- 1Key Specialty of Clinical Pharmacy, The First Affiliated Hospital of Guangdong Pharmaceutical University, Guangzhou, China
- 2NMPA Key Laboratory for Technology Research and Evaluation of Pharmacovigilance, Guangdong Pharmaceutical University, Guangzhou, China
- 3School of Clinical Pharmacy, Guangdong Pharmaceutical University, Guangzhou, China
Prostate cancer (PCa) is a top-incidence malignancy, and the second most common cause of death amongst American men and the fifth leading cause of cancer death in men around the world. Androgen receptor (AR), the key transcription factor, is critical for the progression of PCa by regulating a series of target genes by androgen stimulation. A number of co-regulators of AR, including co-activators or co-repressors, have been implicated in AR-mediated gene transcription and PCa progression. Epigenetic regulators, by modifying chromatin integrity and accessibility for transcription regulation without altering DNA sequences, influence the transcriptional activity of AR and further regulate the gene expression of AR target genes in determining cell fate, PCa progression and therapeutic response. In this review, we summarized the structural interaction of AR and epigenetic regulators including histone or DNA methylation, histone acetylation or non-coding RNA, and functional synergy in PCa progression. Importantly, epigenetic regulators have been validated as diagnostic markers and therapeutic targets. A series of epigenetic target drugs have been developed, and have demonstrated the potential to treat PCa alone or in combination with antiandrogens.
Introduction
Prostate cancer (PCa) is the second most commonly diagnosed cancer type and the fifth leading cause of cancer death in men around the world (Bray et al., 2018). Perturbed transcriptional control is one of main mechanisms driving the development of PCa. Multiple key transcription factors (TFs) crucial for PCa progression have been identified, such as androgen receptor (AR), FOXA1, N-myc and the ETS-domain transcription factor family, etc. AR is the most important TF promoting the progression of hormone-dependent and independent PCa. Since the androgen/AR axis plays key roles in driving PCa progression, androgen deprivation therapy (ADT) remains the mainstream therapeutic modality for PCa (Kawakami et al., 2006). However, most PCa patients eventually become refractory to ADT, and the disease inevitably develops to castration-resistant prostate cancer (CRPC). At this stage, bone metastasis develops, the life quality of patients deteriorates, and commonly the life span is less than 1 year (Kirby et al., 2011). Thus, it remains urgent to clarify the molecular mechanism of CRPC and identify new diagnostic and therapeutic targets, so PCa patients can be diagnosed sooner and effectively treated.
Epigenetics refers to biological processes that regulate gene expression and function without altering DNA sequences (Holliday, 1994; Weinhold, 2006). Epigenetic modifications, including DNA methylation, histone modifications and non-coding RNA (Liao and Xu, 2019), regulate gene transcription, including the addition of chemical “tags” on DNA and the posttranslational modification (PTM) of histone proteins, imparting distinct features on chromatin architecture. Currently the key enzymes catalyzing these modifications have been identified as epigenetic regulators and categorized as “writers”, “readers” and “erasers”. “Writers” such as DNA methyltransferases (DNMTs) and histone acetyltransferases (HATs) introduce chemical modifications such as methylation and/or acetylation on DNA and histone proteins. “Readers” are the specialized proteins that identify and interpret those modifications and convey the epigenetic information to downstream effectors. “Erasers” are enzymes proficient in removing these epigenetic markers, and include histone demethylases (KDMs) and histone deacetylase complexes (HDACs) (Yegnasubramanian, 2016).
These epigenetic modifications enhance the establishment of a context-specific transcriptional profile, and aberrations ultimately cause genomic instability. Aberrations of epigenetic regulators such as gene amplification or mutations have been frequently found in PCa (Li et al., 2019), and play vital roles in the initiation and progression of cancer (Graca et al., 2016). The epigenetic regulators, individually or cooperatively with AR, contribute PCa progression as transcriptional co-activators or co-repressors of AR.
In this review, we summarized the known epigenetic regulators, including readers, writers, and erasers working together with AR in PCa progression, and discussed their potential as epigenetic diagnostic and therapeutic targets, as well as future translational applications for PCa.
Androgen/Androgen Receptor Pathways and Epigenetic Co-regulators in Prostate Cancer
Androgen is mainly produced by the conversion of cholesterol in the testes, muscle, adrenal glands, ovaries, skin, adipose tissue and endometrium (Narayanan et al., 2018). Androgen is a major male steroid that binds to AR to determine masculinity and sexual maturation. AR dissociates from heat shock proteins (HSPs), and AR phosphorylation promotes AR translocation to the nuclei where AR binds to the androgen response element (ARE) motifs through zinc finger domains. AR recruits co-regulators, and regulates the transcription of downstream target genes (Eftekharzadeh et al., 2019) (Figure 1).
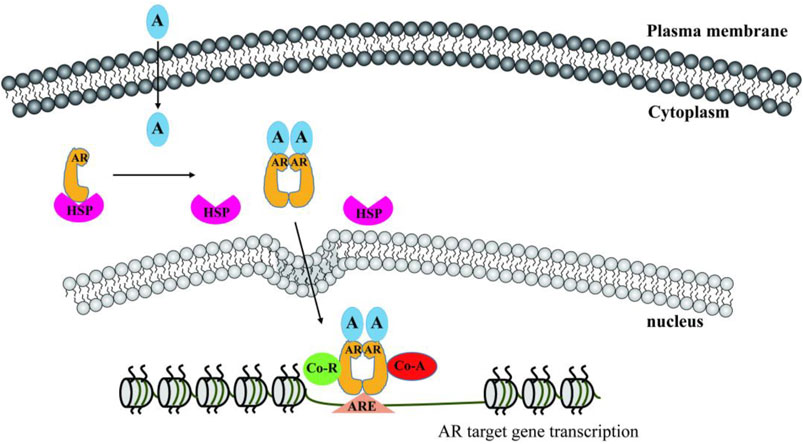
FIGURE 1. Androgen/AR signaling pathway. A, androgen; AR, androgen receptor; HSP, heat shock protein; ARE, androgen response element; Co-R, co-repressor; Co-A, co-activator.
AR, also named NR3C4, is an important member of the nuclear receptor superfamily. Its full-length gene transcript contains eight exons, which synthesize three major functional protein domains with individual functions (Roy et al., 2001; Yu et al., 2020). The N-terminal domain (NTD) is encoded by exon 1, the DNA binding domain (DBD) is encoded by exons 2 and 3, and exons 4 to 8 encode the ligand-binding domain (LBD), which is connected to DBD through the flexure hinge region. The color boxes in Figure 2 represent individual protein domains including NTD, DBD, hinge, and LBD. All these domains are crucial for AR function. Recently, by using cryogenic electron microscopy (Cryo-EM), Yu et al. resolved the three-dimensional (3-D) structure of full-length AR protein (AR-FL), and observed the structural interaction of AR protein and the androgen responsive elements (ARE) binding motif in the promoter of target gene (Yu, et al., 2020).
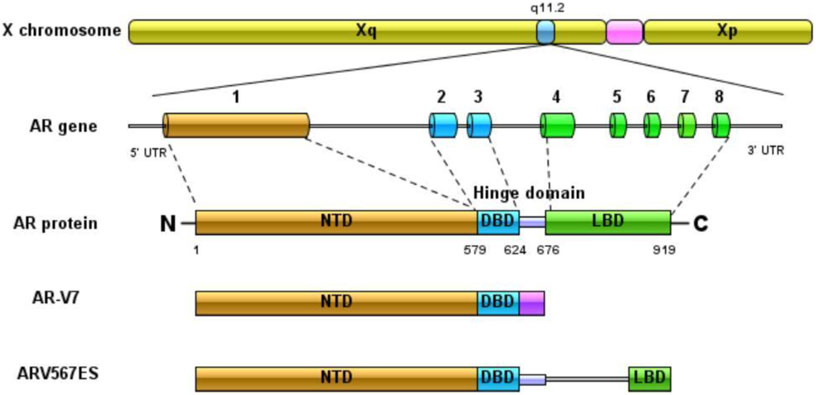
FIGURE 2. The transcriptional structure of full-length AR and major splicing variants AR-V7 and ARV567ES.
AR co-regulators, recruited by the individual domains of AR protein, modify the epigenetic conditions around the ARE binding motifs in the chromatin, thereby affecting the transcriptional activity of AR. Compelling evidence has highlighted the central roles of chromatin structure and histone posttranslational modifications (PTMs) in determining the spectrum of genes regulated by AR and other transcription factors (Dehm and Tindall, 2007; Cai et al., 2013). Multiple histone modifying enzymes, together with demethylation enzymes directly regulate AR expression and its transcriptional activity (Leader et al., 2006). Additionally, non-coding RNAs, including long non-coding RNAs (lncRNAs) and micro RNAs (miRNAs), directly or indirectly modulate the epigenetic status to regulate AR-regulated gene transcription. The dysregulation of gene transcription contributes to PCa progression (Gao and Alumkal, 2010).
The crystal structures of AR-FL, and the domains of hinge/DBD/LBD have been resolved (Figure 3), but that of NTD (AF-1), the strongest active domain has not been resolved yet.
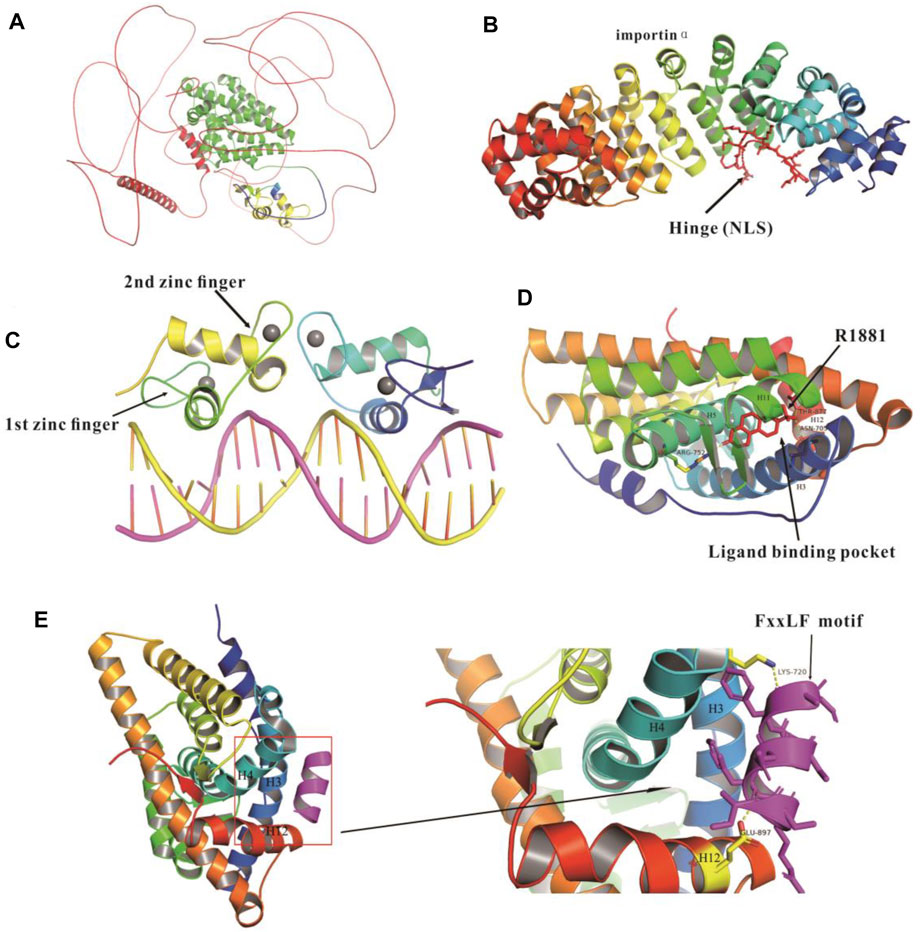
FIGURE 3. Crystal structures of full length, and domains of DBD, hinge and LBD of AR protein. (A) Crystal structure of full-length AR (Uniport: P10275, AlphaFold2). NTD, (amino acid residue 1-555) (red), DNA binding domain (DBD, amino acid residue 556-620) (yellow), hinge domain (amino acid residue 617-668) (blue); hinge and ligand binding domain (hinge-LBD, Amino acid residues 617-918) (green); LBD (amino acid residues 669-919) and AR NLS regions (amino acid residues 617-635) (color gradient). (B) Crystal structure of hinge domain and NLS regions (amino acid 621-635) (red) complexed with importin-α (PDB: 3BTR). (C) Crystal structure of DBD (PDB: 1R4I). (D) Crystallographic structure of protein complex containing LBD and a synthetic androgen metrexone R1881 (PDB: 1E3G), the ligand binding pocket surrounded by the N-terminus of H3, H5, H11 and H12. (E) Structure of AR LBD complex with FxxLF motif peptide (purple), the figure on the right shows the interface between AR LBD and FxxLF motif (PDB:1T7R). Hydrogen bonds are shown in dotted yellow lines.
