- 1Departamento de Genética, Universidad de Sevilla, Seville, Spain
- 2Centro Andaluz de Biología Molecular y Medicina Regenerativa-CABIMER, Universidad de Sevilla-CSIC-Universidad Pablo de Olavide, Seville, Spain
The correct repair of DNA double-strand breaks is essential for maintaining the stability of the genome, thus ensuring the survival and fitness of any living organism. Indeed, the repair of these lesions is a complicated affair, in which several pathways compete for the DNA ends in a complex balance. Thus, the fine-tuning of the DNA double-strand break repair pathway choice relies on the different regulatory layers that respond to environmental cues. Among those different tiers of regulation, RNA modifications have just emerged as a promising field.
Double-Strand Breaks Repair Pathways
When DNA is damaged either physically or chemically, the integrity of the genomic information might be compromised. Therefore, living organisms have many repair pathways that try reverting the damaged DNA to the original DNA sequence or, at least, to minimize the impact of such changes. Particularly difficult-to-manage DNA lesions are DNA double-strand breaks (DSBs), which are produced when both DNA strands break at the same time in close proximity. Such DNA alteration triggers a very complex response, commonly known as the DNA damage response (DDR), that not only initiates the repair of the lesion but causes a complete upheaval of the cellular metabolism (Jackson and Bartek, 2009). In the case of DSBs, several repair pathways coexist. They are classified regarding the use or non-use of extensive homologous sequences as templates (Figure 1). Thus, non-homologous end-joining (NHEJ) requires no homology and process through the religation of both sides of the break (Davis and Chen, 2013; Radhakrishnan et al., 2014), whereas homologous recombination (HR) needs the presence of extensive homologies for the repair (Jasin and Rothstein, 2013). Additionally, alternative non-homologous end-joining (Alt-NHEJ) uses micro-homologous regions. Given that the outcome of each repair pathway is different, the choice between them has to be tightly regulated as errors in this balance can be very deleterious (for a review, see Ceccaldi et al., 2016). It is still not completely understood how cells chose between these alternative pathways, but one of the most important points is the processing of the DNA ends by nucleases to create single-stranded DNA (ssDNA), the so-called DNA end resection step (Figure 1). It consists of the nucleolytic production of long tails of ssDNA, which are promptly covered by the RPA complex that protects the structure (Huertas, 2010; Symington et al., 2014; Cejka, 2015). Such ssDNA is required for HR and Alt-NHEJ but efficiently blocks NHEJ. During classical HR, RAD51 replaces RPA, thus forming the so-called nucleoprotein filament that has the ability to search for homologous DNA (Figure 1) (Daley et al., 2014; Greene, 2016).
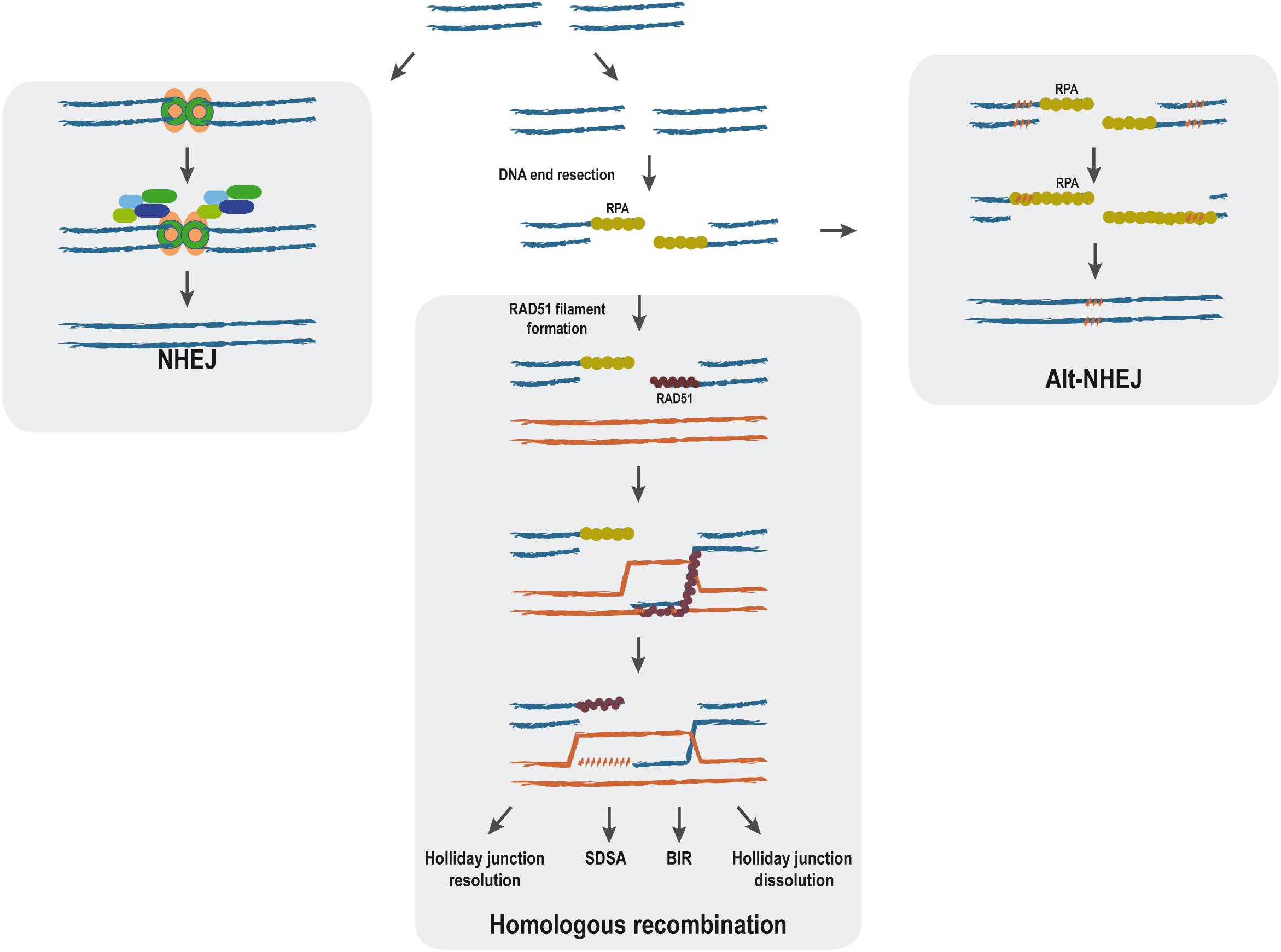
Figure 1. Main double-strand break (DSB) repair pathways. The main steps of non-homologous end joining (NHEJ), alternative non-homologous end-joining, and homologous recombination repair mechanisms are represented. DNA double-strand breaks can be repaired without end processing by NHEJ (left). In response to specific cues, the ends can be processed by a nucleolytic degradation of one strand to form long tails of ssDNA in the process known as resection. When this happens, classical NHEJ is effectively blocked. Sometimes, ssDNA formation will expose short stretches of microhomology (right, punctuated orange pattern), and the break will be repaired by Alt-NHEJ (right). Alternatively, homologous recombination can proceed using the resected DNA. Although HR can proceed through different HR pathways [holiday junction resolution, synthesis-dependent strand annealing (SDSA), break-induced replication (BIR), and holiday junction dissolution], all of them share the initial steps.
