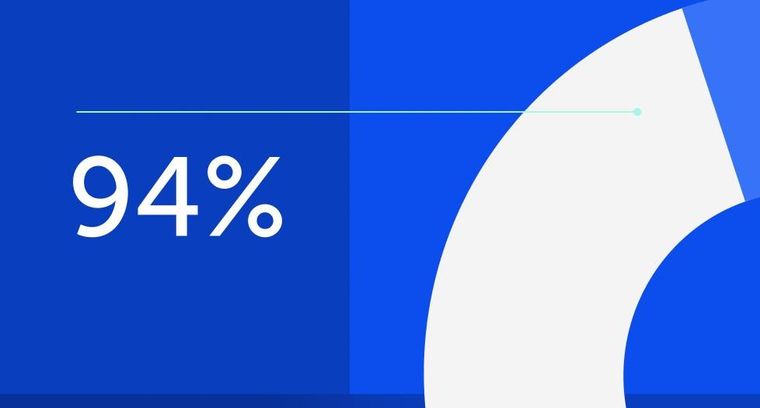
94% of researchers rate our articles as excellent or good
Learn more about the work of our research integrity team to safeguard the quality of each article we publish.
Find out more
REVIEW article
Front. Mol. Biosci., 15 December 2022
Sec. Molecular Diagnostics and Therapeutics
Volume 9 - 2022 | https://doi.org/10.3389/fmolb.2022.1036746
This article is part of the Research TopicExtracellular Vesicles and Cell-cell Communication in Normal Cellular Processes and CancerView all 7 articles
Extracellular vesicles (EV) are vesicular vesicles with phospholipid bilayer, which are present in biological fluids and extracellular microenvironment. Extracellular vesicles serve as pivotal mediators in intercellular communication by delivering lipids, proteins, and RNAs to the recipient cells. Different from extracellular vesicles derived from biofluids and that originate from cell culture, the tissue derived extracellular vesicles (Ti-EVs) send us more enriched and accurate information of tissue microenvironment. Notably, tissue derived extracellular vesicles directly participate in the crosstalk between numerous cell types within microenvironment. Current research mainly focused on the extracellular vesicles present in biological fluids and cell culture supernatant, yet the studies on tissue derived extracellular vesicles are increasing due to the tissue derived extracellular vesicles are promising agents to reflect the occurrence and development of human diseases more accurately. In this review, we aimed to clarify the characteristics of tissue derived extracellular vesicles, specify the isolation methods and the roles of tissue derived extracellular vesicles in various diseases, including tumors. Moreover, we summarized the advances and challenges of tissue derived extracellular vesicles research.
Extracellular vesicles (EVs) are nano lipid bilayer-delimited particles released by a variety of cells (Yanez-Mo et al., 2015). Microvesicles (MVs), exosomes, and apoptotic bodies are the three major subtypes of EVs, which are characterized mainly based on their size, biological properties, and production process (Crescitelli et al., 2013; Shao et al., 2018). In brief, MVs are released by cellular membrane budding with 100–1,000 nm in size, while exosomes (30–150 nm) are formed by the fusion of multivesicular bodies (MVBs) and cell membrane, and apoptotic bodies are delivered during the process of cell apoptosis with a diameter of 500–2000 nm (Raposo and Stoorvogel, 2013; DeLeo and Ikezu, 2018; Gurunathan et al., 2019). However, the three EV subtypes are difficult to distinguish thoroughly because of the overlap in size (Marar et al., 2021). Therefore, they are frequently classified as small, medium, and large EVs according to their size. All lipid-bilayer particles released by cells will be referred to as “EVs” in this review in accordance with the International Society for Extracellular Vesicles (ISEV) nomenclature, with size designations where necessary (Thery et al., 2018).
Before being released, EVs can package multiple lipids, nucleic acids and proteins. Owing to the stable lipid membrane of EVs, these cargoes are prevented from degradation (Andaloussi et al., 2013; Gupta et al., 2021). When EVs are delivered into the extracellular environment, they can directly bind to the surface of recipient cells and subsequently initiate intracellular signaling pathways or deliver their cargoes to the recipient cells through endocytosis (Valadi et al., 2007; Raposo and Stoorvogel, 2013; Mulcahy et al., 2014; Xiao et al., 2014). As a result, by acting as messengers in cell-to-cell communication, EVs play a critical role in various physiological and pathological processes, as well as the morbidity and development of numerous diseases (Mateescu et al., 2017; Hill, 2019; Raposo and Stahl, 2019; Russell et al., 2019; Urabe et al., 2020). For example, the EVs produced by tumor cells encourage the invasion and metastasis of ovarian cancer by delivering miR-106a-5p to recipient cells to target KLF6 (Zheng et al., 2022). Exosomal miR-146a-5p and miR-155-5p communicate with cancer-associated fibroblasts to promote CXCL12/CXCR7-induced metastasis of colorectal cancer (Wang et al., 2022). In wound healing, several studies proved that EVs derived from mesenchymal stromal cells (MSCs) are conducive to assist wound closure (Zhang et al., 2015; Ha et al., 2016). Furthermore, Sun et al. (Sun et al., 2022) found EVs are key mediators during the early hypoxia-induced damage signaling transduction from endothelial cells to cardiomyocytes in acute myocardial infarction (AMI).
EVs widely exist in almost all types of body fluids, including blood, urine, cerebrospinal fluid (CSF), saliva and tears. Thus they are appropriate biomarkers for the early diagnosis and prognosis in clinical application due to these body fluids are relatively easily to collect (Keller et al., 2011; Lasser et al., 2011; Muraoka et al., 2020a; Fringuello et al., 2021; Yu et al., 2021; Liu et al., 2022). As reported, the circulating miR-135a-3p in serum EVs has been characterized as a possible biological marker of non-alcoholic fatty liver disease (Jiang et al., 2021a). In Parkinson’s disease (PD), serum EVs-derived miRNAs (miR-374a-5p, miR-374b-5p, miR-199a-3p, miR-28-5p, miR-22-5p and miR-151a-5p) are promising biomarkers for PD progression and early diagnosis (He et al., 2021). Recently, a database (exoRBase 2.0) has been established to display the atlas of mRNAs and non-coding RNAs in EVs from human biological fluids, which will be beneficial for discovering novel circulating biomarkers to augment the diagnosis and therapy of human diseases (Lai et al., 2022).
Nowadays, researches on EVs have expanded from cells and body fluids to the characterization of EVs from the extracellular spaces within tissues (Perez-Gonzalez et al., 2012; Vella et al., 2017; Li et al., 2021). Increasing evidence have shown the EVs isolated from tissues possess more abundant biological information and are more accurately to reflect the alteration of tissue microenvironment compared to those derived from cells and biological fluids (Asai et al., 2015; Jang et al., 2019). These studies mainly focused on the tissue derived EVs (Ti-EVs) extracted from whole tissues (Perez-Gonzalez et al., 2012; Silverman et al., 2019; Lasser et al., 2021), or short-term culture of tissue explants, such as ex vivo tumors (Mincheva-Nilsson et al., 2016; Lunavat et al., 2017). In this review, we aimed to make a summary of the studies on Ti-EVs in recent years to provide a better understanding of the characteristics and potential values of EVs derived from tissues.
In the past decade, a variety of studies about EVs isolated from in vitro cell culture systems and body fluids have been reported (Nielsen et al., 2017; Breglio et al., 2020; Penas-Martinez et al., 2021). However, the in vitro cell culture environment is a relatively unitary system and cannot fully simulate the complex intrinsic microenvironment. Besides, the process of long-term cell cultivation can change the cell characteristics, and subsequently influence the EVs released from cell lines (Allen et al., 2016; Crescitelli et al., 2020). More important, the cell lines may not be accurately authentic to the tissues of origin. By employing gene profiling and transcriptome analysis in human glioma cell lines, Allen et al. (Allen et al., 2016) found the DNA profile of a commonly used glioma cell line U87MG was distinct from that of the original cells. On the contrary, it might be a cell line of uncertain origin. Through the analysis of more than 40 ovarian cancer cell lines, it was shown that high-grade serous ovarian cancer sample tissues and commonly utilized ovarian cancer cell lines had significantly different molecular profiles, indicating that cell lines are not completely consistent with tissues (Domcke et al., 2013). Besides, although the EVs isolated from biological fluids contain a great deal of information, they are a very mixed collection from multifarious cells and organs (De Wever and Hendrix, 2019; Huang and Xu, 2021). Therefore, the abundant information is confused and it is also difficult to determine the origin of these fluids derived-EVs.
Compared with the cell culture and body fluids derived EVs, the Ti-EVs can carry more original information, and reflect the intercellular communication more truly (Table 1) (Camino et al., 2020). In addition, the source of Ti-EVs is more clear, so they are easier to track. For instance, Ti-EVs can be directly visualized in the interstitial space of a melanoma metastatic tissues under the electron microscopy, suggesting these Ti-EVs might originate from the metastatic foci (Jang et al., 2019). By using the immuno-TEM (Transmission Electron Microscopy), several nanosized (30–100 nm in diameter) EVs which were characterized by the round shape, are observed clearly in the cytoplasm of human benign prostatic hyperplasia (BPH) cells and prostate cancer cells in the tumor microenvironment (Park et al., 2016). Owing to the above reasons, to isolate EVs from different types of tissues (such as brain, heart, lung, and kidney), and explore their roles in multiple cellular process has gained more and more attention (Figure 1) (Li et al., 2021; Chang et al., 2022).