Co-Regulators Recruited by the N-Terminal Domain Domain of Androgen Receptor
The NTD of AR protein, a relatively long and flexible domain, accounts for more than 60% of AR protein, but the crystal structure of the NTD of AR protein has not been resolved yet. Within the NTD is activation function 1 (AF-1), which is essential for AR transactivational activity (Jenster et al., 1995; Dehm and Tindall, 2007). Co-activators of the p160 family such as SRC1 (steroid receptor coactivator 1) have been reported to directly bind and activate AF-1. The AF-1 contains two large domains TAU1 (amino acids 101-370) and TAU5 (amino acids 360-485), which are indispensable for receptor-dependent transactivation (Dehm and Tindall, 2007; Jenster, et al., 1995). Integrity of TAU-5 is a prerequisite for p160 co-activators recruitment by AR (Callewaert et al., 2006). In addition, the Cryo-EM decoded the 3-D structure of the protein complex containing DNA-bound full-length AR and its key co-activators SRC3 and p300, and identified the NTD as the primary site for the recruitment of co-activators (Yu, et al., 2020). HATs P300 and P300/CREB binding protein (CBP) induce AR and histone acetylation, and recruit proteins containing bromodomains such as BRD4, which promote the proliferation, migration and invasion of PCa cells (Gao and Alumkal, 2010; Belkina and Denis, 2012).
Co-Regulators Recruited by the DNA Binding Domain/Hinge Domain of Androgen Receptor
The DBD/hinge domain of AR protein is the most conserved region and is composed of two zinc fingers based on the crystal structure of the DBD (Figure3) (Shaffer et al., 2004). Its functions include AR nuclear localization, dimerization, mediating AR DNA recognition and binding to the AREs (Dehm and Tindall, 2007). The first zinc finger is the P-box, by which AR binds to the nucleotide bases of the DNA major groove, responsible for sequence-specific DNA recognition. The second zinc finger is the D-box, including the AR DBD and carboxy-terminal extension (CTE), and this zinc finger is the AR dimerization interface, recapitulating the selectivity and specificity of the DNA binding site and inducing the dimerization of full-length receptor (Verrijdt et al., 2003; Dehm and Tindall, 2007). AR-DBD binds to the AREs in the prostate specific antigen (PSA) gene enhancers and induces the dimerization of AR DBD from head to head, which may be an appropriate model to explain androgen specificity (Tan et al., 2015). AR regulates the transmembrane protease serine 2 (TMPRSS2) gene by binding to the ARE sites in the promoter, which results in the abnormal overexpression of such ETS family oncogenes as ERG and ETV1. The fusion genes of TMPRSS2 and ETS oncogenes are associated with aggressive lesions, poor prognosis, and early-onset PCa (Tomlins et al., 2006; Tomlins et al., 2005; Verrijdt, et al., 2003). In addition, chromatin modifying enzymes, such as JMJD2C and LSD1, induce chromatin remodeling and modify AR binding to ARE sites to regulate gene transcription (Gao and Alumkal, 2010). Pioneer factors, a special class of TFs including FOXO and GATA2, bind to the compacted chromatin and initiate the recruitment of other TFs, such as ER or AR to access their DNA target sites to regulate gene transcriptional activation (Jozwik and Carroll, 2012).
The hinge region is important for the subcellular distribution of nuclear receptors (Jenster et al., 1993; Zhou et al., 1994). Nuclear localization signals (NLS) located at the intersection of the DBD and hinge region of AR could regulate the nuclear import of nuclear receptors. The crystal structure of importin-α and AR shows that importin-α mediates the nuclear import of AR, and the residues from the major NLS 629-RKLKKL-634 contribute to importin-α binding to AR, and promote the AR transcriptional activity (Clinckemalie et al., 2012; Jenster, et al., 1993). Additionally, the hinge region of AR regulates the epigenetic pathway, especially the acetylation of its 629-RKLKKL-634 motif, which plays key roles in DNA binding, co-activator recruitment and the N/C interaction, and is a target site of acetylation, methylation and ubiquitination (Clinckemalie, et al., 2012; Fu et al., 2006). Multiple proteins, such as SNURF, ASC-1 and BAF57, work as co-activators of AR (Moilanen et al., 1998; Lee et al., 2002; Link et al., 2008), while others work as co-repressors including ARR19 and NcoR by binding to the hinge region Figure 4 (Wang H. et al., 2001; Jeong et al., 2004). In addition, SIRT1 induces the deacetylation of AR at K630, and thereby inhibits AR activity and the growth of PCa (Clinckemalie, et al., 2012).
Co-Regulators Recruited by the Ligand-Binding Domain Domain of Androgen Receptor
The ligand-dependent functional binding domain (AF2) in glucocorticoid receptor (GR) or estrogen receptor (ER) shows strong transcriptional activity (Jenster et al., 1992; Yu, et al., 2020). However, the binding of ligand to AR-LBD initiates significant conformational changes, and forms a hydrophobic groove, which serves as a docking site for a LXXLL motif present in co-regulators, such as SRCs (Heery et al., 1997). The LBD contains the activation function 2 (AF-2, ∼250 amino acids) and mediates the interaction between AR and HSPs (Eftekharzadeh, et al., 2019; He et al., 2000), which prevents AR protein from degradation without androgen stimulation. AF-2 not only is the docking site of coactivators, but also promotes the N/C interaction, the recruitment of coactivators and AR transcriptional activation (Dehm and Tindall, 2007; Klokk et al., 2007). LBD interacts with NTD or other specific co-factors through the FXXLF motif to regulate gene transcription (Dubbink et al., 2006; Messner et al., 2020). The first crystallographic structure of AR-LBD-bound by the synthetic androgen metrexone R1881 has been resolved (Figure 3D) (Matias et al., 2000). R1881, by binding to LBD via hydrogen bonds, thus serves as the AR LBD agonist. LBD is the major binding site of anti-androgen drugs, but drug resistance occurs due to AR mutation and amplification.
The absence of LBD domain in splice variant AR forms (AR-Vs) constitutively sustains active AR signaling after anti-androgen drug treatment (Zhu and Luo, 2020).
AR-V7, a truncated AR splicing variant 7 without LBD, is highly active in CRPC, and promotes abiraterone and enzalutamide resistance (Nakazawa et al., 2014; Aurilio et al., 2020). Its mRNA retains the first three typical exons, and then connects with a mutation-specific recessive exon 3 (CE3). The splicing variation results from the early translation termination of 16 mutation-specific amino acids. In addition, ARv567ES retains the first 4 exons, connecting with the exon8, but misses the exons 5, 6 and 7.
Epigenetic Control of Prostate Cancer
DNA Methylation/Demethylation
DNA methylation is the most extensively studied epigenetic mechanism essential for gene transcription regulation. DNA methylation is primarily catalyzed by a family of DNA methyltransferases: DNMT1, DNMT3A and DNMT3B. DNMT1 is a major DNA methyltransferase for DNA methylation maintanace, while DNMT3A and DNMT3B, as de novo DNA methyltransferases, mainly initiate the DNA methylation (Okano et al., 1999). In PCa, many CpG islands display perturbed hypermethylation. Multiple key tumor suppressor genes such as p53, Rb1 and BRCA1, have undergone CpG islands hypermethylation in their promoter regions (Baylin, 2005). Aberrant DNA methylation has been linked to PCa initiation and progression (Nelson et al., 2009).
It has been reported that AR promoter is hypermethylated, which results in low AR expression in some of the PCa (Ruggero et al., 2018). DNMT1, cooperating with E2F1, down-regulates AR gene transcription in a methylation-independent manner (Valdez et al., 2011). Overexpression of DNMT1 induces DNA hypermethylation and represses the gene transcription of TMPRSS2, an AR target gene in AR-negative PCa cells (Chu et al., 2014). In contrast, DNMT1 knockdown leads to de-repression of endogenous AR in human normal prostate epithelial cells (Valdez, et al., 2011).
DNA methylation patterns are largely erased and then re-established between generations of mammals. Ten-eleven translocation proteins (TET1-3) mediate the oxidation of 5-methylcytosine (5mC) to 5-hydroxymethylcytosine (5hmC), 5-formylcytosine (5fC), and 5-carboxycytosine (5caC) (Tahiliani et al., 2009; He et al., 2011; Ito et al., 2011). And then, the oxidized forms are directly excised by DNA glycosylases such as thymine DNA glycosylase (TDG) (He, et al., 2011). 5hmC, the major oxidized form of 5mC, is profoundly reduced in human cancers, including PCa. DNA hypomethylation is often observed in the metastasized PCa. Hypomethylation in the promoter region of oncogenes leads to activate transcription and promotes cell proliferation. For example, the reduced genome-wide methylation weakens the stability of chromatin and promotes the expression of proto-oncogene MYC and RAS, thereby promoting PCa invasion and metastasis (Gurel et al., 2008; Albany et al., 2011).
Histone Modifications
Histone Methylation
Histone modifications play vital roles in cancer progression (Ruggero, et al., 2018). Methyltransferases (KMTs) and demethylases (KDMs) are important regulators of histone methylation and gene transcription (Table 1) (Coffey et al., 2013; Askew et al., 2017). AR-dependent gene transcription depends on both histone methyltransferase and demethylase activities. The protein arginine methyltransferase (PRMT-4), more commonly known as co-activator-associated arginine methyltransferase 1 (CARM-1), by interacting with SRC co-activators, is essential for specific arginine methylation on histones H3. CARM-1 promotes AR-mediated transactivation completely depending on the presence of SRC proteins. Due to its indirect recruitment to AR, CARM-1 has been classified as a secondary co-activator (Chen et al., 1999). Additionally, PRMT1 is recruited to the AR transcriptional complex and promotes AR-dependent gene expression through SRC proteins (Wang Q. et al., 2001). The methyltransferase G9a is predominantly related to transcriptional repression by regulating the methylation lysine 9 on histone 3 (H3K9). However, G9a functions as a co-activator by synergistically working with TIF-2 and CARM-1 (Lee et al., 2006). LSD1 removes the methyl groups from dimethylated or monomethylated Lys 4 or Lys 9 on histone H3, thereby acting as a co-activator or co-suppressor of AR (Lee, et al., 2006). JMJD2C cooperates with LSD1 to demethylate the repressively trimethylated H3K9 during gene activation by AR (Schulz and Hoffmann, 2009). The histone demethylase JHDM2A cooperates with AR, and induces H3K9 demethylation as well as transcriptional activation (Yamane et al., 2006).
Histone Acetylation
Histone acetylation is generally associated with active transcription, regulated by HATs and HDACs (Table 1) (Mathis et al., 1978; Grunstein, 1997; Abbas and Gupta, 2008).
Tip60, a histone acetyltransferase abnormally overexpressing in CRPC tissues, directly induces the acetylation of H2A, H3 and H4 through the HAT region at the C-terminal, and directly induces AR acetylation through the activation of the intrinsic acetyltransferases (Gaughan et al., 2002). Histone acetyltransferase P300 and PCAF (p300/CBP-associating factor) induce the acetylation of three lysine residues of AR (Lys-630 and Lys-632 and Lys-633), thereby promoting the intrinsic transcriptional activity of AR. The acetylation of AR may be reversed by HDACs including Sirtuin 1, thereby reducing the transcriptional activity of AR (Fu, et al., 2006; Lavery and Bevan, 2011). Downregulation of SIRT2 deacetylase elevated the acetylated Lys 18 on H3, which decreased the sensitivity to ADT (Damodaran et al., 2017). Altogether, HATs and HDACs as co-regulators have been involved in the AR-mediated gene transcription.
Noncoding RNAs
Non-coding RNAs (ncRNAs) refer to RNAs that are not translated into proteins. According to their length, ncRNAs are divided into two categories: small ncRNAs are transcripts with a length of 18–200 nt, and long ncRNAs are those more than 200 nt. NcRNAs participate in epigenetic regulation directly or indirectly (Figure 5).
