- Laboratory of Aging Research and Cancer Drug Target, State Key Laboratory of Biotherapy, National Clinical Research Center for Geriatrics, West China Hospital, Sichuan University, Chengdu, China
Ikaros is a zinc finger transcription factor (TF) of the Krüppel family member, which significantly regulates normal lymphopoiesis and tumorigenesis. Ikaros can directly initiate or suppress tumor suppressors or oncogenes, consequently regulating the survival and proliferation of cancer cells. Over recent decades, a series of studies have been devoted to exploring and clarifying the relationship between Ikaros and associated tumors. Therapeutic strategies targeting Ikaros have shown promising therapeutic effects in both pre-clinical and clinical trials. Nevertheless, the increasingly prominent problem of drug resistance targeted to Ikaros and its analog is gradually appearing in our field of vision. This article reviews the role of Ikaros in tumorigenesis, the mechanism of drug resistance, the progress of targeting Ikaros in both pre-clinical and clinical trials, and the potential use of associated therapy in cancer therapy.
1 Introduction
Ikaros is a zinc finger transcription factor (TF) and a member of the Krüppel family, which is called the IKAROS family zinc finger protein family (IKZF) and consists of other TFs named Ikaros, Helios, Aiolos, Eos, and Pegasus. Ikaros is encoded by the IKZF1 gene (Zhao et al., 2020), exerting an essential effect on regulating normal lymphopoiesis and functions as a tumor suppressor (Winandy et al., 1995; Sigvardsson, 2018). It covers four zinc fingers at the N-terminal for binding to DNA by directly combining with the GGGAA core motif in vitro and at the A/GGAAA core motif in vivo. At the C-terminal of Ikaros, two additional zinc fingers are required to form homo- and hetero-dimerization between isoforms. The mutations in IKZF are associated with recurrent infections, cytopenia (neutropenia, immune thrombocytopenia, and autoimmune hemolytic anemia), autoimmune diseases, and hematological malignancies (Kuehn et al., 2020).
Current knowledge of the Ikaros family suggests that these TFs are primarily concerned with the development of lymphocytes (Heizmann et al., 2018), covering extensive cellular processes like proliferation, differentiation, cell cycle arrest, and apoptosis (Fan and Lu, 2016). Nevertheless, the absence of Ikaros proteins results in a detrimental production of B lymphocytes, T lymphocytes, NK cells, and dendritic cells (Georgopoulos, 2002; Hariri and Hardin, 2020). Germline mutation in IKZF1 has also been reported to be associated with congenital pancytopenia (Goldman et al., 2012).
1.1 Classification and Genome
The Ikaros protein family includes five members named Ikaros, Helios, Aiolos, Eos, and Pegasus. Ikaros, Helios, and Aiolos are principally expressed in some hematopoietic cells and lymphoid cells; notwithstanding, Ikaros is also found in the brain. Eos and Pegasus are widely detected throughout the body, including the brain, liver, skeletal muscle, kidney, and heart (Fan and Lu, 2016) (Table 1). Various IKZF protein TFs emit diverse effects in the maintenance of normal physiological activities or progression of some diseases.
1.1.1 Ikaros
The IKZF1 gene that encodes Ikaros is located on chromosome 7 at 7p12.2 (HGNC, 2021). It consists of 8 exons and codes 519 amino acids. Exon 8 at the C-terminal includes the two domain zinc fingers required to form homo- and hetero-dimerization and four N-terminal DNA-binding zinc fingers for binding to the core motif at DNA (Ruiz et al., 2004; Zhang et al., 2020; Payne, 2011). Different combinations of zinc finger modules influence the capacity of DNA-binding and functional properties (Molnár and Georgopoulos, 1994; Vairy and Tran, 2020). At least 12 isoforms, including Ik1–12, are generated through alternative splicing Ikaros genes encoding a zinc finger protein with eight exons (Molnár and Georgopoulos, 1994; Yamamoto et al., 2005; Hahm et al., 1994) (Figure 1). Among all the isoforms, the largest isoforms are Ik1 or Ik-H, which have four zinc finger domains at the N-terminal and two at the end of the C-terminal (Ronni et al., 2007). In addition, dimerization among Ikaros isoforms either enhances or suppresses its affinity of DNA binding, thus affecting the general Ikaros transcriptional activity through specific mechanisms such as chromatin-remodeling complexes and epigenetic modification (Georgopoulos et al., 1997; McCarty et al., 2003).
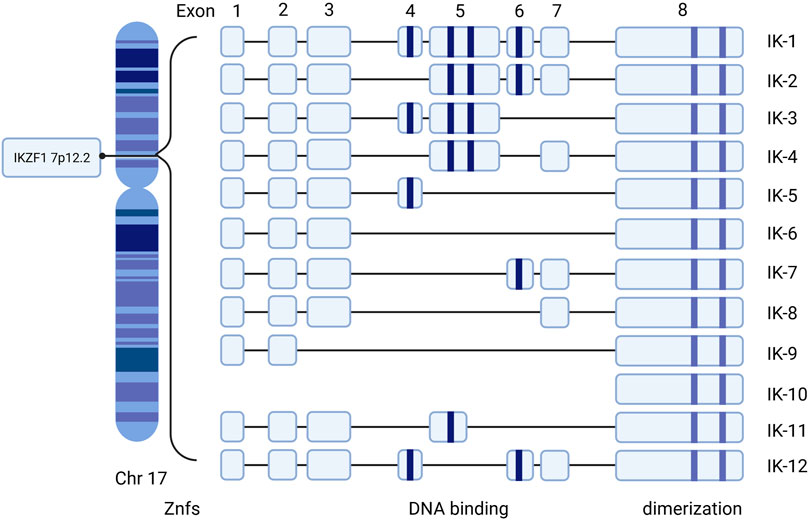
FIGURE 1. Human Ikaros isoforms produced by IKZF1 transcription. Exons 1–8 refer to the encoding exons. The N-terminal zinc fingers are shown in blue column bars and C-terminal zinc fingers are shown in purple column bars. Znfs: zinc-fingers.
Isoforms with at least three DNA binding domains can combine with Ikaros conservative DNA binding sites, while isoforms with less than three DNA binding domains are not able to bind these motifs and remain in the cytoplasm (Hahm et al., 1994). Hence, the functional characteristics and subcellular localization of Ikaros isotypes may be different. Besides, the expression of Ikaros is correlated to the morphology of the nucleus. When cytogenetic outcomes are not available, the morphology of the cuplike nucleus can help to indicate the presence of IKZF1 deletion at a high predictive value (Li et al., 2019). IK6, due to the deficiency of exons 4–7, lacks four N-terminal zinc fingers but retains the C-terminal dimerization domain, which is most closely related to oncogenicity (Vairy and Tran, 2020). The presence of certain isoforms may be associated with the occurrence and prognosis of corresponding clinical diseases. Ik6 and Ik10 are highly related to a poor clinical outcome in B-lineage acute lymphoblastic leukemia (ALL) in children (Moreira et al., 2019). There is a correlation between Ik6 expression and t (4; 11) translocation in patients with leukemia (Ruiz et al., 2004; Zhang et al., 2020).
1.1.2 Helios
Helios, also called IKZF2, is of immediate clinical significance due to the fact that it plays a similar function to Ikaros in regulating the functional property of immune cells and the progression of hematological malignancies. Helios is a marker of stable and inhibitory T regulated cells (Tregs) (Thornton and Shevach, 2019), acting as an essential part to preferentially strengthen the differentiation of fetal Tregs and fine-adjusting of fetal Tregs (Ng et al., 2019). Helios-deficient Tregs show an unstable phenotype in the process of inflammation, characterized by decreased expression of FoxP3 and increased expression of effector cytokines following weakened activation of the STAT5 pathway (Kim et al., 2015).
In addition, the high expression of IKZF2 and low level of interleukin-7 receptor (IL7R) are identifiable transcriptional spectra demonstrated in CD16 + CD8 T cells, which are related to chronic untreated HIV-1 infection (Naluyima et al., 2019). Furthermore, Helios was found to be expressed in acute myeloid leukemia (AML) cells, and its depletion in AML cells resulted in decreased colony formation and slowed down oncogenesis (Park et al., 2019). The elevating Helios levels are related to the capacity of Tregs in malignant pleural effusion due to the downregulation of miR-4772-3p (Yu et al., 2019).
1.1.3 Aiolos
Aiolos, encoded by the IKZF3 gene, is located in 17q11.2∼21 and made up of eight exons and seven introns (Hosokawa et al., 1999). The trans-differentiation from innate lymphoid cell 3 (ILC3) to ILC1/NK cells is linked with high expression of Aiolos and can be reduced through lenalidomide (Len) targeting Aiolos and Ikaros (Mazzurana et al., 2019). In addition, it is of great importance in the occurrence, metastasis, and prognosis of certain hematological malignancies and solid tumors (Duhamel et al., 2008; Li et al., 2014). Aiolos has been found to be expressed in lung cancer cells ectopically, which instigates cancer metastasis through reducing expression of many adhesion-related genes, spoiling cell-cell or cell-matrix interactions, and promoting anchorage independence (Wang et al., 2019; Li et al., 2014). Moreover, solid tumors are able to mimic the cellular behavior related to lymphocyte transport during immune surveillance through the “identity theft” of hematopoiesis led by the expression of Aiolos (Li et al., 2014). In addition to increasing invasive and migratory ability in lung cancer cells through upregulation of the phosphatidylinositol-3 kinase (PI3K)/AKT/Twist axis induced by Aiolos overexpression, the overexpression of Aiolos also upregulates cancer stem cell-like properties through the same pathway (Hung et al., 2019).
1.1.4 Eos
Eos, a novel transcriptional regulator encoded by the IKZF4 gene, is required for the suppressive function of Treg cells in vivo (Gokhale et al., 2019). Eos directly interacts with Foxp3, leading to chromatin modifications, which results in gene silencing in Tregs (Pan et al., 2009). While Tregs of mice with an overall absence of Eos were demonstrated to have completely normal suppressive function both in vivo and in vitro (Rieder et al., 2015; Gokhale et al., 2019). In peripheral blood mononuclear cells of individuals with HTLV-1 infection, the aberrant expression of Eos may correlate to the pathological progression of HTLV-1-related adult T-cell leukemia/lymphoma and myelopathy/tropical spastic paraparesis (Naito et al., 2019).
1.1.5 Pegasus
Pegasus is a novel transcriptional regulator encoded by IKZF5, with an unclear physiological function of normal hematopoiesis. It was reported that Pegasus was related to megakaryopoiesis and dominant thrombocytopenia in humans (Lentaigne et al., 2019).
1.2 Ikaros Family Proteins and Related Diseases
Ikaros proteins are identified to serve as suppressors in diverse types of lymphoma or leukemia (Heizmann et al., 2018; Chan, 2019), whereas they are overexpressed in other malignancies to maintain cancer cell proliferation and survival, such as in malignant plasma cells, monoclonal gammopathy of undetermined significance, and multiple myeloma (MM) (Cippitelli et al., 2021). Detailed functional analyses showed that Ikaros could excite or suppress oncogenes or tumor suppressors genes; consequently, Ikaros-mediated transcriptional expression of target genes regulates survival and proliferation of cancer cells (Gowda et al., 2017a). Specifically, Ikaros was reported to control human skin fibroblast cell migration negatively by GSK3β-Ikaros-ANXA4 signaling (Wang et al., 2020a).
It is assumed that Ikaros family deficiency may lead to a variety of immune-associated diseases, including immune thrombocytopenia (Sriaroon et al., 2019), presumed autoimmune hepatitis (Groth et al., 2020), systemic lupus erythematosus (SLE) (Cunninghame Graham et al., 2011; Jeng et al., 2019; Chen et al., 2020), rheumatoid arthritis (Yang et al., 2019), asthma (Igartua et al., 2015), type 1 diabetes (Davidson and Diamond, 2001; Swafford et al., 2011; Lempainen et al., 2013; Khamechian et al., 2018), Graves’ disease (Li et al., 2018a), Hashimoto thyroiditis (Hu et al., 2019), inflammatory bowel disease (Crohn disease) (Barrett et al., 2008; Eskandarian et al., 2019; Sznurkowska et al., 2020), di George syndrome (Klocperk et al., 2014), antiphospholipid syndrome (Dieudonné et al., 2019), Parkinson disease (Daneshvar Kakhaki et al., 2020), Sjogren’s syndrome, and systemic sclerosis (Gorlova et al., 2011).
It also shows that Ikaros can manage myeloid cell proliferation, and somatic Ikaros mutations are related to myeloproliferative disorders (Theocharides et al., 2015). In addition, the occurrence and maintenance of numerous human cancers, such as pediatric B-cell precursor acute lymphoblastic leukemia (BCP-ALL) (Churchman et al., 2018; Stanulla et al., 2018; Tayel et al., 2019), lung cancer (Li et al., 2014; Zhao et al., 2020), breast cancer (Edgren et al., 2011), nasopharyngeal carcinoma (Verhoeven et al., 2019), ovarian (He et al., 2012), liver (Liu et al., 2017), and colorectal cancer (Javierre et al., 2011), are also correlated with the abnormal expression of Ikaros family proteins. Recently, for some solid tumors, it was shown that a higher level of Ikaros is correlated with poor differentiation and advanced stage of ovarian cancer (He et al., 2012), while it functions as an anticancer character in hepatocellular carcinoma through inhibiting CD133 and ANXA4 expression (Liu et al., 2017). Besides, the hypermethylation of Ikaros levels could be considered as a sign of the progression of colorectal cancer (CRC) and inform adequacy of surgical resection about CRC (Javierre et al., 2011; Symonds et al., 2018; Symonds et al., 2020).
For patients with MM, the Ikaros family proteins served as predictors of prognosis for MM patients treated by Len (Kriegsmann et al., 2019; Tachita et al., 2020). However, a correlation between the expression of IKZF1 or IKZF3 and patients’ reaction to Len from immunohistochemical analysis remains obscure (Dimopoulos et al., 2019). It is considered that the effect of low IKZF1 or IKZF3 levels on the adverse outcome of Len therapy results in shorter progression-free survival and overall survival (Zhu et al., 2014; Pourabdollah et al., 2016; Dimopoulos et al., 2019).
In ALL, approximately 50% of adult patients possess IKZF1 genetic mutations, including beyond 80% of patients with BCR-ABL1-positive (Ph+) ALL. A total of 15% of IKZF1 genetic alteration can be found in childhood B-cell ALL, covering about 70% of Ph + ALL patients (Mullighan et al., 2007; Mullighan et al., 2008). Patients with ALL have Ikaros mutations with characteristic resistance to treatment (Marke et al., 2016), high relapse rate (Kuiper et al., 2010; Berry et al., 2020), and poor prognosis (Mullighan et al., 2009; Aref et al., 2020). Genetic and functional abnormalities of IKZF1, including deletion of a single Ikaros, were regarded as new prognostic indicators for high-risk leukemia in clinical trials (NCT00993538; NCT03709719, NCT01431664) (Mi et al., 2012; Tang et al., 2019; Granados-Zamora et al., 2020).
In chronic myelogenous leukemia (CML), the deletions in IKZF1 and codeletion of other genes are identified in a chronic phase CML diagnostic sample (Klumb et al., 2019). Moreover, the deficiency or reduction of Ikaros is deemed as a common step and potential diagnostic precursor of progressive myeloid disease in patients with CML (Beer et al., 2015).
Immunomodulatory drugs (IMiDs), consist of thalidomide, Len, pomalidomide, and an analog, target a ubiquitous protein called CRBN to induce the degradation of Ikaros. The efficacy and safety of those drugs have been verified in a wide range of clinical trials, and it is increasingly clear that the efficacy of IMiDs in the treatment of MM (Gao et al., 2020), myelodysplastic syndrome (MDS) with deletion of chromosome 5q (Fenaux et al., 2011), mantle cell lymphoma (MCL) (Ruan et al., 2018) and chronic lymphocytic leukemia (CLL) (Vitale et al., 2016; Zhou et al., 2020) is promising. Despite the development of Ikaros-targeted therapy, the incidence of drug resistance is increasing. Herein, we summarized the molecular characteristic of Ikaros, the mechanism of the Ikaros-associated pathway, and recent anti-Ikaros drug development based on clinical trials in our review.
2 Ikaros Family Signaling
Ikaros seems to act both as a transcriptional repressor and as an activator by binding to assorted nuclear factors referred to as epigenetic regulation and chromatin remodeling. If recruiting histone remodeling complexes such as nucleosome remodeling and deacetylase complex (NuRD) via direct binding to Mi-2, it will mediate tumor inhibition. If integrating into the ATP-dependent chromatin remodeling complexes SW1/SNF, it will cause gene activation (Dhanyamraju et al., 2020; Payne et al., 2020). Ikaros also directly engages with and recruits distinct histone deacetylase complexes (HDAC1 and HDAC2) to specific promoters of its target genes to modulate gene expression and to exert tumor-suppressive effects (Koipally et al., 1999; Song et al., 2016). Ikaros participates in a NuRD complex with acetyltransferases, methyltransferases, deacetylases, and the chromatin remodeling complex (Oliveira et al., 2019). In addition to the NuRD complex, the positive-transcription elongation factor b and the protein phosphatase 1α (PP1) are required to assist transcription extension of Ikaros target genes and regular differentiation of hematopoietic progenitor cells (Bottardi et al., 2014). In addition, Ikaros manipulates cellular proliferation by means of suppressing the PI3K pathway and genetic expression that promote cell cycle progression (Song et al., 2015).
2.1 Signaling Pathways About Ikaros Family
Studies on signaling pathways about Ikaros have attracted some attention (Figure 2). The most fully studied Ikaros-related pathway is the preBCR (B-cell receptor) signal pathway, which has formed a distinct picture of the regulatory network (Alkhatib et al., 2012). Reciprocally, the activated preBCR pathway eventually decreased the activity of Ikaros; Ikaros can counteract this effect by suppressing two sites in the pathway (Yasuda et al., 2000; Nera et al., 2006; Nakayama et al., 2009).
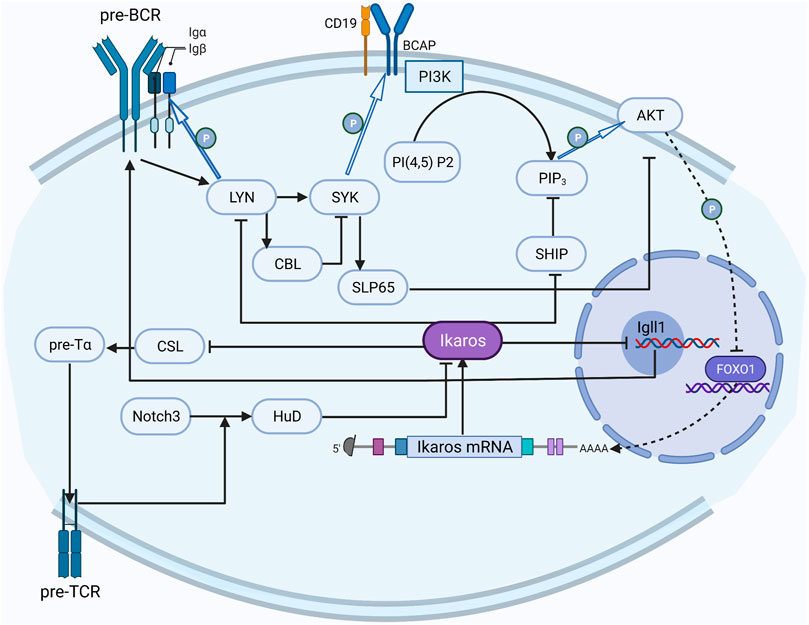
FIGURE 2. The regulation network of Ikaros. The activated preBCR pathway decreases the activity of Ikaros, Ikaros counteracts this inhibition by repressing LYN and SHIP in the pathway; Notch3 upregulates HuD to convert Ikaros to the dominant negative isoform, and Ikaros counteracts the Notch effect on CSL activation through competing with the CSL DNA binding site in the promoter region.
The Notch pathway has also been relatively well studied. Notch3 converts the alternative splicing mode of Ikaros to a dominant-negative isoform by upregulating the expression of RNA binding protein HuD (Bellavia et al., 2007). On the contrary, Ikaros counteracts the Notch effect on CSL activation through competing with the CSL DNA binding site in the promoter region and inhibits the expression of the downstream genes, including the components of preTCR (Dumortier et al., 2006).
