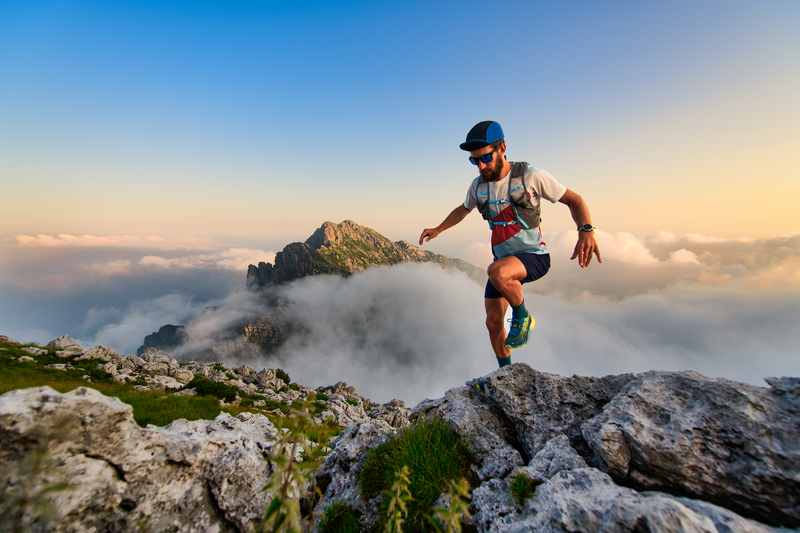
95% of researchers rate our articles as excellent or good
Learn more about the work of our research integrity team to safeguard the quality of each article we publish.
Find out more
REVIEW article
Front. Mol. Biosci. , 28 April 2022
Sec. Molecular Diagnostics and Therapeutics
Volume 9 - 2022 | https://doi.org/10.3389/fmolb.2022.895814
This article is part of the Research Topic The Role of Calcium Channels in Human Health and Disease - Volume II View all 7 articles
Transient receptor potential (TRP) channels are polymodal channels capable of sensing environmental stimuli, which are widely expressed on the plasma membrane of cells and play an essential role in the physiological or pathological processes of cells as sensors. TRPs often form functional homo- or heterotetramers that act as cation channels to flow Na+ and Ca2+, change membrane potential and [Ca2+]i (cytosolic [Ca2+]), and change protein expression levels, channel attributes, and regulatory factors. Under normal circumstances, various TRP channels respond to intracellular and extracellular stimuli such as temperature, pH, osmotic pressure, chemicals, cytokines, and cell damage and depletion of Ca2+ reserves. As cation transport channels and physical and chemical stimulation receptors, TRPs play an important role in regulating secretion, interfering with cell proliferation, and affecting neural activity in these glands and their adenocarcinoma cells. Many studies have proved that TRPs are widely distributed in the pancreas, adrenal gland, and other glands. This article reviews the specific regulatory mechanisms of various TRP channels in some common glands (pancreas, salivary gland, lacrimal gland, adrenal gland, mammary gland, gallbladder, and sweat gland).
Endocrine gland-related diseases, though rare, remain a significant threat. Take the islets as an example, pancreatitis can contribute to diabetes and pancreatic cancer, and the existing treatment options, such as radiotherapy and surgery, are ineffective (Kleeff et al., 2017; Hart and Conwell, 2020). In addition, the cases of pancreatic ductal adenocarcinoma (PDAC) are on the rise year by year, and the fatality rate is very high because most cases are found in the late stage. For non-early-stage patients, existing systemic therapy, radiotherapy, and cytotoxic therapy are not complete cures (Park et al., 2021). In this regard, regulation of TRPs can help regulate the microenvironment of pancreatic cells and improve the possibility of a specific therapy.
On the other hand, standard treatments for salivary and lacrimal gland-associated Sjögren’s syndrome (pSS) include anti-inflammatory drugs, steroids, hormones, immunosuppressants, and biotherapy (Ramos-Casals et al., 2020). Primary adrenal insufficiency or hyperplasia is also often treated with hormone replacement therapy to mimic normal secretion. Nevertheless, the death rate among primary adrenal insufficiency patients continues increasing (El-Maouche et al., 2017; Barthel et al., 2019). For hidradenitis suppurativa (acne inversa), surgical, laser, and antibiotic treatments are often inadequate, new drug targets are needed, and patients are often associated with a range of endocrine diseases such as obesity, diabetes, and metabolic syndrome (Saunte and Jemec, 2017; Sabat et al., 2020).
Moreover, studies have proved that COVID-19 is associated with secretory gland diseases such as diabetes, adrenal insufficiency, and mumps (De-Madaria and Capurso, 2021; Fisher et al., 2021; Zhou et al., 2021). Patients with diabetes, obesity, and primary adrenal insufficiency infected with COVID-19 will have more severe damage to the endocrine glands, resulting in a poor prognosis and the possibility of chronic inflammation, significantly increasing hospitalization, severe illness, and mortality rates (Marazuela et al., 2020; Zhou et al., 2021). In contrast, COVID-19 can induce diabetic onset in patients with hyperglycemia and induce secondary adrenal insufficiency. In addition, the hormone therapy previously used in patients with endocrine diseases should be changed accordingly after COVID-19 infection (Lisco et al., 2021). Here, the TRP channels are also noteworthy as potential drug targets for endocrine glands.
TRP channels have been gradually discovered and studied in the past few decades. The TRP channel was initially named after the photo-transducted channels in Drosophila melanogaster, which is blind to constant bright light (Cosens and Manning, 1969; Hardie, 2011; Samanta et al., 2018). Various TRPs discovered later were classified according to differences in amino acid sequence and topological structure, including seven families in total: TRPC (canonical), TRPV (vanilloid), TRPM (melastatin), TRPA (ankyrin), TRPP (polycystic), TRPML (mucolipin), and TRPN (Drosophila NOMPC) (Hardie, 2011; Li, 2017; Samanta et al., 2018). And then there is TRPY, which was just discovered in yeast and named after it (Amini et al., 2021). Among them, TRPC, TRPV, TRPM, and TRPA are group1 of TRP channels, which have high similarities with drosophila TRP channels. TRPP and TRPML are group 2 of TRP channels, which have distal relevance to drosophila TRP channels. Group1 and 2 are different in the transmembrane domain (Li, 2017; Samanta et al., 2018).
Due to its homology with drosophila TRP sequence, TRPCs were first discovered and had seven members, divided into four subgroups: TRPC1, TRPC2 (a pseudogene in mammals), TRPC3\6\7, and TRPC4\5. TRPCs can be activated by gated receptors, storage operations, and mechanical stimuli to participate in cell regulation (Wes et al., 1995; Vazquez et al., 2004; Ong et al., 2016). TRPVs are named after the activation of TRPV1 by capsaicin in sensory neurons (Caterina et al., 1999). In addition, vanillin and vanillic acid in plants can also activate TRPVs (Li, 2017). TRPVs have six members, divided into two subgroups: TRPV1-4 and TRPV5\6. TRPV1-4 are thermosensitive nonselective nociceptors, which are related to neural plasticity (Satheesh et al., 2016). TRPV5\6 are epithelial channels with high calcium selectivity (Li, 2017). Mammalian TRPMs have eight members divided into four subgroups according to sequence homology: M1\3, M2\8, M4\5, and M6\7. Some TRPMs are distributed in the intima of cells, and their activation patterns, cation selectivity, and tissue distribution vary significantly among different TRPMs members (Kraft and Harteneck, 2005; Li, 2017; Samanta et al., 2018). TRPM is mostly cloned from cancer tissues and is related to tumor genesis, proliferation, and differentiation. They are involved in temperature sensing, Mg2+ homeostasis regulation, and taste conduction (Kraft and Harteneck, 2005; Samanta et al., 2018). TRPA1, the only member of the TRPA family in mammals, is an anchor-like transmembrane protein capable of sensing chemical damage (Zygmunt and Hogestatt, 2014).
For TRPPs, it was found in polycystic kidney disease with the pathogenic locus of autosomal dominant and it had three members: TRPP1-3. TRPPs are composed of intact membrane proteins (Hughes et al., 1995; Mochizuki et al., 1996; Samanta et al., 2018). TRPMLs also has three members: TRPML1-3; TRP channels with the most negligible molecular weight, are associated with vesicle transport (Colletti and Kiselyov, 2011; Flores and Garcia-Anoveros, 2011; Wang et al., 2014; Venkatachalam et al., 2015). However, TRPNs do not encode in mammals, and they are related to mechanical sensory transduction in nematodes, flies, and zebrafish (Lee et al., 2010). It is worth mentioning that TRPs affect sensory function and the development of (inherited) diseases. In addition to cancers and diabetes, TRPs are often involved in heart, bone, kidney, brain, skin, eyes, and nerve diseases. TRPs are also related to the sensory transduction of chronic pain (Julius, 2013; Li, 2017; Samanta et al., 2018). Since TRPs are primarily on the surface of cells, they have also been studied as targets for drugs such as analgesics (Moran, 2018; Koivisto et al., 2022). The following review focuses on the research mechanism and drug progress of TRP channels in the pancreas, salivary gland, lacrimal gland, adrenal gland, mammary gland, gallbladder, and sweat gland (Figure 1).
FIGURE 1. Schematic illustration of the tissue-distribution of TRP channels and their putative roles in human glands.
As a component of the store-operated Ca2+ (SOC) channels, TRPC channels, primarily located in the plasma membrane, can be activated by G protein-coupled receptors (GPRs), protein kinases, or mechanical stimulation to form non-selective Ca2+ osmotic channels, mediating Ca2+ and some monovalent ions influx, and promoting cell depolarization (Chen et al., 2020; Lopez et al., 2020). In the pancreas, TRPC1, TRPC3, and TRPC4 have promoted insulin secretion by β cells. In rat β cells, TRPC1 and Orai1 are coupled to form cationic channels that mediate Ca2+ influx and are regulated by stromal interaction molecule 1(STIM1) (Islam, 2011; Najder et al., 2018; Islam, 2020). STIM1 gates TRPC1 through intermolecular interactions (Islam, 2011; Islam, 2020). In addition, TRPC1 is phosphorylated by protein kinase C-α (PKCα) to promote glucose-induced insulin secretion (Xu et al., 2019). Under normal circumstances, TRPC3 is activated by G protein-coupled receptor 40 (GPR40) in rat and mouse β cells. In rat β cells, Activated TRPC3 enhanced insulin secretion via phospholipase C, PKC, and GPR40, leading to membrane depolarization and Ca2+ influx (Hayes et al., 2013). The high expression level of TRPC3/TRPC6 induces pancreatic α and β cells proliferation via insulin-related transcription factor pancreatic and duodenal homeobox 1 (PDX-1) (Hayes et al., 2013). TRPC4 was expressed in rat and mouse islet β cells and insulinoma cells. In βTC3 cells, intracellular Ca2+ depletion activates TRPC4, which generates a cationic current that stimulates membrane oscillation and promotes Ca2+ influx, thereby increasing insulin secretion (Roe et al., 1998; Islam, 2020). Leptin, the activator of TRPC4, phosphorylates TRPC4 by phosphoinositide 3-kinase. Similarly, in INS-1 cells, protein histidine phosphatase 1 phosphorylates TRPC4, which also translocates K-ATP channels to the plasma membrane typically and promotes insulin secretion (Park et al., 2013; Srivastava et al., 2018).
In addition, in the case of PDAC, TRPC1, TRPC3, and TRPC6 may also be activated by mechanical stimuli to aggravate the disease. In the PDAC pressure microenvironment, TRPC1 transmits the mechanical signal that mediates the activation and migration of pancreatic stellate cells (PSCs) and induces chemotaxis in neutrophils. Furthermore, it was predicted that it cooperated with TRPM7 and TRPV4 to promote the progress of PDAC (Lindemann et al., 2015; Fels et al., 2016; Najder et al., 2018). In the pathological condition of PDAC, activated TRPC3 and KCa3.1 (Ca2+-dependent K+ 3.1) channels in the PDAC matrix jointly promote the migration and taxis of PSCs, and the over-activation of PSCs to produce excessive extracellular matrix proteins is the leading cause of pancreatic cancer fibrosis. Moreover, in the hypoxic tumor microenvironment of PDAC, TRPC6 is not only richly expressed in PSCs but also can be activated by hypoxia, thus promoting the secretion of migration factors, which may also worsen the disease progression, which is also related to its ability to control Ca2+ influx (Nielsen et al., 2017; Storck et al., 2017; Najder et al., 2018).
TRPVs are also predominantly expressed in islet β cells. Among them, TRPV2 and TRPV4 also have similar insulin-secreting effects as mentioned above (Islam, 2011; Uchida and Tominaga, 2011; Sawatani et al., 2019). However, TRPV1 transmitting neural sensation does not directly promote insulin secretion, and the role of TRPV5 and TRPV6 in islet remains to be studied. TRPV1 was expressed in INS-1E cells (Fagelskiold et al., 2012). Although TRPV1 does not directly affect glucose-induced insulin secretion in mouse β cells (Diaz-Garcia et al., 2014), it is expressed in sensory nerve fibers of the mouse pancreas, and its locus is associated with the risk of autoimmune diabetes (Razavi et al., 2006; Najder et al., 2018). During insulin release, TRPV1 is activated to promote the release of calcitonin-gene-related peptide (CGRP) and substance P (SP) by neurons, and the concentration of CGRP and SP are balanced with glucose concentration by regulating insulin concentration (Zhong et al., 2019). In nonobese diabetic mice, neuronal failure to release CGRP and SP due to TRPV1 mutations lead to physiological dysfunction of β cells, resulting in insulin resistance and β cell stress (Razavi et al., 2006; Najder et al., 2018; Zhong et al., 2019). In addition, glucagon-like peptide 1 (GLP-1) can also enhance insulin secretion, but different from the above-mentioned TRP channels, TRPV1 in the ileum is activated by capsaicin, which increases GLP-1 secretion and leads to increased pancreatic insulin secretion (Wang et al., 2012); however, this interpretation should be treated with caution. Although TRPV1 is typically excited by capsaicin, whether TRPV1 promotes insulin secretion has been highly controversial (Zhong et al., 2019). Notably, TRPV1 also expresses and mediates inflammation and pain sensation in afferents of acute pancreatitis, in coordination with TRPA1. It is possible that they regulate [Ca2+]i and increase the release of inflammatory and pain mediators (Schwartz et al., 2011; Schwartz et al., 2013).
Ca2+ permeable TRPV2 is expressed in mouse insulinoma MIN6 cells and β cells (Islam, 2011). When unstimulated, TRPV2 is distributed in the cytoplasm, mainly in the endoplasmic reticulum (Uchida and Tominaga, 2011). Under high glucose conditions, insulin binds with its β cell receptor, phosphatidylinositol 3 kinase (PI3K), to stimulate the translocation of TRPV2 from the cytoplasm to membrane, which increases [Ca2+]i, accelerates insulin secretion, and stimulates β cell growth (Hisanaga et al., 2009; Aoyagi et al., 2010; Uchida and Tominaga, 2011). In addition, the osmotic swelling of cells during high glucose also activates TRPV2 to depolarize cells and increase glucose-induced insulin secretion (Sawatani et al., 2019). It was also reported that the antiaging gene Klotho translocated TRPV2 in MIN-6 (Lin and Sun, 2012).
As a permeable Ca2+ channel, TRPV4 is expressed in human pancreatic non-β cells and, like TRPV2, is a thermosensitive osmotic and mechanical sensor; it is also activated by the swelling of cells stimulated by glucose (Islam, 2011; Uchida and Tominaga, 2011; Marabita and Islam, 2017). Moreover, in mouse β cells, TRPV4 can be activated by sensing mechanical membrane changes induced by human islet amyloid polypeptide, which affect [Ca2+]i and promote insulin secretion (Casas et al., 2008). In addition, the activation of TRPV4 also promotes insulin secretion in INS-1E cells, and extracellular signal-regulated kinase may be involved in this process (Skrzypski et al., 2013; Billert et al., 2017).
TRPV5/6 are the epithelial Ca2+ permeable channels. TRPV5 exists in secretory granules of β cells, but its effect on secretion is unknown. TRPV6 is expressed in the exocrine acinar of the pancreas, especially in human and mouse islet α cells (Hoenderop et al., 2003; Marabita and Islam, 2017). In INS-1 cells, TRPV6 regulates calcium ions to influence insulin mRNA expression and cell proliferation, but does not seem to affect insulin secretion (Skrzypski et al., 2015). Pathologically, TRPV6 overexpression in the cytoplasm of pancreatic cancer cells promotes the migration of cancer tissues (Song et al., 2018).
TRPMs are also expressed in the pancreas. In addition to being activated by their activators, TRPM2, TRPM4, and TRPM5 can also be triggered by the influx of Ca2+ to participate in membrane depolarization, and TRPM2, TRPM7, and TRPM8 are involved in the progression of pancreatic adenocarcinoma (Islam, 2011; Uchida and Tominaga, 2011; Yee et al., 2012a; Uchida et al., 2017; Islam, 2020). TRPM2 is also engaged in β cell apoptosis in animals other than humans. TRPM2 was found to express in mouse and human β cells and rat INS-1 cells. TRPM2 was activated by ADP ribose (ADPR), Nicotinamide adenine dinucleotide (NAD+) concentration, and reactive oxygen species (ROS) to mediate Ca2+ influx and depolarization to regulate glucose-dependent insulin secretion (Pi et al., 2007; Du et al., 2009; Leloup et al., 2009; Islam, 2011; Islam, 2020). Particularly, TRPM2 is a calcium-permeable thermosensitive channel. Before being activated by ADPR, extracellular Ca2+ must be combined with the calcium sensor in the TRPM2 channel, which reflects the leading role of Ca2+ (McHugh et al., 2003; Csanady and Torocsik, 2009; Du et al., 2009). At a high glucose concentration, besides essential ADPR and Ca2+, other activators of TRPM2 include arachidonic acid produced by glucose metabolism, PKA-dependent cyclic adenosine monophosphate (cAMP), intestinal incretin hormone GLP-1, and physical second messenger “heat” (Togashi et al., 2006; Islam, 2011; Yosida et al., 2014). Growth hormone-releasing peptides Ghrelin and epinephrine inhibit TRPM2 by inhibiting cAMP, thus inhibiting insulin secretion (Yosida et al., 2014; Islam, 2020).