FIGURE 1. Sources of tissue derived extracellular vesicles (Ti-EVs). Ti-EVs have been isolated from brain, heart, liver, lung, kidney and intestine tissues, as well as adipose tissue and platelet.
Currently, EVs studies are limited by the shortage of EVs isolation and purification methods (Ramirez et al., 2018; Royo et al., 2020). Up to date, several methods of EVs isolation and purification are commonly used, including differential centrifugation (DC) (Tauro et al., 2013; Pospichalova et al., 2015), ultracentrifugation (UC) (Gardiner et al., 2016), density gradients centrifugation (DGC) (Jeppesen et al., 2019), microfluidic devices (Liga et al., 2015; Konoshenko et al., 2018), size exclusion chromatography (SEC), synthetic polymer–based precipitation (Van Deun et al., 2014), and membrane filtration(UF) (Grant et al., 2011). Even though, they all have limitations, because the size and physicochemical properties of EVs always overlap with lipoproteins, chyle particles and so on. There is still a lack of methods focusing on the separation of tissue derived EVs (Cianciaruso et al., 2019; Huang et al., 2020). Furthermore, how to isolate EVs from tissues but not damage the cell membranes so that to maintain the native shape and function of Ti-EVs during the process of extraction is one of the grand challenges (Thery et al., 2018). Several published methods for isolating EVs from tissues usually involve homogenization and filtration, which can mix the extracellular environment with intracellular vesicles as well as other nanosized particles (Perez-Gonzalez et al., 2012; Gallart-Palau et al., 2016). In order to dissociate the tissues without disrupting cell, and improve the purity of Ti-EVs isolation, the method of combining the high-speed centrifugation, discontinuous sucrose gradient ultracentrifugation, filtration, and following with ultracentrifugation is recommended (Muraoka et al., 2020b; Crescitelli et al., 2021; Ruan et al., 2021). Another possible approach is utilizing collagenase (e.g. type I and III) to dissociate cells from human and animal tissues before centrifugation. This method is applicable for the tissues that are sensitive to damage, such as brain tissues, primary and metastatic tumor tissues (Vella et al., 2017; Crescitelli et al., 2020). Figure 2 illustrates a promising universal method applied for extracting Ti-EVs from diverse tissues (Crescitelli et al., 2021). Besides, extraction of EVs from fresh tissues rather than frozen tissues are more conducive to the purification of Ti-EVs (Crescitelli et al., 2020). This may be because the freezing process will cause damage to cells, resulting in cell rupture and overflow of contents. Although some strategies for separating Ti-EVs have been developed, researchers need to make appropriate adjustments to the separation method based on the type of tissue samples because of the complexity and specificity of different tissues.
FIGURE 2. The promising universal method applied for extracting Ti-EVs from a variety of tissues. At first, fresh tissues are sliced into slices and subsequently dissociated gently for 15–45 min at 37°C. Afterwards, a 70 μm pore-sized filter is used to remove tissue pieces. Centrifugation at a speed of 300–10,000 g at 4°C is conducted to remove apoptotic bodies, remaining cells and debris and the supernatant pass through a 0.22 μm filter to collect large EVs. Next, the filtrate is ultracentrifugated at a speed of 100,000–150,000 g at 4°C (>1 h) to collect small EVs. Finally, the isolated Ti-EVs are resuspended with PBS and can be stored at −80°C for a long time.
In human cancers, significant progress has been made in the study of cell culture and bio-fluid derived EVs (Becker et al., 2016; Weng et al., 2021; Namee and O'Driscoll, 2018; Vaidyanathan et al., 2018). Despite that, increasing evidence has revealed the critical roles of Ti-EVs in multiple tumors (Table 2) (Cianciaruso et al., 2019; Himbert et al., 2020). For example, after collecting 426 human samples, including tissue explants, plasma, and other bodily fluids, the proteomic profile of EVs was conducted to develop improved biopsy method for the detection of cancers. Interestingly, the comparison of Ti-EVs identified proteins that distinguish tumors from normal tissues with a sensitivity of 90% and a specificity of 94%, suggesting that Ti-EVs are reliable biomarkers for the examination of cancer and determination of cancer type (Hoshino et al., 2020).
Malignant melanoma is the most aggressive and life-threatening skin cancer which originates from melanocytes (Cheng et al., 2021). In melanoma, EVs isolated from tumor tissues were found different from the classical cell line-derived EVs. These Ti-EVs have more abundant mitochondrial membrane proteins, and can be applied as potential predictors for the occurrence of melanoma (Jang et al., 2019). Zhang et al. (2018) demonstrated that tumor tissue-derived EVs from gastric cancer could induce higher expression of autophagy related genes ATG7 and BECN1, as well as the activation of NF-κB signaling pathway in neutrophils than EVs derived from non-cancerous tissues. Furthermore, neutrophils treated with EVs isolated from gastric cancer tissues promoted the migration of gastric cancer cells more effectively. Pancreatic ductal adenocarcinoma (PDAC) is a malignant type of pancreatic cancer (Siegel et al., 2021). Because of the insufficient understanding about the occurrence of liver metastasis in PDAC, the options for the therapeutic treatment of PDAC liver metastasis are very limited (Garrido-Laguna and Hidalgo, 2015; Huang et al., 2017). Nevertheless, the EVs derived from PDAC have been confirmed to mediate the crosstalk between the primary PDAC cells and hepatic satellite cells (HSCs). PDAC-EVs carrying CD44v6/C1QBP are essential for maintaining the hepatic fibrosis microenvironment and the liver metastasis of PDAC, indicating that EVs-CD44v6/C1QBP are potential biomarker for the prediction of the prognosis and liver metastasis of PDAC patients (Liang et al., 2021). Muscle-invasive urothelial bladder cancer (UBC) is a malignancy characterized by poor prognosis and high morbidity (Chamie et al., 2013). In order to explore new appropriate biomarkers for the occurrence and development of UBC, the Ti-EVs were extracted from UBC tumor tissues and matched distant tissues for further proteomics analysis. The results showed that 69 most abundant proteins profiled in tissue-derived EVs, regardless of the sites close to or away from the original tumor, were closely associated with the cancer metabolic pathways and poor prognosis (Eldh et al., 2021). These findings confirm the release of malignant EVs in UBC even though the pathologically undetectable tumor type, thus emphasizing the necessity of early radical cystectomy for UBC patients. At present, suitable diagnostic biomarkers for clear cell renal carcinoma (ccRCC) are still inadequate (Wang et al., 2018). To identify appropriate tumor-specific exosomal markers in ccRCC, the expression of CD147 (a pan-cancer-specific protein), CD70 and CA9 (the well characterized proteins in ccRCC) (Jilaveanu et al., 2012; Li et al., 2017; Baniak et al., 2020; Peng et al., 2020) in EVs were examined. Highly expressed CA9, CD70, and CD147 were found in Ti-EVs from tumor tissues compared to that in normal tissues. Therefore, CA9, CD70, and CD147 might function as potential biomarkers for the identification of tumor-specific EVs in ccRCC (Himbert et al., 2020).
Tumor metastasis is a very challenging problem. Recent evidence suggests that there is a complex communication mechanism between primary tumors and metastases, whereas primary tumors can influence the microenvironment of distant organs to induce metastasis (Kaplan et al., 2005; Hara et al., 2017; Ji et al., 2020). The microenvironment modified by primary tumors is referred as the metastatic niche which is characterized by vascular permeability, extracellular matrix remodeling, bone marrow-derived cells recruitment, angiogenesis, and immunosuppression (Dong et al., 2021; Garcia-Silva et al., 2021). EVs secreted by primary tumors are pivotal mediators during the formation of metastatic niche by delivering agents with tumor characteristics to the recipient cells in distant organs (Li et al., 2019a; Lee et al., 2019; Mashouri et al., 2019). For example, the EVs derived from ovarian primary tumor directly promote circulating tumor cells homing, colonization, and outgrowth within the metastatic niche but inhibit the antitumor immune response of host mircoenvironmentt (Feng et al., 2019). In salivary adenoid cystic carcinoma, primary tumor derived EVs activate lung fibroblast and induce the formation of lung metastatic niche which subsequently accelerate the lung metastasis (Kong et al., 2019). Moreover, it was found that EVs derived from highly metastatic murine breast cancer were primarily absorbed by the lungs of mice, and the T-cell proliferation and NK cell cytotoxicity were inhibited obviously. This may explain why the microenvironment in distant organs are always immunosuppressive (Wen et al., 2016; Patel et al., 2018). In summary, the Ti-EVs derived from tumors are tightly involved in the progress and metastasis of cancers, and they are potential targets for developing new diagnostic and therapeutic methods for tumors.