It was regarded that ERK1/2-mediated ETS1 phosphorylation decreased the ability of ETS1 to increase Ikaros expression (Joshi et al., 2014). And the activated integrin signaling pathway was reported to be restrained by Ikaros as well. Ikaros is affected by several interferon regulatory factors (IRFs), among which IRF4 and IRF8 were considered to induce the expression of Ikaros and its homologous gene Aiolos (Ma et al., 2008); however, another study showed that IRF8 rather than IRF4 triggers the IKZF1 promoter, and IRF5 could inhibit this activation (Fang et al., 2012).
2.2 Activation of Ikaros
Ikaros activities are thought to be regulated by post-translational phosphorylation, small ubiquitin-related modifier (SUMOylation), and ubiquitination (Sridharan and Smale, 2007). Besides, the hypomethylated pattern of CpG island in the IKZF1 promoter region may be the basis of abnormal Ikaros expression patterns associated with malignant tumors (Chen et al., 2019; Rahmani et al., 2019). SUMOylation interferes in the interaction of Ikaros with transcriptional co-repressors SIN3A, SIN3B, Mi-2β, and CtBP and weakens the inhibitory activity of Ikaros (Gómez-del Arco et al., 2005). And the participations of Ikaros in HDAC-dependent and HDAC-independent inhibition are disrupted by Ikaros SUMOylation, but the nuclear localization to pericentromeric heterochromatin is not affected (Gómez-del Arco et al., 2005). Ikaros SUMOylation was discovered to exist in B-ALL cells, whereas it did not show up in normal peripheral blood leukocytes, indicating its potential work in leukemia (Chen et al., 2019). The process of deSUMOylation was actively modulated by SUMO-specific protease Senp1, Axam, and yeast Ulp1 (Ihara et al., 2007). Certainly, Ikaros is ubiquitinated by E3 ligase CRBN7 and degraded by proteasome under the induction of IMiDs, while inhibition of Ikaros ubiquitination is correlated to interact with some TFs like runt-related transcription factor families (RUNXs) (Zhou et al., 2019; Liu et al., 2021). Otherwise, the absence of the E120 enhancer led to an evidential decrease in Ikzf1 mRNA. Nevertheless, the epigenetic pattern and 3D topology of this locus are only slightly impacted, emphasizing the complicacy of the regulatory pattern of the Ikzf1 locus (Alomairi et al., 2020).
The effect of carcinogenic casein kinase II (CK2) on the phosphorylation of Ikaros has been widely studied. CK2 is a multipotent serine/threonine kinase, which is overexpressed in various cancers, including leukemia (Cunninghame Graham et al., 2011; Jeng et al., 2019; Chen et al., 2020). Studies have shown that CK2 directly phosphorylates multiple amino acids in the whole Ikaros protein, and hyperphosphorylated Ikaros facilitates self-degradation through the ubiquitin/proteasome pathway (Dovat et al., 2011). The application of phosphomimetic esters and phosphoresistant Ikaros mutants found that the phosphorylation of CK2 phosphate sites seriously decreases the ability of Ikaros to bind to DNA and alters the localization to pericentromeric heterochromatin, resulting in the dysfunction of Ikaros proteins (Gurel et al., 2008). Pharmacological inhibition of CK2 can restore the DNA binding ability and tumor inhibitory activity of Ikaros and cause leukemia cytotoxicity in the high-risk model of xenotransplantation in patients with ALL, highlighting the fact that CK2 inhibitors can be used as potential therapeutic strategies for high-risk pediatric leukemia (Song et al., 2015; Gowda et al., 2017a). Ikaros phosphorylation by CK2 is cell periodicity, indicating that CK2 effects the regulation of Ikaros function during G1/S transition and S phase in human leukemia (Arco et al., 2004; Li et al., 2012). Besides, CK2-mediated phosphorylation of Ikaros was vital to regulate the transcriptionally of the terminal deoxy transferase gene during differentiation of thymocytes (Wang et al., 2014). SYK is able to phosphorylate Ikaros at dissimilar sites, affecting Ikaros’ nuclear localization (Uckun et al., 2012).
CK2-mediated phosphorylation is reversed by PP1 to dephosphorylate Ikaros (Popescu et al., 2009; Song et al., 2011). The mutation of the PP1 interaction site of Ikaros or the pharmacological inhibition of PP1 lead to the hyperphosphorylation of Ikaros, which seriously reduces the DNA binding ability of Ikaros, loses the pericentromeric localization of Ikaros, and increases degradation of Ikaros through the ubiquitous protein pathway (Popescu et al., 2009).
2.3 Molecular Mechanisms of Ikaros in Immune Cells
Ikaros has different effects on the growth, reproduction, and differentiation of many kinds of innate or adaptive lymphocytes in vivo (Figure 3). It has been previously reported that Ikaros is vital for the conversion between the large and small pre-B stages (Schwickert et al., 2014). Ikaros also sustains B-cell proliferation and differentiation through initiating kinase-signaling cascades and collaborating with chromatin protein 4 (Ochiai et al., 2020). In addition, the plasma cell mal-differentiation of sub1 deficient B cells can be saved by Ikaros and IRF4 (Ochiai et al., 2020). For innate lymphoid cells, Ikaros, the significant regulator of ILC3 existence and function, represses the transcriptional activity of aryl hydrocarbon receptors in a zinc finger-dependent manner, inhibits ILC3 in a cellular manner, and controls intestinal immune response in steady-state and disease (Li et al., 2016). Ikaros and Aiolos play a critical role in regulating the trans-differentiation of ILC3-ILC1/NK cells (Bald et al., 2019).
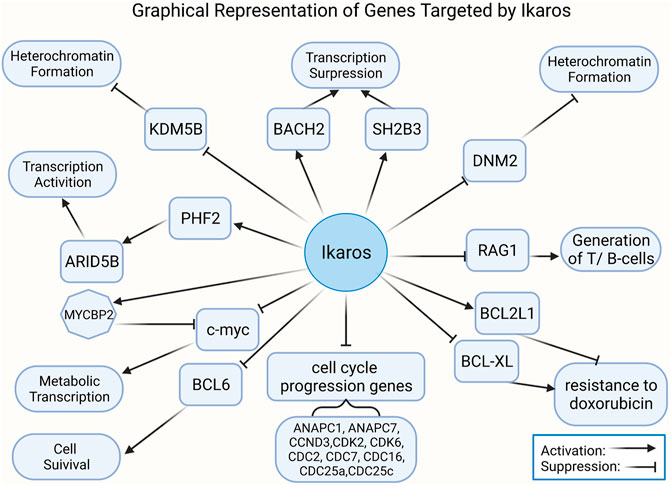
FIGURE 3. Illustration of genes regulated by Ikaros in tumors. Ikaros is a tumor suppressor gene, controlling the transcription of ma genes related to leukemogenesis.
IKZF1 was demonstrated to regulate embryonic T lymphopoiesis of zebrafish via chemokine receptor 9 and IRF4 (Huang et al., 2019). Members of the IKZF family are also involved in the differentiation and property of single T helper cell subsets, covering TH1, TH2, TH17, T follicular, and Tregs (Powell et al., 2019). The function of Ikaros protein in T cell differentiation has been revealed, whereas contradictory results were obtained in certain mouse models. Research utilizing T cells possessing germline Ikaros gene mutations demonstrated that Ikaros promotes Th17 and Treg cell differentiation and suppresses the polarization of Th1 (Cippitelli et al., 2021). Nevertheless, in a mouse model in which Ikaros conditioned knockout in mature T cells, the deficiency of Ikaros is related to the acquirement of Th1, Th2, Th17 but not Treg cells (Lyon de Ana et al., 2019).
Ikaros supports signal-induced downregulation of recombination-activating gene 1 (RAG1) and RAG2 gene expression in CD4 + CD8+ positive thymocytes through a non-redundant manner (Naik et al., 2019). Ikaros plays a role in preventing autoimmunity by administering BCR unresponsiveness and repressing TLR signaling transduction (Schwickert et al., 2019). Although lymphocytes need Ikaros to differentiate, the role of the Ikaros protein family in the myeloid cell is not clear (Park et al., 2015). The mouse model has shown that Ikaros participates in the regulation of differentiation of neutrophils by silencing permissible or specific pathways in the ordinary precursors of macrophage-monocyte evolution (Dumortier et al., 2003). Ikaros modulates early phase differentiation of neutrophils but is optional for mature neutrophils (Dumortier et al., 2003).
2.4 Tumorigenesis and Antitumor Effect of Ikaros
Ikaros appears to function as a transcriptional inhibitor and activator by binding to different targets, such as some nuclear factors related to epigenetic regulation (Figure 4). Ikaros suppresses target gene transcription by directly binding or recruiting HDAC1 (Ikaros-HDAC1 complex) to induce the formation of inhibitory chromatin: the former way results in the increase of H3K9me3 and the reduction of H3K9ac, while the latter complex can promote the affinity for DNA binding to the promoter of lysine [K]-specific demethylase 5B (KDM5B) by CK2 inhibitors and raise formation of H3K27me3 and reduce H3K9ac (Song et al., 2016; Wang et al., 2016). The CK2-Ikaros axis also exerts beneficial control over Ikaros target gene expression, increasing PHD finger protein 2 (PHF2) expression, which forms a complex with AT-rich interactive domain-containing protein 5B (ARID5B) to activate the target genes’ transcription (Ge et al., 2018a; Ge et al., 2018b). The phosphorylation by CK2 overexpressed in B-ALL depresses Ikaros combining and recruiting HDAC1 to the promoter of BCL2L1, which causes repression of BCL2L1 and increases expression of BCL-XL (Schott et al., 2020). Recent studies have indicated that Ikaros has a negative effect on the development of T cell leukemia via globally regulating the enhancer or super-enhancer landscape and pioneering activity (Ding et al., 2019). Ikaros has a critical role in regulating de novo enhancer formation, super-enhancers formation, depletion of enhancers, and stimulation of poised enhancers, demonstrating that Ikaros direct modulates the expression of numerous genes than hypothesized formerly (Gowda et al., 2020). Furthermore, miR-26b expression is induced by the differential expression of Ikaros isoforms and transcriptional regulators of miR-26b modulated by PTEN (Yuan et al., 2017). The negative prognosis of IKZF1 deletion in BCP-ALL may be strengthened by the activation of Janus kinase signal transducer and activator of transcription (JAK/STAT) signaling and recede by ERG deletion (Stanulla et al., 2018). The mutual control of Notch1 and Ikaros in DN2 subsets of the thymus in tumor-bearing mice promotes the early stagnation of T cell development at the DN2a stage and its transfer to dendritic cells lineage (Guha et al., 2020). In terms of metabolism, the expression of functional paired box 5(PAX5) and Ikaros induces the powerful upregulation of glucose-6-phosphate dehydrogenase (G6PD). Interestingly, in clinical trials of patients with lymphoma, patients with high expression of G6PD were linked to poor overall prognosis (Xiao et al., 2018).
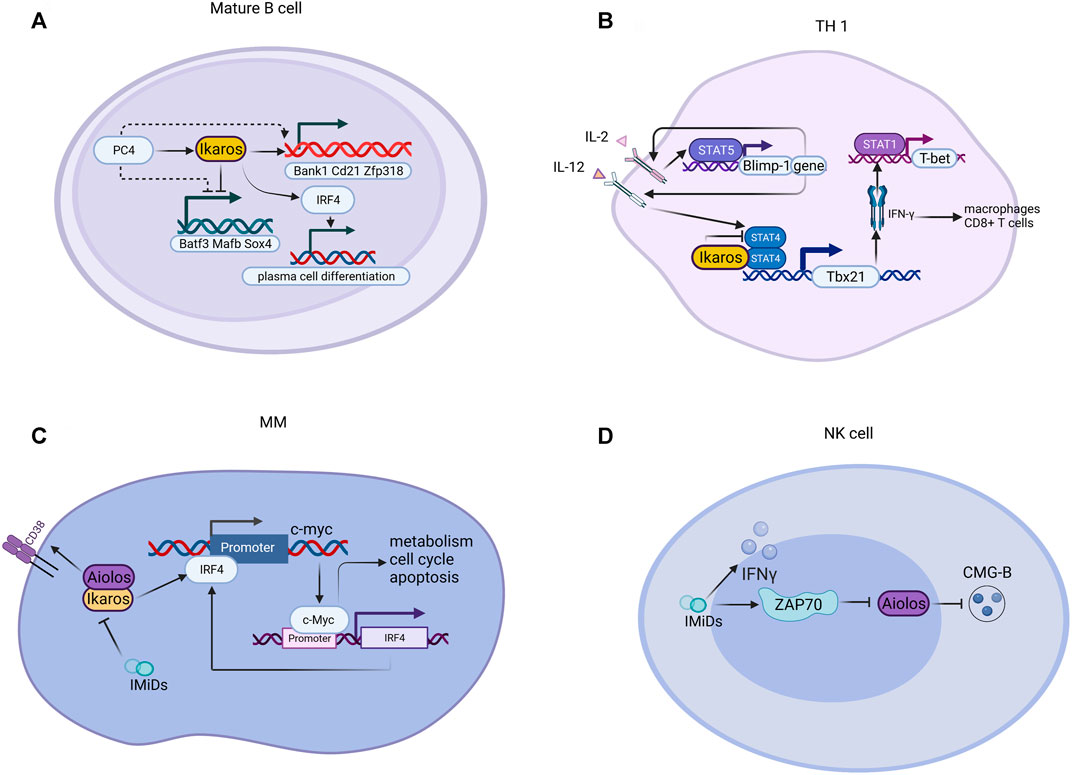
FIGURE 4. Mechanism of Ikaros family proteins in various cells. (A) For mature B cells, PC4 increases and cooperatively assists Ikaros proteins to regulate gene expression; the PC4-IKAROS-IRF4 axis activates genes (e.g., Bank1, Cd21, and Zfp318), represses non-B cell genes (e.g., Batf3, Mafb, and Sox4), and promotes plasma cell differentiation. (B) Ikaros suppresses the polarization of Th1 through suppressing transcription mediated by STATA4 and downstream factors. (C) IMiDs interfere with disease-promoting activities of c-Myc and IRF4 via Aiolos and Ikaros in MM. (D) IMiDs bind to ZAP70, downregulate Aiolos, and enhance CMG-B. CMG-B, cytotoxic molecular granzyme B.
These variants of IKZF1 were distributed across the whole gene, particularly located to the C-terminal zinc finger dimerization domain, whereas in immunodeficient patients, germline IKZF1 variants are restricted to the N-terminal zinc fingers (Churchman et al., 2018). IKZF1 mutations and deletions have been suggested to contribute to the occurrence and poor prognosis of ALL and AML (Mullighan et al., 2008; Mullighan et al., 2009; de Rooij et al., 2015; Zhang et al., 2020). Deletion of IKZF1 was also demonstrated as an acquired convert at the transformation phase from CML to lymphoid blast crisis or ALL (Li et al., 2018b). The IKZF1 deletions lead to haploinsufficiency, dominant-negative Ikaros forms, or complete loss of expression of Ikaros. The principle of this dominant-negative effect may be that the high level of mutant unbinding DNA isomers with less than three N-terminal sequences occurs through the formation of heterodimers with wild-type isomers, resulting in alteration in DNA binding properties and them becoming repressors (Vairy and Tran, 2020). Mutations at the C-terminal zinc fingers could also cause a deep effect on the transcription of target genes (Vairy and Tran, 2020). Even after a comprehensive analysis of DNA binding sites of Ikaros in murine hematopoietic cells, the molecular mechanism of tumor suppressor effects in leukemia regulated by Ikaros-mediated transcription is still not clear (Song et al., 2015).
Overexpression of Ikaros leads to inhibition of transcription of genes supporting the PI3K pathway and generates transcription of genes, like INPP5D, repressing the PI3K pathway (Song et al., 2015). And AKT phosphorylation decreasing by overexpression of Ikaros in leukemia cells is identical to the effect of treatment with imatinib (Gowda et al., 2017a). Nevertheless, the JAK/STAT pathways and PI3K/AKT pathways were proved to activate with Ik6 expression in ALL (Song et al., 2015; Qu et al., 2019). Ikaros overexpression also directly suppresses the promoter activity of these clinically significant cell cycle progression genes, including ANAPC1, ANAPC7, CCND3, CDK2, CDK6, CDC2, CDC7, CDC16, CDC25a, CDC25c, and CCNE2 (Dhanyamraju et al., 2020). In addition, KDM5B expression is downregulated by Ikaros through recruiting HDAC1 to the KDM5B gene promoter, which causes an inhibitory chromatin condition and transcriptional inhibition consequently (Dhanyamraju et al., 2020). Ikaros upregulates the PHF2 expression through chromatin remodeling, as demonstrated by the appearance of increased H3K4me3 in the promoter of the PHF2 gene (Ge et al., 2016a; Ge et al., 2018a; Maciel et al., 2019). The expression of ARID5B is positively adjusted by Ikaros, and the loss of a single copy of IKZF1 is associated with low ARID5B expression (Ge et al., 2018b). Gain- and loss-of-function experiments of Ikaros in ALL shows that Ikaros inhibits c-myc gene transcription but controls transcription of MYC binding protein 2 positively, which suppresses the activity of myc (Ge et al., 2015). STAT5 regulates the super-enhancer of the myc gene by means of competition for binding to target sites or regulating histone acetylation in an opposing way to Ikaros (Katerndahl et al., 2017). It was revealed that the genes related to the BCR signal pathway and the IKZF family are presumed to be upstream of the MYC/IRF4 axis (Tsukamoto et al., 2020). In patients with trisomy 12 CLL, IRF4 by means of inducing Ikaros mediates the overexpression of CD49d, which was considered to identify those patients treated with ibrutinib with the characteristics of inferior nodal responses and shorter clinical outcomes (Fiorcari et al., 2019).
Moreover, Ikaros was also verified to negatively regulate IL7R expression and promote SH2B adaptor protein 3 (SH2B3) transcription (Ge et al., 2016b). Ikaros represses B-cell lymphoma 6 (BCL6) transcription and initiates basic leucine zipper transcription factor 2 (BACH2) gene transcription, suggested by BCL6 and BACH2 functional experiments (Ge et al., 2017). Some data identified that Ikaros inhibits dynamin 2 in leukemia by directly combining with the promoter and inducing heterochromatin formation (Ge et al., 2016). PAX5, RUNX1, and IKZF1 were found to overlap abundant target genes, and a modality with dominant-negative effective of IKZF1 or ETV6-RUNX1 fusion protein cooperates with the loss of heterozygosity of PAX5 to regulate gene expression (Okuyama et al., 2019). Furthermore, RAG1 is considered as an immediate target of Ikaros (Li et al., 2016; Dimopoulos et al., 2019). IKZF1 was defined as a crucial trans-activator of the SLAMF-7 gene, which is mainly expressed in MM cells and deemed as an ideal target for immunotherapy (Kikuchi et al., 2020).
In contrast to the role of IKZF2 as a tumor suppressor gene in hypodiploid B-ALL, it is essential for AML (Park et al., 2019). The deficiency of IKZF2 in AML cells is related to the reduction of colony formation, augment of differentiation and apoptosis, defective leukemic stem cells function, and tardive leukemogenesis (Park et al., 2015; Park et al., 2019). One of the main mechanisms may be that IKZF2 determines a self-renewal gene expression procedure called HOXA9 and represses a C/EBP-driven differentiation program. In addition, this forced reduction of Ikzf2 is accompanied by decreasing HOXA9 and BCL2, but not Mll, Myc, or Meis1.
3 Targeted Therapy for Ikaros
IMiDs, covering thalidomide, Len, and pomalidomide, are clinically approved medicine for the therapy of MM and other malignancies (Table 2). Among them, the relatively well-studied diseases are MM and MDS. These drugs lead to selective ubiquitination and proteasomal degradation of Ikaros and Aiolos through recruiting TFs to the CRL4CRBN E3 ubiquitin ligase, representing an original mechanism of therapy through altering the substrate specificity (Lu et al., 2014; Sievers et al., 2018; Zeidner et al., 2020). Different IMiDs target the degradation of distinct sets of TFs. Furthermore, IMiDs have the ability of direct inhibition of tumor cell growth and strong immunostimulatory characteristics, consequently having multiple implications on the presence of different cellular components in the tumor microenvironment (Cippitelli et al., 2021).