Many different aspects of cellular biology impact the outcome of the DSB repair. Among them, it has become clear in recent years that the RNA itself and RNA-related proteins play some essential roles (for review, see Jimeno et al., 2019). In this review, we focus specifically on the emerging significance of RNA modifications for DSB repair.
Roles of Different RNA Molecules in HR
There is extensive literature on the regulatory effects of different RNAs in DNA repair through in trans effects, that is, by affecting the expression of repair factors. Several comprehensive reviews can be found in the literature (Thapar, 2018; Jimeno et al., 2019; Bader et al., 2020). But additionally, RNA can affect directly the DSB repair in cis by the action of different RNA species, including the role of specific non-coding RNAs (ncRNAs) or by the presence of DNA:RNA hybrids at the site of DNA breaks.
Roles of RNA in the Damage-Induced Recruitment of Repair Factors
The first piece of evidence in this regard was shown by Pryde et al. (2005), when they showed that RNase A treatment caused the disappearance of ionizing radiation (IR)-induced 53BP1 foci and, moreover, that this effect was reverted by the addition of ectopic RNA (Pryde et al., 2005). Thus, it seems that RNA might stabilize some IR-induced foci (IRIF). Indeed, DICER and DROSHA play an RNA-mediated role in the secondary recruitment of DDR factors such as MDC1 and 53BP1 and, thus, in the amplification of the DDR signal (Francia et al., 2016). Recent work has allowed di Fagagna’s lab to propose a model in which the assembly of complete promoters at the sites of DSBs drives RNA synthesis, stimulating the phase separation of DDR factors in the shape of foci that exhibit liquid–liquid phase-separation condensate properties (Pessina et al., 2019). However, this does not apply generally to all IRIF, since RNA is not necessary for the formation of foci of the DDR sensor NBS1 (Francia et al., 2016), and, furthermore, the accumulation of RNA has been shown to be detrimental for the formation of RPA foci (Domingo-Prim et al., 2019).
De Novo Transcription of Long Non-coding RNA at DSB Sites
Although it has been thoroughly described that transcription is generally repressed after the appearance of DSBs (Shanbhag et al., 2010; Pankotai et al., 2012; Kim et al., 2015; Manfrini et al., 2015), some labs have reported de novo transcription around the DSB (Francia et al., 2012; Ohle et al., 2016; Michelini et al., 2017; D’Alessandro et al., 2018; Burger et al., 2019; Pessina et al., 2019; Vítor et al., 2019; Sharma et al., 2021). Thus, after DNA damage, RNA polymerase II is supposed to be recruited to the damaged chromatin and to start transcription around the break. Such transcription creates de novo long lncRNAs. The production of these damage-induced non-coding RNAs (dincRNAs) due to de novo transcription at the sites of the breaks is supposed to be essential for the proper activation of the DDR (Francia et al., 2012) and to fulfill the repair of the DSB, mainly by affecting Rad51 recruitment and thus having an impact on HR efficiency (Gao et al., 2014). In agreement, the inhibition of transcription before the damage induction negatively affects the formation of RPA and Rad51 foci (Yasuhara et al., 2018). However, whether the production of these damage-induced ncRNAs depends on paused polymerases rather than de novo–loaded ones is still open for debate (Puget et al., 2019).