Long Non-Coding RNAs
LncRNAs primarily interact with mRNA, DNA, protein, and miRNA and consequently regulate gene expression through epigenetic mechanisms. LncRNAs repress gene transcription in collaboration with chromatin remodeling complexes or histone modifying enzymes. The polycomb repressive complexes PRC1 and PRC2 as the most common protein partners of lncRNAs, result in chromatin compaction. PRC2 is mainly composed of SUZ12, EED and EZH2. Among them, histone methyltransferase EZH2, responsible for H3K27 methylation, leads to gene silencing (Wei et al., 2017). Multiple lncRNAs bind to PRC2 and function in a PRC2-dependent manner. For example, HOTAIR interacts with PRC2 via its 5′ domain and regulates the epigenetic repression of PRC2 target genes (Rinn et al., 2007). Furthermore, HOTAIR contains another binding domain at the 3’ end for LSD1/CoREST, a histone deacetylase complex that facilitates transcriptional repression (Tsai et al., 2010). Similarly, in addition to PRC2 proteins, ANRIL also binds CBX7, a component of PRC1, then recruits PRC1 complexe to the INK4A/ARF locus to inhibit transcription (Yap et al., 2010).
LncRNAs have been involved in transcription activation. PRNCR1 and PCGEM1 bind successively to AR and enhance AR-mediated gene transcription in a ligand-dependent or independent manner (Yang et al., 2013). Linc00675 promotes AR signaling and PCa progression by two ways. Linc00675 binds to the NTD domain of AR protein, and competitively inhibits the binding of MDM2, thereby inhibits AR protein degradation through ubiquitin-proteasome pathways. On the other hand, linc00675 promotes AR signaling by binding and stabilizing GATA2 mRNA (Yao et al., 2020). Table 2 summarizes the lncRNAs involved in PCa.
Micro RNAs
miRNAs, by binding to the 3′ untranslated regions (UTR) of mRNA, repress gene expression. Aberrant miRNAs may be the outcome of epigenetic dysregulation, including CpG islands hypermethylation of promoter (Esteller, 2011). The methylation sensitive E2F transcription factors have been involved in miRNA regulation (Emmrich and Putzer, 2010). For example, the hypermethylation of CpG sites in promoters of miR-200c and miR-141 repress their expression. Conversely, upregulation of miR-34b repressed the expression of DNMTs and HDACs by directly targeting the 3′UTR of their mRNA, and partially induced demethylation and active chromatin modification (Majid et al., 2013).
miRNAs have emerged as the key regulators in diverse biological processes. miR-200 promotes epithelial mesenchymal transformation (EMT) by decreasing ZEB1 and ZEB2 (Park et al., 2008). miR-135a overexpression reduces PCa cell invasion and migration in vivo and in vitro by downregulating ROCK1 and ROCK2 (Kroiss et al., 2015).
The interaction between AR signaling and miRNAs contributes to the progression of PCa. AR binds to miR-21 promoter and increases its expression (Ribas et al., 2009). The upregulation of miR-21 inhibits the expression of transforming growth factor β receptor II (TGFBR2) by binding to its 3′UTR, thus attenuating TGFβ-mediated smad2/3 activation and cell growth inhibition in PCa (Mishra et al., 2014). In addition, AR directly binds to the AR binding site of the miR-135a and activates its transcription (Kroiss, et al., 2015).
Conversely, miRNAs repress AR gene expression. miR-31 directly binds to the mRNA of AR or other cell cycle regulatory genes and inhibit the proliferation of PCa cells (Lin et al., 2013). In addition, miR-34 and AR regulate each other in a feedback loop. miR-34 directly targets the 3’ UTR of AR mRNA and inhibits AR gene expression. In contrast, miR-34 is a direct target of p53 (He et al., 2007). AR acts upstream of p53 in PCa and activates the gene transcription of miR-34 in p53-dependent manner (Rokhlin et al., 2008).
In addition to direct regulation, miRNAs also indirectly regulate the gene expression and transcriptonal activity of AR. For example, miR-141 targets the 3′ UTR of Orphan receptor small heterodimer partner (SHP) mRNA, resulting in the translation inhibition and RNA degradation. SHP is a common suppressor of AR, which inhibits AR transcriptional activity. miR-141 is commonly elevated in PCa, thus indirectly promotes AR transcriptional activity (Xiao et al., 2012). In addition, MYC activates AR transcription by binding to the promoter. miR-let-7c inhibits AR gene expression and activity via targeting the 3’ UTR of MYC, which results in the degradation of MYC mRNA through RISC complex (Nadiminty et al., 2012). Table 3 summarizes the miRNAs involved in PCa.
Non-Coding RNAs and Liquid-Liquid Phase Separation
Liquid liquid phase separation (LLPS) is a biological phenomenon, which means that components with similar properties form droplets and condense in cells. The intracellular components form a reaction chamber through phase separation, so as to establish an environment in which multiple biological reactions occur at the same time (Shin and Brangwynne, 2017). NcRNAs play an important regulatory role in phase separation. NcRNAs contain different RNA domains, which are recognized and bound by specific RNA binding proteins (RBPs), which put together to form a dynamic network called phase separation droplets (Nozawa et al., 2020).
Modifications in LLPS result in a series of epigenetic disorders, then further promote tumorigenesis (Erdel and Rippe, 2018; Nozawa, et al., 2020). Biomolecular condensates are formed through LLPS, which separate and concentrate biomolecules with different physical and chemical properties. Small molecule anti-cancer drugs may be concentrated, and then selectively distributed into the condensate, so as to modify its pharmacodynamic characteristics (Klein et al., 2020). Such oncogenes as RAS and MYC, are considered as undruggable targets due to the lack of protein pockets, can be targeted by the condensates containing small molecules anti-cancer drugs (Dang et al., 2017). Transcriptional co-activators such as BRD4 and MED1 have been shown to form foci at superenhancer (SE) sites, showing the nature of liquid condensate. AR interacts with BRD4 and MED1, and forms foci in AR-positive PCa cells. AR rich foci formation show the characteristics of liquid-like condensate, which may be phase separated condensate. However, foci formation requires the full-length structure of AR, while the truncated ARs are not enough to induce lesions (Zhang et al., 2021). AR NTD containing multiple potential Speckle-type POZ protein (SPOP) interaction motifs ARE sufficient to drive the LLPS of AR (Bouchard et al., 2018). AR NTD form droplets at high concentration in vitro, and SPOP strongly promotes the formation of droplets (Bouchard, et al., 2018). In addition, studies have suggested that AR DBD is the main LLPS driver, which is the smallest region separated from RNA. Without a small amount of RNA, DBD could not drive LLPS alone, and only unstructured RNA make DBD drive LLPS (Ahmed et al., 2021). These studies show that AR drive LLPS to form phase condensate by different mechanisms. Since the unfolding mechanism of AR LLPS may be the key to cancer progression, it is very important to further study the mechanism details of AR LLPS.
Currently, the study of phase separation is far from mature, and many questions need be addressed. For example, how do ncRNAs regulate phase separation? how do ncRNAs regulate the pharmacodynamics of drugs through aggregates? and how do ncRNAs find the phase separation of more proteins? The resolution of these questions is critical to clarify the roles of ncRNAs in cancer progression, and further to identify ncRNAs as novel anti-cancer drug targets.
Epigenetic Regulators as Prostate Cancer Diagnostic Markers
Current methods of PCa diagnosis mainly test the levels of serum PSA. Nevertheless, PSA specificity testing alone leads to false positive results and unnecessary biopsies. Epigenetic regulators may become sensitive and specific diagnostic biomarkers for early PCa detection due to their advantages of stability and availability in body fluids. Epigenetic regulator biomarkers may avoid unnecessary biopsies and reduce the economic burden of high-cost overtreatment (Smith-Palmer et al., 2019).
Epigenetic Modifications of Key Genes as Biomarkers
Methylation of GSTP1 promoter is the most common epigenetic modification in PCa. GSTP1 promoter methylation was found in 90% of adenocarcinomas and 70% of high-grade and pre high-grade PCa niduses, but not in normal or hyperplastic prostatic epithelium (Nakayama et al., 2003). Hypermethylation of GSTP1 promoter can accurately distinguish whether a person has PCa or not, with a significant specificity of 89.5–100% and sensitivity of 21.4–86.3% in urine samples (Cairns et al., 2001). A PCa methylation assay (ProCaM™) assessing GSTP1, APC and RAR in urine has been validated in a multicenter prospective study in which urine samples from men with serum prostate PSA levels of 2.0–10.0 ng/ml were analyzed. This method can detect PCa more accurately than serum PSA (Baden et al., 2011).
Another kind of epigenetic modification that can distinguish normal tissue from PCa is histone modification. The levels of H4Ac, H3Ac, H3K4, H3k9me3, H3K9me2 and H3k4me1 in PCa are significantly decreased. H3K9me2 and H3Ac have almost 90% specificity and 80% sensitivity for distinguishing tumor tissue from non-malignant tissue (Santos et al., 2020).
Non-Coding RNAs as a Prostate Cancer Diagnostic Biomarker
Long Non-Coding RNAs
PCa tissues show increased expression of MALAT-1 compared with normal and benign prostatic hyperplasia tissues (Ren et al., 2012). Downregulation of MALAT-1 inhibited the growth of PCa cells and resulted in cell cycle arrest. Circulating MALAT-1 fragments (MD minirna) were better than PSA for predicting prostate biopsy results, indicating that MALAT-1 is a potential biomarker for PCa diagnosis (Wang et al., 2014).
Progensa™ PCA3 (Gen-Probe Inc., San Diego, CA, United States) has been approved by the United States FDA for detecting the levels of PCA3 in the urine of men over 50 years of age. In most prostate tumors, the level of PCA3 is 60–100 times higher than in adjacent non-tumor tissues, and is not detected in other types of tumors. Compared with serum PSA, the combination of urine PCA3 and fusion gene TMPRSS2-ERG significantly improved the specificity of PCa diagnosis and reduced unnecessary prostate biopsy (Salagierski and Schalken, 2012).
PCA3 is an ideal diagnostic marker, but is not a prognostic marker (Leyten et al., 2014). However, PCAT14 may be a diagnostic and prognostic biomarker, since it is highly expressed in non-malignant tumor tissues, while the absence of PCAT14 promotes proliferation and recurrence of cancer. Its expression inversely correlates with the degree of aggressiveness, and may be used as a conventional clinicopathologic risk factor for PCa prognosis. Expression of PCAT14 is correlated with better biochemical progression-free survival, metastasis-free survival, and PCa-specific survival (Shukla et al., 2016).
Another promising prognostic urine biomarker is lncRNA SChLAP1, which is readily detected in urine sediments by qPCR. The expression of SChLAP1 is specific to PCa and functions as independent risk factor for metastasis (Prensner et al., 2014).
Micro RNAs
miRNAs can be detected in serum, plasma and urine samples, and may become highly specific, stable and sensitive biomarkers for PCa diagnosis. Moya et al. reported that the levels of miR-98-5p, miR-152-3p, miR-326 and miR-4289 are higher in plasma samples of PCa patients than the healthy controls (Moya et al., 2019). The specificity and sensitivity of miR-17-3p and miR-1185-2-3p as a diagnostic tool is over 90% (Urabe et al., 2019). miR-182-5p was significantly upregulated in the tissues and plasma of PCa patients. miR-182-5p expression in PCa had an AUC in tissues of 0.81, a specificity in plasma of 77%, and an NPV of 99% (Bidarra et al., 2019).
Epigenetic Regulators as Therapeutic Targets for Drug Development
Epigenetic drugs have shown great anti-cancer potential in preclinical studies and clinical trials through different pathways. Recently, BET inhibitors targeting BRD4, a newly identified epigenetic molecule, demonstrated significant anti-cancer effects (Mahajan et al., 2017).
JQ1, an inhibitor of BET, inhibits the binding of BRD4 to AR enhancers, and thereby represses AR signaling and AR-regulated gene transcription (Lochrin et al., 2014). However BET inhibitors did not show satisfactory survival benefit for PCa patients in the clinical trials. In CRPC, EZH2 functions as a co-activator of AR to regulate gene transcription. PI3K/AKT phosphorylates EZH2 at S21 and reduces the H3K27me3 activity of EZH2 (Xu et al., 2012). Phosphorylated EZH2 interacts with AR and methylates AR at the sites of lysine 630 and 632, which increase its transcriptional activity (Deb et al., 2013; Xu, et al., 2012). EZH2 inhibitors alone or in combination with other inhibitors, such as NF-κB inhibitors, have demonstrated great therapeutic potential for PCa (Wu et al., 2016; Jin et al., 2021). EZH2 or AR inhibitors alone did not demonstrate significant anti-cancer efficacy, and easily induced drug resistance due to the reciprocal feedback activation loop (Shankar et al., 2020). However, the combination of EZH2 and AR inhibitors synergistically inhibited the growth of CRPC (Kim et al., 2018; Shankar, et al., 2020).
The histone methyltransferase inhibitor (HMTi) 3-dezaneplanocin-A (DZNeP) showed significant antitumor efficacy by inhibiting the activity of multiple histone methyltransferases including EZH2 (Miranda et al., 2009). LSD1, as a key histone demethytransferase, is upregulated in CRPC, and is highly and positively correlated with the prognosis of CRPC patients. LSD1 inhibitors have been developed (Metzger, et al., 2005; Yang et al., 2018), and demonstrated significant anti-cancer efficacy by inhibiting cancer cell proliferation, differentiation, invasion and migration (Fang et al., 2019).