Neurodegenerative disorders are a group of central nervous system (CNS) diseases related to brain function degeneration, including Alzheimer’s Disease (AD), Parkinson’s Disease (PD), Amyotrophic Lateral Sclerosis (ALS) and Huntington’s Disease (Gao et al., 2021). Alzheimer’s disease (AD) is the most common neurodegenerative disorder, which is characterized by the amyloid plaques and the intracellular accumulation of neurofibrillary tangles (NFTs) in brain tissue (Goedert et al., 1988; Duyckaerts et al., 2009). The role of EVs in AD has been widely studied, however, the EVs from brain tissues have not been well explored (Hill, 2019; Nogueras-Ortiz et al., 2020). Tau and Aβ oligomers are the most AD related pathogenic proteins which have also been detected in brain tissue derived EVs (BDEVs), indicating that BDEVs might participate in the AD pathogenesis (Guo et al., 2016; DeLeo and Ikezu, 2018). With the deepening of research, a quantitative proteomic analysis of the BDEVs isolated from AD patients and control ones was conducted. The proteomic analysis elucidated that ANXA5, GPM6A, VGF, and ACTZ could distinguish AD EVs from controls with high accuracy, thus providing novel biomarkers for AD (Muraoka et al., 2020c). Moreover, by isolating BDEVs from the frontal cortex of AD patients and control subjects, Cheng et al. (2020) found that BDEVs derived from AD patients contain more disease-associated miRNAs. Although there are few studies about the interplay of RNAs in BDEVs, the above findings will be beneficial for clarifying the early pathological changes of AD. Amyotrophic lateral sclerosis (ALS) is a neurodegenerative disease caused by the deposition of ubiquitinated and aggregated proteins in lewy body-like hyaline, or skein-like inclusions in the upper and lower motor neurons cytoplasm (Taylor et al., 2002; Arai et al., 2006). In order to explore the potential roles of Ti-EVs in ALS, the motor cortex derived EVs (MCEVs) were isolated from the brain tissues of ALS patients and neurological controls to perform proteomic analysis. As the result of proteomic analysis shown, 16 proteins (including several stress granule dynamics related proteins, such as STAU1 and DHX30) were found to be differentially expressed in ALS MCEVs compared to that of controls, suggesting that these MCEVs are promising targets for treating ALS. Moreover, the finding of these proteins in MCEVs reveals that ALS related stress particles might be tightly involved in the package of MCEVs thereby highlighting the indispensability of EVs in the pathogenesis of ALS (Vassileff et al., 2020). Parkinson’s Disease (PD) is an important member of neurodegeneration, and some studies have elucidated the role of EVs in PD (Leggio et al., 2021; Huang et al., 2022). However, the research on brain derived EVs in PD is still insufficient, and more in-depth research is necessary. In addition, further studies are needed to detect these brain tissue-EVs relevant proteins and RNAs in EVs from CSF and blood, so as to evaluate their potential value as diagnostic markers.
In acute myocardial infarction (AMI), the reperfusion can induce irreversible myocardial injury, which is also known as myocardial ischemia-reperfusion (IR) injury (Hausenloy and Yellon, 2013). To date, the underlying mechanism of IR injury remains unclear. As reported, the cardiac IR induced EVs could accelerate IR injury. A novel IR-EVs-miR-155-5p-M1 polarization pathway was found in heart tissue during the progression of IR, shedding lights on the therapeutic potential of cardiac Ti-EVs for the treatment of IR injury (Ge et al., 2021). By establishing mice IR model, Zhou et al. extracted Ti-EVs from the IR heart tissues, and illustrated a specific profile of circRNAs in Ti-EVs, providing evidences about the role of Ti-EVs in the development of cardiac IR (Ge et al., 2019). When the acute myocardial infarction occurs, a number of inflammatory cells will be rapidly recruited to the ischemic areas, leading to the massive release of cytokines, soluble chemokines, and growth factors (Silvestre et al., 2013). By comparing the Ti-EVs isolated from the heart tissues of coronary artery ligation and sham mice. It was found that AMI increased the release of cardiac EVs which were immediately absorbed by infiltrating monocytes and then to modulate the local inflammatory responses (Loyer et al., 2018). These findings indicated that the local Ti-EVs formation in the infarcted heart are closely related to the inflammation after myocardial infarction.
Adipose tissue (AT) has been regarded as an endocrine organ, which can secrete adipokines, such as proinflammatory cytokines, anti-inflammatory cytokines and metabolism regulatory cytokines (Cui et al., 2017; Packer, 2018; Wei et al., 2020). Adipose tissue can release EVs and these EVs are considered to be the main source of circulating EVs (Wei et al., 2020). In adipose tissue-secreted EVs (AT-EVs), abundant miRNAs have been detected, suggesting that AT-EVs may convey intercellular information and signals (Thomou et al., 2017; Lee et al., 2021). As reported before, the obese mice could release enriched AT-EVs (derived from perivascular adipose tissues, PVAT) which packed miR-221-3p. The receipt of these EVs in lean mice aroused the response of inflammation in PVAT and vascular phenotypic switching in abdominal aorta, highlighting the importance of AT-EVs in cellular crosstalk to participate obesity-associated inflammation (Li et al., 2019b). Adipose tissue-derived stem cells (ADSCs) can internalize the EVs derived from adipose tissues and induced adipogenesis. Mechanically, AT-EVs reduced the expression of adipogenesis related protein WISP2 in ADSCs by transferring miR-450a-5p to them (Zhang et al., 2017). Brown adipose tissue (BAT) is a major kind of adipose tissue. Interestingly, the sEVs secreted by BAT are involved in exercise cardioprotection via delivering the cardioprotective miRNAs into the heart. This result showed the BAT-sEVs are critical mediator in the interaction between BAT and cardiomyocyte (Zhao et al., 2022). Besides, AT-EVs obtained from gestational diabetes mellitus (GDM) and normal glucose tolerant (NGT) have been confirmed to have differently expressed proteins, which may contribute to fetal overgrowth in GDM (Jayabalan et al., 2019).
The stability and severity of the plaque is closely related to the crosstalk between itself and the microenvironment. Electron microscopic analysis of ultrathin sections has verified the presence of EVs in human atherosclerotic plaque, indicating that lesional smooth muscle cells (SMCs) and endothelial cells within the plaque are able to release EVs into the extracellular space. This finding provides a novel aspect of intercellular communication in plaque environment (Perrotta and Aquila, 2016). In another study about metabolic syndrome (MetS), EVs isolated from the atherosclerotic plaques of mouse and human both contained abundant Rap1, which is crucial for the inflammatory response of vascular (Metrich et al., 2010). Researchers investigated the excessive production of Ti-EVs enriched in Rap1 could promote the development of atherosclerosis in MetS by inducing the remodel and inflammation of vascular (Perdomo et al., 2020). Nicotine is the cigarette smoke’s main component which could directly stimulate plaque cell migration and proliferation as well as the communication of cytokines between macrophages and VSMCs via EVs, thus accelerating atherogenesis (Liu et al., 2017; Ren et al., 2018). Furthermore, nicotine could facilitate atherosclerotic lesion progression and cause plaque derived EVs to remain in vivo, which might mediate the migration and proliferation of VSMC (Zhu et al., 2019).
In the inner ear, cochlea is a vital organ that responsible for the auditory signal transduction. Cochlea develops during the period of embryonic day 9 to postnatal day 21 (Wright et al., 2003; Burns et al., 2012). Both the detection of sound waves and the transmission of sound information to the brain are relied on cochlear hair cells (HCs) (LeMasurier and Gillespie, 2005). In spite of the widely research about EVs in cancer and some other diseases, there are few studies to reveal the role of EVs in cochlea. Lately, (Jiang et al. (2022) has isolated cochlear tissue-derived EVs from mice of different ages to analyze and characterize the protein and miRNA contents of EVs. More than five hundred miRNAs and five thousand proteins have been detected in the EVs derived from cochlear. Among them, about two hundred miRNAs and three thousand proteins are expressed differentially at different periods, which are probably involved in the maturation of HCs. These findings authenticated that EVs are present in the cochlea tissues and important for the development of auditory system. Meanwhile, these EVs-miRNAs and proteins are promising novel targets for studying the mechanism of cochlear development.
Compared with EVs isolated from cell culture supernatant and body fluids, Ti-EVs may play a more crucial role in different sorts of diseases. Due to Ti-EVs are located in the tissue microenvironment, they have abundant cell crosstalk information and can authentically reflect the intercommunication between cells within tissue microenvironment. Moreover, the contents of Ti-EVs are relative pure because of its single tissue source. Based on these advantages, Ti-EVs have attracted increasing attention and have been studied a lot in basic research and clinical applications. Although some progress has been achieved, there are still some challenges and limitations needed to overcome. Firstly, Ti-EVs isolated by existing methods are often contaminated by EVs released from other broken cells. Therefore, it is urgent to develop optimal techniques to isolate and purify Ti-EVs. Furthermore, the tissue microenvironment contains diverse cell types, to elucidate the accurate original cell type of Ti-EVs will provide deep understanding for the intercellular communication within microenvironment. To further investigate the chemical, physical, and biological characteristics of Ti-EVs may help us distinguish Ti-EVs from “impurities”. Secondly, some researches have demonstrated the potential of Ti-EVs to be diagnostic biomarkers, however, the isolation methods are too complex to apply in clinical practices and the exploration of Ti-EVs in the pathophysiological process of various diseases are still in the initial stage. How to balance the purity and convenience of Ti-EVs extraction is a major challenge in the future. Thirdly, considering the spatiotemporal specificity of tissue development, to illustrate the spatiotemporal profiles of Ti-EVs in all kinds of tissues will help us understand the development process deeply.