Messenger RNA as a Repair Template
Another attractive possibility is that the mRNA molecule might act as a template, that is, a source of homology for the HR repair, but an unequivocal proof of such a role still remains evasive. In budding yeast RNaseH1 and RNAseH2 double mutants, HR can proceed directly using RNA templates, albeit with very low efficiency (Keskin et al., 2014). Indeed, in vitro, both yeast and human Rad52 could catalyze direct annealing of RNA to a DSB-like DNA end (Keskin et al., 2014). Furthermore, human and yeast Rad52 and Rad51 show the inverse strand exchange activity (Wahba et al., 2013; Mazina et al., 2017). This DNA–RNA strand exchange activity has also been described for PALB2, which supports not only the forward but also the inverse strand exchange in coordination or in the absence of RAD51 (Mazina et al., 2017; Deveryshetty et al., 2019). This homology-directed RNA–templated DNA repair seems to require transcription to provide the homologous transcript, which in turn forms an active ribonucleoprotein complex with RAD52 (McDevitt et al., 2018). Also, a Cockayne Syndrome group B (CSB)-mediated de novo transcription–dependent HR pathway has been found to rely on the capacity of RAD52 to bring an RNA template to the break site (Wei et al., 2015). Moreover, in postmitotic neurons, RAD52 is recruited to DSBs in a fashion that is dependent on the presence of nascent mRNA in the form of an R-loop (Welty et al., 2018). However, not only HR can use the RNA as an intermediate for repair, but even during NHEJ, an RNA molecule could be used to promote error-free repair at transcribed regions (Chakraborty et al., 2016). Furthermore, three additional DNA repair pathways that require the RNA molecule have been described recently in yeast. RNA- and cDNA-templated DSB repair pathways (R-TDR and c-TDR) use either an RNA transcript or a DNA copy of the RNA transcript for repair of induced DSBs, whereas RNA-templated DNA modification (R-TDM) acts on spontaneous or mutagen-induced breaks (Meers et al., 2020). Both R-TDR and R-TDM require the action of the RNA polymerase zeta. Despite the relevance of these findings, it is important to note that both R-TDM and R-TDR are observed only when RNase H1 and H2 are mutated, raising the possibility that these might not be the relevant repair routes in a physiological background.
DNA:RNA Hybrids on DSB Repair
On the other hand, the formation of persistent DNA:RNA hybrids or R-loops at the sites of the break seems to play both positive and negative roles during HR, even earlier to the strand invasion step (Ohle et al., 2016; Cohen et al., 2018; Costantino and Koshland, 2018; Lu et al., 2018; García-Muse and Aguilera, 2019; Jimeno et al., 2019; Paull, 2019; Puget et al., 2019; Domingo-Prim et al., 2020). The role of those R-loops is still controversial and requires further study for clarification. Although this is a very hot topic currently in the field of DNA repair, due to length constraints in this review, we will only summarize some general findings briefly at this point (Figure 2), and later in the context of RNA modifications, that is, the focus of this manuscript (see below). Especially, the role of R-loops in DSB repair and their consequences have been extensively discussed by others and us in recent reviews (for further details, see Jimeno et al., 2019; Puget et al., 2019; Domingo-Prim et al., 2020). For example, in Schizosaccharomyces pombe, it has been shown that DNA:RNA hybrids are required for DNA end resection (Ohle et al., 2016) and also that persistent R-loops block the resection prompting asymmetric DNA end processing (Costantino and Koshland, 2018). In human cells, an increase of R-loops close to DSBs located at transcribed regions has also been observed (Cohen et al., 2018; Lu et al., 2018; Bader and Bushell, 2020). We have previously suggested an interpretation of all the published data (Jimeno et al., 2019) that is shown in Figure 2. We propose that the accumulation of the DNA:RNA hybrids has different impacts on the DNA resection process depending on the localization in time and space of such structures. If pre-existent R-loops are present at the break locus before resection starts, they might act as a roadblock and hamper the resection processivity (Figure 2(1)). Then, the activities of proteins such as the helicase SETX would be required in order to facilitate the resection. On the contrary, R-loops close to DSBs can also recruit Rad52 and BRCA1, in turn counteracting the anti-resection activity of the Shieldin complex (Figure 2(2)). This will expedite DNA end processivity (Figure 2(3)). Finally, after the DNA short-range resection has switched to the long-range resection, de novo transcription of the ssDNA would create DNA:RNA hybrids that will not interfere with the DNA processing but might be required for a full DDR (Figure 2(4)). However, the removal of the RNA molecule bound to the ssDNA would be required to allow RPA loading onto ssDNA (Figure 2(5)).
