- 1Department of Steroids and Proteofactors, Institute of Endocrinology, Prague, Czechia
- 2Department of Neurology and Center of Clinical Neuroscience, First Faculty of Medicine, Charles University and General University Hospital in Prague, Prague, Czechia
Steroid sulfation and desulfation participates in the regulation of steroid bioactivity, metabolism and transport. The authors focused on sulfation and desulfation balance in three neurodegenerative diseases: Alzheimer´s disease (AD), Parkinson´s disease (PD), and multiple sclerosis (MS). Circulating steroid conjugates dominate their unconjugated counterparts, but unconjugated steroids outweigh their conjugated counterparts in the brain. Apart from the neurosteroid synthesis in the central nervous system (CNS), most brain steroids cross the blood-brain barrier (BBB) from the periphery and then may be further metabolized. Therefore, steroid levels in the periphery partly reflect the situation in the brain. The CNS steroids subsequently influence the neuronal excitability and have neuroprotective, neuroexcitatory, antidepressant and memory enhancing effects. They also exert anti-inflammatory and immunoprotective actions. Like the unconjugated steroids, the sulfated ones modulate various ligand-gated ion channels. Conjugation by sulfotransferases increases steroid water solubility and facilitates steroid transport. Steroid sulfates, having greater half-lives than their unconjugated counterparts, also serve as a steroid stock pool. Sulfotransferases are ubiquitous enzymes providing massive steroid sulfation in adrenal zona reticularis and zona fasciculata.. Steroid sulfatase hydrolyzing the steroid conjugates is exceedingly expressed in placenta but is ubiquitous in low amounts including brain capillaries of BBB which can rapidly hydrolyze the steroid sulfates coming across the BBB from the periphery. Lower dehydroepiandrosterone sulfate (DHEAS) plasma levels and reduced sulfotransferase activity are considered as risk factors in AD patients. The shifted balance towards unconjugated steroids can participate in the pathophysiology of PD and anti-inflammatory effects of DHEAS may counteract the MS.
Introduction
Steroids are important components of endogenous signaling in the organism. Steroidogenesis takes place prominently in peripheral glands such as adrenals, gonads, placenta. However, some other tissues and organs, including the brain are also able to metabolize cholesterol to steroid molecules and exert effects in autocrine and paracrine manner (Labrie, 1991; Pluchino et al., 2019; Giatti et al., 2020a). The group of steroids that can influence actions in nervous system are called neuroactive steroids (NAS), with the subgroup of steroids–neurosteroids—that act in the nervous system and are also synthesized there (Corpéchot et al., 1981).
Under physiological conditions, NAS influence a broad spectrum of functions, such as brain development, sexual behavior, stress response, emotions, memory, and cognition (Vallée et al., 1997; Serra et al., 2000; Frye, 2001; Darnaudéry et al., 2002; Johansson et al., 2002; Hampl et al., 2015). In various pathophysiological states of the central nervous system (CNS), such as epilepsy, depression, anxiety and neurodegenerative diseases neuroactive steroid levels are altered (Hillen et al., 2000; Kancheva et al., 2010; Hill et al., 2011; MacKenzie and Maguire, 2013; Kanceva et al., 2015).
The most discussed NAS in humans include metabolites of progesterone, i.e., pregnanolone isomers, 5α/β-reduced metabolites of cortisol, sulfates of pregnanolone isomers, dehydroepiandrosterone sulfate (DHEAS) and pregnenolone sulfate (PregS). Furthermore, classical steroid hormones such as 17β-estradiol (E2) (Xu et al., 2008; Jiang et al., 2009) testosterone (Park-Chung et al., 1999), and progesterone (Wu et al., 1990) can act as NAS in the brain (reviewed in (Giatti et al., 2019)).
Biosynthesis of NAS, especially pregnane steroids, shows marked differences depending on the gender, age, menstrual cycle and pregnancy status (Kancheva et al., 2007). In premenopausal women, the largest proportion of pregnanolone isomers arise from progesterone synthesized in corpus luteum with the dominant metabolite allopregnanolone (3α-hydroxy-5α-pregnan-20-one) (Hill et al., 2005; Ottander et al., 2005; Hill and Očenášková, 2007). Gonadal pregnane may easily surpass the blood-brain barrier (BBB) as the brain concentrations reflect the ovarian production (Bixo et al., 1997).
Outside of pregnancy and the luteal phase of the menstrual cycle, the adrenal cortex produces most NAS and their precursors. Human zona fasciculata produces PregS (Hill and Očenášková, 2007) in relatively high concentrations. In addition, zona fasciculata synthesizes mainly cortisol whose 5α/β-reduced metabolites can act as NAS (Strömberg et al., 2005); zona reticularis primarily produces massive amounts of DHEAS (Mueller et al., 2015).
Additionally, the CNS can synthesize neurosteroids directly in the CNS or from peripheral precursors (Corpéchot et al., 1981; Corpéchot et al., 1983; Luchetti et al., 2011). Maintaining a pool of the bioavailable steroids in the site of action is a dynamic process where different steroidogenic enzymes are involved. One of the systems regulating the activity of steroids are sulfotransferases (SULTs) and steroid sulfatase (STS), which add or detach sulfate moiety, respectively. This balance could be of importance in neurodegenerative processes as well as for the transport of soluble steroid conjugates to the respective active sites.
The role of unconjugated NAS in various neurodegenerative diseases were intensively reviewed recently (Melcangi et al., 2016; Yilmaz et al., 2019; Giatti et al., 2020b; Kudova, 2021) as well as in the past (Luchetti et al., 2011; Melcangi et al., 2008; Wang et al., 2001; Melcangi and Panzica, 2009; Melcangi et al., 2011; di Michele et al., 2003). In this review, we focused mainly on sulfated steroids and sulfation and desulfation pathways in three neurodegenerative diseases: Alzheimer´s disease (AD), Parkinson’s disease (PD), multiple sclerosis (MS), while the dysregulation of sulfation processes can change the bioavailability and activity of NAS and may influence the pathogenesis and progression of some diseases.
Unconjugated Steroids
Unconjugated (free) steroids are predominantly lipophilic compounds, which can enter the cells and pass the BBB by simple nonsaturable diffusion. A major fraction of numerous steroids in circulation is bound to albumin. However, steroids can easily dissociate from the albumin complex and pass the BBB as well (Pardridge and Mietus, 1979; Pardridge and Mietus, 1980). Steroids that are bound to selective transport proteins (CBD-transcortin, SHBG-sex hormone binding globulin), are not transported through the BBB (Compagnone and Mellon, 2000). Part of steroids can be also synthesized de novo in CNS (Corpéchot et al., 1983). However, a substantial part of steroids can be synthesized from steroid precursors from periphery or can be transported from the periphery thus partly reflecting the circulating levels (Bixo et al., 1995; Kancheva et al., 2010; Kancheva et al., 2011). Therefore, the contribution of peripheral steroids to the steroid pool in CNS is important and changes in the steroid milieu in the CNS can subsequently have an impact on neuronal activity in brain (Vaňková et al., 2016). The steroid levels in cerebrospinal fluid (CSF) are generally lower than in circulation, while some CNS steroids (especially DHEA and some of its metabolites; allopregnanolone and cortisol) correlate with their peripheral levels (Kancheva et al., 2010).
Main unconjugated NAS include metabolites of progesterone (allopregnanolone, isopregnanolone, pregnanolone, epipregnanolone and pregnanediols), DHEA, E2, testosterone and their metabolites (androsterone, epiandrosterone, etiocholanolone, 3α-hydroxy-5α/β,17β-diols), 5α/β-reduced metabolites of glucocorticoids (3α-hydroxy, 5α/β-tetrahydro-cortisols, 3α-hydroxy-5α/β-tetrahydro-cortisones). Basic scheme of steroidogenesis is shown in Figure 1.
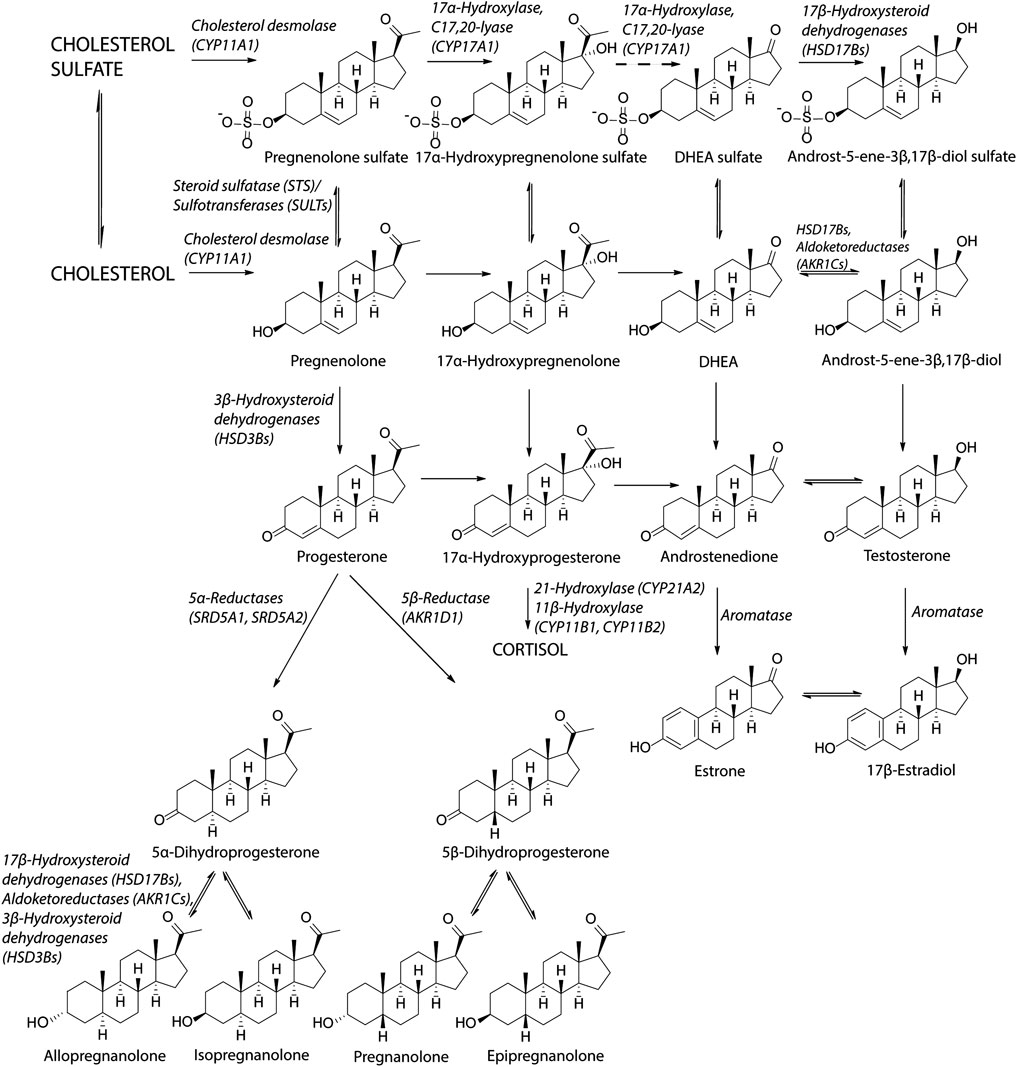
FIGURE 1. Scheme of steroidogenesis. Steroidogenic pathway for sulfated steroids is similar as the biosynthesis of unconjugated steroids. Cholesterol can be sulfated by sulfotransferase (SULT2B1b) to cholesterol sulfate (Fuda et al., 2002). Cholesterol sulfate can be converted to pregnenolone sulfate by cholesterol desmolase while cholesterol sulfate serves as a better substrate than unconjugated cholesterol (Tuckey, 1990). Pregnenolone sulfate can be subsequently converted to 17α-hydroxypregnenolone sulfate by CYP17A1 in the same way as pregnenolone (Neunzig et al., 2014). The lyase reaction of CYP17A1 has not been confirmed to take place by recent studies (Neunzig et al., 2014; Sánchez-Guijo et al., 2016; Neunzig and Bernhardt, 2018), although earlier studies indicated this conversion (Lamont et al., 1970; Jaffe et al., 1972). DHEAS can be converted to androst-5-ene-3β,17β-diol sulfate by 17β-hydroxysteroid dehydrogenases (Sánchez-Guijo et al., 2016; Qaiser et al., 2017). Regarding the unconjugated steroids, the reactions between DHEA and androst-5-ene-3β,17β-diol, androstenedione and testosterone and estrone and estradiol are preferentially conducted in the reductase direction (Purohit and Foster, 2012; Mostaghel, 2013).
DHEA is mainly of adrenal origin, but it can also be synthesized in gonads (10–20%) (Nieschlag et al., 1973) and most probably also in the CNS (Stárka et al., 2015). DHEA is a substrate for testosterone production and subsequently for estrogen synthesis. These processes occur also in the human brain (Steckelbroeck et al., 2002) and may subsequently influence the nervous system by genomic as well as by non-genomic pathways. Furthermore, DHEA itself serves as neuroactive steroid (Stárka et al., 2015). DHEA has neuroprotective, anti-glucocorticoid, anti-apoptotic, anti-inflammatory and anti-oxidative properties, increases neurite growth and has impact on neurogenesis and catecholamine synthesis and secretion. However, it can also be neurotoxic (reviewed in (Maninger et al., 2009; Stárka et al., 2015)).