Although the widely clinical application of EVs is still difficult because of the problems in EVs preparation, transmission, targeting and retention, some achievements have been made. A variety of studies have shown diverse biomaterials for delivering EVs efficiently, including implantable scaffolds (Jiang et al., 2021b), injectable hydrogels (Wong et al., 2020), and chemical crosslinking (Heirani-Tabasi et al., 2021). Modifying EVs to meet different needs is another strategy. These engineered EVs can carry cell targeted ligands and a high level of specific agents, which are expected to improve their therapeutic effects, especially in multiple tumors (Zhan et al., 2020; Zhao et al., 2020; Wong et al., 2021). In addition, to insert imaging molecules in engineered EVs is an appropriate method for determining the metabolism and distribution of EVs in vivo (Luan et al., 2017; Liang et al., 2021).
Without a doubt, a deeper comprehension of Ti-EVs biology and the development of standardized techniques for Ti-EVs quantification, extraction and storage, molecular characterization, and potency assays will greatly advance the prospects of Ti-EVs-based diagnostic and therapeutic applications in the future. Challenges do exist at present, but with the improvement of technology and development of research, these difficulties will eventually be overcome. The clinical application of Ti-EVs will gradually come true.
WT proposed and designed the study. ZZ and QS wrote the draft. WT and ZZ collected the data. All authors contributed to the design and interpretation of the study and to further drafts. ZZ and QS revised the manuscript. All authors read and approved the final manuscript.
The authors declare that the research was conducted in the absence of any commercial or financial relationships that could be construed as a potential conflict of interest.
All claims expressed in this article are solely those of the authors and do not necessarily represent those of their affiliated organizations, or those of the publisher, the editors and the reviewers. Any product that may be evaluated in this article, or claim that may be made by its manufacturer, is not guaranteed or endorsed by the publisher.
Allen, M., Bjerke, M., Edlund, H., Nelander, S., and Westermark, B. (2016). Origin of the U87MG glioma cell line: Good news and bad news. Sci. Transl. Med. 8, 354re3. doi:10.1126/scitranslmed.aaf6853
Andaloussi, S. E., Mager, I., Breakefield, X. O., and Wood, M. J. (2013). Extracellular vesicles: Biology and emerging therapeutic opportunities. Nat. Rev. Drug Discov. 12, 347–357. doi:10.1038/nrd3978
Arai, T., Hasegawa, M., Akiyama, H., Ikeda, K., Nonaka, T., Mori, H., et al. (2006). TDP-43 is a component of ubiquitin-positive tau-negative inclusions in frontotemporal lobar degeneration and amyotrophic lateral sclerosis. Biochem. Biophys. Res. Commun. 351, 602–611. doi:10.1016/j.bbrc.2006.10.093
Asai, H., Ikezu, S., Tsunoda, S., Medalla, M., Luebke, J., Haydar, T., et al. (2015). Depletion of microglia and inhibition of exosome synthesis halt tau propagation. Nat. Neurosci. 18, 1584–1593. doi:10.1038/nn.4132
Baniak, N., Flood, T. A., Buchanan, M., Dal Cin, P., and Hirsch, M. S. (2020). Carbonic anhydrase IX (CA9) expression in multiple renal epithelial tumour subtypes. Histopathology 77, 659–666. doi:10.1111/his.14204
Becker, A., Thakur, B. K., Weiss, J. M., Kim, H. S., Peinado, H., and Lyden, D. (2016). Extracellular vesicles in cancer: Cell-to-Cell mediators of metastasis. Cancer Cell 30, 836–848. doi:10.1016/j.ccell.2016.10.009
Breglio, A. M., May, L. A., Barzik, M., Welsh, N. C., Francis, S. P., Costain, T. Q., et al. (2020). Exosomes mediate sensory hair cell protection in the inner ear. J. Clin. Invest. 130, 2657–2672. doi:10.1172/JCI128867
Burns, J. C., Cox, B. C., Thiede, B. R., Zuo, J., and Corwin, J. T. (2012). In vivo proliferative regeneration of balance hair cells in newborn mice. J. Neurosci. 32, 6570–6577. doi:10.1523/JNEUROSCI.6274-11.2012
Camino, T., Lago-Baameiro, N., Martis-Sueiro, A., Couto, I., Santos, F., Baltar, J., et al. (2020). Vesicles shed by pathological murine adipocytes spread pathology: Characterization and functional role of insulin resistant/hypertrophied adiposomes. Int. J. Mol. Sci. 21, 2252. doi:10.3390/ijms21062252
Chamie, K., Litwin, M. S., Bassett, J. C., Daskivich, T. J., Lai, J., Hanley, J. M., et al. (2013). Recurrence of high-risk bladder cancer: A population-based analysis. Cancer 119, 3219–3227. doi:10.1002/cncr.28147
Chang, W., Li, M., Song, L., Miao, S., Yu, W., and Wang, J. (2022). Noncoding RNAs from tissue-derived small extracellular vesicles: Roles in diabetes and diabetic complications. Mol. Metab. 58, 101453. doi:10.1016/j.molmet.2022.101453
Cheng, L., Vella, L. J., Barnham, K. J., McLean, C., Masters, C. L., and Hill, A. F. (2020). Small RNA fingerprinting of Alzheimer's disease frontal cortex extracellular vesicles and their comparison with peripheral extracellular vesicles. J. Extracell. Vesicles 9, 1766822. doi:10.1080/20013078.2020.1766822
Cheng, Y. C., Chang, Y. A., Chen, Y. J., Sung, H. M., Bogeski, I., Su, H. L., et al. (2021). The roles of extracellular vesicles in malignant melanoma. Cells 10, 2740. doi:10.3390/cells10102740
Cianciaruso, C., Beltraminelli, T., Duval, F., Nassiri, S., Hamelin, R., Mozes, A., et al. (2019). Molecular profiling and functional analysis of macrophage-derived tumor extracellular vesicles. Cell Rep. 27, 3062–3080. doi:10.1016/j.celrep.2019.05.008
Crescitelli, R., Lasser, C., Jang, S. C., Cvjetkovic, A., Malmhall, C., Karimi, N., et al. (2020). Subpopulations of extracellular vesicles from human metastatic melanoma tissue identified by quantitative proteomics after optimized isolation. J. Extracell. Vesicles 9, 1722433. doi:10.1080/20013078.2020.1722433
Crescitelli, R., Lasser, C., and Lotvall, J. (2021). Isolation and characterization of extracellular vesicle subpopulations from tissues. Nat. Protoc. 16, 1548–1580. doi:10.1038/s41596-020-00466-1
Crescitelli, R., Lasser, C., Szabo, T. G., Kittel, A., Eldh, M., Dianzani, I., et al. (2013). Distinct RNA profiles in subpopulations of extracellular vesicles: Apoptotic bodies, microvesicles and exosomes. J. Extracell. Vesicles 2, 20677. doi:10.3402/jev.v2i0.20677
Cui, H., Lopez, M., and Rahmouni, K. (2017). The cellular and molecular bases of leptin and ghrelin resistance in obesity. Nat. Rev. Endocrinol. 13, 338–351. doi:10.1038/nrendo.2016.222
De Wever, O., and Hendrix, A. (2019). A supporting ecosystem to mature extracellular vesicles into clinical application. EMBO J. 38, e101412. doi:10.15252/embj.2018101412
DeLeo, A. M., and Ikezu, T. (2018). Extracellular vesicle biology in alzheimer's disease and related tauopathy. J. Neuroimmune Pharmacol. 13, 292–308. doi:10.1007/s11481-017-9768-z
Domcke, S., Sinha, R., Levine, D. A., Sander, C., and Schultz, N. (2013). Evaluating cell lines as tumour models by comparison of genomic profiles. Nat. Commun. 4, 2126. doi:10.1038/ncomms3126
Dong, Q., Liu, X., Cheng, K., Sheng, J., Kong, J., and Liu, T. (2021). Pre-metastatic niche formation in different organs induced by tumor extracellular vesicles. Front. Cell Dev. Biol. 9, 733627. doi:10.3389/fcell.2021.733627
Duyckaerts, C., Delatour, B., and Potier, M. C. (2009). Classification and basic pathology of Alzheimer disease. Acta Neuropathol. 118, 5–36. doi:10.1007/s00401-009-0532-1