3.1 Antitumor Effect of IMiDs in MM
The deletion of Ikaros and Aiolos maintains the occurrence and progress of MM by contributing to downregulate c-Myc and IRF4 (Bjorklund et al., 2015). There is a positive feedback loop between IRF4 and c-Myc; IRF4 combines with the region of c-myc promoter and promotes c-myc expression, while the C-Myc protein transactivates the IRF4 gene directly (Shaffer et al., 2008). The target genes of C-Myc cover genes that regulate cell metabolism (GLUT1), cell cycle (CDKs, cyclins, and E2FTF), and apoptosis (Jovanović et al., 2018). On the side, Krüppel-like factor 2 (KLF2) and B lymphocyte maturation inducing protein-1 (Blimp-1) exert a significant effect on regulating the growth and survival of MM. In the positive feedback loop, IRF4 and KLF2 transactivate and promote the expression of each other and are also upregulated by KDM3A via removing H3K9me marks at promoters (Ohguchi et al., 2016). Therefore, the lessening of each protein induced by IMiDs represses the expression of integrin alpha-4/beta-7, thus affecting the migration and homing of MM cells to bone marrow. In addition, the expression and activity of Blimp-1 decreased by IMiDs through a variety of mechanisms: 1) Blimp-1 is repressed at the transcriptional level as the target gene of IRF4; 2) Aiolos interacts with Blimp-1 and synergistically inhibits apoptosis-related genes; and 3) ubiquitination and proteasome degradation of Blimp-1 can be enhanced directly (Hung et al., 2016). Moreover, the degradation of Ikaros and Aiolos induced by IMiDs can upregulate the surface expression of CD38 in MM cells, preparing for the antibody-dependent cytotoxicity induced by daratumab in NK cells (Fedele et al., 2018).
3.2 Immunomodulatory Activity of IMiDs in MM
IKZF TFs are central to regulate the innate or adaptive immune response in patients with MM. The ILCs consist of lymphoid tissue inducer cells, NK cells, ILC1s, ILC2s, and ILC3s (Vivier et al., 2018; Krabbendam et al., 2021). For NK cells, their activity induced by IMids is due to the fact that T lymphocytes are stimulated to produce IL-2 (Gandhi et al., 2014). Drugs also directly bind to activate kinase ZAP70, downregulate Aiolos, and enhance the expression of cytotoxic molecular granzyme B (Hideshima et al., 2021). Len directly enhances the activity of NK cells by increasing the proportion of synapses that can penetrate IFN-γ vesicles and increasing the production of IFN-γ (Lagrue et al., 2015). Len and pomalidomide increased the MICA expression of NK cell-mediated immune surveillance molecule NKG2D ligand and PVR/CD155 of dNaM-1 ligand on the human MM cell membrane, thus enhancing the sensitivity to the identification and killing of MM cells (Fionda et al., 2015). As for ILCs, it was demonstrated that the activity of ILCs was enhanced by reducing Ikaros and Aiolos protein (Bald et al., 2019). The 2 TFs are significant regulators for the conversion of ILC3 into ILC1/NK cells. Len increased the expression of certain ILC3-related genes (such as rorc, baff, il22, and nrp1) and upregulated the proportion of ILC3 producing IL-22. Concomitantly, a process of transdifferentiation of ILC3 to ILC1 was inhibited by Len with the downregulation of Aiolos and depression of ILC1-associated transcripts (prf1, gzmb, cd244, lef1, and ncr3). After treatment with Len, DC differentiated from peripheral blood and bone marrow monocytes in patients with MM expressed higher standards of cytokines and chemokines (IL-8, TNF, CCL2, and CCL5) and strengthened the function to stimulate the proliferation of allogeneic T cells (Costa et al., 2017).
IMiDs promote specific CD4+ and CD8+ T cell responses in MM, whereas they inhibit the expansion and function of Tregs. T cells with less expression of Ikaros require less TCR to participate in immune cell activation, show greater proliferation response to IL-2, and are less sensitive to inhibitors of TCR and IL-2R signal transduction, suggesting that the lower the expression of Ikaros, the lower the T cell activation threshold (Avitahl et al., 1999). Besides, the loss of IKZFs is the reason for the increase of IFN-γ, IL-21, and IL-2 production by T cells treated with IMiDs (Gandhi et al., 2014; Brissot et al., 2015). In addition, Len also decreased the levels of Ikaros and Aiolos in chimeric antigen receptor (CAR) T-cells, which helped to enhance the ability of CAR T-cells to restore IL-2 gene transcription and facilitate IL-2 secretion against solid cancer cells (Wang et al., 2020b).
4 Clinical Applications
4.1 Immunomodulatory Drugs
IMiDs, including thalidomide, Len, and pomalidomide, are effective in treating certain hematological malignancies, in combination with steroids, proteasome inhibitors (PIs), or monoclonal antibodies, such as MM (Gao et al., 2020), MDS with deletion of chromosome 5q (Fenaux et al., 2011), MCL (Ruan et al., 2018), and CLL (Vitale et al., 2016). IMiDs exert their antitumor activity directly through different treatment-related effects, such as inhibiting angiogenesis, repressing cancer cell proliferation, and inducing apoptosis (Le Roy et al., 2018). The cellular target of IMiDs is CRBN, a ubiquitous protein that acts as a substrate receptor for Cullin-4-RING E3 ubiquitin ligase complexes, as well as DDB1, ROC1, and CUL4.
4.1.1 Treatment of IMiDs in MM
Different IMiDs have been applied to different conditions. Thalidomide was the first IMiD to be discovered, and its history served as a lesson in the history of drug development (Franks et al., 2004). Len is commonly used in new MM patients, maintenance therapy after transplant, and relapsed/refractory MM (RRMM) patients, while pomalidomide is only applied to RRMM (Zou et al., 2020).
Patients with newly diagnosed MM (NDMM) who were unsuitable for stem cell transplantation are associated with a considerable benefit in progression-free survival (PFS) [lenalidomide-dexamethasone vs. melphalan-prednisone-thalidomide (MPT); HR, 0.72; p < 0.001] and overall survival (OS) at the interim analysis and less frequent grade 3 or 4 toxic and adverse events (70 vs. 78%), with continuous lenalidomide-dexamethasone intervention until disease progression compared with MPT (NCT00689936) (Benboubker et al., 2014). For patients with MM after transplantation, maintenance therapy with Len significantly extended PFS (41 months, vs. 23 months with placebo; HR, 0.50; p < 0.001), while differences in OS were not discovered in two cohorts (NCT00430365) (Attal et al., 2012). Identically, Len maintenance significantly improved PFS in patients with NDMM but did not enhance OS in the trial population (ISRCTN49407852) (Jackson et al., 2019). Early intervention with Len in smoldering MM notably decelerates progression to symptomatic MM and the damage of target-organ. The 1-, 2-, and 3-years progression-free survival for the lenalidomide and observation arm was 98 vs. 89%, 93 vs. 76%, and 91 vs. 66%, respectively (NCT01169337) (Lonial et al., 2020). Moreover, in contrast to high-dose dexamethasone, pomalidomide plus low-dose dexamethasone significantly prolonged median PFS (4.0 months, vs. 1.9 months with high-dose dexamethasone; HR, 0.48; CI, 0.39–0.60; p < 0.0001), and is considered a new medical strategy for patients with RRMM, despite the fact that the occurrence of grades 3–4 neutropenia were higher (NCT01311687) (Miguel et al., 2013). In another phase III trial, on the basis of BTZ and dexamethasone, pomalidomide was added in the treatment of patients with RRMM to significantly improve PFS (11.20 vs. 7.10 months; HR, 0.61; 95%CI, 0·49–0.77; p < 0·0001), with acceptable hemocyte toxicity (NCT01734928) (Richardson et al., 2019). In addition to malignancy, pomalidomide is a well-tolerated and efficient treatment for advanced steroid-refractory chronic graft-versus-host disease (cGVHD), indicating the antifibrotic effects of pomalidomide are related to the increased levels of blood regulatory T cells and IL-2 (NCT01688466) (Curtis et al., 2021).
Subcutaneous BTZ delivery + lenalidomide + dexamethasone (VRD) is a safe and effective therapeutic schedule for induction in NDMM with valuable partial responses (55.6% by cycle 3, 63.8% by cycle 4, 68.3% by cycle 5, and 70.4% after induction) (NCT01916252) (Rosiñol et al., 2019). Furthermore, pretreatment of cells with BTZ, a PI, leads to the accumulation of Ikaros, thus suppressing the efficacy of Len (Shi et al., 2015). Nevertheless, with the therapy of Len combined with BTZ, Ikaros was demonstrated to be degraded by a pathway independent from the autophagy and proteasome pathways, such as activating calpain and caspase to downregulate Ikaros by calcium flux (Ganesan et al., 2020).
Pan-proviral insertion in murine malignancy kinase inhibitors covering SGI1776 and LGH447 presented inspiriting results in patients with RRMM, which was associated with upregulation of CRBN and downregulation of Ikaros and Aiolos (Zheng et al., 2019). Nonselective HDAC inhibitors like A452 induce synergistic cytotoxicity of MM without modifying CRBN expression combined with IMiDs, thus downregulating IKZF1/3, c-Myc, and IRF4 (Won et al., 2019).
4.1.2 Treatment of IMiDs in Leukemia
IMiDs are conventionally used for the therapy of MM, MDS, and B-cell lymphoma; nevertheless, little is realized about the efficacy of IMiDs in AML (Le Roy et al., 2018) (Table 3). Len induces degradation of Ikaros, reducing the expression of GPR68 and RCAN1, which upregulates the Ca2+/calpain pro-apoptotic pathway and inhibits the CaN pro-survival pathway, respectively. Hence, cyclosporine, a CaN inhibitor, is able to extend the therapeutic potential of Len to MDS as well as AML without affecting immune function (Dou and Fang, 2020). Compared to azacytidine (AZA) monotherapy, patients with higher-risk MDS treated with AZA in conjunction with Len or Vorinostat achieved an overall response rate (ORR). In stratified analysis, patients with CMML could benefit from AZA plus Len (NCT01522976) (Sekeres et al., 2017). In patients with 5q-deletion-related MDS, Len rendered significant clinical outcomes compared with thalidomide and pomalidomide, which was related to induced degradation of CK1 α (Krönke et al., 2015; Petzold et al., 2016). Len was revealed to be given safely in patients with a relapsed AML/MDS post-allograft combined with AZA without reversing impaired INF-γ/TNF-α production; 7 of 15 (47%) patients ultimately reached a major clinical response after LEN/AZA therapy (ISCRCTN98163167) (Craddock et al., 2019). For patients with AML, the results of the current study illustrated that the addition of Len to standard remission induction chemotherapy (cytarabine + daunorubicin) could not ameliorate the therapeutic effect of elderly patients with AML (NTR2294) (Ossenkoppele et al., 2020).
In a phase I trial, following timed sequential induction therapy (TST), pomalidomide administration in the early stages of lymphocyte recovery has been revealed to be well tolerated in patients with newly diagnosed AML and adverse cytogenetics of MDS with particularly high CR rates (NCT02029950). The exact mechanism of benefit from pomalidomide application after chemotherapy remains obscure. It is of probability that pomalidomide remarkably decreases Aiolos expression in peripheral blood and bone marrow CD4+/CD8+ T cells, strengthens T cell differentiation and proliferation, and promotes cytokine production (Zeidner et al., 2020). Additionally, pomalidomide may be serviceable for the treatment of HTLV-1 and EBV-induced tumors by causing infected cells to become more vulnerable to innate and adaptive host immune responses (Davis et al., 2019).
Lenalidomide has been approved for application with rituximab in patients with relapsed/refractory follicular lymphoma in the United States. Lenalidomide + rituximab was demonstrated to be a safe and effective chemotherapy-free approach that improves upon single-agent rituximab and may become a substitution to chemoimmunotherapy (Flowers et al., 2020). Efficacy results of rituximab plus lenalidomide were similar to rituximab plus chemotherapy and both regimens were followed by rituximab maintenance therapy among patients with untreated follicular lymphoma. In contrast, the two groups presented different safety profiles. A higher percentage of grades 3 or 4 neutropenia appeared in the rituximab + chemotherapy group (32 vs. 50%) (NCT01476787 and NCT01650701) (Morschhauser et al., 2018). PFS of patients with recurrent indolent lymphoma was notably promoted for lenalidomide plus rituximab compared with rituximab plus placebo (HR, 0.46; 95% CI, 0.34–0.62; p < 0.001), and the safety profile of the test group was acceptable (NCT01938001) (Leonard et al., 2019). For patients with DLBCL who achieved CR or PR by R-CHOP induction, a randomized phase III trial demonstrated that Len maintenance monotherapy in elderly patients with DLBCL is safe and effective (mPFS was not reached, vs. 58.9 months with placebo; HR, 0.708; 95% CI, 0.537–0.933; p = 0.01) (Thieblemont et al., 2017). Despite inescapable toxicity (63%, vs. 12% in observation group; p < 0·0001), Len improved PFS in patients with an MCL post-autograft (NCT02354313) (Ladetto et al., 2021).
Long-term IMiDs used continuously, like maintenance treatment for MM, may raise the frequency of uncontrolled immunostimulatory diseases by inducing a chronic inflammatory response, resulting in certain autoimmune diseases covering vasculitis, rashes, optic neuritis, interstitial pneumonitis, Graves’ disease, and polymyositis (Montefusco et al., 2014; Brissot et al., 2015; Shao et al., 2017; Phan et al., 2020; Shafi et al., 2020; Cippitelli et al., 2021).
4.2 Treatment of Cereblon-Modulating Agent
CRBN E3 ligase modulating drugs (CELMoDs), such as avadomide (CC-122), iberdomide (CC-220), CC-885, CC-92480, and novel thalidomide analogs, are in current clinical trials as a monotherapy and in combination (NCT01421524; NCT02773030; NCT03374085) (Table 4). The CELMoDs have strong antitumor and immunostimulatory capabilities in patients with hematological malignancies (Matyskiela et al., 2016; Matyskiela et al., 2018; Ito and Handa, 2019; Rasco et al., 2019; Gao et al., 2020; Hansen et al., 2020).
CC-122 (avadomide) was combined with CRL4CRBN E3 ligase to conduce the degradation of Ikaros and Aiolos in MM cells and DLBCL cells (Hagner et al., 2015). CC-122 has been applied to a variety of clinical trials for diverse diseases, including non-Hodgkin’s lymphoma, MM, HCC, melanoma, and CLL/SLL (Rasco et al., 2019). It was found in research in pre-clinical experiments and a phase I clinical trial that avadomide + obinutuzumab was a tolerable therapeutic regimen for plenty of patients with manageable toxicity. Nevertheless, the predetermined activity threshold was not reached in this trial. There is no denying that cereblon-modulators plus anti-CD20 antibodies should be investigated further as a chemotherapy-free approach for relapsed or refractory non-Hodgkin lymphoma (NCT02417285) (Michot et al., 2020). In another phase I clinical trial, avadomide monotherapy presented manageable toxicity and promising pharmacokinetics in patients with solid tumors, NHL, and multiple myeloma. Of five patients with NHL, one realized CR, and two achieved PR (NCT01421524) (Rasco et al., 2019).
CC-220 (iberdomide) is another analog of thalidomide, which enhances the efficacy to downregulate Ikaros and Aiolos by tightly binding to CRL4CRBN E3 ligase for the treatment of RRMM and SLE (Matyskiela et al., 2018). The peripheral blood mononuclear cells of patients with SLE showed strikingly higher levels of IKZF1 (2.1-fold) and IKZF3 (4.1-fold) mRNA than a healthy person. And iberdomide significantly decreased levels of Ikaros and Aiolos protein in B cells, T cells, and monocytes, supporting its further clinical development for treating SLE (NCT01733875) (Schafer et al., 2018).
In addition to the pharmacologic function of CC-220, CC-885 can also induce CRL4CRBN-dependent delegation of translation termination factor GSPT1, which cannot be degraded by neither Len nor pomalidomide, demonstrating the spectrum of the different substrates of CC-885 from pomalidomide and Len (Matyskiela et al., 2016). Hence, CC-885 may have the extra potential for AML therapy. Relevant pre-clinical and clinical trials are ongoing and being analyzed due to their novelty. CC-92480 is another novel protein degrader acting on the refractory/relapsed MM (RRMM) of Len (Hansen et al., 2020).
4.3 Treatment of Proteasome Inhibitors
PIs are a class of important drugs for the treatment of MM and MCL, and are being studied for the treatment of other diseases. BTZ is the first PI to be approved by the Food and Drug Administration (FDA) of the United States. Carfilzomib and ixazomib have been approved successively, and more drugs are in development (Fricker, 2020). The phase III GIMEMA-MMY-3006 trial illuminated the superiority of the BTZ + thalidomide + dexamethasone (VTD) regimen over thalidomide and dexamethasone (TD) in improving CR and prolonging PFS (HR, 0·60; 95% CI, 0·48–0.76; p < 0·0001) as an induction regimen before and intensification therapy after double autologous hematopoietic stem-cell transplantation (ASCT) for patients with NDMM (NCT01134484) (Cavo et al., 2010; Tacchetti et al., 2020). The randomized phase III trial SWOG S0777 and long-term follow-up of SWOG S0777 revealed a similar pattern that the addition of BTZ to Len and Dex for induction therapy led to a statistically and clinically meaningful increase in PFS as well as OS (NCT00644228) (Durie et al., 2017; Durie et al., 2020). Another trial demonstrated that the first single-agent ixazomib option in patients with NDMM not undergoing ASCT promoted mPFS (17.4 vs. 9.4 months; HR, 0.659; 95% CI, 0.542–0.801; p < 0.001; median follow-up, 21.1 months) without unexpected toxicity (NCT02312258) (Dimopoulos et al., 2020a). For combination therapy of ixazomib, the addition of ixazomib to a Len and Dex regimen was correlated to longer PFS (20.6 vs. 14.7 months in Len and Dex; HR, 0.74; p = 0.01) with limited toxic effects (NCT01564537) (Moreau et al., 2016). Besides, in patients with RRMM, carfilzomib in conjunction with Len and Dex (KRd) generated markedly improved mPFS (26.3 months, vs. 17.6 months in the Len and Dex group; HR, 0.69; 95% CI, 0.57–0.83; p = 0.0001) at the interim analysis and presented an improving risk-benefit profile (NCT01080391) (Stewart et al., 2015). However, the KRd scheme cannot effectively improve PFS (34·6 months vs. 34·4 months in the Len and Dex group; HR, 1·04; 95% CI, 0.83–1.31; p = 0·74) compared with a control group in patients with NDMM, and had more toxicity (NCT01863550) (Kumar et al., 2020).
4.4 Immunotherapy
Immunotherapy is one of the most promising treatments for several cancers. In future clinical trials, early identification of high-risk patients with ALL is essential for the best treatment, and to ensure innovative treatments, such as immunotherapy, are introduced in a timely manner (Stanulla et al., 2018). In some studies about certain solid tumors that lack IKZF1 expression, overexpression of Ikaros leads to increased immune recruitment infiltration and tumor sensitivity to cytotoxic T lymphocyte-associated antigen-4 (CTLA4) and programmed cell death protein 1 (PD1) inhibitors (Chen et al., 2015; Chen et al., 2018). Nevertheless, meaningful MM regression was not achieved by single-drug PD-1 blockade in early clinical studies. Besides, the FDA established that the risks of the pembrolizumab plus pomalidomide and dexamethasone combination outweighed its benefits for patients with RRMM and halted the study (NCT02576977) (Mateos et al., 2019). Equally, the interim analysis results presented that the benefit-risk event of pembrolizumab plus lenalidomide and dexamethasone is adverse for patients with NDMM. Survival and long-term safety follow-up are ongoing (NCT02579863) (Usmani et al., 2019). CAR-T immunotherapy faces many problems in the treatment of solid tumors, and T-cell dysfunction or failure is one of them. Len improved CAR-T function by inducing the degradation of Ikaros and Aiolos, promoting the killing of CD133-CAR-T and increasing the secretion of the cytokine and the proliferation of CD133-CAR-T (Wang et al., 2020b).
4.5 Targeted Therapy
Adding imatinib to the therapy for patients with BCR-ABL1 fusion to strengthen the treatment of IKZF1-del patients can eliminate the adverse effect of IKZF1-del in contemporary ALL treatment (Yeoh et al., 2018). Imatinib, as an intensifying therapy for childhood B-ALL with IKZF1-del, significantly decreased the risk of recurrence and improved the 5-years OS from 69.6% in MS2003 to 91.6% in MS 2010 (p = 0.007) (NCT0289464) (Yeoh et al., 2018). Besides, ibrutinib also presented enduring single-agent efficacy in patients with relapsed or refractory MCL. A CR rate of 21% and a PR rate of 47% were detected. And the most common adverse events related to treatment were diarrhea, fatigue, and nausea (NCT01236391) (Wang et al., 2013).