Pregnanolone isomers are progesterone metabolites originated through the action of ubiquitous 5α-reductase (SRD5As) and liver 5β-reductase (AKR1D1) forming 5α- and 5β-dihydroprogesterone, respectively (Havlíková et al., 2006). The subsequent metabolism to individual pregnanolone isomers is provided by a system of subfamily 1C aldoketoreductases (AKR1Cs) and 17β-hydroxysteroid dehydrogenases (HSD17Bs) (Miller and Auchus, 2011; Rižner and Penning, 2014; He et al., 2019) previously known as 3α- and 3β-hydroxysteroid oxidoreductases. Reduced metabolites of progesterone exert anxiolytic, sedative, hypnotic and anticonvulsive effects (Frye, 1995; Klein and Herzog, 1998; Hill et al., 2010).
Sex hormones are also active in the CNS. Particularly E2 exert pleiotropic effects there, facilitating learning and memory (Frick, 2015; Sun et al., 2019; Luine and Frankfurt, 2012; Tozzi et al., 2020; Dieni et al., 2020), as well as influencing emotional (Altemus, 2019) and sexual behavior (Diotel et al., 2018). They generally act as neuroprotective substances (Fargo et al., 2009; Spence and Voskuhl, 2012; Duong et al., 2020; Yang et al., 2020) and promote neurogenesis and neuro-regeneration (Pillerová et al., 2021; Azcoitia et al., 2019). These processes in the brain take place through classical nuclear steroid receptors (estrogen receptor α and β-ERα and ERβ, androgen receptor-AR, progesterone receptor-PR), non-classical membrane-associated steroid receptors (AR, ERα, ERβ) and transmembrane receptors (zinc transporter protein 9, G protein coupled estrogen receptor 1) (Pillerová et al., 2021). Finally, E2 can exert its action through neurotransmitter receptors such as serotonin receptor (Wetzel et al., 1998), L-type voltage gated channel (Sribnick et al., 2009; Vega-Vela et al., 2017; Sánchez et al., 2014) or N-methyl-D-aspartate (NMDA) receptor (Weaver et al., 1997; Foy et al., 1999). The action of E2 on L-type voltage channel as well as NMDA receptor appears to be concentration dependent (Table 1). Progesterone has also therapeutic benefits such as reduction of inflammation and edema, preventing myelin degradation and reducing excitotoxic neuronal death (Luoma et al., 2012).
Conjugated Steroids
Conjugated steroids predominantly include steroid sulfates and glucuronides. Namely the sulfates have an important role in the regulation of steroid metabolism and transport. Steroid sulfates are hydrophilic compounds. Therefore, their passive diffusion through BBB is limited when compared with their unconjugated counterparts. Organic anion transporters (OATs) belonging to the solute carrier (SLC) transporters superfamily are the primary transporters for cellular influx of steroid sulfates. On the other hand, multidrug resistance proteins (MRP) from the ATP-binding cassette (ABC) transporter superfamily provides efflux of steroid sulfates (Sodani et al., 2012; Mueller et al., 2015). Steroid sulfates are transported from the cell mainly through MRP1 and MRP4. The same types of transporters (ABC and SLC transporters) are present on the BBB (Grube et al., 2018). It is assumed that there is a predominant influx of steroid sulfates from the circulation across the BBB to the brain due to huge concentration gradient (Wang et al., 1997; Qaiser et al., 2017; Grube et al., 2018).
The most important conjugated NAS include DHEAS, PregS and conjugated 5α/β reduced pregnane and androstane isomers. The steroid conjugates in the blood dominate over their free counterparts from one to four orders of magnitude. On the other hand, the levels of unconjugated DHEA, Preg, and reduced 5α-pregnane steroids were found in substantially higher amounts in all brain regions compared to their conjugated counterparts (Weill-Engerer et al., 2002). Looking more closely to individual brain regions, the highest levels of DHEAS were found in striatum, hypothalamus and cerebellum and those of PregS in striatum and hypothalamus (Weill-Engerer et al., 2002).
DHEAS exert neuroprotective, neuroexcitatory, antidepressant and memory enhancing effects. Together with DHEA, DHEAS has anti-inflammatory and immunomodulating effects, positive effects on neurite growth, neurogenesis and neuronal survival as described earlier (reviewed in (Maninger et al., 2009; Stárka et al., 2015)).
PregS, similarly as DHEAS, is an excitatory NAS. It has enhancing effects on the adult hippocampal neurogenesis, neurite growth and the survival of newborn neurons (Xu et al., 2012). It plays a role in the modulation of memory and learning (Smith et al., 2014; Wong et al., 2015).
Mechanism of Action of Neuroactive Steroids
Genomic Action
Steroids can surpass the BBB from periphery to the brain either by passive diffusion (unconjugated steroids) or in cooperation with transporter proteins mentioned above (steroid conjugates). Unconjugated or deconjugated steroids can bind to intracellular receptors in the brain and act as transcriptional factors regulating gene expression (Rupprecht et al., 1996). This action may be preceded by intracellular metabolization of the steroids (Rupprecht, 2003). This genomic effect is generally delayed in the onset, because it is limited by the rate of protein synthesis, but it has longer lasting effects.
Non-Genomic Action
In addition to this classical genomic effect, NAS (unconjugated as well as conjugated) are able to bind to various membrane receptors where they can act as their allosteric modulators and induce fast nongenomic effects in values from milliseconds to seconds (McEwen, 1991; Joëls, 1997). Both fast and delayed actions can potentially/subsequently alter membrane excitability (Joëls, 1997).
The mechanism of action of NAS lies mainly in affecting the excitability of the nervous cells. They are able to modulate permeability of ion channels. In the CNS, the best-known receptors modulated by NAS are type A γ-aminobutyric acid (GABAA) receptors and glutamate receptors including NMDA receptors, α-amino-3-hydroxy-5-methyl-4-isoxazolepropionic acid (AMPA) receptors and kainate receptors (Joëls, 1997; Wu and Chen, 1997; Wu et al., 1998; Yaghoubi et al., 1998; Beyenburg et al., 2001; Rupprecht, 2003; Shirakawa et al., 2005). Furthermore, interactions of NAS with glycine, transient receptor potential (TRP), nicotinic acetylcholine, muscarinic acetylcholine, sigma (σ)-receptors and several types of voltage-gated calcium channel were reported (Klangkalya and Chan, 1988; Valera et al., 1992; Monnet et al., 1995; Hu et al., 2007; Xu et al., 2008; Majeed et al., 2012; Bukanova et al., 2020).
Stereoselectivity is an important property when binding to GABAA and NMDA receptors. The presence of a 3α-hydroxy group on the A ring is necessary for the positive modulation of GABAA receptor. The GABAA receptor positive modulators include 3α-pregnane steroids (Majewska et al., 1986), including the tetrahydrodeoxycorticosterone (THDOC) isomers, as well as 3α-androstane metabolites (Turner et al., 1989; Kaminski et al., 2005). These substances act via increasing the frequency and opening time of the GABAA receptor (for chloride ions). The influx of chlorides into nerve cells reduce the neuronal excitability. Thus, these substances are neuroinhibitory and exhibit sedative, hypnotic, anesthetic, anxiolytic and anticonvulsant properties. The 3β-pregnane steroids (Wang et al., 2000), and particularly their conjugates (Park-Chung et al., 1999) as well as the Δ5 steroid sulfates (PregS, DHEAS) act as negative GABAA receptor modulators and activate the neuronal activity in this way. Nanomolar concentrations of steroids are necessary for the positive modulation of GABAAR, while the antagonists act only in micromolar amounts (Park-Chung et al., 1999). Steroid modulators of selected membrane receptors are shown in Table 1.
Positive and negative steroid modulators are also known for the NMDA receptor. Positive modulators, upon binding to the receptor, increase the influx of calcium ions into the cell and thus cause activation of the neuron. These include Δ5 steroid sulfates (Wu et al., 1991; Irwin et al., 1992) and polar conjugates of 5α-pregnane steroids (predominantly sulfates) (Weaver et al., 2000). Polar conjugates of 5β-pregnane steroids have the opposite effect (Park-Chung et al., 1994; Yaghoubi et al., 1998; Weaver et al., 2000). These substances are summarized in Table 1 together with positive and negative modulators of further receptors.
Conjugation and Deconjugation
Steroids can be conjugated either by sulfotransferases (SULTs) to form sulfates or by uridine 5′-diphospo (UDP)-glucuronosyltransferases to form glucuronides (Figure 2). These processes increase their polarity and water solubility and facilitate the excretion in urine and bile (Schiffer et al., 2019). Furthermore, sulfates having greater half-lives than their unconjugated counterparts, also function as a steroid pool in the circulation (mainly DHEAS and estrone sulfate). Finally, sulfated steroids (e.g., PregS and DHEAS) may modulate some ligand-gated ion channels in the CNS as was described above.
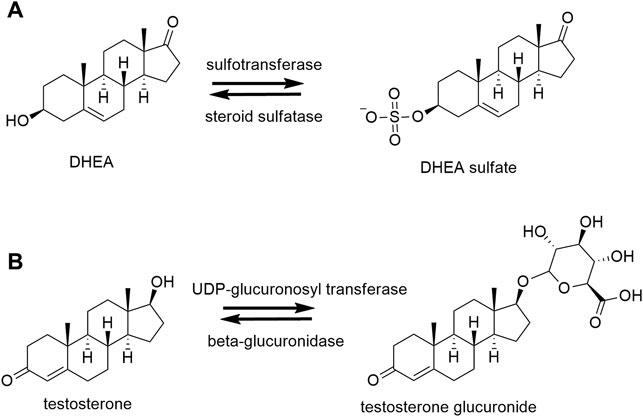
FIGURE 2. Conjugation and deconjugation reactions. (A) Example of sulfation and desulfation reactions of dehydroepiandrosterone (DHEA) by sulfotransferase and steroid sulfatase, respectively. (B) Example of glucuronidation and deglucuronidation of testosterone by uridine 5′-diphospho (UDP)-glucuronosyltransferase and β-glucuronidase, respectively.
The form of conjugation (sulfation/glucuronidation) depends on the structure of the steroid. Sulfation occurs mainly in ∆5 steroids such as DHEA, pregnenolone and estrogens (estrone). Alternatively, glucuronidation takes place mainly in the phase 2 metabolism of ∆4 steroids (Mueller et al., 2015; Schiffer et al., 2019).
Sulfotransferases
Sulfation takes place by two-step enzymatic reactions; 1) activation of the sulfate group in the form of 3′phosphoadenosine-5′-phosphosulfate (PAPS) by PAPS synthase and 2) transfer of activated sulfate on hydroxyl group of the steroid by SULT (Schiffer et al., 2019). Five cytoplasmic SULTs are known to be involved in steroid metabolism–SULT1A1, SULT1E1, SULT2A1 and 2 isoforms of SULT2B1 (SULT2B1a a SULT2B1b) (Hempel et al., 2000; Fuda et al., 2002; Chang et al., 2004; Gamage et al., 2005). They possess broad substrate specificity; instead of steroid sulfation they can also metabolize phenolic drugs and catecholamines (SULT1A), thyroid hormones (SULT1B) and sterols (SULT2B) (Strott, 2002). Regarding steroids, they can have preferred substrate e.g. SULT1E1 preferentially sulfates estrogens and SULT2A1 most androgens and pregnenolone (reviewed in (Mueller et al., 2015)) and SULT2B1 isoforms stereo-specifically sulfate 3β-hydroxysteroids (e.g., pregnenolone, cholesterol) (Meloche and Falany, 2001; Fuda et al., 2002). SULTs are ubiquitous enzymes with the highest concentrations found in the liver and intestine compared to the kidney and lung (Riches et al., 2009). SULT2A1 is strongly expressed in adrenal zona reticularis, zona fasciculata and the liver. SULT2A1 probably has a dual function: in adrenals it is responsible for massive sulfation of DHEA and pregnenolone and it detoxifies xenobiotics in the liver (Falany and Rohn-Glowacki, 2013).
Regarding the detailed expression of SULTs in brain, SULT1A1 expression was detected in several brain regions (Salman et al., 2009). SULT2A1 was detected exclusively in the thalamus and hypothalamus (Shimizu and Tamura, 2002), it was not detected in other brain regions. These findings are in agreement with the those of Salman et al. who analyzed specimens of prefrontal cortex, hippocampus, and cerebellum (Salman et al., 2011). The results on SULT2B1b expression are ambiguous. No mRNA expression of SULT2B1b in the brain was reported by some authors (Her et al., 1998; Meloche and Falany, 2001), conversely, SULT2B1b mRNA expression was reported in a large number of sections of the human brain by other research groups (Shimizu and Tamura, 2002; Salman et al., 2011). Although SULT2B1a mRNA was detected by Salman et al. (but not by (Shimizu and Tamura, 2002)), no SULT2B1a immunoreactive protein was observed. SULT1E1 expression was not found in human brain in one study (Salman et al., 2011). Taken together, some types of SULTs are apparently available in the brain and therefore may play a crucial role in neurosteroid sulfation and control.
Steroid Sulfatase
Sulfation is reversible; steroid sulfate can be desulfated by steroid sulfatase (EC 3.1.6.2, STS, aryl sulfatase C) to unconjugated steroids in the target tissue. STS belongs to the sulfatase family containing 17 members, while only STS uses steroids as substrates (Diez-Roux and Ballabio, 2005). It is expressed as a membrane associated enzyme. Immunohistochemical techniques showed localization mainly in the rough endoplasmic reticulum. Furthermore, it is localized in Golgi cisternal, trans-Golgi reticulum, plasma membranes, endosomes and lysosomes (Willemsen et al., 1988; Stein et al., 1989). The main substrates for STS are estrone sulfate, DHEAS, PregS and cholesterol sulfate (Mueller et al., 2015). It is most abundantly expressed in placenta, however, it is believed to be ubiquitous in low amounts (Reed et al., 2005) in other tissues including the brain (Iwamori et al., 1976; Steckelbroeck et al., 2004). Within the brain the high activity of STS was observed in the thalamus, hypothalamus, hippocampus and temporal lobe (Perumal and Robins, 1973; Steckelbroeck et al., 2004). Furthermore, reports from rat studies indicate that STS is present in brain capillaries of BBB and can rapidly desulfate DHEAS that comes across the BBB from the circulation (Nicolas and Fry, 2007; Qaiser et al., 2017).