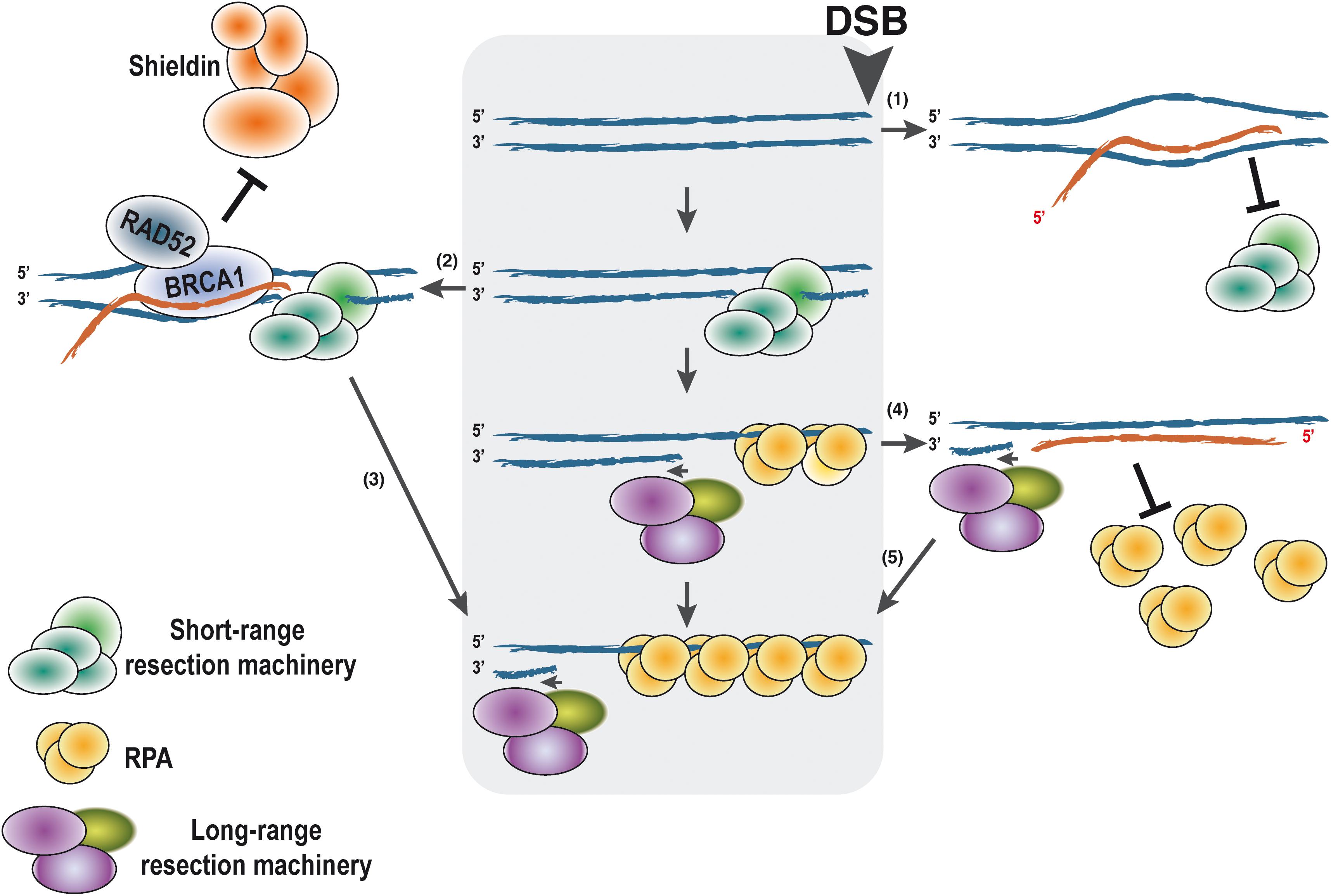
Figure 2. Putative effects of DNA:RNA hybrid presence on DNA end resection according to the timing of their appearance. DNA end resection proceeds unimpaired in the absence of the DNA:RNA hybrid (central gray box). For simplicity, only one end of the DNA is shown, with the DSB on the right side as marked. When an R-loop is present at the site of the break, it might block DNA end resection by blocking the first steps of the process (1). On the contrary, other studies indicate that if resection can indeed be started, the presence of a DNA:RNA hybrid can stimulate resection by attracting RAD52 and BRCA1 (2). They in turn will antagonize the anti-resection function of the Shieldin complex, thus stimulating resection processivity (3). Once resection is fully activated and the long-range resection is engaged, de novo transcription would create ncRNAs that can pair with the ssDNA, forming DNA:RNA hybrids (4). Such hybrids will not affect resection and will facilitate a full DDR. The presence of such structures might block RPA loading. Thus, elimination of the RNA will be required for activation of homologous recombination and limit DNA end resection (5). In all cases, the polarity of the DNA and RNA is shown. DDR, DNA damage response.
RNA Modifications in DNA Repair
RNA modification is emerging as another important layer of epigenetic regulation. It consists of the posttranscriptional chemical modification of the RNA molecule by different enzymes, creating a plethora of possible alternative epitranscriptomic signatures (Table 1).
Ribosomal RNA (rRNA) and transfer RNA (tRNA) are heavily modified RNA molecules (Roundtree et al., 2017), but in recent years, modifications in other RNA families, such as mRNA, micro RNA (miRNA), or lncRNA, have been readily observed (Shelton et al., 2016; Zhao et al., 2016; Esteller and Pandolfi, 2017). More than 100 different RNA modifications have been found in RNA, N6-methyladenosine (m6A), N1-methyladenosine (m1A), 5-methylcytosine (m5C), internal 7-methylguanosine (m7G), 2′-O-methylation (2′-OMe), pseudouridine (ψ), uridylation, ADP-ribosylation, and adenosine deamination to inosine being some of the most common ones (Barbieri and Kouzarides, 2020). For some of these modifications, the proteins that catalyze the modifications (writers), the ones that remove the modifications (erasers), and the ones that bind specifically to these modifications (readers) have been identified. The most prominent examples of RNA modifications, their writers, readers, and erasers, and their connection with DNA repair can be found in Table 1.