DD-03–171 is an optimized Bruton tyrosine kinase (BTK) inhibitor analog, which showed an enhanced anti-proliferation outcome on MCL cells in vitro through the degradation of BTK, Ikaros, and Aiolos (Dobrovolsky et al., 2019). Acalabrutinib, a selective second-generation BTK inhibitor, provided superior durable responses and an acceptable toxicity profile in patients with relapsed or refractory MCL. At the median follow-up of 15.2 months, 81% of patients attained an overall response, and 40% of patients achieved a CR. The most common adverse events were headache (38%), diarrhea (31%), fatigue (27%), and myalgia (21%) (NCT02213926) (Wang et al., 2018).
The CK2 inhibitor CX4945 can restore Ikaros function and exert the antileukemia effect in vitro or in pre-clinical leukemia models (Gowda et al., 2017b; Borgo et al., 2021). There are also many cancer clinical trials currently running (NCT01199718, NCT00891280, NCT03897036, NCT02128282, NCT03904862, and NCT03571438).
Bromodomain-containing protein 4 (BRD4) is a chromatin-binding protein that was considered to immediately regulate a range of genes involved in the BCR signal pathway, covering Aiolos, B-cell linker, PAX5, and several oncogenes, like MYB. Thalidomide derivatives to various Brd4 small molecule inhibitors through different junctions were used to synthesize CRL4CRBN E3-based PROTAC targeting Brd4. For example, the synthesis of many pre-clinical trials like dBET1, ARV-825, BETd-246, BETd-260, and QCA570. Of course, there are other degradation pathways, such as the CRL2VHL E3-based pathway (Duan et al., 2018). BRD4 inhibitors were suggested to enhance the anti-MCL effect of Len or ibrutinib synergistically. It works even in BTZ-resistant MCL cells (Tsukamoto et al., 2020).
In breast cancer, the application of ginseng polysaccharide was able to suppress MDA-MB-231 cell proliferation by the activation of IKZF1 (Zhou et al., 2020). The anticancer capability of imatinib in Ik6+ and Ph + ALL can be strengthened by plant elements such as Huaier extract (Qu et al., 2019). The use of caffeic acid phenethyl ester analogs in the so-called Achilles heel of myeloma, aimed at the Ikaros/IRF4 axis, showed positive clinical outcomes (Murugesan et al., 2020).
Daratumumab, a monoclonal antibody targeting CD38, has presented substantial efficacy as a monotherapy in heavily pretreated and refractory patients with MM and in conjunction with bortezomib in patients with NDMM as well as with IMiD in patients with RRMM. Overall responses were noted in 29.2% of refractory patients with MM treated with daratumumab monotherapy (NCT01985126) (Lonial et al., 2016; Usmani et al., 2020). In addition, the combined administration may be an ideal backbone for the future research of anti-CD38 monoclonal antibodies in terms of their good safety and oral administration. The addition of daratumumab with Len-Dex induced a high ORR and PFS, markedly decreasing the risk for progression and associated death in patients with RRMM and NDMM who were ineligible for ASCT in contrast to Len-Dex therapy (Dimopoulos et al., 2016). And after 44.3 months of follow-up, triple therapy significantly prolonged PFS in the treated population (44.5 vs. 17.5 months; HR, 0.44; 95% CI, 0.35–0.55; p < 0.0001). In terms of adverse profiles, a higher frequency of neutropenia and pneumonia was noticed in the daratumumab group (NCT02076009, NCT02252172) (Facon et al., 2019; Bahlis et al., 2020). Moreover, daratumumab plus Pom-Dex could also reduce the risk of disease progression and improve mPFS (12·4 vs. 6·9 months; HR, 0·63; 95% CI, 0·47–0.85) in patients with RRMM compared to Pom-Dex, and can be considered a new therapeutic strategy (NCT03180736) (Chari et al., 2017; Dimopoulos et al., 2021a). Besides, daratumumab applied with carfilzomib and Dex in patients with RRMM was associated with a promising benefit-risk profile and prolonging PFS (NCT03158688) (Dimopoulos et al., 2020b). In quadruple therapy, induction and remarkable consolidation treatment of daratumumab plus Len, BTZ, and Dex improved response depth and PFS with acceptable safety in patients with transplant-eligible NDMM (NCT02874742, NCT02541383) (Moreau et al., 2019; Voorhees et al., 2020). Isatuximab, another monoclonal antibody targeting CD38, has been evaluated as a monotherapy and combined with Dex in patients with RRMM. The combination of isatuximab and Dex promoted ORR (43.6 vs. 23.9% in the Isa arm) and survival outcomes with no adverse effect on safety (NCT01084252) (Dimopoulos et al., 2021b). On the basis of Pom-Dex, the addition of isatuximab was able to importantly improve PFS in patients with RRMM, particularly for patients who are refractory to Len and a PI (NCT02990338) (Attal et al., 2019).
In phase Ib-III clinical studies, elotuzumab, an immunostimulatory antibody against signaling lymphocytic activation molecule F7 (SLAMF7), achieved a reduction of 30% in the risk of disease progression or death in patients with RRMM combined with Len and Dex (NCT01239797) (Lonial et al., 2015; Dimopoulos et al., 2020c). Particularly among patients with MM who failed after treatment with Len and a PI, the addition of elotuzumab to Pom-Dex tremendously lowered the risk of disease progression or death and prolonged mPFS (10.3 vs. 4.7 months in the control group) versus Pom-Dex alone (NCT02654132) (Dimopoulos et al., 2018). Nevertheless, in the SWOG-1211 trial, elotuzumab combined with BTZ, Len, and Dex did not improve clinical outcomes of patients with NDMM as induction and maintenance treatments (Usmani et al., 2021) (NCT01668719).
4.6 Reversal of Drug Resistance
The majority of newly diagnosed patients respond to therapy of an immunomodulator, and yet most develop resistance to administered therapies eventually. Strategies to surmount resistance have been proposed (Mogollón et al., 2019). In MM, distinguishing mechanisms of resistance to both Len and Pom are apparent, therefore, tumors with acquired resistance to pomalidomide react to Len and vice versa (Ocio et al., 2015). STAT3 and MEK1/2 inhibitors have been testified to overcome IMiDs resistance effectively in pre-clinical studies (Ocio et al., 2015; Zhu et al., 2019). Enhancing degradation of CRBN has also been proved to be more sensitive to IMiDs (Kim et al., 2020). Protecting IKZFs from degradation through RUNXs inhibition resulted in conquering the resistance of IMiDs in MM (Zhou et al., 2019). In addition, a synthesized HDAC6 selective inhibitor, A452, is a strategy to overcome resistance to IMiDs (He et al., 2020). Furthermore, Cys reinforced the sensitivity of MDS/AML cells to Len without affecting the activation of T cells (He et al., 2020). It has been reported that the addition of cyclophosphamide to an IMiD could enhance the efficacy of IMiDs, prolong PFS and OS, and even save patients with Len-refractory disease, regardless of whether they have pre-accepted ASCT (NCT02244125) (Garderet et al., 2018). In patients with Len-refractory MM, the combination of pomalidomide, cyclophosphamide, and Dex confer a superior ORR and PFS than pomalidomide and Dex (NCT01432600) (Baz et al., 2016). In ALL, treatment with retinoid receptor agonists is able to fortify the sensitivity of IKZF1-aberrant BCR-ABL1 ALL patients to tyrosine kinase inhibitor therapy (Churchman et al., 2015).
5 Conclusion
Ikaros and its analogues play an important role in regulating normal lymphopoiesis, immune diseases, and generation of some tumors. In terms of categories, the Ikaros protein family consists of five members named Ikaros, Helios, Aiolos, Eos, and Pegasus. Ikaros acts both as a transcriptional repressor and as an activator by binding to assorted nuclear factors referred to epigenetic regulation and chromatin remodeling in different malignancies. And its own activities are thought to be regulated by post-translational phosphorylation, SUMOylation, and ubiquitination. Studies on signaling pathways about Ikaros have clarified that the preBCR signal pathway, Notch pathway, integrin signaling pathway, and IRFs are related to Ikaros signal transduction. For lymphocytes in healthy subjects, the Ikaros protein family exerts different effects on the growth, reproduction, and differentiation of many kinds of innate or adaptive lymphocytes in vivo and prevents autoimmunity by administering BCR unresponsiveness and repressing TLR signaling transduction.
Ikaros appears to function as a transcriptional inhibitor and activator by binding to different nuclear factors related to epigenetic regulation or targeting genes directly. The Ikaros-HDAC1 complex, inducing the formation of inhibitory chromatin, suppresses the transcription of its target genes accordingly. Ikaros has an adverse effect on the development of leukemia via globally regulating the enhancer or super-enhancer landscape and pioneer ring activity. Analogically, Ikaros is able to directly act on some target genes, such as those that activate the target genes’ transcription, cause transcription suppression, determine cell cycle progression, control cell survival, and develop drug resistance. Ikaros induces the powerful upregulation of G6PD, effecting malignancies through glucose metabolism.
IMiDs lead to selective ubiquitination and proteasomal degradation of Ikaros and Aiolos through recruiting TFs to the CRL4CRBN E3 ubiquitin ligase, achieving the purpose of therapy for MM and other malignancies. Novel thalidomide analogs, are currently in clinical trials as a monotherapy and in combination with other drugs, illustrating that CELMoDs have strong antitumor and immunostimulatory capabilities in patients with hematological malignancies. Even so, developing resistance to administered therapies is a huge obsession. Combination therapy and developing drugs against new targets may be potential treatments. Other treatments, such as protease kinase inhibitors, monoclonal antibodies, and phytochemicals were also applied to patients with IKZF-missing phenotypes, but the efficacy remains to be verified.
Author Contributions
YW and XW developed the main direction and offered significant guidance for this manuscript. RX and YC drafted the manuscript. XH illustrated the figures and tables for the manuscript. All authors approved the final manuscript.
Funding
This work is supported by the National Natural Science Foundation Regional Innovation and Development (No. U19A2003), the National Major Scientific and Technological Special Project for “Significant New Drugs Development” (No. 2018ZX09733001), the Excellent Youth Foundation of Sichuan Scientific Committee grant in China (No. 2019JDJQ008), and the Development Program of China (No. 2016YFA0201402).
Conflict of Interest
The authors declare that the research was conducted in the absence of any commercial or financial relationships that could be construed as a potential conflict of interest.
Publisher’s Note
All claims expressed in this article are solely those of the authors and do not necessarily represent those of their affiliated organizations, or those of the publisher, the editors and the reviewers. Any product that may be evaluated in this article, or claim that may be made by its manufacturer, is not guaranteed or endorsed by the publisher.
References
Alkhatib, A., Werner, M., Hug, E., Herzog, S., Eschbach, C., Faraidun, H., et al. (2012). FoxO1 Induces Ikaros Splicing to Promote Immunoglobulin Gene Recombination. J. Exp. Med. 209, 395–406. doi:10.1084/jem.20110216
Alomairi, J., Molitor, A. M., Sadouni, N., Hussain, S., Torres, M., Saadi, W., et al. (2020). Integration of High-Throughput Reporter Assays Identify a Critical Enhancer of the Ikzf1 Gene. PloS one 15, e0233191. doi:10.1371/journal.pone.0233191
Arco, P. G.-d., Maki, K., and Georgopoulos, K. (2004). Phosphorylation Controls Ikaros's Ability to Negatively Regulate the G 1 -S Transition. Mol. Cell Biol 24, 2797–2807. doi:10.1128/mcb.24.7.2797-2807.2004
Aref, S., Khaled, N., Menshawy, N. E., Sabry, M., and Agder, M. A. (2020). Clinical Value of RAG1 Expression and IKZF1 Deletions in Philadelphia Negative Pediatric B Cell Precursor Acute Lymphoblastic Leukemia. Pediatr. Hematol. Oncol. 37, 380–389. doi:10.1080/08880018.2020.1767739
Attal, M., Richardson, P. G., Rajkumar, S. V., San-Miguel, J., Beksac, M., Spicka, I., et al. (2019). Isatuximab Plus Pomalidomide and Low-Dose Dexamethasone versus Pomalidomide and Low-Dose Dexamethasone in Patients with Relapsed and Refractory Multiple Myeloma (ICARIA-MM): a Randomised, Multicentre, Open-Label, Phase 3 Study. Lancet 394, 2096–2107. doi:10.1016/S0140-6736(19)32556-5
Attal, M., Lauwers-Cances, V., Marit, G., Caillot, D., Moreau, P., Facon, T., et al. (2012). Lenalidomide Maintenance after Stem-Cell Transplantation for Multiple Myeloma. N. Engl. J. Med. 366, 1782–1791. doi:10.1056/nejmoa1114138
Avitahl, N., Winandy, S., Friedrich, C., Jones, B., Ge, Y., and Georgopoulos, K. (1999). Ikaros Sets Thresholds for T Cell Activation and Regulates Chromosome Propagation. Immunity 10, 333–343. doi:10.1016/s1074-7613(00)80033-3
Bahlis, N. J., Dimopoulos, M. A., White, D. J., Benboubker, L., Cook, G., Leiba, M., et al. (2020). Daratumumab Plus Lenalidomide and Dexamethasone in Relapsed/refractory Multiple Myeloma: Extended Follow-Up of POLLUX, a Randomized, Open-Label, Phase 3 Study. Leukemia 34, 1875–1884. doi:10.1038/s41375-020-0711-6
Bald, T., Wagner, M., Gao, Y., Koyasu, S., and Smyth, M. J. (2019). Hide and Seek: Plasticity of Innate Lymphoid Cells in Cancer. Semin. Immunol. 41, 101273. doi:10.1016/j.smim.2019.04.001
Barrett, J. C., Hansoul, S., Hansoul, S., Nicolae, D. L., Cho, J. H., Duerr, R. H., et al. (2008). Genome-wide Association Defines More Than 30 Distinct Susceptibility Loci for Crohn's Disease. Nat. Genet. 40, 955–962. doi:10.1038/ng.175
Baz, R. C., Martin, T. G., Lin, H.-Y., Zhao, X., Shain, K. H., Cho, H. J., et al. (2016). Randomized Multicenter Phase 2 Study of Pomalidomide, Cyclophosphamide, and Dexamethasone in Relapsed Refractory Myeloma. Blood 127, 2561–2568. doi:10.1182/blood-2015-11-682518
Beer, P. A., Knapp, D. J. H. F., Miller, P. H., Kannan, N., Sloma, I., Heel, K., et al. (2015). Disruption of IKAROS Activity in Primitive Chronic-phase CML Cells Mimics Myeloid Disease Progression. Blood 125, 504–515. doi:10.1182/blood-2014-06-581173
Bellavia, D., Mecarozzi, M., Campese, A. F., Grazioli, P., Talora, C., Frati, L., et al. (2007). Notch3 and the Notch3-Upregulated RNA-Binding Protein HuD Regulate Ikaros Alternative Splicing. Embo J. 26, 1670–1680. doi:10.1038/sj.emboj.7601626
Benboubker, L., Dimopoulos, M. A., Dispenzieri, A., Catalano, J., Belch, A. R., Cavo, M., et al. (2014). Lenalidomide and Dexamethasone in Transplant-Ineligible Patients with Myeloma. N. Engl. J. Med. 371, 906–917. doi:10.1056/nejmoa1402551
Berry, N. K., Scott, R. J., Sutton, R., Law, T., Trahair, T. N., Dalla-Pozza, L., et al. (2020). Enrichment of Atypical Hyperdiploidy and IKZF1 Deletions Detected by SNP-Microarray in High-Risk Australian AIEOP-BFM B-Cell Acute Lymphoblastic Leukaemia Cohort. Cancer Genet. 242, 8–14. doi:10.1016/j.cancergen.2020.01.051
Bjorklund, C. C., Lu, L., Kang, J., Hagner, P. R., Havens, C. G., Amatangelo, M., et al. (2015). Rate of CRL4CRBN Substrate Ikaros and Aiolos Degradation Underlies Differential Activity of Lenalidomide and Pomalidomide in Multiple Myeloma Cells by Regulation of C-Myc and IRF4. Blood Cancer J. 5, e354. doi:10.1038/bcj.2015.66
Borgo, C., D’Amore, C., Sarno, S., Salvi, M., and Ruzzene, M. (2021). Protein Kinase CK2: a Potential Therapeutic Target for Diverse Human Diseases. Sig Transduct Target. Ther. 6, 183. doi:10.1038/s41392-021-00567-7
Bottardi, S., Mavoungou, L., Pak, H., Daou, S., Bourgoin, V., Lakehal, Y. A., et al. (2014). The IKAROS Interaction with a Complex Including Chromatin Remodeling and Transcription Elongation Activities Is Required for Hematopoiesis. Plos Genet. 10, e1004827. doi:10.1371/journal.pgen.1004827
Brissot, E., Clavert, A., Blin, N., Roland, V., Guillaume, T., Dubruille, V., et al. (2015). Impact of Lenalidomide on Immune Functions in the Setting of Maintenance Therapy for Multiple Myeloma. Leukemia 29, 2098–2100. doi:10.1038/leu.2015.64
Cavo, M., Tacchetti, P., Patriarca, F., Petrucci, M. T., Pantani, L., Galli, M., et al. (2010). Bortezomib with Thalidomide Plus Dexamethasone Compared with Thalidomide Plus Dexamethasone as Induction Therapy before, and Consolidation Therapy after, Double Autologous Stem-Cell Transplantation in Newly Diagnosed Multiple Myeloma: a Randomised Phase 3 Study. The Lancet 376, 2075–2085. doi:10.1016/s0140-6736(10)61424-9
Chan, S. M. (2019). The Making of a Leukemic Stem Cell: A Novel Role for IKZF2 in AML Stemness and Differentiation. Cell Stem Cell 24, 5–6. doi:10.1016/j.stem.2018.12.007
Chari, A., Suvannasankha, A., Fay, J. W., Arnulf, B., Kaufman, J. L., Ifthikharuddin, J. J., et al. (2017). Daratumumab Plus Pomalidomide and Dexamethasone in Relapsed And/or Refractory Multiple Myeloma. Blood 130, 974–981. doi:10.1182/blood-2017-05-785246
Chen, J. C., Cerise, J. E., Jabbari, A., Clynes, R., and Christiano, A. M. (2015). Master Regulators of Infiltrate Recruitment in Autoimmune Disease Identified through Network-Based Molecular Deconvolution. Cell Syst. 1, 326–337. doi:10.1016/j.cels.2015.11.001
Chen, J. C., Perez-Lorenzo, R., Saenger, Y. M., Drake, C. G., and Christiano, A. M. (2018). IKZF1 Enhances Immune Infiltrate Recruitment in Solid Tumors and Susceptibility to Immunotherapy. Cell Syst. 7, 92–103. doi:10.1016/j.cels.2018.05.020
Chen, L., Niu, Q., Huang, Z., Yang, B., Wu, Y., and Zhang, J. (2020). IKZF1 Polymorphisms Are Associated with Susceptibility, Cytokine Levels, and Clinical Features in Systemic Lupus Erythematosus. Medicine 99, e22607. doi:10.1097/md.0000000000022607
Chen, Q., Shi, Y., Chen, Y., Ji, T., Li, Y., and Yu, L. (2019). Multiple Functions of Ikaros in Hematological Malignancies, Solid Tumor and Autoimmune Diseases. Gene 684, 47–52. doi:10.1016/j.gene.2018.10.045
Churchman, M. L., Low, J., Qu, C., Paietta, E. M., Kasper, L. H., Chang, Y., et al. (2015). Efficacy of Retinoids in IKZF1-Mutated BCR-ABL1 Acute Lymphoblastic Leukemia. Cancer cell 28, 343–356. doi:10.1016/j.ccell.2015.07.016