Mutations in the gene for STS result in X-linked ichthyosis, which is a rare skin disorder. Several extracutaneous manifestations have been associated with this disease including corneal opacities, cryptorchidism and chondrodysplasia punctata (Fernandes et al., 2010). While STS is expressed in various brain structures, it can be hypothesized that alterations in brain function might be present. Neurological (mental retardation, epilepsy), neurodevelopmental (attention deficit hyperactivity disorder, autism) and mood disorders are more frequent in individuals with X-linked ichthyosis compared to the general population (Fernandes et al., 2010; Chatterjee et al., 2016). From neuroanatomical point of view, structural changes in basal ganglia (reduced right putamen and pallidum volume, and left accumbens volume) in female carriers were reported (Brcic et al., 2020). The described structural changes seem to be also involved in the degeneration in PD. The recent results of Hickman et al. (2022) showed that neurodevelopmental and neurodegenerative diseases may overlap. The genetic alterations which can lead to AD and PD were also described in detail by the authors (Hickman et al., 2022).
Uridine 5′-Diphospho-Glucuronosyl Transferases and β-glucuronidase
For completeness, except for sulfation, steroids may be conjugated to steroid glucuronides by UDP-glucuronosyl transferases (UGT), with the UGT1 a 2. However, this process is irreversible in the humans except for the activity of certain gut bacteria that possess β-glucuronidase activity (Schiffer et al., 2019). The process of glucuronidation is used for excretion through bile and urine and steroid glucuronides are not neuroactive.
Balances Between Conjugated and Unconjugated Neuroactive Steroids
Balances between conjugated and unconjugated NAS, except for adrenal sulfated Δ5 steroids, are ensured mainly by hepatic STS, SULTs, and possibly UDP-glucuronosyltransferases. Sulfation pathways prevail in healthy brain, colon, adrenal, and kidney while desulfation dominates in breast, ovary, prostate, testis, placenta and uterus (Mueller et al., 2015). These balances may be of great importance as unconjugated and sulfated steroids act in many cases in opposite ways on the same receptors.
For example, 5α/β-reduced metabolites with a hydroxy group in the 3α-position are positive modulators of GABAA receptors. However their sulfation reverses their action from the positive to negative modulation (Park-Chung et al., 1999). Additionally, the sulfation in the 3α-position enables steroid modulation at NMDA receptor. These data suggest that sulfation and desulfation might be the critical point in steroid regulation of GABAergic and glutamatergic neurotransmission (Park-Chung et al., 1994; Park-Chung et al., 1999). Sulfation also increases the polarity of substances and contributes to their better solubility in the circulation, while rather inhibiting their passage through the BBB.
Sulfation in Neurodegenerative Diseases
Neurodegenerative diseases including multiple sclerosis (MS), Alzheimer’s disease (AD) and Parkinson’s disease (PD) are generally characterized by progressive alterations in the brain and the spinal cord (Bianchi et al., 2020). These diseases are usually accompanied by neuroinflammation, which may contribute to neurodegeneration (Yilmaz et al., 2019). In addition, neurosteroid synthesis can be affected by neuroinflammation and vice versa, neuroactive steroids can influence neuroinflammation. Alterations in neurosteroids (mainly unconjugated) in the aforementioned neurogenerative diseases were thoroughly reviewed in 2011 by Luchetti et al. (2011). The current review presents the results of some of the later published studies.
Alzheimer’s Disease
The pathophysiology of Alzheimer’s disease (AD) is characterized by a formation of extracellular amyloid plaques in the cortex and limbic system, aggregation of hyperphosphorylated τ-protein causing intracellular neurofibrillary tangles and is often accompanied by reactive microgliosis and loss of synapses, cholinergic, serotonergic, and noradrenergic function together with glutamatergic dysfunction (López and DeKosky, 2008; Reitz and Mayeux, 2014; Vaňková et al., 2016). The clinical picture is formed by memory loss and cognitive impairment that are often accompanied by various neurological and psychiatric symptoms (López and DeKosky, 2008).
The study of Vaňková et al. (2016) examined 16 AD female patients and 22 sex- and age-matched heathy controls. The measurement of 30 unconjugated steroids and 17 conjugates in the circulation of the AD patients showed altered various steps of the steroidogenesis. The authors found a shift from conjugated to free (unconjugated) steroids in the AD patients probably due to the reduced SULT2A1 activity in the liver and the adrenal zona reticularis. Despite this finding, the relative overproduction of C21 steroids was sufficient to maintain higher levels of sulfates such as PregS, allopregnanolone sulfate, conjugated pregnanolone and conjugated 5β-pregnane-3α,20α-diol in AD patients (Vaňková et al., 2016). The same findings of attenuated sulfotransferase activity measured as the ratio between conjugated and corresponding unconjugated steroids were confirmed in the cohort of 18 male and 16 female AD patients compared to corresponding age- and gender-controls (Vaňková et al., 2015).
Generally, lower plasma levels of DHEAS in AD patients are reported when compared with control subjects (Näsman et al., 1991; Genedani et al., 2004; Aldred and Mecocci, 2010; Pan et al., 2019). Besides the lower plasma levels of DHEAS, Yanase et al. also found lower values of DHEAS/DHEA ratio in patients with AD and cerebrovascular dementia. This indicates decreased peripheral sulfotransferase activity in dementias in general (Yanase et al., 1996). A recent meta-analysis showed lower DHEAS plasma levels in AD patients (Pan et al., 2019), in accordance with reduced sulfotransferase activity in AD patients. Furthermore, AD patients with higher DHEAS plasma levels were more successful in some memory tasks than patients with lower DHEAS levels (Carlson et al., 1999). Moreover, the results from a prospective study supported the role of lower DHEAS as a risk factor for AD (Hillen et al., 2000) and indicates attenuated sulfotransferase activity even before the development of the disease.
The conclusions drawn from the examinations of the circulating steroids are in line with the observations in CSF, where the steroid levels may also reflect the steroid production and metabolism in the brain. Higher DHEA but lower DHEAS levels in CSF were reported in patients with AD and vascular dementia (Kim et al., 2003). This indicates the attenuated sulfation in the brain of patients with dementia and suggests that DHEA itself does not protect from neurodegeneration. However, it may be a compensatory mechanism against the neurodegenerative process. Lower DHEAS and PregS levels were found in certain brain regions of AD patients examined postmortem together with negative correlation of these sulfates with key proteins involved in plaque formation (Weill-Engerer et al., 2002). These results allow to speculate about the neuroprotective role of sulfated steroids and/or the sulfation in AD.
Data from genome wide association study (GWAS) showed downregulation of STS gene in patients with AD (Wu et al., 2019). Further GWAS revealed eight independent single nucleotide polymorphisms (SNPs) associated with serum DHEAS concentration. The results elucidated a certain role for SULT2A1 gene which provides information about key mechanisms of degeneration and aging (Zhai et al., 2011).
STS inhibitors influence the ratio between sulfated and non-sulfated steroids which subsequently modulate brain function. Administration of the STS inhibitor DU-14 to rats increased plasma DHEAS, decreased plasma DHEA and enhanced hippocampal acetylcholine release and memory (Rhodes et al., 1997). Additionally, significantly higher levels of serotonin in the striatum and hippocampus were reported in mice lacking STS gene (Trent et al., 2012). Therefore, steroid sulfation may influence processes in the hippocampus (one of the earliest sites affected in AD (Braak et al., 1993; Mu and Gage, 2011)) by multiple mechanisms, including an alteration of the cholinergic and serotoninergic signaling (Trent et al., 2012).
A recent study of Pérez-Jiménez et al. showed that the treatment with STS inhibitor STX64 (Irosustat) resulted in higher pool of sulfated steroids and subsequently increased longevity, improved cognitive symptoms and plaque formation in a chronic AD murine model (Pérez-Jiménez et al., 2021). Furthermore, the use of another STS inhibitor DU-14 in chronic AD murine model decreased the cognitive deficits in spatial learning and memory and protected hippocampal synaptic plasticity (Yue et al., 2016). These data may be of importance for treatment of age-related diseases such as AD in humans. Interestingly, the concept of inhibition of STS has been longer studied in the context of hormone dependent cancers (reviewed in (Foster, 2021)). The use of STS inhibitor STX64 in phase II clinical trial was efficient for the treatment of breast cancer with an acceptable safety profile (Palmieri et al., 2017).
Parkinson’s Disease
Parkinson’s disease (PD) is a neurodegenerative disorder that predominantly presents in later life with bradykinesia and at least one other symptom of resting tremor or rigidity. People with PD can develop cognitive impairment, including memory loss and dementia. Parkinson’s dementia is the second most common dementia after AD and is characterized by neurodegeneration in areas related to motor control, coordination and cognitive function (Mendell and MacLusky, 2018). These features are caused by a massive loss of dopaminergic neurons in the substantia nigra pars compacta and consequent striatal dopamine deficiency (di Michele et al., 2013). The pathological hallmark is α-synuclein aggregation into intraneuronal inclusions named Lewy bodies (Mahul-Mellier et al., 2020). Prevalence of PD is twice higher in men than in women, however, women have higher mortality rate and faster progression of the disease (Cerri et al., 2019).
The mechanism how steroid sulfation may be involved in the pathophysiology of PD might lie in the modulation of dopaminergic neurons in substantia nigra that are also under control of the excitatory glutamatergic and inhibitory GABAergic systems (Cobb and Abercrombie, 2002).
The few studies which examined alterations in neurosteroid levels in PD were mostly focusing on unconjugated NAS such as allopregnanolone (di Michele et al., 2003). While DHEAS was found to be lower in AD and vascular dementia (Yanase et al., 1996), no changes were observed in DHEAS levels in PD patients when compared with healthy controls in circulation (Genedani et al., 2004). However, the expression of SULT2B1 was downregulated in substantia nigra of PD patients with no changes in sulfatase expression (Luchetti et al., 2010). These results indicate that there can be brain region-specific changes in the bioavailability of neuroactive sulfate steroids, which may consequently affect the balance between GABAergic and glutamatergic systems and finally worsen the degeneration of dopaminergic cells (di Michele et al., 2013). The question is whether the decreased levels of neuroprotective NAS contribute to the neurodegeneration or they may be the primary cause of it (Luchetti et al., 2011).
STS inhibitors as well as mutations in STS gene were tested in Caenorhabditis elegans PD model. Mutation in STS gene or administration of STX64 improved mobility and decreased the number of α-synuclein aggregates (Pérez-Jiménez et al., 2021) indicating that the use of STS inhibitors in PD could be also a promising treatment option.
Multiple Sclerosis
Multiple sclerosis (MS) is an autoimmune inflammatory and demyelinating disease of the central nervous system with symptoms occurring most often between age of 20–40. Clinical representation is variable with either cognitive and/or motor impairment depending on the localization of the lesion(s). Compared to PD, the prevalence of MS is, on the contrary, at least twice higher in women than in men (Luchetti et al., 2014).
Several studies examined changes in unconjugated NAS in the brain of MS patients. DHEA and allopregnanolone levels in the white matter of MS patients were reported to be decreased (Noorbakhsh et al., 2011; Boghozian et al., 2017). Gender-dependent changes in progesterone and estradiol synthesis and signaling in MS lesions have been described where estrogen pathways were predominantly activated in MS lesions of male patients whereas progesterone pathways were predominantly activated in MS lesions of female patients (Luchetti et al., 2014). In CSF of MS patients, increased levels of pregnenolone and DHEA compared to control groups were reported (Orefice et al., 2016). Additionally, an increase in pregnenolone and isopregnanolone levels together with a decrease in dihydroprogesterone and allopregnanolone levels in CSF of male MS patients were observed in the study of Caruso el al. (Caruso et al., 2014). Dysregulation in biosynthesis of allopregnanolone of MS patients and its potentially therapeutic role was recently reviewed (Melcangi and Panzica, 2014; Noorbakhsh et al., 2014; Balan et al., 2019). To the best of the authors’ knowledge, no information about sulfated steroids and sulfation pathways in MS patients in brain tissue or CSF is available.
At the peripheral level, low plasma testosterone was found in men as well as in women with MS (Foster et al., 2003; Tomassini et al., 2005; Foroughipour et al., 2012). A recent study of Cheng et al. found changes in isopregnanolone and allopregnanolone plasma levels when comparing patients with relapsing-remitting MS and patients with a clinically isolated syndrome (Cheng et al., 2021).
In a study of Kancheva et al., 51 steroids and steroid polar conjugates were analyzed in 12 women with MS and 6 sex-and age-matched controls in follicular phase of menstrual cycle. Intriguingly, the results showed higher levels of C21 steroids including pregnenolone and 3α/β pregnane isomers and also higher levels of conjugated steroids such as PregS, 20α-dihydropregnenolone sulfate, conjugates of 3α/β pregnane isomers and certain bioactive C19 steroids (androsterone, 5-androsten-3β,7α,17β-triol) in MS patients (Kanceva et al., 2015) similarly as in AD patients (Vaňková et al., 2016). The altered levels of these steroids may influence neural activity by interacting with various neurotransmitter receptors on a neuronal membrane and affect the balance between neuroprotection and excitotoxicity.
The results regarding DHEAS levels in MS are ambiguous. Levels of DHEAS did not differ between MS and control patients in the above mentioned study of Kanceva et al. (2015). On the other hand, two studies found lower levels of DHEAS in MS in comparison with healthy subjects (Ramsaransing et al., 2005; Foroughipour et al., 2012) and one study found higher DHEAS in MS patients (Ysrraelit et al., 2008). Further, lower serum levels of DHEAS and DHEA were found in patients with fatigue in comparison with those without fatigue within the progressive form of MS (Téllez et al., 2006).