Moreover, TRPM2 was associated with β cell apoptosis. In the case of oxidative stress induced by free fatty acids or cytokines, TRPM2 is activated by ROS, which promotes mitochondrial destruction and leads to apoptosis. Still, human β cells resist such apoptosis (Wehage et al., 2002; Scharenberg, 2009; Li et al., 2017a; Islam, 2020). In addition, TRPM2 is distributed on the lysosomal membrane, gating Ca2+ and Zn2+ and affecting the externalization of phosphatidylserine (Mirnikjoo et al., 2009; Li et al., 2017a). In the case of pathological PADC, TRPM2 of neutrophils in tumor stroma may also be activated by ROS (Uchida and Tominaga, 2011; Faouzi and Penner, 2014).
TRPM3 has the permeability of Na+, Zn2+, and Ca2+, and has a strong absorption effect on Zn2+. In pancreatic β cells such as INS-1 cells, TRPM3, which is activated by pregnenolone sulfate (PregS), mediates Ca2+, Zn2+ influx, and induces the biosynthesis of zinc finger transcription factor Egr-1, thereby promoting glucose-induced insulin synthesis and release (Wagner et al., 2008; Colsoul et al., 2011; Mendez-Resendiz et al., 2020). Although the effect of TRPM3 is rapid and reversible, it does not participate in Ca2+ signaling in β cells, probably because PregS depolarizes with Na+ current and thus activates voltage-gated calcium channels (Islam, 2020). In addition to PregS, TRPM3 is also regulated by phosphatidylinositol 4,5-biphosphate (PIP2) and heat (Badheka et al., 2015).
Both TRPM4 and TRPM5 are intracellular Ca2+ dependent, and they are activated by increased [Ca2+]i during insulin release (Colsoul et al., 2010; Uchida and Tominaga, 2011). Unlike TRPM2, TRPM4/5 are not Ca2+ permeable, but monovalent cation channels. Activated by Ca2+ influx, TRPM4/5 depolarize the membrane through Na + action and open voltage-gated calcium channels, thereby increasing glucose-induced insulin secretion (Islam, 2011; Uchida and Tominaga, 2011). In addition to being activated by [Ca2+]i, TRPM4/5 can also be activated by PKC, leading to membrane depolarization and increasing insulin secretion (Uchida and Tominaga, 2011; Shigeto et al., 2015; Uchida et al., 2017). TRPM4 and TRPM5 are also different. TRPM4 is expressed in both human and mouse β cells and INS-1 cells, and its depolarization mechanism is almost similar (Uchida et al., 2017). In addition to the PKC pathway, PIP2 also maintains TRPM4 sensitivity to [Ca2+]i concentration, and TRPM4 is inhibited by glibenclamide (Colsoul et al., 2011). Particularly, in pancreatic acinar cells (PACs), the depolarization of TRPM4 inhibits Ca2+ influx (Diszhazi et al., 2021). TRPM4 is also involved in glucagon secretion in DTC1-6 of islet α cell line (Colsoul et al., 2011). TRPM5 was highly expressed in rat β cells and insulinoma cells but low in human β cells (Islam, 2020). Stevioside can regulate TRPM5, which may be related to its hypoglycemic effect (Steinritz et al., 2018). Interestingly, TRPM5 acts as a taste receptor and activation of sweet-taste receptors in mouse β cells is mediated by TRPM5, and TRPM5 knockout mice have less sweet taste preference and higher glucose tolerance (Larsson et al., 2015; Islam, 2020).
TRPM6 is not expressed in human β cells, while TRPM7, with the permeability of Mg2+, Ca2+, and Zn2+, is exceptionally abundant in human and mouse β cells, and can regulate [Mg2+]i to promote insulin secretion (Islam, 2020). Importantly, in the case of human pathological pancreatic adenocarcinoma, TRPM7 and TRPM8 are overexpressed and are necessary biomarkers for cancer cell proliferation (Yee et al., 2011; Yee et al., 2012a). In pancreatic adenocarcinoma, sweetbread (SWD) mutations overexpress the suppressor of cytokine signaling 3a (socs3a) gene, resulting in cell cycle arrest in G0-G1 phases, inhibiting pancreatic epithelial cell growth, causing hypoplastic acini and dysmorphic ducts. In this process, TRPM7 of some cells is activated by SWD mutations, which mediates Mg2+ influx to inhibit socs3a, thus protecting cells from growth defects and enabling normal aging (Yee et al., 2011; Yee et al., 2012b). On the contrary, TRPM8 can prevent gene-induced senescence of cancer cells, which means that it is beneficial to the proliferation and anti-senescence of pancreatic adenocarcinoma cells and promotes the progression of the disease (Yee et al., 2010).
TRPA1 is a calcium-permeable non-selective cation channel, dual thermoreceptor, which is highly expressed in rat pancreatic cells. It is activated by glycolytic products at high glucose concentrations and positively regulated by estrogens and their metabolites (Uchida et al., 2017; Mendez-Resendiz et al., 2020). In mouse islet β cells and rat INS-1 cells, TRPA1 is directly activated by hydroxylated catechol estrogens such as 2-hydroxyestradiol, which accelerates membrane depolarization and calcium influx through synergistic K-ATP channel closure, thereby increasing glucose-induced insulin secretion (Colsoul et al., 2011; Ma et al., 2019; Mendez-Resendiz et al., 2020). However, long-term stimulation of TRPA1 interferes with the transcription of insulin-related factors such as pancreatic and duodenal homeobox 1 (PDX-1) and reduces insulin secretion (Steinritz et al., 2018).
TRPML1, TRPML3, and TRPP1 are expressed in human pancreatic β cells and islets (Marabita and Islam, 2017). TRPML1 is also a Ca2+ osmotic channel, mainly located in intracellular vesicles. Since the activity of TRPML is related to pH, it tends to exist in lysosomes with low pH and can be activated by phosphatidylinositol 3,5-bisphosphate, which is rich in lysosomes. It plays a role in vesicle transport and lysosomal exocrine secretion (Li et al., 2017b; Di Paola et al., 2018). Moreover, TRPML1 mutation can lead to the loss of lysosome function (Sun et al., 2000; Dong et al., 2009). Finally, TRPP1 acts as a Ca2+ permeable non-selective cation channel, which may also promote β cell depolarization (Islam, 2020). The detailed molecular mechanism of TRP channels in the pancreas is shown in Figures 2, 3.
FIGURE 2. Activation of TRP channels in the pancreas. Here is pancreatic β cell or adenoma cell. TRPC1, TRPC3, TRPC4, and TRPA1 are activated by different pathways, mediating extracellular Ca2+ influx in the presence of Ca2+ deficiency in the ER and depolarizing to stimulate insulin secretion. TRPC6 is associated with cell proliferation and tumor migration. TRPV2, which existed on vesicles and ER, was activated by insulin synthesis or altered intracellular and extracellular osmotic pressure under high glucose conditions, translocated to the plasma membrane and increased Ca2+ influx.
FIGURE 3. Activation of TRP channels in the pancreas Ⅱ. Here is pancreatic β cell or adenoma cell. TRPM2, TRPM3, TRPM4, and TRPM7 are also activated to promote insulin secretion. It is noteworthy that TRPM2 needs to bind to Ca2+, TRPM3 mainly mediates Na+ and Zn2+ influx, TRPM4 mainly mediates Na+ influx, and TRPM7 mediates Mg2+ influx. They mainly mediate extracellular Ca2+ influx by changing membrane potential and activating voltage-gated calcium channels. Similar to TRPV2, TRPV4 was activated by osmotic pressure under high glucose conditions. Although TRPV6 exists in INS-1 and inhibits insulin mRNA expression, it does not affect insulin secretion alone.
Salivary gland secretion depends on the increase of Ca2+ in salivary gland cells; when both extracellular and endoplasmic reticulum (ER) Ca2+ are insufficient to maintain the concentration gradient, the Store-Operated Ca2+ Entry (SOCE) mechanism mediates [Ca2+]i (Ambudkar, 2016; Liu et al., 2018). Thus, ion transporters and channels such as Na+/K+/2Cl− cotransporter 1 (NKCC1), Anoctamin 1 (ANO1), and KCa are opened to regulate intracellular and extracellular Cl− concentration and maintain osmotic gradient, allowing water to be secreted through the water channel Aquaporin 5 (AQP5) in the apical membrane (Ambudkar, 2012; Ambudkar, 2014; Liu et al., 2018). In this process, the channels involved in SOCE are mainly TRPC1 and Orai1. Orai1 is an important component of store-operated calcium release-activated calcium and SOC channels, and the role of TRPC1 depends on the activity of the Orai1 channel (Liu et al., 2000; Liu et al., 2007; Cheng et al., 2008; Cheng et al., 2011; Hong et al., 2011; Ambudkar, 2016). Orai1 is first activated to mediate Ca2+ influx, which stimulates the movement of TRPC1 to the plasma membrane after the acinar cells are stimulated by neurotransmitters. Moreover the two channels are coupled into a single channel to increase Ca2+ influx and salivary secretion by activating an ER- Ca2+ binding protein- STIM1 and Caveolin-1 (Zeng et al., 2008; Pani et al., 2009; Cheng et al., 2011; Hong et al., 2011; Pani et al., 2013; Choi et al., 2014; Ambudkar, 2016; Liu et al., 2018).
Except for TRPC1, TRPC3 and TRPC4 also participate in SOCE, but whether TRPC3 participates in SOCE seems to be related to cell type and expression level (Liu et al., 2018). In salivary gland ductal cells, TRPC3 acts in a similar way to TRPC1 that TRPC3 is also associated with Orai1 after being activated by STIM1. However, TRPC3 must bind to TRPC1 before activation; that is, TRPC3 is dependent on TRPC1 to form TRPC3-TRPC1 heteromeric channel (Lee et al., 2014; Ong et al., 2014; Ambudkar, 2016). In pathological conditions, the generation of cytotoxicity in salivary acinar cells during inflammation is related to the Ca2+ influx mediated by TRPC3, which is reflected in ROS production and membrane damage. This phenomenon is similar with the increased of [Ca2+]i in pancreatic acinar cells aggravating pancreatitis. It is suspected that TRPC1 is also involved in this cell damage process (Kim et al., 2011; Ambudkar, 2016).
Loss of salivary gland function leads to xerostomia, often caused by radiation therapy or chronic autoimmune disease Sjögren’s syndrome (pSS), which is associated with an unregulated increase in intracellular Ca2+ (Konings et al., 2005; Mavragani and Moutsopoulos, 2014; Liu et al., 2018). For the loss of salivary gland function caused by pSS, pSS itself leads to the overexpression of TNF-α, interleukin, γ-IFN, and other inflammatory factors (Baturone et al., 2009; Bikker et al., 2010). Moreover, activated granulocytes also increase ROS during inflammation, which is regulated by TRPM2. TRPM2 regulates immune inflammation by regulating the release of inflammatory factors and the growth of dendritic cells (Gasser et al., 2006; Yamamoto et al., 2008; Sumoza-Toledo et al., 2011; Knowles et al., 2013).
TRPM2 is the ROS receptor existing in mouse salivary acinar cells, which may be involved in radiation-induced cell damage and pSS (Sumoza-Toledo and Penner, 2011; Liu et al., 2013; Liu et al., 2018). TRPM2 is activated by NAD+ and its ADPR generated after hydrolysis to guide Ca2+ influx and participate in cell damage when the radiation creates an excessive ROS microenvironment (Perraud et al., 2003; Sumoza-Toledo and Penner, 2011; Ambudkar, 2016; Liu et al., 2018). TRPM2 plays a key role in the relationship between cell damage after radiation and dry mouth (Liu et al., 2013; Teos et al., 2016; Liu et al., 2018). In conclusion, TRPM2 mediates irreversible salivary gland damage after radiation by excessive consumption of STIM1 and dysregulation of SOCE (Liu et al., 2013; Liu et al., 2017). For the recovery of damaged glands after radiation, inhibition of TRPM2 activation can promote the regeneration of glandular cells by keeping STIM1 and SOCE at normal levels (Jang et al., 2016; Liu et al., 2017). Moreover, TRPM2 was not only associated with salivary gland secretion loss, but also inhibited the abnormal shrinkage of acinar cell volume, which is related to the change of osmotic pressure during salivary gland secretion (Ambudkar, 2016). For example, TRPM2 is activated after radiation to regulate ion transporters, changes osmotic gradients, and normalizes cell size through regulatory volume increase (RVI) under the condition of being excited by agonists such as carbachol (CCh) (Liu et al., 2018). In contrast to TRPM2’s RVI, swollen cells that absorb water can return to normal size by the opposite route, known as regulatory volume decrease (RVD). TRPV4 can be activated under hypotonic conditions and play an RVD role by regulating osmotic gradient by guiding Ca2+ influx as well (Liu et al., 2006; Liu et al., 2018). Additionally, TRPV4 also can be activated by 4α-phorbol-12,13 didecanoate, Muscarine, GSK1016790A, and heat. Muscarine leads to salivary secretion by activating TRPV4 through heat, which opens the Cl− transport channel ANO1 (Zhang et al., 2012a; Sobhan et al., 2013; Derouiche et al., 2018).
Among them, TRPV1, TRPM8, TRPV3 and TRPV6 are also found in salivary glands. TRPV1 agonists-capsaicin, piperine, or TRPM8 agonist -menthol increased CCh-induced salivary secretion. Allyl isothiocyanate, the co-antagonist of TRPM8 and TRPA1, decreased the salivary secretion, indicating that the roles of these TRP channels are different (Peng, 2011; Sobhan et al., 2013; Liu et al., 2018; Houghton et al., 2020). TRPC1, TRPV4, TRPM8, and TRPV3 are also involved in the early tubular structure differentiation of salivary gland cells (Fujiseki et al., 2017). The detailed molecular mechanism of TRP channels in the salivary gland is shown in Figure 4.
FIGURE 4. Activation of TRP channels in salivary glands. Here is salivary acinar cell (TRPC3 is present in duct cells). When TRPM2, TRPC1, TRPC3, or TRPV4 are activated and mediate Ca2+ influx, ANO1 and other ion transport channels can be activated to change osmotic pressure and secrete or absorb water through AQP5. TRPA1, TRPV1, and TRPM8 also affect cell secretion but remain to be studied. In addition, too much ROS can damage gland cells, resulting in secretion disorders.
The adrenal gland is a vital endocrine organ of the human body. The gland is divided into two parts, the adrenal cortex and the adrenal medulla (AM). The chromaffin cells in the medulla secrete catecholamine hormones, and the cortex mainly secretes corticosteroids, including aldosterone and glucocorticoids. These hormones always affect the function of the human body, and their balance is of great significance to human health. Many subfamilies of TRP channels are expressed in the adrenal gland and play a significant role in adrenal physiology and pathology.
It has been confirmed that TRPC1, TRPC4, and TRPC5 are expressed in guinea pig AM cells at the protein level. Keita et al. found that STIM1 promotes the formation of TRPC1 with TRPC4 heteromer channels as well as insertion into the cell membrane in PC12 cells and guinea pig AM cells (Harada et al., 2019). TRPC1—TRPC4 heteromeric channels function as store-operated Ca2+ entry channels in endothelial cells (Sundivakkam et al., 2012). And TRPC3 (Goel et al., 2007), TRPC5 (Bezzerides et al., 2004), and TRPC6 (Cayouette et al., 2004) are inserted into the cell membrane in response to stimulation by G protein-coupled receptors (GPCRs) or receptor tyrosine kinases. TRPC1—TRPC4 heteromeric channels, therefore, mediate Ca2+ influx in response to stimulation of muscarinic receptors in guinea pig AM cells.
TRPC channels expression in the adrenal gland is increased in patients with metabolic syndrome, which may be a potential risk of inducing cardiovascular disease (Hu et al., 2009). Renin-angiotensin-aldosterone system activity is significantly increased in metabolic syndrome (Krug and Ehrhart-Bornstein, 19792008; Sarzani et al., 2008). High concentrations of aldosterone can regulate gene expression through corresponding receptors, which include binding to the glucocorticoid response element (GRE) half-site sequence (Olivos and Artalejo, 2008; Bhargava et al., 2004). Mineralocorticoid and glucocorticoid receptors bind to the same response element in the promoter, the GRE. Several GRE-like half-site motifs (AGAACA) were present in the putative promoter region of TRPC1, TRPC5, and TRPC6 channels. These channels are up-regulated in patients with metabolic syndrome. When these channels are activated via the Gq/11-PLCβ pathway or Ca2+ store depleting pathway (Marom et al., 2011), an influx of Ca2+ into chromaffin cells causes depolarization of chromaffin cells and may activate voltage-gated Ca2+ channels to promote their secretion of epinephrine (Burgoyne et al., 1993; Cheek and Barry, 1993). The detailed molecular mechanism is shown in Figure 5. In addition, circulating hormones, such as histamine and angiotensin II, can also stimulate catecholamine exocytosis from adrenal chromaffin cells by activating voltage-independent circulating hormone-operated cation channels (Livett and Marley, 1993; Olivos and Artalejo, 2008; Sala et al., 2008). TRPC1, TRPC5, and TRPC6 are candidates for increasing hormone-induced exocrine secretion in chromaffin cells (Obukhov and Nowycky, 2002). It is well-known that excessive release of epinephrine from the adrenal gland increases the risk of myocardial infarction, cerebrovascular accident, arrhythmia, stroke, while up-regulated TRPC1, TRPC5, and TRPC6 channels are likely to cause cardiovascular disease in patients with metabolic syndrome. Therefore, these channels in the adrenal gland may be a therapeutic intervention target in patients with metabolic syndrome. However, it is not clear whether aldosterone is the only regulator of TRPC channels in the adrenal medulla, and additional molecular factors that further up-regulate TRPC channels may exist during metabolic syndrome.
FIGURE 5. Mineralocorticoid-mediated up-regulation of TRPC5. The GRE-like half-site (AGAACA) in the TRPC5 gene binds to the mineralocorticoid receptor, prompting the up-regulation of TRPC5 channels. TRPC5 is activated to mediate the influx of Ca2+ into chromaffin cells, depolarizing chromaffin cells and prompting their secretion of catecholamines.