Churchman, M. L., Qian, M., Te Kronnie, G., Zhang, R., Yang, W., Zhang, H., et al. (2018). Germline Genetic IKZF1 Variation and Predisposition to Childhood Acute Lymphoblastic Leukemia. Cancer Cell 33, 937–948. e8. doi:10.1016/j.ccell.2018.03.021
Cippitelli, M., Stabile, H., Kosta, A., Petillo, S., Gismondi, A., Santoni, A., et al. (2021). Role of Aiolos and Ikaros in the Antitumor and Immunomodulatory Activity of IMiDs in Multiple Myeloma: Better to Lose Than to Find Them. Int. J. Mol. Sci. 22. doi:10.3390/ijms22031103
Costa, F., Vescovini, R., Bolzoni, M., Marchica, V., Storti, P., Toscani, D., et al. (2017). Lenalidomide Increases Human Dendritic Cell Maturation in Multiple Myeloma Patients Targeting Monocyte Differentiation and Modulating Mesenchymal Stromal Cell Inhibitory Properties. Oncotarget 8, 53053–53067. doi:10.18632/oncotarget.18085
Craddock, C., Slade, D., De Santo, C., Wheat, R., Ferguson, P., Hodgkinson, A., et al. (2019). Combination Lenalidomide and Azacitidine: A Novel Salvage Therapy in Patients Who Relapse after Allogeneic Stem-Cell Transplantation for Acute Myeloid Leukemia. Jco 37, 580–588. doi:10.1200/jco.18.00889
Cunninghame Graham, D. S., Morris, D. L., Bhangale, T. R., Criswell, L. A., Syvänen, A.-C., Rönnblom, L., et al. (2011). Association of NCF2, IKZF1, IRF8, IFIH1, and TYK2 with Systemic Lupus Erythematosus. Plos Genet. 7, e1002341. doi:10.1371/journal.pgen.1002341
Curtis, L. M., Ostojic, A., Venzon, D. J., Holtzman, N. G., Pirsl, F., Kuzmina, Z. J., et al. (2021). A Randomized Phase 2 Trial of Pomalidomide in Subjects Failing Prior Therapy for Chronic Graft-Versus-Host Disease. Blood 137, 896–907. doi:10.1182/blood.2020006892
Daneshvar Kakhaki, R., Kouchaki, E., Dadgostar, E., Behnam, M., Tamtaji, O. R., Nikoueinejad, H., et al. (2020). The Correlation of Helios and Neuropilin-1 Frequencies with Parkinson Disease Severity. Clin. Neurol. Neurosurg. 192, 105833. doi:10.1016/j.clineuro.2020.105833
Davidson, A., and Diamond, B. (2001). Autoimmune Diseases. N. Engl. J. Med. 345, 340–350. doi:10.1056/nejm200108023450506
Davis, D. A., Shrestha, P., Aisabor, A. I., Stream, A., Galli, V., Pise-Masison, C. A., et al. (2019). Pomalidomide Increases Immune Surface Marker Expression and Immune Recognition of Oncovirus-Infected Cells. Oncoimmunology 8, e1546544. doi:10.1080/2162402x.2018.1546544
de Rooij, J. D. E., Beuling, E., van den Heuvel-Eibrink, M. M., Obulkasim, A., Baruchel, A., Trka, J., et al. (2015). Recurrent Deletions of IKZF1 in Pediatric Acute Myeloid Leukemia. Haematologica 100, 1151–1159. doi:10.3324/haematol.2015.124321
Dhanyamraju, P. K., Iyer, S., Smink, G., Bamme, Y., Bhadauria, P., Payne, J. L., et al. (2020). Transcriptional Regulation of Genes by Ikaros Tumor Suppressor in Acute Lymphoblastic Leukemia. Int. J. Mol. Sci. 21. doi:10.3390/ijms21041377
Dieudonné, Y., Guffroy, A., Vollmer, O., Carapito, R., and Korganow, A.-S. (2019). IKZF1 Loss-Of-Function Variant Causes Autoimmunity and Severe Familial Antiphospholipid Syndrome. J. Clin. Immunol. 39, 353–357. doi:10.1007/s10875-019-00643-2
Dimopoulos, K., Fibiger Munch-Petersen, H., Winther Eskelund, C., Dissing Sjö, L., Ralfkiaer, E., Gimsing, P., et al. (2019). Expression of CRBN, IKZF1, and IKZF3 Does Not Predict Lenalidomide Sensitivity and Mutations in the Cereblon Pathway Are Infrequent in Multiple Myeloma. Leuk. Lymphoma 60, 180–188. doi:10.1080/10428194.2018.1466290
Dimopoulos, M. A., Dytfeld, D., Grosicki, S., Moreau, P., Takezako, N., Hori, M., et al. (2018). Elotuzumab Plus Pomalidomide and Dexamethasone for Multiple Myeloma. N. Engl. J. Med. 379, 1811–1822. doi:10.1056/nejmoa1805762
Dimopoulos, M. A., Lonial, S., White, D., Moreau, P., Weisel, K., San-Miguel, J., et al. (2020). Elotuzumab, Lenalidomide, and Dexamethasone in RRMM: Final Overall Survival Results from the Phase 3 Randomized ELOQUENT-2 Study. Blood Cancer J. 10, 91. doi:10.1038/s41408-020-00357-4
Dimopoulos, M. A., Oriol, A., Nahi, H., San-Miguel, J., Bahlis, N. J., Usmani, S. Z., et al. (2016). Daratumumab, Lenalidomide, and Dexamethasone for Multiple Myeloma. N. Engl. J. Med. 375, 1319–1331. doi:10.1056/nejmoa1607751
Dimopoulos, M. A., Špička, I., Quach, H., Oriol, A., Hájek, R., Garg, M., et al. (2020). Ixazomib as Postinduction Maintenance for Patients with Newly Diagnosed Multiple Myeloma Not Undergoing Autologous Stem Cell Transplantation: The Phase III TOURMALINE-MM4 Trial. Jco 38, 4030–4041. doi:10.1200/jco.20.02060
Dimopoulos, M. A., Terpos, E., Boccadoro, M., Delimpasi, S., Beksac, M., Katodritou, E., et al. (2021). Daratumumab Plus Pomalidomide and Dexamethasone versus Pomalidomide and Dexamethasone Alone in Previously Treated Multiple Myeloma (APOLLO): an Open-Label, Randomised, Phase 3 Trial. Lancet Oncol. 22, 801–812. doi:10.1016/s1470-2045(21)00128-5
Dimopoulos, M., Bringhen, S., Anttila, P., Capra, M., Cavo, M., Cole, C., et al. (2021). Isatuximab as Monotherapy and Combined with Dexamethasone in Patients with Relapsed/refractory Multiple Myeloma. Blood 137, 1154–1165. doi:10.1182/blood.2020008209
Dimopoulos, M., Quach, H., Mateos, M.-V., Landgren, O., Leleu, X., Siegel, D., et al. (2020). Carfilzomib, Dexamethasone, and Daratumumab versus Carfilzomib and Dexamethasone for Patients with Relapsed or Refractory Multiple Myeloma (CANDOR): Results from a Randomised, Multicentre, Open-Label, Phase 3 Study. The Lancet 396, 186–197. doi:10.1016/s0140-6736(20)30734-0
Ding, Y., Zhang, B., Payne, J. L., Song, C., Ge, Z., Gowda, C., et al. (2019). Ikaros Tumor Suppressor Function Includes Induction of Active Enhancers and Super-enhancers along with Pioneering Activity. Leukemia 33, 2720–2731. doi:10.1038/s41375-019-0474-0
Dobrovolsky, D., Wang, E. S., Morrow, S., Leahy, C., Faust, T., Nowak, R. P., et al. (2019). Bruton Tyrosine Kinase Degradation as a Therapeutic Strategy for Cancer. Blood 133, 952–961. doi:10.1182/blood-2018-07-862953
Dou, A., and Fang, J. (2020). Cyclosporine Broadens the Therapeutic Potential of Lenalidomide in Myeloid Malignancies. J. Cell Immunol 2, 237–244. doi:10.33696/immunology.2.049
Dovat, S., Song, C., Payne, K. J., and Li, Z. (2011). Ikaros, CK2 Kinase, and the Road to Leukemia. Mol. Cell Biochem 356, 201–207. doi:10.1007/s11010-011-0964-5
Duan, Y., Guan, Y., Qin, W., Zhai, X., Yu, B., and Liu, H. (2018). Targeting Brd4 for Cancer Therapy: Inhibitors and Degraders. Med. Chem. Commun. 9, 1779–1802. doi:10.1039/c8md00198g
Duhamel, M., Arrouss, I., Merle-Béral, H., and Rebollo, A. (2008). The Aiolos Transcription Factor Is Up-Regulated in Chronic Lymphocytic Leukemia. Blood 111, 3225–3228. doi:10.1182/blood-2007-09-113191
Dumortier, A., Jeannet, R., Kirstetter, P., Kleinmann, E., Sellars, M., dos Santos, N. R., et al. (2006). Notch Activation Is an Early and Critical Event during T-Cell Leukemogenesis in Ikaros-Deficient Mice. Mol. Cell Biol 26, 209–220. doi:10.1128/mcb.26.1.209-220.2006
Dumortier, A., Kirstetter, P., Kastner, P., and Chan, S. (2003). Ikaros Regulates Neutrophil Differentiation. Blood 101, 2219–2226. doi:10.1182/blood-2002-05-1336
Durie, B. G. M., Hoering, A., Abidi, M. H., Rajkumar, S. V., Epstein, J., Kahanic, S. P., et al. (2017). Bortezomib with Lenalidomide and Dexamethasone versus Lenalidomide and Dexamethasone Alone in Patients with Newly Diagnosed Myeloma without Intent for Immediate Autologous Stem-Cell Transplant (SWOG S0777): a Randomised, Open-Label, Phase 3 Trial. The Lancet 389, 519–527. doi:10.1016/s0140-6736(16)31594-x
Durie, B. G. M., Hoering, A., Sexton, R., Abidi, M. H., Epstein, J., Rajkumar, S. V., et al. (2020). Longer Term Follow-Up of the Randomized Phase III Trial SWOG S0777: Bortezomib, Lenalidomide and Dexamethasone vs. Lenalidomide and Dexamethasone in Patients (Pts) with Previously Untreated Multiple Myeloma without an Intent for Immediate Autologous Stem Cell Transplant (ASCT). Blood Cancer J. 10, 53. doi:10.1038/s41408-020-0311-8
Edgren, H., Murumagi, A., Kangaspeska, S., Nicorici, D., Hongisto, V., Kleivi, K., et al. (2011). Identification of Fusion Genes in Breast Cancer by Paired-End RNA-Sequencing. Genome Biol. 12, R6. doi:10.1186/gb-2011-12-1-r6
Eskandarian, Z., Fliegauf, M., Bulashevska, A., Proietti, M., Hague, R., Smulski, C. R., et al. (2019). Assessing the Functional Relevance of Variants in the IKAROS Family Zinc Finger Protein 1 (IKZF1) in a Cohort of Patients with Primary Immunodeficiency. Front. Immunol. 10, 568. doi:10.3389/fimmu.2019.00568
Facon, T., Kumar, S., Plesner, T., Orlowski, R. Z., Moreau, P., Bahlis, N., et al. (2019). Daratumumab Plus Lenalidomide and Dexamethasone for Untreated Myeloma. N. Engl. J. Med. 380, 2104–2115. doi:10.1056/nejmoa1817249
Fan, Y., and Lu, D. (2016). The Ikaros Family of Zinc-finger Proteins. Acta Pharmaceutica Sinica. B 6, 513–521. doi:10.1016/j.apsb.2016.06.002
Fang, C.-M., Roy, S., Nielsen, E., Paul, M., Maul, R., Paun, A., et al. (2012). Unique Contribution of IRF-5-Ikaros axis to the B-Cell IgG2a Response. Genes Immun. 13, 421–430. doi:10.1038/gene.2012.10
Fedele, P. L., Willis, S. N., Liao, Y., Low, M. S., Rautela, J., Segal, D. H., et al. (2018). IMiDs Prime Myeloma Cells for Daratumumab-Mediated Cytotoxicity through Loss of Ikaros and Aiolos. Blood 132, 2166–2178. doi:10.1182/blood-2018-05-850727
Fenaux, P., Giagounidis, A., Selleslag, D., Beyne-Rauzy, O., Mufti, G., Mittelman, M., et al. (2011). A randomized phase 3 study of lenalidomide versus placebo in RBC transfusion-dependent patients with Low-/Intermediate-1-risk myelodysplastic syndromes with del5q. Blood 118, 3765–3776. doi:10.1182/blood-2011-01-330126
Fionda, C., Abruzzese, M. P., Zingoni, A., Cecere, F., Vulpis, E., Peruzzi, G., et al. (2015). The IMiDs Targets IKZF-1/3 and IRF4 as Novel Negative Regulators of NK Cell-Activating Ligands Expression in Multiple Myeloma. Oncotarget 6, 23609–23630. doi:10.18632/oncotarget.4603
Fiorcari, S., Benatti, S., Zucchetto, A., Zucchini, P., Gattei, V., Luppi, M., et al. (2019). Overexpression of CD49d in Trisomy 12 Chronic Lymphocytic Leukemia Patients Is Mediated by IRF4 through Induction of IKAROS. Leukemia 33, 1278–1302. doi:10.1038/s41375-018-0296-5
Flowers, C. R., Leonard, J. P., and Fowler, N. H. (2020). Lenalidomide in Follicular Lymphoma. Blood 135, 2133–2136. doi:10.1182/blood.2019001751
Franks, M. E., Macpherson, G. R., and Figg, W. D. (2004). Thalidomide. The Lancet 363, 1802–1811. doi:10.1016/s0140-6736(04)16308-3
Fricker, L. D. (2020). Proteasome Inhibitor Drugs. Annu. Rev. Pharmacol. Toxicol. 60, 457–476. doi:10.1146/annurev-pharmtox-010919-023603
Gandhi, A. K., Kang, J., Havens, C. G., Conklin, T., Ning, Y., Wu, L., et al. (2014). Immunomodulatory Agents Lenalidomide and Pomalidomide Co‐stimulate T Cells by Inducing Degradation of T Cell Repressors I Karos and A Iolos via Modulation of the E 3 Ubiquitin Ligase Complex CRL 4 CRBN. Br. J. Haematol. 164, 811–821. doi:10.1111/bjh.12708
Ganesan, S., Palani, H. K., Balasundaram, N., David, S., Devasia, A. J., George, B., et al. (2020). Combination Lenalidomide/Bortezomib Treatment Synergistically Induces Calpain-dependent Ikaros Cleavage and Apoptosis in Myeloma Cells. Mol. Cancer Res. 18, 529–536. doi:10.1158/1541-7786.mcr-19-0431
Gao, S., Wang, S., and Song, Y. (2020). Novel Immunomodulatory Drugs and Neo-Substrates. Biomark Res. 8, 2. doi:10.1186/s40364-020-0182-y
Garderet, L., Kuhnowski, F., Berge, B., Roussel, M., Escoffre-Barbe, M., Lafon, I., et al. (2018). Pomalidomide, Cyclophosphamide, and Dexamethasone for Relapsed Multiple Myeloma. Blood 132, 2555–2563. doi:10.1182/blood-2018-07-863829
Ge, Z., Gu, Y., Han, Q., Sloane, J., Ge, Q., Gao, G., et al. (2018). Plant Homeodomain finger Protein 2 as a Novel IKAROS Target in Acute Lymphoblastic Leukemia. Epigenomics 10, 59–69. doi:10.2217/epi-2017-0092
Ge, Z., Gu, Y., Han, Q., Zhao, G., Li, M., Li, J., et al. (2016). Targeting High Dynamin-2 (DNM2) Expression by Restoring Ikaros Function in Acute Lymphoblastic Leukemia.. Sci Rep. 6, 38004. doi:10.1038/srep38004
Ge, Z., Gu, Y., Xiao, L., Han, Q., Li, J., Chen, B., et al. (2016). Co-existence of IL7R High and SH2B3 Low Expression Distinguishes a Novel High-Risk Acute Lymphoblastic Leukemia with Ikaros Dysfunction. Oncotarget 7, 46014–46027. doi:10.18632/oncotarget.10014
Ge, Z., Gu, Y., Zhao, G., Li, J., Chen, B., Han, Q., et al. (2016). High CRLF2 Expression Associates with IKZF1 Dysfunction in Adult Acute Lymphoblastic Leukemia without CRLF2 Rearrangement. Oncotarget 7, 49722–49732. doi:10.18632/oncotarget.10437
Ge, Z., Guo, X., Li, J., Hartman, M., Kawasawa, Y. I., Dovat, S., et al. (2015). Clinical Significance of High C-MYC and Low MYCBP2 Expression and Their Association with Ikaros Dysfunction in Adult Acute Lymphoblastic Leukemia. Oncotarget 6, 42300–42311. doi:10.18632/oncotarget.5982
Ge, Z., Han, Q., Gu, Y., Ge, Q., Ma, J., Sloane, J., et al. (2018). Aberrant ARID5B Expression and its Association with Ikaros Dysfunction in Acute Lymphoblastic Leukemia. Oncogenesis 7, 84. doi:10.1038/s41389-018-0095-x
Ge, Z., Zhou, X., Gu, Y., Han, Q., Li, J., Chen, B., et al. (2017). Ikaros Regulation of the BCL6/BACH2 axis and its Clinical Relevance in Acute Lymphoblastic Leukemia. Oncotarget 8, 8022–8034. doi:10.18632/oncotarget.14038
Georgopoulos, K. (2002). Haematopoietic Cell-Fate Decisions, Chromatin Regulation and Ikaros. Nat. Rev. Immunol. 2, 162–174. doi:10.1038/nri747
Georgopoulos, K., Winandy, S., and Avitahl, N. (1997). The Role of the Ikaros Gene in Lymphocyte Development and Homeostasis. Annu. Rev. Immunol. 15, 155–176. doi:10.1146/annurev.immunol.15.1.155
Gokhale, A. S., Gangaplara, A., Lopez-Occasio, M., Thornton, A. M., and Shevach, E. M. (2019). Selective Deletion of Eos (Ikzf4) in T-Regulatory Cells Leads to Loss of Suppressive Function and Development of Systemic Autoimmunity. J. Autoimmun. 105, 102300. doi:10.1016/j.jaut.2019.06.011
Goldman, F. D., Gurel, Z., Al-Zubeidi, D., Fried, A. J., Icardi, M., Song, C., et al. (2012). Congenital Pancytopenia and Absence of B Lymphocytes in a Neonate with a Mutation in the Ikaros Gene. Pediatr. Blood Cancer 58, 591–597. doi:10.1002/pbc.23160
Gómez-del Arco, P., Koipally, J., and Georgopoulos, K. (2005). Ikaros SUMOylation: Switching Out of Repression. Mol. Cell Biol 25, 2688–2697. doi:10.1128/MCB.25.7.2688-2697.2005
Gorlova, O., Martin, J.-E., Rueda, B., Koeleman, B. P. C., Ying, J., Teruel, M., et al. (2011). Identification of Novel Genetic Markers Associated with Clinical Phenotypes of Systemic Sclerosis through a Genome-wide Association Strategy. Plos Genet. 7, e1002178. doi:10.1371/journal.pgen.1002178
Gowda, C., Sachdev, M., Muthusami, S., Kapadia, M., Petrovic-Dovat, L., Hartman, M., et al. (2017). Casein Kinase II (CK2) as a Therapeutic Target for Hematological Malignancies. Curr. Pharm. Des. 23, 95–107. doi:10.2174/1381612822666161006154311
Gowda, C., Soliman, M., Kapadia, M., Ding, Y., Payne, K., and Dovat, S. (2017). Casein Kinase II (CK2), Glycogen Synthase Kinase-3 (GSK-3) and Ikaros Mediated Regulation of Leukemia. Adv. Biol. Regul. 65, 16–25. doi:10.1016/j.jbior.2017.06.001
Gowda, C., Song, C., Ding, Y., Iyer, S., Dhanyamraju, P. K., McGrath, M., et al. (2020). Cellular Signaling and Epigenetic Regulation of Gene Expression in Leukemia. Adv. Biol. Regul. 75, 100665. doi:10.1016/j.jbior.2019.100665
Granados-Zamora, M., Chaves-Herrera, K., Morera-Araya, E., Granados-Alfaro, P., Valverde-Muñoz, K., Soto-Herrera, G., et al. (2020). IKZF1 Deletions as a Prognostic Factor in Costa Rican Patients with Pediatric B-Cell Acute Lymphoblastic Leukemia. J. Pediatr. hematology/oncology 42, e401–e406. doi:10.1097/mph.0000000000001807
Groth, D. J., Lakkaraja, M. M., Ferreira, J. O., Feuille, E. J., Bassetti, J. A., and Kaicker, S. M. (2020). Management of Chronic Immune Thrombocytopenia and Presumed Autoimmune Hepatitis in a Child with IKAROS Haploinsufficiency. J. Clin. Immunol. 40, 653–657. doi:10.1007/s10875-020-00781-y
Guha, I., Bhuniya, A., Shukla, D., Patidar, A., Nandi, P., Saha, A., et al. (2020). Tumor Arrests DN2 to DN3 Pro T Cell Transition and Promotes its Conversion to Thymic Dendritic Cells by Reciprocally Regulating Notch1 and Ikaros Signaling. Front. Immunol. 11, 898. doi:10.3389/fimmu.2020.00898
Gurel, Z., Ronni, T., Ho, S., Kuchar, J., Payne, K. J., Turk, C. W., et al. (2008). Recruitment of Ikaros to Pericentromeric Heterochromatin Is Regulated by Phosphorylation. J. Biol. Chem. 283, 8291–8300. doi:10.1074/jbc.m707906200
Hagner, P. R., Man, H.-W., Fontanillo, C., Wang, M., Couto, S., Breider, M., et al. (2015). CC-122, a Pleiotropic Pathway Modifier, Mimics an Interferon Response and Has Antitumor Activity in DLBCL. Blood 126, 779–789. doi:10.1182/blood-2015-02-628669
Hahm, K., Ernst, P., Lo, K., Kim, G. S., Turck, C., and Smale, S. T. (1994). The Lymphoid Transcription Factor LyF-1 Is Encoded by Specific, Alternatively Spliced mRNAs Derived from the Ikaros Gene. Mol. Cel. Biol. 14, 7111–7123. doi:10.1128/mcb.14.11.7111
Hansen, J. D., Correa, M., Nagy, M. A., Alexander, M., Plantevin, V., Grant, V., et al. (2020). Discovery of CRBN E3 Ligase Modulator CC-92480 for the Treatment of Relapsed and Refractory Multiple Myeloma. J. Med. Chem. 63, 6648–6676. doi:10.1021/acs.jmedchem.9b01928
Hariri, L., and Hardin, C. C. (2020). Covid-19, Angiogenesis, and ARDS Endotypes. N. Engl. J. Med. 383, 182–183. doi:10.1056/nejme2018629
He, L.-C., Gao, F.-H., Xu, H.-Z., Zhao, S., Ma, C.-M., Li, J., et al. (2012). Ikaros Inhibits Proliferation and, through Upregulation of Slug, Increases Metastatic Ability of Ovarian Serous Adenocarcinoma Cells. Oncol. Rep. 28, 1399–1405. doi:10.3892/or.2012.1946
He, X., Dou, A., Feng, S., Roman-Rivera, A., Hawkins, C., Lawley, L., et al. (2020). Cyclosporine Enhances the Sensitivity to Lenalidomide in MDS/AML In Vitro. Exp. Hematol. 86, 21–27. doi:10.1016/j.exphem.2020.05.001
Heizmann, B., Kastner, P., and Chan, S. (2018). The Ikaros Family in Lymphocyte Development. Curr. Opin. Immunol. 51, 14–23. doi:10.1016/j.coi.2017.11.005
Hgnc, (2021). The Resource for Approved Human Gene Nomenclature. www.genenames.org.