HDAC molecules regulate gene expression by altering chromatin structure and regulating the functions of non-histone proteins (Chao and Goodman, 2014). Several HDAC inhibitors (HDACi) have been developed, and demonstrated anti-cancer potential by inducing cell cycle arrest, apoptosis and autophagy, and inhibiting the production of reactive oxygen species (ROS) (Rana et al., 2020). Inhibition of HDAC6 results in the hyperacetylation of HSP90, the loss of ATP binding, and the dissociation and degradation of AR (Rosati et al., 2016).
Additionally, natural compounds like anacardic acid, from cashew nut shell liquid, and a polyisoprenylated benzophenone derivative from the fruit rind of garcinia indica, known as garcinol, inhibit the HAT activity of both p300 and PCAF (Balasubramanyam et al., 2004). Small molecule synthetic inhibitors such as the γ-butyrolactone MB-3 are cell-permeable GCN5 inhibitor, and a series of isothiazolones have been found to inhibit p300 and PCAF activity (Biel et al., 2005). The epigenetic targets of flavonoids include oncogenes and tumor suppressor genes. They are indirectly regulated by epigenetic enzymes such as DNMTs, HATs and HDACs. As natural epigenetic regulators, they have been used for chemoprevention, which is a promising and effective strategy to inhibit carcinogenesis and progression (Izzo et al., 2020). Bioactive molecules in the diet, such as the edible plant Celtis sagittata, demonstrated remarkable anti-cancer efficacy by regulating epigenetic signaling pathways and altering tumor-associated immune response. These compounds have anticancer functions in various cancer types by specifically inhibiting the activities of DNMTs and HDACs (Pradhan et al., 2019). In addition, many epigenetic drugs have been tested in various stages of clinical trials (Table 4).
Conclusion
The androgen/AR signaling pathway is one of the most important drivers for PCa initiation and progression, as well as the transformation to CRPC. Therefore, the anti-androgen/AR drugs have become the first line therapy for PCa.
With a deeper understanding of PCa biology and epigenetics, additional epigenetic regulators critical for the development of PCa have been identified. Epigenetic regulators, individually or in combination with AR, promote PCa progression and have the potential to become novel PCa diagnostic markers and therapeutic targets. Although the development of epigenetic drugs is still in its infancy, undoubtedly, they will become increasingly important therapeutic tools for PCa, particularly for CRPC and neuroendocrine prostate cancer (NEPC) when used alone or in combination with anti-androgen/AR drugs.
Author Contributions
DTG designed the total frames of manuscript and tables of the manuscript, and wrote the sections of Introduction, DNA methylation and Conclusiont; YX wrote the sections of Androgen/AR pathways, epigenetic co-regulators, Epigenetic regulators as therapeutic targets doe drug development; YD wrote the section of Non-coding RNA and diagnostic and therapeutic markers; LX wrote the section of Histone methylation and drew Figure 1; XL designed the project, supervised the total process, and wrote the remaining sections of manuscript. All authors read and approved the final manuscript.
Funding
This work was supported by grants from the National Natural Science Foundation of China (NSFC, 81572542 and NSFC, 81874096). Research Project of Key Discipline of Guangdong Province (2019GDXK0010).
Conflict of Interest
The authors declare that the research was conducted in the absence of any commercial or financial relationships that could be construed as a potential conflict of interest.
Publisher’s Note
All claims expressed in this article are solely those of the authors and do not necessarily represent those of their affiliated organizations, or those of the publisher, the editors, and the reviewers. Any product that may be evaluated in this article, or claim that may be made by its manufacturer, is not guaranteed or endorsed by the publisher.
Acknowledgments
This study was supported by National Key Clinical Specialty Construction Project (Clinical Pharmacy) and High Level Clinical Key Specialty (Clinical Pharmacy) in Guangdong Province.
References
Aarnisalo, P., Palvimo, J. J., and Janne, O. A. (1998). CREB-binding Protein in Androgen Receptor-Mediated Signaling. Proc. Natl. Acad. Sci. 95 (95), 2122–2127. doi:10.1073/pnas.95.5.2122
Abbas, A., and Gupta, S. (2008). The Role of Histone Deacetylases in Prostate Cancer. Epigenetics 3, 300–309. doi:10.4161/epi.3.6.7273
Ahmed, J., Meszaros, A., Lazar, T., and Tompa, P. (2021). DNA‐binding Domain as the Minimal Region Driving RNA‐dependent Liquid-Liquid Phase Separation of Androgen Receptor. Protein Sci. : a Publ. Protein Soc. 30, 1380–1392. doi:10.1002/pro.4100
Albany, C., Alva, A. S., Aparicio, A. M., Singal, R., Yellapragada, S., Sonpavde, G., et al. (20112011). Epigenetics in Prostate Cancer. Prostate Cancer 2011, 1–12. doi:10.1155/2011/580318
Alen, P., Claessens, F., Verhoeven, G., Rombauts, W., and Peeters, B. (1999). The Androgen Receptor Amino-Terminal Domain Plays a Key Role in P160 Coactivator-Stimulated Gene Transcription. Mol. Cel Biol 19, 6085–6097. doi:10.1128/mcb.19.9.6085
Askew, E. B., Bai, S., Parris, A. B., Minges, J. T., and Wilson, E. M. (2017). Androgen Receptor Regulation by Histone Methyltransferase Suppressor of Variegation 3-9 Homolog 2 and Melanoma Antigen-A11. Mol. Cell. Endocrinol. 443, 42–51. doi:10.1016/j.mce.2016.12.028
Aurilio, G., Cimadamore, A., Mazzucchelli, R., Lopez-Beltran, A., Verri, E., Scarpelli, M., et al. (2020). Androgen Receptor Signaling Pathway in Prostate Cancer: From Genetics to Clinical Applications. Cells 9, 2653. doi:10.3390/cells9122653
Baden, J., Adams, S., Astacio, T., Jones, J., Markiewicz, J., Painter, J., et al. (2011). Predicting Prostate Biopsy Result in Men with Prostate Specific Antigen 2.0 to 10.0 Ng/ml Using an Investigational Prostate Cancer Methylation Assay. J. Urol. 186, 2101–2106. doi:10.1016/j.juro.2011.06.052
Balasubramanyam, K., Altaf, M., Varier, R. A., Swaminathan, V., Ravindran, A., Sadhale, P. P., et al. (2004). Polyisoprenylated Benzophenone, Garcinol, a Natural Histone Acetyltransferase Inhibitor, Represses Chromatin Transcription and Alters Global Gene Expression. J. Biol. Chem. 279, 33716–33726. doi:10.1074/jbc.M402839200
Baylin, S. B. (2005). DNA Methylation and Gene Silencing in Cancer. Nat. Rev. Clin. Oncol. 2 (2 Suppl. 1), S4–S11. doi:10.1038/ncponc0354
Belkina, A. C., and Denis, G. V. (2012). BET Domain Co-regulators in Obesity, Inflammation and Cancer. Nat. Rev. Cancer 12, 465–477. doi:10.1038/nrc3256
Bhatia, V., Yadav, A., Tiwari, R., Nigam, S., Goel, S., Carskadon, S., et al. (2019). Epigenetic Silencing of miRNA-338-5p and miRNA-421 Drives SPINK1-Positive Prostate Cancer. Clin. Cancer Res. 25, 2755–2768. doi:10.1158/1078-0432.CCR-18-3230
Bidarra, D., Constâncio, V., Barros-Silva, D., Ramalho-Carvalho, J., Moreira-Barbosa, C., Antunes, L., et al. (2019). Circulating MicroRNAs as Biomarkers for Prostate Cancer Detection and Metastasis Development Prediction. Front. Oncol. 9, 900. doi:10.3389/fonc.2019.00900
Biel, M., Wascholowski, V., and Giannis, A. (2005). Epigenetics-An Epicenter of Gene Regulation: Histones and Histone-Modifying Enzymes. Angew. Chem. Int. Ed. 44, 3186–3216. doi:10.1002/anie.200461346
Bouchard, J. J., Otero, J. H., Scott, D. C., Szulc, E., Martin, E. W., Sabri, N., et al. (2018). Cancer Mutations of the Tumor Suppressor SPOP Disrupt the Formation of Active, Phase-Separated Compartments. Mol. Cel 72 (72), 19–36. doi:10.1016/j.molcel.2018.08.027
Bray, F., Ferlay, J., Soerjomataram, I., Siegel, R. L., Torre, L. A., and Jemal, A. (2018). Global Cancer Statistics 2018: GLOBOCAN Estimates of Incidence and Mortality Worldwide for 36 Cancers in 185 Countries. CA: A Cancer J. Clinicians 68, 394–424. doi:10.3322/caac.21492
Cai, C., Yuan, X., and Balk, S. P. (2013). Androgen Receptor Epigenetics. Transl Androl. Urol. 2, 148–157. doi:10.3978/j.issn.2223-4683.2013.09.02
Cairns, P., Esteller, M., Herman, J. G., Schoenberg, M., Jeronimo, C., Sanchez-Cespedes, M., et al. (2001). Molecular Detection of Prostate Cancer in Urine by GSTP1 Hypermethylation. Clin. Cancer Res. 7, 2727–2730.
