- Department of Pharmacy and Biotechnology, University of Bologna, Bologna, Italy
F-type ATP synthases are transmembrane enzymes, which play a central role in the metabolism of all aerobic and photosynthetic cells and organisms, being the major source of their ATP synthesis. Catalysis occurs via a rotary mechanism, in which the free energy of a transmembrane electrochemical ion gradient is converted into the free energy of ATP phosphorylation from ADP and Pi, and vice versa. An ADP, tightly bound to one of the three catalytic sites on the stator head, is associated with catalysis inhibition, which is relieved by the transmembrane proton gradient and by ATP. By preventing wasteful ATP hydrolysis in times of low osmotic energy and low ATP/ADP ratio, such inhibition constitutes a classical regulatory feedback effect, likely to be an integral component of in vivo regulation. The present miniview focuses on an additional putative regulatory phenomenon, which has drawn so far little attention, consisting in a substrate-induced tuning of the H+/ATP coupling ratio during catalysis, which might represent an additional key to energy homeostasis in the cell. Experimental pieces of evidence in support of such a phenomenon are reviewed.
1 Introduction
F-type ATP synthases (“ATP synthases” in the following) are highly conserved transmembrane enzymes, found in most eubacteria, mitochondria and chloroplasts (Boyer, 1997; Kinosita et al., 1998; Leslie and Walker, 2000; Junge and Nelson, 2015; Kühlbrandt, 2019), where they represent the main source of ADP phosphorylation. They convert the free energy of the transmembrane electrochemical H+-gradient (“H+-gradient” in the following)—or Na+-gradient, in some organisms (Dimroth, 1990)—into the free energy of ATP phosphorylation from ADP and Pi, and vice versa, as first proposed by the chemiosmotic theory (Mitchell, 1961). They do so by a rotary mechanism, in which, viewed from the hydrophobic transmembrane FO sector to the hydrophilic F1 head, the rotor moves either clockwise (synthesis), or anti-clockwise (hydrolysis) (Diez et al., 2004; Iino and Noji, 2006; Noji and Ueno, 2022).
F1 contains a ring of three alternating α- and β-subunits, the latter bearing the three catalytic sites, at which ATP synthesis/hydrolysis occurs (Figure 1). The H+ translocate within FO, at the interface between the hydrophobic stator and rotor parts. The hydrophobic rotor part is a ring-shaped oligomer of c-subunits, which, during synthesis, transmits the torque to the hydrophilic rotor subunits γ and ε. The latters in turn rotate within the central cavity of the α3β3-barrel, thus inducing the cyclic conformational changes in the β-subunits, which elicit substrate binding and product release. The number of c-subunits varies in different organisms, from 8 in the bovine mitochondrial enzyme, to 17 in the human pathogen Burkholderia pseudomallei (Kühlbrandt, 2019).
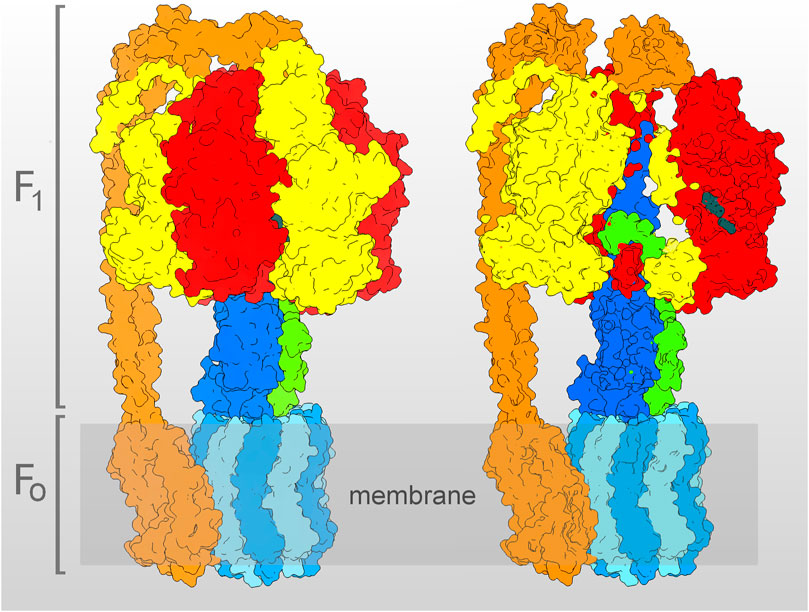
FIGURE 1. Molecular model of the E. coli ATP synthase, determined by cryo-EM (Sobti et al., 2020, PDB entry 6oqr, with the complex in the ε-subunit “up” state–see Section 3). On the left, the whole model is shown, on the right, the same model is clipped, to show the γ- (blue) and ε- (green) subunits filling the inner cavity of the α3β3-barrel (α-subunit in yellow, β-subunit in red). The c10-oligomer (light blue), together with the γ- and ε-subunits, build the rotor, while the stator is constituted by the α3β3-barrel and by the peripheral stalk (orange), composed by the δ-, b2-, and a-subunits. In the clipped model, the ADP and Pi bound to the catalytic site of the β1 are visible (dark gray). The model was represented using USCF Chimera (Pettersen et al., 2004).
The rotary mechanism of F-type ATP synthases is shared by the related type-A ATP synthases (Archaebacteria) and type-V ATPases (vacuolar and other cellular membranes), the latter functioning in vivo for ATP hydrolysis (Zubareva et al., 2020). The three enzyme types also share some regulatory features, in particular the non-competitive inhibition by low ADP concentrations, which locks the enzyme in an inhibited conformation (Vasilyeva and Forgac, 1998; Feniouk and Yoshida, 2008; Sielaff et al., 2018; Zubareva et al., 2020). In the F-type enzymes, the tightly bound ADP is released, thus recovering the catalytic activity, at high ATP concentrations or high H+-gradients (Lapashina and Feniouk, 2018), by switching to a different conformation, which is metastable in the absence of a H+-gradient (Fischer et al., 2000, and references therein). By preventing the thermodynamically favored ATP hydrolysis at low ATP/ADP ratio and low H+-gradient, such regulation appears to be functional for cellular energy conservation in times of energy depletion.