The research also reveals the role of glutamate in the pathophysiology of MS. Higher glutamate concentrations were found in acute lesions and normal-appearing white matter (Srinivasan et al., 2005) and appear to contribute to the progression of the disease (Azevedo et al., 2014). GWAS which used in vivo glutamate concentration as a quantitative trait discovered that several SNPs are associated with glutamate concentrations. One of these SNPs is rs794185 (p < 6.44 × 10-7), which is within the sulfatase modifiying factor 1 (SUMF1) gene (Baranzini et al., 2010). SUMF1 is an essential factor for sulfatase activities, including the one of STS. The dysregulation of SUMF1 can lead to its diminished activity (Fraldi et al., 2007). Subsequently, an imbalance between conjugated and unconjugated steroids that can modulate glutamatergic receptors occurs.
The available data concerning the NAS and particularly sulfation pathways in association to MS in humans are limited. Therefore, the data from animal models may be helpful. In the mouse model of MS–experimental autoimmune encephalomyelitis (EAE)—a protective effect of unconjugated DHEA on the development and severity of the EAE was repeatedly reported (Du et al., 2001; Aggelakopoulou et al., 2016). Similarly, DHEAS administration to mice ameliorated EAE severity and improved neurological outcomes in EAE, possibly through anti-inflammatory effects (Boghozian et al., 2017).
Conclusion
The expression of SULTs and STS is brain region specific, the explanation of these differences across brain region remains to be elucidated. The balance between steroid sulfation and desulfation is critical to maintaining the balance between unconjugated and conjugated steroids, especially when their CNS action is reversed. In neurodegenerative diseases such as Parkinson’s disease and Alzheimer’s disease the reduced SULT expression and lower levels of steroid sulfates were reported in the brain. In multiple sclerosis, no information about steroid sulfation and steroid sulfate levels is available yet. However, as with other neurodegenerative diseases, changes in steroid sulfation could be expected. Changes in sulfation in neurodegenerative diseases occur also at the peripheral level as mainly documented by changed ratios of conjugated steroids to their unconjugated counterparts. These alterations may subsequently affect the neuronal activity in the CNS, as the circulating unconjugated steroids and to a lesser extent also the steroid conjugates from periphery surpass the BBB and enter the brain. Therefore, further research of the steroid sulfation in periphery and in brain deserves attention.
Future studies aiming to decipher the relative contributions of the effects of steroid sulfation on neurodegeneration by neurochemical/inflammatory/developmental/general health process may choose different approaches to answer these scientific questions. Animal experiments may be one of the options. However, the limitations of the animal studies are due to the different steroidogenesis in humans and commonly used laboratory animals. In fact, compared to primates, laboratory rodents have negligible steroid sulfate production and generally very different adrenal steroidogenesis (Schuler, 2021). Another route may be GWAS or transcriptomic studies, which are promising approaches suitable for studying the genome or transcriptome and the pathophysiology of human diseases. However, their disadvantage might be the need for a larger sample size (Hong and Park, 2012). Finally, steroidomic studies may also be designed to explore the mutual association between the metabolites studied.
STS inhibitors are gaining increased attention in the context of aging and age-related diseases. Use of STS inhibitors in animal studies shows promising results in increasing longevity and reducing protein aggregation in protein aggregation diseases such as AD and PD (Pérez-Jiménez et al., 2021). Based on the promising animal results clinical studies in humans are justifiable and warranted.
Author Contributions
The authors confirm contribution to the paper as follows: draft manuscript preparation–JV; study conception and supervision–RK; visualization and critical review of the manuscript–MH; literature review and critical review of the manuscript–LK; EKH–critical review and the revision of the manuscript.
Funding
The work was supported by a grant NU20-04-00450 of the Ministry of Health of the Czech Republic.
Conflict of Interest
The authors declare that the research was conducted in the absence of any commercial or financial relationships that could be construed as a potential conflict of interest.
Publisher’s Note
All claims expressed in this article are solely those of the authors and do not necessarily represent those of their affiliated organizations, or those of the publisher, the editors, and the reviewers. Any product that may be evaluated in this article, or claim that may be made by its manufacturer, is not guaranteed or endorsed by the publisher.
References
Aggelakopoulou, M., Kourepini, E., Paschalidis, N., Simoes, D. C. M., Kalavrizioti, D., Dimisianos, N., et al. (2016). Erβ-Dependent Direct Suppression of Human and Murine Th17 Cells and Treatment of Established Central Nervous System Autoimmunity by a Neurosteroid. J. Immunol. 197 (7), 2598–2609. doi:10.4049/jimmunol.1601038
Aldred, S., and Mecocci, P. (2010). Decreased Dehydroepiandrosterone (DHEA) and Dehydroepiandrosterone Sulfate (DHEAS) Concentrations in Plasma of Alzheimer's Disease (AD) Patients. Arch. Gerontol. Geriatr. 51 (1), e16–e18. doi:10.1016/j.archger.2009.07.001
Altemus, M. (2019). Neuroendocrine Networks and Functionality. Med. Clin. North America 103 (4), 601–612. doi:10.1016/j.mcna.2019.03.003
Azcoitia, I., Barreto, G. E., and Garcia-Segura, L. M. (2019). Molecular Mechanisms and Cellular Events Involved in the Neuroprotective Actions of Estradiol. Analysis of Sex Differences. Front. Neuroendocrinology 55, 100787. doi:10.1016/j.yfrne.2019.100787
Azevedo, C. J., Kornak, J., Chu, P., Sampat, M., Okuda, D. T., Cree, B. A., et al. (2014). In Vivo evidence of Glutamate Toxicity in Multiple Sclerosis. Ann. Neurol. 76 (2), 269–278. doi:10.1002/ana.24202
Balan, I., Beattie, M. C., O’Buckley, T. K., Aurelian, L., and Morrow, A. L. (2019). Endogenous Neurosteroid (3α,5α)3-Hydroxypregnan-20-One Inhibits Toll-Like-4 Receptor Activation and Pro-inflammatory Signaling in Macrophages and Brain. Sci. Rep. 9 (1), 1220. doi:10.1038/s41598-018-37409-6
Baranzini, S. E., Srinivasan, R., Khankhanian, P., Okuda, D. T., Nelson, S. J., Matthews, P. M., et al. (2010). Genetic Variation Influences Glutamate Concentrations in Brains of Patients with Multiple Sclerosis. Brain 133 (9), 2603–2611. doi:10.1093/brain/awq192
Beyenburg, S., Stoffel-Wagner, B., Bauer, J., Watzka, M., Blümcke, I., Bidlingmaier, F., et al. (2001). Neuroactive Steroids and Seizure Susceptibility. Epilepsy Res. 44 (2-3), 141–153. doi:10.1016/s0920-1211(01)00194-2
Bianchi, V. E., Rizzi, L., Bresciani, E., Omeljaniuk, R. J., and Torsello, A. (2020). Androgen Therapy in Neurodegenerative Diseases. J. Endocr. Soc. 4 (11), bvaa120. doi:10.1210/jendso/bvaa120
Bixo, M., Andersson, A., Winblad, B., Purdy, R. H., and Bäckström, T. (1997). Progesterone, 5alpha-Pregnane-3,20-Dione and 3alpha-Hydroxy-5alpha-Pregnane-20-One in Specific Regions of the Human Female Brain in Different Endocrine States. Brain Res. 764 (1-2), 173–178. doi:10.1016/s0006-8993(97)00455-1
Bixo, M., Bäckström, T., Winblad, B., and Andersson, A. (1995). Estradiol and Testosterone in Specific Regions of the Human Female Brain in Different Endocrine States. J. Steroid Biochem. Mol. Biol. 55 (3-4), 297–303. doi:10.1016/0960-0760(95)00179-4
Boghozian, R., McKenzie, B. A., Saito, L. B., Mehta, N., Branton, W. G., Lu, J., et al. (2017). Suppressed Oligodendrocyte Steroidogenesis in Multiple Sclerosis: Implications for Regulation of Neuroinflammation. Glia 65 (10), 1590–1606. doi:10.1002/glia.23179
Braak, H., Braak, E., and Bohl, J. (1993). Staging of Alzheimer-Related Cortical Destruction. Eur. Neurol. 33 (6), 403–408. doi:10.1159/000116984
Brcic, L., Underwood, J. F., Kendall, K. M., Caseras, X., Kirov, G., and Davies, W. (2020). Medical and Neurobehavioural Phenotypes in Carriers of X-Linked Ichthyosis-Associated Genetic Deletions in the UK Biobank. J. Med. Genet. 57 (10), 692–698. doi:10.1136/jmedgenet-2019-106676
Brewer, L. D., Dowling, A. L. S., Curran-Rauhut, M. A., Landfield, P. W., Porter, N. M., and Blalock, E. M. (2009). Estradiol Reverses a Calcium-Related Biomarker of Brain Aging in Female Rats. J. Neurosci. 29 (19), 6058–6067. doi:10.1523/jneurosci.5253-08.2009
Bukanova, J. V., Solntseva, E. I., and Kudova, E. (2020). Neurosteroids as Selective Inhibitors of Glycine Receptor Activity: Structure-Activity Relationship Study on Endogenous Androstanes and Androstenes. Front. Mol. Neurosci. 13, 44. doi:10.3389/fnmol.2020.00044
Carlson, L. E., Sherwin, B. B., and Chertkow, H. M. (1999). Relationships between Dehydroepiandrosterone Sulfate (DHEAS) and Cortisol (CRT) Plasma Levels and Everyday Memory in Alzheimer's Disease Patients Compared to Healthy Controls. Horm. Behav. 35 (3), 254–263. doi:10.1006/hbeh.1999.1518
Caruso, D., Melis, M., Fenu, G., Giatti, S., Romano, S., Grimoldi, M., et al. (2014). Neuroactive Steroid Levels in Plasma and Cerebrospinal Fluid of Male Multiple Sclerosis Patients. J. Neurochem. 130 (4), 591–597. doi:10.1111/jnc.12745
Cerri, S., Mus, L., and Blandini, F. (2019). Parkinson's Disease in Women and Men: What's the Difference. J. Parkinsons Dis. 9 (3), 501–515. doi:10.3233/jpd-191683
Chang, H.-J., Shi, R., Rehse, P., and Lin, S.-X. (2004). Identifying Androsterone (ADT) as a Cognate Substrate for Human Dehydroepiandrosterone Sulfotransferase (DHEA-ST) Important for Steroid Homeostasis. J. Biol. Chem. 279 (4), 2689–2696. doi:10.1074/jbc.M310446200
Chatterjee, S., Humby, T., and Davies, W. (2016). Behavioural and Psychiatric Phenotypes in Men and Boys with X-Linked Ichthyosis: Evidence from a Worldwide Online Survey. PLoS One 11 (10), e0164417. doi:10.1371/journal.pone.0164417
Chen, S.-C., Chang, T.-J., and Wu, F.-S. (2004). Competitive Inhibition of the Capsaicin Receptor-Mediated Current by Dehydroepiandrosterone in Rat Dorsal Root Ganglion Neurons. J. Pharmacol. Exp. Ther. 311 (2), 529–536. doi:10.1124/jpet.104.069096
Cheng, C., Gomez, D., McCombe, J. A., Smyth, P., Giuliani, F., Blevins, G., et al. (2021). Disability Progression in Multiple Sclerosis Is Associated with Plasma Neuroactive Steroid Profile. Neurol. Sci. 42, 5241–5247. doi:10.1007/s10072-021-05203-4
Cobb, W. S., and Abercrombie, E. D. (2002). Distinct Roles for Nigral GABA and Glutamate Receptors in the Regulation of Dendritic Dopamine Release under normal Conditions and in Response to Systemic Haloperidol. J. Neurosci. 22 (4), 1407–1413. doi:10.1523/jneurosci.22-04-01407.2002
Compagnone, N. A., and Mellon, S. H. (2000). Neurosteroids: Biosynthesis and Function of These Novel Neuromodulators. Front. Neuroendocrinology 21 (1), 1–56. doi:10.1006/frne.1999.0188
Corpéchot, C., Robel, P., Axelson, M., Sjövall, J., and Baulieu, E. E. (1981). Characterization and Measurement of Dehydroepiandrosterone Sulfate in Rat Brain. Proc. Natl. Acad. Sci. 78 (8), 4704–4707. doi:10.1073/pnas.78.8.4704
Corpéchot, C., Synguelakis, M., Talha, S., Axelson, M., Sjövall, J., Vihko, R., et al. (1983). Pregnenolone and its Sulfate Ester in the Rat Brain. Brain Research 270 (1), 119–125. doi:10.1016/0006-8993(83)90797-7
Darnaudéry, M., Pallarès, M., Piazza, P.-V., Le Moal, M., and Mayo, W. (2002). The Neurosteroid Pregnenolone Sulfate Infused into the Medial Septum Nucleus Increases Hippocampal Acetylcholine and Spatial Memory in Rats. Brain Res. 951 (2), 237–242. doi:10.1016/s0006-8993(02)03166-9
di Michele, F., Longone, P., Romeo, E., Lucchetti, S., Brusa, L., Pierantozzi, M., et al. (2003). Decreased Plasma and Cerebrospinal Fluid Content of Neuroactive Steroids in Parkinson's Disease. Neurol. Sci. 24 (3), 172–173. doi:10.1007/s10072-003-0115-1
di Michele, F., Luchetti, S., Bernardi, G., Romeo, E., and Longone, P. (2013). Neurosteroid and Neurotransmitter Alterations in Parkinson's Disease. Front. Neuroendocrinology 34 (2), 132–142. doi:10.1016/j.yfrne.2013.03.001
Dieni, C. V., Contemori, S., Biscarini, A., and Panichi, R. (2020). De Novo Synthesized Estradiol: A Role in Modulating the Cerebellar Function. Int. J. Mol. Sci. 21 (9), 3316. doi:10.3390/ijms21093316
Diez-Roux, G., and Ballabio, A. (2005). Sulfatases and Human Disease. Annu. Rev. Genom. Hum. Genet. 6, 355–379. doi:10.1146/annurev.genom.6.080604.162334
Diotel, N., Charlier, T. D., Lefebvre d'Hellencourt, C., Couret, D., Trudeau, V. L., Nicolau, J. C., et al. (2018). Steroid Transport, Local Synthesis, and Signaling within the Brain: Roles in Neurogenesis, Neuroprotection, and Sexual Behaviors. Front. Neurosci. 12, 84. doi:10.3389/fnins.2018.00084
Du, C., Khalil, M. W., and Sriram, S. (2001). Administration of Dehydroepiandrosterone Suppresses Experimental Allergic Encephalomyelitis in SJL/J Mice. J. Immunol. 167 (12), 7094–7101. doi:10.4049/jimmunol.167.12.7094
Duong, P., Tenkorang, M. A. A., Trieu, J., McCuiston, C., Rybalchenko, N., and Cunningham, R. L. (2020). Neuroprotective and Neurotoxic Outcomes of Androgens and Estrogens in an Oxidative Stress Environment. Biol. Sex. Differ. 11 (1), 12. doi:10.1186/s13293-020-0283-1
Earl, D. E., and Tietz, E. I. (2011). Inhibition of Recombinant L-type Voltage-Gated Calcium Channels by Positive Allosteric Modulators of GABAA Receptors. J. Pharmacol. Exp. Ther. 337 (1), 301–311. doi:10.1124/jpet.110.178244
Falany, C. N., and Rohn-Glowacki, K. J. (2013). SULT2B1: Unique Properties and Characteristics of a Hydroxysteroid Sulfotransferase Family. Drug Metab. Rev. 45 (4), 388–400. doi:10.3109/03602532.2013.835609
Fargo, K. N., Foecking, E. M., Jones, K. J., and Sengelaub, D. R. (2009). Neuroprotective Actions of Androgens on Motoneurons. Front. Neuroendocrinology 30 (2), 130–141. doi:10.1016/j.yfrne.2009.04.005
Fernandes, N. F., Janniger, C. K., and Schwartz, R. A. (2010). X-linked Ichthyosis: an Oculocutaneous Genodermatosis. J. Am. Acad. Dermatol. 62 (3), 480–485. doi:10.1016/j.jaad.2009.04.028
Fodor, L., Boros, A., Dezso, P., and Maksay, G. (2006). Expression of Heteromeric glycine Receptor-Channels in Rat Spinal Cultures and Inhibition by Neuroactive Steroids. Neurochem. Int. 49 (6), 577–583. doi:10.1016/j.neuint.2006.04.013
Foroughipour, A., Norbakhsh, V., Najafabadi, S. H., and Meamar, R. (2012). Evaluating Sex Hormone Levels in Reproductive Age Women with Multiple Sclerosis and Their Relationship with Disease Severity. J. Res. Med. Sci. 17 (9), 882–885. https://www.ncbi.nlm.nih.gov/pmc/articles/PMC3697216/pdf/JRMS-17-882.pdf.
