- 1Department of Otolaryngology, The Second Affiliated Hospital of Nanchang University, NanChang, China
- 2Jiangxi Province Key Laboratory of Molecular Medicine, Nanchang, China
Ferroptosis is a newly discovered type of programmed cell death, which is closely related to the imbalance of iron metabolism and oxidative stress. Ferroptosis has become an important research topic in the fields of cardiomyopathy, tumors, neuronal injury disorders, and ischemia perfusion disorders. As an important part of non-coding RNA, microRNAs regulate various metabolic pathways in the human body at the post-transcriptional level and play a crucial role in the occurrence and development of many diseases. The present review introduces the mechanisms of ferroptosis and describes the relevant pathways by which microRNAs affect cardiomyopathy, tumors, neuronal injury disorders and ischemia perfusion disorders through regulating ferroptosis. In addition, it provides important insights into ferroptosis-related microRNAs, aiming to uncover new methods for treatment of the above diseases, and discusses new ideas for the implementation of possible microRNA-based ferroptosis-targeted therapies in the future.
Introduction
Ferroptosis is a newly discovered programmed cell death mechanism with characteristics that are different from those of autophagy, apoptosis and necrosis. Several studies have demonstrated that ferroptosis is involved in the occurrence and development of cardiomyopathy, tumors, neuronal injury disorders, and ischemia perfusion disorders (Masaldan et al., 2019; Jiang et al., 2021; Van Coillie et al., 2022). MicroRNAs are a class of endogenous non-coding small RNA molecules, generally 21–25 nucleotides in length, which regulate various metabolic pathways in the human body at the post-transcriptional and translational levels, including regulation of tumor cell growth and induction of chemotherapy resistance (Ambros, 2001). Noncoding RNAs play a significant role in ferroptosis of various cells (Zhang X. et al., 2020; Zhi et al., 2021; Zuo et al., 2022). Previous reviews have mainly focused on the role of noncoding RNAs in cancer through regulating ferroptosis (Valashedi et al., 2022), but the role of microRNAs in other diseases through regulating ferroptosis has not been summarized.
The present review first discusses the mechanisms of ferroptosis and then summarizes the diseases and related pathways regulated by microRNAs through regulating ferroptosis. MicroRNAs have great potential as therapeutic targets. This report provides reference information for more in-depth studies of microRNAs in the field of ferroptosis.
Overview of ferroptosis
In 2012, Dixon et al. first used the concept of ferroptosis to describe the mode of cell death, which is caused by the accumulation of lipid peroxides (Dixon et al., 2012).
Ferroptosis is an iron-dependent mode of cell death that involves lipid reactive oxygen species (ROS) accumulation (Stockwell et al., 2017). The mechanism of its occurrence has been partially uncovered (Jhelum et al., 2020). First, the overloaded iron ions generate a lot of free hydroxyl groups via the Fenton reaction. Iron is a vital trace element involved in many physiological processes in the human body. Excess iron, however, increases the oxidative sensitivity of cells (Chen et al., 2019). Cells take up Fe3+ primarily through the transferrin receptor protein 1 complex (Gao et al., 2015). The six-transmembrane epithelial antigen of the prostate 3 (Steap3) reduces intracellular Fe3+ to Fe2+. Ferroportin (FPN), also known as solute carrier family 40 member 1 (SLC40A1), is involved in the regulation of iron balance by expelling Fe2+ out of the cells (Geng et al., 2018). Overexpression of ferritin heavy chain1 (FTH1) inhibits erastin-induced ferroptosis by regulating Fe2+ (Gao et al., 2016; Hou et al., 2016).
Next, lipid peroxidation induction leads to lipid metabolism disorders, and the accumulation of lipid oxides and ROS is responsible for ferroptosis. Fe2+ overload causes a series of oxidative stress reactions in the cell, resulting in the destruction of the nucleus, membrane, organelles, and proteins. Esterified polyunsaturated fatty acids (PUFAs) are the most common substrates in lipid peroxidation (Kagan et al., 2017). Acyl-CoA synthetase long-chain family member 4 (ACSL4) is involved in the biosynthesis and reassembly of PUFAs on the cell membrane (Chen et al., 2021b). Lysophosphatidylcholine acyltransferase 3 then mediates PUFA activation to induce ferroptosis (Doll et al., 2017).
Finally, System Xc− - glutathione - glutathione peroxidase 4 (Xc−-GSH-GPX4) is considered a crucial antioxidant axis in ferroptosis. Xc− complex is a cysteine-glutamate reverse transporter composed of two-subunit solute carrier family 7 member 11 (SLC7A11/xCT) along with solute carrier family 3 member 2 (SLC3A2) (Koppula et al., 2018). The main function of Xc− is to regulate the transport balance of glutamate and cysteine and participate in the synthesis of GSH (Koppula et al., 2021). GSH is a cofactor for GPX4. It protects cells from oxidative damage. GPX4 is a selenoprotein that neutralizes toxic lipid peroxides and inhibits ferroptosis (Forcina and Dixon, 2019; Weaver and Skouta, 2022). GPX4 inactivation is a pivotal condition for the occurrence of ferroptosis (Jhelum et al., 2020). Large amounts of GSH are depleted, resulting in decreased glutathione peroxidase 4 (GPX4) activity. Certain intracellular components, including oncoprotein activating transcription factor 4 (ATF4), nuclear factor erythroid 2-like factor 2 (NFE2L2/NRF2), and Beclin-1, can also regulate iron by affecting systems Xc− and GSH death level (Habib et al., 2015; Song et al., 2018). In addition, the classic tumor suppressor gene P53 affects the occurrence and development of various diseases, such as tumors, by regulating GPX4-dependent and GPX4-independent ferroptosis pathways (Liu and Gu, 2022).
At present, numerous genetic hallmarks and protein hallmarks of ferroptosis are available for detection, but their specificity remains limited (Chen et al., 2021a).
Other auxiliary evidence, such as detection of cell activity, iron levels, GSH levels in cells and tissues, ROS and ROS product content, malondialdehyde (MDA), and mitochondrial membrane potential (MMP) is also often used to demonstrate the occurrence of ferroptosis. Moreover, changes in cell morphology, particularly mitochondrial morphology, observed under transmission electron microscopy, are the main features that distinguish ferroptosis from other forms of programmed cell death, such as apoptosis, autophagy, and necrosis. Mitochondrial shrinkage, high membrane density, diminished or absent cristae, and exterior membrane rupture are common in cells undergoing ferroptosis (Agmon and Stockwell, 2017; Stockwell, 2022).
More research on ferroptosis has continued to accumulate over the last few years. Ferroptosis has been shown to take part in the development of various diseases, such as tumors, cardiovascular diseases, and autoimmune diseases (Nguyen et al., 2020; Guo et al., 2022; Lai et al., 2022). Next, the role of microRNAs was explored in ferroptosis separately from microRNA-involved cardiomyopathy, tumors, nervous system diseases, and ischemia perfusion disorders.