TRPV1 is a polymodal receptor activated by physical and chemical stimulation, including heat (above 43°C) and changes in pH (both acidic and alkaline), endovanilloids, such as anandamine, N-acyldopamines, and a variety of exogenous agonists, which mainly consist of capsaicin (Zsombok, 2013; Moran and Szallasi, 2018). TRPV1 was upregulated in chromaffin cells of neuropathic animals (Arribas-Blázquez et al., 2019). Meanwhile, Pablo et al. found that stress significantly increased the mRNA levels of TRPV1 channels in adrenal cells (Surkin et al., 2018). TRPV1 is a ligand-gated, non-selective cation channel with high permeability to Ca2+ and therefore Ca2+ influx in adrenocortical cells when TRPV1 is activated (Moran and Szallasi, 2018). High levels of intracellular Ca2+ concentration may suppress glucocorticoid production (Matthews and Saffran, 1973). Capsaicin inhibits lipopolysaccharide-induced intracellular steroidogenesis in adrenocortical cells by activating TRPV1 and increasing intracellular Ca2+ levels, which may prevent the development of central nervous system disorders in patients with severe sepsis (Ferreira et al., 2019). In summary, TRPV1 channels in the adrenal gland are upregulated when the body is under stress or neuropathological conditions. Excessive expression of TRPV1 after activation by its agonists may attenuate the release of corticosteroids to some extent and thus protect the body. Finally, TRPV1 is a driver to increase the rapid release of corticosterone and epinephrine. Dekel et al. proposed an alternative strategy to modulate peripheral organ function (Rosenfeld et al., 2020). They developed a magnetothermal switch for the on-demand release of the adrenal hormones epinephrine and corticosterone by controlling TRPV1 activation in vitro. They use this approach to control adrenal hormone secretion in genetically intact rats wirelessly. Since alterations in the levels of these hormones are associated with psychiatric disorders such as post-traumatic stress disorder and depression, their approach may be helpful to study the physical and psychological effects of stress. While TRPV1 (and other thermosensitive TRP channels) was shown to be expressed in other organs deep in the body, such as peripheral nerves, gastrointestinal tract, pancreas, and heart (Akbar et al., 2008; Schwartz et al., 2011; Randhawa and Jaggi, 2017), the potential application of magnetothermal deep organ stimulation as means to study organ function paves the way for the development of bioelectronic medicines.
TRPA1 is a Ca2+ -permeable non-selective cation channel that can be activated by various toxic or irritating substances in nature. Allyl isothiocyanate (AITC) and cinnamaldehyde (CAN) increase epinephrine secretion by activating TRPA1-expressing adrenal sympathetic nerves in rats (Iwasaki et al., 2008). TRPA1 is frequently coexpressed with TRPV1, raising the possibility that TRPA1 and TRPV1 mediate the function of a class of polymodal nociceptors (Liedtke and Heller, 2007). Yuriko et al. found that oleuropein aglycone (OA) stimulates norepinephrine secretion by activating TRPA1 and TRPV1, thereby enhancing UCP1 receptor expression in brown adipose tissue (BAT) (Oi-Kano et al., 2017). BAT is the organ responsible for heat production in the human body, and activation of adrenergic receptors expressed in BAT can increase UCP1 receptor expression (Himms-Hagen et al., 1994; Nagase et al., 1996; Cannon and Nedergaard, 2004). The activation of TRPV1 is a key link in capsaicin-induced epinephrine secretion. Capsaicin improves body energy metabolism by promoting catecholamine secretion and up-regulating UCP1 receptor in rats (Kawada et al., 1986; Watanabe et al., 1987; Watanabe et al., 1988; Kobayashi et al., 1998). Also, AITC increases body heat production and expression of UCP1 receptors by activating TRPA1 channels (Yoshida et al., 1988). We hypothesize that TRPA1, when expressed alone, can be activated by its agonist AITC, thereby inducing catecholamine secretion from the adrenal gland. Activation of TRPA1 is therefore likely to be one of the pathways by which the body increases energy metabolism as well as increases heat production, but there is currently insufficient evidence to elucidate the mechanism by which TRPA1 mediates catecholamine secretion after activation. However, not all TRPA1 activation can mediate the increased activity of adrenal sympathetic nerves. Some studies have found that β-eudesmol inhibits adrenal sympathetic nerve activity (ASNA) by activating TRPA1 (Ohara et al., 2018). Kazuaki et al. identified three key amino acid residues in TRPA1, namely threonine 813, tyrosine 840, and serine 873, which can be activated by β-eudesmol (Ohara et al., 2015).
TRPM7 has a very broad tissue distribution as a member of the TRP channel superfamily. Its gene encodes a persistently open calcium channel regulated by intracellular Mg2+/ATP. It has been shown that calcium influx caused by TRPM7 plays a key role in the survival of cells (Nadler et al., 2001). Bonnie et al. found that rat adrenal pheochromocytoma (PC12) cells expressing the receptor (LEPRb) showed significant induction of promoter activity and TRPM7 expression after leptin treatment (Yeung et al., 2021). They found that activated pSTAT3 epigenetically regulates the transcription of TRPM7 through DNA methylation and histone modifications. Specifically, it is manifested in a reduction in CpG site-specific methylation and H3K27 (H3 [histone 3] K27 [lysine 27]) trimethylation and an increase in H3K27 acetylation and H3K4 (H3 lysine 4) trimethylation at the TRPM7 promoter. The detailed molecular mechanism is shown in Figure 6. Therefore, TRPM7 channels may be up-regulated when plasma levels are increased in vivo due to obesity, and we speculated that when this event occurs in adrenal chromaffin cells, more Ca2+ influx due to up-regulation of TRPM7 channels may induce increased catecholamine secretion, which is likely to be one of the reasons associated with hypertension. Fortunately, epigenetic changes are reversible, and epigenetic modifications targeting TRPM7 may serve as a novel therapeutic approach for the treatment of obese hypertension.
FIGURE 6. Leptin induces epigenetic regulation of TRPM7. Activated pSTAT3 epigenetically regulates transcription of TRPM7 through DNA methylation and histone modifications. Specifically, it is manifested in a reduction in CpG site-specific methylation and H3K27 trimethylation and an increase in H3K27 acetylation and H3K4 trimethylation at the TRPM7 promoter.
TRPM4 has been demonstrated to be expressed in mouse adrenal medulla (AM) cells at the mRNA levels (Mathar et al., 2010a). Masumi et al. found that pituitary adenylate cyclase-activating polypeptide (PACAP) induced catecholamine secretion in mouse AM cells, which may be due to the generation of depolarizing inward currents caused by TRPM4 channel activation (Inoue et al., 2020). Application of the endogenous PKC activator 1-oleoyl-2-actyl-sn-glycerol mimics PACAP activation of Na+ -permeable cation channels in bovine AM cells (Tanaka et al., 1996), and therefore, PACAP may also activate TRPM4 in an AM-cell PKA-dependent manner. Differently, PACAP does not directly induce the secretion of catecholamines in guinea-pig AM cells, but rather promotes muscarinic receptor-mediated activation of Ca2+-activated nonselective cation (NSC) channels, and this promotion is mainly due to the increased insertion of heteromeric TRPC1-TRPC4 channels into the cell membrane (Harada et al., 2019). Ilka et al. found that mice with Trpm4 gene deletion lost long-term regulation of blood pressure stability, which they concluded was caused by elevated plasma levels of epinephrine and norepinephrine after excluding the cause of altered cardiac function (Mathar et al., 2010b). However, the molecular mechanism of how TRPM4 regulates or promotes release from chromaffin cells remains to be clarified but may include direct dependence on TRPM4 to regulate vesicle release, as shown for another TRPM7 in cholinergic synaptic vesicles (Krapivinsky et al., 2006). These results suggest that TRPM4 activity may be beneficial in limiting elevated blood pressure in hypertensive patients. Hypertension is a potential risk factor for cardiovascular disease. In most cases, its pathogenesis remains unclear. The above lists two TRPM channels that affect the body’s blood pressure level by changing the secretion of catecholamines by adrenal chromaffin cells, demonstrating the feasibility of TRP channels as a therapeutic target for hypertension, but there are still not many studies in this area and the mechanism by which TRP channels regulate blood pressure needs to be further explored.
The mammary gland is an accessory gland to the skin. Adult women have no secretory activity of the mammary gland when they are not pregnant, called the quiescent mammary gland. Mammary gland hyperplasia during pregnancy and exuberant secretion during lactation are called active mammary glands. TRP channels are expressed in normal mammary epithelial cell, and play an important role in Ca2+ transport in mammary epithelial cells. Anantamongkol et al. found that the expression of TRPC1, TRPC5, and TRPC7 increased during early lactation (Anantamongkol et al., 2009). Mammalian TRPC channels can be activated by receptor-operated or store-operated mechanisms to mediate Ca2+ influx, thereby prompting breast milk secretion (Ambudkar, 2006). It is therefore likely that TRPC channels that are upregulated prepare for extensive and sustained epithelial cell activity to support milk production and/or secretion. However, another study reported decreased expression of TRPC1 during lactation and early mammary involution stages in mice (McAndrew et al., 2011). VanHouten et al. showed that TRPV5 expression was lower in mammary epithelial cells during pregnancy and lactation than in quiescent mammary epithelial cells. In addition, TRPV6 is expressed in the mammary gland of quiescent mice, but its expression shows a decreasing trend throughout pregnancy and is not expressed during lactation (VanHouten and Wysolmerski, 2007).
The widely distributed TRPV4 cationic channel participates in the transduction of mechanical and/or osmotic stimuli in different tissues (Liedtke and Friedman, 2003; Gao et al., 2003). TRPV4 is selectively localized in the basolateral membrane compartment, where it is activated by 4-a-phorbol12,13-didecanoat (4a-PDD) (Vriens et al., 2007), resulting in calcium entry, which on the one hand activates Ca2+-activated K+ channels (BK) and provides a transcellular ion pathway. On the other hand, it increases the permeability of mammary epithelial cells, which is created by a down-regulation of sealing-type claudin proteins accompanied by an altered tight junction structure (Reiter et al., 2006). The detailed molecular mechanism is shown in Figure 7. Md Aminul et al. found that both low permeability tight junctions of mammary epithelial cells and milk production were enhanced after a mild heat treatment at 39°C. TRPV4 activity was increased during mild heat treatment at 39°C. Activation of TRPV4 by GSK1016790A leads to Ca2+ influx, promotes Ca2+ release from the endoplasmic reticulum (ER), and depletes Ca2+ stores, at which point the unfolded protein response (UPR) is initiated and upregulates the transcript levels of Xbp1s through PERK, IRE1, and ATF6 receptor-mediated signaling pathways (Harding et al., 2000; Celli et al., 2011; Shen et al., 2019). Upregulation of the Xbp1 gene increases the expression of β-casein (β-casein is a differentiation marker of HC11 cells and is a representative milk protein), Zo-1, ocln, and Cldn3 mRNA, and thereby regulates the expression of TJ protein-coding genes. Interestingly, TRPV4 expression is increased during pregnancy but decreased during lactation (Islam et al., 2020). Mammary epithelial cells are exposed to temperatures above body temperature during lactation due to metabolism (Berman et al., 1985). Heat stress induces ER stress and causes UPR (Xu et al., 2011; Kim et al., 2013). Elevated transcript levels of Chop decrease the viability of cells and therefore cause reduced lactation (Urra et al., 2013). The detailed molecular mechanism is shown in Figure 8. TRP channels cover the temperature sensing range, TRPV4 is activated at 39°C, but there may be other TRPs that are activated at higher temperatures, and although there is no evidence so far, it provides direction and ideas for later studies.
FIGURE 7. Regulation of transcellular and paracellular pathways by TRPV4 in HC11 cells. TRPV4 induces Ca2+ influx by activation by 4-PDD, which activates BK channels to provide transcellular ion channels. The process down-regulates sealed claudin proteins and alters the structure of tight junctions, increasing paracellular permeability and facilitating milk production.
FIGURE 8. Regulation of TRP Channels on breast cells at different temperatures. The activity of TRPV4 increased at 39 °C. Activation of TRPPV4 by GSK1016790A leads to Ca2+ influx, promotes Ca2+ release from the ER, and depletes Ca2+ stores, at which point the UPR is initiated to upregulate transcript levels of Xbp1s via the PERK, IRE1, and ATF6 signaling pathways. This increased the expression of Zo-1, occln and Cldn3 mRNA and enhanced the expression of β-casein and TJ protein-coding genes. The presence of a certain TRP channel up-regulates the apoptosis transcription factor CHOP and promotes apoptosis through the UPR pathway at 41 °C.
TRPV6 is expressed in normal breast and breast cancer cell lines. A positive correlation between expression of the transcription factor zinc finger homeobox 3 (ZFHX3) and promoter activity of TRPV6 was found by Dan et al. (Zhao et al., 2019). Previous studies have shown that Ca2+ influx mediated by TRPV6 activation is able to induce keratinocyte differentiation (Lehen’kyi et al., 2011; Lehen’kyi et al., 2007). Ca2+ has been shown to be involved in controlling the growth of breast cancer cells through its interaction with calmodulin (Strobl et al., 1995). TRPV6 channel-mediated Ca2+ influx is involved in cell proliferation, which may be associated with breast cancer cell migration (Bolanz et al., 2008; Bolanz et al., 2009).
TRPC1 expression is increased in invasive ductal carcinoma (Guilbert et al., 2008; Dhennin-Duthille et al., 2011; Mandavilli et al., 2012). TGFβ-induced Epithelial-to-Mesenchymal Transition (EMT) is dependent on Ca2+ entry via the TRPC1- Stromal Interaction Molecule 1 (STIM1) complex that leads to the activation of calpains and matrix metalloproteinases (MMPs), which target proteins involved in cellular adherence and promote their migration (Schaar et al., 2016). The detailed molecular mechanism is shown in Figure 9. Therefore, inhibition of the TRPC1-STIM1 complex may be an attractive target for the treatment of breast cancer metastasis. Naoya et al. found that LPA/LPAR3 signaling and subsequent TRPC3 activation leaded to an increase in the number of breast cancer stem cells (BCSCs) through calcium-dependent transcriptional activation of IL-8 by NFAT (Hirata et al., 2022). Polyunsaturated fatty acids (PUFA) and TRPC3 antagonists continuously inhibit the proliferation and migration of breast cancer cells (Zhang et al., 2012b), but the mechanism is still unclear. As one of the biomarkers of breast cancer development and migration, TRPC3 represents a potential target for a new class of anticancer drugs. In the future, specific therapeutic approaches to reduce tumorigenesis may be established by targeting the LPA/LPAR3 pathway and TRPC3 channels. Overexpression of TRPC5 induces chemoresistance by up-regulating of P-glycoprotein (P-gp) and hypoxia-inducible factor-1α in chemoresistant breast cancer cells (Zhu et al., 2015; Ma et al., 2012a). TRPC5-mediated Ca2+ entry stimulates P-gp overproduction through NFATc3 in breast cancer cells (Ma et al., 2012b). P-gp expels intracellular Adriamycin (ADM) into the extracellular matrix, leading to drug resistance. The detailed molecular mechanism of TRPC3 and TRPC5 is shown in Figure 10. In addition, TRPC5 mediates cytoprotective autophagy through the CaMKKβ/AMPKα/mTOR pathway, causing drug resistance in breast cancer cells (Zhang et al., 2017). Inhibition of P-gp activity and avoidance of autophagy is therefore an attractive approach to overcome multidrug resistance in cancer chemotherapy, and TRPC5 is a valuable target.
FIGURE 9. TRPC1-STIM1 complex mediates TGF-β-induced EMT. STIM1 aggregates to the endoplasmic reticulum-plasma membrane (ER-PM) junctions, forming the TRPC1-STIM1 complex with TRPC1, mediating the down-regulation of E-cadherin and leading to the activation of calproteinases and MMPs, which target proteins involved in cell adhesion and promote their migration.
FIGURE 10. TRPC3 and TRPC5 mediate breast cancer cell proliferation and drug resistance. Ca2+ entry through TRPC3 and TRPC5 channels, via calmodulin-calcineurin dependent NFAT signal pathway, upregulating IL-8 and P-gp expression respectively. IL-8 mediates BCSC proliferation through CXC receptor 2. Up-regulated P-gp expels intracellular ADM into the extracellular matrix, leading to drug resistance.
The gallbladder is an exocrine digestive gland of the human body that mainly serves to concentrate and store bile. The contractile emptying of the gallbladder is regulated by hormones, and cholecystokinin stimulates contraction of the gallbladder smooth muscle (GBSM) layer and excretion of bile after eating. TRPP2 channel protein belongs to the superfamily of transient receptor potential (TRP) channels and is widely expressed in the smooth muscle of the digestive tract, including gallbladder smooth muscle. TRPP2 can not only mediate intracellular Ca2+ release from Ca2+ stores, but also regulate extracellular Ca2+ influx and enhance intracellular Ca2+ concentration ([Ca2+]i) (González-Perrett et al., 2001; Koulen et al., 2002).
It has been shown that TRPP2 channels are widely distributed in the plasma membrane and endoplasmic reticulum (ER) (Tsiokas, 2009). Xingguo et al. found that knockdown of TRPP2 protein in GBSM of guinea pigs significantly decreased Ca2+ release and extracellular Ca2+ influx evoked by carnosine (CCh), thereby inhibiting endothelin-1 (ET-1) and cholecystokinin-induced gallbladder contraction (Zhong et al., 2016). Cholecystokinin (CCK) and ET-1 receptors play an important role in GBSM contraction. CCK and ET-1 receptors, as members of the G protein-coupled receptor (GPCR) superfamily, can activate phospholipase C (PLC) and guide the hydrolysis of phosphatidylinositol 4,5-bisphosphate to produce diacylglycerol and inositol 1,4,5-triphosphate (IP3) (Noble et al., 1999). IP3 increases local cytosolic Ca2+ concentration by activating the IP3 receptor to release Ca2+ from Ca2+ stores, which may activate TRPP2 causing the further release of Ca2+ (Li et al., 2005; Sammels et al., 2010). Depletion of Ca2+ stores will subsequently initiate SOCE. An increase in [Ca2+]i is a key event in eliciting smooth muscle contraction in response to agonists (Karaki et al., 1997). In addition, studies have shown that TRPP2 is also present in the plasma membrane and mediates Ca2+ influx (Du et al., 2012; Narayanan et al., 2013). In summary, TRPP2-mediated Ca2+ release expressed on the ER membrane plays an important role in agonist-induced GBSM contraction. GPCRs are key points in initiating TRPP2 opening through the PLC-IP3 pathway. The detailed molecular mechanism is shown in Figure 11.