Eldh, M., Mints, M., Hiltbrunner, S., Ladjevardi, S., Alamdari, F., Johansson, M., et al. (2021). Proteomic profiling of tissue exosomes indicates continuous release of malignant exosomes in urinary bladder cancer patients, even with pathologically undetectable tumour. Cancers 13, 3242. doi:10.3390/cancers13133242
Feng, W., Dean, D. C., Hornicek, F. J., Shi, H., and Duan, Z. (2019). Exosomes promote pre-metastatic niche formation in ovarian cancer. Mol. Cancer 18, 124. doi:10.1186/s12943-019-1049-4
Fringuello, A., Tatman, P. D., Wroblewski, T., Thompson, J. A., Yu, X., Lillehei, K. O., et al. (2021). Cytokine-laden extracellular vesicles predict patient prognosis after cerebrovascular accident. Int. J. Mol. Sci. 22, 7847. doi:10.3390/ijms22157847
Gallart-Palau, X., Serra, A., and Sze, S. K. (2016). Enrichment of extracellular vesicles from tissues of the central nervous system by PROSPR. Mol. Neurodegener. 11, 41. doi:10.1186/s13024-016-0108-1
Gao, P., Li, X., Du, X., Liu, S., and Xu, Y. (2021). Diagnostic and therapeutic potential of exosomes in neurodegenerative diseases. Front. Aging Neurosci. 13, 790863. doi:10.3389/fnagi.2021.790863
Garcia-Silva, S., Benito-Martin, A., Nogues, L., Hernandez-Barranco, A., Mazariegos, M. S., Santos, V., et al. (2021). Melanoma-derived small extracellular vesicles induce lymphangiogenesis and metastasis through an NGFR-dependent mechanism. Nat. Cancer 2, 1387–1405. doi:10.1038/s43018-021-00272-y
Gardiner, C., Di Vizio, D., Sahoo, S., Thery, C., Witwer, K. W., Wauben, M., et al. (2016). Techniques used for the isolation and characterization of extracellular vesicles: Results of a worldwide survey. J. Extracell. Vesicles 5, 32945. doi:10.3402/jev.v5.32945
Garrido-Laguna, I., and Hidalgo, M. (2015). Pancreatic cancer: From state-of-the-art treatments to promising novel therapies. Nat. Rev. Clin. Oncol. 12, 319–334. doi:10.1038/nrclinonc.2015.53
Ge, X., Meng, Q., Wei, L., Liu, J., Li, M., Liang, X., et al. (2021). Myocardial ischemia-reperfusion induced cardiac extracellular vesicles harbour proinflammatory features and aggravate heart injury. J. Extracell. Vesicles 10, e12072. doi:10.1002/jev2.12072
Ge, X., Meng, Q., Zhuang, R., Yuan, D., Liu, J., Lin, F., et al. (2019). Circular RNA expression alterations in extracellular vesicles isolated from murine heart post ischemia/reperfusion injury. Int. J. Cardiol. 296, 136–140. doi:10.1016/j.ijcard.2019.08.024
Goedert, M., Wischik, C. M., Crowther, R. A., Walker, J. E., and Klug, A. (1988). Cloning and sequencing of the cDNA encoding a core protein of the paired helical filament of alzheimer disease: Identification as the microtubule-associated protein tau. Proc. Natl. Acad. Sci. U. S. A. 85, 4051–4055. doi:10.1073/pnas.85.11.4051
Grant, R., Ansa-Addo, E., Stratton, D., Antwi-Baffour, S., Jorfi, S., Kholia, S., et al. (2011). A filtration-based protocol to isolate human plasma membrane-derived vesicles and exosomes from blood plasma. J. Immunol. Methods 371, 143–151. doi:10.1016/j.jim.2011.06.024
Guo, B. B., Bellingham, S. A., and Hill, A. F. (2016). Stimulating the release of exosomes increases the intercellular transfer of prions. J. Biol. Chem. 291, 5128–5137. doi:10.1074/jbc.M115.684258
Gupta, D., Zickler, A. M., and El Andaloussi, S. (2021). Dosing extracellular vesicles. Adv. Drug Deliv. Rev. 178, 113961. doi:10.1016/j.addr.2021.113961
Gurunathan, S., Kang, M. H., Jeyaraj, M., Qasim, M., and Kim, J. H. (2019). Review of the isolation, characterization, biological function, and multifarious therapeutic approaches of exosomes. Cells 8, 307. doi:10.3390/cells8040307
Ha, D., Yang, N., and Nadithe, V. (2016). Exosomes as therapeutic drug carriers and delivery vehicles across biological membranes: Current perspectives and future challenges. Acta Pharm. Sin. B 6, 287–296. doi:10.1016/j.apsb.2016.02.001
Hara, T., Nakaoka, H. J., Hayashi, T., Mimura, K., Hoshino, D., Inoue, M., et al. (2017). Control of metastatic niche formation by targeting APBA3/Mint3 in inflammatory monocytes. Proc. Natl. Acad. Sci. U. S. A. 114, E4416–E4424. doi:10.1073/pnas.1703171114
Hausenloy, D. J., and Yellon, D. M. (2013). Myocardial ischemia-reperfusion injury: A neglected therapeutic target. J. Clin. Invest. 123, 92–100. doi:10.1172/JCI62874
He, S., Huang, L., Shao, C., Nie, T., Xia, L., Cui, B., et al. (2021). Several miRNAs derived from serum extracellular vesicles are potential biomarkers for early diagnosis and progression of Parkinson's disease. Transl. Neurodegener. 10, 25. doi:10.1186/s40035-021-00249-y
Heirani-Tabasi, A., Hosseinzadeh, S., Rabbani, S., Ahmadi Tafti, S. H., Jamshidi, K., Soufizomorrod, M., et al. (2021). Cartilage tissue engineering by co-transplantation of chondrocyte extracellular vesicles and mesenchymal stem cells, entrapped in chitosan-hyaluronic acid hydrogel. Biomed. Mat. 16, 055003. doi:10.1088/1748-605X/ac0cbf
Hill, A. F. (2019). Extracellular vesicles and neurodegenerative diseases. J. Neurosci. 39, 9269–9273. doi:10.1523/JNEUROSCI.0147-18.2019
Himbert, D., Zeuschner, P., Ayoubian, H., Heinzelmann, J., Stockle, M., and Junker, K. (2020). Characterization of CD147, CA9, and CD70 as tumor-specific markers on extracellular vesicles in clear cell renal cell carcinoma. Diagn. (Basel) 10, 1034. doi:10.3390/diagnostics10121034
Hoshino, A., Kim, H. S., Bojmar, L., Gyan, K. E., Cioffi, M., Hernandez, J., et al. (2020). Extracellular vesicle and particle biomarkers define multiple human cancers. Cell 182, 1044–1061. doi:10.1016/j.cell.2020.07.009
Huang, C., Li, N., Li, Z., Chang, A., Chen, Y., Zhao, T., et al. (2017). Tumour-derived Interleukin 35 promotes pancreatic ductal adenocarcinoma cell extravasation and metastasis by inducing ICAM1 expression. Nat. Commun. 8, 14035. doi:10.1038/ncomms14035
Huang, Y., Cheng, L., Turchinovich, A., Mahairaki, V., Troncoso, J. C., Pletnikova, O., et al. (2020). Influence of species and processing parameters on recovery and content of brain tissue-derived extracellular vesicles. J. Extracell. Vesicles 9, 1785746. doi:10.1080/20013078.2020.1785746
Huang, Y., Liu, Z., Li, N., Tian, C., Yang, H., Huo, Y., et al. (2022). Parkinson's disease derived exosomes aggravate neuropathology in SNCA*A53T mice. Ann. Neurol. 92, 230–245. doi:10.1002/ana.26421
Huang, Z., and Xu, A. (2021). Adipose extracellular vesicles in intercellular and inter-organ crosstalk in metabolic health and diseases. Front. Immunol. 12, 608680. doi:10.3389/fimmu.2021.608680
Jang, S. C., Crescitelli, R., Cvjetkovic, A., Belgrano, V., Olofsson Bagge, R., Sundfeldt, K., et al. (2019). Mitochondrial protein enriched extracellular vesicles discovered in human melanoma tissues can be detected in patient plasma. J. Extracell. Vesicles 8, 1635420. doi:10.1080/20013078.2019.1635420
Jayabalan, N., Lai, A., Ormazabal, V., Adam, S., Guanzon, D., Palma, C., et al. (2019). Adipose tissue exosomal proteomic profile reveals a role on placenta glucose metabolism in gestational diabetes mellitus. J. Clin. Endocrinol. Metab. 104, 1735–1752. doi:10.1210/jc.2018-01599
Jeppesen, D. K., Fenix, A. M., Franklin, J. L., Higginbotham, J. N., Zhang, Q., Zimmerman, L. J., et al. (2019). Reassessment of exosome composition. Cell 177, 428–445. doi:10.1016/j.cell.2019.02.029
Ji, Q., Zhou, L., Sui, H., Yang, L., Wu, X., Song, Q., et al. (2020). Primary tumors release ITGBL1-rich extracellular vesicles to promote distal metastatic tumor growth through fibroblast-niche formation. Nat. Commun. 11, 1211. doi:10.1038/s41467-020-14869-x