The role of microRNA in cardiomyopathy via regulating ferroptosis
As mentioned earlier, GPX4 is critical for the regulation of ferroptosis (Ursini et al., 2022). Zhuang et al. used an ischemia-reperfusion (I/R) rat model and cardiac fibrosis cell model induced by angiotensin II to determine that miR-375–3p promotes ferroptosis and accelerates cardiac fibrosis by inhibiting GPX4 (Zhuang et al., 2022). Fan et al. have showed that inhibition of miR-15a-5p decreases ferroptosis through GPX4 and thus alleviates myocardial injury in acute myocardial infarction (Fan et al., 2021). Inhibition of miR-1224 has also been found to alleviate hypoxia/reoxygenation myocardial injury by upregulating GPX4 (Li G. et al., 2021). GPX4 is a critical target in ferroptosis. MiR-375-3p, miR-15a-5p or miR-1224 inhibition may protect cardiomyocytes and alleviate myocardial injury by increasing GPX4 and decreasing ferroptosis. As a part of the Xc− transporter, SLC7A11 also plays a significant role in the antioxidant system (Jyotsana et al., 2022). The study of Liu et al. inhibited cardiac fibroblast-derived exon-miR-23a-3p by using the exosome inhibitor GW4869, and inhibition of miR-23a-3p resulted in upregulation of SLC7A11, thereby reducing ferroptosis in H9c2 cardiomyocytes and preventing continued development of atrial flutter (Liu D. et al., 2022). It suggests that miR-23a-3p inhibition increases intracellular cystine and GSH levels, thereby neutralizing ROS and treating atrial fibrillation. Glutaminase 2 (GLS2) can cause ROS accumulation through mitochondria by accelerating glutamate formation (Matés et al., 2020). After myocardial infarction, cardiomyocytes often undergo cell death and pathological remodeling, which easily lead to heart failure (Duan, 2020). Zhou et al. have suggested that miR-190a-5p inhibits cardiomyocyte ferroptosis by inhibiting GLS2 and decreases the levels of ROS, MDA, and Fe2+ in H9c2 cells, thus playing a protective role in myocardial infarction (Zhou et al., 2021). MiR-190a-5p reduces lipid peroxidation by inhibiting GLS2 mRNA, thereby inhibiting ferroptosis and lowering the risk of myocardial infarction. Autophagy related protein 5 (ATG5) has been suggested to inhibit ferroptosis by inhibiting autophagy (Liang et al., 2022). Tang et al. have found that overexpression of miR-30d promotes ferroptosis after myocardial infarction by targeting ATG5, thus inhibiting autophagy in cardiomyocytes (Tang et al., 2020). Therefore, crosstalk may exist between ferroptosis and autophagy. The incidence of cardiovascular disease is high worldwide. Decreasing cardiomyocyte death and repairing damaged cardiac tissue are urgent clinical needs (Xu S. et al., 2021). Ferroptosis decreases reduces the overall cardioprotective effect of ischemia/reperfusion (I/R) injury. Ferroptosis suppression decreases inflammation and limits the extent of left ventricular remodeling after I/R injury (Komai et al., 2022).
Common clinical cardiovascular diseases include atherosclerosis, myocardial infarction, heart failure, and arrhythmia. Ferroptosis plays a vital role in the development of cardiovascular disease (Guo et al., 2022). The mechanism of microRNA in ferroptosis occurs through regulation of the antioxidant system and lipid oxidation. Most existing studies have shown that microRNA overexpression damages cardiomyocytes by promoting ferroptosis. However, because microRNAs have diverse functions, further research is needed to reveal more details. Table 1 shows microRNAs that affect cardiomyopathy through regulating ferroptosis.
The role of microRNA in tumors via regulating ferroptosis
GPX4 remains an important target of microRNAs in tumor diseases (Gao et al., 2022). The experiments by Xu et al. showed that miR-15a overexpression can inhibit cell proliferation, increase the release of lactate dehydrogenase, MDA, Fe2+, and ROS, and then destroy MMP by inhibiting GPX4 in prostate cancer cells (Xu P. et al., 2022). In colorectal cancer cells, Liu et al. reported that miR-15a-3p overexpression can also promote ferroptosis by inhibiting GPX4 (Liu L. et al., 2022). Xu et al. found that miR-1287–5p still targets GPX4 to promote ferroptosis in osteosarcoma cells (Xu Z. et al., 2021). Deng et al. study suggested that miR-324–3p targets inhibition of GPX4, promotes ferroptosis in lung adenocarcinoma cells, and reverses their resistance to cisplatin (Deng et al., 2021). These microRNAs directly bound to the 3′-UTR of GPX4 mRNA and inhibited its expression, causing ROS accumulation in tumor cells and acting as tumor suppressor genes. Inhibition of the ATF4-HSPA5-GPX4 pathway reduces GPX4 levels and induces ferroptosis. Loss of ATF4 leads to increased ferroptosis (Chen et al., 2017; Zhu et al., 2017). The study by Bai et al. reported that miR-214–3p targets ATF4 to promote ferroptosis in hepatoma cells (Bai et al., 2020). Gomaa et al. found that overexpression of miR-4715–3p can inhibit aurora kinase A (AURKA) and GPX4, inducing ferroptosis in upper gastrointestinal adenocarcinoma cells (Gomaa et al., 2019). GPX4 content is a key factor in tumor ferroptosis, and these microRNAs have been shown to promote ferroptosis in various tumor cells by inhibiting target genes involved in GPX4 synthesis. Ferroptosis suppressor protein 1 (FSP1) exerts antioxidant effects parallel to GPX4, and FSP1-CoQ10-NAD(P)H is another pathway that inhibits ferroptosis (Bersuker et al., 2019; Doll et al., 2019). One experiment showed that exosomal miR-4443 targets methyltransferase 3 (METT3) to regulate FSP1 expression, thereby inhibiting ferroptosis in non-small cell lung cancer (Song et al., 2021). In the absence of GPX4, FSP1 can be used as an oxidoreductase to inhibit ROS and alleviate ferroptosis.
SLC7A11 is widely expressed in various tumor tissues, and plays a significant role in inhibiting ferroptosis during the occurrence and development of tumors by controlling cysteine transport (Tang et al., 2022). Sun et al. found that the miR-34c-3p/SLC7A11 axis can potentiate erastin-induced ferroptosis in oral squamous cell carcinoma (Sun et al., 2022). Yadav et al. reported that overexpression of miR-5096 can promote ferroptosis by targeting SLC7A11 and inhibit breast cancer cell growth (Yadav et al., 2021). The study by Ni et al. showed that miR-375/SLC7A11 inhibits gastric cancer stem cells by triggering ferroptosis. They believe that miR-375 can reduce the stemness of gastric cancer cells by inducing ferroptosis (Ni et al., 2021). SLC3A2, like SLC11A7, is an important functional subunit of the Xc− system (Liu M. et al., 2022). Hu et al. found that exosomal miR-142–3p secreted by hepatocellular carcinoma cells targets SLC3A2 to promote ferroptosis in MI-type macrophages, thus, accelerating the development of hepatocellular carcinoma (Hu et al., 2022). The microRNAs mentioned above reduced GSH levels by regulating cystine transport into tumor cells by targeting SLC7A11 and SLC3A2. Dickkopf-related protein 1 (DKK1) inhibits the occurrence of ferroptosis and protects cells from ferroptosis by enhancing the expression of SLC7A11 (Wu M. et al., 2022). Liao et al. found that miR-130b-3p targets DKK1 to inhibit ferroptosis in melanoma cells (Liao et al., 2021). MicroRNAs essentially regulated tumor ferroptosis via the antioxidant system, whether by regulating GPX4, FSP1, or GSH.
ACSL4 can promote lipid peroxidation of PUFAs to promote ferroptosis, and is also an important target of many microRNAs (Chen et al., 2021b; Liu et al., 2021). Bao et al. demonstrated that miR-670–3p can inhibit ferroptosis in glioblastoma cells by targeting ACSL4. Furthermore, miR-670–3p inhibitor-treated U87MG and A172 cells increase chemosensitivity to temozolomide (Bao et al., 2021). The study by Ma et al. showed that miR-424–5p targeting ACSL4 negatively regulates ferroptosis in ovarian cancer cells (Ma et al., 2021). They inhibited ROS production from PUFAs by targeting ACSL4 mRNA, thus resulting in ferroptosis inhibition.