As an example of how some RNA modifications work, we can take the methylation of adenosine at position 6 to give N6-methyladenosine (m6A), arguably the best studied of them. First, a writer complex modifies the RNA substrate. In the case of m6A, it is mostly dependent on the methyltransferase complex formed by METTL3 and METTL14 (Liu et al., 2014) although other writers exist (Pendleton et al., 2017; Ma et al., 2019; van Tran et al., 2019). In agreement with an epitranscriptomic function, m6A methylation is reversible by the action of an eraser, in this case, the demethylases FTO (fat mass and obesity-associated protein) and ALKBH5 (Jia et al., 2011; Zheng et al., 2013). On the other hand, in order to be erased or to perform its varied functions, when a RNA presents m6A, it has to be recognized by a reader. Five components of the YTH domain family (YTHDF1-3 and YTHDC1-2), insulin-like growth factor 2 mRNA-binding protein (IGF2BP), heterogeneous nuclear ribonucleoproteins A2/B1(HNRNPA2B1), and proline-rich and coiled-coil containing protein 2A (PRRC2A) can bind to RNAs bearing the m6A modification (Alarcón et al., 2015; Huang et al., 2018; Liao et al., 2018; Wu et al., 2019) and elicit different responses that have an impact in general on RNA metabolism and other processes such as the ones discussed under DNA repair.
Recently, it has become clear that RNA modifications also affect DNA repair (Ketley and Gullerova, 2020) (Table 1). The first example was the role of m6A RNA methylation in the repair of DNA damage induced by UV facilitating the rapid recruitment of Pol κ to UV-induced DNA damage (Xiang et al., 2017). Roles of m6A RNA methylation in DSB repair have been also described (Zhang et al., 2020a). Indeed, the RNA methyltransferase METTL3, the m6A RNA reader YTHDC1, and m6A-modified RNA accumulate at DSBs promoting HR (Figure 3A). This effect seems to depend on the modulation of stability of DNA:RNA hybrids at DSBs (Zhang et al., 2020a). Along the same lines, Abakir et al. (2020) in a study non-related with DSB induction, showed that most DNA:RNA hybrids are, indeed, m6A modified and that such RNA modifications on R-loops are important for the maintenance of genetic stability in human cells. However, other studies showed that RNA bearing the m6A modification promotes the formation of co-transcriptional R-loops at transcription termination sites to avoid readthrough by the RNA polymerase II (Yang et al., 2019). Albeit these two studies are at odd in the pro- or anti-stability of m6A for the R-loop, something that should be clarified further, they clearly involve such modification in R-loop metabolism. Furthermore, 5-methylcytosine accumulates at damaged sites and helps DNA repair. As for m6A, the m5C role in this process seems to happen in the context of R-loops and requires transcription and the recruitment of a writer factor, TRDMT1, at sites of DNA damage. Importantly, in the absence of TRDMT1, repair by HR is hampered (Chen et al., 2020) (Figure 3A).
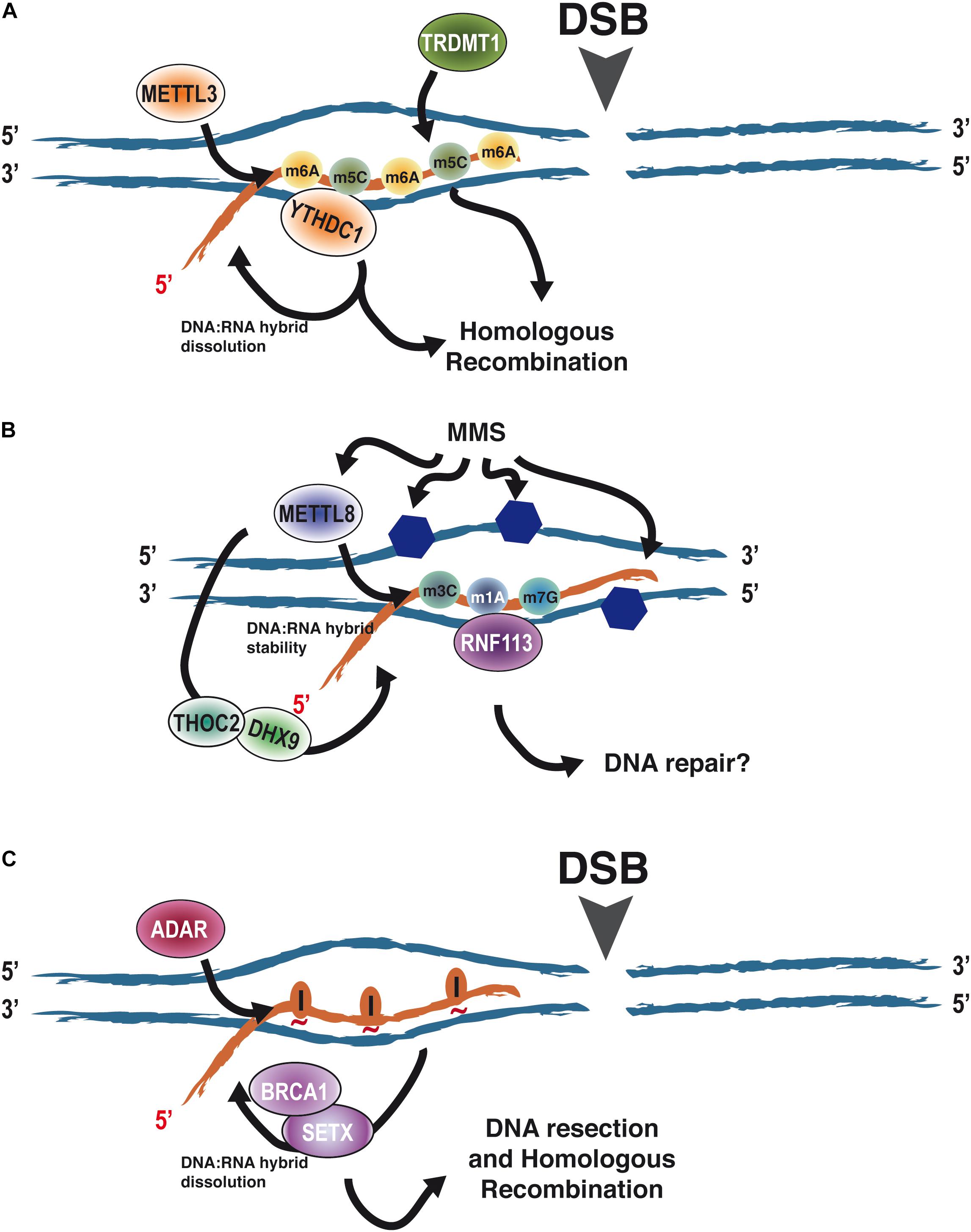