Jiang, H., Qian, Y., Shen, Z., Liu, Y., He, Y., Gao, R., et al. (2021). Circulating microRNA135a3p in serum extracellular vesicles as a potential biological marker of nonalcoholic fatty liver disease. Mol. Med. Rep. 24, 498. doi:10.3892/mmr.2021.12137
Jiang, P., Ma, X., Han, S., Ma, L., Ai, J., Wu, L., et al. (2022). Characterization of the microRNA transcriptomes and proteomics of cochlear tissue-derived small extracellular vesicles from mice of different ages after birth. Cell. Mol. Life Sci. 79, 154. doi:10.1007/s00018-022-04164-x
Jiang, S., Tian, G., Yang, Z., Gao, X., Wang, F., Li, J., et al. (2021). Enhancement of acellular cartilage matrix scaffold by Wharton's jelly mesenchymal stem cell-derived exosomes to promote osteochondral regeneration. Bioact. Mat. 6, 2711–2728. doi:10.1016/j.bioactmat.2021.01.031
Jilaveanu, L. B., Sznol, J., Aziz, S. A., Duchen, D., Kluger, H. M., and Camp, R. L. (2012). CD70 expression patterns in renal cell carcinoma. Hum. Pathol. 43, 1394–1399. doi:10.1016/j.humpath.2011.10.014
Kaplan, R. N., Riba, R. D., Zacharoulis, S., Bramley, A. H., Vincent, L., Costa, C., et al. (2005). VEGFR1-positive haematopoietic bone marrow progenitors initiate the pre-metastatic niche. Nature 438, 820–827. doi:10.1038/nature04186
Keller, S., Ridinger, J., Rupp, A. K., Janssen, J. W., and Altevogt, P. (2011). Body fluid derived exosomes as a novel template for clinical diagnostics. J. Transl. Med. 9, 86. doi:10.1186/1479-5876-9-86
Kong, J., Tian, H., Zhang, F., Zhang, Z., Li, J., Liu, X., et al. (2019). Extracellular vesicles of carcinoma-associated fibroblasts creates a pre-metastatic niche in the lung through activating fibroblasts. Mol. Cancer 18, 175. doi:10.1186/s12943-019-1101-4
Konoshenko, M. Y., Lekchnov, E. A., Vlassov, A. V., and Laktionov, P. P. (2018). Isolation of extracellular vesicles: General methodologies and latest trends. Biomed. Res. Int. 2018, 8545347. doi:10.1155/2018/8545347
Lai, H., Li, Y., Zhang, H., Hu, J., Liao, J., Su, Y., et al. (2022). exoRBase 2.0: an atlas of mRNA, lncRNA and circRNA in extracellular vesicles from human biofluids. Nucleic Acids Res. 50, D118–D128. doi:10.1093/nar/gkab1085
Lasser, C., Alikhani, V. S., Ekstrom, K., Eldh, M., Paredes, P. T., Bossios, A., et al. (2011). Human saliva, plasma and breast milk exosomes contain RNA: Uptake by macrophages. J. Transl. Med. 9, 9. doi:10.1186/1479-5876-9-9
Lasser, C., Kishino, Y., Park, K. S., Shelke, G. V., Karimi, N., Suzuki, S., et al. (2021). Immune-associated proteins are enriched in lung tissue-derived extracellular vesicles during allergen-induced eosinophilic airway inflammation. Int. J. Mol. Sci. 22, 4718. doi:10.3390/ijms22094718
Lee, J. W., Stone, M. L., Porrett, P. M., Thomas, S. K., Komar, C. A., Li, J. H., et al. (2019). Hepatocytes direct the formation of a pro-metastatic niche in the liver. Nature 567, 249–252. doi:10.1038/s41586-019-1004-y
Lee, K. S., Lee, J., Kim, H. K., Yeom, S. H., Woo, C. H., Jung, Y. J., et al. (2021). Extracellular vesicles from adipose tissue-derived stem cells alleviate osteoporosis through osteoprotegerin and miR-21-5p. J. Extracell. Vesicles 10, e12152. doi:10.1002/jev2.12152
Leggio, L., Paterno, G., Vivarelli, S., Falzone, G. G., Giachino, C., Marchetti, B., et al. (2021). Extracellular vesicles as novel diagnostic and prognostic biomarkers for Parkinson's disease. Aging Dis. 12, 1494–1515. doi:10.14336/AD.2021.0527
LeMasurier, M., and Gillespie, P. G. (2005). Hair-cell mechanotransduction and cochlear amplification. Neuron 48, 403–415. doi:10.1016/j.neuron.2005.10.017
Li, H., Xi, Z., Dai, X., Wu, W., Li, Y., Liu, Y., et al. (2017). CD147 and glioma: A meta-analysis. J. Neurooncol. 134, 145–156. doi:10.1007/s11060-017-2499-4
Li, K., Chen, Y., Li, A., Tan, C., and Liu, X. (2019). Exosomes play roles in sequential processes of tumor metastasis. Int. J. Cancer 144, 1486–1495. doi:10.1002/ijc.31774
Li, S. R., Man, Q. W., Gao, X., Lin, H., Wang, J., Su, F. C., et al. (2021). Tissue-derived extracellular vesicles in cancers and non-cancer diseases: Present and future. J. Extracell. Vesicles 10, e12175. doi:10.1002/jev2.12175
Li, X., Ballantyne, L. L., Yu, Y., and Funk, C. D. (2019). Perivascular adipose tissue-derived extracellular vesicle miR-221-3p mediates vascular remodeling. FASEB J. 33, 12704–12722. doi:10.1096/fj.201901548R
Liang, Y., Duan, L., Lu, J., and Xia, J. (2021). Engineering exosomes for targeted drug delivery. Theranostics 11, 3183–3195. doi:10.7150/thno.52570
Liga, A., Vliegenthart, A. D., Oosthuyzen, W., Dear, J. W., and Kersaudy-Kerhoas, M. (2015). Exosome isolation: A microfluidic road-map. Lab. Chip 15, 2388–2394. doi:10.1039/c5lc00240k
Liu, H., Yuan, W., Pang, Q., Xue, C., and Yan, X. (2022). Single-particle analysis of tear fluid reveals abundant presence of tissue factor-exposing extracellular vesicles with strong coagulation activity. Talanta 239, 123089. doi:10.1016/j.talanta.2021.123089
Liu, L., Wu, H., Cao, Q., Guo, Z., Ren, A., and Dai, Q. (2017). Stimulation of Alpha7 nicotinic acetylcholine receptor attenuates nicotine-induced upregulation of MMP, MCP-1, and RANTES through modulating ERK1/2/AP-1 signaling pathway in RAW264.7 and MOVAS cells. Mediat. Inflamm. 2017, 2401027. doi:10.1155/2017/2401027
Loyer, X., Zlatanova, I., Devue, C., Yin, M., Howangyin, K. Y., Klaihmon, P., et al. (2018). Intra-cardiac release of extracellular vesicles shapes inflammation following myocardial infarction. Circ. Res. 123, 100–106. doi:10.1161/CIRCRESAHA.117.311326
Luan, X., Sansanaphongpricha, K., Myers, I., Chen, H., Yuan, H., and Sun, D. (2017). Engineering exosomes as refined biological nanoplatforms for drug delivery. Acta Pharmacol. Sin. 38, 754–763. doi:10.1038/aps.2017.12
Lunavat, T. R., Cheng, L., Einarsdottir, B. O., Olofsson Bagge, R., Veppil Muralidharan, S., Sharples, R. A., et al. (2017). BRAF(V600) inhibition alters the microRNA cargo in the vesicular secretome of malignant melanoma cells. Proc. Natl. Acad. Sci. U. S. A. 114, E5930–E5939. doi:10.1073/pnas.1705206114
Marar, C., Starich, B., and Wirtz, D. (2021). Extracellular vesicles in immunomodulation and tumor progression. Nat. Immunol. 22, 560–570. doi:10.1038/s41590-021-00899-0
Mashouri, L., Yousefi, H., Aref, A. R., Ahadi, A. M., Molaei, F., and Alahari, S. K. (2019). Exosomes: Composition, biogenesis, and mechanisms in cancer metastasis and drug resistance. Mol. Cancer 18, 75. doi:10.1186/s12943-019-0991-5
Mateescu, B., Kowal, E. J., van Balkom, B. W., Bartel, S., Bhattacharyya, S. N., Buzas, E. I., et al. (2017). Obstacles and opportunities in the functional analysis of extracellular vesicle RNA - an ISEV position paper. J. Extracell. Vesicles 6, 1286095. doi:10.1080/20013078.2017.1286095
Metrich, M., Berthouze, M., Morel, E., Crozatier, B., Gomez, A. M., and Lezoualc'h, F. (2010). Role of the cAMP-binding protein Epac in cardiovascular physiology and pathophysiology. Pflugers Arch. 459, 535–546. doi:10.1007/s00424-009-0747-y
Mincheva-Nilsson, L., Baranov, V., Nagaeva, O., and Dehlin, E. (2016). Isolation and characterization of exosomes from cultures of tissue explants and cell lines. Curr. Protoc. Immunol. 115 (14 42 1), 1–14. doi:10.1002/cpim.17
Mulcahy, L. A., Pink, R. C., and Carter, D. R. (2014). Routes and mechanisms of extracellular vesicle uptake. J. Extracell. Vesicles 3, 24641. doi:10.3402/jev.v3.24641