FIGURE 11. TRPP2 mediates gallbladder smooth muscle contraction. CCK and ET-1 receptors can activate PLC and guide the hydrolysis of phosphatidylinositol 4,5-bisphosphate to produce diacylglycerol and IP3. IP3 increases local [Ca2+]i by activating IP3R to release Ca2+ from Ca2+ stores, which in turn activates TRPP2 to cause further release of Ca2+. Depletion of Ca2+ stores will subsequently initiate SOCE. The increase in [Ca2+]i causes contraction of gallbladder smooth muscle.
However, in addition to TRPP2 channels, many other ion channels similarly mediate smooth muscle Ca2+ homeostasis and contractility. An example is a physical and functional interaction between TRPC1 or TRPC4 channels and STIM1 thought to contribute to SOCE (Sours-Brothers et al., 2009). TRPC4 and TRPC6 channels depolarize muscarinic receptor-coupled intestinal smooth muscle cells and voltage-activated Ca2+ influx and contraction, thereby accelerating small intestinal motility in vivo (Tsvilovskyy et al., 2009). Sara Morales et al. found that guinea pig gallbladder smooth muscle contains mRNAs encoding TRPC1, TRPC2, TRPC3, and TRPC4 proteins whose abundance depends on cytosolic Ca2+ concentration. They found that lowering the level of cellular Ca2+ with the use of Ca2+ chelators such as EGTA and BAPTA AM leads to a decrease in the expression of all TRPC members found in GBSM, whereas elevations in cellular Ca2+ as a result of Ca2+ influx or store depletion leads to an increase in TRPC gene expression (Morales et al., 2007). Therefore, we cannot deny that there are other ion channels mediating Ca2+ homeostasis in gallbladder smooth muscle cells, and the interaction between these ion channels still needs to be explored in the future, and the detailed mechanism of gallbladder smooth muscle contraction still needs to be further elucidated.
Human skin has more than two million sweat glands and plays a great role in excreting waste products and maintaining water and salt balance. Sufficient studies have shown that TRP channels are abundantly expressed in the skin, and various TRP channels are involved in the formation and maintenance of the skin barrier, the growth of HF, and the immune and inflammatory processes of the skin, thereby maintaining skin homeostasis and promoting the occurrence of a variety of skin diseases. More importantly, several skin-expressed TRP channels act as primary sensors for temperature, mechanical, and chemical stimuli, modulating our temperature, touch, itch, and pain perception under physiological and pathological conditions. TRPV4 channels are present in cutaneous vascular endothelial cells and eccrine secretory cells (Kida et al., 2012; Fusi et al., 2014; Olivan-Viguera et al., 2018), and activation of the channel increases the concentration of intracellular Ca2+. If it has an important function in the cell types described above, it leads to vasodilatation (Félétou and Vanhoutte, 2009) and sweat secretion (Sato and Sato, 1981; Metzler-Wilson et al., 2014). Naoto et al. showed that TRPV4 channel activation mediates cutaneous vasodilatation but has no effect on sweating and that activation of TRPV4 channels alone is not sufficient to induce sweat secretion in humans. However, TRPV4 channel activation may increase calcitonin gene-related peptide (Gao and Wang, 2010), a peptide that has been shown to increase muscarinic sweating in humans (Schlereth et al., 2006). TRPV6 is involved in the uptake of ca ions and regulates intracellular ca ion levels in keratinocytes. Extracellular ca ion-induced differentiation up-regulated the mRNA and protein levels of TRPV6, and keratinocyte differentiation was affected after siRNA silencing of TRPV6 in human primary keratinocytes (Lehen’kyi et al., 2007). However, there are no excessive studies to elucidate the link between TRP channels and sweat glands, and there may be other TRP channels other than TRPV4 that mediate the secretion of sweat glands.
In lacrimal glands, some TRP channels are involved in tear secretion and inflammation. Among them, TRPM3 located in the apical membrane of lacrimal gland epithelium may promote the development of lacrimal gland in the early mouse embryo (Kanewska et al., 2020). TRPV1 is also expressed in lacrimal glands and may play a role in regulating Ca2+ and water transport (Martinez-Garcia et al., 2013). In addition, the role of TRPV4 in the lacrimal gland is the same as that in the salivary gland, which is also activated at moderate temperature and regulates the transporter ANO1 through the influx of Ca2+ to enhance the secretion of the lacrimal gland, which is manifested by the tearing effect of muscarinic (Derouiche et al., 2018). However, additional studies on the specific mechanisms of TRP channels in lacrimal glands remain to be conducted.
In this article we review the specific regulatory mechanisms of various TRP channels in some common glands (pancreas, salivary gland, lacrimal gland, adrenal gland, gallbladder, sweat gland). Except the tissues discussed above, TRP channels are also reported to have important functions in other tissues. TRPC5 together with TRPA1 are capable for cold sensing in teeth (Bernal et al., 2021). Meanwhile TRPC5, which is expressed in dopamine ARC neurons, has also been reported to regulate prolactin homeostasis (Blum et al., 2019). TRPM2, which is expressed in a subpopulation of neurons in hypothalamic, is a temperature sensor and able to regulates the fever response (Song et al., 2016).
As summarized above, TRP channels are been participated in pathology of diverse system. Therefore, drug discovery targeting TRP channels to cure different disorders has grown. Moreover, majority of TRP channels are located at the cell surface, which means they are easily accessible target. Many members of the TRP channel superfamily are abundantly expressed in the pancreas, and their activity is closely related to the physiological and pathological processes of the pancreas. TRPA1 can be activated by a variety of substances to promote Ca2+ influx to induce insulin secretion, but maintaining the activated state for a long time will instead inhibit insulin secretion (Steinritz et al., 2018). TRPV1 is expressed in sensory nerve fibers in the mouse pancreas and does not mediate insulin secretion (Diaz-Garcia et al., 2014), but is closely linked to the physiological function of β-cells (Razavi et al., 2006; Najder et al., 2018; Zhong et al., 2019), in addition to mediating the release of inflammatory mediators and the production of pain sensation in acute pancreatitis (Schwartz et al., 2011; Schwartz et al., 2013). There is no disease-modifying treatment for people with type 2 diabetes mellitus. Accumulating evidence suggests that pharmacological blockade of TRPV1 with the small molecule antagonist BCTC291 improves oral glucose tolerance and glucose-stimulated insulin secretion. Another TRPV1 antagonist, XEN-D0501, is currently undergoing phase II clinical trials in T2DM patients with good tolerability and safety. The combination of TRPV1 and TRPA1 antagonists also protects sensory nerves and prevents cognitive decline in patients with T2DM. TRPC1, TRPC3, and TRPC4 is expressed in pancreatic β-cells and can be activated by various pathways such as G-protein-coupled receptors and mechanical stimuli to mediate insulin secretion under physiological conditions (Hayes et al., 2013; Park et al., 2013; Srivastava et al., 2018), but the activation of these TRPCs in PDAC is a major cause of PSCs migration and chemotaxis, which will aggravate the development of the disease. These TRPCs have the potential to be a biological indicator for the diagnosis and treatment of PDAC, but there is no excessive literature to support the conjecture. The TRPM subfamily is the most reported TRP channel expressed in the pancreas, in which TRPM2, TRPM4, and TRPM5 mediates Ca2+ influx with significant differences in activation patterns, while TRPM3 and TRPM7 is also permeable to Zn2+, and they all play a role in insulin secretion. It is worth noting that TRPM2, TRPM7, and TRPM8 is involved in the progression of pancreatic cancer, and TRPM7 and TRPM8 is overexpressed in pancreatic cancer tissues and has become a marker for judging cancer cell proliferation in clinical practice (Yee et al., 2011; Yee et al., 2012a). Interestingly, they play an opposite role in pancreatic cancer. TRPM7 has a protective effect on pancreatic cancer, but TRPM8 can promote the progression of the disease, which provides a new target and idea for the treatment of pancreatic cancer. In addition, other TRPMs channels have not been reported in pancreatic diseases, and their role in the disease is still unknown. Many TRPC members are expressed in the mammary gland and are closely associated with the development and metastasis of breast cancer. Adriamycin is one of the drugs for the treatment of breast cancer in clinical practice, but the up-regulation of TRPC5 makes tumor cells resistant to adriamycin and promotes the metastasis of tumor cells (Ma et al., 2012b; Zhang et al., 2017). Blocking TRPC5 activation may provide new clinical treatment options for adriamycin-resistant breast cancer. In addition, digoxin may have a therapeutic effect on Triple-negative breast cancers (TNBC) cell lines with high TRPC1 and TRPC4 expression (Grant et al., 2019). Some other diseases and related drugs currently studied are summarized in Supplementary Table S1.
Much of our understanding of the contribution of TRP channels to disease comes from studies of in vitro systems or preclinical rodent models, which do not always reflect human disease. In this context, endocrine-related diseases caused by abnormal TRP channels provide some uncertainty. In terms of drug discovery, diseases caused by functional overactivation of TRP channels may be easier to treat because small molecules (e.g., targeted nanodrugs) can inhibit overactivation of TRP channels on the surface of cell membranes; Diseases caused by loss of TRP channel function, especially gene mutations, are difficult to target with small molecules and may require a less-validated approach, such as gene therapy, DNA-based nanostructures, or organoid therapy, to restore normal TRP channel function. In addition to directly reversing dysfunctional TRP channels, there may be benefits in regulating the function of intact TRP channels through therapeutic interventions when diseases associated with these channel diseases are caused by other genetic mutations or environmental factors.
In summary, due to the diversity and specificity of TRP channels, a variety of customized TRP channel-based structures have been created. The mechanism of action of endocrine-related TRP channels summarized in this paper provides valuable directions for biomedical development. On salivary secretion, the role of TRPC1, TRPM2 and TRPV4 in the physiological and pathological processes of salivary glands has been to be explored (Ambudkar, 2016; Liu et al., 2018). Some TRP channel superfamily members are expressed in the lacrimal gland and mainly mediate the development and secretion of the lacrimal gland, but the mechanism remains to be studied (Martinez-Garcia et al., 2013; Derouiche et al., 2018; Kanewska et al., 2020). TRPA1, TRPV1, TRPM4 and TRPM7 has been shown to affect adrenaline production (Iwasaki et al., 2008; Mathar et al., 2010b; Ferreira et al., 2019; Yeung et al., 2021). TRP channels are also expressed in sweat and lacrimal glands, and there is currently insufficient evidence for the association of TRP channels with sweat gland secretion, TRPV6 and TRPM3 promote cell development in sweat and lacrimal glands, respectively, and TRPV4 plays a role in enhancing lacrimal gland secretion (Derouiche et al., 2018; Lehen’kyi et al., 2007; Kanewska et al., 2020). TRPM7, TRPM8, and TRPV6 have been used as diagnostic and prognostic markers of breast cancer in clinical practice (Lehen’kyi et al., 2012; Van Haute et al., 2010; Chan et al., 1991; Adams et al., 2003; Zhuang et al., 2002; Tsavaler et al., 2001; Wang et al., 2009), but because the molecular markers are still insufficient in the judgment of prognosis, and it is necessary to explore the correlation between more types of TRP channels and breast cancer, in addition to the lack of specific drug inhibitors of these channels is also an obstacle that must be overcome.
These problems are real, but may not be insurmountable, and the potential benefits are considerable. If drug discovery companies can find creative ways to harness TRP pathways in diseases, they may be able to develop novel, state-of-the-art drugs.
YLi and YLy collected the materials and wrote the manuscript. HW designed the research and revised the manuscript.
This work was financially supported by the National Natural Science Foundation of China (81602327), and the Zhishan Scholars Programs of Southeast University (2242021R41070).
The authors declare that the research was conducted in the absence of any commercial or financial relationships that could be construed as a potential conflict of interest.
All claims expressed in this article are solely those of the authors and do not necessarily represent those of their affiliated organizations, or those of the publisher, the editors and the reviewers. Any product that may be evaluated in this article, or claim that may be made by its manufacturer, is not guaranteed or endorsed by the publisher.
The Supplementary Material for this article can be found online at: https://www.frontiersin.org/articles/10.3389/fmolb.2022.895814/full#supplementary-material
AM, adrenal medulla; ADPR, ADP ribose; AITC, Allyl isothiocyanate; ANO1, Anoctamin 1; AQP5, Aquaporin 5; ASNA, adrenal sympathetic nerve activity; BK, Ca2+ -activated K+ channels; BAT, brown adipose tissue; cAMP, cyclic adenosine monophosphate; CAN, cinnamaldehyde; CCh, carbachol; DAG, diacyl glycerol; CGRP, calcitonin-gene-related peptide; CLICs, intracellular chloride channels; [Ca2+]i, cytosolic [Ca2+]; CCK, Cholecystokinin; ER, endoplasmic reticulum; GBC, gallbladder cancer; EMT, epithelial-to-mesenchymal transition; ET-1, endothelin 1; GPCR, G protein-coupled receptor; GBSM, gallbladder smooth muscle; GPR, G protein-coupled receptor; GLP-1, glucagon-like peptide 1; GRE, glucocorticoid response element; IDC, invasive ductal carcinoma; IP3, inositol 1,4,5-triphosphate; KCa, Ca2+-dependent K+; NAD+, Nicotinamide adenine dinucleotide; NKCC1, Na+/K+/2Cl− cotransporter 1; OA, oleuropein aglycone; NSC, nonselective cation channels; PACAP, pituitary adenylate cyclase-activating polypeptide; PDAC, pancreatic ductal adenocarcinoma; PDX-1, pancreatic and duodenal homeobox 1; PIP2, phosphatidylinositol bisphosphate; PregS, pregnenolone sulfate; pSS, Sjögren’s syndromeSP, substance P; STIM, stromal interaction molecule; SWD, sweetbread; socs3a, suppressor of cytokine signaling 3a; PKC, protein kinase C; PLC, phospholipase C; PSC, pancreatic stellate cell; ROS, reactive oxygen species; RVI, regulatory volume increase; RVD, regulatory volume decrease; SOC, store-operated Ca2+; SOCE, store-operated Ca2+ entry; TRP, transient receptor potential; TRPC, transient receptor potential canonical; TRPV, transient receptor potential vanilloid, TRPM, transient receptor potential melastatin, TRPA, transient receptor potential ankyrin; TRPP, transient receptor potential polycystic; TRPML, transient receptor potential mucolipin; Xbp1s, X-box binding proteins; UPR, unfolded protein response; ZFHX3, zinc finger homeobox 3; 4a-PDD, 4-a-phorbol12,13-didecanoat.
Adams, M., Meijer, O. C., Wang, J., Bhargava, A., and Pearce, D. (2003). Homodimerization of the Glucocorticoid Receptor Is Not Essential for Response Element Binding: Activation of the Phenylethanolaminen -methyltransferase Gene by Dimerization-Defective Mutants. Mol. Endocrinol. 17, 2583–2592. doi:10.1210/me.2002-0305
Akbar, A., Yiangou, Y., Facer, P., Walters, J. R. F., Anand, P., and Ghosh, S. (2008). Increased Capsaicin Receptor Trpv1-Expressing Sensory Fibres in Irritable Bowel Syndrome and Their Correlation with Abdominal Pain. Gut 57, 923–929. doi:10.1136/gut.2007.138982
Ambudkar, I. S. (2014). Ca(2)(+) Signaling and Regulation of Fluid Secretion in Salivary Gland Acinar Cells. Cell Calcium 55, 297–305. doi:10.1016/j.ceca.2014.02.009
Ambudkar, I. S. (2006). Ca2+ Signaling Microdomains:platforms for the Assembly and Regulation of Trpc Channels. Trends Pharmacol. Sci. 27, 25–32. doi:10.1016/j.tips.2005.11.008
Ambudkar, I. S. (2016). Calcium Signalling in Salivary Gland Physiology and Dysfunction. J. Physiol. 594, 2813–2824. doi:10.1113/JP271143
Ambudkar, I. S. (2012). Polarization of Calcium Signaling and Fluid Secretion in Salivary Gland Cells. Curr. Med. Chem. 19, 5774–5781. doi:10.2174/092986712804143321
Amini, M., Chang, Y., Wissenbach, U., Flockerzi, V., Schlenstedt, G., and Beck, A. (2021). Activity of the Yeast Vacuolar Trp Channel Trpy1 Is Inhibited by Ca(2+)-Calmodulin Binding. J. Biol. Chem. 297, 101126. doi:10.1016/j.jbc.2021.101126
Anantamongkol, U., Charoenphandhu, N., Wongdee, K., Teerapornpuntakit, J., Suthiphongchai, T., Prapong, S., et al. (2009). Transcriptome Analysis of Mammary Tissues Reveals Complex Patterns of Transporter Gene Expression during Pregnancy and Lactation. Cell Biol Int 34, 67–74. doi:10.1042/CBI20090023
Aoyagi, K., Ohara-Imaizumi, M., Nishiwaki, C., Nakamichi, Y., and Nagamatsu, S. (2010). Insulin/phosphoinositide 3-kinase Pathway Accelerates the Glucose-Induced First-phase Insulin Secretion through Trpv2 Recruitment in Pancreatic Beta-Cells. Biochem. J. 432, 375–386. doi:10.1042/BJ20100864
Arribas-Blázquez, M., Olivos-Oré, L. A., Barahona, M. V., Sánchez De La Muela, M., Solar, V., Jiménez, E., et al. (2019). Overexpression of P2x3 and P2x7 Receptors and Trpv1 Channels in Adrenomedullary Chromaffin Cells in a Rat Model of Neuropathic Pain. Int. J. Mol. Sci. 20, 155. doi:10.3390/ijms20010155
Badheka, D., Borbiro, I., and Rohacs, T. (2015). Transient Receptor Potential Melastatin 3 Is a Phosphoinositide-dependent Ion Channel. J. Gen. Physiol. 146, 65–77. doi:10.1085/jgp.201411336
Barthel, A., Benker, G., Berens, K., Diederich, S., Manfras, B., Gruber, M., et al. (2019). An Update on addison's Disease. Exp. Clin. Endocrinol. Diabetes 127, 165–175. doi:10.1055/a-0804-2715
Baturone, R., Soto, M. J., Marquez, M., Macias, I., de Oca, M. M., Medina, F., et al. (2009). Health-related Quality of Life in Patients with Primary Sjogren's Syndrome: Relationship with Serum Levels of Proinflammatory Cytokines. Scand. J. Rheumatol. 38, 386–389. doi:10.1080/03009740902973821
Berman, A., Folman, Y., Kaim, M., Mamen, M., Herz, Z., Wolfenson, D., et al. (1985). Upper Critical Temperatures and Forced Ventilation Effects for High-Yielding Dairy Cows in a Subtropical Climate. J. Dairy Sci. 68, 1488–1495. doi:10.3168/jds.s0022-0302(85)80987-5
Bernal, L., Sotelo-Hitschfeld, P., König, C., Sinica, V., Wyatt, A., Winter, Z., et al. (2021). Odontoblast Trpc5 Channels Signal Cold Pain in Teeth. Sci. Adv. 7, eabf5567. doi:10.1126/sciadv.abf5567
Bezzerides, V. J., Ramsey, I. S., Kotecha, S., Greka, A., and Clapham, D. E. (2004). Rapid Vesicular Translocation and Insertion of Trp Channels. Nat. Cel Biol 6, 709–720. doi:10.1038/ncb1150
Bhargava, A., Wang, J., and Pearce, D. (2004). Regulation of Epithelial Ion Transport by Aldosterone through Changes in Gene Expression. Mol. Cel Endocrinol 217, 189–196. doi:10.1016/j.mce.2003.10.020
Bikker, A., van Woerkom, J. M., Kruize, A. A., Wenting-van, W. M., de Jager, W., Bijlsma, J. W., et al. (2010). Increased Expression of Interleukin-7 in Labial Salivary Glands of Patients with Primary Sjogren's Syndrome Correlates with Increased Inflammation. Arthritis Rheum. 62, 969–977. doi:10.1002/art.27318
Billert, M., Skrzypski, M., Sassek, M., Szczepankiewicz, D., Wojciechowicz, T., Mergler, S., et al. (2017). Trpv4 regulates insulin mrna expression and ins-1e cell death via erk1/2 and no-dependent mechanisms. Cell Signal 35, 242–249. doi:10.1016/j.cellsig.2017.03.018
Blum, T., Moreno-Pérez, A., Pyrski, M., Bufe, B., Arifovic, A., Weissgerber, P., et al. (2019). Trpc5 Deficiency Causes Hypoprolactinemia and Altered Function of Oscillatory Dopamine Neurons in the Arcuate Nucleus. P Natl. Acad. Sci. Usa 116, 15236–15243. doi:10.1073/pnas.1905705116
Bolanz, K. A., Hediger, M. A., and Landowski, C. P. (2008). The Role of Trpv6 in Breast Carcinogenesis. Mol. Cancer Ther. 7, 271–279. doi:10.1158/1535-7163.MCT-07-0478
Bolanz, K. A., Kovacs, G. G., Landowski, C. P., and Hediger, M. A. (2009). Tamoxifen Inhibits Trpv6 Activity via Estrogen Receptor-independent Pathways in Trpv6-Expressing Mcf-7 Breast Cancer Cells. Mol. Cancer Res. : MCR 7, 2000–2010. doi:10.1158/1541-7786.MCR-09-0188
Burgoyne, R. D., Morgan, A., Robinson, I., Pender, N., and Cheek, T. R. (1993). Exocytosis in Adrenal Chromaffin Cells. J. Anat. 183 (Pt 2), 309–314.