Since every c-subunit bears one H+ binding site, and the α3β3-barrel bears three catalytic sites, the rotary mechanism implies that the maximum possible H+/ATP stoichiometry is given by the number of c-subunit divided by three (Läuger, 1984; Junge, 1999; Watt et al., 2010). Bulk measurements in the chloroplast and mitochondrial enzymes, near to the thermodynamic equilibrium between the free energies of H+-gradient and ADP phosphorylation, have yielded values of the effective number of H+ translocated for each ATP molecule hydrolyzed/synthesized—the “H+/ATP coupling ratio” (Läuger, 1984)—close to, but 10%–15% smaller than, their respective c/β ratios (Van Walraven et al., 1996; Petersen et al., 2012, but see Soga et al., 2017, for even closer values in Bacillus PS3). Such less-than-c/β values are eventually consistent with some internal energy dissipation within the molecular motor (Martin et al., 2014; Hou and Wang, 2021), including, e.g., the H+-gradient energy used for maintaining otherwise metastable enzyme states (Turina et al., 2016), or the ATP binding/hydrolysis energy used for other “priming” reactions (see Section 3).
Since the rotary mechanism of the ATP synthase has been experimentally shown (Noji et al., 1997), much attention has been devoted to investigating, in single ATP synthase molecule experiments, the remarkably high tightness featured by its chemomechanical coupling (Kinosita et al., 2000, 2004; Toyabe et al., 2011; Zimmermann and Seifert, 2012; Saita et al., 2015). While such high efficiency in chemomechanical coupling can indeed be considered an evolutionary optimized feature of this “splendid molecular machine” (Boyer, 1997), on the other hand, cellular systems have also been shown to harbor sophisticated devices for fine-tuning the overall energy conversion efficiency. The mitochondria themselves are a prime example of regulated H+-leak mechanisms, with the Uncoupling Protein 1 and the ADP/ATP carrier considered to play the main role in the regulation of thermogenesis and reactive oxygen species production (Cadenas, 2018; Demine et al., 2019; Bertholet and Kirichok, 2022), or with their permeability transition pore deciding between cell life and death (Bernardi et al., 2021). The close cousins V-type ATPases have been shown to work with variable coupling ratios, proposed to be functional to variable acidification needs (Davies et al., 1994; Müller and Taiz, 2002; Kettner et al., 2003; Forgac, 2007; Saroussi and Nelson, 2009). In addition, variable coupling ratios in the ATP synthase, according to energy conditions, have been reported in whole bacterial cells (Gober and Kashket, 1984; Vink et al., 1984; Cook and Russell, 1994). Possibly, the marvel at the high tightness of energy conversion within the ATP synthase has let researchers in the field assume that its evolution, at a given c/β stoichiometry, had only taken place towards the highest possible coupling ratio. To my knowledge, very little work has been devoted so far to investigating the possibility that such coupling ratio could itself be subjected to regulation.
Purpose of the present work is to propose the existence of a built-in mechanism able to modulate the H+/ATP coupling ratio within the F-type ATP synthases, eventually useful for in vivo energy homeostasis, by highlighting some experimental evidence which is consistent with such hypothesis.
2 Nucleotide- and ligand-induced changes in the H+/ATP coupling ratio
2.1 Nucleotides
A change in coupling ratio, dependent on the ADP concentration, has been reported and investigated in bacterial ATP synthases (Turina et al., 2004; D’Alessandro et al., 2008). Measurements were carried out in the hydrolysis direction, progressively reducing the ADP concentration by an ADP trap at constant ATP concentration, thereby observing a corresponding reduction in the number of H+ pumped per hydrolyzed ATP. In both tested bacterial species, the less-coupled hydrolysis was inhibited by Fo inhibitors, oligomycin in Rb. capsulatus, DCCD in E. coli. The data could be most easily explained by the ATP synthase existing in, at least, two interconvertible states, differing by their own coupling ratios, with the higher coupling-ratio state (EC) favored by ADP binding, and the lower coupling-ratio state (EU) favored by ATP binding. ATP and ADP would compete for the same site, the occupancy of which, with either nucleotide, would determine the switch between the two conformations. That site could thus constitute a direct regulatory “sensor” of the cellular ATP/ADP ratio.
In D’Alessandro et al. (2011), D’Alessandro et al. (2017) two ADP binding sites were identified by their different functional effects and different apparent Kds, both in the sub-micromolar range, the tighter site being associated with the change in coupling ratio, and the other one with inhibition of ATP hydrolysis. Given the high-affinity binding of ADP to one of the three catalytic sites on E. coli F1 (Cingolani and Duncan, 2011), a possible scenario is that the two ADP binding sites can be identified as the same catalytic site, showing different properties in the two different enzyme conformations (EU and EC).
In a nutshell, the above scenario implies that EU is stabilized by ATP and that EC is stabilized by ADP. We know that the tightly bound ADP fully inhibits catalysis, and that an H+-gradient is needed to release the tightly bound ADP and let the (fully coupled) rotary catalysis take place for several turnovers. Most likely, also the EU needs some triggering event to get rid of the bound ATP, to be able to engage in its less coupled, but still rotary, catalysis. ATP binding/hydrolysis to another catalytic site could be such an event.
Previously, measurements of ATP hydrolysis in parallel with H+ pumping by (Shigalowa et al., 1985; Strotmann et al., 1986) had also supported the existence of an uncoupled form of the ATP synthase. According to the authors, the data could be best explained by postulating that a non-hydrolyzed ATP, bound to the ATP synthase in place of a tightly bound ADP, would induce a partially uncoupled ATP hydrolysis.
Interestingly, high concentrations of ATP were reported to induce a partial uncoupling of the V-ATPase pump (Arai et al., 1989; Shao and Forgac, 2004).
2.2 Sulfite
The most evident effect of sulfite on the ATP synthase is a strong increase of the hydrolysis activity, which has been associated with the release of the inhibitory, tightly bound ADP (Vasilyeva et al., 1982; Larson et al., 1989; Murataliev and Boyer, 1992). While the presence of ATP could slowly release the tightly bound ADP during catalysis, the presence of sulfite significantly accelerated the ATP-dependent reactivation of the ADP-inhibited complex. However, sulfite was shown to inhibit ATP synthesis, in a way not readily explained by competition with Pi (Pacheco-Moisés et al., 2002) and, consistently with the latter inhibition, was also shown to decrease the H+/ATP coupling ratio, as measured in the hydrolysis direction, both in the V-type ATPase (Kibak et al., 1993), and in the ATP synthase (Cappellini et al., 1997). The sulfite-activated hydrolysis was still completely inhibited by FO inhibitors, such as oligomycin, venturicidin, DCCD (Moyle and Mitchell, 1975; Zhang et al., 1993; Cappellini et al., 1997; Pacheco-Moisés et al., 2000; Pacheco-Moisés et al., 2002).
