- 1Instituto de Biología Funcional y Genómica, Consejo Superior de Investigaciones Científicas, Universidad de Salamanca, Salamanca, Spain
- 2Departamento de Biología Experimental-Genética, Universidad de Jaén, Jaén, Spain
- 3Centro de Estudios Avanzados en Aceite de Oliva y Olivar, Universidad de Jaén, Jaén, Spain
Evolutionarily conserved kinases and phosphatases regulate RNA polymerase II (RNAPII) transcript synthesis by modifying the phosphorylation status of the carboxyl-terminal domain (CTD) of Rpb1, the largest subunit of RNAPII. Proper levels of Rpb1-CTD phosphorylation are required for RNA co-transcriptional processing and to coordinate transcription with other nuclear processes, such as chromatin remodeling and histone modification. Whether other RNAPII subunits are phosphorylated and influences their role in gene expression is still an unanswered question. Much less is known about RNAPI and RNAPIII phosphorylation, whose subunits do not contain functional CTDs. However, diverse studies have reported that several RNAPI and RNAPIII subunits are susceptible to phosphorylation. Some of these phosphorylation sites are distributed within subunits common to all three RNAPs whereas others are only shared between RNAPI and RNAPIII. This suggests that the activities of all RNAPs might be finely modulated by phosphorylation events and raises the idea of a tight coordination between the three RNAPs. Supporting this view, the transcription by all RNAPs is regulated by signaling pathways that sense different environmental cues to adapt a global RNA transcriptional response. This review focuses on how the phosphorylation of RNAPs might regulate their function and we comment on the regulation by phosphorylation of some key transcription factors in the case of RNAPI and RNAPIII. Finally, we discuss the existence of possible common mechanisms that could coordinate their activities.
Introduction
The transcription of cellular RNAs is carried out by DNA-dependent RNA polymerases (RNAPs). In bacteria and archaea, only one RNAP transcribes all RNAs. In Eukarya, three RNAPs (RNAPI, -II and -III) are required for RNA transcription, except plants containing two other RNAPs (RNAPIV and -V). RNAPI synthesizes the precursor ribosomal RNA (rRNA 35S in yeast, 47S in mammals), RNAPIII produces 5S rRNA and transfer RNAs (tRNAs) and RNAPII transcribes all the protein-coding genes synthesizing messenger RNAs (mRNAs). Additionally, RNAPII and RNAPIII can synthesize other types of transcripts, such as small non-coding RNAs (ncRNAs), whose specific synthesis may differ depending on the species (Huet et al., 1985; Dieci et al., 2007). Finally, RNAPIV and RNAPV produce small interfering (siRNAs) and ncRNAs in plants (Onodera et al., 2005; Zhang et al., 2007; Haag and Pikaard, 2011; Lopez et al., 2011; Wang and Ma, 2015). All RNAPs are related at the evolutionary level, displaying common structures and functions. The minimum preserved structure of RNAPs is that of bacteria, consisting of five subunits. Archaeal RNAP has 12 subunits and eukaryotic RNAPs are complexes of 12 (RNAPII), 14 (RNAPI) and 17 (RNAPIII) subunits (Cramer et al., 2008; Werner and Grohmann, 2011; Wang and Ma, 2015; Cramer, 2019b). They all have a structurally conserved core formed by 10 subunits, with additional factors located on the polymerase complex periphery. Moreover, they all share five subunits (Rpb5, Rpb6, Rpb8, Rpb10, and Rpb12) with common functions but also with specific roles in their corresponding RNAPs (Cramer et al., 2008; Cuevas-Bermúdez et al., 2017). The structures of the three eukaryotic RNAPs, first solved in Saccharomyces cerevisiae, are highly conserved and their resolution has tremendously helped to understand the mechanism of transcription (Cramer et al., 2000; Armache et al., 2005; Engel et al., 2013; Fernandez-Tornero et al., 2013; Hoffmann et al., 2015; Sainsbury et al., 2015; Ramsay et al., 2020; Schier and Taatjes, 2020). The correct regulation of gene transcription depends on mechanisms that regulate the formation of large multiprotein complexes (RNAPs and their cognate factors) and their dynamics through all the transcription process. One of the most prominent mechanisms is post-translational modification (PTM) of proteins (Deribe et al., 2010), phosphorylation being the most frequent (Beltrao et al., 2013). A clear example is the dynamic phosphorylation of the carboxyl-terminal domain (CTD) of Rpb1, key for gene transcription (Buratowski, 2009; Calvo and García, 2012; Hsin and Manley, 2012; Eick and Geyer, 2013; Harlen and Churchman, 2017). Unfortunately, while most of the available data refer mainly to the phospho-regulation of transcription factors implicated in the modulation of all RNAP activities, little is known about the phosphorylation of other RNAP subunits and their implications in RNA biogenesis. Here, we have compiled all the phospho-sites identified to date for S. cerevisiae and human RNAPs (Supplementary Tables S1, S2). We discuss the localization and possible roles of the three RNAP subunit phosphorylations in budding yeast, as the structures of the different transcription complexes are better known in this organism. Finally, we review the possible conservation of RNAP phospho-regulation with evolution.
RNAPII Phosphorylation
RNAPII is the best known of the eukaryotic RNA polymerases. Transcription by RNAPII is a very complex, dynamic and finely regulated process. A sophisticated network of protein–protein and protein–nucleic acid interactions is established, producing conformational and activity changes in RNAPII through the transcription cycle. Thus, a pre-initiation complex (PIC), composed basically of general transcription factors (GTFs: TFIIA, B, D, E, F, and H), Mediator and RNAPII, is assembled at the gene promoters, opening the DNA to initiate transcription (Greber and Nogales, 2019; Schier and Taatjes, 2020). Other factors acting as activators/co-activators and repressors/co-repressors can modulate the transcription activity (Ho and Shuman, 1999; Thomas and Chiang, 2006; Hahn and Young, 2011; Roeder, 2019). Subsequently, RNAPII activity is regulated by elongation and termination factors (Kwak and Lis, 2013). Because pre-mRNA maturation (capping, splicing and polyadenylation) occurs co-transcriptionally, a set of processing factors also interacts with the transcription machinery. Moreover, chromatin and histone modifiers act to facilitate and regulate the passage of RNAPII through the genes being transcribed in concert with the transcription complex. It is well known that the correct orchestration of all these processes involved in mRNA biogenesis is coordinated and fine-tuned by the phosphorylation status of the Rpb1-CTD (Perales and Bentley, 2009; Calvo and García, 2012; Hsin and Manley, 2012; Harlen and Churchman, 2017).
Functional and structural studies with S. cerevisiae have provided the majority of the existing knowledge about RNAPII transcription mechanisms, regulation and coordination with other cellular processes (Cramer, 2019a; Cramer, 2019b; Roeder, 2019). Recent structural data combined with functional studies have advanced our understanding of RNAPII transcription in general and that of PIC function, structure and dynamics in particular (Greber and Nogales, 2019; Schier and Taatjes, 2020). Resolution of the RNAPII structure by X-ray crystallography about 20 years ago showed that its twelve subunits are folded and assembled into four mobile modules: the core module, formed by the active center (Rpb1 and Rpb2) and assembly platform (Rpb3, Rpb10, Rpb11, and Rpb12); the jaw-lobe module, made up of Rpb1 and Rpb9; the shelf module containing the foot and cleft domains of Rpb1 and the lower jaw and assembly domains of Rpb5; and the stalk module, formed by Rpb4 and Rpb7, which in the case of S. cerevisiae can be dissociated from the 10-subunit core polymerase (Cramer et al., 2000; Cramer et al., 2001; Gnatt et al., 2001; Armache et al., 2003; Bushnell and Kornberg, 2003). Within these modules there are some key structural domains with basic roles in transcription, such as the active site, cleft, clamp, wall, protrusion, funnel and RNA exit channel. Movement of these regions is accompanied by binding of the GTFs with essential roles in transcription initiation. Subsequent binding of elongation factors replaces the GTFs, thus regulating further steps of the transcription cycle. How all these events take place is not fully understood, although some are explained by conformational changes of the transcription complex and/or phosphorylation of specific factors and the Rpb1-CTD (Wang et al., 2010; Larochelle et al., 2012; He et al., 2013; Sainsbury et al., 2015; He et al., 2016; Harlen and Churchman, 2017; Nogales et al., 2017; Greber and Nogales, 2019; Nogales and Greber, 2019; Patel et al., 2019; Schier and Taatjes, 2020).
The Rpb1-CTD is an unstructured and flexible domain that is crucial for the regulation of RNAPII transcription. It consists of multiple repeats of the heptapeptide sequence Tyr1-Ser2-Pro3-Thr4-Ser5-Pro6-Ser7, which is not present in other RNAPs. It is evolutionarily well conserved from protozoa to metazoa. The number of repeats ranges from 26 repetitions in S. cerevisiae to 52 in mammals (Corden et al., 1985; Chapman et al., 2008). Five of the seven residues are susceptible to phosphorylation: Tyr1; Ser2, -5, and -7; and Thr4 (Buratowski, 2003; Hsin et al., 2011; Hsin and Manley, 2012; Allepuz-Fuster et al., 2014; Yurko and Manley, 2018). Prior to transcription initiation, the Rpb1-CTD likely interacts with Mediator and helps to recruit it to the promoter (Kim et al., 1994; Naar et al., 2002; Robinson et al., 2016). During initiation, the CTD becomes phosphorylated by transcription-associated kinases, generating phospho-marks required for the binding of elongation and RNA processing factors, among others, and to proceed to a productive elongation phase. As this subject has been extensively reviewed (Perales and Bentley, 2009; Calvo and García, 2012; Hsin and Manley, 2012; Harlen and Churchman, 2017), we will focus on this section on the phosphorylations of other RNAPII subunits (Supplementary Table S1), although in most cases a role in transcription and/or RNA processing has not been yet stated.