Cannon, B., and Nedergaard, J. (2004). Brown Adipose Tissue: Function and Physiological Significance. Physiol. Rev. 84, 277–359. doi:10.1152/physrev.00015.2003
Casas, S., Novials, A., Reimann, F., Gomis, R., and Gribble, F. M. (2008). Calcium Elevation in Mouse Pancreatic Beta Cells Evoked by Extracellular Human Islet Amyloid Polypeptide Involves Activation of the Mechanosensitive Ion Channel Trpv4. Diabetologia 51, 2252–2262. doi:10.1007/s00125-008-1111-z
Caterina, M. J., Rosen, T. A., Tominaga, M., Brake, A. J., and Julius, D. (1999). A Capsaicin-Receptor Homologue with a High Threshold for Noxious Heat. Nature 398, 436–441. doi:10.1038/18906
Cayouette, S., Lussier, M. P., Mathieu, E., Bousquet, S. M., and Boulay, G. (2004). Exocytotic Insertion of Trpc6 Channel into the Plasma Membrane upon Gq Protein-Coupled Receptor Activation. J. Biol. Chem. 279, 7241–7246. doi:10.1074/jbc.m312042200
Celli, A., Mackenzie, D. S., Crumrine, D. S., Tu, C. L., Hupe, M., Bikle, D. D., et al. (2011). Endoplasmic Reticulum Ca2+ Depletion Activates Xbp1 and Controls Terminal Differentiation in Keratinocytes and Epidermis. Br. J. Dermatol. 164, 16–25. doi:10.1111/j.1365-2133.2010.10046.x
Chan, G. C., Hess, P., Meenakshi, T., Carlstedt-Duke, J., Gustafsson, J. A., and Payvar, F. (1991). Delayed Secondary Glucocorticoid Response Elements. Unusual Nucleotide Motifs Specify Glucocorticoid Receptor Binding to Transcribed Regions of Alpha 2u-Globulin Dna. J. Biol. Chem. 266, 22634–22644. doi:10.1016/s0021-9258(18)54618-4
Cheek, T. R., and Barry, V. A. (1993). Stimulus-secretion Coupling in Excitable Cells: a central Role for Calcium. J. Exp. Biol. 184, 183–196. doi:10.1242/jeb.184.1.183
Chen, X., Sooch, G., Demaree, I. S., White, F. A., and Obukhov, A. G. (2020). Transient Receptor Potential Canonical (Trpc) Channels: Then and Now. Cells-Basel 9, 1983. doi:10.3390/cells9091983
Cheng, K. T., Liu, X., Ong, H. L., and Ambudkar, I. S. (2008). Functional Requirement for Orai1 in Store-Operated Trpc1-Stim1 Channels. J. Biol. Chem. 283, 12935–12940. doi:10.1074/jbc.C800008200
Cheng, K. T., Liu, X., Ong, H. L., Swaim, W., and Ambudkar, I. S. (2011). Local Ca(2)+ Entry via Orai1 Regulates Plasma Membrane Recruitment of Trpc1 and Controls Cytosolic Ca(2)+ Signals Required for Specific Cell Functions. Plos Biol. 9, e1001025. doi:10.1371/journal.pbio.1001025
Choi, S., Maleth, J., Jha, A., Lee, K. P., Kim, M. S., So, I., et al. (2014). The Trpcs-Stim1-Orai Interaction. Handb Exp. Pharmacol. 223, 1035–1054. doi:10.1007/978-3-319-05161-1_13
Colletti, G. A., and Kiselyov, K. (2011). Trpml1. Adv. Exp. Med. Biol. 704, 209–219. doi:10.1007/978-94-007-0265-3_11
Colsoul, B., Schraenen, A., Lemaire, K., Quintens, R., Van Lommel, L., Segal, A., et al. (2010). Loss of High-Frequency Glucose-Induced Ca2+ Oscillations in Pancreatic Islets Correlates with Impaired Glucose Tolerance in Trpm5-/- Mice. Proc. Natl. Acad. Sci. U S A. 107, 5208–5213. doi:10.1073/pnas.0913107107
Colsoul, B., Vennekens, R., and Nilius, B. (2011). Transient Receptor Potential Cation Channels in Pancreatic Beta Cells. Rev. Physiol. Biochem. Pharmacol. 161, 87–110. doi:10.1007/112_2011_2
Cosens, D. J., and Manning, A. (1969). Abnormal Electroretinogram from a drosophila Mutant. Nature 224, 285–287. doi:10.1038/224285a0
Csanady, L., and Torocsik, B. (2009). Four Ca2+ Ions Activate Trpm2 Channels by Binding in Deep Crevices Near the Pore but Intracellularly of the Gate. J. Gen. Physiol. 133, 189–203. doi:10.1085/jgp.200810109
De-Madaria, E., and Capurso, G. (2021). Covid-19 and Acute Pancreatitis: Examining the Causality. Nat. Rev. Gastroenterol. Hepatol. 18, 3–4. doi:10.1038/s41575-020-00389-y
Derouiche, S., Takayama, Y., Murakami, M., and Tominaga, M. (2018). Trpv4 Heats up Ano1-dependent Exocrine Gland Fluid Secretion. Faseb J. 32, 1841–1854. doi:10.1096/fj.201700954R
Dhennin-Duthille, I., Gautier, M., Faouzi, M., Guilbert, A., Brevet, M., Vaudry, D., et al. (2011). High Expression of Transient Receptor Potential Channels in Human Breast Cancer Epithelial Cells and Tissues: Correlation with Pathological Parameters. Cell Physiol. Biochem. : Int. J. Exp. Cell. Physiol. Biochem. Pharmacol. 28, 813–822. doi:10.1159/000335795
Di Paola, S., Scotto-Rosato, A., and Medina, D. L. (2018). Trpml1: the Ca((2+))retaker of the Lysosome. Cell Calcium 69, 112–121. doi:10.1016/j.ceca.2017.06.006
Diaz-Garcia, C. M., Morales-Lazaro, S. L., Sanchez-Soto, C., Velasco, M., Rosenbaum, T., and Hiriart, M. (2014). Role for the Trpv1 Channel in Insulin Secretion from Pancreatic Beta Cells. J. Membr. Biol. 247, 479–491. doi:10.1007/s00232-014-9658-8
Diszhazi, G., Magyar, Z. E., Lisztes, E., Toth-Molnar, E., Nanasi, P. P., Vennekens, R., et al. (2021). Trpm4 Links Calcium Signaling to Membrane Potential in Pancreatic Acinar Cells. J. Biol. Chem. 297, 101015. doi:10.1016/j.jbc.2021.101015
Dong, X. P., Wang, X., Shen, D., Chen, S., Liu, M., Wang, Y., et al. (2009). Activating Mutations of the Trpml1 Channel Revealed by Proline-Scanning Mutagenesis. J. Biol. Chem. 284, 32040–32052. doi:10.1074/jbc.M109.037184
Du, J., Wong, W., Sun, L., Huang, Y., and Yao, X. (2012). Protein Kinase G Inhibits Flow-Induced Ca2+ Entry into Collecting Duct Cells. J. Am. Soc. Nephrol. : JASN 23, 1172–1180. doi:10.1681/ASN.2011100972
Du, J., Xie, J., and Yue, L. (2009). Intracellular Calcium Activates Trpm2 and its Alternative Spliced Isoforms. Proc. Natl. Acad. Sci. U S A. 106, 7239–7244. doi:10.1073/pnas.0811725106
El-Maouche, D., Arlt, W., and Merke, D. P. (2017). Congenital Adrenal Hyperplasia. Lancet 390, 2194–2210. doi:10.1016/S0140-6736(17)31431-9
Fagelskiold, A. J., Kannisto, K., Bostrom, A., Hadrovic, B., Farre, C., Eweida, M., et al. (2012). Insulin-secreting ins-1e cells express functional trpv1 channels. Islets 4, 56–63. doi:10.4161/isl.18915
Faouzi, M., and Penner, R. (2014). Trpm2. Handb Exp. Pharmacol. 222, 403–426. doi:10.1007/978-3-642-54215-2_16
Félétou, M., and Vanhoutte, P. M. (2009). Edhf: an Update. Clin. Sci. (London) 117, 139–155. doi:10.1042/CS20090096
Fels, B., Nielsen, N., and Schwab, A. (2016). Role of Trpc1 Channels in Pressure-Mediated Activation of Murine Pancreatic Stellate Cells. Eur. Biophys. J. 45, 657–670. doi:10.1007/s00249-016-1176-4
Ferreira, L. G. B., Prevatto, J. P., Freitas, H. R., Reis, R. A. M., Silva, P. M. R., Martins, M. A., et al. (2019). Capsaicin Inhibits Lipopolysaccharide-Induced Adrenal Steroidogenesis by Raising Intracellular Calcium Levels. Endocrine 64, 169–175. doi:10.1007/s12020-019-01849-5
Fisher, J., Monette, D. L., Patel, K. R., Kelley, B. P., and Kennedy, M. (2021). Covid-19 Associated Parotitis. Am. J. Emerg. Med. 39, 251–254. doi:10.1016/j.ajem.2020.06.059
Flores, E. N., and Garcia-Anoveros, J. (2011). Trpml2 and the Evolution of Mucolipins. Adv. Exp. Med. Biol. 704, 221–228. doi:10.1007/978-94-007-0265-3_12
Fujiseki, M., Yamamoto, M., Ubaidus, S., Shinomiya, T., Abe, S., Tazaki, M., et al. (2017). Localization and Expression Patterns of Trp Channels in Submandibular Gland Development. Arch. Oral Biol. 74, 46–50. doi:10.1016/j.archoralbio.2016.09.011
Fusi, C., Materazzi, S., Minocci, D., Maio, V., Oranges, T., Massi, D., et al. (2014). Transient Receptor Potential Vanilloid 4 (Trpv4) Is Downregulated in Keratinocytes in Human Non-melanoma Skin Cancer. J. Invest. Dermatol. 134, 2408–2417. doi:10.1038/jid.2014.145
Gao, F., and Wang, D. H. (2010). Hypotension Induced by Activation of the Transient Receptor Potential Vanilloid 4 Channels: Role of Ca2+-Activated K+ Channels and Sensory Nerves. J. Hypertens. 28, 102–110. doi:10.1097/HJH.0b013e328332b865
Gao, X., Wu, L., and O'Neil, R. G. (2003). Temperature-modulated Diversity of Trpv4 Channel Gating: Activation by Physical Stresses and Phorbol Ester Derivatives through Protein Kinase C-dependent and -independent Pathways. J. Biol. Chem. 278, 27129–27137. doi:10.1074/jbc.m302517200
Gasser, A., Glassmeier, G., Fliegert, R., Langhorst, M. F., Meinke, S., Hein, D., et al. (2006). Activation of T Cell Calcium Influx by the Second Messenger Adp-Ribose. J. Biol. Chem. 281, 2489–2496. doi:10.1074/jbc.M506525200
Goel, M., Sinkins, W. G., Zuo, C., Hopfer, U., and Schilling, W. P. (2007). Vasopressin-induced Membrane Trafficking of Trpc3 and Aqp2 Channels in Cells of the Rat Renal Collecting Duct. Am. J. Physiol. Ren. Physiol. 293, F1476–F1488. doi:10.1152/ajprenal.00186.2007
González-Perrett, S., Kim, K., Ibarra, C., Damiano, A. E., Zotta, E., Batelli, M., et al. (2001). Polycystin-2, the Protein Mutated in Autosomal Dominant Polycystic Kidney Disease (Adpkd), Is a Ca2+-Permeable Nonselective Cation Channel. P Natl. Acad. Sci. Usa 98, 1182–1187. doi:10.1073/pnas.98.3.1182
Grant, C. V., Carver, C. M., Hastings, S. D., Ramachandran, K., Muniswamy, M., Risinger, A. L., et al. (2019). Triple-negative Breast Cancer Cell Line Sensitivity to Englerin a Identifies a New, Targetable Subtype. Breast Cancer Res. Tr 177, 345–355. doi:10.1007/s10549-019-05324-7
Guilbert, A., Dhennin-Duthille, I., Hiani, Y. E., Haren, N., Khorsi, H., Sevestre, H., et al. (2008). Expression of Trpc6 Channels in Human Epithelial Breast Cancer Cells. Bmc Cancer 8, 125. doi:10.1186/1471-2407-8-125
Harada, K., Matsuoka, H., and Inoue, M. (2019). Stim1-dependent Membrane Insertion of Heteromeric Trpc1/4 Channels in Response to Muscarinic Receptor Stimulation. J. Cel Sci 132, jcs227389. doi:10.1242/jcs.227389
Hardie, R. C. (2011). A Brief History of Trp: Commentary and Personal Perspective. Pflugers Arch. 461, 493–498. doi:10.1007/s00424-011-0922-9
Harding, H. P., Novoa, I., Zhang, Y., Zeng, H., Wek, R., Schapira, M., et al. (2000). Regulated Translation Initiation Controls Stress-Induced Gene Expression in Mammalian Cells. Mol. Cel 6, 1099–1108. doi:10.1016/s1097-2765(00)00108-8
Hart, P. A., and Conwell, D. L. (2020). Chronic Pancreatitis: Managing a Difficult Disease. Am. J. Gastroenterol. 115, 49–55. doi:10.14309/ajg.0000000000000421
Hayes, H. L., Moss, L. G., Schisler, J. C., Haldeman, J. M., Zhang, Z., Rosenberg, P. B., et al. (2013). Pdx-1 Activates Islet Alpha- and Beta-Cell Proliferation via a Mechanism Regulated by Transient Receptor Potential Cation Channels 3 and 6 and Extracellular Signal-Regulated Kinases 1 and 2. Mol. Cel Biol 33, 4017–4029. doi:10.1128/MCB.00469-13
Himms-Hagen, J., Cui, J., Danforth, E. J., Taatjes, D. J., Lang, S. S., Waters, B. L., et al. (1994). Effect of Cl-316,243, a Thermogenic Beta 3-agonist, on Energy Balance and Brown and white Adipose Tissues in Rats. Am. J. Physiol. 266, R1371–R1382. doi:10.1152/ajpregu.1994.266.4.r1371
Hirata, N., Yamada, S., Yanagida, S., Ono, A., Yasuhiko, Y., Nishida, M., et al. (2022). Lysophosphatidic Acid Promotes the Expansion of Cancer Stem Cells via Trpc3 Channels in Triple-Negative Breast Cancer. Int. J. Mol. Sci. 23, 1967. doi:10.3390/ijms23041967
Hisanaga, E., Nagasawa, M., Ueki, K., Kulkarni, R. N., Mori, M., and Kojima, I. (2009). Regulation of Calcium-Permeable Trpv2 Channel by Insulin in Pancreatic Beta-Cells. Diabetes 58, 174–184. doi:10.2337/db08-0862
Hoenderop, J. G., Nilius, B., and Bindels, R. J. (2003). Epithelial Calcium Channels: from Identification to Function and Regulation. Pflugers Arch. 446, 304–308. doi:10.1007/s00424-003-1045-8
Hong, J. H., Li, Q., Kim, M. S., Shin, D. M., Feske, S., Birnbaumer, L., et al. (2011). Polarized but Differential Localization and Recruitment of Stim1, Orai1 and Trpc Channels in Secretory Cells. Traffic 12, 232–245. doi:10.1111/j.1600-0854.2010.01138.x
Houghton, J. W., Carpenter, G., Hans, J., Pesaro, M., Lynham, S., and Proctor, G. (2020). Agonists of Orally Expressed Trp Channels Stimulate Salivary Secretion and Modify the Salivary Proteome. Mol. Cel Proteomics 19, 1664–1676. doi:10.1074/mcp.RA120.002174
Hu, G., Oboukhova, E. A., Kumar, S., Sturek, M., and Obukhov, A. G. (2009). Canonical Transient Receptor Potential Channels Expression Is Elevated in a Porcine Model of Metabolic Syndrome. Mol. Endocrinol. 23, 689–699. doi:10.1210/me.2008-0350
Hughes, J., Ward, C. J., Peral, B., Aspinwall, R., Clark, K., San, M. J., et al. (1995). The Polycystic Kidney Disease 1 (Pkd1) Gene Encodes a Novel Protein with Multiple Cell Recognition Domains. Nat. Genet. 10, 151–160. doi:10.1038/ng0695-151
Inoue, M., Harada, K., and Matsuoka, H. (2020). Mechanisms for Pituitary Adenylate Cyclase-Activating Polypeptide-Induced Increase in Excitability in guinea-pig and Mouse Adrenal Medullary Cells. Eur. J. Pharmacol. 872, 172956. doi:10.1016/j.ejphar.2020.172956
Islam, M. A., Mizusawa, M., Sharmin, M. M., Hayashi, S., and Yonekura, S. (2020). Trpv4 Increases the Expression of Tight junction Protein-Encoding Genes via Xbp1 in Mammary Epithelial Cells. Animals 10, 1174. doi:10.3390/ani10071174
Islam, M. S. (2020). Molecular Regulations and Functions of the Transient Receptor Potential Channels of the Islets of Langerhans and Insulinoma Cells. Cells-Basel 9, 685. doi:10.3390/cells9030685
Islam, M. S. (2011). Trp Channels of Islets. Adv. Exp. Med. Biol. 704, 811–830. doi:10.1007/978-94-007-0265-3_42
Iwasaki, Y., Tanabe, M., Kobata, K., and Watanabe, T. (2008). Trpa1 Agonists-Aallyl Isothiocyanate and Cinnamaldehyde-Iinduce Adrenaline Secretion. Biosci. Biotechnol. Biochem. 72, 2608–2614. doi:10.1271/bbb.80289
Jang, S. I., Ong, H. L., Liu, X., Alevizos, I., and Ambudkar, I. S. (2016). Up-regulation of Store-Operated Ca2+ Entry and Nuclear Factor of Activated T Cells Promote the Acinar Phenotype of the Primary Human Salivary Gland Cells. J. Biol. Chem. 291, 8709–8720. doi:10.1074/jbc.M115.701607
Julius, D. (2013). Trp Channels and Pain. Annu. Rev. Cel Dev Biol 29, 355–384. doi:10.1146/annurev-cellbio-101011-155833
Kanewska, A., Ito, M., Karasawa, Y., Inada, M., Garreis, F., Paulsen, F., et al. (2020). Developmental Change in the Gene Expression of Transient Receptor Potential Melastatin Channel 3 (Trpm3) in Murine Lacrimal Gland. Ann. Anat. 231, 151551. doi:10.1016/j.aanat.2020.151551
Karaki, H., Ozaki, H., Hori, M., Mitsui-Saito, M., Amano, K., Harada, K., et al. (1997). Calcium Movements, Distribution, and Functions in Smooth Muscle. Pharmacol. Rev. 49, 157–230.