The effects of sulfite can be interpreted in light of the scenario put forward in Section 2.1. The contiguity of ADP and sulfite in the tight ADP-binding site can be envisaged to mimic the structure of an ATP, and therefore to trigger the EC → EU conversion in the ADP-inhibited ATP synthase molecules. Such sulfite-induced conversion would cause the observed decrease in the H+/ATP ratio of hydrolysis, and the lower synthesis rate at constant H+-gradient. The proposal by Moyle and Mitchell (1975) and Pacheco-Moisés et al. (2000), that the sulfite-induced active state is associated with structural modifications of the enzyme, supports the above interpretation.
Other oxyanions, structurally similar to sulfite, have been less investigated (Walker, 1994). Selenite is known to increase hydrolysis (see, e.g., Jarman et al., 2021), and a systematic investigation might show that it is uncoupling as well. Carbonate has long been known to be activating for hydrolysis, and more recently it has also been reported to inhibit synthesis in submitochondrial particles (Lodeyro et al., 2001). The structurally close chloroform was also reported to change the H+/ATP ratio (Rottenberg, 1983).
2.3 ATP-Pi exchange
The ATP-Pi exchange is due to the ATP synthesis catalyzed by the ATP synthase in the presence of Pi and of a high ATP/ADP ratio, which induces an ATP-generated H+-gradient. It is called that way since the newly formed ATP is detected as the radioactive ATP generated when the only radioactive reagent at the start of the reaction was Pi. Lodeyro et al. (2001) excluded the possibility of an uncoupling effect of carbonate, based on their finding that it activated the ATP-Pi exchange. An alternative interpretation can be proposed on the basis of the EU ↔ EC hypothesis, since the carbonate-induced higher EU/EC ratio would decrease the rates under the experimental conditions normally used for measuring ATP synthesis (low ATP/ADP ratio), but could increase the H+-gradient, and thus the ATP synthesis rates, during ATP-Pi exchange (high ATP/ADP ratio, see below). Notice that sulfite has also been shown to increase the rate of ATP-Pi exchange (Pacheco-Moisés et al., 2002).
Even though a high ATP/ADP ratio is a most frequent condition in the cell, the experimentally observed ATP-Pi exchange still represents an energetic conundrum for an ATP synthase with a homogeneous H+/ATP ratio, since substantial ATP synthesis is found under nearly prohibitive conditions for such reaction (very high ATP/ADP ratio and low H+-gradient). However, the co-occurrence of EC and EU during the ATP-Pi exchange could represent an easy solution for that enigma, since EU, if its lower H+/ATP coupling ratio was due to a lower H+/ATP stoichiometry (Section 4), would represent the steeper H+-gradient producer, and EC the better H+-gradient consumer, thus allowing substantial synthesis rates by concomitant maximal measurable hydrolysis rates. The co-occurrence of two different active conformations (EC and EU) would also agree with the results obtained by (Shoshan and Shavit, 1979), who showed that Fab fragments against F1 inhibited ATP-Pi exchange, but not light-driven synthesis, implying that an additional ATPase conformation (EU) played a major role during ATP-Pi exchange, different from the one (EC) catalyzing the light-driven synthesis.
2.4 Ca2+
The ATP synthesis/hydrolysis, catalyzed by the ATP synthase, requires Mg2+. When Mg2+ is substituted by Ca2+, the synthesis is inhibited, while the hydrolysis remains high, and still sensitive to the FO-inhibitors DCCD, oligomycin and venturicidin, but it becomes uncoupled from H+-translocation, as reported by several groups (e.g., Pick and Weiss, 1988; Strid and Nyrén, 1989; Casadio and Melandri, 1996; Papageorgiou et al., 1998). Uncoupling by Ca2+ has also been reported in V-type ATPases (Crider and Xie, 2003). The hydrolysis of CaATP was later shown capable of sustaining the rotary motions of the γ-subunit in single molecule detection, with a rate comparable to that induced by MgATP (Tucker et al., 2004). More recently, in purified and reconstituted bovine ATP synthase, Urbani et al. (2019) showed that Ca2+ causes channel-like dissipation of the ATP hydrolysis-induced H+-gradient, and does so even more in the presence of activators of the permeability transition pore.
Similar as proposed for sulfite (Section 2.2), the underlying reasons for Ca2+-uncoupling might be related to the ADP/ATP occupancy of the high-affinity catalytic site. Both by direct binding experiments (Maldonado et al., 1998) and by measuring the extent of water oxygen incorporation into released ATP (Kohlbrenner and Boyer, 1983), the same conclusion was reached, that Ca2+ in place of Mg2+ strongly accelerated ADP release from the high-affinity catalytic site on the enzyme. The EC ↔ EU interconversion would then be significantly shifted by Ca2+ toward the less coupled EU.
2.5 Other ligands
The human hormon 17β-Estradiol (E2) was found to directly bind to a subunit of the mitochondrial ATP synthase (Zheng and Ramirez, 1999) and to inhibit ATP synthesis (Massart et al., 2002). More recently, in simultaneous measurements of H+-gradient and ATP synthesis, Moreno et al. (2013) have reported that E2 decreased the H+/ATP ratio in the synthesis direction, and that such an effect was dependent on the presence of non-hydrolyzed ATP. As proposed above (Sections 2.1–2.4), such decrease could be due to a shift of the EC ↔ EU equilibrium toward the less coupled EU, induced by E2 binding to the enzyme.
Interestingly, the mitochondrial ATP synthase has also been reported to be a target for several phytoestrogens, such as quercetin, resveratrol, curcumin (Zheng and Ramirez, 2000), for the anorexigenic peptides enterostatin (Berger et al., 2002; Lindqvist et al., 2008) and Angt_Human [448–462] (Sasaki et al., 2022), as well as for the anti-apoptotic peptide Bcl-xL, whose binding to the β-subunit was reported to decrease an ATP-dependent ion leak within the ATP synthase (Alavian et al., 2011).
The phytotoxin tentoxin showed also an interesting behavior, reminiscent of that of sulfite. At low concentrations, it was reported to increase the affinity of the non-hydrolyzable ATP analog AMPPNP for the high-affinity nucleotide-binding site (Selman and Selman-Reimer, 1979), thus blocking the enzyme, but then favoring the EU conformation at higher concentrations. Consistently, higher tentoxin concentrations reactivated hydrolysis and rotation (Meiss et al., 2008), and at a lower H+/ATP ratio (Sigalat et al., 1995).