Several phospho-proteomic studies have identified at least 75 new phospho-sites in 10 of the 12 subunits of S. cerevisiae RNAPII (Supplementary Table S1), 55 of them distributed along specific RNAPII subunits (Rpb1, Rpb2, Rpb3 Rpb4, and Rpb9) and 20 in shared subunits with RNAPI and RNAPIII (Rpb5, Rpb6, Rpb8, Rpb10, and Rpb12) (Albuquerque et al., 2008; Pultz et al., 2012; Swaney et al., 2013; Sostaric et al., 2018; MacGilvray et al., 2020; Lanz et al., 2021; Richard et al., 2021). However, it is unknown whether all these residues are phosphorylated in vivo and if they form part of a regulatory mechanism to control the biogenesis of RNAPII transcripts. Localization of these residues on the RNAPII structure (Figure 1A, upper panel) shows a broad distribution, with 50 of the 75 phospho-sites localized in structured regions. Notably, many of them correspond to defined RNAPII regions, suggesting that post-translational modifications by phosphorylation may influence how these specific regions act during the transcription steps (i.e., DNA contact, NTP addition, clamp movement, etc.). It is worth noting that 39 phospho-sites are exposed on the surface of the enzyme (Figure 1A, bottom panel, and Figure 1C). Thus, it is tempting to speculate that the phosphorylation of these residues might be important for protein–protein interaction between RNAPII and different transcriptional regulators. In fact, these exposed residues localize in regions that are described to contact the GTFs (TFIIB, TBP, TFIIA, TFIIE, TFIIF, TFIIH or TFIIS; Figure 1B), Mediator and elongation factor Spt5/4 (Cai et al., 2010; Martinez-Rucobo et al., 2011; Nogales et al., 2017; Greber and Nogales, 2019; Schier and Taatjes, 2020). Interestingly, there are three phospho-sites (S1793, T1471, and Y1473) within the Rpb1 linker, an unstructured region that connects the CTD to the rest of the protein, whose phosphorylation is required for Spt6 interaction with RNAPII and the re-assembly of repressive chromatin during transcription (Sdano et al., 2017).
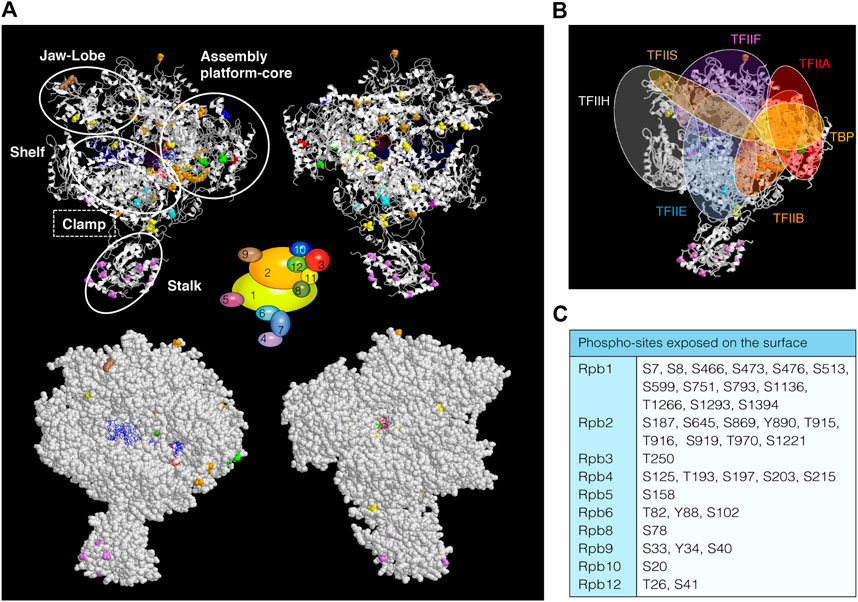
FIGURE 1. RNAPII phospho-sites. (A) Upper, schematic views (ribbon representation) of Saccharomyces cerevisiae RNAPII (PDB: 1y1w), displaying phospho-sites that have been labelled in different colours according to the 12 subunits diagram shown in the middle of the figures. RNAPII mobile modules are indicated with white open circles. DNA is represented in blue and RNA in red. Surface (bottom) views showing exposed phospho-sites. (B) Schematic representation of GTFs localizations according to published works (i.e., (Sainsbury et al., 2015; Schier and Taatjes, 2020)). (C) Table with phospho-sites exposed on the surface of RNAPII whose phosphorylation status could be important for the association/dissociation of transcription regulators.
Rpb2 phosphorylation sites lie in the external 1, protrusion, fork, wall, hybrid binding and anchor domains. In the wall, near the active site, the RNA:DNA hybrid separates and upstream DNA makes a 90° turn to exit RNAPII (Cramer et al., 2001). The protrusion is an external, positively charged domain, placed above the wall where the DNA exits from the cleft. Re-annealing of transcribed DNA occurs as it exits the enzyme, and the protrusion may participate in this process. Therefore, phosphorylation of residues lying in these domains could be involved in the separation of the RNA:DNA hybrid, the re-annealing of the transcribed DNA as it exits the enzyme and/or in the association/dissociation of transcription and processing factors (Pappas and Hampsey, 2000). Another example is the association and function of TFIIB and TFIIF during transcription initiation, factors important to position the DNA over the RNAPII active center cleft (Sainsbury et al., 2015; Greber and Nogales, 2019; Schier and Taatjes, 2020). Indeed, TFIIB interacts with the clamp, with the dock and cleft (Rpb1), and with the wall and protrusion domains (Rpb2) (Kostrewa et al., 2009; Liu et al., 2010; Sainsbury et al., 2015). TFIIF binds upstream and downstream DNA and RNAPII near the Rpb2 lobe and protrusion domains (Muhlbacher et al., 2014; Plaschka et al., 2015). Again, phosphorylation of residues in TFIIB and TFIIF binding regions could be involved in their association with the RNAPII.
Rpb4 and Rpb7 form a heterodimer known as the stalk domain, and only Rpb4 contains phosphorylation sites (Richard et al., 2021). The stalk extends from the foot domain at the base of the RNAPII enzyme and its movement helps to coordinate opening and closing of the clamp (Armache et al., 2003; Bushnell and Kornberg, 2003). It is contacted by initiation and elongation factors (Cai et al., 2010; Martinez-Rucobo et al., 2011; Li et al., 2014; Plaschka et al., 2015; Greber and Nogales, 2019; Schier and Taatjes, 2020). Rpb4 contains several phospho-sites whose phosphorylation may be important for interaction with Rpb7 and/or the 10-subunit polymerase. Accordingly, the Rpb4 S125 residue resides within a region exclusively present in S. cerevisiae that could regulate specific functions in this organism, such as dissociation of Rpb4/7 from the core polymerase (Sharma and Kumari, 2013; Duek et al., 2018). Moreover, exposed residues could mediate the association of different factors (Babbarwal et al., 2014; Garavis et al., 2017; Allepuz-Fuster et al., 2019; Calvo, 2020), depending on their phosphorylation status, such as TFIIE, TFIIF, Mediator (Cai et al., 2010; Sainsbury et al., 2015), Spt5/4 (Martinez-Rucobo et al., 2011; Li et al., 2014) some CTD phosphatases (Kimura et al., 2002; Allepuz-Fuster et al., 2014), and termination factors (Mitsuzawa et al., 2003; Runner et al., 2008), and thus regulate the function of Rpb4/7. Similarly to Rpb4/7, Rpb3 forms a heterodimer with Rpb11 (Cramer et al., 2001). Moreover, some phospho-sites fall in a region comprising the heterodimerization domain of Rpb3. This suggests that phosphorylation of this region might be important for the formation of the heterodimer. Rpb9 phosphorylated residues localized in the jaw and linker domains (Cramer et al., 2001) and, because TFIIH interacts with RNAPII near the Rpb9 jaw, we could speculate that modification of these residues could be functionally linked to this factor (Muhlbacher et al., 2014; Plaschka et al., 2015).
The subunits shared by the three RNAPs (Rpb5, Rpb6, Rpb8, Rpb10, and Rpb12) are also phospho-proteins (Supplementary Table S1) (Albuquerque et al., 2008; Swaney et al., 2013; Sostaric et al., 2018; MacGilvray et al., 2020; Lanz et al., 2021). For instance, Rpb5 and Rpb6 contain phospho-sites (S158, and Y88 and T82, respectively) localized in regions important for Rpb5 and Rpb6 assembly to RNAPII (Cramer et al., 2001; Tan et al., 2003; Zaros et al., 2007).
Modification by phosphorylation of some residues of RNAPII subunits could be important not only for the association of different factors along the transcription cycle but also for exchange of factors occupying the same or close surfaces on RNAPII. This is the case of initiation and elongation factors that compete during the transcription cycle for binding to the polymerase complex, for instance TFIIE and Spt5 (Li et al., 2014). How these mutually exclusive interactions of the transcription factors with RNAPII are regulated without affecting the efficiency of all the transcription steps (initiation, pausing and elongation) remains to be understood. Recently, it has been shown that the Rpb1-CTD undergoes liquid-phase separation, which could explain the association of initiation and elongation factors (Boehning et al., 2018; Cramer, 2019b). First, a dynamic condensate is formed near the promoter during initiation that contains a non-phosphorylated RNAPII and initiation factors. This condensate facilitates transcription initiation, RNA synthesis and Rpb1-CTD phosphorylation. Second, a transient condensate containing phosphorylated RNAPII and elongation factors is produced and maintained until RNAPII reaches the end of the genes, where RNAPII is dephosphorylated, recycled and transferred to the first condensate. As the transfer of RNAPII from one condensate to another is controlled by CTD phosphorylation, it is possible that this mechanism might be crucial for optimal transcriptional regulation (Boehning et al., 2018; Guo et al., 2019; Peng et al., 2020). However, we cannot rule out that the exchange of factors during the initiation/elongation transition could be regulated by the phosphorylation of other RNAPII subunits and/or even that the transfer of RNAPII between both condensates could require the post-translational modification of additional subunits.