Callewaert, L., Van Tilborgh, N., and Claessens, F. (2006). Interplay between Two Hormone-independent Activation Domains in the Androgen Receptor. Cancer Res. 66, 543–553. doi:10.1158/0008-5472.CAN-05-2389
Cao, P., Deng, Z., Wan, M., Huang, W., Cramer, S. D., Xu, J., et al. (2010). MicroRNA-101 Negatively Regulates Ezh2 and its Expression Is Modulated by Androgen Receptor and HIF-1α/HIF-1β. Mol. Cancer 9, 108. doi:10.1186/1476-4598-9-108
Chakravarty, D., Sboner, A., Nair, S. S., Giannopoulou, E., Li, R., Hennig, S., et al. (2014). The Oestrogen Receptor Alpha-Regulated lncRNA NEAT1 Is a Critical Modulator of Prostate Cancer. Nat. Commun. 5 (5), 5383. doi:10.1038/ncomms6383
Chao, O. S., and Goodman, O. B. (2014). Synergistic Loss of Prostate Cancer Cell Viability by Coinhibition of HDAC and PARP. Mol. Cancer Res. 12, 1755–1766. doi:10.1158/1541-7786.MCR-14-0173
Chen, D., Ma, H., Hong, H., Koh, S. S., Huang, S.-M., Schurter, B. T., et al. (1999). Regulation of Transcription by a Protein Methyltransferase. Science 284, 2174–2177. doi:10.1126/science.284.5423.2174
Chng, K. R., Chang, C. W., Tan, S. K., Yang, C., Hong, S. Z., Sng, N. Y. W., et al. (2012). A Transcriptional Repressor Co-regulatory Network Governing Androgen Response in Prostate Cancers. EMBO J. 31, 2810–2823. doi:10.1038/emboj.2012.112
Chu, M., Chang, Y., Wang, N., Li, W., Li, P., and Gao, W.-Q. (2014). Hypermethylation-mediated Transcriptional Repression of TMPRSS2 in Androgen Receptor-Negative Prostate Cancer Cells. Exp. Biol. Med. (Maywood) 239, 823–828. doi:10.1177/1535370214531880
Clinckemalie, L., Vanderschueren, D., Boonen, S., and Claessens, F. (2012). The Hinge Region in Androgen Receptor Control. Mol. Cell. Endocrinol. 358, 1–8. doi:10.1016/j.mce.2012.02.019
Coffey, K., Rogerson, L., Ryan-Munden, C., Alkharaif, D., Stockley, J., Heer, R., et al. (2013). The Lysine Demethylase, KDM4B, Is a Key Molecule in Androgen Receptor Signalling and Turnover. Nucleic Acids Res. 41, 4433–4446. doi:10.1093/nar/gkt106
Dai, X., Liu, L., Liang, Z., Guo, K., Xu, S., and Wang, H. (2019). Silencing of lncRNA MALAT1 Inhibits Cell Cycle Progression via Androgen Receptor Signaling in Prostate Cancer Cells. Pathol. - Res. Pract. 215, 712–721. doi:10.1016/j.prp.2019.01.011
Damodaran, S., Damaschke, N., Gawdzik, J., Yang, B., Shi, C., Allen, G. O., et al. (2017). Dysregulation of Sirtuin 2 (SIRT2) and Histone H3K18 Acetylation Pathways Associates with Adverse Prostate Cancer Outcomes. BMC cancer 17, 874. doi:10.1186/s12885-017-3853-9
Dang, C. V., Reddy, E. P., Shokat, K. M., and Soucek, L. (2017). Drugging the 'undruggable' Cancer Targets. Nat. Rev. Cancer 17, 502–508. doi:10.1038/nrc.2017.36
Deb, G., Thakur, V. S., and Gupta, S. (2013). Multifaceted Role of EZH2 in Breast and Prostate Tumorigenesis. Epigenetics 8, 464–476. doi:10.4161/epi.24532
Dehm, S. M., and Tindall, D. J. (2007). Androgen Receptor Structural and Functional Elements: Role and Regulation in Prostate Cancer. Mol. Endocrinol. 21, 2855–2863. doi:10.1210/me.2007-0223
Ding, X. F., Anderson, C. M., Ma, H., Hong, H., Uht, R. M., Kushner, P. J., et al. (1998). Nuclear Receptor-Binding Sites of Coactivators Glucocorticoid Receptor Interacting Protein 1 (GRIP1) and Steroid Receptor Coactivator 1 (SRC-1): Multiple Motifs with Different Binding Specificities. Mol. Endocrinol. 12, 302–313. doi:10.1210/mend.12.2.0065
Dubbink, H. J., Hersmus, R., Pike, A. C. W., Molier, M., Brinkmann, A. O., Jenster, G., et al. (2006). Androgen Receptor Ligand-Binding Domain Interaction and Nuclear Receptor Specificity of FXXLF and LXXLL Motifs as Determined by L/F Swapping. Mol. Endocrinol. 20, 1742–1755. doi:10.1210/me.2005-0348
Eftekharzadeh, B., Banduseela, V. C., Chiesa, G., Martínez-Cristóbal, P., Rauch, J. N., Nath, S. R., et al. (2019). Hsp70 and Hsp40 Inhibit an Inter-domain Interaction Necessary for Transcriptional Activity in the Androgen Receptor. Nat. Commun. 10, 3562. doi:10.1038/s41467-019-11594-y
Emmrich, S., and Pützer, B. M. (2010). Checks and Balances: E2F-microRNA Crosstalk in Cancer Control. Cell cycle 9, 2555–2567. doi:10.4161/cc.9.13.12061
Erdel, F., and Rippe, K. (2018). Formation of Chromatin Subcompartments by Phase Separation. Biophysical J. 114, 2262–2270. doi:10.1016/j.bpj.2018.03.011
Esteller, M. (2011). Non-coding RNAs in Human Disease. Nat. Rev. Genet. 12 (12), 861–874. doi:10.1038/nrg3074
Fang, Y., Liao, G., and Yu, B. (2019). LSD1/KDM1A Inhibitors in Clinical Trials: Advances and Prospects. J. Hematol. Oncol. 12, 129. doi:10.1186/s13045-019-0811-9
Fang, Z., Xu, C., Li, Y., Cai, X., Ren, S., Liu, H., et al. (2016). A Feed-Forward Regulatory Loop between Androgen Receptor and PlncRNA-1 Promotes Prostate Cancer Progression. Cancer Lett. 374, 62–74. doi:10.1016/j.canlet.2016.01.033
Fletcher, C. E., Sulpice, E., Combe, S., Shibakawa, A., Leach, D. A., Hamilton, M. P., et al. (2019). Androgen Receptor-Modulatory microRNAs Provide Insight into Therapy Resistance and Therapeutic Targets in Advanced Prostate Cancer. Oncogene 38, 5700–5724. doi:10.1038/s41388-019-0823-5
Fu, M., Liu, M., Sauve, A. A., Jiao, X., Zhang, X., Wu, X., et al. (2006). Hormonal Control of Androgen Receptor Function through SIRT1. Mol. Cel Biol 26, 8122–8135. doi:10.1128/MCB.00289-06
Fu, M., Rao, M., Wang, C., Sakamaki, T., Wang, J., Di Vizio, D., et al. (2003). Acetylation of Androgen Receptor Enhances Coactivator Binding and Promotes Prostate Cancer Cell Growth. Mol. Cel Biol 23, 8563–8575. doi:10.1128/mcb.23.23.8563-8575.2003
Fu, M., Wang, C., Reutens, A. T., Wang, J., Angeletti, R. H., Siconolfi-Baez, L., et al. (2000). p300 and p300/cAMP-Response Element-Binding Protein-Associated Factor Acetylate the Androgen Receptor at Sites Governing Hormone-dependent Transactivation. J. Biol. Chem. 275, 20853–20860. doi:10.1074/jbc.M000660200
Gao, L., and Alumkal, J. (2010). Epigenetic Regulation of Androgen Receptor Signaling in Prostate Cancer. Epigenetics 5 (5), 100–104. doi:10.4161/epi.5.2.10778
Gaughan, L., Brady, M. E., Cook, S., Neal, D. E., and Robson, C. N. (2001). Tip60 Is a Co-activator Specific for Class I Nuclear Hormone Receptors. J. Biol. Chem. 276, 46841–46848. doi:10.1074/jbc.M103710200
Gaughan, L., Logan, I. R., Cook, S., Neal, D. E., and Robson, C. N. (2002). Tip60 and Histone Deacetylase 1 Regulate Androgen Receptor Activity through Changes to the Acetylation Status of the Receptor. J. Biol. Chem. 277, 25904–25913. doi:10.1074/jbc.M203423200
Graça, I., Pereira-Silva, E., Henrique, R., Packham, G., Crabb, S. J., and Jerónimo, C. (2016). Epigenetic Modulators as Therapeutic Targets in Prostate Cancer. Clin. Epigenet 8, 98. doi:10.1186/s13148-016-0264-8
Grunstein, M. (1997). Histone Acetylation in Chromatin Structure and Transcription. Nature 389, 349–352. doi:10.1038/38664
Gu, P., Chen, X., Xie, R., Han, J., Xie, W., Wang, B., et al. (2017). lncRNA HOXD-AS1 Regulates Proliferation and Chemo-Resistance of Castration-Resistant Prostate Cancer via Recruiting WDR5. Mol. Ther. 25, 1959–1973. doi:10.1016/j.ymthe.2017.04.016
Gu, P., Chen, X., Xie, R., Xie, W., Huang, L., Dong, W., et al. (2019). A Novel AR Translational Regulator lncRNA LBCS Inhibits Castration Resistance of Prostate Cancer. Mol. Cancer 18, 109. doi:10.1186/s12943-019-1037-8
Guo, H., Ahmed, M., Zhang, F., Yao, C. Q., Li, S., Liang, Y., et al. (2016). Modulation of Long Noncoding RNAs by Risk SNPs Underlying Genetic Predispositions to Prostate Cancer. Nat. Genet. 48, 1142–1150. doi:10.1038/ng.3637
Gurel, B., Iwata, T., M Koh, C., Jenkins, R. B., Lan, F., Van Dang, C., et al. (2008). Nuclear MYC Protein Overexpression Is an Early Alteration in Human Prostate Carcinogenesis. Mod. Pathol. 21, 1156–1167. doi:10.1038/modpathol.2008.111
He, B., Kemppainen, J. A., and Wilson, E. M. (2000). FXXLF and WXXLF Sequences Mediate the NH2-terminal Interaction with the Ligand Binding Domain of the Androgen Receptor. J. Biol. Chem. 275, 22986–22994. doi:10.1074/jbc.M002807200
He, L., He, X., Lim, L. P., de Stanchina, E., Xuan, Z., Liang, Y., et al. (2007). A microRNA Component of the P53 Tumour Suppressor Network. Nature 447, 1130–1134. doi:10.1038/nature05939
He, Y.-F., Li, B.-Z., Li, Z., Liu, P., Wang, Y., Tang, Q., et al. (2011). Tet-mediated Formation of 5-carboxylcytosine and its Excision by TDG in Mammalian DNA. Science 333, 1303–1307. doi:10.1126/science.1210944
He, Z., Shen, F., Qi, P., Zhai, Z., and Wang, Z. (2021). miR-541-3p Enhances the Radiosensitivity of Prostate Cancer Cells by Inhibiting HSP27 Expression and Downregulating β-catenin. Cell Death Discov. 7, 18. doi:10.1038/s41420-020-00387-8
Heery, D. M., Kalkhoven, E., Hoare, S., and Parker, M. G. (1997). A Signature Motif in Transcriptional Co-activators Mediates Binding to Nuclear Receptors. Nature 387, 733–736. doi:10.1038/42750
Hosohata, K., Li, P., Hosohata, Y., Qin, J., Roeder, R. G., and Wang, Z. (2003). Purification and Identification of a Novel Complex Which Is Involved in Androgen Receptor-dependent Transcription. Mol. Cel Biol 23, 7019–7029. doi:10.1128/mcb.23.19.7019-7029.2003
Huang, Q., Ma, B., Su, Y., Chan, K., Qu, H., Huang, J., et al. (2020). miR-197-3p Represses the Proliferation of Prostate Cancer by Regulating the VDAC1/AKT/β-catenin Signaling Axis. Int. J. Biol. Sci. 16, 1417–1426. doi:10.7150/ijbs.42019
Huang, W., Su, X., Yan, W., Kong, Z., Wang, D., Huang, Y., et al. (2018). Overexpression of AR-regulated lncRNA TMPO-AS1 Correlates with Tumor Progression and Poor Prognosis in Prostate Cancer. Prostate 78, 1248–1261. doi:10.1002/pros.23700
Huang, Z.-Q., Li, J., Sachs, L. M., Cole, P. A., and Wong, J. (2003). A Role for Cofactor-Cofactor and Cofactor-Histone Interactions in Targeting P300, SWI/SNF and Mediator for Transcription. EMBO J. 22, 2146–2155. doi:10.1093/emboj/cdg219
Hulf, T., Sibbritt, T., Wiklund, E. D., Patterson, K., Song, J. Z., Stirzaker, C., et al. (2013). Epigenetic-induced Repression of microRNA-205 Is Associated with MED1 Activation and a Poorer Prognosis in Localized Prostate Cancer. Oncogene 32, 2891–2899. doi:10.1038/onc.2012.300
Ito, S., Shen, L., Dai, Q., Wu, S. C., Collins, L. B., Swenberg, J. A., et al. (2011). Tet Proteins Can Convert 5-methylcytosine to 5-formylcytosine and 5-carboxylcytosine. Science 333, 1300–1303. doi:10.1126/science.1210597