Kawada, T., Watanabe, T., Takaishi, T., Tanaka, T., and Iwai, K. (1986). “From Society for Experimental Biology and Medicine. Proc. Soc. Exp. Biol. Med. 182 (2), 250–256. doi:10.3181/00379727-183-42414
Kida, N., Sokabe, T., Kashio, M., Haruna, K., Mizuno, Y., Suga, Y., et al. (2012). Importance of Transient Receptor Potential Vanilloid 4 (Trpv4) in Epidermal Barrier Function in Human Skin Keratinocytes. Pflugers Archiv : Eur. J. Physiol. 463, 715–725. doi:10.1007/s00424-012-1081-3
Kim, J., Park, S., Kim, T., Park, H., Park, J., Kim, B. K., et al. (2013). Testicular Hyperthermia Induces Unfolded Protein Response Signaling Activation in Spermatocyte. Biochem. Bioph Res. Co 434, 861–866. doi:10.1016/j.bbrc.2013.04.032
Kim, M. S., Lee, K. P., Yang, D., Shin, D. M., Abramowitz, J., Kiyonaka, S., et al. (2011). Genetic and Pharmacologic Inhibition of the Ca2+ Influx Channel Trpc3 Protects Secretory Epithelia from Ca2+-dependent Toxicity. Gastroenterology 140, 2107–2115. doi:10.1053/j.gastro.2011.02.052
Kleeff, J., Whitcomb, D. C., Shimosegawa, T., Esposito, I., Lerch, M. M., and Gress, T. (2017). Chronic Pancreatitis. Nat. Rev. Dis. Primers 3, 17060. doi:10.1038/nrdp.2017.60
Knowles, H., Li, Y., and Perraud, A. L. (2013). The Trpm2 Ion Channel, an Oxidative Stress and Metabolic Sensor Regulating Innate Immunity and Inflammation. Immunol. Res. 55, 241–248. doi:10.1007/s12026-012-8373-8
Kobayashi, A., Osaka, T., Namba, Y., Inoue, S., Lee, T. H., and Kimura, S. (1998). Capsaicin Activates Heat Loss and Heat Production Simultaneously and Independently in Rats. Am. J. Physiol. 275, R92–R98. doi:10.1152/ajpregu.1998.275.1.R92
Koivisto, A. P., Belvisi, M. G., Gaudet, R., and Szallasi, A. (2022). Advances in Trp Channel Drug Discovery: from Target Validation to Clinical Studies. Nat. Rev. Drug Discov. 21, 41–59. doi:10.1038/s41573-021-00268-4
Konings, A. W., Coppes, R. P., and Vissink, A. (2005). On the Mechanism of Salivary Gland Radiosensitivity. Int. J. Radiat. Oncol. Biol. Phys. 62, 1187–1194. doi:10.1016/j.ijrobp.2004.12.051
Koulen, P., Cai, Y., Geng, L., Maeda, Y., Nishimura, S., Witzgall, R., et al. (2002). Polycystin-2 Is an Intracellular Calcium Release Channel. Nat. Cel Biol 4, 191–197. doi:10.1038/ncb754
Kraft, R., and Harteneck, C. (2005). The Mammalian Melastatin-Related Transient Receptor Potential Cation Channels: an Overview. Pflugers Arch. 451, 204–211. doi:10.1007/s00424-005-1428-0
Krapivinsky, G., Mochida, S., Krapivinsky, L., Cibulsky, S. M., and Clapham, D. E. (2006). The Trpm7 Ion Channel Functions in Cholinergic Synaptic Vesicles and Affects Transmitter Release. Neuron 52, 485–496. doi:10.1016/j.neuron.2006.09.033
Krug, A. W., and Ehrhart-Bornstein, M. (19792008)., 51. Dallas, Tex, 1252–1258. doi:10.1161/HYPERTENSIONAHA.107.109439Aldosterone and Metabolic Syndrome: Is Increased Aldosterone in Metabolic Syndrome Patients an Additional Risk Factor?Hypertension
Larsson, M. H., Hakansson, P., Jansen, F. P., Magnell, K., and Brodin, P. (2015). Ablation of Trpm5 in Mice Results in Reduced Body Weight Gain and Improved Glucose Tolerance and Protects from Excessive Consumption of Sweet Palatable Food when Fed High Caloric Diets. Plos One 10, e138373. doi:10.1371/journal.pone.0138373
Lee, J., Moon, S., Cha, Y., and Chung, Y. D. (2010). Drosophila Trpn(=nompc) Channel Localizes to the Distal End of Mechanosensory Cilia. Plos One 5, e11012. doi:10.1371/journal.pone.0011012
Lee, K. P., Choi, S., Hong, J. H., Ahuja, M., Graham, S., Ma, R., et al. (2014). Molecular Determinants Mediating Gating of Transient Receptor Potential Canonical (Trpc) Channels by Stromal Interaction Molecule 1 (Stim1). J. Biol. Chem. 289, 6372–6382. doi:10.1074/jbc.M113.546556
Lehen'kyi, V., Beck, B., Polakowska, R., Charveron, M., Bordat, P., Skryma, R., et al. (2007). Trpv6 Is a Ca2+ Entry Channel Essential for Ca2+-Induced Differentiation of Human Keratinocytes. J. Biol. Chem. 282, 22582–22591. doi:10.1074/jbc.M611398200
Lehen'kyi, V., Raphaël, M., and Prevarskaya, N. (2012). The Role of the Trpv6 Channel in Cancer. J. Physiol. 590, 1369–1376. doi:10.1113/jphysiol.2011.225862
Lehen'kyi, V., Vandenberghe, M., Belaubre, F., Julié, S., Castex-Rizzi, N., Skryma, R., et al. (2011). Acceleration of Keratinocyte Differentiation by Transient Receptor Potential Vanilloid (Trpv6) Channel Activation. J. Eur. Acad. Dermatol. Venereol. : JEADV 25 (Suppl. 1), 12–18. doi:10.1111/j.1468-3083.2010.03894.x
Leloup, C., Tourrel-Cuzin, C., Magnan, C., Karaca, M., Castel, J., Carneiro, L., et al. (2009). Mitochondrial Reactive Oxygen Species Are Obligatory Signals for Glucose-Induced Insulin Secretion. Diabetes 58, 673–681. doi:10.2337/db07-1056
Li, F., Munsey, T. S., and Sivaprasadarao, A. (2017). Trpm2-mediated Rise in Mitochondrial Zn(2+) Promotes Palmitate-Induced Mitochondrial Fission and Pancreatic Beta-Cell Death in Rodents. Cell Death Differ 24, 1999–2012. doi:10.1038/cdd.2017.118
Li, H. (2017). Trp Channel Classification. Adv. Exp. Med. Biol. 976, 1–8. doi:10.1007/978-94-024-1088-4_1
Li, M., Zhang, W. K., Benvin, N. M., Zhou, X., Su, D., Li, H., et al. (2017). Structural Basis of Dual Ca(2+)/ph Regulation of the Endolysosomal Trpml1 Channel. Nat. Struct. Mol. Biol. 24, 205–213. doi:10.1038/nsmb.3362
Li, Y., Wright, J. M., Qian, F., Germino, G. G., and Guggino, W. B. (2005). Polycystin 2 Interacts with Type I Inositol 1,4,5-trisphosphate Receptor to Modulate Intracellular Ca2+ Signaling. J. Biol. Chem. 280, 41298–41306. doi:10.1074/jbc.m510082200
Liedtke, W., and Friedman, J. M. (2003). Abnormal Osmotic Regulation in Trpv4-/- Mice. P Natl. Acad. Sci. Usa 100, 13698–13703. doi:10.1073/pnas.1735416100
Liedtke, W. B., and Heller, S. (2007). Trp Ion Channel Function in Sensory Transduction and Cellular Signaling Cascades. Boca Raton, London, New York: CRC Press Taylor and Francis Group.
Lin, Y., and Sun, Z. (2012). Antiaging Gene Klotho Enhances Glucose-Induced Insulin Secretion by Up-Regulating Plasma Membrane Levels of Trpv2 in Min6 Beta-Cells. Endocrinology 153, 3029–3039. doi:10.1210/en.2012-1091
Lindemann, O., Strodthoff, C., Horstmann, M., Nielsen, N., Jung, F., Schimmelpfennig, S., et al. (2015). Trpc1 Regulates Fmlp-Stimulated Migration and Chemotaxis of Neutrophil Granulocytes. Biochim. Biophys. Acta 1853, 2122–2130. doi:10.1016/j.bbamcr.2014.12.037
Lisco, G., De Tullio, A., Stragapede, A., Solimando, A. G., Albanese, F., Capobianco, M., et al. (2021). Covid-19 and the Endocrine System: a Comprehensive Review on the Theme. J. Clin. Med. 10, 2920. doi:10.3390/jcm10132920
Liu, X., Bandyopadhyay, B. C., Nakamoto, T., Singh, B., Liedtke, W., Melvin, J. E., et al. (2006). A Role for Aqp5 in Activation of Trpv4 by Hypotonicity: Concerted Involvement of Aqp5 and Trpv4 in Regulation of Cell Volume Recovery. J. Biol. Chem. 281, 15485–15495. doi:10.1074/jbc.M600549200
Liu, X., Cheng, K. T., Bandyopadhyay, B. C., Pani, B., Dietrich, A., Paria, B. C., et al. (2007). Attenuation of Store-Operated Ca2+ Current Impairs Salivary Gland Fluid Secretion in Trpc1(-/-) Mice. Proc. Natl. Acad. Sci. U S A. 104, 17542–17547. doi:10.1073/pnas.0701254104
Liu, X., Cotrim, A., Teos, L., Zheng, C., Swaim, W., Mitchell, J., et al. (2013). Loss of Trpm2 Function Protects against Irradiation-Induced Salivary Gland Dysfunction. Nat. Commun. 4, 1515. doi:10.1038/ncomms2526
Liu, X., Gong, B., de Souza, L. B., Ong, H. L., Subedi, K. P., Cheng, K. T., et al. (2017). Radiation Inhibits Salivary Gland Function by Promoting Stim1 Cleavage by Caspase-3 and Loss of Soce through a Trpm2-dependent Pathway. Sci. Signal. 10, eaal4064. doi:10.1126/scisignal.aal4064
Liu, X., Ong, H. L., and Ambudkar, I. (2018). Trp Channel Involvement in Salivary Glands-Some Good, Some Bad. Cells-Basel 7, 74. doi:10.3390/cells7070074
Liu, X., Wang, W., Singh, B. B., Lockwich, T., Jadlowiec, J., O'Connell, B., et al. (2000). Trp1, a Candidate Protein for the Store-Operated Ca(2+) Influx Mechanism in Salivary Gland Cells. J. Biol. Chem. 275, 3403–3411. doi:10.1074/jbc.275.5.3403
Livett, B. G., and Marley, P. D. (1993). Noncholinergic Control of Adrenal Catecholamine Secretion. J. Anat. 183 (Pt 2), 277–289.
