLRP3 inflammasome, metabolism reprogramming, macrophages, targeted therapy
Citation: Luo R, Li X and Wang D (2022) Reprogramming Macrophage Metabolism and its Effect on NLRP3 Inflammasome Activation in Sepsis. Front. Mol. Biosci. 9:917818. doi: 10.3389/fmolb.2022.917818
Received: 11 April 2022; Accepted: 09 June 2022;
Published: 29 June 2022.
Edited by:
Zichao Luo, National University of Singapore, SingaporeReviewed by:
Rishabh Charan Choudhary, Northwell Health, United StatesPradeep Bist, Duke-NUS Medical School, Singapore
Copyright © 2022 Luo, Li and Wang. This is an open-access article distributed under the terms of the Creative Commons Attribution License (CC BY). The use, distribution or reproduction in other forums is permitted, provided the original author(s) and the copyright owner(s) are credited and that the original publication in this journal is cited, in accordance with accepted academic practice. No use, distribution or reproduction is permitted which does not comply with these terms.
*Correspondence: Dan Wang, MTY4MzAxMDEzQGNzdS5lZHUuY24=