Solute carrier family 1 member 5 (SLC1A5) transports glutamine into the cell during ferroptosis, and may increase cell sensitivity to ferroptosis (Xu F. et al., 2022; Zhu D. et al., 2022). Luo et al. reported that overexpression of miR-137, which results in decreased glutamine and MDA levels, can inhibit ferroptosis in melanoma cells by targeting SLC1A5 (Luo et al., 2018). Aspartate aminotransaminase (GOT1) is an enzyme involved in glutamate metabolism, which catalyzes the production of α-ketoglutarate (Kremer et al., 2021). Zhang et al. have shown that overexpression of miR-9 inhibits ferroptosis in melanoma cells by directly binding to the 3′-UTR of GOT1 (Zhang et al., 2018). These findings suggest that miR-137 and miR-9 speed up the progression of melanoma by inhibiting ferroptosis through reducing lipid peroxidation.
Iron-responsive element-binding protein 2 (IREB2) is related to intracellular iron ion concentration, and suppression of IREB2 can inhibit the level of Fe2+, thus inhibiting ferroptosis (Li et al., 2022). Fan et al. showed that miR-19a inhibits ferroptosis in colorectal cancer cells HT29 by inhibiting IREB2 (Fan et al., 2022). Transferrin (TF) mediates Fe2+ entry into the cells, and TF blocking can reduce Fe2+ overload and inhibit ferroptosis (Pandrangi et al., 2022). Zheng et al. found that miR-545 inhibition can reduce ferroptosis in rectal cancer cells by regulating TF (Sixin Zheng, Lingling Hu, Qingwen Song et al., 2021). Intracellular Fe2+ can transport Fe2+ out of the cells via FPN1/SLC40A1. FPN participates in ferroptosis by regulating intracellular Fe2+ concentration (Hao et al., 2021; Wang et al., 2021; Pandrangi et al., 2022). Wei et al. reported that miR-302a-3p can strengthen ferroptosis in non-small cell lung cancer by targeting FPN (Wei et al., 2021). Zhu et al. showed that miR-4735–3p targets SLC40A1 to promote ferroptosis in clear cell renal cell carcinoma, thereby inhibiting tumor proliferation (Zhu C. et al., 2022). Ferroptosis is characterized by iron overload. Different microRNAs have different effects on iron metabolism target genes, resulting in ferroptosis regulation.
Tumor necrosis factor-α-induced protein 8 (TNFAIP8/TIPE) can participate in ferroptosis by inhibiting p53. One prior study showed that miR-539 overexpression can promote ferroptosis in colorectal cancer cells by inhibiting TIPE (Yang et al., 2021). Two studies by Tomita et al. demonstrated that miR-7-5p knockdown can reduce radioresistance in radioresistant cancer cells by regulating ferroptosis through changes in Fe2+ content (Tomita et al., 2019; Tomita et al., 2021). Tumor cells change their own microenvironment to achieve continuous proliferation (Xu et al., 2020), and microRNAs affect the proliferation of various tumor cells through the regulation of ferroptosis. The function of microRNA can be accomplished by the degradation or the translation inhibition of targeted mRNA after binding to the 3′-UTR region. However, this regulatory effect is complex, in that it presents two aspects of tumor-promoting and tumor-suppression. Table 2 lists microRNAs that affect tumors through regulating ferroptosis.
The role of microRNA in neuronal injury disorder via regulating ferroptosis
FSP1 acts as a complementary antioxidant pathway and is also a target gene of microRNA-672–3p. Inhibition of miR-672–3p was reported to inhibit ferroptosis by upregulating FSP1, thereby promoting neural repair in spinal cord injury (Wang F. et al., 2022). The SLC7A11/GPX4 signaling pathway is a major antioxidant pathway in ferroptosis-induced nerve injury (Fu et al., 2022). Wang et al. reported that inhibition of miR-378a-3p reverses lead exposure-induced ferroptosis by targeting SLC7A11 in HT22 cells (Wang W. et al., 2022). The two microRNAs mentioned above induced ferroptosis by inhibiting key molecules in the antioxidant pathway. It suggests that blocking them can reduce nerve cell death and alleviate specific neuronal injury disorders.
BTB and CNC homology 1(Bach1) is an oxidative stress-responsive transcription factor in ferroptosis, which promotes it by inhibiting the antioxidant system (GSH-GPX4 and FSP1-CoQ10 pathways) (Nishizawa et al., 2022). Li et al. showed that miR-194-loaded mesenchymal exosomes can inhibit neurovascular endothelial cell ferroptosis by targeting Bach1, resulting in neuroprotection (Li X. et al., 2021). Prostaglandin peroxidase synthase-2(Ptgs2) is a regulatory gene in ferroptosis lipid oxidation (Macías-Rodríguez et al., 2020). Xiao et al. reported that overexpression of miR-212–5p overexpression can inhibit ferroptosis in neuronal cells by inhibiting Ptgs2, thereby attenuating traumatic brain injury (Xiao X. et al., 2019). Overall, miR-194 and miR-212-5p reduced ROS production or increased ROS decomposition by targeting key lipid peroxidation targets.
FTH1, an important subunit of ferritin, plays a crucial role in Fe2+ metabolism as an iron chelator (Muhoberac and Vidal, 2019). Previous studies have shown that inhibiting neurotransmitters such as glutamate A may help alleviate movement disorders (Tugan Yildiz and Tuncel Berktas, 2021). Li et al. reported that miR-335 enhances ferroptosis in vitro and in vivo models of Parkinson’s disease by degrading FTH1 (Xinrong Li et al., 2021). The pathogenesis of stroke is complex and is thought to potentially be related to glutamate-induced oxidative stress (Wu and Prentice, 2021). It was confirmed that exosomal miR-137 can inhibit oxyhemoglobin-induced ferroptosis in SH-SY5Y cells, thereby initiating protection (Li et al., 2020). Studies have shown that iron ion imbalance, oxidative stress, and abnormal glutamate have important effects on ferroptosis and nerve damage. Therefore, ferroptosis in neurological diseases is increasingly being studied. Studies have shown that the roles of microRNAs in ferroptosis of neurological diseases are involved in the complex of bidirectional regulation of neurological diseases (Wu et al., 2018). Table 3 lists microRNAs that affect neuronal injury disorder through regulating ferroptosis.
The role of microRNA in ischemia perfusion disorders via regulating ferroptosis
Ferroptosis is an important mechanism leading to I/R injury in multiple organs (Chen Y. et al., 2021). GPX4 and SLC7A11 are key ferroptosis enzymes that act as target genes for multiple microRNAs in ischemic perfusion disease. Cell and animal experiments by, Zhou et al. demonstrated that miR-214–3p inhibition inhibits ferroptosis through GPX4, thereby reducing renal tubular damage (Zhou et al., 2022). Ding et al. reported that miR-182–5p and miR-378a-3p contribute to the activation of ferroptosis in I/R renal injury by inhibiting GPX4 and SLC7A11 (Ding et al., 2020). Zhang et al. showed that miR-30b-5p targets paired box protein 3 (PAX3) and SLC7A11 to enhance trophoblast ferroptosis, and that miR-30b-5p inhibition alleviates symptoms in a rat model (Zhang H. et al., 2020). GPX4 and SLC7A11 are still important microRNA target genes in ischemic perfusion disorders. This is related to GPX4’s critical antioxidant role in ferroptosis.
Steap3 is involved in ferroptosis as a key regulator of iron metabolism (Yan et al., 2021). A prior study demonstrated that miR-124–3p reduces the degree of ferroptosis in HO-1-modified bone marrow mesenchymal stem cells by inhibiting Steap3, alleviating the risk of hepatic I/R injury (Wu L. et al., 2022). Nuclear factor erythroid 2-related factor 2/heme oxygenase 1 (Nrf2/HO-1) signaling axis inhibits ROS generation and prevents oxidative stress damage (Yan et al., 2021). Tao et al. reported that miR-3587 inhibition could upregulates HO-1 by regulating HMOX1, thereby attenuating I/R-induced ferroptosis in kidney tissue (Tao et al., 2021). MiR3587 inhibition increases HO-1, a key antioxidant that reduces intracellular ROS and ferroptosis.