Foster, P. A. (2021). Steroid Sulphatase and its Inhibitors: Past, Present and Future. Molecules 26 (10), 2852. doi:10.3390/molecules26102852
Foster, S. C., Daniels, C., Bourdette, D. N., and Bebo, B. F. (2003). Dysregulation of the Hypothalamic-Pituitary-Gonadal axis in Experimental Autoimmune Encephalomyelitis and Multiple Sclerosis. J. Neuroimmunol. 140 (1-2), 78–87. doi:10.1016/s0165-5728(03)00177-2
Foy, M. R., Xu, J., Xie, X., Brinton, R. D., Thompson, R. F., and Berger, T. W. (1999). 17β-Estradiol Enhances NMDA Receptor-Mediated EPSPs and Long-Term Potentiation. J. Neurophysiol. 81 (2), 925–929. doi:10.1152/jn.1999.81.2.925
Fraldi, A., Biffi, A., Lombardi, A., Visigalli, I., Pepe, S., Settembre, C., et al. (2007). SUMF1 Enhances Sulfatase Activities In Vivo in Five Sulfatase Deficiencies. Biochem. J. 403 (2), 305–312. doi:10.1042/bj20061783
Frick, K. M. (2015). Molecular Mechanisms Underlying the Memory-Enhancing Effects of Estradiol. Horm. Behav. 74, 4–18. doi:10.1016/j.yhbeh.2015.05.001
Frye, C. A. (1995). The Neurosteroid 3 Alpha, 5 Apha-THP Has Antiseizure and Possible Neuroprotective Effects in an Animal Model of Epilepsy. Brain Res. 696 (1-2), 113–120. doi:10.1016/0006-8993(95)00793-p
Frye, C. A. (2001). The Role of Neurosteroids and Non-genomic Effects of Progestins and Androgens in Mediating Sexual Receptivity of Rodents. Brain Res. Brain Res. Rev. 37 (1-3), 201–222. doi:10.1016/s0165-0173(01)00119-9
Fuda, H., Lee, Y. C., Shimizu, C., Javitt, N. B., and Strott, C. A. (2002). Mutational Analysis of Human Hydroxysteroid Sulfotransferase SULT2B1 Isoforms Reveals that Exon 1B of the SULT2B1 Gene Produces Cholesterol Sulfotransferase, whereas Exon 1A Yields Pregnenolone Sulfotransferase. J. Biol. Chem. 277 (39), 36161–36166. doi:10.1074/jbc.M207165200
Gamage, N. U., Tsvetanov, S., Duggleby, R. G., McManus, M. E., and Martin, J. L. (2005). The Structure of Human SULT1A1 Crystallized with Estradiol. J. Biol. Chem. 280 (50), 41482–41486. doi:10.1074/jbc.M508289200
Genedani, S., Rasio, G., Cortelli, P., Antonelli, F., Guidolin, D., Galantucci, M., et al. (2004). Studies on Homocysteine and Dehydroepiandrosterone Sulphate Plasma Levels in Alzheimer's Disease Patients and in Parkinson's Disease Patients. Neurotox Res. 6 (4), 327–332. doi:10.1007/bf03033443
Giatti, S., Diviccaro, S., Falvo, E., Garcia-Segura, L. M., and Melcangi, R. C. (2020). Physiopathological Role of the Enzymatic Complex 5α-Reductase and 3α/β-Hydroxysteroid Oxidoreductase in the Generation of Progesterone and Testosterone Neuroactive Metabolites. Front. Neuroendocrinology 57, 100836. doi:10.1016/j.yfrne.2020.100836
Giatti, S., Diviccaro, S., Serafini, M. M., Caruso, D., Garcia-Segura, L. M., Viviani, B., et al. (2020). Sex Differences in Steroid Levels and Steroidogenesis in the Nervous System: Physiopathological Role. Front. Neuroendocrinology 56, 100804. doi:10.1016/j.yfrne.2019.100804
Giatti, S., Garcia-Segura, L. M., Barreto, G. E., and Melcangi, R. C. (2019). Neuroactive Steroids, Neurosteroidogenesis and Sex. Prog. Neurobiol. 176, 1–17. doi:10.1016/j.pneurobio.2018.06.007
Grube, M., Hagen, P., and Jedlitschky, G. (2018). Neurosteroid Transport in the Brain: Role of ABC and SLC Transporters. Front. Pharmacol. 9, 354. doi:10.3389/fphar.2018.00354
Hampl, R., Bičíková, M., and Sosvorová, L. (2015). Hormones and the Blood-Brain Barrier. Horm. Mol. Biol. Clin. Investig. 21 (3), 159–164. doi:10.1515/hmbci-2014-0042
Havlíková, H., Hill, M., Kancheva, L., Vrbíková, J., Pouzar, V., Cerny, I., et al. (2006). Serum Profiles of Free and Conjugated Neuroactive Pregnanolone Isomers in Nonpregnant Women of fertile Age. J. Clin. Endocrinol. Metab. 91 (8), 3092–3099. doi:10.1210/jc.2005-2785
He, X.-Y., Dobkin, C., and Yang, S.-Y. (2019). 17β-Hydroxysteroid Dehydrogenases and Neurosteroid Metabolism in the central Nervous System. Mol. Cell Endocrinol. 489, 92–97. doi:10.1016/j.mce.2018.10.002
Hempel, N., Barnett, A. C., Bolton-Grob, R. M., Liyou, N. E., and McManus, M. E. (2000). Site-directed Mutagenesis of the Substrate-Binding Cleft of Human Estrogen Sulfotransferase. Biochem. Biophysical Res. Commun. 276 (1), 224–230. doi:10.1006/bbrc.2000.3473
Her, C., Wood, T. C., Eichler, E. E., Mohrenweiser, H. W., Ramagli, L. S., Siciliano, M. J., et al. (1998). Human Hydroxysteroid Sulfotransferase SULT2B1: Two Enzymes Encoded by a Single Chromosome 19 Gene. Genomics 53 (3), 284–295. doi:10.1006/geno.1998.5518
Hickman, R. A., O’Shea, S. A., Mehler, M. F., and Chung, W. K. (2022). Neurogenetic Disorders across the Lifespan: from Aberrant Development to Degeneration. Nat. Rev. Neurol. doi:10.1038/s41582-021-00595-5 https://www.nature.com/articles/s41582-021-00595-5
Hill, M. (2007). “Neuroaktivní Pregnanové Deriváty - Fyziologie a Patofyziologie,” in Pokroky V Endokrinologii. Editor J. Očenášková (Prague: Maxdorf).
Hill, M., Popov, P., Havlíková, H., Kancheva, L., Vrbíková, J., Kancheva, R., et al. (2005). Altered Profiles of Serum Neuroactive Steroids in Premenopausal Women Treated for Alcohol Addiction. Steroids 70 (8), 515–524. doi:10.1016/j.steroids.2005.02.013
Hill, M., Vrbíková, J., Zárubová, J., Kancheva, R., Velíková, M., Kancheva, L., et al. (2011). The Steroid Metabolome in Lamotrigine-Treated Women with Epilepsy. Steroids 76 (12), 1351–1357. doi:10.1016/j.steroids.2011.07.002
Hill, M., Zárubová, J., Marusič, P., Vrbíková, J., Velíková, M., Kancheva, R., et al. (2010). Effects of Valproate and Carbamazepine Monotherapy on Neuroactive Steroids, Their Precursors and Metabolites in Adult Men with Epilepsy. J. Steroid Biochem. Mol. Biol. 122 (4), 239–252. doi:10.1016/j.jsbmb.2010.06.003
Hillen, T., Lun, A., Reischies, F. M., Borchelt, M., Steinhagen-Thiessen, E., and Schaub, R. T. (2000). DHEA-S Plasma Levels and Incidence of Alzheimer's Disease. Biol. Psychiatry 47 (2), 161–163. doi:10.1016/s0006-3223(99)00217-6
Hong, E. P., and Park, J. W. (2012). Sample Size and Statistical Power Calculation in Genetic Association Studies. Genomics Inform. 10 (2), 117–122. doi:10.5808/gi.2012.10.2.117
Hu, A.-Q., Wang, Z.-M., Lan, D.-M., Fu, Y.-M., Zhu, Y.-H., Dong, Y., et al. (2007). Inhibition of Evoked Glutamate Release by Neurosteroid Allopregnanolone via Inhibition of L-type Calcium Channels in Rat Medial Prefrontal Cortex. Neuropsychopharmacol 32 (7), 1477–1489. doi:10.1038/sj.npp.1301261
Irwin, R. P., Maragakis, N. J., Rogawski, M. A., Purdy, R. H., Farb, D. H., and Paul, S. M. (1992). Pregnenolone Sulfate Augments NMDA Receptor Mediated Increases in Intracellular Ca2+ in Cultured Rat Hippocampal Neurons. Neurosci. Lett. 141 (1), 30–34. doi:10.1016/0304-3940(92)90327-4
Iwamori, M., Moser, H. W., and Kishimoto, Y. (1976). Steroid Sulfatase in Brain: Comparison of Sulfohydrolase Activities for Various Steroid Sulfates in normal and Pathological Brains, Including the Various Forms of Metachromatic Leukodystrophy. J. Neurochem. 27 (6), 1389–1395. doi:10.1111/j.1471-4159.1976.tb02620.x
Jaffe, R. B., Pérez-palacios, G., and Diczfalusy, E. (1972). Conversion of Pregnenolone and Pregnenolone Sulfate to Other Steroid Sulfates by the Human Fetus Perfused at Midgestation1. J. Clin. Endocrinol. Metab. 35 (5), 646–654. doi:10.1210/jcem-35-5-646
Jiang, P., Kong, Y., Zhang, X.-B., Wang, W., Liu, C.-F., and Xu, T.-L. (2009). Glycine Receptor in Rat Hippocampal and Spinal Cord Neurons as a Molecular Target for Rapid Actions of 17-β-Estradiol. Mol. Pain 5, 1744–8069. doi:10.1186/1744-8069-5-2
Jiang, P., Yang, C.-X., Wang, Y.-T., and Xu, T.-L. (2006). Mechanisms of Modulation of Pregnanolone on Glycinergic Response in Cultured Spinal Dorsal Horn Neurons of Rat. Neuroscience 141 (4), 2041–2050. doi:10.1016/j.neuroscience.2006.05.009
Joëls, M. (1997). Steroid Hormones and Excitability in the Mammalian Brain. Front. Neuroendocrinology 18 (1), 2–48. doi:10.1006/frne.1996.0144
Johansson, I.-M., Birzniece, V., Lindblad, C., Olsson, T., and Bäckström, T. (2002). Allopregnanolone Inhibits Learning in the Morris Water Maze. Brain Res. 934 (2), 125–131. doi:10.1016/s0006-8993(02)02414-9
Kaminski, R. M., Marini, H., Kim, W.-J., and Rogawski, M. A. (2005). Anticonvulsant Activity of Androsterone and Etiocholanolone. Epilepsia 46 (6), 819–827. doi:10.1111/j.1528-1167.2005.00705.x
Kanceva, R., Stárka, L., Kancheva, L., Hill, M., Veliková, M., and Havrdová, E. (2015). Increased Serum Levels of C21 Steroids in Female Patients with Multiple Sclerosis. Physiol. Res. 64 (Suppl. 2), S247–S254. doi:10.33549/physiolres.933145
Kancheva, R., Hill, M., Cibula, D., Včeláková, H., Kancheva, L., Vrbíková, J., et al. (2007). Relationships of Circulating Pregnanolone Isomers and Their Polar Conjugates to the Status of Sex, Menstrual Cycle, and Pregnancy. J. Endocrinol. 195 (1), 67–78. doi:10.1677/joe-06-0192
Kancheva, R., Hill, M., Novák, Z., Chrastina, J., Kancheva, L., and Stárka, L. (2011). Neuroactive Steroids in Periphery and Cerebrospinal Fluid. Neuroscience 191, 22–27. doi:10.1016/j.neuroscience.2011.05.054
Kancheva, R., Hill, M., Novák, Z., Chrastina, J., Velíková, M., Kancheva, L., et al. (2010). Peripheral Neuroactive Steroids May Be as Good as the Steroids in the Cerebrospinal Fluid for the Diagnostics of CNS Disturbances. J. Steroid Biochem. Mol. Biol. 119 (1-2), 35–44. doi:10.1016/j.jsbmb.2009.12.006
Kim, S.-B., Hill, M., Kwak, Y.-T., Hampl, R., Jo, D.-H., and Morfin, R. (2003). Neurosteroids: Cerebrospinal Fluid Levels for Alzheimer's Disease and Vascular Dementia Diagnostics. J. Clin. Endocrinol. Metab. 88 (11), 5199–5206. doi:10.1210/jc.2003-030646
Klangkalya, B., and Chan, A. (1988). Structure-activity Relationships of Steroid Hormones on Muscarinic Receptor Binding. J. Steroid Biochem. 29 (1), 111–118. doi:10.1016/0022-4731(88)90384-6
Klein, P., and Herzog, A. G. (1998). Hormonal Effects on Epilepsy in Women. Epilepsia 39 (Suppl. 8), S9–S16. doi:10.1111/j.1528-1157.1998.tb02602.x