Finally, the high sequence conservation within the RNAPII core between yeast and humans suggests similar mechanisms of RNA synthesis. However, sequences are more divergent toward the exterior/surface residues, suggesting that biochemically distinct interfaces interact with different factors (Cramer et al., 2001; He et al., 2013; He et al., 2016; Nogales et al., 2017; Schier and Taatjes, 2020). Accordingly, phospho-sites localized in the surface of RNAPII may contribute to the association/dissociation of species-specific factors.
RNAPI Phosphorylation
Initially, 15 phospho-sites were identified in S. cerevisiae distributed to five of the 14 subunits. Mutation of 13 of these phospho-sites indicated that most are non-essential PTMs, suggesting that they might contribute to non-essential RNAPI functions. Only one residue, Rpa190-S685, was suggested to play a role in rRNA cleavage/elongation or termination (Gerber et al., 2008). To date, 115 site-specific phosphorylations have been identified, mostly in phospho-proteomic studies, distributed along all the 14 RNAPI subunits (Ficarro et al., 2002; Albuquerque et al., 2008; Holt et al., 2009; Soulard et al., 2010; Pultz et al., 2012; Swaney et al., 2013; Sostaric et al., 2018; MacGilvray et al., 2020; Lanz et al., 2021). We have compiled all these sites in Supplementary Table S1. In summary, 81 sites reside in specific subunits and 34 are shared: 20 with RNAPII and RNAPIII and 14 with RNAPIII. Among these 81 phospho-sites, 63 are localized in regions of solved structure (Figure 2A, upper panel). Remarkably, 49 sites are exposed on the surface of the enzyme (Figure 2A, bottom panel, and Figure 2C), which again suggests a role for the association of RNAPI with transcription regulators, for instance Rrn3 (Figure 2B) (Torreira et al., 2017).
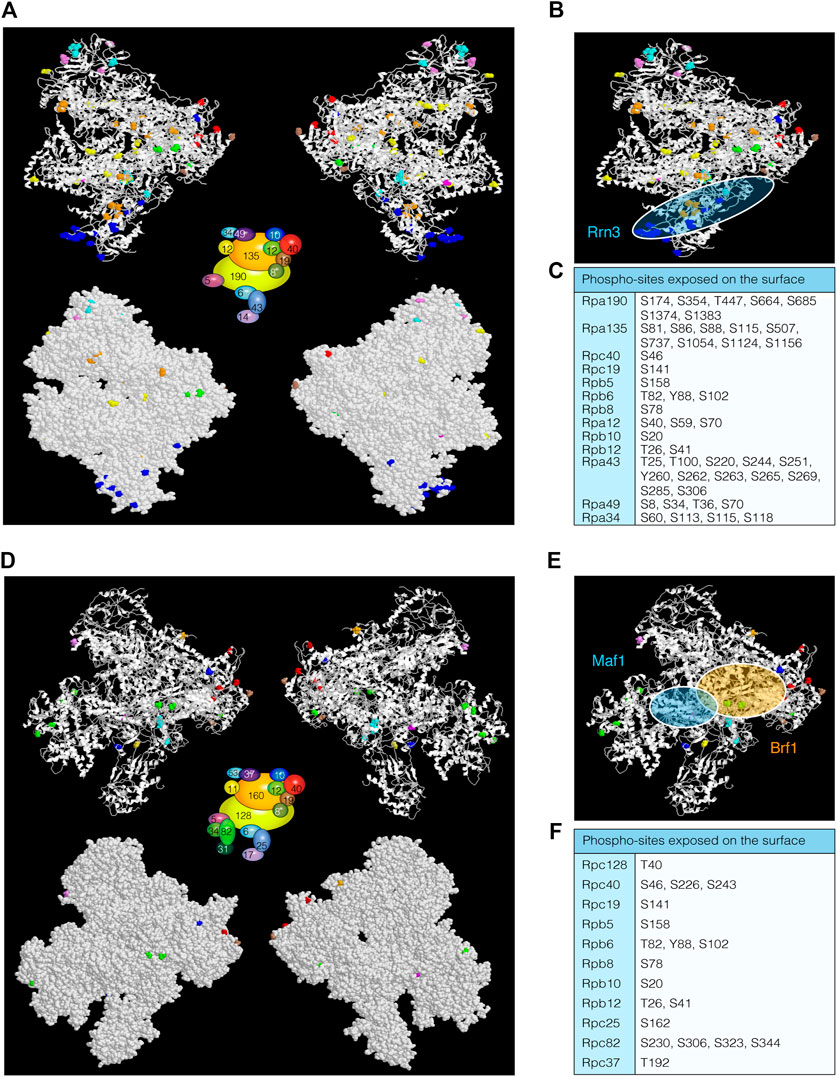
FIGURE 2. RNAPI and RNAPIII phospho-sites. (A) Ribbon (upper) and surface (bottom) schematic views of RNAPI (PDB: 4c3h) from Saccharomyces cerevisiae, displaying phospho-sites labelled in different colours according to the subunit diagram shown in the middle of the figures. (B) Schematic representation of Rrn3 localization (Torreira et al., 2017). (C) Table with phospho-sites exposed on the surface of RNAPI. (D) Ribbon (upper) and surface (bottom) representations of RNAPIII (PDB: 5fj9) displaying coloured phospho-sites. (E) Schematic representation of Maf1 and Brf1 associations with RNAPIII (Vorlander et al., 2020a; Vorlander et al., 2020b). (F) Table with RNAPIII phospho-sites exposed on the surface. As in the case of RNAPII, these residues could be important for the interaction with transcription regulators.
One of the first observations implicating protein phosphorylation in regulating RNAPI activity was the discovery that Fcp1, a Rpb1-CTD phosphatase, interacted with the RNAPI transcription machinery and was essential for rDNA efficient transcription, probably by facilitating RNAPI dephosphorylation and chain elongation during rRNA synthesis (Fath et al., 2004). Later, it was documented that the Rpa43 subunit was phosphorylated in several specific residues (S208, S220, S262, S263, S285) (Gerber et al., 2008). This subunit, together with Rpa14, forms the stalk domain and creates a platform for binding initiation factors and newly synthesized RNA (Fernandez-Tornero et al., 2013; Torreira et al., 2017). This stalk domain is required for RNAPI homodimerization and transcription inactivation (Torreira et al., 2017). However, it is unknown if Rpa43 phosphorylation levels play a role in this process. Nonetheless, it was reported that Cdc14 dephosphorylates Rpa43 in mitosis to exclude it from the nucleolus, thereby restraining rDNA transcription and facilitating condensin loading, an essential step for correct segregation of the nucleolus (Clemente-Blanco et al., 2009).
Rpa43 interacts with Rrn3 (Milkereit and Tschochner, 1998; Moorefield et al., 2000), a crucial RNAPI factor whose phosphorylation has been implicated in the regulation of the holoenzyme, after activation of growth factor signaling pathways that connect nutrient availability and rDNA production. Rrn3 is the yeast homologue of the mammalian growth-dependent rRNA synthesis factor TIF-IA (Grummt and Voit, 2010). This interaction depends on the phosphorylation of RNAPI and on Rrn3-P/TIF-IA association, and is essential to establish a competent transcriptional initiation complex (Fath et al., 2001; Cavanaugh et al., 2002; Torreira et al., 2017). Interestingly, in mice, casein kinase 2 (C1K2) has been implicated in Rrn3/TIF-IA phosphorylation at S170/172 to trigger its release from the RNAPI complex after transcription initiation, a prerequisite for transcription elongation (Bierhoff et al., 2008). This suggests that Rrn3/TIF-IA is subjected to a complex phospho-code that regulates its interaction with the RNAPI holoenzyme during ribosome biogenesis. Importantly, human RNAPI activity is also controlled in response to different types of environmental stresses throughout the phosphorylation of Rrn3/TIF-IA. Under glucose restriction, Rrn3/TIF-IA phosphorylation by the AMPK kinase prevents the assembly of a functional PIC (Hoppe et al., 2009). On the other hand, Rrn3/TIF-IA phosphorylation by the JNK kinase in mice restrains its interaction with RNAPI in response to oxidative stress, thus abrogating the formation of new PICs (Mayer et al., 2005).
RNAPIII Phosphorylation
In terms of structural composition, RNAPIII is the largest eukaryotic RNA polymerase complex in mass and molecular conformation (Vannini and Cramer, 2012). It is formed by 17 subunits, 10 of which are unique to RNAPIII. Novel phospho-proteomic studies have shed light on the post-transcriptional phospho-mapping of multiple RNAPIII subunits (Albuquerque et al., 2008; Holt et al., 2009; Swaney et al., 2013; MacGilvray et al., 2020; Lanz et al., 2021). Fifteen of the 17 subunits are phosphorylated in both yeast and humans (Supplementary Table S1, S2). In the case of S. cerevisiae, there are 76 phospho-sites, 42 of them localized in specific subunits. Only 28 residues are localized in regions of known structure (Figure 2D) and 19 are exposed on the surface of the polymerase complex (Figure 2D, bottom, and Figure 2F). Three specific residues of Rpc53 (S224, T228 and T232) are of known function (see below) (Lee et al., 2012). Therefore, it is intuitive to think that RNAPIII activity might also be highly regulated by phosphorylation events, as described for RNAPI and RNAPII complexes. Interestingly, in S. cerevisiae only two phospho-sites in the two largest subunits have been shown to be phosphorylated, whereas 32 phospho-sites distributed along these subunits have been identified in humans (Supplementary Table S2). This observation suggests that regulation of RNAPIII activity by phosphorylation could be species specific.