3 Nucleotide-induced changes in the ATP synthase conformation under unisite conditions
When the ratio between substrates and ATP synthase is less than one, catalysis has been shown to occur at the high-affinity site, both in synthesis and hydrolysis direction—the so-called “unisite” catalysis, which is several orders of magnitude slower than multisite steady-state catalysis (Penefsky and Cross, 1991). Though unisite catalysis has often been considered a step cooperatively integrated to each turnover of the multisite catalysis, it has also been shown to take place in the absence of rotation (García and Capaldi, 1998), a result in agreement with those of Bullough et al. (1987), and with a recent cryo-EM study, which indicates that unisite catalysis is an initial reaction that is distinguished from steady-state rotary catalysis (Nakano et al., 2022). In addition, Sakaki et al. (2005) measured the same rotation rates from mM down to nM ATP concentrations, without detecting any transition from multisite to unisite rates.
In (Turina and Capaldi, 1994), the kinetics of unisite catalysis was found to match the kinetics of the emission changes of a fluorescent probe, attached to a site-directed cysteine in the γ-subunit as a reporter of conformational changes. The kinetics analysis indicated at least two conformations, one of which induced by ATP binding to the high-affinity catalytic site, the other one induced by the ensuing ADP still bound at the same site after hydrolysis.
Based on the above results, the unisite catalysis can be hypothesized to constitute a “priming” reaction, which sets the stage for the subsequent rotary catalysis. Such a priming reaction at the high affinity site would generate either an ATP-bound or an ADP-bound conformation. Those two conformations may be taken to coincide with EU and EC, respectively, since they are similarly induced by the ATP or ADP occupancy of the high-affinity site.
Cryo-EM studies in the presence or absence of multisite nucleotides (Sobti et al., 2019, 2020) showed that, after exposure to ATP, the E. coli enzyme adopts a different conformation, with a catalytic subunit (βDP) changing from open to closed, and the ε-subunit C-terminal domain (εCTD) converting from the “up” to the “down” state. Conversely, exposure to ADP induced only a partial closure of the βDP subunit, and maintained the εCTD “up” state. Interestingly, in crosslinking studies, an “up” conformation of the bacterial enzyme was reported to retain synthesis but to be inhibited in hydrolysis, while the “down” conformation retained both (Tsunoda et al., 2001; Suzuki et al., 2003). The transition between the two forms was determined by the H+-gradient and by the ATP/ADP ratio (Suzuki et al., 2003). Though the εCTD-less enzyme retained the nucleotide-dependent uncoupling (D’Alessandro et al., 2017), suggesting that the εCTD is not the primary motor of the EC ↔ EU change, such two largely different εCTD states imply two largely different ATP synthase conformations even in the εCTD-less enzyme.
4 Leak, slip, or lower H+/ATP stoichiometry?
The lower H+/ATP coupling ratio of EU could be due to a leak (a passive H+ channel), to more frequent slippage (a stochastic “deviation” from the usual coupled reaction pathway), or to a different coupling mechanism, featuring a lower H+/ATP stoichiometry—or to a combination of the three. Leak or higher slippage would mean that energy dissipation within EU is significantly higher than energy dissipation within EC. Possibly, only the case of a lower H+/ATP stoichiometry, which does not necessarily involve a higher energy dissipation in EU, could succeed in explaining the mystery of ATP-Pi exchange (Section 2.3). A variable H+/ATP stoichiometry, on top of a slippage, has been reported in V-ATPases, which was able to explain the very steep H+-gradient found in the vacuoles of lemon fruit (Müller and Taiz, 2002). Future experimental work might confirm the V-ATPase result and eventually find similar evidence in the ATP synthase as well.
5 Conclusion
In the present work, some functional evidence is reviewed, indicating that the ATP synthase might adopt two different conformations, characterized by different H+/ATP coupling ratios, and that the ATP or ADP occupancy of its high-affinity catalytic site may be the key for the conformational switch (Section 2). In addition, structural evidence is reviewed, which supports the existence of two largely different conformations, according to the ATP or ADP occupancy of the high-affinity catalytic site (Section 3). Future work may confirm or not the idea that those two ATP synthase conformations differently couple nucleotide synthesis/hydrolysis with H+-translocation through FO. Should such modulation by the ATP/ADP ratio of the coupling degree between the two main energy currencies in the cell—ATP and the transmembrane H+-gradient—be confirmed, it would open yet another new perspective on the subtlety and elegance of both ATP synthase and of metabolism regulation.
Author contributions
PT analyzed the data and wrote the text.
Conflict of interest
The authors declare that the research was conducted in the absence of any commercial or financial relationships that could be construed as a potential conflict of interest.
Publisher’s note
All claims expressed in this article are solely those of the authors and do not necessarily represent those of their affiliated organizations, or those of the publisher, the editors and the reviewers. Any product that may be evaluated in this article, or claim that may be made by its manufacturer, is not guaranteed or endorsed by the publisher.