Figure 3. The role of RNA modifications in the context of DNA:RNA hybrids on DNA repair. (A) RNA modifications such as m6A and m5C have been shown to accumulate upon the appearance of a DNA DSB on DNA:RNA hybrids due to the recruitment of the writers METTL3 and TRDMT1. Such modifications promote homologous recombination. At least in the case of m6A, this relies on the regulation of DNA:RNA hybrid stability through the eraser YTHDC1. (B) MMS causes the alkylation of DNA (blue hexagons) and the induction of the modifications m3C, m1A, and m7G on RNA by METTL8. On the other hand, METTL8 has been proposed to modulate DNA:RNA hybrid stability through THOC2 and DHX9, thus opening the possibility of such modifications happening in the context of R-loops. Furthermore, RNF113 has been shown to interact with m3C, m1A, and m7G after MMS treatment, stimulating DNA repair. Whether, in addition to its role in the spliceosome, this happens in the context of R-loops is an open question. (C) The appearance of a DSB stimulates the transient recruitment of ADAR proteins. Its A-to-I editing activity is required to facilitate DNA:RNA hybrid removal by SETX and BRCA1 prior to DNA end resection. We propose that this is mediated by loosening the interaction between the RNA and DNA moieties as inosines will not pair with the opposing thymines (red tilde). Abbreviations: adenosine deaminases acting on RNA.
Less clear are the connections of other RNA modifications with the repair of the DNA molecule. H2O2 treatment, which causes DNA oxidation, causes specific changes in the patterns of N1-methyladenosine on RNA (Li et al., 2016). Additionally, UV-irradiation decreases the cellular levels of m1A in RNAs (Svobodová Kovaříková et al., 2020). Strikingly, this global RNA demethylation is carried out by the eraser ALKBH3, an enzyme that has been involved in DNA repair (Dango et al., 2011; Mohan et al., 2019). Furthermore, MMS treatment induces the association of RNF113A, a ubiquitin ligase involved in the repair of alkylating agents (Brickner et al., 2017), with RNAs harboring the three major MMS-induced modifications mediated by METTL8 (m3C, m1A, and m7G), suggesting a role of such RNA modifications in response to DNA damage caused by alkylating agents (Brickner et al., 2020). Albeit so far RNF113A role in DNA repair seems to be connected with its role in the spliceosome (Shostak et al., 2020). The fact that METTL8 has been also proposed to modulate the R-loop stability through its association with THOC2 and DHX9 (Zhang et al., 2020b) opens the possibility that m1A and/or other METTL8-mediated RNA modifications might also play a role in DNA repair of different DNA lesions as well as DDR by regulating R-loop biology at the breaks (Figure 3B).
Human ALKBH8, which catalyzes methylation of tRNA to form mcm5 U, has also been connected with the DDR. Not only its expression is upregulated upon exposure to DNA damaging agents in an ATM0 dependent fashion but also its depletion sensitizes cells to MMS and bleomycin treatments (Fu et al., 2010). Interestingly, such a role is broadly conserved in evolution (Zdzalik et al., 2014). This function has been associated so far with tRNA modification but opens the possibility that the effect could also be mediated by altering other RNAs.