Muraoka, S., DeLeo, A. M., Sethi, M. K., Yukawa-Takamatsu, K., Yang, Z., Ko, J., et al. (2020). Proteomic and biological profiling of extracellular vesicles from Alzheimer's disease human brain tissues. Alzheimers Dement. 16, 896–907. doi:10.1002/alz.12089
Muraoka, S., Jedrychowski, M. P., Yanamandra, K., Ikezu, S., Gygi, S. P., and Ikezu, T. (2020). Proteomic profiling of extracellular vesicles derived from cerebrospinal fluid of alzheimer's disease patients: A pilot study. Cells 9, 1959. doi:10.3390/cells9091959
Muraoka, S., Lin, W., Chen, M., Hersh, S. W., Emili, A., Xia, W., et al. (2020). Assessment of separation methods for extracellular vesicles from human and mouse brain tissues and human cerebrospinal fluids. Methods 177, 35–49. doi:10.1016/j.ymeth.2020.02.002
Namee, N. M., and O'Driscoll, L. (2018). Extracellular vesicles and anti-cancer drug resistance. Biochim. Biophys. Acta. Rev. Cancer 1870, 123–136. doi:10.1016/j.bbcan.2018.07.003
Nielsen, M. R., Frederiksen-Moller, B., Zachar, R., Jorgensen, J. S., Hansen, M. R., Ydegaard, R., et al. (2017). Urine exosomes from healthy and hypertensive pregnancies display elevated level of alpha-subunit and cleaved alpha- and gamma-subunits of the epithelial sodium channel-ENaC. Pflugers Arch. 469, 1107–1119. doi:10.1007/s00424-017-1977-z
Nogueras-Ortiz, C. J., Mahairaki, V., Delgado-Peraza, F., Das, D., Avgerinos, K., Eren, E., et al. (2020). Astrocyte- and neuron-derived extracellular vesicles from alzheimer's disease patients effect complement-mediated neurotoxicity. Cells 9, 1618. doi:10.3390/cells9071618
Packer, M. (2018). Epicardial adipose tissue may mediate deleterious effects of obesity and inflammation on the myocardium. J. Am. Coll. Cardiol. 71, 2360–2372. doi:10.1016/j.jacc.2018.03.509
Park, Y. H., Shin, H. W., Jung, A. R., Kwon, O. S., Choi, Y. J., Park, J., et al. (2016). Prostate-specific extracellular vesicles as a novel biomarker in human prostate cancer. Sci. Rep. 6, 30386. doi:10.1038/srep30386
Patel, S., Fu, S., Mastio, J., Dominguez, G. A., Purohit, A., Kossenkov, A., et al. (2018). Unique pattern of neutrophil migration and function during tumor progression. Nat. Immunol. 19, 1236–1247. doi:10.1038/s41590-018-0229-5
Penas-Martinez, J., Barrachina, M. N., Cuenca-Zamora, E. J., Luengo-Gil, G., Bravo, S. B., Caparros-Perez, E., et al. (2021). Qualitative and quantitative comparison of plasma exosomes from neonates and adults. Int. J. Mol. Sci. 22, 1926. doi:10.3390/ijms22041926
Peng, J., Jiang, H., Guo, J., Huang, J., Yuan, Q., Xie, J., et al. (2020). CD147 expression is associated with tumor proliferation in bladder cancer via GSDMD. Biomed. Res. Int. 2020, 7638975. doi:10.1155/2020/7638975
Perdomo, L., Vidal-Gomez, X., Soleti, R., Vergori, L., Duluc, L., Chwastyniak, M., et al. (2020). Large extracellular vesicle-associated Rap1 accumulates in atherosclerotic plaques, correlates with vascular risks and is involved in atherosclerosis. Circ. Res. 127, 747–760. doi:10.1161/CIRCRESAHA.120.317086
Perez-Gonzalez, R., Gauthier, S. A., Kumar, A., and Levy, E. (2012). The exosome secretory pathway transports amyloid precursor protein carboxyl-terminal fragments from the cell into the brain extracellular space. J. Biol. Chem. 287, 43108–43115. doi:10.1074/jbc.M112.404467
Perrotta, I., and Aquila, S. (2016). Exosomes in human atherosclerosis: An ultrastructural analysis study. Ultrastruct. Pathol. 40, 101–106. doi:10.3109/01913123.2016.1154912
Pospichalova, V., Svoboda, J., Dave, Z., Kotrbova, A., Kaiser, K., Klemova, D., et al. (2015). Simplified protocol for flow cytometry analysis of fluorescently labeled exosomes and microvesicles using dedicated flow cytometer. J. Extracell. Vesicles 4, 25530. doi:10.3402/jev.v4.25530
Ramirez, M. I., Amorim, M. G., Gadelha, C., Milic, I., Welsh, J. A., Freitas, V. M., et al. (2018). Technical challenges of working with extracellular vesicles. Nanoscale 10, 881–906. doi:10.1039/c7nr08360b
Raposo, G., and Stahl, P. D. (2019). Extracellular vesicles: A new communication paradigm? Nat. Rev. Mol. Cell Biol. 20, 509–510. doi:10.1038/s41580-019-0158-7
Raposo, G., and Stoorvogel, W. (2013). Extracellular vesicles: Exosomes, microvesicles, and friends. J. Cell Biol. 200, 373–383. doi:10.1083/jcb.201211138
Ren, A., Wu, H., Liu, L., Guo, Z., Cao, Q., and Dai, Q. (2018). Nicotine promotes atherosclerosis development in apolipoprotein E-deficient mice through α1-nAChR. J. Cell. Physiology 234, 14507–14518. doi:10.1002/jcp.27728
Royo, F., Thery, C., Falcon-Perez, J. M., Nieuwland, R., and Witwer, K. W. (2020). Methods for separation and characterization of extracellular vesicles: Results of a worldwide survey performed by the ISEV rigor and standardization subcommittee. Cells 9, 1955. doi:10.3390/cells9091955
Ruan, Z., Pathak, D., Venkatesan Kalavai, S., Yoshii-Kitahara, A., Muraoka, S., Bhatt, N., et al. (2021). Alzheimer's disease brain-derived extracellular vesicles spread tau pathology in interneurons. Brain. 144, 288–309. doi:10.1093/brain/awaa376
Russell, A. E., Sneider, A., Witwer, K. W., Bergese, P., Bhattacharyya, S. N., Cocks, A., et al. (2019). Biological membranes in EV biogenesis, stability, uptake, and cargo transfer: An ISEV position paper arising from the ISEV membranes and EVs workshop. J. Extracell. Vesicles 8, 1684862. doi:10.1080/20013078.2019.1684862
Shao, H., Im, H., Castro, C. M., Breakefield, X., Weissleder, R., and Lee, H. (2018). New Technologies for analysis of extracellular vesicles. Chem. Rev. 118, 1917–1950. doi:10.1021/acs.chemrev.7b00534
Siegel, R. L., Miller, K. D., Fuchs, H. E., and Jemal, A. (2021). Cancer statistics, 2017. Ca. Cancer J. Clin. 71, 7–30. doi:10.3322/caac.21387
Silverman, J. M., Christy, D., Shyu, C. C., Moon, K. M., Fernando, S., Gidden, Z., et al. (2019). CNS-derived extracellular vesicles from superoxide dismutase 1 (SOD1)(G93A) ALS mice originate from astrocytes and neurons and carry misfolded SOD1. J. Biol. Chem. 294, 3744–3759. doi:10.1074/jbc.RA118.004825
Silvestre, J. S., Smadja, D. M., and Levy, B. I. (2013). Postischemic revascularization: From cellular and molecular mechanisms to clinical applications. Physiol. Rev. 93, 1743–1802. doi:10.1152/physrev.00006.2013
Sun, P., Wang, C., Mang, G., Xu, X., Fu, S., Chen, J., et al. (2022). Extracellular vesicle-packaged mitochondrial disturbing miRNA exacerbates cardiac injury during acute myocardial infarction. Clin. Transl. Med. 12, e779. doi:10.1002/ctm2.779
Tauro, B. J., Greening, D. W., Mathias, R. A., Mathivanan, S., Ji, H., and Simpson, R. J. (2013). Two distinct populations of exosomes are released from LIM1863 colon carcinoma cell-derived organoids. Mol. Cell. Proteomics 12, 587–598. doi:10.1074/mcp.M112.021303
Taylor, J. P., Hardy, J., and Fischbeck, K. H. (2002). Toxic proteins in neurodegenerative disease. Science 296, 1991–1995. doi:10.1126/science.1067122
Thery, C., Witwer, K. W., Aikawa, E., Alcaraz, M. J., Anderson, J. D., Andriantsitohaina, R., et al. (2018). Minimal information for studies of extracellular vesicles 2018 (MISEV2018): A position statement of the international society for extracellular vesicles and update of the MISEV2014 guidelines. J. Extracell. Vesicles 7, 1535750. doi:10.1080/20013078.2018.1535750
Thomou, T., Mori, M. A., Dreyfuss, J. M., Konishi, M., Sakaguchi, M., Wolfrum, C., et al. (2017). Adipose-derived circulating miRNAs regulate gene expression in other tissues. Nature 542, 450–455. doi:10.1038/nature21365