Xiao et al. found that miR-17–92 overexpression can affect ACSL4 expression by targeting tumor necrosis factor, alpha-induced protein 3(A20), thereby reducing ferroptosis in endothelial cells (Xiao F. J. et al., 2019). Liu et al. showed that miR-132 overexpression can accelerate the progression of atherosclerosis by reducing the level MMP, increasing ROS production, and promoting ferroptosis (Zexin et al., 2022). Ferroptosis is involved in the ischemic injury of various organs and tissues (von Samson-Himmelstjerna et al., 2022). Existing studies have shown that the mechanism through which microRNAs act on ischemic perfusion diseases mainly involve promotion of ferroptosis. Therefore, interventions may potentially target microRNA to achieve protection of target organs. Table 4 lists microRNAs that affect ischemia perfusion disorders through regulating ferroptosis.
Summary and outlook
Many studies have confirmed that microRNAs are involved in the occurrence of cardiomyopathy, tumors, neuronal injury disorder and ischemia perfusion disorders by promoting or inhibiting ferroptosis (Li N. et al., 2021; Maslov et al., 2022; Zuo et al., 2022). Figure 1 shows their relevant mechanisms. A significant number of studies have demonstrated that microRNAs can participate in the ferroptosis process through key targets, such as GPX4, SLC7A11, and ACSL4 (Chen et al., 2021b). Most of the current microRNA research explores its impact on a key target in the ferroptosis pathway alone, and does not explore the mechanism of action related to ferroptosis. The mechanism of microRNA’s impact on disease through regulating ferroptosis remains largely unexplored. Therefore, it is still necessary to further analyze the relevant mechanisms of microRNA from the aspects of metabolic pathways and epigenetic modifications in order to be able to, intervene in the development of diseases.
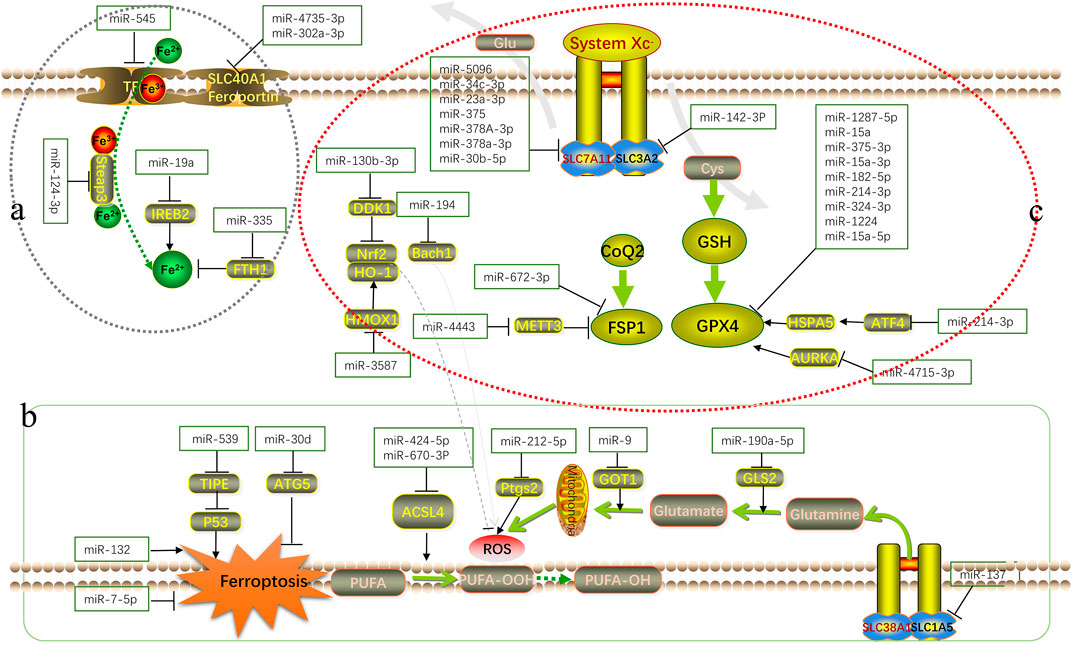
FIGURE 1. Schematic overview of the mechanism of microRNA in ferroptosis. MicroRNAs regulate various biological processes in the occurrence and development of ferroptosis by interfering with iron metabolism ((A): accumulation process of intracellular Fe2+), lipid peroxidation ((B): accumulation process of ROS and other peroxidation products) and antioxidant systems ((C): antioxidant axis dominated by Xc−-GSH-GPX4).
Author contributions
LG and QZ contributed to the preparation and writing of the manuscript. YL contributed to the editing of the manuscript.
Funding
The study was supported by the National Natural Science Fund of China (grant No. 81760184 and 82060185) and Natural Science Fund of Jiangxi Province (grant No. 20192ACB20027).
Conflict of interest
The authors declare that the research was conducted in the absence of any commercial or financial relationships that could be construed as a potential conflict of interest.
Publisher’s note
All claims expressed in this article are solely those of the authors and do not necessarily represent those of their affiliated organizations, or those of the publisher, the editors and the reviewers. Any product that may be evaluated in this article, or claim that may be made by its manufacturer, is not guaranteed or endorsed by the publisher.
References
Agmon, E., and Stockwell, B. R. (2017). Lipid homeostasis and regulated cell death. Curr. Opin. Chem. Biol. 39, 83–89. doi:10.1016/j.cbpa.2017.06.002
Bai, T., Liang, R., Zhu, R., Wang, W., Zhou, L., and Sun, Y. (2020). MicroRNA-214-3p enhances erastin-induced ferroptosis by targeting ATF4 in hepatoma cells. J. Cell Physiol. 235 (7-8), 5637–5648. doi:10.1002/jcp.29496
Bao, C., Zhang, J., Xian, S.-Y., and Chen, F. (2021). MicroRNA-670-3p suppresses ferroptosis of human glioblastoma cells through targeting ACSL4. Free Radic. Res. 55 (7), 743–754. doi:10.1080/10715762.2021.1962009
Bersuker, K., Hendricks, J. M., Li, Z., Magtanong, L., Ford, B., Tang, P. H., et al. (2019). The CoQ oxidoreductase FSP1 acts parallel to GPX4 to inhibit ferroptosis. Nature 575 (7784), 688–692. doi:10.1038/s41586-019-1705-2
Chen, D., Fan, Z., Rauh, M., Buchfelder, M., Eyupoglu, I. Y., and Savaskan, N. (2017). ATF4 promotes angiogenesis and neuronal cell death and confers ferroptosis in a xCT-dependent manner. Oncogene 36 (40), 5593–5608. doi:10.1038/onc.2017.146
Chen, X., Comish, P. B., Tang, D., and Kang, R. (2021a). Characteristics and biomarkers of ferroptosis. Front. Cell Dev. Biol. 9, 637162. doi:10.3389/fcell.2021.637162
Chen, X., Li, J., Kang, R., Klionsky, D. J., and Tang, D. (2021b). Ferroptosis: Machinery and regulation. Autophagy 17 (9), 2054–2081. doi:10.1080/15548627.2020.1810918
Chen, Y., Fan, H., Wang, S., Tang, G., Zhai, C., and Shen, L. (2021c). Ferroptosis: A novel therapeutic target for ischemia-reperfusion injury. Front. Cell Dev. Biol. 9, 688605. doi:10.3389/fcell.2021.688605
Chen, Y., Fan, Z., Yang, Y., and Gu, C. (2019). Iron metabolism and its contribution to cancer (Review). Int. J. Oncol. 54 (4), 1143–1154. doi:10.3892/ijo.2019.4720
Deng, S. H., Wu, D. M., Li, L., Liu, T., Zhang, T., Li, J., et al. (2021). miR-324-3p reverses cisplatin resistance by inducing GPX4-mediated ferroptosis in lung adenocarcinoma cell line A549. Biochem. Biophys. Res. Commun. 549, 54–60. doi:10.1016/j.bbrc.2021.02.077
Ding, C., Ding, X., Zheng, J., Wang, B., Li, Y., Xiang, H., et al. (2020). miR-182-5p and miR-378a-3p regulate ferroptosis in I/R-induced renal injury. Cell Death Dis. 11 (10), 929. doi:10.1038/s41419-020-03135-z
Dixon, S. J., Lemberg, K. M., Lamprecht, M. R., Skouta, R., Zaitsev, E. M., Gleason, C. E., et al. (2012). Ferroptosis: An iron-dependent form of nonapoptotic cell death. Cell 149 (5), 1060–1072. doi:10.1016/j.cell.2012.03.042
Doll, S., Freitas, F. P., Shah, R., Aldrovandi, M., da Silva, M. C., Ingold, I., et al. (2019). FSP1 is a glutathione-independent ferroptosis suppressor. Nature 575 (7784), 693–698. doi:10.1038/s41586-019-1707-0