Additionally, it has been recently demonstrated that RNA can be a target of reversible mono-ADP-ribosylation. Indeed, PARP10, PARP11, and PARP15, as well as a PARP homolog TRPT1, are able to ADP-ribosylate RNA-phosphorylated ends. This ADP-ribosylation of RNA is efficiently reversed in vitro by several ADP-ribosylhydrolases (Munnur et al., 2019). PARylation of proteins by PARP1, 2, and 3 has important roles in DNA repair, both in single-strand breaks (SSBs) and DSBs repair (Caldecott, 2014). So, the role of ADP-ribosylated RNA in the response to DNA breaks is worth exploring in the future.
Another form of RNA modification is RNA editing, caused by the deamination of either C or A, to change the RNA sequence to either U or I, respectively. Unlike other RNA modifications, these are not reversible. However, on the other hand, it can elicit many different responses, such as changes in RNA stability, splicing, or even altering the coding sequence. In principle, every mammalian transcript can be subjected to RNA editing (Gallo et al., 2017). Interestingly, we have observed that A-to-I deamination by ADAR proteins is required for efficient DNA end resection and HR (Jimeno et al., 2021). As for m6A and m5C, it seems to happen in the context of DNA:RNA hybrids, as the phenotype is suppressed by RNaseH overexpression, and is essential for R-loop dissolution and clearance prior DNA end resection. Indeed, our data suggest DNA:RNA hybrids, resulting either from pre-existing transcription or from newly transcribed RNA after DNA damage, block DNA resection. Then, transient recruitment of ADAR to sites of DNA DSBs where its adenine deamination activity is required to, in turn, facilitate hybrid removal by SETX and BRCA1 in order to expedite resection (Jimeno et al., 2021) (Figure 3C).
The Role of RNA Modifications on DSB Repair by DNA:RNA Hybrids Stability
When RNA acts as a donor of information to fulfill HR, a DNA:RNA hybrid has to be formed. These DNA–RNA structures also form as a consequence of damage-induced transcription at DSBs sites. Thus, the formation, stability, and regulation of these DSBs-related R-loops play a central role in many aspects of the DNA repair process (Ohle et al., 2016; Costantino and Koshland, 2018; García-Muse and Aguilera, 2019; Paull, 2019; Puget et al., 2019). As mentioned before, the impact of DNA:RNA hybrids on DNA resection is still under debate since not only negative but also positive roles have been proposed for those structures on this first step of the HR process (Ohle et al., 2016; Costantino and Koshland, 2018; Jimeno et al., 2019; Domingo-Prim et al., 2020).
One way to reconcile these observations is that R-loops might vary their impact on DNA end resection and HR, depending on modifications on the RNA moiety. Thus, RNA modifications of R-loops will bring an extra epitranscriptomic layer of control to DSB repair, affecting the balance between resection-mediated repair, such as HR, and those repair pathways that are resection-independent like NHEJ. This new level of regulation might rely on the stabilization/destabilization of R-loops at DSBs as a response to different cellular cues, such as DNA chromatin structure, cell cycle position, time after the break formation, etc. In this model, R-loops will initially block DNA end resection, providing an initial window of opportunity for NHEJ to act, but if the break cannot be repaired or in the presence of pro-HR signals, the modification of the RNA will facilitate its removal, allowing resection to proceed. Moreover, prior to DNA:RNA dissolution, such modifications could enhance the recruitment of HR proteins, thus explaining why some authors have seen a positive effect of R-loop formation in recombination. So, modifications such as the aforementioned m6A, m5C, or A-to-I editing will facilitate HR (Chen et al., 2020; Zhang et al., 2020a; Jimeno et al., 2021) (Figure 3). To add an extra layer of complexity, it is known that these RNA modifications crosstalk, and, indeed, they regulate each other reciprocally (Xiang et al., 2018). In this scenario, an “R-loop code” based on several RNA modifications could fine-tune DSB repair, controlling the licensing of DNA end resection, thus channeling the DNA ends toward the most appropriate repair route.
RNA Modification, DNA Repair, and Cancer
Defective DNA repair is recognized as a hallmark of cancer cells, as it will increase the mutation rate associated with tumorigenesis (Hanahan and Weinberg, 2011). Strikingly, RNA modifications have also been associated with cancer development (Barbieri and Kouzarides, 2020). Despite that several roles of RNA modification have been already linked with cancer development, we wonder if part of this connection could be due to defective DNA repair when RNA modification levels are altered.
For example, the m6A writer METTL3 has been found to be overexpressed in several cancer types (Lin et al., 2016; Barbieri et al., 2017; Vu et al., 2017; Chen et al., 2018). METTL3-mediated m6A methylation of mRNAs promotes the expression of oncogenes, and the degradation of the mRNAs coding for tumor suppressors participates in essential processes such as cell proliferation, maintenance of undifferentiated phenotype, or the epithelial to mesenchymal transition (Lin et al., 2016; Barbieri et al., 2017; Chen and Yu, 2018; Barbieri and Kouzarides, 2020). But as mentioned, METTL3 also plays a relevant role in DSB repair, thus opening the possibility that some of those phenotypes are indirect, acquired by mutations due to incorrect DNA repair.