Lopez, J. J., Jardin, I., Sanchez-Collado, J., Salido, G. M., Smani, T., and Rosado, J. A. (2020). Trpc Channels in the Soce Scenario. Cells-Basel 9, 126. doi:10.3390/cells9010126
Ma, W., Chen, X., Cerne, R., Syed, S. K., Ficorilli, J. V., Cabrera, O., et al. (2019). Catechol Estrogens Stimulate Insulin Secretion in Pancreatic Beta-Cells via Activation of the Transient Receptor Potential A1 (Trpa1) Channel. J. Biol. Chem. 294, 2935–2946. doi:10.1074/jbc.RA118.005504
Ma, X., Cai, Y., He, D., Zou, C., Zhang, P., Lo, C. Y., et al. (2012). Transient Receptor Potential Channel Trpc5 Is Essential for P-Glycoprotein Induction in Drug-Resistant Cancer Cells. P Natl. Acad. Sci. Usa 109, 16282–16287. doi:10.1073/pnas.1202989109
Ma, X., Cai, Y., He, D., Zou, C., Zhang, P., Lo, C. Y., et al. (2012). Transient Receptor Potential Channel Trpc5 Is Essential for P-Glycoprotein Induction in Drug-Resistant Cancer Cells. Proc. Natl. Acad. Sci. 109, 16282–16287. doi:10.1073/pnas.1202989109
Mandavilli, S., Singh, B. B., and Sahmoun, A. E. (2012). Serum Calcium Levels, Trpm7, Trpc1, Microcalcifications, and Breast Cancer Using Breast Imaging Reporting and Data System Scores. Breast Cancer (Dove Med. Press) 2013, 1–7. doi:10.2147/BCTT.S37436
Marabita, F., and Islam, M. S. (2017). Expression of Transient Receptor Potential Channels in the Purified Human Pancreatic Beta-Cells. Pancreas 46, 97–101. doi:10.1097/MPA.0000000000000685
Marazuela, M., Giustina, A., and Puig-Domingo, M. (2020). Endocrine and Metabolic Aspects of the Covid-19 Pandemic. Rev. Endocr. Metab. Disord. 21, 495–507. doi:10.1007/s11154-020-09569-2
Marom, M., Birnbaumer, L., and Atlas, D. (2011). Membrane Depolarization Combined with Gq-Activated G-Protein-Coupled Receptors Induce Transient Receptor Potential Channel 1 (Trpc1)- Dependent Potentiation of Catecholamine Release. Neuroscience 189, 132–145. doi:10.1016/j.neuroscience.2011.05.007
Martinez-Garcia, M. C., Martinez, T., Paneda, C., Gallego, P., Jimenez, A. I., and Merayo, J. (2013). Differential Expression and Localization of Transient Receptor Potential Vanilloid 1 in Rabbit and Human Eyes. Histol. Histopathol 28, 1507–1516. doi:10.14670/HH-28.1507
Mathar, I., Vennekens, R., Meissner, M., Kees, F., Van der Mieren, G., Camacho Londoño, J. E., et al. (2010). Increased Catecholamine Secretion Contributes to Hypertension in Trpm4-Deficient Mice. J. Clin. Invest. 120, 3267–3279. doi:10.1172/JCI41348
Mathar, I., Vennekens, R., Meissner, M., Kees, F., Van der Mieren, G., Camacho Londoño, J. E., et al. (2010). Increased Catecholamine Secretion Contributes to Hypertension in Trpm4-Deficient Mice. J. Clin. Invest. 120, 3267–3279. doi:10.1172/JCI41348
Matthews, E. K., and Saffran, M. (1973). Ionic Dependence of Adrenal Steroidogenesis and Acth-Induced Changes in the Membrane Potential of Adrenocortical Cells. J. Physiol. 234, 43–64. doi:10.1113/jphysiol.1973.sp010333
Mavragani, C. P., and Moutsopoulos, H. M. (2014). Sjogren's Syndrome. Annu. Rev. Pathol. 9, 273–285. doi:10.1146/annurev-pathol-012513-104728
McAndrew, D., Grice, D. M., Peters, A. A., Davis, F. M., Stewart, T., Rice, M., et al. (2011). Orai1-mediated Calcium Influx in Lactation and in Breast Cancer. Mol. Cancer Ther. 10, 448–460. doi:10.1158/1535-7163.MCT-10-0923
McHugh, D., Flemming, R., Xu, S. Z., Perraud, A. L., and Beech, D. J. (2003). Critical Intracellular Ca2+ Dependence of Transient Receptor Potential Melastatin 2 (Trpm2) Cation Channel Activation. J. Biol. Chem. 278, 11002–11006. doi:10.1074/jbc.M210810200
Mendez-Resendiz, K. A., Enciso-Pablo, O., Gonzalez-Ramirez, R., Juarez-Contreras, R., Rosenbaum, T., and Morales-Lazaro, S. L. (2020). Steroids and Trp Channels: a Close Relationship. Int. J. Mol. Sci. 21, 3819. doi:10.3390/ijms21113819
Metzler-Wilson, K., Sammons, D. L., Ossim, M. A., Metzger, N. R., Jurovcik, A. J., Krause, B. A., et al. (2014). Extracellular Calcium Chelation and Attenuation of Calcium Entry Decrease In Vivo Cholinergic-Induced Eccrine Sweating Sensitivity in Humans. Exp. Physiol. 99, 393–402. doi:10.1113/expphysiol.2013.076547
Mirnikjoo, B., Balasubramanian, K., and Schroit, A. J. (2009). Mobilization of Lysosomal Calcium Regulates the Externalization of Phosphatidylserine during Apoptosis. J. Biol. Chem. 284, 6918–6923. doi:10.1074/jbc.M805288200
Mochizuki, T., Wu, G., Hayashi, T., Xenophontos, S. L., Veldhuisen, B., Saris, J. J., et al. (1996). Pkd2, a Gene for Polycystic Kidney Disease that Encodes an Integral Membrane Protein. Science 272, 1339–1342. doi:10.1126/science.272.5266.1339
Morales, S., Diez, A., Puyet, A., Camello, P. J., Camello-Almaraz, C., Bautista, J. M., et al. (2007). Calcium Controls Smooth Muscle Trpc Gene Transcription via the Camk/calcineurin-dependent Pathways. Am. J. Physiol-cell Ph. 292, C553–C563. doi:10.1152/ajpcell.00096.2006
Moran, M. M., and Szallasi, A. (2018). Targeting Nociceptivetransient Receptor Potential Channels to Treat Chronic Pain: Current State of the Field. Br. J Pharmacol 175, 2185–2203. doi:10.1111/bph.14044
Moran, M. M. (2018). Trp Channels as Potential Drug Targets. Annu. Rev. Pharmacol. Toxicol. 58, 309–330. doi:10.1146/annurev-pharmtox-010617-052832
Nadler, M. J., Hermosura, M. C., Inabe, K., Perraud, A. L., Zhu, Q., Stokes, A. J., et al. (2001). Ltrpc7 Is a mg.Atp-Regulated Divalent Cation Channel Required for Cell Viability. Nature 411, 590–595. doi:10.1038/35079092
Nagase, I., Yoshida, T., Kumamoto, K., Umekawa, T., Sakane, N., Nikami, H., et al. (1996). Expression of Uncoupling Protein in Skeletal Muscle and white Fat of Obese Mice Treated with Thermogenic Beta 3-adrenergic Agonist. J. Clin. Invest. 97, 2898–2904. doi:10.1172/jci118748
Najder, K., Musset, B., Lindemann, O., Bulk, E., Schwab, A., and Fels, B. (2018). The Function of Trp Channels in Neutrophil Granulocytes. Pflugers Arch. 470, 1017–1033. doi:10.1007/s00424-018-2146-8
Narayanan, D., Bulley, S., Leo, M. D., Burris, S. K., Gabrick, K. S., Boop, F. A., et al. (2013). Smooth Muscle Cell Transient Receptor Potential Polycystin-2 (Trpp2) Channels Contribute to the Myogenic Response in Cerebral Arteries. J. Physiol. 591, 5031–5046. doi:10.1113/jphysiol.2013.258319
Nielsen, N., Kondratska, K., Ruck, T., Hild, B., Kovalenko, I., Schimmelpfennig, S., et al. (2017). Trpc6 Channels Modulate the Response of Pancreatic Stellate Cells to Hypoxia. Pflugers Arch. 469, 1567–1577. doi:10.1007/s00424-017-2057-0
Noble, F., Wank, S. A., Crawley, J. N., Bradwejn, J., Seroogy, K. B., Hamon, M., et al. (1999). International union of Pharmacology. Xxi. Structure, Distribution, and Functions of Cholecystokinin Receptors. Pharmacol. Rev. 51, 745–781.
Obukhov, A. G., and Nowycky, M. C. (2002). Trpc4 Can Be Activated by G-Protein-Coupled Receptors and Provides Sufficient Ca2+ to Trigger Exocytosis in Neuroendocrine Cells. J. Biol. Chem. 277, 16172–16178. doi:10.1074/jbc.M111664200
Ohara, K., Fukuda, T., Okada, H., Kitao, S., Ishida, Y., Kato, K., et al. (2015). Identification of Significant Amino Acids in Multiple Transmembrane Domains of Human Transient Receptor Potential Ankyrin 1 (Trpa1) for Activation by Eudesmol, an Oxygenized Sesquiterpene in Hop Essential Oil. J. Biol. Chem. 290, 3161–3171. doi:10.1074/jbc.M114.600932
Ohara, K., Katayama, M., and Nagai, K. (2018). Β-Eudesmol, an Oxygenized Sesquiterpene, Affects Efferent Adrenal Sympathetic Nerve Activity via Transient Receptor Potential Ankyrin 1 in Rats. Neurosci. Lett. 684, 18–24. doi:10.1016/j.neulet.2018.06.057
Oi-Kano, Y., Iwasaki, Y., Nakamura, T., Watanabe, T., Goto, T., Kawada, T., et al. (2017). Oleuropein Aglycone Enhances Ucp1 Expression in Brown Adipose Tissue in High-Fat-Diet-Induced Obese Rats by Activating β-adrenergic Signaling. J. Nutr. Biochem. 40, 209–218. doi:10.1016/j.jnutbio.2016.11.009
Olivan-Viguera, A., Garcia-Otin, A. L., Lozano-Gerona, J., Abarca-Lachen, E., Garcia-Malinis, A. J., Hamilton, K. L., et al. (2018). Pharmacological Activation of Trpv4 Produces Immediate Cell Damage and Induction of Apoptosis in Human Melanoma Cells and Hacat Keratinocytes. Plos One 13, e190307. doi:10.1371/journal.pone.0190307
Olivos, L., and Artalejo, A. R. (2008). Muscarinic Excitation-Secretion Coupling in Chromaffin Cells. Acta Physiol. 192, 213–220. doi:10.1111/j.1748-1716.2007.01816.x
Ong, H. L., de Souza, L. B., and Ambudkar, I. S. (2016). Role of Trpc Channels in Store-Operated Calcium Entry. Adv. Exp. Med. Biol. 898, 87–109. doi:10.1007/978-3-319-26974-0_5
Ong, H. L., de Souza, L. B., Cheng, K. T., and Ambudkar, I. S. (2014). Physiological Functions and Regulation of Trpc Channels. Handb Exp. Pharmacol. 223, 1005–1034. doi:10.1007/978-3-319-05161-1_12
Pani, B., Liu, X., Bollimuntha, S., Cheng, K. T., Niesman, I. R., Zheng, C., et al. (2013). Impairment of Trpc1-Stim1 Channel Assembly and Aqp5 Translocation Compromise Agonist-Stimulated Fluid Secretion in Mice Lacking Caveolin1. J. Cel Sci 126, 667–675. doi:10.1242/jcs.118943
Pani, B., Ong, H. L., Brazer, S. C., Liu, X., Rauser, K., Singh, B. B., et al. (2009). Activation of Trpc1 by Stim1 in Er-Pm Microdomains Involves Release of the Channel from its Scaffold Caveolin-1. Proc. Natl. Acad. Sci. U S A. 106, 20087–20092. doi:10.1073/pnas.0905002106
Park, S. H., Ryu, S. Y., Yu, W. J., Han, Y. E., Ji, Y. S., Oh, K., et al. (2013). Leptin Promotes K(atp) Channel Trafficking by Ampk Signaling in Pancreatic Beta-Cells. Proc. Natl. Acad. Sci. U S A. 110, 12673–12678. doi:10.1073/pnas.1216351110
Park, W., Chawla, A., and O'Reilly, E. M. (2021). Pancreatic Cancer: a Review. JAMA 326, 851–862. doi:10.1001/jama.2021.13027
Peng, J. B. (2011). Trpv5 and Trpv6 in Transcellular Ca(2+) Transport: Regulation, Gene Duplication, and Polymorphisms in African Populations. Adv. Exp. Med. Biol. 704, 239–275. doi:10.1007/978-94-007-0265-3_14
Perraud, A. L., Shen, B., Dunn, C. A., Rippe, K., Smith, M. K., Bessman, M. J., et al. (2003). Nudt9, a Member of the Nudix Hydrolase Family, Is an Evolutionarily Conserved Mitochondrial Adp-Ribose Pyrophosphatase. J. Biol. Chem. 278, 1794–1801. doi:10.1074/jbc.M205601200
Pi, J., Bai, Y., Zhang, Q., Wong, V., Floering, L. M., Daniel, K., et al. (2007). Reactive Oxygen Species as a Signal in Glucose-Stimulated Insulin Secretion. Diabetes 56, 1783–1791. doi:10.2337/db06-1601
Ramos-Casals, M., Brito-Zeron, P., Bombardieri, S., Bootsma, H., De Vita, S., Dorner, T., et al. (2020). Eular Recommendations for the Management of Sjogren's Syndrome with Topical and Systemic Therapies. Ann. Rheum. Dis. 79, 3–18. doi:10.1136/annrheumdis-2019-216114
Randhawa, P. K., and Jaggi, A. S. (2017). Trpv(1) Channels in Cardiovascular System: a Double Edged Sword? Int. J. Cardiol. 228, 103–113. doi:10.1016/j.ijcard.2016.11.205
Razavi, R., Chan, Y., Afifiyan, F. N., Liu, X. J., Wan, X., Yantha, J., et al. (2006). Trpv1+ Sensory Neurons Control Beta Cell Stress and Islet Inflammation in Autoimmune Diabetes. Cell 127, 1123–1135. doi:10.1016/j.cell.2006.10.038
Reiter, B., Kraft, R., Günzel, D., Zeissig, S., Schulzke, J. D., Fromm, M., et al. (2006). Trpv4‐mediated Regulation of Epithelial Permeability. FASEB J. 20, 1802–1812. doi:10.1096/fj.06-5772com
Roe, M. W., Worley, J. R., Qian, F., Tamarina, N., Mittal, A. A., Dralyuk, F., et al. (1998). Characterization of a Ca2+ Release-Activated Nonselective Cation Current Regulating Membrane Potential and [ca2+]i Oscillations in Transgenically Derived Beta-Cells. J. Biol. Chem. 273, 10402–10410. doi:10.1074/jbc.273.17.10402
Rosenfeld, D., Senko, A. W., Moon, J., Yick, I., Varnavides, G., Gregurec, D., et al. (2020). Transgene-free Remote Magnetothermal Regulation of Adrenal Hormones. Sci. Adv. 6, z3734. doi:10.1126/sciadv.aaz3734
Sabat, R., Jemec, G., Matusiak, L., Kimball, A. B., Prens, E., and Wolk, K. (2020). Hidradenitis Suppurativa. Nat. Rev. Dis. Primers 6, 18. doi:10.1038/s41572-020-0149-1
Sala, F., Nistri, A., and Criado, M. (2008). Nicotinic Acetylcholine Receptors of Adrenal Chromaffin Cells. Acta Physiol. 192, 203–212. doi:10.1111/j.1748-1716.2007.01804.x
Samanta, A., Hughes, T., and Moiseenkova-Bell, V. Y. (2018). Transient Receptor Potential (Trp) Channels. Subcell Biochem. 87, 141–165. doi:10.1007/978-981-10-7757-9_6
Sammels, E., Devogelaere, B., Mekahli, D., Bultynck, G., Missiaen, L., Parys, J. B., et al. (2010). Polycystin-2 Activation by Inositol 1,4,5-Trisphosphate-Induced Ca2+ Release Requires its Direct Association with the Inositol 1,4,5-trisphosphate Receptor in a Signaling Microdomain. J. Biol. Chem. 285, 18794–18805. doi:10.1074/jbc.M109.090662
Sarzani, R., Salvi, F., Dessì-Fulgheri, P., and Rappelli, A. (2008). Renin-angiotensin System, Natriuretic Peptides, Obesity, Metabolic Syndrome, and Hypertension: an Integrated View in Humans. J. Hypertens. 26, 831–843. doi:10.1097/HJH.0b013e3282f624a0
Satheesh, N. J., Uehara, Y., Fedotova, J., Pohanka, M., Busselberg, D., and Kruzliak, P. (2016). Trpv Currents and Their Role in the Nociception and Neuroplasticity. Neuropeptides 57, 1–8. doi:10.1016/j.npep.2016.01.003
Sato, K., and Sato, F. (1981). Role of Calcium in Cholinergic and Adrenergic Mechanisms of Eccrine Sweat Secretion. Am. J. Physiol. 241, C113–C120. doi:10.1152/ajpcell.1981.241.3.c113
Saunte, D., and Jemec, G. (2017). Hidradenitis Suppurativa: Advances in Diagnosis and Treatment. JAMA 318, 2019–2032. doi:10.1001/jama.2017.16691
Sawatani, T., Kaneko, Y. K., Doutsu, I., Ogawa, A., and Ishikawa, T. (2019). Trpv2 Channels Mediate Insulin Secretion Induced by Cell Swelling in Mouse Pancreatic Beta-Cells. Am. J. Physiol. Cel Physiol 316, C434–C443. doi:10.1152/ajpcell.00210.2017
Schaar, A., Sukumaran, P., Sun, Y., Dhasarathy, A., and Singh, B. B. (2016). Trpc1-stim1 Activation Modulates Transforming Growth Factor Beta-Induced Epithelial-To-Mesenchymal Transition. Oncotarget 7, 80554–80567. doi:10.18632/oncotarget.12895
Scharenberg, A. M. (2009). Trpm2 and Pancreatic Beta-Cell Responses to Oxidative Stress. Islets 1, 165–166. doi:10.4161/isl.1.2.9434
Schlereth, T., Dittmar, J. O., Seewald, B., and Birklein, F. (2006). Peripheral Amplification of Sweating-Aa Role for Calcitonin Gene-Related Peptide. J. Physiol. 576, 823–832. doi:10.1113/jphysiol.2006.116111
Schwartz, E. S., Christianson, J. A., Chen, X., La, J. H., Davis, B. M., Albers, K. M., et al. (2011). Synergistic Role of Trpv1 and Trpa1 in Pancreatic Pain and Inflammation. Gastroenterology 140, 1283–1291. doi:10.1053/j.gastro.2010.12.033
Schwartz, E. S., La, J. H., Scheff, N. N., Davis, B. M., Albers, K. M., and Gebhart, G. F. (2013). Trpv1 and Trpa1 Antagonists Prevent the Transition of Acute to Chronic Inflammation and Pain in Chronic Pancreatitis. J. Neurosci. 33, 5603–5611. doi:10.1523/JNEUROSCI.1806-12.2013
Shen, J., Tu, L., Chen, D., Tan, T., Wang, Y., and Wang, S. (2019). Trpv4 Channels Stimulate Ca(2+)-Induced Ca(2+) Release in Mouse Neurons and Trigger Endoplasmic Reticulum Stress after Intracerebral Hemorrhage. Brain Res. Bull. 146, 143–152. doi:10.1016/j.brainresbull.2018.11.024
Shigeto, M., Ramracheya, R., Tarasov, A. I., Cha, C. Y., Chibalina, M. V., Hastoy, B., et al. (2015). Glp-1 Stimulates Insulin Secretion by Pkc-dependent Trpm4 and Trpm5 Activation. J. Clin. Invest. 125, 4714–4728. doi:10.1172/JCI81975
Skrzypski, M., Kakkassery, M., Mergler, S., Grotzinger, C., Khajavi, N., Sassek, M., et al. (2013). Activation of trpv4 channel in pancreatic ins-1e beta cells enhances glucose-stimulated insulin secretion via calcium-dependent mechanisms. Febs Lett. 587, 3281–3287. doi:10.1016/j.febslet.2013.08.025
Skrzypski, M., Khajavi, N., Mergler, S., Szczepankiewicz, D., Kolodziejski, P. A., Metzke, D., et al. (2015). Trpv6 channel modulates proliferation of insulin secreting ins-1e beta cell line. Biochim. Biophys. Acta 1853, 3202–3210. doi:10.1016/j.bbamcr.2015.09.012
Sobhan, U., Sato, M., Shinomiya, T., Okubo, M., Tsumura, M., Muramatsu, T., et al. (2013). Immunolocalization and Distribution of Functional Temperature-Sensitive Trp Channels in Salivary Glands. Cell Tissue Res 354, 507–519. doi:10.1007/s00441-013-1691-x
Song, H., Dong, M., Zhou, J., Sheng, W., Li, X., and Gao, W. (2018). Expression and Prognostic Significance of Trpv6 in the Development and Progression of Pancreatic Cancer. Oncol. Rep. 39, 1432–1440. doi:10.3892/or.2018.6216
Song, K., Wang, H., Kamm, G. B., Pohle, J., Reis, F. D. C., Heppenstall, P., et al. (2016). The Trpm2 Channel Is a Hypothalamic Heat Sensor that Limits Fever and Can Drive Hypothermia. Science (New York, N.Y.) 353, 1393–1398. doi:10.1126/science.aaf7537
Sours-Brothers, S., Ding, M., Graham, S., and Ma, R. (2009). Interaction between Trpc1/trpc4 Assembly and Stim1 Contributes to Store-Operated Ca2+ Entry in Mesangial Cells. Exp. Biol. Med. (Maywood, N.J.) 234, 673–682. doi:10.3181/0809-RM-279
Srivastava, S., Li, Z., Soomro, I., Sun, Y., Wang, J., Bao, L., et al. (2018). Regulation of Katp Channel Trafficking in Pancreatic Beta-Cells by Protein Histidine Phosphorylation. Diabetes 67, 849–860. doi:10.2337/db17-1433
Steinritz, D., Stenger, B., Dietrich, A., Gudermann, T., and Popp, T. (2018). Trps in Tox: Involvement of Transient Receptor Potential-Channels in Chemical-Induced Organ Toxicity-A Structured Review. Cells-Basel 7, 98. doi:10.3390/cells7080098
Storck, H., Hild, B., Schimmelpfennig, S., Sargin, S., Nielsen, N., Zaccagnino, A., et al. (2017). Ion Channels in Control of Pancreatic Stellate Cell Migration. Oncotarget 8, 769–784. doi:10.18632/oncotarget.13647
Strobl, J. S., Wonderlin, W. F., and Flynn, D. C. (1995). Mitogenic Signal Transduction in Human Breast Cancer Cells. Gen. Pharmacol. 26, 1643–1649. doi:10.1016/0306-3623(95)00062-3
Sumoza-Toledo, A., Lange, I., Cortado, H., Bhagat, H., Mori, Y., Fleig, A., et al. (2011). Dendritic Cell Maturation and Chemotaxis Is Regulated by Trpm2-Mediated Lysosomal Ca2+ Release. Faseb J. 25, 3529–3542. doi:10.1096/fj.10-178483
Sumoza-Toledo, A., and Penner, R. (2011). Trpm2: a Multifunctional Ion Channel for Calcium Signalling. J. Physiol. 589, 1515–1525. doi:10.1113/jphysiol.2010.201855
Sun, M., Goldin, E., Stahl, S., Falardeau, J. L., Kennedy, J. C., Acierno, J. J., et al. (2000). Mucolipidosis Type Iv Is Caused by Mutations in a Gene Encoding a Novel Transient Receptor Potential Channel. Hum. Mol. Genet. 9, 2471–2478. doi:10.1093/hmg/9.17.2471
Sundivakkam, P. C., Freichel, M., Singh, V., Yuan, J. P., Vogel, S. M., Flockerzi, V., et al. (2012). The Ca(2+) Sensor Stromal Interaction Molecule 1 (Stim1) Is Necessary and Sufficient for the Store-Operated Ca(2+) Entry Function of Transient Receptor Potential Canonical (Trpc) 1 and 4 Channels in Endothelial Cells. Mol. Pharmacol. 81, 510–526. doi:10.1124/mol.111.074658
Surkin, P. N., Gallino, S. L., Luce, V., Correa, F., Fernandez-Solari, J., and De Laurentiis, A. (2018). Pharmacological Augmentation of Endocannabinoid Signaling Reduces the Neuroendocrine Response to Stress. Psychoneuroendocrino 87, 131–140. doi:10.1016/j.psyneuen.2017.10.015
Tanaka, K., Shibuya, I., Nagamoto, T., Yamashita, H., and Kanno, T. (1996). Pituitary Adenylate Cyclase-Activating Polypeptide Causes Rapid Ca2+ Release from Intracellular Stores and Long Lasting Ca2+ Influx Mediated by Na+ Influx-dependent Membrane Depolarization in Bovine Adrenal Chromaffin Cells. Endocrinology 137, 956–966. doi:10.1210/endo.137.3.8603609
Teos, L. Y., Zheng, C. Y., Liu, X., Swaim, W. D., Goldsmith, C. M., Cotrim, A. P., et al. (2016). Adenovirus-mediated Haqp1 Expression in Irradiated Mouse Salivary Glands Causes Recovery of Saliva Secretion by Enhancing Acinar Cell Volume Decrease. Gene Ther. 23, 572–579. doi:10.1038/gt.2016.29
Togashi, K., Hara, Y., Tominaga, T., Higashi, T., Konishi, Y., Mori, Y., et al. (2006). Trpm2 Activation by Cyclic Adp-Ribose at Body Temperature Is Involved in Insulin Secretion. Embo J. 25, 1804–1815. doi:10.1038/sj.emboj.7601083
Tsavaler, L., Shapero, M. H., Morkowski, S., and Laus, R. (2001). Trp-p8, a Novel Prostate-specific Gene, Is Up-Regulated in Prostate Cancer and Other Malignancies and Shares High Homology with Transient Receptor Potential Calcium Channel Proteins. Cancer Res. 61, 3760–3769.