Hideshima, T., Ogiya, D., Liu, J., Harada, T., Kurata, K., Bae, J., et al. (2021). Immunomodulatory Drugs Activate NK Cells via Both Zap-70 and Cereblon-dependent Pathways. Leukemia 35, 177–188. doi:10.1038/s41375-020-0809-x
Hosokawa, Y., Maeda, Y., Takahashi, E.-i., Suzuki, M., and Seto, M. (1999). Human Aiolos, an Ikaros-Related Zinc finger DNA Binding Protein: cDNA Cloning, Tissue Expression Pattern, and Chromosomal Mapping. Genomics 61, 326–329. doi:10.1006/geno.1999.5949
Hu, Y., Zhang, L., Chen, H., Liu, X., Zheng, X., Shi, H., et al. (2019). Analysis of Regulatory T Cell Subsets and Their Expression of Helios and PD-1 in Patients with Hashimoto Thyroiditis. Int. J. Endocrinol. 2019, 5368473. doi:10.1155/2019/5368473
Huang, Y., Lu, Y., He, Y., Feng, Z., Zhan, Y., Huang, X., et al. (2019). Ikzf1 Regulates Embryonic T Lymphopoiesis via Ccr9 and Irf4 in Zebrafish. J. Biol. Chem. 294, 16152–16163. doi:10.1074/jbc.ra119.009883
Hung, J.-J., Kao, Y.-S., Huang, C.-H., and Hsu, W.-H. (2019). Overexpression of Aiolos Promotes Epithelial-Mesenchymal Transition and Cancer Stem Cell-like Properties in Lung Cancer Cells. Sci. Rep. 9, 2991. doi:10.1038/s41598-019-39545-z
Hung, K.-H., Su, S.-T., Chen, C.-Y., Hsu, P.-H., Huang, S.-Y., Wu, W.-J., et al. (2016). Aiolos Collaborates with Blimp-1 to Regulate the Survival of Multiple Myeloma Cells. Cell Death Differ 23, 1175–1184. doi:10.1038/cdd.2015.167
Igartua, C., Myers, R. A., Mathias, R. A., Pino-Yanes, M., Eng, C., Graves, P. E., et al. (2015). Ethnic-specific Associations of Rare and Low-Frequency DNA Sequence Variants with Asthma. Nat. Commun. 6, 5965. doi:10.1038/ncomms6965
Ihara, M., Koyama, H., Uchimura, Y., Saitoh, H., and Kikuchi, A. (2007). Noncovalent Binding of Small Ubiquitin-Related Modifier (SUMO) Protease to SUMO Is Necessary for Enzymatic Activities and Cell Growth. J. Biol. Chem. 282, 16465–16475. doi:10.1074/jbc.m610723200
Ito, T., and Handa, H. (2019). [Cereblon as a Primary Target of IMiDs]. Rinsho Ketsueki 60, 1013–1019. doi:10.11406/rinketsu.60.1013
Jackson, G. H., Davies, F. E., Pawlyn, C., Cairns, D. A., Striha, A., Collett, C., et al. (2019). Lenalidomide Maintenance versus Observation for Patients with Newly Diagnosed Multiple Myeloma (Myeloma XI): a Multicentre, Open-Label, Randomised, Phase 3 Trial. Lancet Oncol. 20, 57–73. doi:10.1016/s1470-2045(18)30687-9
Javierre, B. M., Rodriguez-Ubreva, J., Al-Shahrour, F., Corominas, M., Graña, O., Ciudad, L., et al. (2011). Long-range Epigenetic Silencing Associates with Deregulation of Ikaros Targets in Colorectal Cancer Cells. Mol. Cancer Res. 9, 1139–1151. doi:10.1158/1541-7786.mcr-10-0515
Jeng, M. Y., Mumbach, M. R., Granja, J. M., Satpathy, A. T., Chang, H. Y., and Chang, A. L. S. (2019). Enhancer Connectome Nominates Target Genes of Inherited Risk Variants from Inflammatory Skin Disorders. J. Invest. Dermatol. 139, 605–614. doi:10.1016/j.jid.2018.09.011
Joshi, I., Yoshida, T., Jena, N., Qi, X., Zhang, J., Van Etten, R. A., et al. (2014). Loss of Ikaros DNA-Binding Function Confers Integrin-dependent Survival on Pre-B Cells and Progression to Acute Lymphoblastic Leukemia. Nat. Immunol. 15, 294–304. doi:10.1038/ni.2821
Jovanović, K. K., Roche-Lestienne, C., Ghobrial, I. M., Facon, T., Quesnel, B., and Manier, S. (2018). Targeting MYC in Multiple Myeloma. Leukemia 32, 1295–1306. doi:10.1038/s41375-018-0036-x
Katerndahl, C. D. S., Heltemes-Harris, L. M., Willette, M. J. L., Henzler, C. M., Frietze, S., Yang, R., et al. (2017). Antagonism of B Cell Enhancer Networks by STAT5 Drives Leukemia and Poor Patient Survival. Nat. Immunol. 18, 694–704. doi:10.1038/ni.3716
Khamechian, T., Irandoust, B., Mohammadi, H., Nikoueinejad, H., and Akbari, H. (2018). Association of Regulatory T Cells with Diabetes Type-1 and its Renal and Vascular Complications Based on the Expression of Forkhead Box Protein P3 (FoxP3), Helios and Neurophilin-1. Iran J. Allergy Asthma Immunol. 17, 151–157.
Kikuchi, J., Hori, M., Iha, H., Toyama-Sorimachi, N., Hagiwara, S., Kuroda, Y., et al. (2020). Soluble SLAMF7 Promotes the Growth of Myeloma Cells via Homophilic Interaction with Surface SLAMF7. Leukemia 34, 180–195. doi:10.1038/s41375-019-0525-6
Kim, G. W., Yoo, J., Won, H.-R., Yeon, S.-K., Lee, S. W., Lee, D. H., et al. (2020). A452, HDAC6-Selective Inhibitor Synergistically Enhances the Anticancer Activity of Immunomodulatory Drugs in IMiDs-Resistant Multiple Myeloma. Leuk. Res. 95, 106398. doi:10.1016/j.leukres.2020.106398
Kim, H.-J., Barnitz, R. A., Kreslavsky, T., Brown, F. D., Moffett, H., Lemieux, M. E., et al. (2015). Stable Inhibitory Activity of Regulatory T Cells Requires the Transcription Factor Helios. Science 350, 334–339. doi:10.1126/science.aad0616
Klocperk, A., Grecová, J., Šišmová, K., Kayserová, J., Froňková, E., and Šedivá, A. (2014). Helios expression in T-regulatory cells in patients with di George Syndrome. J. Clin. Immunol. 34, 864–870. doi:10.1007/s10875-014-0071-y
Klumb, C. E., Barbosa, T. d. C., Nestal de Moraes, G., Schramm, M. T., Emerenciano, M., and Maia, R. C. (2019). IKZF1 Deletion and Co-occurrence with Other Aberrations in a Child with Chronic Myeloid Leukemia Progressing to Acute Lymphoblastic Leukemia. Pediatr. Blood Cancer 66, e27570. doi:10.1002/pbc.27570
Koipally, J., Renold, A., Kim, J., and Georgopoulos, K. (1999). Repression by Ikaros and Aiolos Is Mediated through Histone Deacetylase Complexes. EMBO J. 18, 3090–3100. doi:10.1093/emboj/18.11.3090
Krabbendam, L., Bernink, J. H., and Spits, H. (2021). Innate Lymphoid Cells: from Helper to Killer. Curr. Opin. Immunol. 68, 28–33. doi:10.1016/j.coi.2020.08.007
Kriegsmann, K., Baertsch, M.-A., Awwad, M. H. S., Merz, M., Hose, D., Seckinger, A., et al. (2019). Cereblon-binding Proteins Expression Levels Correlate with Hyperdiploidy in Newly Diagnosed Multiple Myeloma Patients. Blood Cancer J. 9, 13. doi:10.1038/s41408-019-0174-z
Krönke, J., Fink, E. C., Hollenbach, P. W., MacBeth, K. J., Hurst, S. N., Udeshi, N. D., et al. (2015). Lenalidomide induces ubiquitination and degradation of CK1α in del(5q) MDS. Nature 523, 183–188. doi:10.1038/nature14610
Kuehn, H. S., Niemela, J. E., Stoddard, J., Ciullini Mannurita, S., Shahin, T., Goel, S., et al. (2020). Germline IKAROS Dimerization Haploinsufficiency Causes Hematologic Cytopenias and Malignancies. Blood 137 (3), 349–363. doi:10.1182/blood.2020007292
Kuiper, R. P., Waanders, E., van der Velden, V. H. J., van Reijmersdal, S. V., Venkatachalam, R., Scheijen, B., et al. (2010). IKZF1 Deletions Predict Relapse in Uniformly Treated Pediatric Precursor B-ALL. Leukemia 24, 1258–1264. doi:10.1038/leu.2010.87
Kumar, S. K., Jacobus, S. J., Cohen, A. D., Weiss, M., Callander, N., Singh, A. K., et al. (2020). Carfilzomib or Bortezomib in Combination with Lenalidomide and Dexamethasone for Patients with Newly Diagnosed Multiple Myeloma without Intention for Immediate Autologous Stem-Cell Transplantation (ENDURANCE): a Multicentre, Open-Label, Phase 3, Randomised, Controlled Trial. Lancet Oncol. 21, 1317–1330. doi:10.1016/s1470-2045(20)30452-6
Ladetto, M., Cortelazzo, S., Ferrero, S., Evangelista, A., Mian, M., Tavarozzi, R., et al. (2021). Lenalidomide Maintenance after Autologous Haematopoietic Stem-Cell Transplantation in Mantle Cell Lymphoma: Results of a Fondazione Italiana Linfomi (FIL) Multicentre, Randomised, Phase 3 Trial. Lancet Haematol. 8, e34–e44. doi:10.1016/s2352-3026(20)30358-6
Lagrue, K., Carisey, A., Morgan, D. J., Chopra, R., and Davis, D. M. (2015). Lenalidomide Augments Actin Remodeling and Lowers NK-Cell Activation Thresholds. Blood 126, 50–60. doi:10.1182/blood-2015-01-625004
Le Roy, A., Prébet, T., Castellano, R., Goubard, A., Riccardi, F., Fauriat, C., et al. (2018). Immunomodulatory Drugs Exert Anti-leukemia Effects in Acute Myeloid Leukemia by Direct and Immunostimulatory Activities. Front. Immunol. 9, 977. doi:10.3389/fimmu.2018.00977
Lempainen, J., Härkönen, T., Laine, A., Knip, M., and Ilonen, J. (2013). Associations of Polymorphisms in Non-HLA Loci with Autoantibodies at the Diagnosis of Type 1 diabetes:INSandIKZF4associate with Insulin Autoantibodies. Pediatr. Diabetes 14, 490–496. doi:10.1111/pedi.12046
Lentaigne, C., Greene, D., Sivapalaratnam, S., Favier, R., Seyres, D., Thys, C., et al. (2019). Germline Mutations in the Transcription Factor IKZF5 Cause Thrombocytopenia. Blood 134, 2070–2081. doi:10.1182/blood.2019000782
Leonard, J. P., Trneny, M., Izutsu, K., Fowler, N. H., Hong, X., Zhu, J., et al. (2019). AUGMENT: A Phase III Study of Lenalidomide Plus Rituximab versus Placebo Plus Rituximab in Relapsed or Refractory Indolent Lymphoma. Jco 37, 1188–1199. doi:10.1200/jco.19.00010
Li, L., Ding, X., Wang, X., Yao, Q., Shao, X., An, X., et al. (2018). Polymorphisms of IKZF3 Gene and Autoimmune Thyroid Diseases: Associated with Graves' Disease but Not with Hashimoto's Thyroiditis. Cell Physiol Biochem 45, 1787–1796. doi:10.1159/000487870
Li, S., Heller, J. J., Bostick, J. W., Lee, A., Schjerven, H., Kastner, P., et al. (2016). Ikaros Inhibits Group 3 Innate Lymphoid Cell Development and Function by Suppressing the Aryl Hydrocarbon Receptor Pathway. Immunity 45, 185–197. doi:10.1016/j.immuni.2016.06.027
Li, W., Cooley, L. D., August, K. J., Richardson, A. I., Shao, L., Ahmed, A. A., et al. (2019). Cuplike Nuclear Morphology Is Highly Associated with IKZF1 Deletion in Pediatric Precursor B-Cell ALL. Blood 134, 324–329. doi:10.1182/blood.2019000604
Li, X., Xu, Z., Du, W., Zhang, Z., Wei, Y., Wang, H., et al. (2014). Aiolos Promotes anchorage independence by Silencing p66Shc Transcription in Cancer Cells. Cancer Cell 25, 575–589. doi:10.1016/j.ccr.2014.03.020
Li, Z., Li, S.-P., Li, R.-Y., Zhu, H., Liu, X., Guo, X.-L., et al. (2018). Leukaemic Alterations of IKZF1 Prime Stemness and Malignancy Programs in Human Lymphocytes. Cell Death Dis 9, 526. doi:10.1038/s41419-018-0600-3
Li, Z., Song, C., Ouyang, H., Lai, L., Payne, K. J., and Dovat, S. (2012). Cell Cycle-specific Function of Ikaros in Human Leukemia. Pediatr. Blood Cancer 59, 69–76. doi:10.1002/pbc.23406
Liu, M., Jin, J., Ji, Y., Shan, H., Zou, Z., Cao, Y., et al. (2021). Hsp90/C Terminal Hsc70-Interacting Protein Regulates the Stability of Ikaros in Acute Myeloid Leukemia Cells. China: Life sciences.
Liu, Y. Y., Ge, C., Tian, H., Jiang, J. Y., Zhao, F. Y., Li, H., et al. (2017). The Transcription Factor Ikaros Inhibits Cell Proliferation by Downregulating ANXA4 Expression in Hepatocellular Carcinoma. Am. J. Cancer Res. 7, 1285–1297.