Doll, S., Proneth, B., Tyurina, Y. Y., Panzilius, E., Kobayashi, S., Ingold, I., et al. (2017). ACSL4 dictates ferroptosis sensitivity by shaping cellular lipid composition. Nat. Chem. Biol. 13 (1), 91–98. doi:10.1038/nchembio.2239
Duan, B. (2020). Concise review: Harnessing iPSC-derived cells for ischemic heart disease treatment. J. Transl. Int. Med. 8 (1), 20–25. doi:10.2478/jtim-2020-0004
Fan, H., Ai, R., Mu, S., Niu, X., Guo, Z., and Liu, L. (2022). MiR-19a suppresses ferroptosis of colorectal cancer cells by targeting IREB2. Bioengineered 13 (5), 12021–12029. doi:10.1080/21655979.2022.2054194
Fan, K., Huang, W., Qi, H., Song, C., He, C., Liu, Y., et al. (2021). The Egr-1/miR-15a-5p/GPX4 axis regulates ferroptosis in acute myocardial infarction. Eur. J. Pharmacol. 909, 174403. doi:10.1016/j.ejphar.2021.174403
Forcina, G. C., and Dixon, S. J. (2019). GPX4 at the crossroads of lipid homeostasis and ferroptosis. Proteomics 19 (18), doi:e1800311doi:10.1002/pmic.201800311
Fu, C., Wu, Y., Liu, S., Luo, C., Lu, Y., Liu, M., et al. (2022). Rehmannioside A improves cognitive impairment and alleviates ferroptosis via activating PI3K/AKT/Nrf2 and SLC7A11/GPX4 signaling pathway after ischemia. J. Ethnopharmacol. 289, doi:115021doi:10.1016/j.jep.2022.115021
Gao, M., Fan, K., Chen, Y., Zhang, G., Chen, J., and Zhang, Y. (2022). Understanding the mechanistic regulation of ferroptosis in cancer: Gene matters. J. Genet. Genomics. doi:10.1016/j.jgg.2022.06.002
Gao, M., Monian, P., Pan, Q., Zhang, W., Xiang, J., and Jiang, X. (2016). Ferroptosis is an autophagic cell death process. Cell Res. 26 (9), 1021–1032. doi:10.1038/cr.2016.95
Gao, M., Monian, P., Quadri, N., Ramasamy, R., and Jiang, X. (2015). Glutaminolysis and transferrin regulate ferroptosis. Mol. Cell 59 (2), 298–308. doi:10.1016/j.molcel.2015.06.011
Geng, N., Shi, B. J., Li, S. L., Zhong, Z. Y., Li, Y. C., Xua, W. L., et al. (2018). Knockdown of ferroportin accelerates erastin-induced ferroptosis in neuroblastoma cells. Eur. Rev. Med. Pharmacol. Sci. 22 (12), 3826–3836. doi:10.26355/eurrev_201806_15267
Gomaa, A., Peng, D., Chen, Z., Soutto, M., Abouelezz, K., Corvalan, A., et al. (2019). Epigenetic regulation of AURKA by miR-4715-3p in upper gastrointestinal cancers. Sci. Rep. 9 (1), doi:16970doi:10.1038/s41598-019-53174-6
Guo, Y., Zhang, W., Zhou, X., Zhao, S., Wang, J., Guo, Y., et al. (2022). Roles of ferroptosis in cardiovascular diseases. Front. Cardiovasc Med. 9, doi:911564doi:10.3389/fcvm.2022.911564
Habib, E., Linher-Melville, K., Lin, H. X., and Singh, G. (2015). Expression of xCT and activity of system xc(-) are regulated by NRF2 in human breast cancer cells in response to oxidative stress. Redox Biol. 5, 33–42. doi:10.1016/j.redox.2015.03.003
Hao, L., Mi, J., Song, L., Guo, Y., Li, Y., Yin, Y., et al. (2021). SLC40A1 mediates ferroptosis and cognitive dysfunction in type 1 diabetes. Neuroscience 463, 216–226. doi:10.1016/j.neuroscience.2021.03.009
Hou, W., Xie, Y., Song, X., Sun, X., Lotze, M. T., Zeh, H. J., et al. (2016). Autophagy promotes ferroptosis by degradation of ferritin. Autophagy 12 (8), 1425–1428. doi:10.1080/15548627.2016.1187366
Hu, Z., Yin, Y., Jiang, J., Yan, C., Wang, Y., Wang, D., et al. (2022). Exosomal miR-142-3p secreted by Hepatitis B virus (HBV)-hepatocellular carcinoma (HCC) cells promotes ferroptosis of M1-type macrophages through SLC3A2 and the mechanism of HCC progression. J. Gastrointest. Oncol. 13 (2), 754–767. doi:10.21037/jgo-21-916
Jhelum, P., Santos-Nogueira, E., Teo, W., Haumont, A., Lenoël, I., Stys, P. K., et al. (2020). Ferroptosis mediates cuprizone-induced loss of oligodendrocytes and demyelination. J. Neurosci. 40 (48), 9327–9341. doi:10.1523/jneurosci.1749-20.2020
Jiang, X., Stockwell, B. R., and Conrad, M. (2021). Ferroptosis: Mechanisms, biology and role in disease. Nat. Rev. Mol. Cell Biol. 22 (4), 266–282. doi:10.1038/s41580-020-00324-8
Jyotsana, N., Ta, K. T., and DelGiorno, K. E. (2022). The role of cystine/glutamate antiporter slc7a11/xCT in the pathophysiology of cancer. Front. Oncol. 12, doi:858462doi:10.3389/fonc.2022.858462
Kagan, V. E., Mao, G., Qu, F., Angeli, J. P., Doll, S., Croix, C. S., et al. (2017). Oxidized arachidonic and adrenic PEs navigate cells to ferroptosis. Nat. Chem. Biol. 13 (1), 81–90. doi:10.1038/nchembio.2238
Komai, K., Kawasaki, N. K., Higa, J. K., and Matsui, T. (2022). The role of ferroptosis in adverse left ventricular remodeling following acute myocardial infarction. Cells 11 (9). doi:10.3390/cells11091399
Koppula, P., Zhang, Y., Zhuang, L., and Gan, B. (2018). Amino acid transporter SLC7A11/xCT at the crossroads of regulating redox homeostasis and nutrient dependency of cancer. Cancer Commun. (Lond) 38 (1), 12. doi:10.1186/s40880-018-0288-x
Koppula, P., Zhuang, L., and Gan, B. (2021). Cystine transporter slc7a11/xCT in cancer: Ferroptosis, nutrient dependency, and cancer therapy. Protein Cell 12 (8), 599–620. doi:10.1007/s13238-020-00789-5
Kremer, D. M., Nelson, B. S., Lin, L., Yarosz, E. L., Halbrook, C. J., Kerk, S. A., et al. (2021). GOT1 inhibition promotes pancreatic cancer cell death by ferroptosis. Nat. Commun. 12 (1), 4860. doi:10.1038/s41467-021-24859-2
Lai, B., Wu, C. H., Wu, C. Y., Luo, S. F., and Lai, J. H. (2022). Ferroptosis and autoimmune diseases. Front. Immunol. 13, 916664. doi:10.3389/fimmu.2022.916664
Li, G., Jin, J., Liu, S., Ding, K., and Qian, C. (2021a). Inhibition of miR-1224 suppresses hypoxia/reoxygenation-induced oxidative stress and apoptosis in cardiomyocytes through targeting GPX4. Exp. Mol. Pathol. 121, 104645. doi:10.1016/j.yexmp.2021.104645
Li, N., Jiang, W., Wang, W., Xiong, R., Wu, X., and Geng, Q. (2021b). Ferroptosis and its emerging roles in cardiovascular diseases. Pharmacol. Res. 166, 105466. doi:10.1016/j.phrs.2021.105466
Li, Xinrong, Wenwen, S. I., Zhan, L. I., Xuelei, L. I. U., Shanyu, Y. E., et al. (2021). MiR-335 promotes ferroptosis by targeting ferritin heavy chain 1 in in vivo and in vitro models of Parkinson's disease. Int. J. Mol. Med. 47 (61), 1–11.