Urabe, F., Kosaka, N., Ito, K., Kimura, T., Egawa, S., and Ochiya, T. (2020). Extracellular vesicles as biomarkers and therapeutic targets for cancer. Am. J. Physiol. Cell Physiol. 318, C29–C39. doi:10.1152/ajpcell.00280.2019
Vaidyanathan, R., Soon, R. H., Zhang, P., Jiang, K., and Lim, C. T. (2018). Cancer diagnosis: From tumor to liquid biopsy and beyond. Lab. Chip 19, 11–34. doi:10.1039/c8lc00684a
Valadi, H., Ekstrom, K., Bossios, A., Sjostrand, M., Lee, J. J., and Lotvall, J. O. (2007). Exosome-mediated transfer of mRNAs and microRNAs is a novel mechanism of genetic exchange between cells. Nat. Cell Biol. 9, 654–659. doi:10.1038/ncb1596
Van Deun, J., Mestdagh, P., Sormunen, R., Cocquyt, V., Vermaelen, K., Vandesompele, J., et al. (2014). The impact of disparate isolation methods for extracellular vesicles on downstream RNA profiling. J. Extracell. Vesicles 3, 24858. doi:10.3402/jev.v3.24858
Vassileff, N., Vella, L. J., Rajapaksha, H., Shambrook, M., Kenari, A. N., McLean, C., et al. (2020). Revealing the proteome of motor cortex derived extracellular vesicles isolated from amyotrophic lateral sclerosis human postmortem tissues. Cells 9, 1709. doi:10.3390/cells9071709
Vella, L. J., Scicluna, B. J., Cheng, L., Bawden, E. G., Masters, C. L., Ang, C. S., et al. (2017). A rigorous method to enrich for exosomes from brain tissue. J. Extracell. Vesicles 6, 1348885. doi:10.1080/20013078.2017.1348885
Wang, D., Wang, X., Song, Y., Si, M., Sun, Y., Liu, X., et al. (2022). Exosomal miR-146a-5p and miR-155-5p promote CXCL12/CXCR7-induced metastasis of colorectal cancer by crosstalk with cancer-associated fibroblasts. Cell Death Dis. 13, 380. doi:10.1038/s41419-022-04825-6
Wang, Y. H., Ji, J., Wang, B. C., Chen, H., Yang, Z. H., Wang, K., et al. (2018). Tumor-derived exosomal long noncoding RNAs as promising diagnostic biomarkers for prostate cancer. Cell. Physiol. biochem. 46, 532–545. doi:10.1159/000488620
Wei, M., Gao, X., Liu, L., Li, Z., Wan, Z., Dong, Y., et al. (2020). Visceral adipose tissue derived exosomes exacerbate colitis severity via pro-inflammatory MiRNAs in high fat diet fed mice. ACS Nano 14, 5099–5110. doi:10.1021/acsnano.0c01860
Wen, S. W., Sceneay, J., Lima, L. G., Wong, C. S., Becker, M., Krumeich, S., et al. (2016). The biodistribution and immune suppressive effects of breast cancer-derived exosomes. Cancer Res. 76, 6816–6827. doi:10.1158/0008-5472.CAN-16-0868
Weng, Z., Zhang, B., Wu, C., Yu, F., Han, B., Li, B., et al. (2021). Therapeutic roles of mesenchymal stem cell-derived extracellular vesicles in cancer. J. Hematol. Oncol. 14, 136. doi:10.1186/s13045-021-01141-y
Wong, K. L., Zhang, S., Wang, M., Ren, X., Afizah, H., Lai, R. C., et al. (2020). Intra-articular injections of mesenchymal stem cell exosomes and hyaluronic acid improve structural and mechanical properties of repaired cartilage in a rabbit model. Arthroscopy 36, 2215–2228. doi:10.1016/j.arthro.2020.03.031
Wong, S. H. D., Xu, X., Chen, X., Xin, Y., Xu, L., Lai, C. H. N., et al. (2021). Manipulation of the nanoscale presentation of integrin ligand produces cancer cells with enhanced stemness and robust tumorigenicity. Nano Lett. 21, 3225–3236. doi:10.1021/acs.nanolett.1c00501
Wright, T. J., Hatch, E. P., Karabagli, H., Karabagli, P., Schoenwolf, G. C., and Mansour, S. L. (2003). Expression of mouse fibroblast growth factor and fibroblast growth factor receptor genes during early inner ear development. Dev. Dyn. 228, 267–272. doi:10.1002/dvdy.10362
Xiao, H., Lasser, C., Shelke, G. V., Wang, J., Radinger, M., Lunavat, T. R., et al. (2014). Mast cell exosomes promote lung adenocarcinoma cell proliferation - role of KIT-stem cell factor signaling. Cell Commun. Signal. 12, 64. doi:10.1186/s12964-014-0064-8
Yanez-Mo, M., Siljander, P. R., Andreu, Z., Zavec, A. B., Borras, F. E., Buzas, E. I., et al. (2015). Biological properties of extracellular vesicles and their physiological functions. J. Extracell. Vesicles 4, 27066. doi:10.3402/jev.v4.27066
Yu, S., Huang, M., Wang, J., Zheng, Y., and Xu, H. (2021). Extracellular vesicles in tumor diagnosis: A mini-review. Curr. Mol. Med. 21, 596–606. doi:10.2174/1573405616666201209103154
Zhan, Q., Yi, K., Qi, H., Li, S., Li, X., Wang, Q., et al. (2020). Engineering blood exosomes for tumor-targeting efficient gene/chemo combination therapy. Theranostics 10, 7889–7905. doi:10.7150/thno.45028
Zhang, J., Guan, J., Niu, X., Hu, G., Guo, S., Li, Q., et al. (2015). Exosomes released from human induced pluripotent stem cells-derived MSCs facilitate cutaneous wound healing by promoting collagen synthesis and angiogenesis. J. Transl. Med. 13, 49. doi:10.1186/s12967-015-0417-0
Zhang, X., Shi, H., Yuan, X., Jiang, P., Qian, H., and Xu, W. (2018). Tumor-derived exosomes induce N2 polarization of neutrophils to promote gastric cancer cell migration. Mol. Cancer 17, 146. doi:10.1186/s12943-018-0898-6
Zhang, Y., Yu, M., Dai, M., Chen, C., Tang, Q., Jing, W., et al. (2017). miR-450a-5p within rat adipose tissue exosome-like vesicles promotes adipogenic differentiation by targeting WISP2. J. Cell Sci. 130, 1158–1168. doi:10.1242/jcs.197764
Zhao, H., Chen, X., Hu, G., Li, C., Guo, L., Zhang, L., et al. (2022). Small extracellular vesicles from Brown adipose tissue mediate exercise cardioprotection. Circ. Res. 130, 1490–1506. doi:10.1161/CIRCRESAHA.121.320458
Zhao, X., Wu, D., Ma, X., Wang, J., Hou, W., and Zhang, W. (2020). Exosomes as drug carriers for cancer therapy and challenges regarding exosome uptake. Biomed. Pharmacother. 128, 110237. doi:10.1016/j.biopha.2020.110237
Zheng, Y., Zhu, K., and Wang, G. (2022). miR-106a-5p carried by tumor-derived extracellular vesicles promotes the invasion and metastasis of ovarian cancer by targeting KLF6. Clin. Exp. Metastasis 39, 603–621. doi:10.1007/s10585-022-10165-8
Keywords: extracellular vesicles, tissue derived extracellular vesicles, microenvironment, biomarker, tumor
Citation: Zhi Z, Sun Q and Tang W (2022) Research advances and challenges in tissue-derived extracellular vesicles. Front. Mol. Biosci. 9:1036746. doi: 10.3389/fmolb.2022.1036746
Received: 05 September 2022; Accepted: 05 December 2022;
Published: 15 December 2022.
Edited by:
Abhimanyu Thakur, The University of Chicago, United StatesReviewed by:
Pietro Parisse, National Research Council (CNR), ItalyCopyright © 2022 Zhi, Sun and Tang. This is an open-access article distributed under the terms of the Creative Commons Attribution License (CC BY). The use, distribution or reproduction in other forums is permitted, provided the original author(s) and the copyright owner(s) are credited and that the original publication in this journal is cited, in accordance with accepted academic practice. No use, distribution or reproduction is permitted which does not comply with these terms.
*Correspondence: Weibing Tang, dHdiY25AbmptdS5lZHUuY24=
†These authors have contributed equally to this work
Disclaimer: All claims expressed in this article are solely those of the authors and do not necessarily represent those of their affiliated organizations, or those of the publisher, the editors and the reviewers. Any product that may be evaluated in this article or claim that may be made by its manufacturer is not guaranteed or endorsed by the publisher.
Research integrity at Frontiers
Learn more about the work of our research integrity team to safeguard the quality of each article we publish.