Lonial, S., Dimopoulos, M., Palumbo, A., White, D., Grosicki, S., Spicka, I., et al. (2015). Elotuzumab Therapy for Relapsed or Refractory Multiple Myeloma. N. Engl. J. Med. 373, 621–631. doi:10.1056/nejmoa1505654
Lonial, S., Jacobus, S., Fonseca, R., Weiss, M., Kumar, S., Orlowski, R. Z., et al. (2020). Randomized Trial of Lenalidomide versus Observation in Smoldering Multiple Myeloma. Jco 38, 1126–1137. doi:10.1200/jco.19.01740
Lonial, S., Weiss, B. M., Usmani, S. Z., Singhal, S., Chari, A., Bahlis, N. J., et al. (2016). Daratumumab Monotherapy in Patients with Treatment-Refractory Multiple Myeloma (SIRIUS): an Open-Label, Randomised, Phase 2 Trial. The Lancet 387, 1551–1560. doi:10.1016/s0140-6736(15)01120-4
Lu, G., Middleton, R. E., Sun, H., Naniong, M., Ott, C. J., Mitsiades, C. S., et al. (2014). The Myeloma Drug Lenalidomide Promotes the Cereblon-dependent Destruction of Ikaros Proteins. Science 343, 305–309. doi:10.1126/science.1244917
Lyon de Ana, C., Arakcheeva, K., Agnihotri, P., Derosia, N., and Winandy, S. (2019). Lack of Ikaros Deregulates Inflammatory Gene Programs in T Cells. J.I. 202, 1112–1123. doi:10.4049/jimmunol.1801270
Ma, S., Pathak, S., Trinh, L., and Lu, R. (2008). Interferon Regulatory Factors 4 and 8 Induce the Expression of Ikaros and Aiolos to Down-Regulate Pre-B-cell Receptor and Promote Cell-Cycle Withdrawal in Pre-B-cell Development. Blood 111, 1396–1403. doi:10.1182/blood-2007-08-110106
Maciel, A. L. T., Poubel, C. P., Noronha, E. P., Pombo‐de‐Oliveira, M. S., Mansur, M. B., and Emerenciano, M. (2019). CRLF2 Expression Associates with ICN1 Stabilization in T‐cell Acute Lymphoblastic Leukemia. Genes Chromosomes Cancer 58, 396–401. doi:10.1002/gcc.22723
Marke, R., Havinga, J., Cloos, J., Demkes, M., Poelmans, G., Yuniati, L., et al. (2016). Tumor Suppressor IKZF1 Mediates Glucocorticoid Resistance in B-Cell Precursor Acute Lymphoblastic Leukemia. Leukemia 30, 1599–1603. doi:10.1038/leu.2015.359
Mateos, M.-V., Blacklock, H., Schjesvold, F., Oriol, A., Simpson, D., George, A., et al. (2019). Pembrolizumab Plus Pomalidomide and Dexamethasone for Patients with Relapsed or Refractory Multiple Myeloma (KEYNOTE-183): a Randomised, Open-Label, Phase 3 Trial. Lancet Haematol. 6, e459–e469. doi:10.1016/s2352-3026(19)30110-3
Matyskiela, M. E., Lu, G., Ito, T., Pagarigan, B., Lu, C.-C., Miller, K., et al. (2016). A Novel Cereblon Modulator Recruits GSPT1 to the CRL4CRBN Ubiquitin Ligase. Nature 535, 252–257. doi:10.1038/nature18611
Matyskiela, M. E., Zhang, W., Man, H.-W., Muller, G., Khambatta, G., Baculi, F., et al. (2018). A Cereblon Modulator (CC-220) with Improved Degradation of Ikaros and Aiolos. J. Med. Chem. 61, 535–542. doi:10.1021/acs.jmedchem.6b01921
Mazzurana, L., Forkel, M., Rao, A., Van Acker, A., Kokkinou, E., Ichiya, T., et al. (2019). Suppression of Aiolos and Ikaros Expression by Lenalidomide Reduces Human ILC3−ILC1/NK Cell Transdifferentiation. Eur. J. Immunol. 49, 1344–1355. doi:10.1002/eji.201848075
McCarty, A. S., Kleiger, G., Eisenberg, D., and Smale, S. T. (2003). Selective Dimerization of a C2H2 Zinc finger Subfamily. Mol. Cel. 11, 459–470. doi:10.1016/s1097-2765(03)00043-1
Mi, J.-Q., Wang, X., Yao, Y., Lu, H.-J., Jiang, X.-X., Zhou, J.-F., et al. (2012). Newly Diagnosed Acute Lymphoblastic Leukemia in China (II): Prognosis Related to Genetic Abnormalities in a Series of 1091 Cases. Leukemia 26, 1507–1516. doi:10.1038/leu.2012.23
Michot, J.-M., Bouabdallah, R., Vitolo, U., Doorduijn, J. K., Salles, G., Chiappella, A., et al. (2020). Avadomide Plus Obinutuzumab in Patients with Relapsed or Refractory B-Cell Non-hodgkin Lymphoma (CC-122-NHL-001): a Multicentre, Dose Escalation and Expansion Phase 1 Study. Lancet Haematol. 7, e649–e659. doi:10.1016/s2352-3026(20)30208-8
Miguel, J. S., Weisel, K., Moreau, P., Lacy, M., Song, K., Delforge, M., et al. (2013). Pomalidomide Plus Low-Dose Dexamethasone versus High-Dose Dexamethasone Alone for Patients with Relapsed and Refractory Multiple Myeloma (MM-003): a Randomised, Open-Label, Phase 3 Trial. Lancet Oncol. 14, 1055–1066. doi:10.1016/s1470-2045(13)70380-2
Mogollón, P., Díaz-Tejedor, A., Algarín, E. M., Paíno, T., Garayoa, M., and Ocio, E. M. (2019). Biological Background of Resistance to Current Standards of Care in Multiple Myeloma. Cells 8, 1. doi:10.3390/cells8111432
Molnár, A., and Georgopoulos, K. (1994). The Ikaros Gene Encodes a Family of Functionally Diverse Zinc finger DNA-Binding Proteins. Mol. Cel. Biol. 14, 8292–8303. doi:10.1128/mcb.14.12.8292
Montefusco, V., Galli, M., Spina, F., Stefanoni, P., Mussetti, A., Perrone, G., et al. (2014). Autoimmune Diseases during Treatment with Immunomodulatory Drugs in Multiple Myeloma: Selective Occurrence after Lenalidomide. Leuk. Lymphoma 55, 2032–2037. doi:10.3109/10428194.2014.914203
Moreau, P., Attal, M., Hulin, C., Arnulf, B., Belhadj, K., Benboubker, L., et al. (2019). Bortezomib, Thalidomide, and Dexamethasone with or without Daratumumab before and after Autologous Stem-Cell Transplantation for Newly Diagnosed Multiple Myeloma (CASSIOPEIA): a Randomised, Open-Label, Phase 3 Study. The Lancet 394, 29–38. doi:10.1016/s0140-6736(19)31240-1
Moreau, P., Masszi, T., Grzasko, N., Bahlis, N. J., Hansson, M., Pour, L., et al. (2016). Oral Ixazomib, Lenalidomide, and Dexamethasone for Multiple Myeloma. N. Engl. J. Med. 374, 1621–1634. doi:10.1056/nejmoa1516282
Moreira, L. B. P., Queiróz, R. P., Suazo, V. K., Perna, E., Brandalise, S. R., Yunes, J. A., et al. (2019). Detection by a Simple and Cheaper Methodology of Ik6 and Ik10 Isoforms of the IKZF1 Gene Is Highly Associated with a Poor Prognosis in B-Lineage Paediatric Acute Lymphoblastic Leukaemia. Br. J. Haematol. 187, e58–e61. doi:10.1111/bjh.16172
Morschhauser, F., Fowler, N. H., Feugier, P., Bouabdallah, R., Tilly, H., Palomba, M. L., et al. (2018). Rituximab Plus Lenalidomide in Advanced Untreated Follicular Lymphoma. N. Engl. J. Med. 379, 934–947. doi:10.1056/nejmoa1805104
Mullighan, C. G., Goorha, S., Radtke, I., Miller, C. B., Coustan-Smith, E., Dalton, J. D., et al. (2007). Genome-wide Analysis of Genetic Alterations in Acute Lymphoblastic Leukaemia. Nature 446, 758–764. doi:10.1038/nature05690
Mullighan, C. G., Miller, C. B., Radtke, I., Phillips, L. A., Dalton, J., Ma, J., et al. (2008). BCR-ABL1 Lymphoblastic Leukaemia Is Characterized by the Deletion of Ikaros. Nature 453, 110–114. doi:10.1038/nature06866
Mullighan, C. G., Su, X., Zhang, J., Radtke, I., Phillips, L. A. A., Miller, C. B., et al. (2009). Deletion ofIKZF1and Prognosis in Acute Lymphoblastic Leukemia. N. Engl. J. Med. 360, 470–480. doi:10.1056/nejmoa0808253
Murugesan, A., Lassalle-Claux, G., Hogan, L., Vaillancourt, E., Selka, A., Luiker, K., et al. (2020). Antimyeloma Potential of Caffeic Acid Phenethyl Ester and its Analogues through Sp1 Mediated Downregulation of IKZF1-IRF4-MYC Axis. J. Nat. Prod. 83 (12), 3526–3535. doi:10.1021/acs.jnatprod.0c00350
Naik, A. K., Byrd, A. T., Lucander, A. C. K., and Krangel, M. S. (2019). Hierarchical Assembly and Disassembly of a Transcriptionally Active RAG Locus in CD4+CD8+ Thymocytes. J. Exp. Med. 216, 231–243. doi:10.1084/jem.20181402
Naito, T., Ushirogawa, H., Fukushima, T., Tanaka, Y., and Saito, M. (2019). EOS, an Ikaros Family Zinc finger Transcription Factor, Interacts with the HTLV-1 Oncoprotein Tax and Is Downregulated in Peripheral Blood Mononuclear Cells of HTLV-1-Infected Individuals, Irrespective of Clinical Statuses. Virol. J. 16, 160. doi:10.1186/s12985-019-1270-1
Nakayama, J., Yamamoto, M., Hayashi, K., Satoh, H., Bundo, K., Kubo, M., et al. (2009). BLNK Suppresses Pre-B-cell Leukemogenesis through Inhibition of JAK3. Blood 113, 1483–1492. doi:10.1182/blood-2008-07-166355
Naluyima, P., Lal, K. G., Costanzo, M. C., Kijak, G. H., Gonzalez, V. D., Blom, K., et al. (2019). Terminal Effector CD8 T Cells Defined by an IKZF2+IL-7R- Transcriptional Signature Express FcγRIIIA, Expand in HIV Infection, and Mediate Potent HIV-specific Antibody-dependent Cellular Cytotoxicity. J. Immunol. 203, 2210–2221. doi:10.4049/jimmunol.1900422
Nera, K.-P., Alinikula, J., Terho, P., Narvi, E., Törnquist, K., Kurosaki, T., et al. (2006). Ikaros Has a Crucial Role in Regulation of B Cell Receptor Signaling. Eur. J. Immunol. 36, 516–525. doi:10.1002/eji.200535418
Ng, M. S. F., Roth, T. L., Mendoza, V. F., Marson, A., and Burt, T. D. (2019). Helios Enhances the Preferential Differentiation of Human Fetal CD4+ Naïve T Cells into Regulatory T Cells. Sci. Immunol. 4. doi:10.1126/sciimmunol.aav5947
Ochiai, K., Yamaoka, M., Swaminathan, A., Shima, H., Hiura, H., Matsumoto, M., et al. (2020). Chromatin Protein PC4 Orchestrates B Cell Differentiation by Collaborating with IKAROS and IRF4. Cell Rep. 33, 108517. doi:10.1016/j.celrep.2020.108517
Ocio, E. M., Fernández-Lázaro, D., San-Segundo, L., López-Corral, L., Corchete, L. A., Gutiérrez, N. C., et al. (2015). In Vivo murine Model of Acquired Resistance in Myeloma Reveals Differential Mechanisms for Lenalidomide and Pomalidomide in Combination with Dexamethasone. Leukemia 29, 705–714. doi:10.1038/leu.2014.238
Ohguchi, H., Hideshima, T., Bhasin, M. K., Gorgun, G. T., Santo, L., Cea, M., et al. (2016). The KDM3A-KLF2-IRF4 axis Maintains Myeloma Cell Survival. Nat. Commun. 7, 10258. doi:10.1038/ncomms10258
Okuyama, K., Strid, T., Kuruvilla, J., Somasundaram, R., Cristobal, S., Smith, E., et al. (2019). PAX5 Is Part of a Functional Transcription Factor Network Targeted in Lymphoid Leukemia. Plos Genet. 15, e1008280. doi:10.1371/journal.pgen.1008280
Oliveira, V. C. d., Lacerda, M. P. d., Moraes, B. B. M., Gomes, C. P., Maricato, J. T., Souza, O. F., et al. (2019). Deregulation of Ikaros Expression in B‐1 Cells: New Insights in the Malignant Transformation to Chronic Lymphocytic Leukemia. J. Leukoc. Biol. 106, 581–594. doi:10.1002/jlb.ma1118-454r
Ossenkoppele, G. J., Breems, D. A., Stuessi, G., van Norden, Y., Bargetzi, M., Biemond, B. J., et al. (2020). Lenalidomide Added to Standard Intensive Treatment for Older Patients with AML and High-Risk MDS. Leukemia 34, 1751–1759. doi:10.1038/s41375-020-0725-0
Pan, F., Yu, H., Dang, E. V., Barbi, J., Pan, X., Grosso, J. F., et al. (2009). Eos Mediates Foxp3-dependent Gene Silencing in CD4 + Regulatory T Cells. Science 325, 1142–1146. doi:10.1126/science.1176077
Park, S.-M., Cho, H., Thornton, A. M., Barlowe, T. S., Chou, T., Chhangawala, S., et al. (2019). IKZF2 Drives Leukemia Stem Cell Self-Renewal and Inhibits Myeloid Differentiation. Cell stem cell 24, 153–165. e7. doi:10.1016/j.stem.2018.10.016
Park, S.-M., Gönen, M., Vu, L., Minuesa, G., Tivnan, P., Barlowe, T. S., et al. (2015). Musashi2 Sustains the Mixed-Lineage Leukemia-Driven Stem Cell Regulatory Program. J. Clin. Invest. 125, 1286–1298. doi:10.1172/jci78440
Payne, J. L., Song, C., Ding, Y., Dhanyamraju, P. K., Bamme, Y., Schramm, J. W., et al. (2020). Regulation of Small GTPase Rab20 by Ikaros in B-Cell Acute Lymphoblastic Leukemia. Int. J. Mol. Sci. 21. doi:10.3390/ijms21051718
Payne, M. A. (2011). Zinc finger Structure-Function in Ikaros Marvin A Payne. Wjbc 2, 161–166. doi:10.4331/wjbc.v2.i6.161
Petzold, G., Fischer, E. S., and Thomä, N. H. (2016). Structural Basis of Lenalidomide-Induced CK1α Degradation by the CRL4CRBN Ubiquitin Ligase. Nature 532, 127–130. doi:10.1038/nature16979
Phan, V., Ito, T., Inaba, M., Azuma, Y., Kibata, K., Inagaki-Katashiba, N., et al. (2020). Immunomodulatory Drugs Suppress Th1-Inducing Ability of Dendritic Cells but Enhance Th2-Mediated Allergic Responses. Blood Adv. 4, 3572–3585. doi:10.1182/bloodadvances.2019001410
Popescu, M., Gurel, Z., Ronni, T., Song, C., Hung, K. Y., Payne, K. J., et al. (2009). Ikaros Stability and Pericentromeric Localization Are Regulated by Protein Phosphatase 1. J. Biol. Chem. 284, 13869–13880. doi:10.1074/jbc.m900209200
Pourabdollah, M., Bahmanyar, M., Atenafu, E. G., Reece, D., Hou, J., and Chang, H. (2016). High IKZF1/3 Protein Expression Is a Favorable Prognostic Factor for Survival of Relapsed/refractory Multiple Myeloma Patients Treated with Lenalidomide. J. Hematol. Oncol. 9, 123. doi:10.1186/s13045-016-0354-2
Powell, M. D., Read, K. A., Sreekumar, B. K., and Oestreich, K. J. (2019). Ikaros Zinc Finger Transcription Factors: Regulators of Cytokine Signaling Pathways and CD4+ T Helper Cell Differentiation. Front. Immunol. 10, 1299. doi:10.3389/fimmu.2019.01299
Qu, P., Han, J., Qiu, Y., Yu, H., Hao, J., Jin, R., et al. (2019). Huaier Extract Enhances the Treatment Efficacy of Imatinib in Ik6+ Ph+ Acute Lymphoblastic Leukemia. Biomed. Pharmacother. 117, 109071. doi:10.1016/j.biopha.2019.109071
Rahmani, M., Fardi, M., Hagh, M. F., Feizi, A. A. H., Talebi, M., and Solali, S. (2019). An Investigation of Methylation Pattern Changes in the IKZF1 Promoter in Patients with Childhood B-Cell Acute Lymphoblastic Leukemia. Blood Res. 54, 144–148. doi:10.5045/br.2019.54.2.144
Rasco, D. W., Papadopoulos, K. P., Pourdehnad, M., Gandhi, A. K., Hagner, P. R., Li, Y., et al. (2019). A First-In-Human Study of Novel Cereblon Modulator Avadomide (CC-122) in Advanced Malignancies. Clin. Cancer Res. 25, 90–98. doi:10.1158/1078-0432.ccr-18-1203
Richardson, P. G., Oriol, A., Beksac, M., Liberati, A. M., Galli, M., Schjesvold, F., et al. (2019). Pomalidomide, Bortezomib, and Dexamethasone for Patients with Relapsed or Refractory Multiple Myeloma Previously Treated with Lenalidomide (OPTIMISMM): a Randomised, Open-Label, Phase 3 Trial. Lancet Oncol. 20, 781–794. doi:10.1016/S1470-2045(19)30152-4
Rieder, S. A., Metidji, A., Glass, D. D., Thornton, A. M., Ikeda, T., Morgan, B. A., et al. (2015). Eos Is Redundant for Regulatory T Cell Function but Plays an Important Role in IL-2 and Th17 Production by CD4+ Conventional T Cells. J. Immunol. 195, 553–563. doi:10.4049/jimmunol.1500627
Ronni, T., Payne, K. J., Ho, S., Bradley, M. N., Dorsam, G., and Dovat, S. (2007). Human Ikaros Function in Activated T Cells Is Regulated by Coordinated Expression of its Largest Isoforms. J. Biol. Chem. 282, 2538–2547. doi:10.1074/jbc.m605627200
Rosiñol, L., Oriol, A., Rios, R., Sureda, A., Blanchard, M. J., Hernández, M. T., et al. (2019). Bortezomib, Lenalidomide, and Dexamethasone as Induction Therapy Prior to Autologous Transplant in Multiple Myeloma. Blood 134, 1337–1345. doi:10.1182/blood.2019000241
Ruan, J., Martin, P., Christos, P., Cerchietti, L., Tam, W., Shah, B., et al. (2018). Five-year Follow-Up of Lenalidomide Plus Rituximab as Initial Treatment of Mantle Cell Lymphoma. Blood 132, 2016–2025. doi:10.1182/blood-2018-07-859769
Ruiz, A., Jiang, J., Kempski, H., and Brady, H. J. M. (2004). Overexpression of the Ikaros 6 Isoform Is Restricted to T(4;11) Acute Lymphoblastic Leukaemia in Children and Infants and Has a Role in B-Cell Survival. Br. J. Haematol. 125, 31–37. doi:10.1111/j.1365-2141.2004.04854.x
Schafer, P. H., Ye, Y., Wu, L., Kosek, J., Ringheim, G., Yang, Z., et al. (2018). Cereblon Modulator Iberdomide Induces Degradation of the Transcription Factors Ikaros and Aiolos: Immunomodulation in Healthy Volunteers and Relevance to Systemic Lupus Erythematosus. Ann. Rheum. Dis. 77, 1516–1523. doi:10.1136/annrheumdis-2017-212916
Schott, C. A., Ascoli, C., Huang, Y., Perkins, D. L., and Finn, P. W. (2020). Declining Pulmonary Function in Interstitial Lung Disease Linked to Lymphocyte Dysfunction. Am. J. Respir. Crit. Care Med. 201, 610–613. doi:10.1164/rccm.201910-1909le
Schwickert, T. A., Tagoh, H., Gültekin, S., Dakic, A., Axelsson, E., Minnich, M., et al. (2014). Stage-specific Control of Early B Cell Development by the Transcription Factor Ikaros. Nat. Immunol. 15, 283–293. doi:10.1038/ni.2828
Schwickert, T. A., Tagoh, H., Schindler, K., Fischer, M., Jaritz, M., and Busslinger, M. (2019). Ikaros Prevents Autoimmunity by Controlling Anergy and Toll-like Receptor Signaling in B Cells. Nat. Immunol. 20, 1517–1529. doi:10.1038/s41590-019-0490-2
Sekeres, M. A., Othus, M., List, A. F., Odenike, O., Stone, R. M., Gore, S. D., et al. (2017). Randomized Phase II Study of Azacitidine Alone or in Combination with Lenalidomide or with Vorinostat in Higher-Risk Myelodysplastic Syndromes and Chronic Myelomonocytic Leukemia: North American Intergroup Study SWOG S1117. Jco 35, 2745–2753. doi:10.1200/jco.2015.66.2510
Shaffer, A. L., Emre, N. C. T., Lamy, L., Ngo, V. N., Wright, G., Xiao, W., et al. (2008). IRF4 Addiction in Multiple Myeloma. Nature 454, 226–231. doi:10.1038/nature07064
Shafi, A., Rehman, J. U., Nawaz, A., and Aljohani, N. I. (2020). Lenalidomide-induced Interstitial Pneumonitis. J. Coll. Physicians Surg. Pak 30, 1117–1118. doi:10.29271/jcpsp.2020.10.1117
Shao, C., Yang, J., Kong, Y., Cheng, C., Lu, W., Guan, H., et al. (2017). Overexpression of Dominant-Negative Ikaros 6 Isoform Is Associated with Resistance to TKIs in Patients with Philadelphia Chromosome Positive Acute Lymphoblastic Leukemia. Exp. Ther. Med. 14, 3874–3879. doi:10.3892/etm.2017.4941