Li, X., Wu, L., Tian, X., Zheng, W., Yuan, M., Tian, X., et al. (2022). MiR-29a-3p in exosomes from heme oxygenase-1 modified bone marrow mesenchymal stem cells alleviates steatotic liver ischemia-reperfusion injury in rats by suppressing ferroptosis via iron responsive element binding protein 2. Oxid. Med. Cell Longev. 2022, 6520789. doi:10.1155/2022/6520789
Li, X., Zhang, X., Liu, Y., Pan, R., Liang, X., Huang, L., et al. (2021c). Exosomes derived from mesenchyml stem cells ameliorate oxygen-glucose deprivation/reoxygenation-induced neuronal injury via transferring MicroRNA-194 and targeting Bach1. Tissue Cell 73, 101651. doi:10.1016/j.tice.2021.101651
Li, Y., Wang, J., Chen, S., Wu, P., Xu, S., Wang, C., et al. (2020). MiR-137 boosts the neuroprotective effect of endothelial progenitor cell-derived exosomes in oxyhemoglobin-treated SH-SY5Y cells partially via COX2/PGE2 pathway. Stem Cell Res. Ther. 11 (1). doi:10.1186/s13287-020-01836-y
Liang, Y., Deng, Y., Zhao, J., Liu, L., Wang, J., Chen, P., et al. (2022). Ferritinophagy is involved in experimental subarachnoid hemorrhage-induced neuronal ferroptosis. Neurochem. Res. 47 (3), 692–700. doi:10.1007/s11064-021-03477-w
Liao, Y., Jia, X., Ren, Y., Deji, Z., Gesang, Y., Ning, N., et al. (2021). Suppressive role of microRNA-130b-3p in ferroptosis in melanoma cells correlates with DKK1 inhibition and Nrf2-HO-1 pathway activation. Hum. Cell 34 (5), 1532–1544. doi:10.1007/s13577-021-00557-5
Liu, D., Yang, M., Yao, Y., He, S., Wang, Y., Cao, Z., et al. (2022a). Cardiac fibroblasts promote ferroptosis in atrial fibrillation by secreting exo-miR-23a-3p targeting SLC7A11. Oxidative Med. Cell. Longev. 2022, 1–31. doi:10.1155/2022/3961495
Liu, J., Kang, R., and Tang, D. (2021). Signaling pathways and defense mechanisms of ferroptosis. Febs J. doi:10.1111/febs.16059
Liu, L., Yao, H., Zhou, X., Chen, J., Chen, G., Shi, X., et al. (2022b). MiR-15a-3p regulates ferroptosis via targeting glutathione peroxidase GPX4 in colorectal cancer. Mol. Carcinog. 61 (3), 301–310. doi:10.1002/mc.23367
Liu, M., Kong, X. Y., Yao, Y., Wang, X. A., Yang, W., Wu, H., et al. (2022c). The critical role and molecular mechanisms of ferroptosis in antioxidant systems: A narrative review. Ann. Transl. Med. 10 (6), 368. doi:10.21037/atm-21-6942
Liu, Y., and Gu, W. (2022). p53 in ferroptosis regulation: the new weapon for the old guardian. Cell Death Differ. 29 (5), 895–910. doi:10.1038/s41418-022-00943-y
Luo, M., Wu, L., Zhang, K., Wang, H., Zhang, T., Gutierrez, L., et al. (2018). miR-137 regulates ferroptosis by targeting glutamine transporter SLC1A5 in melanoma. Cell Death Differ. 25 (8), 1457–1472. doi:10.1038/s41418-017-0053-8
Ma, L. L., Liang, L., Zhou, D., and Wang, S. W. (2021). Tumor suppressor miR-424-5p abrogates ferroptosis in ovarian cancer through targeting ACSL4. Neoplasma 68 (1), 165–173. doi:10.4149/neo_2020_200707N705
Macías-Rodríguez, R. U., Inzaugarat, M. E., Ruiz-Margáin, A., Nelson, L. J., Trautwein, C., and Cubero, F. J. (2020). Reclassifying hepatic cell death during liver damage: Ferroptosis-A novel form of non-apoptotic cell death? Int. J. Mol. Sci. 21 (5). doi:10.3390/ijms21051651
Masaldan, S., Bush, A. I., Devos, D., Rolland, A. S., and Moreau, C. (2019). Striking while the iron is hot: Iron metabolism and ferroptosis in neurodegeneration. Free Radic. Biol. Med. 133, 221–233. doi:10.1016/j.freeradbiomed.2018.09.033
Maslov, L. N., Popov, S. V., Naryzhnaya, N. V., Mukhomedzyanov, A. V., Kurbatov, B. K., Derkachev, I. A., et al. (2022). The regulation of necroptosis and perspectives for the development of new drugs preventing ischemic/reperfusion of cardiac injury. Apoptosis. doi:10.1007/s10495-022-01760-x
Matés, J. M., Campos-Sandoval, J. A., de Los Santos-Jiménez, J., and Márquez, J. (2020). Glutaminases regulate glutathione and oxidative stress in cancer. Arch. Toxicol. 94 (8), 2603–2623. doi:10.1007/s00204-020-02838-8
Muhoberac, B. B., and Vidal, R. (2019). Iron, ferritin, hereditary ferritinopathy, and neurodegeneration. Front. Neurosci. 13, 1195. doi:10.3389/fnins.2019.01195
Nguyen, T. H. P., Mahalakshmi, B., and Velmurugan, B. K. (2020). Functional role of ferroptosis on cancers, activation and deactivation by various therapeutic candidates-an update. Chem. Biol. Interact. 317, 108930. doi:10.1016/j.cbi.2019.108930
Ni, H., Qin, H., Sun, C., Liu, Y., Ruan, G., Guo, Q., et al. (2021). MiR-375 reduces the stemness of gastric cancer cells through triggering ferroptosis. Stem Cell Res. Ther. 12 (1), 325. doi:10.1186/s13287-021-02394-7
Nishizawa, H., Yamanaka, M., and Igarashi, K. (2022). Ferroptosis: Regulation by competition between NRF2 and BACH1 and propagation of the death signal. Febs J. doi:10.1111/febs.16382
Pandrangi, S. L., Chittineedi, P., Chikati, R., Lingareddy, J. R., Nagoor, M., and Ponnada, S. K. (2022). Role of dietary iron revisited: In metabolism, ferroptosis and pathophysiology of cancer. Am. J. Cancer Res. 12 (3), 974–985.