Kudova, E. (2021). Rapid Effects of Neurosteroids on Neuronal Plasticity and Their Physiological and Pathological Implications. Neurosci. Lett. 750, 135771. doi:10.1016/j.neulet.2021.135771
Labrie, F. (1991). Intracrinology. Mol. Cell Endocrinol. 78 (3), C113–C118. doi:10.1016/0303-7207(91)90116-a
Lamont, K. G., Pérez-Palacios, G., Pérez, A. E., and Jaffe, R. B. (1970). Pregnenolone and Pregnenolone Sulfate Metabolism by Human Fetal Testes In Vitro. Steroids 16 (1), 127–140. doi:10.1016/s0039-128x(70)80101-5
López, O. L., and DeKosky, S. T. (2008). “Clinical Symptoms in Alzheimer's Disease,” in Handbook of Clinical Neurology (Amsterdam, Netherlands: Elsevier), 207–216. doi:10.1016/s0072-9752(07)01219-5
Luchetti, S., Bossers, K., Frajese, G. V., and Swaab, D. F. (2010). Neurosteroid Biosynthetic Pathway Changes in Substantia Nigra and Caudate Nucleus in Parkinson's Disease. Brain Pathol. 20 (5), 945–951. doi:10.1111/j.1750-3639.2010.00396.x
Luchetti, S., Huitinga, I., and Swaab, D. F. (2011). Neurosteroid and GABA-A Receptor Alterations in Alzheimer's Disease, Parkinson's Disease and Multiple Sclerosis. Neuroscience 191, 6–21. doi:10.1016/j.neuroscience.2011.04.010
Luchetti, S., van Eden, C. G., Schuurman, K., van Strien, M. E., Swaab, D. F., and Huitinga, I. (2014). Gender Differences in Multiple Sclerosis. J. Neuropathol. Exp. Neurol. 73 (2), 123–135. doi:10.1097/nen.0000000000000037
Luine, V. N., and Frankfurt, M. (2012). Estrogens Facilitate Memory Processing through Membrane Mediated Mechanisms and Alterations in Spine Density. Front. Neuroendocrinology 33 (4), 388–402. doi:10.1016/j.yfrne.2012.07.004
Lundgren, P., Strömberg, J., Bäckström, T., and Wang, M. (2003). Allopregnanolone-stimulated GABA-Mediated Chloride Ion Flux Is Inhibited by 3β-Hydroxy-5α-Pregnan-20-One (Isoallopregnanolone). Brain Res. 982 (1), 45–53. doi:10.1016/s0006-8993(03)02939-1
Luoma, J. I., Stern, C. M., and Mermelstein, P. G. (2012). Progesterone Inhibition of Neuronal Calcium Signaling Underlies Aspects of Progesterone-Mediated Neuroprotection. J. Steroid Biochem. Mol. Biol. 131 (1-2), 30–36. doi:10.1016/j.jsbmb.2011.11.002
MacKenzie, G., and Maguire, J. (2013). Neurosteroids and GABAergic Signaling in Health and Disease. Biomol. Concepts 4 (1), 29–42. doi:10.1515/bmc-2012-0033
Mahul-Mellier, A.-L., Burtscher, J., Maharjan, N., Weerens, L., Croisier, M., Kuttler, F., et al. (2020). The Process of Lewy Body Formation, rather Than Simply α-synuclein Fibrillization, Is One of the Major Drivers of Neurodegeneration. Proc. Natl. Acad. Sci. USA 117 (9), 4971–4982. doi:10.1073/pnas.1913904117
Majeed, Y., Tumova, S., Green, B. L., Seymour, V. A. L., Woods, D. M., Agarwal, A. K., et al. (2012). Pregnenolone Sulphate-independent Inhibition of TRPM3 Channels by Progesterone. Cell Calcium 51 (1), 1–11. doi:10.1016/j.ceca.2011.09.005
Majewska, M. D., and Schwartz, R. D. (1987). Pregnenolone-sulfate: an Endogenous Antagonist of the Gamma-Aminobutyric Acid Receptor Complex in Brain. Brain Res. 404 (1-2), 355–360. doi:10.1016/0006-8993(87)91394-1
Majewska, M. D., Demirgo¨ren, S., Spivak, C. E., and London, E. D. (1990). The Neurosteroid Dehydroepiandrosterone Sulfate Is an Allosteric Antagonist of the GABAA Receptor. Brain Res. 526 (1), 143–146. doi:10.1016/0006-8993(90)90261-9
Majewska, M. D., Harrison, N. L., Schwartz, R. D., Barker, J. L., and Paul, S. M. (1986). Steroid Hormone Metabolites Are Barbiturate-like Modulators of the GABA Receptor. Science 232 (4753), 1004–1007. doi:10.1126/science.2422758
Maksay, G., Laube, B., and Betz, H. (2001). Subunit-specific Modulation of glycine Receptors by Neurosteroids. Neuropharmacology 41 (3), 369–376. doi:10.1016/s0028-3908(01)00071-5
Maninger, N., Wolkowitz, O. M., Reus, V. I., Epel, E. S., and Mellon, S. H. (2009). Neurobiological and Neuropsychiatric Effects of Dehydroepiandrosterone (DHEA) and DHEA Sulfate (DHEAS). Front. Neuroendocrinology 30 (1), 65–91. doi:10.1016/j.yfrne.2008.11.002
McEwen, B. S. (1991). Non-genomic and Genomic Effects of Steroids on Neural Activity. Trends Pharmacol. Sci. 12 (4), 141–147. doi:10.1016/0165-6147(91)90531-v
Melcangi, R. C., Garcia-Segura, L. M., and Mensah-Nyagan, A. G. (2008). Neuroactive Steroids: State of the Art and New Perspectives. Cell. Mol. Life Sci. 65 (5), 777–797. doi:10.1007/s00018-007-7403-5
Melcangi, R. C., Giatti, S., and Garcia-Segura, L. M. (2016). Levels and Actions of Neuroactive Steroids in the Nervous System under Physiological and Pathological Conditions: Sex-specific Features. Neurosci. Biobehavioral Rev. 67, 25–40. doi:10.1016/j.neubiorev.2015.09.023
Melcangi, R. C., and Panzica, G. C. (2014). Allopregnanolone: State of the Art. Prog. Neurobiol. 113, 1–5. doi:10.1016/j.pneurobio.2013.09.005
Melcangi, R. C., Panzica, G., and Garcia-Segura, L. M. (2011). Neuroactive Steroids: Focus on Human Brain. Neuroscience 191, 1–5. doi:10.1016/j.neuroscience.2011.06.024
Melcangi, R. C., and Panzica, G. (2009). Neuroactive Steroids: an Update of Their Roles in central and Peripheral Nervous System. Psychoneuroendocrinology 34 (Suppl. 1), S1–S8. doi:10.1016/j.psyneuen.2009.11.001
Meloche, C. A., and Falany, C. N. (2001). Expression and Characterization of the Human 3 Beta-Hydroxysteroid Sulfotransferases (SULT2B1a and SULT2B1b). J. Steroid Biochem. Mol. Biol. 77 (4-5), 261–269. doi:10.1016/s0960-0760(01)00064-4
Mendell, A. L., and MacLusky, N. J. (2018). Neurosteroid Metabolites of Gonadal Steroid Hormones in Neuroprotection: Implications for Sex Differences in Neurodegenerative Disease. Front. Mol. Neurosci. 11, 359. doi:10.3389/fnmol.2018.00359
Miller, W. L., and Auchus, R. J. (2011). The Molecular Biology, Biochemistry, and Physiology of Human Steroidogenesis and its Disorders. Endocr. Rev. 32 (1), 81–151. doi:10.1210/er.2010-0013
Monnet, F. P., Mahé, V., Robel, P., and Baulieu, E. E. (1995). Neurosteroids, via Sigma Receptors, Modulate the [3H]norepinephrine Release Evoked by N-Methyl-D-Aspartate in the Rat hippocampus. Proc. Natl. Acad. Sci. 92 (9), 3774–3778. doi:10.1073/pnas.92.9.3774
Mostaghel, E. A. (2013). Steroid Hormone Synthetic Pathways in Prostate Cancer. Transl Androl. Urol. 2 (3), 212–227. doi:10.3978/j.issn.2223-4683.2013.09.16
Mu, Y., and Gage, F. H. (2011). Adult Hippocampal Neurogenesis and its Role in Alzheimer's Disease. Mol. Neurodegeneration 6, 85. doi:10.1186/1750-1326-6-85
Mueller, J. W., Gilligan, L. C., Idkowiak, J., Arlt, W., and Foster, P. A. (2015). The Regulation of Steroid Action by Sulfation and Desulfation. Endocr. Rev. 36 (5), 526–563. doi:10.1210/er.2015-1036
Näsman, B., Olsson, T., Bäckström, T., Eriksson, S., Grankvist, K., Viitanen, M., et al. (1991). Serum Dehydroepiandrosterone Sulfate in Alzheimer's Disease and in Multi-Infarct Dementia. Biol. Psychiatry 30 (7), 684–690. doi:10.1016/0006-3223(91)90013-c
Neunzig, J., and Bernhardt, R. (2018). Effect of Sulfonated Steroids on Steroidogenic Cytochrome P450-dependent Steroid Hydroxylases. J. Steroid Biochem. Mol. Biol. 179, 3–7. doi:10.1016/j.jsbmb.2017.07.004
Neunzig, J., Sánchez-Guijo, A., Mosa, A., Hartmann, M. F., Geyer, J., Wudy, S. A., et al. (2014). A Steroidogenic Pathway for Sulfonated Steroids: the Metabolism of Pregnenolone Sulfate. J. Steroid Biochem. Mol. Biol. 144 B, 324–333. doi:10.1016/j.jsbmb.2014.07.005
Nicolas, L. B., and Fry, J. P. (2007). The Steroid Sulfatase Inhibitor COUMATE Attenuates rather Than Enhances Access of Dehydroepiandrosterone Sulfate to the Brain in the Mouse. Brain Res. 1174, 92–96. doi:10.1016/j.brainres.2007.07.078
Nieschlag, E., Loriaux, D. L., Ruder, H. J., Zucker, I. R., Kirschner, M. A., and Lipsett, M. B. (1973). The Secretion of Dehydroepiandrosterone and Dehydroepiandrosterone Sulphate in Man. J. Endocrinol. 57 (1), 123–134. doi:10.1677/joe.0.0570123
Noorbakhsh, F., Baker, G. B., and Power, C. (2014). Allopregnanolone and Neuroinflammation: a Focus on Multiple Sclerosis. Front. Cel. Neurosci. 8, 134. doi:10.3389/fncel.2014.00134
Noorbakhsh, F., Ellestad, K. K., Maingat, F., Warren, K. G., Han, M. H., Steinman, L., et al. (2011). Impaired Neurosteroid Synthesis in Multiple Sclerosis. Brain 134 (Pt 9), 2703–2721. doi:10.1093/brain/awr200
Orefice, N., Carotenuto, A., Mangone, G., Bues, B., Rehm, R., Cerillo, I., et al. (2016). Assessment of Neuroactive Steroids in Cerebrospinal Fluid Comparing Acute Relapse and Stable Disease in Relapsing-Remitting Multiple Sclerosis. J. Steroid Biochem. Mol. Biol. 159, 1–7. doi:10.1016/j.jsbmb.2016.02.012
Ottander, U., Poromaa, I. S., Bjurulf, E., Skytt, A., Bäckström, T., and Olofsson, J. I. (2005). Allopregnanolone and Pregnanolone Are Produced by the Human Corpus Luteum. Mol. Cell Endocrinol. 239 (1-2), 37–44. doi:10.1016/j.mce.2005.04.007
Palmieri, C., Stein, R. C., Stein, R. C., Liu, X., Hudson, E., Nicholas, H., et al. (2017). IRIS Study: a Phase II Study of the Steroid Sulfatase Inhibitor Irosustat when Added to an Aromatase Inhibitor in ER-Positive Breast Cancer Patients. Breast Cancer Res. Treat. 165 (2), 343–353. doi:10.1007/s10549-017-4328-z
Pan, X., Wu, X., Kaminga, A. C., Wen, S. W., and Liu, A. (2019). Dehydroepiandrosterone and Dehydroepiandrosterone Sulfate in Alzheimer's Disease: A Systematic Review and Meta-Analysis. Front. Aging Neurosci. 11, 61. doi:10.3389/fnagi.2019.00061
Pardridge, W. M., and Mietus, L. J. (1979). Transport of Steroid Hormones through the Rat Blood-Brain Barrier. J. Clin. Invest. 64 (1), 145–154. doi:10.1172/jci109433
Pardridge, W. M., and Mietus, L. J. (1980). Transport of Thyroid and Steroid Hormones through the Blood-Brain Barrier of the Newborn Rabbit: Primary Role of Protein-Bound Hormone*. Endocrinology 107 (6), 1705–1710. doi:10.1210/endo-107-6-1705
Park-Chung, M., Wu, F. S., and Farb, D. H. (1994). 3 Alpha-Hydroxy-5 Beta-Pregnan-20-One Sulfate: a Negative Modulator of the NMDA-Induced Current in Cultured Neurons. Mol. Pharmacol. 46 (1), 146–150.