Izzo, S., Naponelli, V., and Bettuzzi, S. (2020). Flavonoids as Epigenetic Modulators for Prostate Cancer Prevention. Nutrients 12, 1010. doi:10.3390/nu12041010
Jenster, G., Trapman, J., and Brinkmann, A. O. (1993). Nuclear Import of the Human Androgen Receptor. Biochem. J. 293 (Pt 3), 761–768. doi:10.1042/bj2930761
Jenster, G., van der Korput, H. A. G. M., Trapman, J., and Brinkmann, A. O. (1995). Identification of Two Transcription Activation Units in the N-Terminal Domain of the Human Androgen Receptor. J. Biol. Chem. 270, 7341–7346. doi:10.1074/jbc.270.13.7341
Jenster, G., van der Korput, J. A. G. M., Trapman, J., and Brinkmann, A. O. (1992). Functional Domains of the Human Androgen Receptor. J. Steroid Biochem. Mol. Biol. 41, 671–675. doi:10.1016/0960-0760(92)90402-5
Jeong, B.-C., Hong, C. Y., Chattopadhyay, S., Park, J. H., Gong, E.-Y., Kim, H.-J., et al. (2004). Androgen Receptor Corepressor-19 kDa (ARR19), a Leucine-Rich Protein that Represses the Transcriptional Activity of Androgen Receptor through Recruitment of Histone Deacetylase. Mol. Endocrinol. 18, 13–25. doi:10.1210/me.2003-0065
Jia, J., Li, F., Tang, X.-S., Xu, S., Gao, Y., Shi, Q., et al. (2016). Long Noncoding RNA DANCR Promotes Invasion of Prostate Cancer through Epigenetically Silencing Expression of TIMP2/3. Oncotarget 7, 37868–37881. doi:10.18632/oncotarget.9350
Jiang, Z., Zhang, Y., Chen, X., Wu, P., and Chen, D. (2020). Long Non‐coding RNA LINC00673 Silencing Inhibits Proliferation and Drug Resistance of Prostate Cancer Cells via Decreasing KLF4 Promoter Methylation. J. Cel Mol Med 24, 1878–1892. doi:10.1111/jcmm.14883
Jin, M., Duan, J., Liu, W., Ji, J., Liu, B., and Zhang, M. (2021). Feedback Activation of NF-KB Signaling Leads to Adaptive Resistance to EZH2 Inhibitors in Prostate Cancer Cells. Cancer Cel Int 21, 191. doi:10.1186/s12935-021-01897-w
Jozwik, K. M., and Carroll, J. S. (2012). Pioneer Factors in Hormone-dependent Cancers. Nat. Rev. Cancer 12, 381–385. doi:10.1038/nrc3263
Karvonen, U., Jänne, O. A., and Palvimo, J. J. (2006). Androgen Receptor Regulates Nuclear Trafficking and Nuclear Domain Residency of Corepressor HDAC7 in a Ligand-dependent Fashion. Exp. Cel. Res. 312, 3165–3183. doi:10.1016/j.yexcr.2006.06.018
Kawakami, J., Cowan, J. E., Elkin, E. P., Latini, D. M., DuChane, J., Carroll, P. R., et al. (2006). Androgen-deprivation Therapy as Primary Treatment for Localized Prostate Cancer. Cancer 106, 1708–1714. doi:10.1002/cncr.21799
Kim, J., Lee, Y., Lu, X., Song, B., Fong, K.-W., Cao, Q., et al. (2018). Polycomb- and Methylation-independent Roles of EZH2 as a Transcription Activator. Cel Rep. 25, 2808–2820. e2804. doi:10.1016/j.celrep.2018.11.035
Kirby, M., Hirst, C., and Crawford, E. D. (2011). Characterising the Castration-Resistant Prostate Cancer Population: a Systematic Review. Int. J. Clin. Pract. Nov 65, 1180–1192. doi:10.1111/j.1742-1241.2011.02799.x
Klein, I. A., Boija, A., Afeyan, L. K., Hawken, S. W., Fan, M., Dall'Agnese, A., et al. (2020). Partitioning of Cancer Therapeutics in Nuclear Condensates. Science 368, 1386–1392. doi:10.1126/science.aaz4427
Klokk, T. I., Kurys, P., Elbi, C., Nagaich, A. K., Hendarwanto, A., Slagsvold, T., et al. (2007). Ligand-Specific Dynamics of the Androgen Receptor at its Response Element in Living Cells. Mol. Cel Biol 27, 1823–1843. doi:10.1128/MCB.01297-06
Kroiss, A., Vincent, S., Decaussin-Petrucci, M., Meugnier, E., Viallet, J., Ruffion, A., et al. (2015). Androgen-regulated microRNA-135a Decreases Prostate Cancer Cell Migration and Invasion through Downregulating ROCK1 and ROCK2. Oncogene 34, 2846–2855. doi:10.1038/onc.2014.222
Lavery, D. N., and Bevan, C. L. (2011). Androgen Receptor Signalling in Prostate Cancer: the Functional Consequences of Acetylation. J. Biomed. Biotechnol. 2011, 862125. doi:10.1155/2011/862125
Leader, J. E., Wang, C., Fu, M., and Pestell, R. G. (2006). Epigenetic Regulation of Nuclear Steroid Receptors. Biochem. Pharmacol. 72 (72), 1589–1596. doi:10.1016/j.bcp.2006.05.024
Lee, D. Y., Northrop, J. P., Kuo, M.-H., and Stallcup, M. R. (2006). Histone H3 Lysine 9 Methyltransferase G9a Is a Transcriptional Coactivator for Nuclear Receptors. J. Biol. Chem. 281, 8476–8485. doi:10.1074/jbc.M511093200
Lee, Y. J., Oh, H., Kim, E., Ahn, B., Lee, J. H., Lee, Y., et al. (2019). Long Noncoding RNA HOTTIP Overexpression: A Potential Prognostic Biomarker in Prostate Cancer. Pathol. - Res. Pract. 215, 152649. doi:10.1016/j.prp.2019.152649
Lee, Y. S., Kim, H.-J., Lee, H. J., Lee, J. W., Chun, S.-Y., Ko, S.-K., et al. (2002). Activating Signal Cointegrator 1 Is Highly Expressed in Murine Testicular Leydig Cells and Enhances the Ligand-dependent Transactivation of Androgen Receptor1. Biol. Reprod. 67, 1580–1587. doi:10.1095/biolreprod.102.006155
Leyten, G. H. J. M., Hessels, D., Jannink, S. A., Smit, F. P., de Jong, H., Cornel, E. B., et al. (2014). Prospective Multicentre Evaluation of PCA3 and TMPRSS2-ERG Gene Fusions as Diagnostic and Prognostic Urinary Biomarkers for Prostate Cancer. Eur. Urol. 65, 534–542. doi:10.1016/j.eururo.2012.11.014
Li, H. Y., Jiang, F. Q., Chu, L., and Wei, X. (2020). Long Non-coding RNA BLACAT1 Inhibits Prostate Cancer Cell Proliferation through Sponging miR-361. Eur. Rev. Med. Pharmacol. Sci. 24, 74–85. doi:10.26355/eurrev_202001_19897
Li, Y., Li, Z., and Zhu, W.-G. (2019). Molecular Mechanisms of Epigenetic Regulators as Activatable Targets in Cancer Theranostics. Cmc 26, 1328–1350. doi:10.2174/0929867324666170921101947
Lin, P.-C., Chiu, Y.-L., Banerjee, S., Park, K., Mosquera, J. M., Giannopoulou, E., et al. (2013). Epigenetic Repression of miR-31 Disrupts Androgen Receptor Homeostasis and Contributes to Prostate Cancer Progression. Cancer Res. 73, 1232–1244. doi:10.1158/0008-5472.CAN-12-2968
Lin, Q., Cai, J., and Wang, Q.-Q. (2020). The Significance of Circular RNA DDX17 in Prostate Cancer. Biomed. Res. Int. 2020, 1–16. doi:10.1155/2020/1878431
Ling, Z., Wang, X., Tao, T., Zhang, L., Guan, H., You, Z., et al. (2017). Involvement of Aberrantly Activated HOTAIR/EZH2/miR-193a Feedback Loop in Progression of Prostate Cancer. J. Exp. Clin. Cancer Res. 36, 159. doi:10.1186/s13046-017-0629-7
Lingadahalli, S., Jadhao, S., Sung, Y. Y., Chen, M., Hu, L., Chen, X., et al. (2018). Novel lncRNA LINC00844 Regulates Prostate Cancer Cell Migration and Invasion through AR Signaling. Mol. Cancer Res. 16, 1865–1878. doi:10.1158/1541-7786.MCR-18-0087
Link, K. A., Balasubramaniam, S., Sharma, A., Comstock, C. E. S., Godoy-Tundidor, S., Powers, N., et al. (2008). Targeting the BAF57 SWI/SNF Subunit in Prostate Cancer: a Novel Platform to Control Androgen Receptor Activity. Cancer Res. 68, 4551–4558. doi:10.1158/0008-5472.CAN-07-6392
Liu, B., Sun, Y., Tang, M., Liang, C., Huang, C.-P., Niu, Y., et al. (2020). The miR-361-3p Increases Enzalutamide (Enz) Sensitivity via Targeting the ARv7 and MKNK2 to Better Suppress the Enz-Resistant Prostate Cancer. Cell Death Dis 11, 11807. doi:10.1038/s41419-020-02932-w
Liu, H.-t., Fang, L., Cheng, Y.-x., and Sun, Q. (2016). LncRNA PVT1 Regulates Prostate Cancer Cell Growth by Inducing the Methylation of miR-146a. Cancer Med. 5, 3512–3519. doi:10.1002/cam4.900
Lochrin, S. E., Price, D. K., and Figg, W. D. (2014). BET Bromodomain Inhibitors-A Novel Epigenetic Approach in Castration-Resistant Prostate Cancer. Cancer Biol. Ther. 15, 1583–1585. doi:10.4161/15384047.2014.962297
Luo, G., Liu, D., Huang, C., Wang, M., Xiao, X., Zeng, F., et al. (2017). LncRNA GAS5 Inhibits Cellular Proliferation by Targeting P27Kip1. Mol. Cancer Res. 15, 789–799. doi:10.1158/1541-7786.MCR-16-0331
Lynch, S. M., O'Neill, K. M., McKenna, M. M., Walsh, C. P., and McKenna, D. J. (2016). Regulation of miR-200c and miR-141 by Methylation in Prostate Cancer. Prostate 76, 1146–1159. doi:10.1002/pros.23201
Ma, H., Hong, H., Huang, S.-M., Irvine, R. A., Webb, P., Kushner, P. J., et al. (1999). Multiple Signal Input and Output Domains of the 160-kilodalton Nuclear Receptor Coactivator Proteins. Mol. Cel Biol 19, 6164–6173. doi:10.1128/mcb.19.9.6164
Mahajan, K., Malla, P., Lawrence, H. R., Chen, Z., Kumar-Sinha, C., Malik, R., et al. (2017). ACK1/TNK2 Regulates Histone H4 Tyr88-Phosphorylation and AR Gene Expression in Castration-Resistant Prostate Cancer. Cancer cell 31, 790–803. doi:10.1016/j.ccell.2017.05.003
Majid, S., Dar, A. A., Saini, S., Arora, S., Shahryari, V., Zaman, M. S., et al. (2012). miR-23b Represses Proto-Oncogene Src Kinase and Functions as Methylation-Silenced Tumor Suppressor with Diagnostic and Prognostic Significance in Prostate Cancer. Cancer Res. 72, 6435–6446. doi:10.1158/0008-5472.CAN-12-2181
Majid, S., Dar, A. A., Saini, S., Shahryari, V., Arora, S., Zaman, M. S., et al. (2013). miRNA-34b Inhibits Prostate Cancer through Demethylation, Active Chromatin Modifications, and AKT Pathways. Clin. Cancer Res. 19, 73–84. doi:10.1158/1078-0432.CCR-12-2952
Majumder, S., Liu, Y., Ford, O. H., Mohler, J. L., and Whang, Y. E. (2006). Involvement of Arginine Methyltransferase CARM1 in Androgen Receptor Function and Prostate Cancer Cell Viability. Prostate 66, 1292–1301. doi:10.1002/pros.20438
Mathis, D. J., Oudet, P., Wasylyk, B., and Chambon, P. (1978). Effect of Histone Acetylation on Structure and In Vitro Transcription of Chromatin. Nucl. Acids Res. 5, 3523–3548. doi:10.1093/nar/5.10.3523
Matias, P. M., Donner, P., Coelho, R., Thomaz, M., Peixoto, C., Macedo, S., et al. (2000). Structural Evidence for Ligand Specificity in the Binding Domain of the Human Androgen Receptor. J. Biol. Chem. 275, 26164–26171. doi:10.1074/jbc.M004571200
Mazzu, Y. Z., Yoshikawa, Y., Nandakumar, S., Chakraborty, G., Armenia, J., Jehane, L. E., et al. (2019). Methylation‐associated miR‐193b Silencing Activates Master Drivers of Aggressive Prostate Cancer. Mol. Oncol. 13, 1944–1958. doi:10.1002/1878-0261.12536
Mehra, R., Udager, A. M., Ahearn, T. U., Cao, X., Feng, F. Y., Loda, M., et al. (2016). Overexpression of the Long Non-coding RNA SChLAP1 Independently Predicts Lethal Prostate Cancer. Eur. Urol. 70, 549–552. doi:10.1016/j.eururo.2015.12.003