Probably the best-known regulator of RNAPIII is the repressor Maf1 (Willis and Moir, 2018; Vorlander et al., 2020a), whose activity is controlled by its phosphorylation at multiple sites by protein kinase A, the rapamycin-sensitive TOR kinase (TORC1) and the TORC1-regulated kinase Sch9. Phosphorylation of Maf1 by these kinases leads to changes in its subcellular localization, a mechanism that ensures the accurate activation/repression of RNAPIII (Moir et al., 2006; Lee et al., 2009; Wei and Zheng, 2009; Willis and Moir, 2018). Additionally, casein kinase 2 (CK2) phosphorylation of Maf1 in favorable growth conditions releases this protein from the RNAPIII complex bound to genes for tRNAs, thus activating their transcription (Graczyk et al., 2011). Maf1 regulation also depends on protein phosphatases. It has been postulated that in response to nutrient starvation, poor carbon sources or several cellular stresses, Maf1 is dephosphorylated in a PP4/PP2A-dependent manner and translocated to the nucleus, thus repressing RNAPIII activity (Oler and Cairns, 2012; Ahn et al., 2019). Interestingly, nuclear localization of Maf1 is not enough to completely inhibit RNAPIII activity, suggesting the existence of alternative mechanisms that co-regulate RNAPIII transcription under these conditions (Huber et al., 2009). In agreement with this observation, recent studies have demonstrated that the RNAPIII subunit Rpc53 is also subjected to a phosphorylation switch in response to nutrient limitation and other types of cellular stress. Rpc53 phosphorylation by the two conserved kinases Kns1 and Mck1 modifies the ability of RNAPIII to interact with the DNA molecule, thus avoiding recycling rounds of transcription and allowing dephosphorylated Maf1 to join and inhibit RNAPIII activity (Lee et al., 2012). Another component of RNAPIII controlled by phosphorylation is its Rpc82 subunit, whose concomitant phosphorylation with the TFIIIB subunit Bdp1 by the Sch9 and CK2 kinases opposes Maf1-mediated transcriptional repression (Lee et al., 2015). Finally, it has been reported that the TATA-binding protein (TBP) is also a preferred substrate of CK2 in vitro, which suggests a new mechanism to regulate RNAPIII transcription by phosphorylation in vivo (Ghavidel and Schultz, 1997).
It is important to remark that RNAPIII transcription is regulated in response to environmental cues and during the different stages encompassed in the cell cycle. It has been reported that tRNA levels fluctuate during the cell cycle in a process controlled by the Cdk1/Clb5 kinase complex, boosting tRNA expression during the S phase. This is attained by the cycling phosphorylation of Bdp1, an event that triggers the recruitment of TFIIIC to the genes for tRNAs, stimulates interaction between TFIIIB and TFIIIC and enhances RNAPIII activity (Herrera et al., 2018). However, the physiological significance of cell cycle regulation of RNAPIII transcription remains to be elucidated and undoubtedly will be a fascinating question for the future.
Coordination of RNAP Activities: Adapting Gene Expression to Environmental Conditions
RNAP activities are essential for cellular viability and a limiting step in regulating gene expression. All RNAPs respond to growth cell conditions and nutrient availability. In actively growing cells, the majority of the transcriptional output is due to RNAPI and RNAPIII activities, which are required for the synthesis of ribosomes. The activity of RNAPII is also essential because it transcribes all ribosomal protein genes and genes encoding factors required for ribosome assembly (Ribi regulon) (Warner, 1999; de la Cruz et al., 2018). How eukaryotic RNAPs are regulated has been extensively studied and is still a field of great interest. However, less is known about the mechanisms coordinating and communicating with the three RNAP machineries to adapt cell growth to environmental conditions. Factors that coordinate at least the function of two RNAPs have been identified. For instance, Spt4/5, Paf1C and Ccr4 regulate both RNAPI and RNAPII transcription (Zhang et al., 2010; Anderson et al., 2011; Hartzog and Fu, 2013; Laribee et al., 2015). Similarly, TFIIS and Sub1 influence RNAPII and RNAPIII (Guglielmi et al., 2007; Ghavi-Helm et al., 2008; Carriere et al., 2012; Garcia et al., 2012; Garavis et al., 2017; Calvo, 2018). Recently, the yeast prefoldin-like Bud27 has been shown to be a regulator of the three RNAPs, most likely via its association with a common subunit, Rpb5 (Martinez-Fernandez et al., 2020). One possibility is that RNAPs might be coordinated through regulation of the phosphorylation state of their shared subunits. In support of this hypothesis, Rpb5, Rpb6, Rpb8, Rpb10, and Rpb12 contain phospho-sites (Albuquerque et al., 2008; Swaney et al., 2013; Sostaric et al., 2018; MacGilvray et al., 2020) (Supplementary Table S1).
TOR serine/threonine kinases play an essential role in controlling many aspects of living cells, such as growth, proliferation and survival in response to nutrients (Loewith and Hall, 2011; Kim and Guan, 2019; Laribee and Weisman, 2020). Initially, it was reported that TOR proteins only localized in the cytoplasm, with a crucial role in regulating protein synthesis (Barbet et al., 1996; Gingras et al., 2004). We currently know that TOR and its associated proteins also localize in the nucleus, where they regulate gene expression to guarantee the appropriate ribogenesis (Tsang and Zheng, 2007; Laribee, 2018; Laribee and Weisman, 2020). When S. cerevisiae is grown under nutrient-replete conditions, Tor1 localizes in both the cytoplasm and the nucleus. In the nucleus, Tor1 and Kog1 (the Raptor subunit in S. cerevisiae) bind to the 35S (RNAPI) and 5S (RNAPIII) promoters. However, after starvation or rapamycin treatment, they are removed from these regions, thus inhibiting transcription (Li et al., 2006). In mammals, mTOR and Raptor also interact with the RNAPIII factor TFIIIC to induce 5S and tRNA transcription (Kantidakis et al., 2010). This and other evidence suggest that TORC1 complexes are RNAPI and RNAPIII regulators and likely coordinators of these two RNAP activities. Whether any of the RNAPI or RNAPIII subunits are phosphorylated by TORC1 is unknown. Nevertheless, RNAPIII transcription is also activated by TORC1 via phosphorylation of Maf1 in yeasts (Huber et al., 2009; Lee et al., 2009) and mammals (Kantidakis et al., 2010; Michels et al., 2010). Whereas mTOR and Raptor contribute to RNAPII transcription regulation of a number of genes in mammals (Cunningham et al., 2007; Chaveroux et al., 2013; Laribee, 2018), in budding yeast only the HMO1 gene is known to be directly activated by Tor1 (Panday et al., 2017). Hmo1 activates the transcription of genes regulated by TORC1, including RP, 5S and 35S genes (Gadal et al., 2002; Hall et al., 2006). Both Tor1 and Hmo1 bind to the HMO1 promoter, facilitating its transcription. After rapamycin treatment or DNA damage, Tor1 and Hmo1 are released, thus inhibiting transcription (Panday et al., 2017). Interestingly, promoter binding by the Tor1 kinase is a prerequisite for transcription inhibition, which suggests that Tor1 may phosphorylate a specific target to repress transcription in response to stress conditions. One of these targets might be Paf1C, whose activity is needed to attenuate RNAPI transcription after TORC1 inhibition (Zhang et al., 2010). Similarly, Ccr4 couples nutrient signaling through TORC1 with Rrn3-RNAPI transcription inhibition (Laribee et al., 2015). It would be reasonable to think that Tor1 kinases could also phosphorylate RNAPs, maybe common subunits, to coordinate and modulate their activities in response to environmental conditions.
Author Contributions
All authors listed have made a substantial, direct, and intellectual contribution to the work and approved it for publication.
Funding
Funding was provided by the Spanish Ministry of Economy and Competitiveness (MINECO) and ERDF with Grants conceded to OC (BFU2017-84694-P), AC-B (PGC2018-097963-B-100), FN (BFU2016-77728-C3-2-P), and by Spanish Ministry of Science and Innovation (MICINN) and ERDF to OC and FN (RED2018-102467-T); and by the Junta de Andalucía-Universidad de Jaén (FEDER-UJA 1260360), and the Junta de Andalucía (BIO258) to FN, AG-J, and AC were supported by pre-doctoral contracts from “Junta de Castilla y León”. The IBFG is supported in part by an institutional grant from the “Junta y Castilla y León” (Programa “Escalera de Excelencia” de la Junta de Castilla y León, CLU-2017-03), cofunded by ERDF OP de Castilla y León 14-20.
Conflict of Interest
The authors declare that the research was conducted in the absence of any commercial or financial relationships that could be construed as a potential conflict of interest.
Acknowledgments
We thank members of our laboratories for helpful discussions on the manuscript.