References
Alavian, K. N., Li, H., Collis, L., Bonanni, L., Zeng, L., Sacchetti, S., et al. (2011). Bcl-xL regulates metabolic efficiency of neurons through interaction with the mitochondrial F1FO ATP synthase. Nat. Cell Biol. 13, 1224–1233. doi:10.1038/ncb2330
Arai, H., Pink, S., and Forgac, M. (1989). Interaction of anions and ATP with the coated vesicle proton pump. Biochemistry 28, 3075–3082. doi:10.1021/bi00433a051
Berger, K., Sivars, U., Winzell, M. S., Johansson, P., Hellman, U., Rippe, C., et al. (2002). Mitochondrial ATP synthase--a possible target protein in the regulation of energy metabolism in vitro and in vivo. Nutr. Neurosci. 5, 201–210. doi:10.1080/10284150290008604
Bernardi, P., Carraro, M., and Lippe, G. (2021). The mitochondrial permeability transition: Recent progress and open questions. FEBS J. 16254 doi:10.1111/febs.16254
Bertholet, A. M., and Kirichok, Y. (2022). Mitochondrial H+ leak and thermogenesis. Annu. Rev. Physiol. 84, 381–407. doi:10.1146/annurev-physiol-021119-034405
Boyer, P. D. (1997). The ATP synthase--a splendid molecular machine. Annu. Rev. Biochem. 66, 717–749. doi:10.1146/annurev.biochem.66.1.717
Bullough, D. A., Verburg, J. G., Yoshida, M., and Allison, W. S. (1987). Evidence for functional heterogeneity among the catalytic sites of the bovine heart mitochondrial F1-ATPase. J. Biol. Chem. 262, 11675–11683. doi:10.1016/s0021-9258(18)60863-4
Cadenas, S. (2018). Mitochondrial uncoupling, ROS generation and cardioprotection. Biochim. Biophys. Acta. Bioenerg. 1859, 940–950. doi:10.1016/j.bbabio.2018.05.019
Cappellini, P., Turina, P., Fregni, V., and Melandri, B. A. (1997). Sulfite stimulates the ATP hydrolysis activity of but not proton translocation by the ATP synthase of Rhodobacter capsulatus and interferes with its activation by delta muH+. Eur. J. Biochem. 248, 496–506. doi:10.1111/j.1432-1033.1997.00496.x
Casadio, R., and Melandri, B. A. (1996). CaATP inhibition of the MgATP-dependent proton pump (H+-ATPase) in bacterial photosynthetic membranes with a mechanism of alternative substrate inhibition. J. Biol. Inorg. Chem. 1, 284–291. doi:10.1007/s007750050055
Cingolani, G., and Duncan, T. M. (2011). Structure of the ATP synthase catalytic complex (F(1)) from Escherichia coli in an autoinhibited conformation. Nat. Struct. Mol. Biol. 18, 701–707. doi:10.1038/nsmb.2058
Cook, G. M., and Russell, J. B. (1994). Energy-spilling reactions of Streptococcus bovis and resistance of its membrane to proton conductance. Appl. Environ. Microbiol. 60, 1942–1948. doi:10.1128/aem.60.6.1942-1948.1994
Crider, B. P., and Xie, X.-S. (2003). Characterization of the functional coupling of bovine brain vacuolar-type H(+)-translocating ATPase. Effect of divalent cations, phospholipids, and subunit H (SFD). J. Biol. Chem. 278, 44281–44288. doi:10.1074/jbc.M307372200
D’Alessandro, M., Turina, P., Melandri, B. A., and Dunn, S. D. (2017). Modulation of coupling in the Escherichia coli ATP synthase by ADP and Pi: Role of the ε subunit C-terminal domain. Biochim. Biophys. Acta. Bioenerg. 1858, 34–44. doi:10.1016/j.bbabio.2016.10.004
D’Alessandro, M., Turina, P., and Melandri, B. A. (2008). Intrinsic uncoupling in the ATP synthase of Escherichia coli. Biochim. Biophys. Acta 1777, 1518–1527. doi:10.1016/j.bbabio.2008.09.011
D’Alessandro, M., Turina, P., and Melandri, B. A. (2011). Quantitative evaluation of the intrinsic uncoupling modulated by ADP and P(i) in the reconstituted ATP synthase of Escherichia coli. Biochim. Biophys. Acta 1807, 130–143. doi:10.1016/j.bbabio.2010.08.011
Davies, J. M., Hunt, I., and Sanders, D. (1994). Vacuolar H(+)-pumping ATPase variable transport coupling ratio controlled by pH. Proc. Natl. Acad. Sci. U. S. A. 91, 8547–8551. doi:10.1073/pnas.91.18.8547
Demine, S., Renard, P., and Arnould, T. (2019). Mitochondrial uncoupling: A key controller of biological processes in physiology and diseases. Cells 8, E795. doi:10.3390/cells8080795
Diez, M., Zimmermann, B., Börsch, M., König, M., Schweinberger, E., Steigmiller, S., et al. (2004). Proton-powered subunit rotation in single membrane-bound F0F1-ATP synthase. Nat. Struct. Mol. Biol. 11, 135–141. doi:10.1038/nsmb718
Dimroth, P. (1990). Mechanisms of sodium transport in bacteria. Philos. Trans. R. Soc. Lond. B Biol. Sci. 326, 465–477. doi:10.1098/rstb.1990.0025
Feniouk, B. A., and Yoshida, M. (2008). Regulatory mechanisms of proton-translocating F(O)F (1)-ATP synthase. Results Probl. Cell Differ. 45, 279–308. doi:10.1007/400_2007_043
Fischer, S., Graber, P., and Turina, P. (2000). The activity of the ATP synthase from Escherichia coli is regulated by the transmembrane proton motive force. J. Biol. Chem. 275, 30157–30162. doi:10.1074/jbc.275.39.30157
Forgac, M. (2007). Vacuolar ATPases: Rotary proton pumps in physiology and pathophysiology. Nat. Rev. Mol. Cell Biol. 8, 917–929. doi:10.1038/nrm2272
García, J. J., and Capaldi, R. A. (1998). Unisite catalysis without rotation of the gamma-epsilon domain in Escherichia coli F1-ATPase. J. Biol. Chem. 273, 15940–15945. doi:10.1074/jbc.273.26.15940
Gober, J. W., and Kashket, E. R. (1984). H+/ATP stoichiometry of cowpea Rhizobium sp. strain 32H1 cells grown under nitrogen-fixing and nitrogen-nonfixing conditions. J. Bacteriol. 160, 216–221. doi:10.1128/jb.160.1.216-221.1984
Hou, R., and Wang, Z. (2021). Thermodynamic marking of FOF1 ATP synthase. Biochim. Biophys. Acta. Bioenerg. 1862, 148369. doi:10.1016/j.bbabio.2021.148369