Meng, D., Yang, S., Wan, X., Zhang, Y., Huang, W., Zhao, P., et al. (2016). A Transcriptional Target of Androgen Receptor, miR-421 Regulates Proliferation and Metabolism of Prostate Cancer Cells. Int. J. Biochem. Cel Biol. 73, 30–40. doi:10.1016/j.biocel.2016.01.018
Messner, E. A., Steele, T. M., Tsamouri, M. M., Hejazi, N., Gao, A. C., Mudryj, M., et al. (2020). The Androgen Receptor in Prostate Cancer: Effect of Structure, Ligands and Spliced Variants on Therapy. Biomedicines 8, 422. doi:10.3390/biomedicines8100422
Metzger, E., Wissmann, M., Yin, N., Müller, J. M., Schneider, R., Peters, A. H. F. M., et al. (2005). LSD1 Demethylates Repressive Histone marks to Promote Androgen-receptor-dependent Transcription. Nature 437, 436–439. doi:10.1038/nature04020
Miranda, T. B., Cortez, C. C., Yoo, C. B., Liang, G., Abe, M., Kelly, T. K., et al. (2009). DZNep Is a Global Histone Methylation Inhibitor that Reactivates Developmental Genes Not Silenced by DNA Methylation. Mol. Cancer Ther. 8, 1579–1588. doi:10.1158/1535-7163.MCT-09-0013
Misawa, A., Takayama, K.-i., Urano, T., and Inoue, S. (2016). Androgen-induced Long Noncoding RNA (lncRNA) SOCS2-AS1 Promotes Cell Growth and Inhibits Apoptosis in Prostate Cancer Cells. J. Biol. Chem. 291, 17861–17880. doi:10.1074/jbc.M116.718536
Mishra, S., Deng, J. J., Gowda, P. S., Rao, M. K., Lin, C.-L., Chen, C. L., et al. (2014). Androgen Receptor and microRNA-21 axis Downregulates Transforming Growth Factor Beta Receptor II (TGFBR2) Expression in Prostate Cancer. Oncogene 33, 4097–4106. doi:10.1038/onc.2013.374
Moilanen, A.-M., Poukka, H., Karvonen, U., Häkli, M., Jänne, O. A., and Palvimo, J. J. (1998). Identification of a Novel RING finger Protein as a Coregulator in Steroid Receptor-Mediated Gene Transcription. Mol. Cel Biol 18, 5128–5139. doi:10.1128/MCB.18.9.5128
Moya, L., Meijer, J., Schubert, S., Matin, F., and Batra, J. (2019). Assessment of miR-98-5p, miR-152-3p, miR-326 and miR-4289 Expression as Biomarker for Prostate Cancer Diagnosis. Ijms 20, 1154. doi:10.3390/ijms20051154
Murata, T., Takayama, K., Katayama, S., Urano, T., Horie-Inoue, K., Ikeda, K., et al. (2010). miR-148a Is an Androgen-Responsive microRNA that Promotes LNCaP Prostate Cell Growth by Repressing its Target CAND1 Expression. Prostate Cancer Prostatic Dis. 13, 356–361. doi:10.1038/pcan.2010.32
Nadiminty, N., Tummala, R., Lou, W., Zhu, Y., Zhang, J., Chen, X., et al. (2012). MicroRNA Let-7c Suppresses Androgen Receptor Expression and Activity via Regulation of Myc Expression in Prostate Cancer Cells. J. Biol. Chem. 287, 1527–1537. doi:10.1074/jbc.M111.278705
Nakayama, M., Bennett, C. J., Hicks, J. L., Epstein, J. I., Platz, E. A., Nelson, W. G., et al. (2003). Hypermethylation of the Human Glutathione S-Transferase-π Gene (GSTP1) CpG Island Is Present in a Subset of Proliferative Inflammatory Atrophy Lesions but Not in Normal or Hyperplastic Epithelium of the Prostate. Am. J. Pathol. 163, 923–933. doi:10.1016/s0002-9440(10)63452-9
Nakazawa, M., Antonarakis, E. S., and Luo, J. (2014). Androgen Receptor Splice Variants in the Era of Enzalutamide and Abiraterone. Horm. Canc 5, 265–273. doi:10.1007/s12672-014-0190-1
Narayanan, R., Coss, C. C., and Dalton, J. T. (2018). Development of Selective Androgen Receptor Modulators (SARMs). Mol. Cell. Endocrinol. 465, 134–142. doi:10.1016/j.mce.2017.06.013
Nelson, W. G., De Marzo, A. M., and Yegnasubramanian, S. (2009). Minireview: Epigenetic Alterations in Human Prostate Cancers. Endocrinology 150, 3991–4002. doi:10.1210/en.2009-0573
Nozawa, R. S., Yamamoto, T., Takahashi, M., Tachiwana, H., Maruyama, R., Hirota, T., et al. (2020). Nuclear Microenvironment in Cancer: Control through Liquid‐liquid Phase Separation. Cancer Sci. 111, 3155–3163. doi:10.1111/cas.14551
Okano, M., Bell, D. W., Haber, D. A., and Li, E. (1999). DNA Methyltransferases Dnmt3a and Dnmt3b Are Essential for De Novo Methylation and Mammalian Development. Cell 99, 247–257. doi:10.1016/s0092-8674(00)81656-6
Park, S.-M., Gaur, A. B., Lengyel, E., and Peter, M. E. (2008). The miR-200 Family Determines the Epithelial Phenotype of Cancer Cells by Targeting the E-Cadherin Repressors ZEB1 and ZEB2. Genes Dev. 22 (22), 894–907. doi:10.1101/gad.1640608
Parolia, A., Crea, F., Xue, H., Wang, Y., Mo, F., Ramnarine, V. R., et al. (2015). The Long Non-coding RNA PCGEM1 Is Regulated by Androgen Receptor Activity In Vivo. Mol. Cancer 14, 1446. doi:10.1186/s12943-015-0314-4
Pradhan, N., Parbin, S., Kausar, C., Kar, S., Mawatwal, S., Das, L., et al. (2019). Paederia Foetida Induces Anticancer Activity by Modulating Chromatin Modification Enzymes and Altering Pro-inflammatory Cytokine Gene Expression in Human Prostate Cancer Cells. Food Chem. Toxicol. 130, 161–173. doi:10.1016/j.fct.2019.05.016
Prensner, J. R., Zhao, S., Erho, N., Schipper, M., Iyer, M. K., Dhanasekaran, S. M., et al. (2014). RNA Biomarkers Associated with Metastatic Progression in Prostate Cancer: a Multi-Institutional High-Throughput Analysis of SChLAP1. Lancet Oncol. 15, 1469–1480. doi:10.1016/S1470-2045(14)71113-1
Ramalho-Carvalho, J., Martins, J. B., Cekaite, L., Sveen, A., Torres-Ferreira, J., Graça, I., et al. (2017). Epigenetic Disruption of miR-130a Promotes Prostate Cancer by Targeting SEC23B and DEPDC1. Cancer Lett. 385, 150–159. doi:10.1016/j.canlet.2016.10.028
Ramberg, H., Alshbib, A., Berge, V., Svindland, A., and Taskén, K. A. (2011). Regulation of PBX3 Expression by Androgen and Let-7d in Prostate Cancer. Mol. Cancer 10, 50. doi:10.1186/1476-4598-10-50
Rana, Z., Diermeier, S., Hanif, M., and Rosengren, R. J. (2020). Understanding Failure and Improving Treatment Using HDAC Inhibitors for Prostate Cancer. Biomedicines 8, 22. doi:10.3390/biomedicines8020022
Rayasam, G. V., Wendling, O., Angrand, P. O., Mark, M., Niederreither, K., Song, L., et al. (2003). NSD1 Is Essential for Early post-implantation Development and Has a Catalytically Active SET Domain. EMBO J. 22, 3153–3163. doi:10.1093/emboj/cdg288
Ren, S., Peng, Z., Mao, J.-H., Yu, Y., Yin, C., Gao, X., et al. (2012). RNA-seq Analysis of Prostate Cancer in the Chinese Population Identifies Recurrent Gene Fusions, Cancer-Associated Long Noncoding RNAs and Aberrant Alternative Splicings. Cell Res 22, 806–821. doi:10.1038/cr.2012.30
Ribas, J., Ni, X., Haffner, M., Wentzel, E. A., Salmasi, A. H., Chowdhury, W. H., et al. (2009). miR-21: an Androgen Receptor-Regulated microRNA that Promotes Hormone-dependent and Hormone-independent Prostate Cancer Growth. Cancer Res. 69, 7165–7169. doi:10.1158/0008-5472.CAN-09-1448
Rinn, J. L., Kertesz, M., Wang, J. K., Squazzo, S. L., Xu, X., Brugmann, S. A., et al. (2007). Functional Demarcation of Active and Silent Chromatin Domains in Human HOX Loci by Noncoding RNAs. Cell 129, 1311–1323. doi:10.1016/j.cell.2007.05.022
Rokhlin, O. W., Scheinker, V. S., Taghiyev, A. F., Bumcrot, D., Glover, R. A., and Cohen, M. B. (2008). MicroRNA-34 Mediates AR-dependent P53-Induced Apoptosis in Prostate Cancer. Cancer Biol. Ther. 7, 1288–1296. doi:10.4161/cbt.7.8.6284
Rosati, R., Chen, B., Patki, M., McFall, T., Ou, S., Heath, E., et al. (2016). Hybrid Enzalutamide Derivatives with Histone Deacetylase Inhibitor Activity Decrease Heat Shock Protein 90 and Androgen Receptor Levels and Inhibit Viability in Enzalutamide-Resistant C4-2 Prostate Cancer Cells. Mol. Pharmacol. 90, 225–237. doi:10.1124/mol.116.103416
Roy, A. K., Tyagi, R. K., Song, C. S., Lavrovsky, Y., Ahn, S. C., Oh, T.-S., et al. (2001). Androgen Receptor: Structural Domains and Functional Dynamics after Ligand-Receptor Interaction. Ann. N. Y Acad. Sci. Dec 949, 44–57. doi:10.1111/j.1749-6632.2001.tb04001.x
Ruggero, K., Farran-Matas, S., Martinez-Tebar, A., and Aytes, A. (2018). Epigenetic Regulation in Prostate Cancer Progression. Curr. Mol. Bio Rep. 4, 101–115. doi:10.1007/s40610-018-0095-9
Sakurai, K., Reon, B. J., Anaya, J., and Dutta, A. (2015). The lncRNA DRAIC/PCAT29 Locus Constitutes a Tumor-Suppressive Nexus. Mol. Cancer Res. 13, 828–838. doi:10.1158/1541-7786.MCR-15-0016-T
Salagierski, M., and Schalken, J. A. (2012). Molecular Diagnosis of Prostate Cancer: PCA3 and TMPRSS2:ERG Gene Fusion. J. Urol. 187, 795–801. doi:10.1016/j.juro.2011.10.133
Santos, P. B., Patel, H., Henrique, R., and Félix, A. (2020). Can Epigenetic and Inflammatory Biomarkers Identify Clinically Aggressive Prostate Cancer? Wjco 11 (11), 43–52. doi:10.5306/wjco.v11.i2.43
Sato, S., Katsushima, K., Shinjo, K., Hatanaka, A., Ohka, F., Suzuki, S., et al. (2016). Histone Deacetylase Inhibition in Prostate Cancer Triggers miR-320-Mediated Suppression of the Androgen Receptor. Cancer Res. 76, 4192–4204. doi:10.1158/0008-5472.CAN-15-3339
Schulz, W. A., and Hoffmann, M. J. (2009). Epigenetic Mechanisms in the Biology of Prostate Cancer. Semin. Cancer Biol. 19, 172–180. doi:10.1016/j.semcancer.2009.02.006
Shaffer, P. L., Jivan, A., Dollins, D. E., Claessens, F., and Gewirth, D. T. (2004). Structural Basis of Androgen Receptor Binding to Selective Androgen Response Elements. Proc. Natl. Acad. Sci. 101, 4758–4763. doi:10.1073/pnas.0401123101
Shang, Z., Yu, J., Sun, L., Tian, J., Zhu, S., Zhang, B., et al. (2019). LncRNA PCAT1 Activates AKT and NF-Κb Signaling in Castration-Resistant Prostate Cancer by Regulating the PHLPP/FKBP51/IKKα Complex. Nucleic Acids Res. 47, 4211–4225. doi:10.1093/nar/gkz108
Shankar, E., Franco, D., Iqbal, O., Moreton, S., Kanwal, R., and Gupta, S. (2020). Dual Targeting of EZH2 and Androgen Receptor as a Novel Therapy for Castration-Resistant Prostate Cancer. Toxicol. Appl. Pharmacol. 404, 115200. doi:10.1016/j.taap.2020.115200
Sharma, M., Zarnegar, M., Li, X., Lim, B., and Sun, Z. (2000). Androgen Receptor Interacts with a Novel MYST Protein, HBO1. J. Biol. Chem. 275, 35200–35208. doi:10.1074/jbc.M004838200
Shin, Y., and Brangwynne, C. P. (2017). Liquid Phase Condensation in Cell Physiology and Disease. Science 357, 357. doi:10.1126/science.aaf4382
Shukla, S., Zhang, X., Niknafs, Y. S., Xiao, L., Mehra, R., Cieślik, M., et al. (2016). Identification and Validation of PCAT14 as Prognostic Biomarker in Prostate Cancer. Neoplasia 18, 489–499. doi:10.1016/j.neo.2016.07.001
Sikand, K., Slaibi, J. E., Singh, R., Slane, S. D., and Shukla, G. C. (2011). miR 488* Inhibits Androgen Receptor Expression in Prostate Carcinoma Cells. Int. J. Cancer 129, 810–819. doi:10.1002/ijc.25753