Song, X., Zhu, S., Chen, P., Hou, W., Wen, Q., Liu, J., et al. (2018). AMPK-mediated BECN1 phosphorylation promotes ferroptosis by directly blocking system X(c)(-) activity. Curr. Biol. 28 (15), 2388–2399. e2385. doi:10.1016/j.cub.2018.05.094
Song, Z., Jia, G., Ma, P., and Cang, S. (2021). Exosomal miR-4443 promotes cisplatin resistance in non-small cell lung carcinoma by regulating FSP1 m6A modification-mediated ferroptosis. Life Sci. 276, 119399. doi:10.1016/j.lfs.2021.119399
Stockwell, B. R. (2022). Ferroptosis turns 10: Emerging mechanisms, physiological functions, and therapeutic applications. Cell 185 (14), 2401–2421. doi:10.1016/j.cell.2022.06.003
Stockwell, B. R., Friedmann Angeli, J. P., Bayir, H., Bush, A. I., Conrad, M., Dixon, S. J., et al. (2017). Ferroptosis: A regulated cell death nexus linking metabolism, redox biology, and disease. Cell 171 (2), 273–285. doi:10.1016/j.cell.2017.09.021
Sun, K., Ren, W., Li, S., Zheng, J., Huang, Y., Zhi, K., et al. (2022). MiR-34c-3p upregulates erastin-induced ferroptosis to inhibit proliferation in oral squamous cell carcinomas by targeting SLC7A11. Pathol. Res. Pract. 231, 153778. doi:10.1016/j.prp.2022.153778
Tang, S., Wang, Y., Ma, T., Lu, S., Huang, K., Li, Q., et al. (2020). MiR-30d inhibits cardiomyocytes autophagy promoting ferroptosis after myocardial infarction. Panminerva Med.. doi:10.23736/s0031-0808.20.03979-8
Tang, X., Chen, W., Liu, H., Liu, N., Chen, D., Tian, D., et al. (2022). Research progress on SLC7A11 in the regulation of cystine/cysteine metabolism in tumors. Oncol. Lett. 23 (2), 47. doi:10.3892/ol.2021.13165
Tao, W., Liu, F., Zhang, J., Fu, S., Zhan, H., and Qian, K. (2021). miR-3587 inhibitor attenuates ferroptosis following renal ischemia-reperfusion through HO-1. Front. Mol. Biosci. 8, 789927. doi:10.3389/fmolb.2021.789927
Tomita, K., Fukumoto, M., Itoh, K., Kuwahara, Y., Igarashi, K., Nagasawa, T., et al. (2019). MiR-7-5p is a key factor that controls radioresistance via intracellular Fe(2+) content in clinically relevant radioresistant cells. Biochem. Biophys. Res. Commun. 518 (4), 712–718. doi:10.1016/j.bbrc.2019.08.117
Tomita, K., Nagasawa, T., Kuwahara, Y., Torii, S., Igarashi, K., Roudkenar, M. H., et al. (2021). MiR-7-5p is involved in ferroptosis signaling and radioresistance thru the generation of ROS in radioresistant HeLa and SAS cell lines. Int. J. Mol. Sci. 22 (15). doi:10.3390/ijms22158300
Tugan Yildiz, B., and Tuncel Berktas, D. (2021). Experiences on the administration of botulinum toxin in movement disorders. J. Transl. Int. Med. 9 (1), 52–56. doi:10.2478/jtim-2021-0003
Ursini, F., Bosello Travain, V., Cozza, G., Miotto, G., Roveri, A., Toppo, S., et al. (2022). A white paper on Phospholipid Hydroperoxide Glutathione Peroxidase (GPx4) forty years later. Free Radic. Biol. Med. 188, 117–133. doi:10.1016/j.freeradbiomed.2022.06.227
Valashedi, M. R., Bamshad, C., Najafi-Ghalehlou, N., Nikoo, A., Tomita, K., Kuwahara, Y., et al. (2022). Non-coding RNAs in ferroptotic cancer cell death pathway: Meet the new masters. Hum. Cell 35 (4), 972–994. doi:10.1007/s13577-022-00699-0
Van Coillie, S., Van San, E., Goetschalckx, I., Wiernicki, B., Mukhopadhyay, B., Tonnus, W., et al. (2022). Targeting ferroptosis protects against experimental (multi)organ dysfunction and death. Nat. Commun. 13 (1), 1046. doi:10.1038/s41467-022-28718-6
von Samson-Himmelstjerna, F. A., Kolbrink, B., Riebeling, T., Kunzendorf, U., and Krautwald, S. (2022). Progress and setbacks in translating a decade of ferroptosis research into clinical practice. Cells 11 (14). doi:10.3390/cells11142134
Wang, F., Li, J., Zhao, Y., Guo, D., Liu, D., Chang, S. e., et al. (2022a). miR-672-3p promotes functional recovery in rats with contusive spinal cord injury by inhibiting ferroptosis suppressor protein 1. Oxidative Med. Cell. Longev. 2022, 1–19. doi:10.1155/2022/6041612
Wang, W., Shi, F., Cui, J., Pang, S., Zheng, G., and Zhang, Y. (2022b). MiR-378a-3p/SLC7A11 regulate ferroptosis in nerve injury induced by lead exposure. Ecotoxicol. Environ. Saf. 239. doi:10.1016/j.ecoenv.2022.113639
Wang, Y., Zhao, Y., Ye, T., Yang, L., Shen, Y., and Li, H. (2021). Ferroptosis signaling and regulators in atherosclerosis. Front. Cell Dev. Biol. 9, doi:809457doi:10.3389/fcell.2021.809457
Weaver, K., and Skouta, R. (2022). The selenoprotein glutathione peroxidase 4: From molecular mechanisms to novel therapeutic opportunities. Biomedicines 10 (4). doi:10.3390/biomedicines10040891
Wei, D., Ke, Y. Q., Duan, P., Zhou, L., Wang, C. Y., and Cao, P. (2021). MicroRNA-302a-3p induces ferroptosis of non-small cell lung cancer cells via targeting ferroportin. Free Radic. Res. 55 (7), 821–830. doi:10.1080/10715762.2021.1947503
Wu, J. R., Tuo, Q. Z., and Lei, P. (2018). Ferroptosis, a recent defined form of critical cell death in neurological disorders. J. Mol. Neurosci. 66 (2), 197–206. doi:10.1007/s12031-018-1155-6
Wu, J. Y., and Prentice, H. (2021). Potential new therapeutic intervention for ischemic stroke. J. Transl. Int. Med. 9 (1), 1–3. doi:10.2478/jtim-2021-0014
Wu, L., Tian, X., Zuo, H., Zheng, W., Li, X., Yuan, M., et al. (2022a). miR-124-3p delivered by exosomes from heme oxygenase-1 modified bone marrow mesenchymal stem cells inhibits ferroptosis to attenuate ischemia–reperfusion injury in steatotic grafts. J. Nanobiotechnology 20 (1). doi:10.1186/s12951-022-01407-8
Wu, M., Zhang, X., Zhang, W., Chiou, Y. S., Qian, W., Liu, X., et al. (2022b). Cancer stem cell regulated phenotypic plasticity protects metastasized cancer cells from ferroptosis. Nat. Commun. 13 (1), 1371. doi:10.1038/s41467-022-29018-9
Xiao, F. J., Zhang, D., Wu, Y., Jia, Q. H., Zhang, L., Li, Y. X., et al. (2019a). miRNA-17-92 protects endothelial cells from erastin-induced ferroptosis through targeting the A20-ACSL4 axis. Biochem. Biophys. Res. Commun. 515 (3), 448–454. doi:10.1016/j.bbrc.2019.05.147