Supplementary Material
The Supplementary Material for this article can be found online at: https://www.frontiersin.org/articles/10.3389/fmolb.2021.681865/full#supplementary-material
References
Ahn, C. S., Lee, D.-H., and Pai, H.-S. (2019). Characterization of Maf1 in Arabidopsis: Function under Stress Conditions and Regulation by the TOR Signaling Pathway. Planta 249 (2), 527–542. doi:10.1007/s00425-018-3024-5
Albuquerque, C. P., Smolka, M. B., Payne, S. H., Bafna, V., Eng, J., and Zhou, H. (2008). A Multidimensional Chromatography Technology for In-Depth Phosphoproteome Analysis. Mol. Cell Proteomics 7 (7), 1389–1396. doi:10.1074/mcp.M700468-MCP200
Allepuz-Fuster, P., Martínez-Fernández, V., Garrido-Godino, A. I., Alonso-Aguado, S., Hanes, S. D., Navarro, F., et al. (2014). Rpb4/7 Facilitates RNA Polymerase II CTD Dephosphorylation. Nucleic Acids Res. 42 (22), 13674–13688. doi:10.1093/nar/gku1227
Allepuz-Fuster, P., O’Brien, M. J., González-Polo, N., Pereira, B., Dhoondia, Z., Ansari, A., et al. (2019). RNA Polymerase II Plays an Active Role in the Formation of Gene Loops through the Rpb4 Subunit. Nucleic Acids Res. 47 (17), 8975–8987. doi:10.1093/nar/gkz597
Anderson, S. J., Sikes, M. L., Zhang, Y., French, S. L., Salgia, S., Beyer, A. L., et al. (2011). The Transcription Elongation Factor Spt5 Influences Transcription by RNA Polymerase I Positively and Negatively. J. Biol. Chem. 286 (21), 18816–18824. doi:10.1074/jbc.M110.202101
Armache, K.-J., Kettenberger, H., and Cramer, P. (2003). Architecture of Initiation-Competent 12-subunit RNA Polymerase II. Proc. Natl. Acad. Sci. 100 (12), 6964–6968. doi:10.1073/pnas.1030608100
Armache, K.-J., Mitterweger, S., Meinhart, A., and Cramer, P. (2005). Structures of Complete RNA Polymerase II and its Subcomplex, Rpb4/7. J. Biol. Chem. 280 (8), 7131–7134. doi:10.1074/jbc.M413038200
Babbarwal, V., Fu, J., and Reese, J. C. (2014). The Rpb4/7 Module of RNA Polymerase II Is Required for Carbon Catabolite Repressor Protein 4-negative on TATA (Ccr4-Not) Complex to Promote Elongation. J. Biol. Chem. 289 (48), 33125–33130. doi:10.1074/jbc.C114.601088
Barbet, N. C., Schneider, U., Helliwell, S. B., Stansfield, I., Tuite, M. F., and Hall, M. N. (1996). TOR Controls Translation Initiation and Early G1 Progression in Yeast. MBoC 7 (1), 25–42. doi:10.1091/mbc.7.1.25
Beltrao, P., Bork, P., Krogan, N. J., and Noort, V. (2013). Evolution and Functional Cross‐talk of Protein post‐translational Modifications. Mol. Syst. Biol. 9, 714. doi:10.1002/msb.201304521
Bierhoff, H., Dundr, M., Michels, A. A., and Grummt, I. (2008). Phosphorylation by Casein Kinase 2 Facilitates rRNA Gene Transcription by Promoting Dissociation of TIF-IA from Elongating RNA Polymerase I. Mcb 28 (16), 4988–4998. doi:10.1128/MCB.00492-08
Boehning, M., Dugast-Darzacq, C., Rankovic, M., Hansen, A. S., Yu, T., Marie-Nelly, H., et al. (2018). RNA Polymerase II Clustering through Carboxy-Terminal Domain Phase Separation. Nat. Struct. Mol. Biol. 25 (9), 833–840. doi:10.1038/s41594-018-0112-y
Buratowski, S. (2009). Progression through the RNA Polymerase II CTD Cycle. Mol. Cel 36 (4), 541–546. doi:10.1016/j.molcel.2009.10.019
Buratowski, S. (2003). The CTD Code. Nat. Struct. Mol. Biol. 10 (9), 679–680. doi:10.1038/nsb0903-679
Bushnell, D. A., and Kornberg, R. D. (2003). Complete, 12-subunit RNA Polymerase II at 4.1-A Resolution: Implications for the Initiation of Transcription. Proc. Natl. Acad. Sci. 100 (12), 6969–6973. doi:10.1073/pnas.1130601100
Cai, G., Imasaki, T., Yamada, K., Cardelli, F., Takagi, Y., and Asturias, F. J. (2010). Mediator Head Module Structure and Functional Interactions. Nat. Struct. Mol. Biol. 17 (3), 273–279. doi:10.1038/nsmb.1757
Calvo, O., and García, A. (2012). “RNA Polymerase II Phosphorylation and Gene Expression Regulation,” in Protein Phosphorylation in Human Cells (Rijeka, Croatia: Intech Open), 151–194.
Calvo, O. (2020). RNA Polymerase II Phosphorylation and Gene Looping: New Roles for the Rpb4/7 Heterodimer in Regulating Gene Expression. Curr. Genet. 66 (5), 927–937. doi:10.1007/s00294-020-01084-w
Calvo, O. (2018). Sub1 and RNAPII, until Termination Does Them Part. Transcription 9 (1), 52–60. doi:10.1080/21541264.2017.1333558
Carrière, L., Graziani, S., Alibert, O., Ghavi-Helm, Y., Boussouar, F., Humbertclaude, H., et al. (2012). Genomic Binding of Pol III Transcription Machinery and Relationship with TFIIS Transcription Factor Distribution in Mouse Embryonic Stem Cells. Nucleic Acids Res. 40 (1), 270–283. doi:10.1093/nar/gkr737
Cavanaugh, A. H., Hirschler-Laszkiewicz, I., Hu, Q., Dundr, M., Smink, T., Misteli, T., et al. (2002). Rrn3 Phosphorylation Is a Regulatory Checkpoint for Ribosome Biogenesis. J. Biol. Chem. 277 (30), 27423–27432. doi:10.1074/jbc.M201232200
Chapman, R. D., Heidemann, M., Hintermair, C., and Eick, D. (2008). Molecular Evolution of the RNA Polymerase II CTD. Trends Genet. 24 (6), 289–296. doi:10.1016/j.tig.2008.03.010
Chaveroux, C., Eichner, L. J., Dufour, C. R., Shatnawi, A., Khoutorsky, A., Bourque, G., et al. (2013). Molecular and Genetic Crosstalks between mTOR and ERRα Are Key Determinants of Rapamycin-Induced Nonalcoholic Fatty Liver. Cel Metab. 17 (4), 586–598. doi:10.1016/j.cmet.2013.03.003
Clemente-Blanco, A., Mayán-Santos, M., Schneider, D. A., Machín, F., Jarmuz, A., Tschochner, H., et al. (2009). Cdc14 Inhibits Transcription by RNA Polymerase I during Anaphase. Nature 458 (7235), 219–222. doi:10.1038/nature07652
Corden, J. L., Cadena, D. L., Ahearn, J. M., and Dahmus, M. E. (1985). A Unique Structure at the Carboxyl Terminus of the Largest Subunit of Eukaryotic RNA Polymerase II. Proc. Natl. Acad. Sci. 82 (23), 7934–7938. doi:10.1073/pnas.82.23.7934
Cramer, P., Armache, K.-J., Baumli, S., Benkert, S., Brueckner, F., Buchen, C., et al. (2008). Structure of Eukaryotic RNA Polymerases. Annu. Rev. Biophys. 37, 337–352. doi:10.1146/annurev.biophys.37.032807.130008
Cramer, P., Bushnell, D. A., Fu, J., Gnatt, A. L., Maier-Davis, B., Thompson, N. E., et al. (2000). Architecture of RNA Polymerase II and Implications for the Transcription Mechanism. Science 288 (5466), 640–649. doi:10.1126/science.288.5466.640
Cramer, P., Bushnell, D. A., and Kornberg, R. D. (2001). Structural Basis of Transcription: RNA Polymerase II at 2.8 Angstrom Resolution. Science 292 (5523), 1863–1876. doi:10.1126/science.1059493
Cramer, P. (2019a). Eukaryotic Transcription Turns 50. Cell 179 (4), 808–812. doi:10.1016/j.cell.2019.09.018
Cramer, P. (2019b). Organization and Regulation of Gene Transcription. Nature 573 (7772), 45–54. doi:10.1038/s41586-019-1517-4
Cuevas-Bermúdez, A., Martinez-Fernandez, V., Garrido-Godino, A. I., and Navarro, F. (2017). “Subunits Common to RNA Polymerases,” in The Yeast Role in Medical Applications (Rijeka, Croatia: Intech Open), 151–165.)
Cunningham, J. T., Rodgers, J. T., Arlow, D. H., Vazquez, F., Mootha, V. K., and Puigserver, P. (2007). mTOR Controls Mitochondrial Oxidative Function through a YY1-PGC-1α Transcriptional Complex. Nature 450 (7170), 736–740. doi:10.1038/nature06322
de la Cruz, J., Gómez-Herreros, F., Rodríguez-Galán, O., Begley, V., de la Cruz Muñoz-Centeno, M., and Chávez, S. (2018). Feedback Regulation of Ribosome Assembly. Curr. Genet. 64 (2), 393–404. doi:10.1007/s00294-017-0764-x
Deribe, Y. L., Pawson, T., and Dikic, I. (2010). Post-translational Modifications in Signal Integration. Nat. Struct. Mol. Biol. 17 (6), 666–672. doi:10.1038/nsmb.1842
Dieci, G., Fiorino, G., Castelnuovo, M., Teichmann, M., and Pagano, A. (2007). The Expanding RNA Polymerase III Transcriptome. Trends Genet. 23 (12), 614–622. doi:10.1016/j.tig.2007.09.001
Duek, L., Barkai, O., Elran, R., Adawi, I., and Choder, M. (2018). Dissociation of Rpb4 from RNA Polymerase II Is Important for Yeast Functionality. PLoS One 13 (10), e0206161. doi:10.1371/journal.pone.0206161
Eick, D., and Geyer, M. (2013). The RNA Polymerase II Carboxy-Terminal Domain (CTD) Code. Chem. Rev. 113 (11), 8456–8490. doi:10.1021/cr400071f