Park-Chung, M., Malayev, A., Purdy, R. H., Gibbs, T. T., and Farb, D. H. (1999). Sulfated and Unsulfated Steroids Modulate γ-aminobutyric acidA Receptor Function through Distinct Sites. Brain Res. 830 (1), 72–87. doi:10.1016/s0006-8993(99)01381-5
Pérez-Jiménez, M. M., Monje-Moreno, J. M., Brokate-Llanos, A. M., Venegas-Calerón, M., Sánchez-García, A., Sansigre, P., et al. (2021). Steroid Hormones Sulfatase Inactivation Extends Lifespan and Ameliorates Age-Related Diseases. Nat. Commun. 12 (1), 49. doi:10.1038/s41467-020-20269-y
Perumal, A. S., and Robins, E. (1973). Regional and Subcellular Distribution of Aryl- and Steroid Sulfatases in Brain. Brain Res. 59, 349–358. doi:10.1016/0006-8993(73)90273-4
Pillerová, M., Borbélyová, V., Hodosy, J., Riljak, V., Renczés, E., Frick, K. M., et al. (2021). On the Role of Sex Steroids in Biological Functions by Classical and Non-classical Pathways. An Update. Front. Neuroendocrinology 62, 100926. doi:10.1016/j.yfrne.2021.100926
Pluchino, N., Ansaldi, Y., and Genazzani, A. R. (2019). Brain Intracrinology of Allopregnanolone during Pregnancy and Hormonal Contraception. Horm. Mol. Biol. Clin. Investig. 37 (1), 20180032. doi:10.1515/hmbci-2018-0032
Purohit, A., and Foster, P. A. (2012). Steroid Sulfatase Inhibitors for Estrogen- and Androgen-dependent Cancers. J. Endocrinol. 212 (2), 99–110. doi:10.1530/joe-11-0266
Qaiser, M. Z., Dolman, D. E. M., Begley, D. J., Abbott, N. J., Cazacu-Davidescu, M., Corol, D. I., et al. (2017). Uptake and Metabolism of Sulphated Steroids by the Blood-Brain Barrier in the Adult Male Rat. J. Neurochem. 142 (5), 672–685. doi:10.1111/jnc.14117
Ramsaransing, G. S. M., Heersema, D. J., and De Keyser, J. (2005). Serum Uric Acid, Dehydroepiandrosterone Sulphate, and Apolipoprotein E Genotype in Benign vs. Progressive Multiple Sclerosis. Eur. J. Neurol. 12 (7), 514–518. doi:10.1111/j.1468-1331.2005.01009.x
Reed, M. J., Purohit, A., Woo, L. W. L., Newman, S. P., and Potter, B. V. L. (2005). Steroid Sulfatase: Molecular Biology, Regulation, and Inhibition. Endocr. Rev. 26 (2), 171–202. doi:10.1210/er.2004-0003
Reitz, C., and Mayeux, R. (2014). Alzheimer Disease: Epidemiology, Diagnostic Criteria, Risk Factors and Biomarkers. Biochem. Pharmacol. 88 (4), 640–651. doi:10.1016/j.bcp.2013.12.024
Rhodes, M. E., Li, P. K., Burke, A. M., and Johnson, D. A. (1997). Enhanced Plasma DHEAS, Brain Acetylcholine and Memory Mediated by Steroid Sulfatase Inhibition. Brain Res. 773 (1-2), 28–32. doi:10.1016/s0006-8993(97)00867-6
Riches, Z., Stanley, E. L., Bloomer, J. C., and Coughtrie, M. W. H. (2009). Quantitative Evaluation of the Expression and Activity of Five Major Sulfotransferases (SULTs) in Human Tissues: The SULT "Pie". Drug Metab. Dispos 37 (11), 2255–2261. doi:10.1124/dmd.109.028399
Rižner, T. L., and Penning, T. M. (2014). Role of Aldo-Keto Reductase Family 1 (AKR1) Enzymes in Human Steroid Metabolism. Steroids 79, 49–63. doi:10.1016/j.steroids.2013.10.012
Rupprecht, R., Berning, B., Hauser, C. A. E., Holsboer, F., and Reul, J. M. H. M. (1996). Steroid Receptor-Mediated Effects of Neuroactive Steroids: Characterization of Structure-Activity Relationship. Eur. J. Pharmacol. 303 (3), 227–234. doi:10.1016/0014-2999(96)00036-2
Rupprecht, R. (2003). Neuroactive Steroids: Mechanisms of Action and Neuropsychopharmacological Properties. Psychoneuroendocrinology 28 (2), 139–168. doi:10.1016/s0306-4530(02)00064-1
Salman, E. D., Faye-Petersen, O., and Falany, C. N. (2011). Hydroxysteroid Sulfotransferase 2B1b Expression and Localization in normal Human Brain. Horm. Mol. Biol. Clin. Investig. 8 (1), 445–454. doi:10.1515/hmbci.2011.117
Salman, E. D., Kadlubar, S. A., and Falany, C. N. (2009). Expression and Localization of Cytosolic Sulfotransferase (SULT) 1A1 and SULT1A3 in normal Human Brain. Drug Metab. Dispos 37 (4), 706–709. doi:10.1124/dmd.108.025767
Sánchez, J. C., López-Zapata, D. F., and Pinzón, O. A. (2014). Effects of 17beta-Estradiol and IGF-1 on L-type Voltage-Activated and Stretch-Activated Calcium Currents in Cultured Rat Cortical Neurons. Neuro Endocrinol. Lett. 35 (8), 724–732. https://www.nel.edu/userfiles/articlesnew/NEL350814A09.pdf
Sánchez-Guijo, A., Neunzig, J., Gerber, A., Oji, V., Hartmann, M. F., Schuppe, H.-C., et al. (2016). Role of Steroid Sulfatase in Steroid Homeostasis and Characterization of the Sulfated Steroid Pathway: Evidence from Steroid Sulfatase Deficiency. Mol. Cell Endocrinol. 437, 142–153. doi:10.1016/j.mce.2016.08.019
Sarkar, S. N., Huang, R.-Q., Logan, S. M., Yi, K. D., Dillon, G. H., and Simpkins, J. W. (2008). Estrogens Directly Potentiate Neuronal L-type Ca2+ Channels. Proc. Natl. Acad. Sci. 105 (39), 15148–15153. doi:10.1073/pnas.0802379105
Schiffer, L., Barnard, L., Baranowski, E. S., Gilligan, L. C., Taylor, A. E., Arlt, W., et al. (2019). Human Steroid Biosynthesis, Metabolism and Excretion Are Differentially Reflected by Serum and Urine Steroid Metabolomes: A Comprehensive Review. J. Steroid Biochem. Mol. Biol. 194, 105439. doi:10.1016/j.jsbmb.2019.105439
Schuler, G. (2021). Steroid Sulfates in Domestic Mammals and Laboratory Rodents. Domest. Anim. Endocrinol. 76, 106622. doi:10.1016/j.domaniend.2021.106622
Serra, M., Pisu, M. G., Littera, M., Papi, G., Sanna, E., Tuveri, F., et al. (2000). Social Isolation-Induced Decreases in Both the Abundance of Neuroactive Steroids and GABAA Receptor Function in Rat Brain. J. Neurochem. 75 (2), 732–740. doi:10.1046/j.1471-4159.2000.0750732.x
Shimizu, M., and Tamura, H.-o. (2002). Identification and Localization of Two Hydroxysteroid Sulfotransferases in the Human Brain. J. Health Sci. 48 (5), 467–472. doi:10.1248/jhs.48.467
Shirakawa, H., Katsuki, H., Kume, T., Kaneko, S., and Akaike, A. (2005). Pregnenolone Sulphate Attenuates AMPA Cytotoxicity on Rat Cortical Neurons. Eur. J. Neurosci. 21 (9), 2329–2335. doi:10.1111/j.1460-9568.2005.04079.x
Smith, C. C., Gibbs, T. T., and Farb, D. H. (2014). Pregnenolone Sulfate as a Modulator of Synaptic Plasticity. PSYCHOPHARMACOLOGY 231 (17), 3537–3556. doi:10.1007/s00213-014-3643-x
Sodani, K., Patel, A., Kathawala, R. J., and Chen, Z.-S. (2012). Multidrug Resistance Associated Proteins in Multidrug Resistance. Chin. J. Cancer 31 (2), 58–72. doi:10.5732/cjc.011.10329
Spence, R. D., and Voskuhl, R. R. (2012). Neuroprotective Effects of Estrogens and Androgens in CNS Inflammation and Neurodegeneration. Front. Neuroendocrinology 33 (1), 105–115. doi:10.1016/j.yfrne.2011.12.001
Sribnick, E. A., Del Re, A. M., Ray, S. K., Woodward, J. J., and Banik, N. L. (2009). Estrogen Attenuates Glutamate-Induced Cell Death by Inhibiting Ca2+ Influx through L-type Voltage-Gated Ca2+ Channels. Brain Res. 1276, 159–170. doi:10.1016/j.brainres.2009.04.022
Srinivasan, R., Sailasuta, N., Hurd, R., Nelson, S., and Pelletier, D. (2005). Evidence of Elevated Glutamate in Multiple Sclerosis Using Magnetic Resonance Spectroscopy at 3 T. Brain 128 (Pt 5), 1016–1025. doi:10.1093/brain/awh467
Stárka, L., Dušková, M., and Hill, M. (2015). Dehydroepiandrosterone: a Neuroactive Steroid. J. Steroid Biochem. Mol. Biol. 145, 254–260. doi:10.1016/j.jsbmb.2014.03.008
Steckelbroeck, S., Nassen, A., Ugele, B., Ludwig, M., Watzka, M., Reissinger, A., et al. (2004). Steroid Sulfatase (STS) Expression in the Human Temporal Lobe: Enzyme Activity, mRNA Expression and Immunohistochemistry Study. J. Neurochem. 89 (2), 403–417. doi:10.1046/j.1471-4159.2004.02336.x
Steckelbroeck, S., Watzka, M., Lütjohann, D., Makiola, P., Nassen, A., Hans, V. H. J., et al. (2002). Characterization of the Dehydroepiandrosterone (DHEA) Metabolism via Oxysterol 7α-Hydroxylase and 17-ketosteroid Reductase Activity in the Human Brain. J. Neurochem. 83 (3), 713–726. doi:10.1046/j.1471-4159.2002.01187.x
Stein, C., Hille, A., Seidel, J., Rijnbout, S., Waheed, A., Schmidt, B., et al. (1989). Cloning and Expression of Human Steroid-Sulfatase. J. Biol. Chem. 264 (23), 13865–13872. doi:10.1016/s0021-9258(18)80080-1
Strömberg, J., Bäckström, T., and Lundgren, P. (2005). Rapid Non-genomic Effect of Glucocorticoid Metabolites and Neurosteroids on the γ-aminobutyric Acid-A Receptor. Eur. J. Neurosci. 21 (8), 2083–2088. doi:10.1111/j.1460-9568.2005.04047.x
Strott, C. A. (2002). Sulfonation and Molecular Action. Endocr. Rev. 23 (5), 703–732. doi:10.1210/er.2001-0040
Sun, T., Liu, Z., Liu, M., Guo, Y., Sun, H., Zhao, J., et al. (2019). Hippocampus-specific Rictor Knockdown Inhibited 17β-Estradiol Induced Neuronal Plasticity and Spatial Memory Improvement in Ovariectomized Mice. Behav. Brain Res. 364, 50–61. doi:10.1016/j.bbr.2019.02.014
Téllez, N., Comabella, M., Julià, E. v., Río, J., Tintoré, M. a., Brieva, L., et al. (2006). Fatigue in Progressive Multiple Sclerosis Is Associated with Low Levels of Dehydroepiandrosterone. Mult. Scler. 12 (4), 487–494. doi:10.1191/135248505ms1322oa
Tomassini, V., Onesti, E., Mainero, C., Giugni, E., Paolillo, A., Salvetti, M., et al. (2005). Sex Hormones Modulate Brain Damage in Multiple Sclerosis: MRI Evidence. J. Neurol. Neurosurg. Psychiatry 76 (2), 272–275. doi:10.1136/jnnp.2003.033324
Tozzi, A., Bellingacci, L., and Pettorossi, V. E. (2020). Rapid Estrogenic and Androgenic Neurosteroids Effects in the Induction of Long-Term Synaptic Changes: Implication for Early Memory Formation. Front. Neurosci. 14, 572511. doi:10.3389/fnins.2020.572511
Trent, S., Cassano, T., Bedse, G., Ojarikre, O. A., Humby, T., and Davies, W. (2012). Altered Serotonergic Function May Partially Account for Behavioral Endophenotypes in Steroid Sulfatase-Deficient Mice. Neuropsychopharmacol 37 (5), 1267–1274. doi:10.1038/npp.2011.314
Tuckey, R. C. (1990). Side-chain Cleavage of Cholesterol Sulfate by Ovarian Mitochondria. J. Steroid Biochem. Mol. Biol. 37 (1), 121–127. doi:10.1016/0960-0760(90)90380-4
Turner, D. M., Ransom, R. W., Yang, J. S., and Olsen, R. W. (1989). Steroid Anesthetics and Naturally Occurring Analogs Modulate the Gamma-Aminobutyric Acid Receptor Complex at a Site Distinct from Barbiturates. J. Pharmacol. Exp. Ther. 248 (3), 960–966.