Iino, R., and Noji, H. (2006). F1-ATPase: A highly coupled reversible rotary motor. Biochem. Soc. Trans. 34, 993–996. doi:10.1042/BST0340993
Jarman, O. D., Biner, O., and Hirst, J. (2021). Regulation of ATP hydrolysis by the ε subunit, ζ subunit and Mg-ADP in the ATP synthase of Paracoccus denitrificans. Biochim. Biophys. Acta. Bioenerg. 1862, 148355. doi:10.1016/j.bbabio.2020.148355
Junge, W. (1999). ATP synthase and other motor proteins. Proc. Natl. Acad. Sci. U. S. A. 96, 4735–4737. doi:10.1073/pnas.96.9.4735
Junge, W., and Nelson, N. (2015). ATP synthase. Annu. Rev. Biochem. 84, 631–657. doi:10.1146/annurev-biochem-060614-034124
Kettner, C., Bertl, A., Obermeyer, G., Slayman, C., and Bihler, H. (2003). Electrophysiological analysis of the yeast V-type proton pump: Variable coupling ratio and proton shunt. Biophys. J. 85, 3730–3738. doi:10.1016/S0006-3495(03)74789-4
Kibak, H., Van Eeckhout, D., Cutler, T., Taiz, S. L., and Taiz, L. (1993). Sulfite both stimulates and inhibits the yeast vacuolar H(+)-ATPase. J. Biol. Chem. 268, 23325–23333. doi:10.1016/s0021-9258(19)49466-0
Kinosita, K., Adachi, K., and Itoh, H. (2004). Rotation of F1-ATPase: How an ATP-driven molecular machine may work. Annu. Rev. Biophys. Biomol. Struct. 33, 245–268. doi:10.1146/annurev.biophys.33.110502.132716
Kinosita, K., Yasuda, R., Noji, H., and Adachi, K. (2000). A rotary molecular motor that can work at near 100% efficiency. Philos. Trans. R. Soc. Lond. B Biol. Sci. 355, 473–489. doi:10.1098/rstb.2000.0589
Kinosita, K., Yasuda, R., Noji, H., Ishiwata, S., and Yoshida, M. (1998). F1-ATPase: A rotary motor made of a single molecule. Cell 93, 21–24. doi:10.1016/s0092-8674(00)81142-3
Kohlbrenner, W. E., and Boyer, P. D. (1983). Probes of catalytic site cooperativity during catalysis by the chloroplast adenosine triphosphate and the adenosine triphosphate synthase. J. Biol. Chem. 258, 10881–10886. doi:10.1016/s0021-9258(17)44358-4
Kühlbrandt, W. (2019). Structure and mechanisms of F-type ATP synthases. Annu. Rev. Biochem. 88, 515–549. doi:10.1146/annurev-biochem-013118-110903
Lapashina, A. S., and Feniouk, B. A. (2018). ADP-inhibition of H+-FOF1-ATP synthase. Biochemistry. 83, 1141–1160. doi:10.1134/S0006297918100012
Larson, E. M., Umbach, A., and Jagendorf, A. T. (1989). Sulfite-stimulated release of [3H]ADP bound to chloroplast thylakoid ATPase. Biochimica Biophysica Acta (BBA) - Bioenergetics 973, 78–85. doi:10.1016/S0005-2728(89)80405-0
Läuger, P. (1984). Thermodynamic and kinetic properties of electrogenic ion pumps. Biochim. Biophys. Acta 779, 307–341. doi:10.1016/0304-4157(84)90015-7
Leslie, A. G., and Walker, J. E. (2000). Structural model of F1-ATPase and the implications for rotary catalysis. Philos. Trans. R. Soc. Lond. B Biol. Sci. 355, 465–471. doi:10.1098/rstb.2000.0588
Lindqvist, A., Berger, K., and Erlanson-Albertsson, C. (2008). Enterostatin up-regulates the expression of the beta-subunit of F(1)F(o)-ATPase in the plasma membrane of INS-1 cells. Nutr. Neurosci. 11, 55–60. doi:10.1179/147683008X301397
Lodeyro, A. F., Calcaterra, N. B., and Roveri, O. A. (2001). Inhibition of steady-state mitochondrial ATP synthesis by bicarbonate, an activating anion of ATP hydrolysis. Biochim. Biophys. Acta 1506, 236–243. doi:10.1016/s0005-2728(01)00221-3
Maldonado, E., Dreyfus, G., García, J. J., Gómez-Puyou, A., and de Gómez-Puyou, M. T. (1998). Unisite ATP hydrolysis by soluble Rhodospirillum rubrum F1-ATPase is accelerated by Ca2+. Biochim. Biophys. Acta 1363, 70–78. doi:10.1016/s0005-2728(97)00083-2
Martin, J. L., Ishmukhametov, R., Hornung, T., Ahmad, Z., and Frasch, W. D. (2014). Anatomy of F1-ATPase powered rotation. Proc. Natl. Acad. Sci. U. S. A. 111, 3715–3720. doi:10.1073/pnas.1317784111
Massart, F., Paolini, S., Piscitelli, E., Brandi, M. L., and Solaini, G. (2002). Dose-dependent inhibition of mitochondrial ATP synthase by 17 beta-estradiol. Gynecol. Endocrinol. 16, 373–377. doi:10.1080/gye.16.5.373.377
Meiss, E., Konno, H., Groth, G., and Hisabori, T. (2008). Molecular processes of inhibition and stimulation of ATP synthase caused by the phytotoxin tentoxin. J. Biol. Chem. 283, 24594–24599. doi:10.1074/jbc.M802574200
Mitchell, P. (1961). Coupling of phosphorylation to electron and hydrogen transfer by a chemi-osmotic type of mechanism. Nature 191, 144–148. doi:10.1038/191144a0
Moreno, A. J. M., Moreira, P. I., Custódio, J. B. A., and Santos, M. S. (2013). Mechanism of inhibition of mitochondrial ATP synthase by 17β-estradiol. J. Bioenerg. Biomembr. 45, 261–270. doi:10.1007/s10863-012-9497-1
Moyle, J., and Mitchell, P. (1975). Active/inactive state transitions of mitochondrial ATPase molecules influenced by Mg2+, anions and aurovertin. FEBS Lett. 56, 55–61. doi:10.1016/0014-5793(75)80110-4
Müller, M. L., and Taiz, L. (2002). Regulation of the lemon-fruit V-ATPase by variable stoichiometry and organic acids. J. Membr. Biol. 185, 209–220. doi:10.1007/s00232-001-0124-z
Murataliev, M. B., and Boyer, P. D. (1992). The mechanism of stimulation of MgATPase activity of chloroplast F1-ATPase by non-catalytic adenine-nucleotide binding. Acceleration of the ATP-dependent release of inhibitory ADP from a catalytic site. Eur. J. Biochem. 209, 681–687. doi:10.1111/j.1432-1033.1992.tb17336.x
Nakano, A., Kishikawa, J., Nakanishi, A., Mitsuoka, K., and Yokoyama, K. (2022). Structural basis of unisite catalysis of bacterial F0F1-ATPase. PNAS Nexus 1. doi:10.1093/pnasnexus/pgac116