Engel, C., Sainsbury, S., Cheung, A. C., Kostrewa, D., and Cramer, P. (2013). RNA Polymerase I Structure and Transcription Regulation. Nature 502 (7473), 650–655. doi:10.1038/nature12712
Fath, S., Kobor, M. S., Philippi, A., Greenblatt, J., and Tschochner, H. (2004). Dephosphorylation of RNA Polymerase I by Fcp1p Is Required for Efficient rRNA Synthesis. J. Biol. Chem. 279 (24), 25251–25259. doi:10.1074/jbc.M401867200
Fath, S., Milkereit, P., Peyroche, G., Riva, M., Carles, C., and Tschochner, H. (2001). Differential Roles of Phosphorylation in the Formation of Transcriptional Active RNA Polymerase I. Proc. Natl. Acad. Sci. 98 (25), 14334–14339. doi:10.1073/pnas.231181398
Fernández-Tornero, C., Moreno-Morcillo, M., Rashid, U. J., Taylor, N. M. I., Ruiz, F. M., Gruene, T., et al. (2013). Crystal Structure of the 14-subunit RNA Polymerase I. Nature 502 (7473), 644–649. doi:10.1038/nature12636
Ficarro, S. B., McCleland, M. L., Stukenberg, P. T., Burke, D. J., Ross, M. M., Shabanowitz, J., et al. (2002). Phosphoproteome Analysis by Mass Spectrometry and its Application to Saccharomyces cerevisiae. Nat. Biotechnol. 20 (3), 301–305. doi:10.1038/nbt0302-301
Gadal, O., Labarre, S., Boschiero, C., and Thuriaux, P. (2002). Hmo1, an HMG-Box Protein, Belongs to the Yeast Ribosomal DNA Transcription System. EMBO J. 21 (20), 5498–5507. doi:10.1093/emboj/cdf539
Garavís, M., González-Polo, N., Allepuz-Fuster, P., Louro, J. A., Fernández-Tornero, C., and Calvo, O. (2017). Sub1 Contacts the RNA Polymerase II Stalk to Modulate mRNA Synthesis. Nucleic Acids Res. 45 (5), 2458–2471. doi:10.1093/nar/gkw1206
García, A., Collin, A., and Calvo, O. (2012). Sub1 Associates with Spt5 and Influences RNA Polymerase II Transcription Elongation Rate. MBoC 23 (21), 4297–4312. doi:10.1091/mbc.E12-04-0331
Gerber, J., Reiter, A., Steinbauer, R., Jakob, S., Kuhn, C.-D., Cramer, P., et al. (2008). Site Specific Phosphorylation of Yeast RNA Polymerase I. Nucleic Acids Res. 36 (3), 793–802. doi:10.1093/nar/gkm1093
Ghavi-Helm, Y., Michaut, M., Acker, J., Aude, J.-C., Thuriaux, P., Werner, M., et al. (2008). Genome-wide Location Analysis Reveals a Role of TFIIS in RNA Polymerase III Transcription. Genes Develop. 22 (14), 1934–1947. doi:10.1101/gad.471908
Ghavidel, A., and Schultz, M. C. (1997). Casein Kinase II Regulation of Yeast TFIIIB Is Mediated by the TATA-Binding Protein. Genes Develop. 11 (21), 2780–2789. doi:10.1101/gad.11.21.2780
Gingras, A.-C., Raught, B., and Sonenberg, N. (2004). mTOR Signaling to Translation. Curr. Top. Microbiol. Immunol. 279, 169–197. doi:10.1007/978-3-642-18930-2_11
Gnatt, A. L., Cramer, P., Fu, J., Bushnell, D. A., and Kornberg, R. D. (2001). Structural Basis of Transcription: an RNA Polymerase II Elongation Complex at 3.3 A Resolution. Science 292 (5523), 1876–1882. doi:10.1126/science.1059495
Graczyk, D., Debski, J., Muszynska, G., Bretner, M., Lefebvre, O., and Boguta, M. (2011). Casein Kinase II-Mediated Phosphorylation of General Repressor Maf1 Triggers RNA Polymerase III Activation. Proc. Natl. Acad. Sci. 108 (12), 4926–4931. doi:10.1073/pnas.1010010108
Greber, B. J., and Nogales, E. (2019). The Structures of Eukaryotic Transcription Pre-initiation Complexes and Their Functional Implications. Subcell Biochem. 93, 143–192. doi:10.1007/978-3-030-28151-9_5
Grummt, I., and Voit, R. (2010). Linking rDNA Transcription to the Cellular Energy Supply. Cell Cycle 9 (2), 225–226. doi:10.4161/cc.9.2.10614
Guglielmi, B., Soutourina, J., Esnault, C., and Werner, M. (2007). TFIIS Elongation Factor and Mediator Act in Conjunction during Transcription Initiation In Vivo. Proc. Natl. Acad. Sci. 104 (41), 16062–16067. doi:10.1073/pnas.0704534104
Guo, Y. E., Manteiga, J. C., Henninger, J. E., Sabari, B. R., Dall’Agnese, A., Hannett, N. M., et al. (2019). Pol II Phosphorylation Regulates a Switch between Transcriptional and Splicing Condensates. Nature 572 (7770), 543–548. doi:10.1038/s41586-019-1464-0
Haag, J. R., and Pikaard, C. S. (2011). Multisubunit RNA Polymerases IV and V: Purveyors of Non-coding RNA for Plant Gene Silencing. Nat. Rev. Mol. Cel Biol 12 (8), 483–492. doi:10.1038/nrm3152
Hahn, S., and Young, E. T. (2011). Transcriptional Regulation in Saccharomyces cerevisiae: Transcription Factor Regulation and Function, Mechanisms of Initiation, and Roles of Activators and Coactivators. Genetics 189 (3), 705–736. doi:10.1534/genetics.111.127019
Hall, D. B., Wade, J. T., and Struhl, K. (2006). An HMG Protein, Hmo1, Associates with Promoters of many Ribosomal Protein Genes and throughout the rRNA Gene Locus in Saccharomyces cerevisiae. Mol. Cel Biol 26 (9), 3672–3679. doi:10.1128/MCB.26.9.3672-3679.2006
Harlen, K. M., and Churchman, L. S. (2017). The Code and beyond: Transcription Regulation by the RNA Polymerase II Carboxy-Terminal Domain. Nat. Rev. Mol. Cel Biol 18 (4), 263–273. doi:10.1038/nrm.2017.10
Hartzog, G. A., and Fu, J. (2013). The Spt4-Spt5 Complex: a Multi-Faceted Regulator of Transcription Elongation. Biochim. Biophys. Acta (Bba) - Gene Regul. Mech. 1829 (1), 105–115. doi:10.1016/j.bbagrm.2012.08.007
He, Y., Fang, J., Taatjes, D. J., and Nogales, E. (2013). Structural Visualization of Key Steps in Human Transcription Initiation. Nature 495 (7442), 481–486. doi:10.1038/nature11991
He, Y., Yan, C., Fang, J., Inouye, C., Tjian, R., Ivanov, I., et al. (2016). Near-atomic Resolution Visualization of Human Transcription Promoter Opening. Nature 533 (7603), 359–365. doi:10.1038/nature17970
Herrera, M. C., Chymkowitch, P., Robertson, J. M., Eriksson, J., Bøe, S. O., Alseth, I., et al. (2018). Cdk1 gates Cell Cycle-dependent tRNA Synthesis by Regulating RNA Polymerase III Activity. Nucleic Acids Res. 46 (22), 11698–11711. doi:10.1093/nar/gky846
Ho, C. K., and Shuman, S. (1999). Distinct Roles for CTD Ser-2 and Ser-5 Phosphorylation in the Recruitment and Allosteric Activation of Mammalian mRNA Capping Enzyme. Mol. Cel 3 (3), 405–411. doi:10.1016/s1097-2765(00)80468-2
Hoffmann, N. A., Jakobi, A. J., Moreno-Morcillo, M., Glatt, S., Kosinski, J., Hagen, W. J. H., et al. (2015). Molecular Structures of Unbound and Transcribing RNA Polymerase III. Nature 528 (7581), 231–236. doi:10.1038/nature16143
Holt, L. J., Tuch, B. B., Villen, J., Johnson, A. D., Gygi, S. P., and Morgan, D. O. (2009). Global Analysis of Cdk1 Substrate Phosphorylation Sites Provides Insights into Evolution. Science 325 (5948), 1682–1686. doi:10.1126/science.1172867
Hoppe, S., Bierhoff, H., Cado, I., Weber, A., Tiebe, M., Grummt, I., et al. (2009). AMP-activated Protein Kinase Adapts rRNA Synthesis to Cellular Energy Supply. Proc. Natl. Acad. Sci. 106 (42), 17781–17786. doi:10.1073/pnas.0909873106
Hsin, J.-P., and Manley, J. L. (2012). The RNA Polymerase II CTD Coordinates Transcription and RNA Processing. Genes Develop. 26 (19), 2119–2137. doi:10.1101/gad.200303.112
Hsin, J.-P., Sheth, A., and Manley, J. L. (2011). RNAP II CTD Phosphorylated on Threonine-4 Is Required for Histone mRNA 3' End Processing. Science 334 (6056), 683–686. doi:10.1126/science.1206034
Huber, A., Bodenmiller, B., Uotila, A., Stahl, M., Wanka, S., Gerrits, B., et al. (2009). Characterization of the Rapamycin-Sensitive Phosphoproteome Reveals that Sch9 Is a central Coordinator of Protein Synthesis. Genes Develop. 23 (16), 1929–1943. doi:10.1101/gad.532109
Huet, J., Riva, M., Sentenac, A., and Fromageot, P. (1985). Yeast RNA Polymerase C and its Subunits. Specific Antibodies as Structural and Functional Probes. J. Biol. Chem. 260 (28), 15304–15310. doi:10.1016/s0021-9258(18)95736-4
Kantidakis, T., Ramsbottom, B. A., Birch, J. L., Dowding, S. N., and White, R. J. (2010). mTOR Associates with TFIIIC, Is Found at tRNA and 5S rRNA Genes, and Targets Their Repressor Maf1. Proc. Natl. Acad. Sci. 107 (26), 11823–11828. doi:10.1073/pnas.1005188107
Kim, J., and Guan, K.-L. (2019). mTOR as a central Hub of Nutrient Signalling and Cell Growth. Nat. Cel Biol 21 (1), 63–71. doi:10.1038/s41556-018-0205-1
Kim, Y.-J., Björklund, S., Li, Y., Sayre, M. H., and Kornberg, R. D. (1994). A Multiprotein Mediator of Transcriptional Activation and its Interaction with the C-Terminal Repeat Domain of RNA Polymerase II. Cell 77 (4), 599–608. doi:10.1016/0092-8674(94)90221-6