Tsiokas, L. (2009). Function and Regulation of Trpp2 at the Plasma Membrane. Am. J. Physiol. Ren. Physiol. 297, F1–F9. doi:10.1152/ajprenal.90277.2008
Tsvilovskyy, V. V., Zholos, A. V., Aberle, T., Philipp, S. E., Dietrich, A., Zhu, M. X., et al. (2009). Deletion of Trpc4 and Trpc6 in Mice Impairs Smooth Muscle Contraction and Intestinal Motility In Vivo. Gastroenterology 137, 1415–1424. doi:10.1053/j.gastro.2009.06.046
Uchida, K., Dezaki, K., Yoneshiro, T., Watanabe, T., Yamazaki, J., Saito, M., et al. (2017). Involvement of Thermosensitive Trp Channels in Energy Metabolism. J. Physiol. Sci. 67, 549–560. doi:10.1007/s12576-017-0552-x
Uchida, K., and Tominaga, M. (2011). The Role of Thermosensitive Trp (Transient Receptor Potential) Channels in Insulin Secretion. Endocr. J. 58, 1021–1028. doi:10.1507/endocrj.ej11-0130
Urra, H., Dufey, E., Lisbona, F., Rojas-Rivera, D., and Hetz, C. (2013). When Er Stress Reaches a Dead End. Biochim. Biophys. Acta 1833, 3507–3517. doi:10.1016/j.bbamcr.2013.07.024
Van Haute, C., De Ridder, D., and Nilius, B. (2010). Trp Channels in Human Prostate. TheScientificWorldJournal 10, 1597–1611. doi:10.1100/tsw.2010.149
VanHouten, J. N., and Wysolmerski, J. J. (2007). Transcellular Calcium Transport in Mammary Epithelial Cells. J. Mammary Gland Biol. 12, 223–235. doi:10.1007/s10911-007-9057-1
Vazquez, G., Wedel, B. J., Aziz, O., Trebak, M., and Putney, J. J. (2004). The Mammalian Trpc Cation Channels. Biochim. Biophys. Acta 1742, 21–36. doi:10.1016/j.bbamcr.2004.08.015
Venkatachalam, K., Wong, C. O., and Zhu, M. X. (2015). The Role of Trpmls in Endolysosomal Trafficking and Function. Cell Calcium 58, 48–56. doi:10.1016/j.ceca.2014.10.008
Vriens, J., Owsianik, G., Janssens, A., Voets, T., and Nilius, B. (2007). Determinants of 4 Alpha-Phorbol Sensitivity in Transmembrane Domains 3 and 4 of the Cation Channel Trpv4. J. Biol. Chem. 282, 12796–12803. doi:10.1074/jbc.m610485200
Wagner, T. F., Loch, S., Lambert, S., Straub, I., Mannebach, S., Mathar, I., et al. (2008). Transient Receptor Potential M3 Channels Are Ionotropic Steroid Receptors in Pancreatic Beta Cells. Nat. Cel Biol 10, 1421–1430. doi:10.1038/ncb1801
Wang, J., Peng, S., Li, J., Wang, Y., Zhang, Z., Cheng, Y., et al. (2009). Identification of Metastasis-Associated Proteins Involved in Gallbladder Carcinoma Metastasis by Proteomic Analysis and Functional Exploration of Chloride Intracellular Channel 1. Cancer Lett. 281, 71–81. doi:10.1016/j.canlet.2009.02.020
Wang, P., Yan, Z., Zhong, J., Chen, J., Ni, Y., Li, L., et al. (2012). Transient Receptor Potential Vanilloid 1 Activation Enhances Gut Glucagon-like Peptide-1 Secretion and Improves Glucose Homeostasis. Diabetes 61, 2155–2165. doi:10.2337/db11-1503
Wang, W., Zhang, X., Gao, Q., and Xu, H. (2014). Trpml1: an Ion Channel in the Lysosome. Handb Exp. Pharmacol. 222, 631–645. doi:10.1007/978-3-642-54215-2_24
Watanabe, T., Kawada, T., Kurosawa, M., Sato, A., and Iwai, K. (1988). Adrenal Sympathetic Efferent Nerve and Catecholamine Secretion Excitation Caused by Capsaicin in Rats. Am. J. Physiol. 255, E23–E27. doi:10.1152/ajpendo.1988.255.1.e23
Watanabe, T., Kawada, T., Yamamoto, M., and Iwai, K. (1987). Capsaicin, a Pungent Principle of Hot Red Pepper, Evokes Catecholamine Secretion from the Adrenal Medulla of Anesthetized Rats. Biochem. Bioph Res. Co 142, 259–264. doi:10.1016/0006-291x(87)90479-7
Wehage, E., Eisfeld, J., Heiner, I., Jungling, E., Zitt, C., and Luckhoff, A. (2002). Activation of the Cation Channel Long Transient Receptor Potential Channel 2 (Ltrpc2) by Hydrogen Peroxide. A Splice Variant Reveals a Mode of Activation Independent of Adp-Ribose. J. Biol. Chem. 277, 23150–23156. doi:10.1074/jbc.M112096200
Wes, P. D., Chevesich, J., Jeromin, A., Rosenberg, C., Stetten, G., and Montell, C. (1995). Trpc1, a Human Homolog of a drosophila Store-Operated Channel. Proc. Natl. Acad. Sci. U S A. 92, 9652–9656. doi:10.1073/pnas.92.21.9652
Xu, J., Zhang, W., Cui, W., Shi, B., and Wang, H. (2019). Pkcalpha promotes insulin secretion via trpc1 phosphorylation in ins-1e cells. Biosci. Biotechnol. Biochem. 83, 1676–1682. doi:10.1080/09168451.2019.1617106
Xu, X., Gupta, S., Hu, W., McGrath, B. C., and Cavener, D. R. (2011). Hyperthermia Induces the Er Stress Pathway. Plos One 6, e23740. doi:10.1371/journal.pone.0023740
Yamamoto, S., Shimizu, S., Kiyonaka, S., Takahashi, N., Wajima, T., Hara, Y., et al. (2008). Trpm2-mediated Ca2+influx Induces Chemokine Production in Monocytes that Aggravates Inflammatory Neutrophil Infiltration. Nat. Med. 14, 738–747. doi:10.1038/nm1758
Yee, N. S., Chan, A. S., Yee, J. D., and Yee, R. K. (2012). Trpm7 and Trpm8 Ion Channels in Pancreatic Adenocarcinoma: Potential Roles as Cancer Biomarkers and Targets. Scientifica (Cairo) 2012, 415158. doi:10.6064/2012/415158
Yee, N. S., Zhou, W., and Lee, M. (2010). Transient Receptor Potential Channel Trpm8 Is Over-expressed and Required for Cellular Proliferation in Pancreatic Adenocarcinoma. Cancer Lett. 297, 49–55. doi:10.1016/j.canlet.2010.04.023
Yee, N. S., Zhou, W., Lee, M., and Yee, R. K. (2012). Targeted Silencing of Trpm7 Ion Channel Induces Replicative Senescence and Produces Enhanced Cytotoxicity with Gemcitabine in Pancreatic Adenocarcinoma. Cancer Lett. 318, 99–105. doi:10.1016/j.canlet.2011.12.007
Yee, N. S., Zhou, W., and Liang, I. C. (2011). Transient Receptor Potential Ion Channel Trpm7 Regulates Exocrine Pancreatic Epithelial Proliferation by Mg2+-Sensitive Socs3a Signaling in Development and Cancer. Dis. Model. Mech. 4, 240–254. doi:10.1242/dmm.004564
Yeung, B. H., Griffiths, K., Berger, L., Paudel, O., Shin, M., Rui, L., et al. (2021). Leptin Induces Epigenetic Regulation of Transient Receptor Potential Melastatin 7 in Rat Adrenal Pheochromocytoma Cells. Am. J. Resp Cel Mol 65, 214–221. doi:10.1165/rcmb.2020-0374OC
Yoshida, T., Yoshioka, K., Wakabayashi, Y., Nishioka, H., and Kondo, M. (1988). Effects of Capsaicin and Isothiocyanate on Thermogenesis of Interscapular Brown Adipose Tissue in Rats. J. Nutr. Sci. Vitaminol 34, 587–594. doi:10.3177/jnsv.34.587
Yosida, M., Dezaki, K., Uchida, K., Kodera, S., Lam, N. V., Ito, K., et al. (2014). Involvement of Camp/epac/trpm2 Activation in Glucose- and Incretin-Induced Insulin Secretion. Diabetes 63, 3394–3403. doi:10.2337/db13-1868
Zeng, W., Yuan, J. P., Kim, M. S., Choi, Y. J., Huang, G. N., Worley, P. F., et al. (2008). Stim1 gates Trpc Channels, but Not Orai1, by Electrostatic Interaction. Mol. Cel 32, 439–448. doi:10.1016/j.molcel.2008.09.020
Zhang, H., Zhou, L., Shi, W., Song, N., Yu, K., and Gu, Y. (2012). A Mechanism Underlying the Effects of Polyunsaturated Fatty Acids on Breast Cancer. Int. J. Mol. Med. 30, 487–494. doi:10.3892/ijmm.2012.1022
Zhang, P., Liu, X., Li, H., Chen, Z., Yao, X., Jin, J., et al. (2017). Trpc5-induced Autophagy Promotes Drug Resistance in Breast Carcinoma via Camkkβ/ampkα/mtor Pathway. Sci. Rep-uk 7, 1. doi:10.1038/s41598-017-03230-w
Zhang, Y., Catalan, M. A., and Melvin, J. E. (2012). Trpv4 Activation in Mouse Submandibular Gland Modulates Ca2+ Influx and Salivation. Am. J. Physiol. Gastrointest. Liver Physiol. 303, G1365–G1372. doi:10.1152/ajpgi.00366.2012
Zhao, D., Han, X., Huang, L., Wang, J., Zhang, X., Jeon, J., et al. (2019). Transcription Factor Zfhx3 Regulates Calcium Influx in Mammary Epithelial Cells in Part via the Trpv6 Calcium Channel. Biochem. Bioph Res. Co 519, 366–371. doi:10.1016/j.bbrc.2019.08.148
Zhong, B., Ma, S., and Wang, D. H. (2019). Trpv1 Mediates Glucose-Induced Insulin Secretion through Releasing Neuropeptides. Vivo 33, 1431–1437. doi:10.21873/invivo.11621
Zhong, X., Fu, J., Song, K., Xue, N., Gong, R., Sun, D., et al. (2016). The Role of Trpp2 in Agonist-Induced Gallbladder Smooth Muscle Contraction. Sci. China Life Sci. 59, 409–416. doi:10.1007/s11427-015-4958-5
Zhou, Y., Chi, J., Lv, W., and Wang, Y. (2021). Obesity and Diabetes as High-Risk Factors for Severe Coronavirus Disease 2019 (Covid-19). Diabetes Metab. Res. Rev. 37, e3377. doi:10.1002/dmrr.3377
Zhu, Y., Pan, Q., Meng, H., Jiang, Y., Mao, A., Wang, T., et al. (2015). Enhancement of Vascular Endothelial Growth Factor Release in Long-Term Drug-Treated Breast Cancer via Transient Receptor Potential Channel 5-Ca(2+)-Hypoxia-Inducible Factor 1α Pathway. Pharmacol. Res. 93, 36–42. doi:10.1016/j.phrs.2014.12.006
Zhuang, L., Peng, J., Tou, L., Takanaga, H., Adam, R. M., Hediger, M. A., et al. (2002). Calcium-selective Ion Channel, Cat1, Is Apically Localized in Gastrointestinal Tract Epithelia and Is Aberrantly Expressed in Human Malignancies. Lab. Invest. a J. Tech. Methods Pathol. 82, 1755–1764. doi:10.1097/01.lab.0000043910.41414.e7
Zsombok, A. (2013). Vanilloid Receptors--Do They Have a Role in Whole Body Metabolism? Evidence from Trpv1. J. Diabetes Complicat 27, 287–292. doi:10.1016/j.jdiacomp.2012.11.006
Keywords: TRP, transient receptor potential channels, gland, endocrine-related diseases, calcium channels
Citation: Liu Y, Lyu Y and Wang H (2022) TRP Channels as Molecular Targets to Relieve Endocrine-Related Diseases. Front. Mol. Biosci. 9:895814. doi: 10.3389/fmolb.2022.895814
Received: 14 March 2022; Accepted: 28 March 2022;
Published: 28 April 2022.
Edited by:
Peng Zhang, Institute of ENT and Shenzhen Key Laboratory of ENT, ChinaReviewed by:
Jiang-Yun Luo, The Chinese University of Hong Kong, ChinaCopyright © 2022 Liu, Lyu and Wang. This is an open-access article distributed under the terms of the Creative Commons Attribution License (CC BY). The use, distribution or reproduction in other forums is permitted, provided the original author(s) and the copyright owner(s) are credited and that the original publication in this journal is cited, in accordance with accepted academic practice. No use, distribution or reproduction is permitted which does not comply with these terms.
*Correspondence: Hongmei Wang, d2FuZ2hvbmdtZWk4NUAxNjMuY29t
†These authors have contributed equally to this work and share first authorship
Disclaimer: All claims expressed in this article are solely those of the authors and do not necessarily represent those of their affiliated organizations, or those of the publisher, the editors and the reviewers. Any product that may be evaluated in this article or claim that may be made by its manufacturer is not guaranteed or endorsed by the publisher.
Research integrity at Frontiers
Learn more about the work of our research integrity team to safeguard the quality of each article we publish.