Noji, H., and Ueno, H. (2022). How does F1-ATPase generate torque?: Analysis from cryo-electron microscopy and rotational catalysis of thermophilic F1. Front. Microbiol. 13, 904084. doi:10.3389/fmicb.2022.904084
Noji, H., Yasuda, R., Yoshida, M., and Kinosita, K. (1997). Direct observation of the rotation of F1-ATPase. Nature 386, 299–302. doi:10.1038/386299a0
Pacheco-Moisés, F., García, J. J., Rodríguez-Zavala, J. S., and Moreno-Sánchez, R. (2000). Sulfite and membrane energization induce two different active states of the Paracoccus denitrificans F0F1-ATPase. Eur. J. Biochem. 267, 993–1000. doi:10.1046/j.1432-1327.2000.01088.x
Pacheco-Moisés, F., Minauro-Sanmiguel, F., Bravo, C., and García, J. J. (2002). Sulfite inhibits the F1F0-ATP synthase and activates the F1F0-ATPase of Paracoccus denitrificans. J. Bioenerg. Biomembr. 34, 269–278. doi:10.1023/a:1020252401675
Papageorgiou, S., Melandri, A. B., and Solaini, G. (1998). Relevance of divalent cations to ATP-driven proton pumping in beef heart mitochondrial F0F1-ATPase. J. Bioenerg. Biomembr. 30, 533–541. doi:10.1023/a:1020528432609
Penefsky, H. S., and Cross, R. L. (1991). Structure and mechanism of FoF1-type ATP synthases and ATPases. Adv. Enzymol. Relat. Areas Mol. Biol. 64, 173–214. doi:10.1002/9780470123102.ch4
Petersen, J., Förster, K., Turina, P., and Gräber, P. (2012). Comparison of the H+/ATP ratios of the H+-ATP synthases from yeast and from chloroplast. Proc. Natl. Acad. Sci. U. S. A. 109, 11150–11155. doi:10.1073/pnas.1202799109
Pettersen, E. F., Goddard, T. D., Huang, C. C., Couch, G. S., Greenblatt, D. M., Meng, E. C., et al. (2004). UCSF Chimera--a visualization system for exploratory research and analysis. J. Comput. Chem. 25, 1605–1612. doi:10.1002/jcc.20084
Pick, U., and Weiss, M. (1988). A light-dependent dicyclohexylcarbodiimide-sensitive Ca-ATPase activity in chloroplasts which is not coupled to proton translocation. Eur. J. Biochem. 173, 623–628. doi:10.1111/j.1432-1033.1988.tb14044.x
Rottenberg, H. (1983). Uncoupling of oxidative phosphorylation in rat liver mitochondria by general anesthetics. Proc. Natl. Acad. Sci. U. S. A. 80, 3313–3317. doi:10.1073/pnas.80.11.3313
Saita, E., Suzuki, T., Kinosita, K., and Yoshida, M. (2015). Tentoxin-induced binding of adenine nucleotides to soluble spinach chloroplast coupling factor 1. Biochim. Biophys. Acta 545, 415–423. doi:10.1016/0005-2728(79)90150-6
Sakaki, N., Shimo-Kon, R., Adachi, K., Itoh, H., Furuike, S., Muneyuki, E., et al. (2005). One rotary mechanism for F1-ATPase over ATP concentrations from millimolar down to nanomolar. Biophys. J. 88, 2047–2056. doi:10.1529/biophysj.104.054668
Saroussi, S., and Nelson, N. (2009). The little we know on the structure and machinery of V-ATPase. J. Exp. Biol. 212, 1604–1610. doi:10.1242/jeb.025866
Sasaki, S., Oba, K., Kodera, Y., Itakura, M., and Shichiri, M. (2022). ANGT_HUMAN[448-462], an anorexigenic peptide identified using plasma peptidomics. J. Endocr. Soc. 6, bvac082. doi:10.1210/jendso/bvac082
Selman, B. R., and Selman-Reimer, S. (1979). Tentoxin-induced adenine nucleotide exchange with soluble and thylakoid membrane-bound chloroplast coupling factor 1. FEBS Lett. 97, 301–304. doi:10.1016/0014-5793(79)80107-6
Shao, E., and Forgac, M. (2004). Involvement of the nonhomologous region of subunit A of the yeast V-ATPase in coupling and in vivo dissociation. J. Biol. Chem. 279, 48663–48670. doi:10.1074/jbc.M408278200
Shigalowa, T., Lehmann, U., Krevet, M., and Strotmann, H. (1985). Transient stimulation of light-triggered ATP hydrolysis by preillumination of chloroplasts in the presence of ATP. Biochimica Biophysica Acta (BBA) - Bioenergetics 809, 57–65. doi:10.1016/0005-2728(85)90167-7
Shoshan, V., and Shavit, N. (1979). ATP synthesis and hydrolysis in chloroplast membranes. Differential inhibition by antibodies to chloroplast coupling factor 1. Eur. J. Biochem. 94, 87–92. doi:10.1111/j.1432-1033.1979.tb12874.x
Sielaff, H., Duncan, T. M., and Börsch, M. (2018). The regulatory subunit ε in Escherichia coli FOF1-ATP synthase. Biochim. Biophys. Acta. Bioenerg. 1859, 775–788. doi:10.1016/j.bbabio.2018.06.013
Sigalat, C., Pitard, B., and Haraux, F. (1995). Proton coupling is preserved in membrane-bound chloroplast ATPase activated by high concentrations of tentoxin. FEBS Lett. 368, 253–256. doi:10.1016/0014-5793(95)00664-u
Sobti, M., Ishmukhametov, R., Bouwer, J. C., Ayer, A., Suarna, C., Smith, N. J., et al. (2019). Cryo-EM reveals distinct conformations of E. coli ATP synthase on exposure to ATP. Elife 8, e43864. doi:10.7554/eLife.43864
Sobti, M., Walshe, J. L., Wu, D., Ishmukhametov, R., Zeng, Y. C., Robinson, C. V., et al. (2020). Cryo-EM structures provide insight into how E. coli F1Fo ATP synthase accommodates symmetry mismatch. Nat. Commun. 11, 2615. doi:10.1038/s41467-020-16387-2
Soga, N., Kimura, K., Kinosita, K., Yoshida, M., and Suzuki, T. (2017). Perfect chemomechanical coupling of FoF1-ATP synthase. Proc. Natl. Acad. Sci. U. S. A. 114, 4960–4965. doi:10.1073/pnas.1700801114
Strid, A., and Nyrén, P. (1989). Division of divalent cations into two groups in relation to their effect on the coupling of the F0F1-ATPase of Rhodospirillum rubrum to the protonmotive force. Biochemistry 28, 9718–9724. doi:10.1021/bi00451a027
Strotmann, H., Kiefer, K., and Altvater-Mackensen, R. (1986). Equilibration of the ATPase reaction of chloroplasts at transition from strong light to weak light. Biochimica Biophysica Acta (BBA) - Bioenergetics 850, 90–96. doi:10.1016/0005-2728(86)90012-5