Kimura, M., Suzuki, H., and Ishihama, A. (2002). Formation of a Carboxy-Terminal Domain Phosphatase (Fcp1)/TFIIF/RNA Polymerase II (Pol II) Complex in Schizosaccharomyces pombe Involves Direct Interaction between Fcp1 and the Rpb4 Subunit of Pol II. Mol. Cel Biol 22 (5), 1577–1588. doi:10.1128/mcb.22.5.1577-1588.2002
Kostrewa, D., Zeller, M. E., Armache, K.-J., Seizl, M., Leike, K., Thomm, M., et al. (2009). RNA Polymerase II-TFIIB Structure and Mechanism of Transcription Initiation. Nature 462 (7271), 323–330. doi:10.1038/nature08548
Kwak, H., and Lis, J. T. (2013). Control of Transcriptional Elongation. Annu. Rev. Genet. 47, 483–508. doi:10.1146/annurev-genet-110711-155440
Lanz, M. C., Yugandhar, K., Gupta, S., Sanford, E. J., Faça, V. M., Vega, S., et al. (2021). In‐depth and 3‐dimensional Exploration of the Budding Yeast Phosphoproteome. EMBO Rep. 22 (2), e51121. doi:10.15252/embr.202051121
Laribee, R. N., Hosni-Ahmed, A., Workman, J. J., and Chen, H. (2015). Ccr4-Not Regulates RNA Polymerase I Transcription and Couples Nutrient Signaling to the Control of Ribosomal RNA Biogenesis. Plos Genet. 11 (3), e1005113. doi:10.1371/journal.pgen.1005113
Laribee, R. N. (2018). Transcriptional and Epigenetic Regulation by the Mechanistic Target of Rapamycin Complex 1 Pathway. J. Mol. Biol. 430 (24), 4874–4890. doi:10.1016/j.jmb.2018.10.008
Laribee, R. N., and Weisman, R. (2020). Nuclear Functions of TOR: Impact on Transcription and the Epigenome. Genes 11 (6), 641. doi:10.3390/genes11060641
Larochelle, S., Amat, R., Glover-Cutter, K., Sansó, M., Zhang, C., Allen, J. J., et al. (2012). Cyclin-dependent Kinase Control of the Initiation-To-Elongation Switch of RNA Polymerase II. Nat. Struct. Mol. Biol. 19 (11), 1108–1115. doi:10.1038/nsmb.2399
Lee, J., Moir, R. D., McIntosh, K. B., and Willis, I. M. (2012). TOR Signaling Regulates Ribosome and tRNA Synthesis via LAMMER/Clk and GSK-3 Family Kinases. Mol. Cel 45 (6), 836–843. doi:10.1016/j.molcel.2012.01.018
Lee, J., Moir, R. D., and Willis, I. M. (2015). Differential Phosphorylation of RNA Polymerase III and the Initiation Factor TFIIIB in Saccharomyces cerevisiae. PLoS One 10 (5), e0127225. doi:10.1371/journal.pone.0127225
Lee, J., Moir, R. D., and Willis, I. M. (2009). Regulation of RNA Polymerase III Transcription Involves SCH9-dependent and SCH9-independent Branches of the Target of Rapamycin (TOR) Pathway. J. Biol. Chem. 284 (19), 12604–12608. doi:10.1074/jbc.C900020200
Li, H., Tsang, C. K., Watkins, M., Bertram, P. G., and Zheng, X. F. S. (2006). Nutrient Regulates Tor1 Nuclear Localization and Association with rDNA Promoter. Nature 442 (7106), 1058–1061. doi:10.1038/nature05020
Li, W., Giles, C., and Li, S. (2014). Insights into How Spt5 Functions in Transcription Elongation and Repressing Transcription Coupled DNA Repair. Nucleic Acids Res. 42 (11), 7069–7083. doi:10.1093/nar/gku333
Liu, X., Bushnell, D. A., Wang, D., Calero, G., and Kornberg, R. D. (2010). Structure of an RNA Polymerase II-TFIIB Complex and the Transcription Initiation Mechanism. Science 327 (5962), 206–209. doi:10.1126/science.1182015
Loewith, R., and Hall, M. N. (2011). Target of Rapamycin (TOR) in Nutrient Signaling and Growth Control. Genetics 189 (4), 1177–1201. doi:10.1534/genetics.111.133363
López, A., Ramírez, V., García-Andrade, J., Flors, V., and Vera, P. (2011). The RNA Silencing Enzyme RNA Polymerase V Is Required for Plant Immunity. Plos Genet. 7 (12), e1002434. doi:10.1371/journal.pgen.1002434
MacGilvray, M. E., Shishkova, E., Place, M., Wagner, E. R., Coon, J. J., and Gasch, A. P. (2020). Phosphoproteome Response to Dithiothreitol Reveals Unique versus Shared Features of Saccharomyces cerevisiae Stress Responses. J. Proteome Res. 19 (8), 3405–3417. doi:10.1021/acs.jproteome.0c00253
Martínez-Fernández, V., Cuevas-Bermúdez, A., Gutiérrez-Santiago, F., Garrido-Godino, A. I., Rodríguez-Galán, O., Jordán-Pla, A., et al. (2020). Prefoldin-like Bud27 Influences the Transcription of Ribosomal Components and Ribosome Biogenesis in Saccharomyces cerevisiae. RNA 26 (10), 1360–1379. doi:10.1261/rna.075507.120
Martinez-Rucobo, F. W., Sainsbury, S., Cheung, A. C., and Cramer, P. (2011). Architecture of the RNA Polymerase-Spt4/5 Complex and Basis of Universal Transcription Processivity. EMBO J. 30 (7), 1302–1310. doi:10.1038/emboj.2011.64
Mayer, C., Bierhoff, H., and Grummt, I. (2005). The Nucleolus as a Stress Sensor: JNK2 Inactivates the Transcription Factor TIF-IA and Down-Regulates rRNA Synthesis. Genes Develop. 19 (8), 933–941. doi:10.1101/gad.333205
Michels, A. A., Robitaille, A. M., Buczynski-Ruchonnet, D., Hodroj, W., Reina, J. H., Hall, M. N., et al. (2010). mTORC1 Directly Phosphorylates and Regulates Human MAF1. Mol. Cel Biol 30 (15), 3749–3757. doi:10.1128/MCB.00319-10
Milkereit, P., and Tschochner, H. (1998). A Specialized Form of RNA Polymerase I, Essential for Initiation and Growth-dependent Regulation of rRNA Synthesis, Is Disrupted during Transcription. EMBO J. 17 (13), 3692–3703. doi:10.1093/emboj/17.13.3692
Mitsuzawa, H., Kanda, E., and Ishihama, A. (2003). Rpb7 Subunit of RNA Polymerase II Interacts with an RNA-Binding Protein Involved in Processing of Transcripts. Nucleic Acids Res. 31 (16), 4696–4701. doi:10.1093/nar/gkg688
Moir, R. D., Lee, J., Haeusler, R. A., Desai, N., Engelke, D. R., and Willis, I. M. (2006). Protein Kinase A Regulates RNA Polymerase III Transcription through the Nuclear Localization of Maf1. Proc. Natl. Acad. Sci. 103 (41), 15044–15049. doi:10.1073/pnas.0607129103
Moorefield, B., Greene, E. A., and Reeder, R. H. (2000). RNA Polymerase I Transcription Factor Rrn3 Is Functionally Conserved between Yeast and Human. Proc. Natl. Acad. Sci. 97 (9), 4724–4729. doi:10.1073/pnas.080063997
Mühlbacher, W., Sainsbury, S., Hemann, M., Hantsche, M., Neyer, S., Herzog, F., et al. (2014). Conserved Architecture of the Core RNA Polymerase II Initiation Complex. Nat. Commun. 5, 4310. doi:10.1038/ncomms5310
Naar, A. M., Taatjes, D. J., Zhai, W., Nogales, E., and Tjian, R. (2002). Human CRSP Interacts with RNA Polymerase II CTD and Adopts a Specific CTD-Bound Conformation. Genes Dev. 16 (11), 1339–1344. doi:10.1101/gad.987602
Nogales, E., and Greber, B. J. (2019). High-resolution Cryo-EM Structures of TFIIH and Their Functional Implications. Curr. Opin. Struct. Biol. 59, 188–194. doi:10.1016/j.sbi.2019.08.002
Nogales, E., Louder, R. K., and He, Y. (2017). Structural Insights into the Eukaryotic Transcription Initiation Machinery. Annu. Rev. Biophys. 46, 59–83. doi:10.1146/annurev-biophys-070816-033751
Oler, A. J., and Cairns, B. R. (2012). PP4 Dephosphorylates Maf1 to Couple Multiple Stress Conditions to RNA Polymerase III Repression. EMBO J. 31 (6), 1440–1452. doi:10.1038/emboj.2011.501
Onodera, Y., Haag, J. R., Ream, T., Nunes, P. C., Pontes, O., and Pikaard, C. S. (2005). Plant Nuclear RNA Polymerase IV Mediates siRNA and DNA Methylation-dependent Heterochromatin Formation. Cell 120 (5), 613–622. doi:10.1016/j.cell.2005.02.007
Panday, A., Gupta, A., Srinivasa, K., Xiao, L., Smith, M. D., and Grove, A. (2017). DNA Damage Regulates Direct Association of TOR Kinase with the RNA Polymerase II-Transcribed HMO1 Gene. MBoC 28 (18), 2449–2459. doi:10.1091/mbc.E17-01-0024
Pappas, D. L., and Hampsey, M. (2000). Functional Interaction between Ssu72 and the Rpb2 Subunit of RNA Polymerase II in Saccharomyces cerevisiae. Mol. Cel Biol 20 (22), 8343–8351. doi:10.1128/mcb.20.22.8343-8351.2000
Patel, A. B., Greber, B. J., and Nogales, E. (2020). Recent Insights into the Structure of TFIID, its Assembly, and its Binding to Core Promoter. Curr. Opin. Struct. Biol. 61, 17–24. doi:10.1016/j.sbi.2019.10.001
Peng, L., Li, E.-M., and Xu, L.-Y. (2020). From Start to End: Phase Separation and Transcriptional Regulation. Biochim. Biophys. Acta (Bba) - Gene Regul. Mech. 1863 (12), 194641. doi:10.1016/j.bbagrm.2020.194641
Perales, R., and Bentley, D. (2009). "Cotranscriptionality": The Transcription Elongation Complex as a Nexus for Nuclear Transactions. Mol. Cel 36 (2), 178–191. doi:10.1016/j.molcel.2009.09.018