Smith-Palmer, J., Takizawa, C., and Valentine, W. (2019). Literature Review of the burden of Prostate Cancer in Germany, France, the United Kingdom and Canada. BMC Urol. 19, 19. doi:10.1186/s12894-019-0448-6
Su, W., Xu, M., Chen, X., Chen, N., Gong, J., Nie, L., et al. (2017). Long Noncoding RNA ZEB1-AS1 Epigenetically Regulates the Expressions of ZEB1 and Downstream Molecules in Prostate Cancer. Mol. Cancer 16, 142. doi:10.1186/s12943-017-0711-y
Tahiliani, M., Koh, K. P., Shen, Y., Pastor, W. A., Bandukwala, H., Brudno, Y., et al. (2009). Conversion of 5-methylcytosine to 5-hydroxymethylcytosine in Mammalian DNA by MLL Partner TET1. Science 324, 930–935. doi:10.1126/science.1170116
Takayama, K.-i., Fujimura, T., Suzuki, Y., and Inoue, S. (2020). Identification of Long Non-coding RNAs in Advanced Prostate Cancer Associated with Androgen Receptor Splicing Factors. Commun. Biol. 3 (3), 393. doi:10.1038/s42003-020-01120-y
Takayama, K.-i., Horie-Inoue, K., Katayama, S., Suzuki, T., Tsutsumi, S., Ikeda, K., et al. (2013). Androgen-responsive Long Noncoding RNA CTBP1-AS Promotes Prostate Cancer. Embo J. 32, 1665–1680. doi:10.1038/emboj.2013.99
Takayama, K.-i., Misawa, A., Suzuki, T., Takagi, K., Hayashizaki, Y., Fujimura, T., et al. (2015). TET2 Repression by Androgen Hormone Regulates Global Hydroxymethylation Status and Prostate Cancer Progression. Nat. Commun. 6, 8219. doi:10.1038/ncomms9219
Tan, M. E., Li, J., Xu, H. E., Melcher, K., and Yong, E.-l. (2015). Androgen Receptor: Structure, Role in Prostate Cancer and Drug Discovery. Acta Pharmacol. Sin 36, 3–23. doi:10.1038/aps.2014.18
Tomlins, S. A., Mehra, R., Rhodes, D. R., Smith, L. R., Roulston, D., Helgeson, B. E., et al. (2006). TMPRSS2:ETV4 Gene Fusions Define a Third Molecular Subtype of Prostate Cancer. Cancer Res. 66, 3396–3400. doi:10.1158/0008-5472.CAN-06-0168
Tomlins, S. A., Rhodes, D. R., Perner, S., Dhanasekaran, S. M., Mehra, R., Sun, X.-W., et al. (2005). Recurrent Fusion of TMPRSS2 and ETS Transcription Factor Genes in Prostate Cancer. Science 310, 644–648. doi:10.1126/science.1117679
Tong, D., Liu, Q., Liu, G., Yuan, W., Wang, L., Guo, Y., et al. (2016). The HIF/PHF8/AR axis Promotes Prostate Cancer Progression. Oncogenesis 5, e283. doi:10.1038/oncsis.2016.74
Tsai, M.-C., Manor, O., Wan, Y., Mosammaparast, N., Wang, J. K., Lan, F., et al. (2010). Long Noncoding RNA as Modular Scaffold of Histone Modification Complexes. Science 329, 689–693. doi:10.1126/science.1192002
Urabe, F., Matsuzaki, J., Yamamoto, Y., Kimura, T., Hara, T., Ichikawa, M., et al. (2019). Large-scale Circulating microRNA Profiling for the Liquid Biopsy of Prostate Cancer. Clin. Cancer Res. 25, 3016–3025. doi:10.1158/1078-0432.CCR-18-2849
Valdez, C. D., Davis, J. N., Odeh, H. M., Layfield, T. L., Cousineau, C. S., Berton, T. R., et al. (2011). Repression of Androgen Receptor Transcription through the E2F1/DNMT1 axis. PloS one 6, e25187. doi:10.1371/journal.pone.0025187
Verrijdt, G., Haelens, A., and Claessens, F. (2003). Selective DNA Recognition by the Androgen Receptor as a Mechanism for Hormone-specific Regulation of Gene Expression. Mol. Genet. Metab. 78, 175–185. doi:10.1016/s1096-7192(03)00003-9
Wang, F., Ren, S., Chen, R., Lu, J., Shi, X., Zhu, Y., et al. (2014). Development and Prospective Multicenter Evaluation of the Long Noncoding RNA MALAT-1 as a Diagnostic Urinary Biomarker for Prostate Cancer. Oncotarget 5, 11091–11102. doi:10.18632/oncotarget.2691
Wang, H., Huang, Z.-Q., Xia, L., Feng, Q., Erdjument-Bromage, H., Strahl, B. D., et al. (2001a). Methylation of Histone H4 at Arginine 3 Facilitating Transcriptional Activation by Nuclear Hormone Receptor. Science 293, 853–857. doi:10.1126/science.1060781
Wang, Q., Lu, J., and Yong, E. L. (2001b). Ligand- and Coactivator-Mediated Transactivation Function (AF2) of the Androgen Receptor Ligand-Binding Domain Is Inhibited by the Cognate Hinge Region. J. Biol. Chem. 276, 7493–7499. doi:10.1074/jbc.M009916200
Wei, J.-W., Huang, K., Yang, C., and Kang, C.-S. (2017). Non-coding RNAs as Regulators in Epigenetics. Oncol. Rep. Jan 37, 3–9. doi:10.3892/or.2016.5236
Weinhold, B. (2006). Epigenetics: the Science of Change. Environ. Health Perspect. 114, A160–A167. doi:10.1289/ehp.114-a160
Wissmann, M., Yin, N., Müller, J. M., Greschik, H., Fodor, B. D., Jenuwein, T., et al. (2007). Cooperative Demethylation by JMJD2C and LSD1 Promotes Androgen Receptor-dependent Gene Expression. Nat. Cel Biol 9, 347–353. doi:10.1038/ncb1546
Wu, C., Jin, X., Yang, J., Yang, Y., He, Y., Ding, L., et al. (2016). Inhibition of EZH2 by Chemo- and Radiotherapy Agents and Small Molecule Inhibitors Induces Cell Death in Castration-Resistant Prostate Cancer. Oncotarget 7 (7), 3440–3452. doi:10.18632/oncotarget.6497
Xiang, Y., Zhu, Z., Han, G., Ye, X., Xu, B., Peng, Z., et al. (2007). JARID1B Is a Histone H3 Lysine 4 Demethylase Up-Regulated in Prostate Cancer. Proc. Natl. Acad. Sci. 104, 19226–19231. doi:10.1073/pnas.0700735104
Xiao, J., Gong, A.-Y., Eischeid, A. N., Chen, D., Deng, C., Young, C. Y. F., et al. (2012). miR-141 Modulates Androgen Receptor Transcriptional Activity in Human Prostate Cancer Cells through Targeting the Small Heterodimer Partner Protein. Prostate 72, 1514–1522. doi:10.1002/pros.22501
Xu, K., and Liao, Y. (2019). Epigenetic Regulation of Prostate Cancer: the Theories and the Clinical Implications. Asian J. Androl. 21, 279–290. doi:10.4103/aja.aja_53_18
Xu, K., Wu, Z. J., Groner, A. C., He, H. H., Cai, C., Lis, R. T., et al. (2012). EZH2 Oncogenic Activity in Castration-Resistant Prostate Cancer Cells Is Polycomb-independent. Science 338, 1465–1469. doi:10.1126/science.1227604
Yamane, K., Toumazou, C., Tsukada, Y.-i., Erdjument-Bromage, H., Tempst, P., Wong, J., et al. (2006). JHDM2A, a JmjC-Containing H3K9 Demethylase, Facilitates Transcription Activation by Androgen Receptor. Cell 125, 483–495. doi:10.1016/j.cell.2006.03.027
Yang, G.-J., Lei, P.-M., Wong, S.-Y., Ma, D.-L., and Leung, C.-H. (2018). Pharmacological Inhibition of LSD1 for Cancer Treatment. Molecules 23, 3194. doi:10.3390/molecules23123194
Yang, L., Lin, C., Jin, C., Yang, J. C., Tanasa, B., Li, W., et al. (2013). lncRNA-dependent Mechanisms of Androgen-Receptor-Regulated Gene Activation Programs. Nature 500, 598–602. doi:10.1038/nature124511
Yao, M., Shi, X., Li, Y., Xiao, Y., Butler, W., Huang, Y., et al. (2020). LINC00675 Activates Androgen Receptor axis Signaling Pathway to Promote Castration-Resistant Prostate Cancer Progression. Cel Death Dis 11, 638. doi:10.1038/s41419-020-02856-5
Yap, K. L., Li, S., Muñoz-Cabello, A. M., Raguz, S., Zeng, L., Mujtaba, S., et al. (2010). Molecular Interplay of the Noncoding RNA ANRIL and Methylated Histone H3 Lysine 27 by Polycomb CBX7 in Transcriptional Silencing of INK4a. Mol. Cel 38, 662–674. doi:10.1016/j.molcel.2010.03.021
Yegnasubramanian, S. (2016). Prostate Cancer Epigenetics and its Clinical Implications. Asian J. Androl. 18, 549–558. doi:10.4103/1008-682X.179859
Yu, X., Yi, P., Hamilton, R. A., Shen, H., Chen, M., Foulds, C. E., et al. (2020). Structural Insights of Transcriptionally Active, Full-Length Androgen Receptor Coactivator Complexes. Mol. Cel 79, 812–823. doi:10.1016/j.molcel.2020.06.031
Zaman, M. S., Chen, Y., Deng, G., Shahryari, V., Suh, S. O., Saini, S., et al. (2010). The Functional Significance of microRNA-145 in Prostate Cancer. Br. J. Cancer 103, 256–264. doi:10.1038/sj.bjc.6605742
Zhai, W., Sun, Y., Guo, C., Hu, G., Wang, M., Zheng, J., et al. (2017). LncRNA-SARCC Suppresses Renal Cell Carcinoma (RCC) Progression via Altering the Androgen Receptor(AR)/miRNA-143-3p Signals. Cell Death Differ 24, 1502–1517. doi:10.1038/cdd.2017.74
Zhang, A., Zhao, J. C., Kim, J., Fong, K.-W., Yang, Y. A., Chakravarti, D., et al. (2015). LncRNA HOTAIR Enhances the Androgen-Receptor-Mediated Transcriptional Program and Drives Castration-Resistant Prostate Cancer. Cel Rep. 13 (13), 209–221. doi:10.1016/j.celrep.2015.08.069
Zhang, F., Wong, S., Lee, J., Lingadahalli, S., Wells, C., Saxena, N., et al. (2021). Dynamic Phase Separation of the Androgen Receptor and its Coactivators to Regulate Gene Expression. bioRxiv, 437301. doi:10.1101/2021.03.27.437301
Zhang, Y., Pitchiaya, S., Cieślik, M., Niknafs, Y. S., Tien, J. C.-Y., Hosono, Y., et al. (2018). Analysis of the Androgen Receptor-Regulated lncRNA Landscape Identifies a Role for ARLNC1 in Prostate Cancer Progression. Nat. Genet. 50, 814–824. doi:10.1038/s41588-018-0120-1
Zhou, Y., Yang, H., Xia, W., Cui, L., Xu, R., Lu, H., et al. (2020). LncRNA MEG3 Inhibits the Progression of Prostate Cancer by Facilitating H3K27 Trimethylation of EN2 through Binding to EZH2. J. Biochem. Mar. 1 167, 295–301. doi:10.1093/jb/mvz097
Zhou, Z. X., Sar, M., Simental, J. A., Lane, M. V., and Wilson, E. M. (1994). A Ligand-dependent Bipartite Nuclear Targeting Signal in the Human Androgen Receptor. Requirement for the DNA-Binding Domain and Modulation by NH2-terminal and Carboxyl-Terminal Sequences. J. Biol. Chem. 269, 13115–13123. doi:10.1016/s0021-9258(17)36806-0
Keywords: prostate cancer, androgen receptor, epigenetic regulators, diagnostic markers, therapeutic targets
Citation: Deng T, Xiao Y, Dai Y, Xie L and Li X (2021) Roles of Key Epigenetic Regulators in the Gene Transcription and Progression of Prostate Cancer. Front. Mol. Biosci. 8:743376. doi: 10.3389/fmolb.2021.743376
Received: 18 July 2021; Accepted: 25 November 2021;
Published: 15 December 2021.
Edited by:
Jing Liu, Central South University, ChinaReviewed by:
Olga S. Sokolova, Lomonosov Moscow State University, RussiaDavid Y Lee, University of New Mexico, United States
Copyright © 2021 Deng, Xiao, Dai, Xie and Li. This is an open-access article distributed under the terms of the Creative Commons Attribution License (CC BY). The use, distribution or reproduction in other forums is permitted, provided the original author(s) and the copyright owner(s) are credited and that the original publication in this journal is cited, in accordance with accepted academic practice. No use, distribution or reproduction is permitted which does not comply with these terms.
*Correspondence: Xiong Li, lixiong@gdpu.edu.cn