Shi, C. X., Kortüm, K. M., Zhu, Y. X., Jedlowski, P., Bruins, L., Braggio, E., et al. (2015). Proteasome Inhibitors Block Ikaros Degradation by Lenalidomide in Multiple Myeloma. Haematologica 100, e315–7. doi:10.3324/haematol.2015.124297
Sievers, Q. L., Petzold, G., Bunker, R. D., Renneville, A., Słabicki, M., Liddicoat, B. J., et al. (2018). Defining the Human C2H2 Zinc finger Degrome Targeted by Thalidomide Analogs through CRBN. Science 362, eaat0572. doi:10.1126/science.aat0572
Sigvardsson, M. (2018). Molecular Regulation of Differentiation in Early B-Lymphocyte Development. Int. J. Mol. Sci. 19. doi:10.3390/ijms19071928
Song, C., Gowda, C., Pan, X., Ding, Y., Tong, Y., Tan, B.-H., et al. (2015). Targeting Casein Kinase II Restores Ikaros Tumor Suppressor Activity and Demonstrates Therapeutic Efficacy in High-Risk Leukemia. Blood 126, 1813–1822. doi:10.1182/blood-2015-06-651505
Song, C., Li, Z., Erbe, A. K., Savic, A., and Dovat, S. (2011). Regulation of Ikaros Function by Casein Kinase 2 and Protein Phosphatase 1. Wjbc 2, 126–131. doi:10.4331/wjbc.v2.i6.126
Song, C., Pan, X., Ge, Z., Gowda, C., Ding, Y., Li, H., et al. (2016). Epigenetic Regulation of Gene Expression by Ikaros, HDAC1 and Casein Kinase II in Leukemia. Leukemia 30, 1436–1440. doi:10.1038/leu.2015.331
Sriaroon, P., Chang, Y., Ujhazi, B., Csomos, K., Joshi, H. R., Zhou, Q., et al. (2019). Familial Immune Thrombocytopenia Associated with a Novel Variant in IKZF1. Front. Pediatr. 7, 139. doi:10.3389/fped.2019.00139
Sridharan, R., and Smale, S. T. (2007). Predominant Interaction of Both Ikaros and Helios with the NuRD Complex in Immature Thymocytes. J. Biol. Chem. 282, 30227–30238. doi:10.1074/jbc.m702541200
Stanulla, M., Dagdan, E., Zaliova, M., Möricke, A., Palmi, C., Cazzaniga, G., et al. (2018). IKZF1plus Defines a New Minimal Residual Disease-dependent Very-Poor Prognostic Profile in Pediatric B-Cell Precursor Acute Lymphoblastic Leukemia. Jco 36, 1240–1249. doi:10.1200/jco.2017.74.3617
Stewart, A. K., Rajkumar, S. V., Dimopoulos, M. A., Masszi, T., Špička, I., Oriol, A., et al. (2015). Carfilzomib, Lenalidomide, and Dexamethasone for Relapsed Multiple Myeloma. N. Engl. J. Med. 372, 142–152. doi:10.1056/nejmoa1411321
Swafford, A. D.-E., Howson, J. M. M., Davison, L. J., Wallace, C., Smyth, D. J., Schuilenburg, H., et al. (2011). An Allele ofIKZF1(Ikaros) Conferring Susceptibility to Childhood Acute Lymphoblastic Leukemia Protects against Type 1 Diabetes. Diabetes 60, 1041–1044. doi:10.2337/db10-0446
Symonds, E. L., Pedersen, S. K., Murray, D., Byrne, S. E., Roy, A., Karapetis, C., et al. (2020). Circulating Epigenetic Biomarkers for Detection of Recurrent Colorectal Cancer. Cancer 126, 1460–1469. doi:10.1002/cncr.32695
Symonds, E. L., Pedersen, S. K., Murray, D. H., Jedi, M., Byrne, S. E., Rabbitt, P., et al. (2018). Circulating Tumour DNA for Monitoring Colorectal Cancer-A Prospective Cohort Study to Assess Relationship to Tissue Methylation, Cancer Characteristics and Surgical Resection. Clin. Epigenet 10, 63. doi:10.1186/s13148-018-0500-5
Sznurkowska, K., Luty, J., Bryl, E., Witkowski, J. M., Hermann-Okoniewska, B., Landowski, P., et al. (2020). Enhancement of Circulating and Intestinal T Regulatory Cells and Their Expression of Helios and Neuropilin-1 in Children with Inflammatory Bowel Disease. Jir 13, 995–1005. doi:10.2147/jir.s268484
Tacchetti, P., Pantani, L., Patriarca, F., Petrucci, M. T., Zamagni, E., Dozza, L., et al. (2020). Bortezomib, Thalidomide, and Dexamethasone Followed by Double Autologous Haematopoietic Stem-Cell Transplantation for Newly Diagnosed Multiple Myeloma (GIMEMA-MMY-3006): Long-Term Follow-Up Analysis of a Randomised Phase 3, Open-Label Study. Lancet Haematol. 7, e861–e873. doi:10.1016/s2352-3026(20)30323-9
Tachita, T., Kinoshita, S., Ri, M., Aoki, S., Asano, A., Kanamori, T., et al. (2020). Expression, Mutation, and Methylation of Cereblon‐pathway Genes at Pre‐ and post‐lenalidomide Treatment in Multiple Myeloma. Cancer Sci. 111, 1333–1343. doi:10.1111/cas.14352
Tang, S. H., Lu, Y., Zhang, P. S., Liu, X. H., Du, X. H., Chen, D., et al. (2019). [Ikaros Family Zinc finger 1 Mutation Is a Poor Prognostic Factor for Adult Philadelphia Chromosome Positive Acute Lymphoblastic Leukemia]. Zhonghua nei ke za zhi 58, 301–306. doi:10.3760/cma.j.issn.0578-1426.2019.04.012
Tayel, S. I., El-Hefnway, S. M., Abo El-Fotoh, W. M. M., and El-Zayat, R. S. (2019). The Genetic Variants of IKZF1 Gene Linked with the Growing Risk of Childhood Acute Lymphoblastic Leukaemia. Cmm 19, 32–39. doi:10.2174/1566524019666190219123900
Theocharides, A. P. A., Dobson, S. M., Laurenti, E., Notta, F., Voisin, V., Cheng, P.-Y., et al. (2015). Dominant-negative Ikaros Cooperates with BCR-ABL1 to Induce Human Acute Myeloid Leukemia in Xenografts. Leukemia 29, 177–187. doi:10.1038/leu.2014.150
Thieblemont, C., Tilly, H., Gomes da Silva, M., Casasnovas, R.-O., Fruchart, C., Morschhauser, F., et al. (2017). Lenalidomide Maintenance Compared with Placebo in Responding Elderly Patients with Diffuse Large B-Cell Lymphoma Treated with First-Line Rituximab Plus Cyclophosphamide, Doxorubicin, Vincristine, and Prednisone. Jco 35, 2473–2481. doi:10.1200/jco.2017.72.6984
Thornton, A. M., and Shevach, E. M. (2019). Helios: Still behind the Clouds. Immunology 158, 161–170. doi:10.1111/imm.13115
Tsukamoto, T., Nakahata, S., Sato, R., Kanai, A., Nakano, M., Chinen, Y., et al. (2020). BRD4-Regulated Molecular Targets in Mantle Cell Lymphoma: Insights into Targeted Therapeutic Approach. Cancer Genomics Proteomics 17, 77–89. doi:10.21873/cgp.20169
Uckun, F. M., Ma, H., Zhang, J., Ozer, Z., Dovat, S., Mao, C., et al. (2012). Serine Phosphorylation by SYK Is Critical for Nuclear Localization and Transcription Factor Function of Ikaros. Proc. Natl. Acad. Sci. 109, 18072–18077. doi:10.1073/pnas.1209828109
Usmani, S. Z., Hoering, A., Ailawadhi, S., Sexton, R., Lipe, B., Hita, S. F., et al. (2021). Bortezomib, Lenalidomide, and Dexamethasone with or without Elotuzumab in Patients with Untreated, High-Risk Multiple Myeloma (SWOG-1211): Primary Analysis of a Randomised, Phase 2 Trial. Lancet Haematol. 8, e45–e54. doi:10.1016/s2352-3026(20)30354-9
Usmani, S. Z., Nahi, H., Plesner, T., Weiss, B. M., Bahlis, N. J., Belch, A., et al. (2020). Daratumumab Monotherapy in Patients with Heavily Pretreated Relapsed or Refractory Multiple Myeloma: Final Results from the Phase 2 GEN501 and SIRIUS Trials. Lancet Haematol. 7, e447–e455. doi:10.1016/s2352-3026(20)30081-8
Usmani, S. Z., Schjesvold, F., Oriol, A., Karlin, L., Cavo, M., Rifkin, R. M., et al. (2019). Pembrolizumab Plus Lenalidomide and Dexamethasone for Patients with Treatment-Naive Multiple Myeloma (KEYNOTE-185): a Randomised, Open-Label, Phase 3 Trial. Lancet Haematol. 6, e448–e458. doi:10.1016/s2352-3026(19)30109-7
Vairy, S., and Tran, T. H. (2020). IKZF1 Alterations in Acute Lymphoblastic Leukemia: The Good, the Bad and the Ugly. Blood Rev. 44, 100677. doi:10.1016/j.blre.2020.100677
Verhoeven, R. J. A., Tong, S., Mok, B. W.-Y., Liu, J., He, S., Zong, J., et al. (2019). Epstein-Barr Virus BART Long Non-coding RNAs Function as Epigenetic Modulators in Nasopharyngeal Carcinoma. Front. Oncol. 9, 1120. doi:10.3389/fonc.2019.01120
Vitale, C., Falchi, L., Ten Hacken, E., Gao, H., Shaim, H., Van Roosbroeck, K., et al. (2016). Ofatumumab and Lenalidomide for Patients with Relapsed or Refractory Chronic Lymphocytic Leukemia: Correlation between Responses and Immune Characteristics. Clin. Cancer Res. 22, 2359–2367. doi:10.1158/1078-0432.ccr-15-2476
Vivier, E., Artis, D., Colonna, M., Diefenbach, A., Di Santo, J. P., Eberl, G., et al. (2018). Innate Lymphoid Cells: 10 Years on. Cell 174, 1054–1066. doi:10.1016/j.cell.2018.07.017
Voorhees, P. M., Kaufman, J. L., Laubach, J., Sborov, D. W., Reeves, B., Rodriguez, C., et al. (2020). Daratumumab, Lenalidomide, Bortezomib, and Dexamethasone for Transplant-Eligible Newly Diagnosed Multiple Myeloma: the GRIFFIN Trial. Blood 136, 936–945. doi:10.1182/blood.2020005288
Wang, H., Xu, Z., Du, W., Lin, Z., and Liu, Z. (2019). N160 of Aiolos Determines its DNA-Binding Activity. Anat. Rec. (Hoboken) 302, 2014–2019. doi:10.1002/ar.24213
Wang, H., Song, C., Ding, Y., Pan, X., Ge, Z., Tan, B.-H., et al. (2016). Transcriptional Regulation of JARID1B/KDM5B Histone Demethylase by Ikaros, Histone Deacetylase 1 (HDAC1), and Casein Kinase 2 (CK2) in B-Cell Acute Lymphoblastic Leukemia. J. Biol. Chem. 291, 4004–4018. doi:10.1074/jbc.m115.679332
Wang, H., Song, C., Gurel, Z., Song, N., Ma, J., Ouyang, H., et al. (2014). Protein Phosphatase 1 (PP1) and Casein Kinase II (CK2) Regulate Ikaros-Mediated Repression ofTdTin Thymocytes and T-Cell Leukemia. Pediatr. Blood Cancer 61, 2230–2235. doi:10.1002/pbc.25221
Wang, M. L., Rule, S., Martin, P., Goy, A., Auer, R., Kahl, B. S., et al. (2013). Targeting BTK with Ibrutinib in Relapsed or Refractory Mantle-Cell Lymphoma. N. Engl. J. Med. 369, 507–516. doi:10.1056/nejmoa1306220
Wang, M., Rule, S., Zinzani, P. L., Goy, A., Casasnovas, O., Smith, S. D., et al. (2018). Acalabrutinib in Relapsed or Refractory Mantle Cell Lymphoma (ACE-LY-004): a Single-Arm, Multicentre, Phase 2 Trial. The Lancet 391, 659–667. doi:10.1016/s0140-6736(17)33108-2
Wang, Y., Zheng, X., Wang, Q., Zheng, M., and Pang, L. (2020). GSK3β-Ikaros-ANXA4 Signaling Inhibits High-Glucose-Induced Fibroblast Migration. Biochem. biophysical Res. Commun. 531, 543–551. doi:10.1016/j.bbrc.2020.07.142
Wang, Z., Zhou, G., Risu, N., Fu, J., Zou, Y., Tang, J., et al. (2020). Lenalidomide Enhances CAR-T Cell Activity against Solid Tumor Cells. Cell Transpl. 29, 963689720920825. doi:10.1177/0963689720920825
Winandy, S., Wu, P., and Georgopoulos, K. (1995). A Dominant Mutation in the Ikaros Gene Leads to Rapid Development of Leukemia and Lymphoma. Cell 83, 289–299. doi:10.1016/0092-8674(95)90170-1
Won, H. R., Lee, D. H., Yeon, S. K., Ryu, H. W., Kim, G. W., and Kwon, S. H. (2019). HDAC6-selective I-nhibitor S-ynergistically E-nhances the A-nticancer A-ctivity of I-mmunomodulatory D-rugs in M-ultiple M-yeloma. Int. J. Oncol. 55, 499–512. doi:10.3892/ijo.2019.4828
Xiao, G., Chan, L. N., Klemm, L., Braas, D., Chen, Z., Geng, H., et al. (2018). B-Cell-Specific Diversion of Glucose Carbon Utilization Reveals a Unique Vulnerability in B Cell Malignancies. Cell 173, 470–484. e18. doi:10.1016/j.cell.2018.02.048
Yamamoto, E., Ito, T., Abe, A., Sido, F., Ino, K., Itakura, A., et al. (2005). Ikaros Is Expressed in Human Extravillous Trophoblasts and Involved in Their Migration and Invasion. Mol. Hum. Reprod. 11, 825–831. doi:10.1093/molehr/gah239
Yang, M., Liu, Y., Mo, B., Xue, Y., Ye, C., Jiang, Y., et al. (2019). Helios but Not CD226, TIGIT and Foxp3 Is a Potential Marker for CD4+ Treg Cells in Patients with Rheumatoid Arthritis. Cell Physiol Biochem 52, 1178–1192. doi:10.33594/000000080
Yasuda, T., Maeda, A., Kurosaki, M., Tezuka, T., Hironaka, K., Yamamoto, T., et al. (2000). Cbl Suppresses B Cell Receptor-Mediated Phospholipase C (Plc)-Γ2 Activation by Regulating B Cell Linker Protein-Plc-Γ2 Binding. J. Exp. Med. 191, 641–650. doi:10.1084/jem.191.4.641
Yeoh, A. E. J., Lu, Y., Chin, W. H. N., Chiew, E. K. H., Lim, E. H., Li, Z., et al. (2018). Intensifying Treatment of Childhood B-Lymphoblastic Leukemia with IKZF1 Deletion Reduces Relapse and Improves Overall Survival: Results of Malaysia-Singapore ALL 2010 Study. Jco 36, 2726–2735. doi:10.1200/jco.2018.78.3050
Yu, W.-Q., Ji, N.-F., Gu, C.-J., Sun, Z.-X., Wang, Z.-X., Chen, Z.-Q., et al. (2019). Downregulation of miR-4772-3p Promotes Enhanced Regulatory T Cell Capacity in Malignant Pleural Effusion by Elevating Helios Levels. Chin. Med. J. 132, 2705–2715. doi:10.1097/cm9.0000000000000517
Yuan, T., Yang, Y., Chen, J., Li, W., Li, W., Zhang, Q., et al. (2017). Regulation of PI3K Signaling in T-Cell Acute Lymphoblastic Leukemia: a Novel PTEN/Ikaros/miR-26b Mechanism Reveals a Critical Targetable Role for PIK3CD. Leukemia 31, 2355–2364. doi:10.1038/leu.2017.80
Zeidner, J. F., Knaus, H. A., Zeidan, A. M., Blackford, A. L., Montiel-Esparza, R., Hackl, H., et al. (2020). Immunomodulation with Pomalidomide at Early Lymphocyte Recovery after Induction Chemotherapy in Newly Diagnosed AML and High-Risk MDS. Leukemia 34, 1563–1576. doi:10.1038/s41375-019-0693-4
Zhang, X., Zhang, X., Li, X., Lv, Y., Zhu, Y., Wang, J., et al. (2020). The Specific Distribution Pattern of IKZF1 Mutation in Acute Myeloid Leukemia. J. Hematol. Oncol. 13, 140. doi:10.1186/s13045-020-00972-5
Zhao, W., Chen, T. B., and Wang, H. (2020). Ikaros Is Heterogeneously Expressed in Lung Adenocarcinoma and Is Involved in its Progression. J. Int. Med. Res. 48, 300060520945860. doi:10.1177/0300060520945860
Zheng, J., Sha, Y., Roof, L., Foreman, O., Lazarchick, J., Venkta, J. K., et al. (2019). Pan-PIM Kinase Inhibitors Enhance Lenalidomide's Anti-myeloma Activity via Cereblon-Ikzf1/3 cascade. Cancer Lett. 440-441, 1–10. doi:10.1016/j.canlet.2018.10.003
Zhou, H., Yan, Y., Zhang, X., Zhao, T., Xu, J., and Han, R. (2020). Ginseng Polysaccharide Inhibits MDA-MB-231 Cell Proliferation by Activating the Inflammatory Response. Exp. Ther. Med. 20, 229. doi:10.3892/etm.2020.9359
Zhou, N., Gutierrez-Uzquiza, A., Zheng, X. Y., Chang, R., Vogl, D. T., Garfall, A. L., et al. (2019). RUNX Proteins Desensitize Multiple Myeloma to Lenalidomide via Protecting IKZFs from Degradation. Leukemia 33, 2006–2021. doi:10.1038/s41375-019-0403-2
Zhu, Y. X., Braggio, E., Shi, C.-X., Kortuem, K. M., Bruins, L. A., Schmidt, J. E., et al. (2014). Identification of Cereblon-Binding Proteins and Relationship with Response and Survival after IMiDs in Multiple Myeloma. Blood 124, 536–545. doi:10.1182/blood-2014-02-557819
Zhu, Y. X., Shi, C.-X., Bruins, L. A., Wang, X., Riggs, D. L., Porter, B., et al. (2019). Identification of Lenalidomide Resistance Pathways in Myeloma and Targeted Resensitization Using Cereblon Replacement, Inhibition of STAT3 or Targeting of IRF4. Blood Cancer J. 9, 19. doi:10.1038/s41408-019-0173-0
Zou, J., Jones, R. J., Wang, H., Kuiatse, I., Shirazi, F., Manasanch, E. E., et al. (2020). The Novel Protein Homeostatic Modulator BTX306 Is Active in Myeloma and Overcomes Bortezomib and Lenalidomide Resistance. J. Mol. Med. 98, 1161–1173. doi:10.1007/s00109-020-01943-6
Glossary
TF transcription factor
IKZF IKAROS family zinc finger
AML acute myeloid leukemia
Len lenalidomide
MM multiple myeloma
BCP-ALL pediatric B-cell precursor acute lymphoblastic leukemia
ALL acute lymphoblastic leukemia
CRBN cereblon
MCL mantle cell lymphoma
IMiDs immunomodulatory drugs
MDS myelodysplastic syndrome
CLL chronic lymphocytic leukemia
NuRD nucleosome remodeling and deacetylase complex
HDAC histone deacetylase complexes
PP1 protein phosphatase 1 protein phosphatase 1α
PI3K phosphatidylinositol-3 kinase
BCR B-cell receptor
TCR T-cell receptor
IRF interferon regulatory factor
SUMOylation small ubiquitin-related modifier
RUNX runt-related transcription factor family
CK2 carcinogenic casein kinase II
ILC innate lymphoid cell
TH T helper cell
Treg T regulated cell
KDM5B lysine [K]-specific demethylase 5B
PHF2 PHD finger protein 2
ARID5B AT-rich interactive domain-containing protein 5B
G6PD glucose-6-phosphate dehydrogenase
JAK-STAT Janus kinase-signal transducer and activator of transcription
IL7R interleukin-7 receptor
BCL6 B-cell lymphoma 6
BACH2 basic leucine zipper transcription factor 2
RAG recombination-activating gene
KLF2 Krüppel-like factor 2
Blimp-1 B lymphocyte maturation inducing protein-1
CAR T-cell chimeric antigen receptor T-cell
CELMoDs CRBN E3 ligase modulating drugs
SLL small lymphocytic lymphoma
RRMM relapsed/refractory multiple myeloma
NDMM newly diagnosed multiple myeloma
BTZ bortezomib
BTK Bruton tyrosine kinase
BRD4 bromodomain-containing protein 4
PAX5 paired box 5
Keywords: Ikaros, Aiolos, hematological malignancies, targeted therapy, immunotherapy
Citation: Xia R, Cheng Y, Han X, Wei Y and Wei X (2021) Ikaros Proteins in Tumor: Current Perspectives and New Developments. Front. Mol. Biosci. 8:788440. doi: 10.3389/fmolb.2021.788440
Received: 02 October 2021; Accepted: 09 November 2021;
Published: 07 December 2021.
Edited by:
Hongming Miao, Army Medical University, ChinaReviewed by:
Tian Xiaohe, Anhui University, ChinaGuihua Wang, Huazhong University of Science and Technology, China
Copyright © 2021 Xia, Cheng, Han, Wei and Wei. This is an open-access article distributed under the terms of the Creative Commons Attribution License (CC BY). The use, distribution or reproduction in other forums is permitted, provided the original author(s) and the copyright owner(s) are credited and that the original publication in this journal is cited, in accordance with accepted academic practice. No use, distribution or reproduction is permitted which does not comply with these terms.
*Correspondence: Yuquan Wei, eXVxdWFud2VpQHNjdS5lZHUuY24=; Xiawei Wei, eGlhd2Vpd2VpQHNjdS5lZHUuY24=
†These authors have contributed equally to this work