Xiao, X., Jiang, Y., Liang, W., Wang, Y., Cao, S., Yan, H., et al. (2019b). miR-212-5p attenuates ferroptotic neuronal death after traumatic brain injury by targeting Ptgs2. Mol. Brain 12 (1), 78. doi:10.1186/s13041-019-0501-0
Xu, F., Wang, H., Pei, H., Zhang, Z., Liu, L., Tang, L., et al. (2022a). SLC1A5 prefers to play as an accomplice rather than an opponent in pancreatic adenocarcinoma. Front. Cell Dev. Biol. 10, 800925. doi:10.3389/fcell.2022.800925
Xu, J., Zhang, J., and Wang, J. (2020). The application of traditional Chinese medicine against the tumor immune escape. J. Transl. Int. Med. 8 (4), 203–204. doi:10.2478/jtim-2020-0032
Xu, P., Wang, Y., Deng, Z., Tan, Z., and Pei, X. (2022b). MicroRNA-15a promotes prostate cancer cell ferroptosis by inhibiting GPX4 expression. Oncol. Lett. 23 (2), 67. doi:10.3892/ol.2022.13186
Xu, S., Qiu, Y., and Tao, J. (2021a). The challenges and optimization of cell-based therapy for cardiovascular disease. J. Transl. Int. Med. 9 (4), 234–238. doi:10.2478/jtim-2021-0017
Xu, Z., Chen, L., Wang, C., Zhang, L., and Xu, W. (2021b). MicroRNA-1287-5p promotes ferroptosis of osteosarcoma cells through inhibiting GPX4. Free Radic. Res. 55 (11-12), 1119–1129. doi:10.1080/10715762.2021.2024816
Yadav, P., Sharma, P., Sundaram, S., Venkatraman, G., Bera, A. K., and Karunagaran, D. (2021). SLC7A11/xCT is a target of miR-5096 and its restoration partially rescues miR-5096-mediated ferroptosis and anti-tumor effects in human breast cancer cells. Cancer Lett. 522, 211–224. doi:10.1016/j.canlet.2021.09.033
Yan, Y., Liang, Q., Xu, Z., Huang, J., Chen, X., Cai, Y., et al. (2021). Downregulated ferroptosis-related gene STEAP3 as a novel diagnostic and prognostic target for hepatocellular carcinoma and its roles in immune regulation. Front. Cell Dev. Biol. 9, doi:743046doi:10.3389/fcell.2021.743046
Yang, Y., Lin, Z., Han, Z., Wu, Z., Hua, J., Zhong, R., et al. (2021). miR-539 activates the SAPK/JNK signaling pathway to promote ferropotosis in colorectal cancer by directly targeting TIPE. Cell Death Discov. 7 (1), 272. doi:10.1038/s41420-021-00659-x
Zexin, L., (2022). MicroRNA-132 promotes atherosclerosis by inducing mitochondrial oxidative stressmediated ferroptosis. Nan Fang. Yi Ke Da Xue Xue Bao 421, 143–149. doi:10.12122/j.issn.1673-4254.2022.01.18
Zhang, H., He, Y., Wang, J. X., Chen, M. H., Xu, J. J., Jiang, M. H., et al. (2020a). miR-30-5p-mediated ferroptosis of trophoblasts is implicated in the pathogenesis of preeclampsia. Redox Biol. 29, 101402. doi:10.1016/j.redox.2019.101402
Zhang, K., Wu, L., Zhang, P., Luo, M., Du, J., Gao, T., et al. (2018). miR-9 regulates ferroptosis by targeting glutamic-oxaloacetic transaminase GOT1 in melanoma. Mol. Carcinog. 57 (11), 1566–1576. doi:10.1002/mc.22878
Zhang, X., Wang, L., Li, H., Zhang, L., Zheng, X., and Cheng, W. (2020b). Crosstalk between noncoding RNAs and ferroptosis: New dawn for overcoming cancer progression. Cell Death Dis. 11 (7), 580. doi:10.1038/s41419-020-02772-8
Zheng, S., Hu, L., Qingwen Song, Y. S., Yin, G., Zhu, H., and Wencheng Kong, C. Z. (2021). miR-545 promotes colorectal cancer by inhibiting transferring in the non-normal ferroptosis signaling. AGING 13 (24), 26137–26147.
Zhi, Y., Gao, L., Wang, B., Ren, W., Liang, K. X., and Zhi, K. (2021). Ferroptosis holds novel promise in treatment of cancer mediated by non-coding RNAs. Front. Cell Dev. Biol. 9, 686906. doi:10.3389/fcell.2021.686906
Zhou, J., Xiao, C., Zheng, S., Wang, Q., Zhu, H., Zhang, Y., et al. (2022). MicroRNA-214-3p aggravates ferroptosis by targeting GPX4 in cisplatin-induced acute kidney injury. Cell Stress Chaperones. doi:10.1007/s12192-022-01271-3
Zhou, X., Zhuo, M., Zhang, Y., Shi, E., Ma, X., and Li, H. (2021). miR-190a-5p regulates cardiomyocytes response to ferroptosis via directly targeting GLS2. Biochem. Biophys. Res. Commun. 566, 9–15. doi:10.1016/j.bbrc.2021.05.100
Zhu, C., Song, Z., Chen, Z., Lin, T., Lin, H., Xu, Z., et al. (2022a). MicroRNA-4735-3p facilitates ferroptosis in clear cell renal cell carcinoma by targeting SLC40A1. Anal. Cell. Pathol. 2022, 1–12. doi:10.1155/2022/4213401
Zhu, D., Wu, S., Li, Y., Zhang, Y., Chen, J., Ma, J., et al. (2022b). Ferroptosis-related gene SLC1A5 is a novel prognostic biomarker and correlates with immune infiltrates in stomach adenocarcinoma. Cancer Cell Int. 22 (1), 124. doi:10.1186/s12935-022-02544-8
Zhu, S., Zhang, Q., Sun, X., Zeh, H. J., Lotze, M. T., Kang, R., et al. (2017). HSPA5 regulates ferroptotic cell death in cancer cells. Cancer Res. 77 (8), 2064–2077. doi:10.1158/0008-5472.Can-16-1979
Zhuang, Y., Yang, D., Shi, S., Wang, L., Yu, M., Meng, X., et al. (2022). MiR-375-3p promotes cardiac fibrosis by regulating the ferroptosis mediated by GPX4. Comput. Intell. Neurosci. 2022, 1–12. doi:10.1155/2022/9629158
Keywords: ferroptosis, lipid peroxidation, microRNA, cardiomyopathy, tumor, neuronal injury disorder, ischemia perfusion disorder
Citation: Guo L, Zhang Q and Liu Y (2022) The role of microRNAs in ferroptosis. Front. Mol. Biosci. 9:1003045. doi: 10.3389/fmolb.2022.1003045
Received: 25 July 2022; Accepted: 20 September 2022;
Published: 12 October 2022.
Edited by:
Xin Wang, National Institutes of Health, United StatesReviewed by:
Yanqing Liu, Columbia University, United StatesXiaoling Chen, Purdue University, United States
Copyright © 2022 Guo, Zhang and Liu. This is an open-access article distributed under the terms of the Creative Commons Attribution License (CC BY). The use, distribution or reproduction in other forums is permitted, provided the original author(s) and the copyright owner(s) are credited and that the original publication in this journal is cited, in accordance with accepted academic practice. No use, distribution or reproduction is permitted which does not comply with these terms.
*Correspondence: Yuehui Liu, bGl1eXVlaHVpY2xhcmtAMjFjbi5jb20=