Suzuki, T., Murakami, T., Iino, R., Suzuki, J., Ono, S., Shirakihara, Y., et al. (2003). F0F1-ATPase/synthase is geared to the synthesis mode by conformational rearrangement of epsilon subunit in response to proton motive force and ADP/ATP balance. J. Biol. Chem. 278, 46840–46846. doi:10.1074/jbc.M307165200
Toyabe, S., Watanabe-Nakayama, T., Okamoto, T., Kudo, S., and Muneyuki, E. (2011). Thermodynamic efficiency and mechanochemical coupling of F1-ATPase. Proc. Natl. Acad. Sci. U. S. A. 108, 17951–17956. doi:10.1073/pnas.1106787108
Tsunoda, S. P., Rodgers, A. J., Aggeler, R., Wilce, M. C., Yoshida, M., and Capaldi, R. A. (2001). Large conformational changes of the epsilon subunit in the bacterial F1F0 ATP synthase provide a ratchet action to regulate this rotary motor enzyme. Proc. Natl. Acad. Sci. U. S. A. 98, 6560–6564. doi:10.1073/pnas.111128098
Tucker, W. C., Schwarz, A., Levine, T., Du, Z., Gromet-Elhanan, Z., Richter, M. L., et al. (2004). Observation of calcium-dependent unidirectional rotational motion in recombinant photosynthetic F1-ATPase molecules. J. Biol. Chem. 279, 47415–47418. doi:10.1074/jbc.C400269200
Turina, P., and Capaldi, R. A. (1994). ATP binding causes a conformational change in the gamma subunit of the Escherichia coli F1ATPase which is reversed on bond cleavage. Biochemistry 33, 14275–14280. doi:10.1021/bi00251a040
Turina, P., Giovannini, D., Gubellini, F., and Melandri, B. A. (2004). Physiological ligands ADP and Pi modulate the degree of intrinsic coupling in the ATP synthase of the photosynthetic bacterium Rhodobacter capsulatus. Biochemistry 43, 11126–11134. doi:10.1021/bi048975+
Turina, P., Petersen, J., and Gräber, P. (2016). Thermodynamics of proton transport coupled ATP synthesis. Biochim. Biophys. Acta 1857, 653–664. doi:10.1016/j.bbabio.2016.02.019
Urbani, A., Giorgio, V., Carrer, A., Franchin, C., Arrigoni, G., Jiko, C., et al. (2019). Purified F-ATP synthase forms a Ca2+-dependent high-conductance channel matching the mitochondrial permeability transition pore. Nat. Commun. 10, 4341. doi:10.1038/s41467-019-12331-1
Van Walraven, H. S., Strotmann, H., Schwarz, O., and Rumberg, B. (1996). The H+/ATP coupling ratio of the ATP synthase from thiol-modulated chloroplasts and two cyanobacterial strains is four. FEBS Lett. 379, 309–313. doi:10.1016/0014-5793(95)01536-1
Vasilyeva, E. A., Minkov, I. B., Fitin, A. F., and Vinogradov, A. D. (1982). Kinetic mechanism of mitochondrial adenosine triphosphatase. Inhibition by azide and activation by sulphite. Biochem. J. 202, 15–23. doi:10.1042/bj2020015
Vasilyeva, E., and Forgac, M. (1998). Interaction of the clathrin-coated vesicle V-ATPase with ADP and sodium azide. J. Biol. Chem. 273, 23823–23829. doi:10.1074/jbc.273.37.23823
Vink, R., Bendall, M. R., Simpson, S. J., and Rogers, P. J. (1984). Estimation of H+ to adenosine 5’-triphosphate stoichiometry of Escherichia coli ATP synthase using 31P NMR. Biochemistry 23, 3667–3675. doi:10.1021/bi00311a015
Walker, J. E. (1994). The regulation of catalysis in ATP synthase. Curr. Opin. Struct. Biol. 4, 912–918. doi:10.1016/0959-440x(94)90274-7
Watt, I. N., Montgomery, M. G., Runswick, M. J., Leslie, A. G. W., and Walker, J. E. (2010). Bioenergetic cost of making an adenosine triphosphate molecule in animal mitochondria. Proc. Natl. Acad. Sci. U. S. A. 107, 16823–16827. doi:10.1073/pnas.1011099107
Zhang, S., Letham, D. D., and Jagendorf, A. T. (1993). Inhibition of thylakoid ATPase by venturicidin as an indicator of CF1-CF0 interaction. Plant Physiol. 101, 127–133. doi:10.1104/pp.101.1.127
Zheng, J., and Ramirez, V. D. (2000). Inhibition of mitochondrial proton F0F1-ATPase/ATP synthase by polyphenolic phytochemicals. Br. J. Pharmacol. 130, 1115–1123. doi:10.1038/sj.bjp.0703397
Zheng, J., and Ramirez, V. D. (1999). Purification and identification of an estrogen binding protein from rat brain: Oligomycin sensitivity-conferring protein (OSCP), a subunit of mitochondrial F0F1-ATP synthase/ATPase. J. Steroid Biochem. Mol. Biol. 68, 65–75. doi:10.1016/S0960-0760(98)00161-7
Zimmermann, E., and Seifert, U. (2012). Efficiencies of a molecular motor: A generic hybrid model applied to the F 1 -ATPase. New J. Phys. 14, 103023. doi:10.1088/1367-2630/14/10/103023
Keywords: ATP synthase, H+/ATP, coupling ratio, unisite catalysis, ATP-Pi exchange, sulfite, 17β-estradiol, energy homeostasis
Citation: Turina P (2022) Modulation of the H+/ATP coupling ratio by ADP and ATP as a possible regulatory feature in the F-type ATP synthases. Front. Mol. Biosci. 9:1023031. doi: 10.3389/fmolb.2022.1023031
Received: 19 August 2022; Accepted: 12 September 2022;
Published: 05 October 2022.
Edited by:
Marialaura Marchetti, University of Parma, ItalyReviewed by:
Boris Feniouk, Lomonosov Moscow State University, RussiaCopyright © 2022 Turina. This is an open-access article distributed under the terms of the Creative Commons Attribution License (CC BY). The use, distribution or reproduction in other forums is permitted, provided the original author(s) and the copyright owner(s) are credited and that the original publication in this journal is cited, in accordance with accepted academic practice. No use, distribution or reproduction is permitted which does not comply with these terms.
*Correspondence: Paola Turina, cGFvbGEudHVyaW5hQHVuaWJvLml0