Valera, S., Ballivet, M., and Bertrand, D. (1992). Progesterone Modulates a Neuronal Nicotinic Acetylcholine Receptor. Proc. Natl. Acad. Sci. 89 (20), 9949–9953. doi:10.1073/pnas.89.20.9949
Vallée, M., Mayo, W., Darnaudéry, M., Corpéchot, C., Young, J., Koehl, M., et al. (1997). Neurosteroids: Deficient Cognitive Performance in Aged Rats Depends on Low Pregnenolone Sulfate Levels in the hippocampus. Proc. Natl. Acad. Sci. 94 (26), 14865–14870. doi:10.1073/pnas.94.26.14865
Vaňková, M., Hill, M., Velíková, M., Včelák, J., Vacínová, G., Dvořáková, K., et al. (2016). Preliminary Evidence of Altered Steroidogenesis in Women with Alzheimer's Disease: Have the Patients "OLDER" Adrenal Zona Reticularis. J. Steroid Biochem. Mol. Biol. 158, 157–177. doi:10.1016/j.jsbmb.2015.12.011
Vaňková, M., Hill, M., Velíková, M., Včelák, J., Vacínová, G., Lukášová, P., et al. (2015). Reduced Sulfotransferase SULT2A1 Activity in Patients with Alzheimer´s Disease. Physiol. Res. 64 (Suppl. 2), S265–S273. doi:10.33549/physiolres.933160
Vega-Vela, N. E., Osorio, D., Avila-Rodriguez, M., Gonzalez, J., García-Segura, L. M., Echeverria, V., et al. (2017). L-type Calcium Channels Modulation by Estradiol. Mol. Neurobiol. 54 (7), 4996–5007. doi:10.1007/s12035-016-0045-6
Wagner, T. F. J., Loch, S., Lambert, S., Straub, I., Mannebach, S., Mathar, I., et al. (2008). Transient Receptor Potential M3 Channels Are Ionotropic Steroid Receptors in Pancreatic β Cells. Nat. Cel Biol 10 (12), 1421–1430. doi:10.1038/ncb1801
Wang, M.-D., Wahlström, G., and Bäckström, T. (1997). The Regional Brain Distribution of the Neurosteroids Pregnenolone and Pregnenolone Sulfate Following Intravenous Infusion. J. Steroid Biochem. Mol. Biol. 62 (4), 299–306. doi:10.1016/s0960-0760(97)00041-1
Wang, M., Bäckström, T., Sundström, I., Wahlström, G., Olsson, T., Zhu, D., et al. (2001). Neuroactive Steroids and central Nervous System Disorders. Int. Rev. Neurobiol. 46, 421–459. doi:10.1016/s0074-7742(01)46071-5
Wang, M. D., Bäckström, T., and Landgren, S. (2000). The Inhibitory Effects of Allopregnanolone and Pregnanolone on the Population Spike, Evoked in the Rat Hippocampal CA1 Stratum Pyramidale In Vitro , Can Be Blocked Selectively by Epiallopregnanolone. Acta Physiol. Scand. 169 (4), 333–341. doi:10.1046/j.1365-201x.2000.00744.x
Wang, M., He, Y., Eisenman, L. N., Fields, C., Zeng, C.-M., Mathews, J., et al. (2002). 3β-Hydroxypregnane Steroids Are Pregnenolone Sulfate-like GABAAReceptor Antagonists. J. Neurosci. 22 (9), 3366–3375. doi:10.1523/jneurosci.22-09-03366.2002
Weaver, C. E., Land, M. B., Purdy, R. H., Richards, K. G., Gibbs, T. T., and Farb, D. H. (2000). Geometry and Charge Determine Pharmacological Effects of Steroids on N-Methyl-D-Aspartate Receptor-Induced Ca(2+) Accumulation and Cell Death. J. Pharmacol. Exp. Ther. 293 (3), 747–754. https://jpet.aspetjournals.org/content/293/3/747
Weaver, C. E., Park-Chung, M., Gibbs, T. T., and Farb, D. H. (1997). 17β-Estradiol Protects against NMDA-Induced Excitotoxicity by Direct Inhibition of NMDA Receptors. Brain Res. 761 (2), 338–341. doi:10.1016/s0006-8993(97)00449-6
Weill-Engerer, S., David, J.-P., Sazdovitch, V., Liere, P., Eychenne, B., Pianos, A., et al. (2002). Neurosteroid Quantification in Human Brain Regions: Comparison between Alzheimer's and Nondemented Patients. J. Clin. Endocrinol. Metab. 87 (11), 5138–5143. doi:10.1210/jc.2002-020878
Weir, C. J., Ling, A. T. Y., Belelli, D., Wildsmith, J. A. W., Peters, J. A., and Lambert, J. J. (2004). The Interaction of Anaesthetic Steroids with Recombinant glycine and GABAA Receptors. Br. J. Anaesth. 92 (5), 704–711. doi:10.1093/bja/aeh125
Wetzel, C. H. R., Hermann, B., Behl, C., Pestel, E., Rammes, G., Zieglgänsberger, W., et al. (1998). Functional Antagonism of Gonadal Steroids at the 5-hydroxytryptamine Type 3 Receptor. Mol. Endocrinol. 12 (9), 1441–1451. doi:10.1210/mend.12.9.0163
Willemsen, R., Kroos, M., Hoogeveen, A. T., van Dongen, J. M., Parenti, G., van der Loos, C. M., et al. (1988). Ultrastructural Localization of Steroid Sulphatase in Cultured Human Fibroblasts by Immunocytochemistry: a Comparative Study with Lysosomal Enzymes and the Mannose 6-phosphate Receptor. Histochem. J. 20 (1), 41–51. doi:10.1007/bf01745968
Wong, P., Sze, Y., Chang, C. C. R., Lee, J., and Zhang, X. (2015). Pregnenolone Sulfate Normalizes Schizophrenia-like Behaviors in Dopamine Transporter Knockout Mice through the AKT/GSK3β Pathway. Transl Psychiatry 5 (3), e528. doi:10.1038/tp.2015.21
Wu, F.-S., and Chen, S.-C. (1997). Mechanism Underlying the Effect of Pregnenolone Sulfate on the Kainate-Induced Current in Cultured Chick Spinal Cord Neurons. Neurosci. Lett. 222 (2), 79–82. doi:10.1016/s0304-3940(97)13350-x
Wu, F. S., Chen, S. C., and Tsai, J. J. (1997). Competitive Inhibition of the Glycine-Induced Current by Pregnenolone Sulfate in Cultured Chick Spinal Cord Neurons. Brain Res. 750 (1-2), 318–320. doi:10.1016/s0006-8993(97)00053-x
Wu, F. S., Gibbs, T. T., and Farb, D. H. (1990). Inverse Modulation of Gamma-Aminobutyric Acid- and Glycine-Induced Currents by Progesterone. Mol. Pharmacol. 37 (5), 597–602.
Wu, F. S., Gibbs, T. T., and Farb, D. H. (1991). Pregnenolone Sulfate: a Positive Allosteric Modulator at the N-Methyl-D-Aspartate Receptor. Mol. Pharmacol. 40 (3), 333–336.
Wu, F. S., Lai, C. P., and Liu, B. C. (2000). Non-competitive Inhibition of 5-HT3 Receptor-Mediated Currents by Progesterone in Rat Nodose Ganglion Neurons. Neurosci. Lett. 278 (1-2), 37–40. doi:10.1016/s0304-3940(99)00883-6
Wu, F. S., Yu, H. M., and Tsai, J. J. (1998). Mechanism Underlying Potentiation by Progesterone of the Kainate-Induced Current in Cultured Neurons. Brain Res. 779 (1-2), 354–358. doi:10.1016/s0006-8993(97)01312-7
Wu, M., Fang, K., Wang, W., Lin, W., Guo, L., and Wang, J. (2019). Identification of Key Genes and Pathways for Alzheimer's Disease via Combined Analysis of Genome-wide Expression Profiling in the hippocampus. Biophys. Rep. 5 (2), 98–109. doi:10.1007/s41048-019-0086-2
Wu, T.-W., Wang, J. M., Chen, S., and Brinton, R. D. (2005). 17β-estradiol Induced Ca2+ Influx via L-type Calcium Channels Activates the Src/ERK/cyclic-AMP Response Element Binding Protein Signal Pathway and BCL-2 Expression in Rat Hippocampal Neurons: A Potential Initiation Mechanism for Estrogen-Induced Neuroprotection. Neuroscience 135 (1), 59–72. doi:10.1016/j.neuroscience.2004.12.027
Xu, B., Yang, R., Chang, F., Chen, L., Xie, G., Sokabe, M., et al. (2012). Neurosteroid PREGS Protects Neurite Growth and Survival of Newborn Neurons in the Hippocampal Dentate Gyrus of APPswe/PS1dE9 Mice. Curr. Alzheimer Res. 9 (3), 361–372. doi:10.2174/156720512800107591
Xu, S., Cheng, Y., Keast, J. R., and Osborne, P. B. (2008). 17β-Estradiol Activates Estrogen Receptor β-Signalling and Inhibits Transient Receptor Potential Vanilloid Receptor 1 Activation by Capsaicin in Adult Rat Nociceptor Neurons. Endocrinology 149 (11), 5540–5548. doi:10.1210/en.2008-0278
Yaghoubi, N., Malayev, A., Russek, S. J., Gibbs, T. T., and Farb, D. H. (1998). Neurosteroid Modulation of Recombinant Ionotropic Glutamate Receptors. Brain Res. 803 (1-2), 153–160. doi:10.1016/s0006-8993(98)00644-1
Yanase, T., Fukahori, M., Taniguchi, S., Nishi, Y., Sakai, Y., Takayanagi, R., et al. (1996). Serum Dehydroepiandrosterone (DHEA) and DHEA-Sulfate (DHEA-S) in Alzheimer's Disease and in Cerebrovascular Dementia. Endocr. J. 43 (1), 119–123. doi:10.1507/endocrj.43.119
Yang, L., Zhou, R., Tong, Y., Chen, P., Shen, Y., Miao, S., et al. (2020). Neuroprotection by Dihydrotestosterone in LPS-Induced Neuroinflammation. Neurobiol. Dis. 140, 104814. doi:10.1016/j.nbd.2020.104814
Yilmaz, C., Karali, K., Fodelianaki, G., Gravanis, A., Chavakis, T., Charalampopoulos, I., et al. (2019). Neurosteroids as Regulators of Neuroinflammation. Front. Neuroendocrinology 55, 100788. doi:10.1016/j.yfrne.2019.100788
Ysrraelit, M. C., Gaitán, M. I., Lopez, A. S., and Correale, J. (2008). Impaired Hypothalamic-Pituitary-Adrenal axis Activity in Patients with Multiple Sclerosis. Neurology 71 (24), 1948–1954. doi:10.1212/01.wnl.0000336918.32695.6b
Yue, X.-H., Tong, J.-Q., Wang, Z.-J., Zhang, J., Liu, X., Liu, X.-J., et al. (2016). Steroid Sulfatase Inhibitor DU-14 Protects Spatial Memory and Synaptic Plasticity from Disruption by Amyloid β Protein in Male Rats. Horm. Behav. 83, 83–92. doi:10.1016/j.yhbeh.2016.05.019
Keywords: steroid sulfotransferases, steroid sulfatase, neuroactive steroids, neurosteroids, brain, Alzheimer’s disease, Parkinson's disease, multiple sclerosis
Citation: Vitku J, Hill M, Kolatorova L, Kubala Havrdova E and Kancheva R (2022) Steroid Sulfation in Neurodegenerative Diseases. Front. Mol. Biosci. 9:839887. doi: 10.3389/fmolb.2022.839887
Received: 20 December 2021; Accepted: 20 January 2022;
Published: 23 February 2022.
Edited by:
Jon Wolf Mueller, University of Birmingham, United KingdomReviewed by:
William Davies, Cardiff University, United KingdomGiovanna Di Nardo, University of Turin, Italy
Copyright © 2022 Vitku, Hill, Kolatorova, Kubala Havrdova and Kancheva. This is an open-access article distributed under the terms of the Creative Commons Attribution License (CC BY). The use, distribution or reproduction in other forums is permitted, provided the original author(s) and the copyright owner(s) are credited and that the original publication in this journal is cited, in accordance with accepted academic practice. No use, distribution or reproduction is permitted which does not comply with these terms.
*Correspondence: Jana Vitku, anZpdGt1QGVuZG8uY3o=