Plaschka, C., Larivière, L., Wenzeck, L., Seizl, M., Hemann, M., Tegunov, D., et al. (2015). Architecture of the RNA Polymerase II-Mediator Core Initiation Complex. Nature 518 (7539), 376–380. doi:10.1038/nature14229
Pultz, D., Bennetzen, M. V., Rødkær, S. V., Zimmermann, C., Enserink, J. M., Andersen, J. S., et al. (2012). Global Mapping of Protein Phosphorylation Events Identifies Ste20, Sch9 and the Cell-Cycle Regulatory Kinases Cdc28/Pho85 as Mediators of Fatty Acid Starvation Responses in Saccharomyces cerevisiae. Mol. Biosyst. 8 (3), 796–803. doi:10.1039/c2mb05356j
Ramsay, E. P., Abascal-Palacios, G., Daiss, J. L., King, H., Gouge, J., Pilsl, M., et al. (2020). Structure of Human RNA Polymerase III. Nat. Commun. 11 (1), 6409. doi:10.1038/s41467-020-20262-5
Richard, S., Gross, L., Fischer, J., Bendalak, K., Ziv, T., Urim, S., et al. (2021). Numerous Post-translational Modifications of RNA Polymerase II Subunit Rpb4/7 Link Transcription to Post-transcriptional Mechanisms. Cel Rep. 34 (2), 108578. doi:10.1016/j.celrep.2020.108578
Robinson, P. J., Trnka, M. J., Bushnell, D. A., Davis, R. E., Mattei, P.-J., Burlingame, A. L., et al. (2016). Structure of a Complete Mediator-RNA Polymerase II Pre-initiation Complex. Cell 166 (6), 1411–1422 e1416. doi:10.1016/j.cell.2016.08.050
Roeder, R. G. (2019). 50+ Years of Eukaryotic Transcription: an Expanding Universe of Factors and Mechanisms. Nat. Struct. Mol. Biol. 26 (9), 783–791. doi:10.1038/s41594-019-0287-x
Runner, V. M., Podolny, V., and Buratowski, S. (2008). The Rpb4 Subunit of RNA Polymerase II Contributes to Cotranscriptional Recruitment of 3′ Processing Factors. Mol. Cel Biol 28 (6), 1883–1891. doi:10.1128/MCB.01714-07
Sainsbury, S., Bernecky, C., and Cramer, P. (2015). Structural Basis of Transcription Initiation by RNA Polymerase II. Nat. Rev. Mol. Cel Biol 16 (3), 129–143. doi:10.1038/nrm3952
Schier, A. C., and Taatjes, D. J. (2020). Structure and Mechanism of the RNA Polymerase II Transcription Machinery. Genes Dev. 34 (7-8), 465–488. doi:10.1101/gad.335679.119
Sdano, M. A., Fulcher, J. M., Palani, S., Chandrasekharan, M. B., Parnell, T. J., Whitby, F. G., et al. (2017). A Novel SH2 Recognition Mechanism Recruits Spt6 to the Doubly Phosphorylated RNA Polymerase II Linker at Sites of Transcription. Elife 6. doi:10.7554/eLife.28723
Sharma, N., and Kumari, R. (2013). Rpb4 and Rpb7: Multifunctional Subunits of RNA Polymerase II. Crit. Rev. Microbiol. 39 (4), 362–372. doi:10.3109/1040841X.2012.711742
Šoštarić, N., O'Reilly, F. J., Giansanti, P., Heck, A. J. R., Gavin, A. C., and van Noort, V. (2018). Effects of Acetylation and Phosphorylation on Subunit Interactions in Three Large Eukaryotic Complexes. Mol. Cel Proteomics 17 (12), 2387–2401. doi:10.1074/mcp.RA118.000892
Soulard, A., Cremonesi, A., Moes, S., Schütz, F., Jenö, P., and Hall, M. N. (2010). The Rapamycin-Sensitive Phosphoproteome Reveals that TOR Controls Protein Kinase A toward Some but Not All Substrates. MBoC 21 (19), 3475–3486. doi:10.1091/mbc.E10-03-0182
Swaney, D. L., Beltrao, P., Starita, L., Guo, A., Rush, J., Fields, S., et al. (2013). Global Analysis of Phosphorylation and Ubiquitylation Cross-Talk in Protein Degradation. Nat. Methods 10 (7), 676–682. doi:10.1038/nmeth.2519
Tan, Q., Prysak, M. H., and Woychik, N. A. (2003). Loss of the Rpb4/Rpb7 Subcomplex in a Mutant Form of the Rpb6 Subunit Shared by RNA Polymerases I, II, and III. Mol. Cel Biol 23 (9), 3329–3338. doi:10.1128/mcb.23.9.3329-3338.2003
Thomas, M. C., and Chiang, C.-M. (2006). The General Transcription Machinery and General Cofactors. Crit. Rev. Biochem. Mol. Biol. 41 (3), 105–178. doi:10.1080/10409230600648736
Torreira, E., Louro, J. A., Pazos, I., González-Polo, N., Gil-Carton, D., Duran, A. G., et al. (2017). The Dynamic Assembly of Distinct RNA Polymerase I Complexes Modulates rDNA Transcription. Elife 6, e20832. doi:10.7554/eLife.20832
Tsang, C. K., and Zheng, X. F. S. (2007). TOR-in(g) the Nucleus. Cell Cycle 6 (1), 25–29. doi:10.4161/cc.6.1.3675
Vannini, A., and Cramer, P. (2012). Conservation between the RNA Polymerase I, II, and III Transcription Initiation Machineries. Mol. Cel 45 (4), 439–446. doi:10.1016/j.molcel.2012.01.023
Vorländer, M. K., Baudin, F., Moir, R. D., Wetzel, R., Hagen, W. J. H., Willis, I. M., et al. (2020a). Structural Basis for RNA Polymerase III Transcription Repression by Maf1. Nat. Struct. Mol. Biol. 27 (3), 229–232. doi:10.1038/s41594-020-0383-y
Vorländer, M. K., Jungblut, A., Karius, K., Baudin, F., Grötsch, H., Kosinski, J., et al. (2020b). Structure of the TFIIIC Subcomplex τA Provides Insights into RNA Polymerase III Pre-initiation Complex Formation. Nat. Commun. 11 (1), 4905. doi:10.1038/s41467-020-18707-y
Wang, Y., Fairley, J. A., and Roberts, S. G. E. (2010). Phosphorylation of TFIIB Links Transcription Initiation and Termination. Curr. Biol. 20 (6), 548–553. doi:10.1016/j.cub.2010.01.052
Wang, Y., and Ma, H. (2015). Step‐wise and Lineage‐specific Diversification of Plant RNA Polymerase Genes and Origin of the Largest Plant‐specific Subunits. New Phytol. 207 (4), 1198–1212. doi:10.1111/nph.13432
Warner, J. R. (1999). The Economics of Ribosome Biosynthesis in Yeast. Trends Biochem. Sci. 24 (11), 437–440. doi:10.1016/s0968-0004(99)01460-7
Wei, Y., and Zheng, X. F. S. (2009). Sch9 Partially Mediates TORC1 Signaling to Control Ribosomal RNA Synthesis. Cell Cycle 8 (24), 4085–4090. doi:10.4161/cc.8.24.10170
Werner, F., and Grohmann, D. (2011). Evolution of Multisubunit RNA Polymerases in the Three Domains of Life. Nat. Rev. Microbiol. 9 (2), 85–98. doi:10.1038/nrmicro2507
Willis, I. M., and Moir, R. D. (2018). Signaling to and from the RNA Polymerase III Transcription and Processing Machinery. Annu. Rev. Biochem. 87, 75–100. doi:10.1146/annurev-biochem-062917-012624
Yurko, N. M., and Manley, J. L. (2018). The RNA Polymerase II CTD "orphan" Residues: Emerging Insights into the Functions of Tyr-1, Thr-4, and Ser-7. Transcription 9 (1), 30–40. doi:10.1080/21541264.2017.1338176
Zaros, C., Briand, J.-F., Boulard, Y., Labarre-Mariotte, S., Garcia-Lopez, M. C., Thuriaux, P., et al. (2007). Functional Organization of the Rpb5 Subunit Shared by the Three Yeast RNA Polymerases. Nucleic Acids Res. 35 (2), 634–647. doi:10.1093/nar/gkl686
Zhang, X., Henderson, I. R., Lu, C., Green, P. J., and Jacobsen, S. E. (2007). Role of RNA Polymerase IV in Plant Small RNA Metabolism. Proc. Natl. Acad. Sci. 104 (11), 4536–4541. doi:10.1073/pnas.0611456104
Zhang, Y., Smith, A. D., Renfrow, M. B., and Schneider, D. A. (2010). The RNA Polymerase-Associated Factor 1 Complex (Paf1C) Directly Increases the Elongation Rate of RNA Polymerase I and Is Required for Efficient Regulation of rRNA Synthesis. J. Biol. Chem. 285 (19), 14152–14159. doi:10.1074/jbc.M110.115220
Keywords: phosphorylation, transcription regulation, gene expression, RNA polymerase I, RNA polymerase II, RNA polymerase III
Citation: González-Jiménez A, Campos A, Navarro F, Clemente-Blanco A and Calvo O (2021) Regulation of Eukaryotic RNAPs Activities by Phosphorylation. Front. Mol. Biosci. 8:681865. doi: 10.3389/fmolb.2021.681865
Received: 17 March 2021; Accepted: 07 June 2021;
Published: 25 June 2021.
Edited by:
Silvia M. L. Barabino, University of Milano-Bicocca, ItalyReviewed by:
Jose C. Reyes, Andalusian Center of Molecular Biology and Regenerative Medicine (CABIMER), SpainJoe Reese, Pennsylvania State University (PSU), United States
Copyright © 2021 González-Jiménez, Campos, Navarro, Clemente-Blanco and Calvo. This is an open-access article distributed under the terms of the Creative Commons Attribution License (CC BY). The use, distribution or reproduction in other forums is permitted, provided the original author(s) and the copyright owner(s) are credited and that the original publication in this journal is cited, in accordance with accepted academic practice. No use, distribution or reproduction is permitted which does not comply with these terms.
*Correspondence: Olga Calvo, b2NhbHZvQHVzYWwuZXM=