The ADAR-mediated adenosine-to-inosine editing has also been linked to several types of cancers. High levels of ADAR1 suppress the immune response and promote cell growth in cancer cells. Therefore, targeting ADAR1 has been proposed as a potential strategy to prevent resistance to immunotherapy (Ishizuka et al., 2019). On the contrary, ADAR2 has been reported to have mainly a tumor suppressor role in cancer. ADAR2 levels are downregulated in the glioblastoma and the high-grade astrocytomas (Cenci et al., 2008; Tomaselli et al., 2013; Gallo et al., 2017). As ADAR-deficient cells are defective in DNA repair (Jimeno et al., 2021), it will be worth exploring if those tumors with overexpression or downregulation of these factors might benefit from specific DNA damage–inducing cancer treatments.
Author Contributions
SJ with the help of FB browsed the literature and collected the source data. SJ with PH wrote the manuscript. All authors contributed to the article and approved the submitted version.
Funding
This work was funded by the R+D+I grant PID2019-104195G from the Spanish Ministry of Science and Innovation-Agencia Estatal de Investigación/10.13039/50110001103. FB is funded by the University of Seville through a postdoctoral contract of the V PPIT-US. CABIMER is supported by the Regional Government of Andalucia (Junta de Andalucía).
Conflict of Interest
The authors declare that the research was conducted in the absence of any commercial or financial relationships that could be construed as a potential conflict of interest.
References
Abakir, A., Giles, T. C., Cristini, A., Foster, J. M., Dai, N., Starczak, M., et al. (2020). N 6-methyladenosine regulates the stability of RNA:DNA hybrids in human cells. Nat. Genet. 52, 48–55. doi: 10.1038/s41588-019-0549-x
Alarcón, C. R., Goodarzi, H., Lee, H., Liu, X., Tavazoie, S., and Tavazoie, S. F. (2015). HNRNPA2B1 Is a Mediator of m6A-Dependent Nuclear RNA Processing Events. Cell 162, 1299–1308. doi: 10.1016/j.cell.2015.08.011
Bader, A. S., and Bushell, M. (2020). DNA:RNA hybrids form at DNA double-strand breaks in transcriptionally active loci. Cell Death Dis. 11:280. doi: 10.1038/s41419-020-2464-6
Bader, A. S., Hawley, B. R., Wilczynska, A., and Bushell, M. (2020). The roles of RNA in DNA double-strand break repair. Br. J. Cancer 122, 613–623. doi: 10.1038/s41416-019-0624-1
Barbieri, I., and Kouzarides, T. (2020). Role of RNA modifications in cancer. Nat. Rev. Cancer 20, 303–322. doi: 10.1038/s41568-020-0253-2
Barbieri, I., Tzelepis, K., Pandolfini, L., Shi, J., Millán-Zambrano, G., Robson, S. C., et al. (2017). Promoter-bound METTL3 maintains myeloid leukaemia by m6A-dependent translation control. Nature 552, 126–131. doi: 10.1038/nature24678
Brickner, J. R., Soll, J. M., Lombardi, P. M., Vågbø, C. B., Mudge, M. C., Oyeniran, C., et al. (2017). A ubiquitin-dependent signalling axis specific for ALKBH-mediated DNA dealkylation repair. Nature 551, 389–393. doi: 10.1038/nature24484
Brickner, J. R., Tsao, N., Rodell, R., Oyeniran, C., and Lukinović, V. (2020). Aberrant RNA methylation triggers recruitment of an alkylation repair complex. bioRxiv [Preprint]. doi: 10.1101/2020.08.28.271874
Burger, K., Schlackow, M., and Gullerova, M. (2019). Tyrosine kinase c-Abl couples RNA polymerase II transcription to DNA double-strand breaks. Nucleic Acids Res. 47, 3467–3484. doi: 10.1093/nar/gkz024
Caldecott, K. W. (2014). Protein ADP-ribosylation and the cellular response to DNA strand breaks. DNA Repair. 19, 108–113. doi: 10.1016/j.dnarep.2014.03.021
Ceccaldi, R., Rondinelli, B., and D’Andrea, A. D. (2016). Repair pathway choices and consequences at the double-strand break. Trends Cell Biol. 26, 52–64. doi: 10.1016/j.tcb.2015.07.009
Cejka, P. (2015). DNA end resection: nucleases team up with the right partners to initiate homologous recombination. J. Biol. Chem. 290, 22931–22938. doi: 10.1074/jbc.R115.675942
Cenci, C., Barzotti, R., Galeano, F., Corbelli, S., Rota, R., Massimi, L., et al. (2008). Down-regulation of RNA editing in pediatric astrocytomas: ADAR2 editing activity inhibits cell migration and proliferation. J. Biol. Chem. 283, 7251–7260. doi: 10.1074/jbc.M708316200
Chakraborty, A., Tapryal, N., Venkova, T., Horikoshi, N., Pandita, R. K., Sarker, A. H., et al. (2016). Classical non-homologous end-joining pathway utilizes nascent RNA for error-free double-strand break repair of transcribed genes. Nat. Commun. 7, 1–12. doi: 10.1038/ncomms13049
Chen, H., Yang, H., Zhu, X., Yadav, T., Ouyang, J., Truesdell, S. S., et al. (2020). m5C modification of mRNA serves a DNA damage code to promote homologous recombination. Nat. Commun. 11, 3–14. doi: 10.1038/s41467-020-16722-7
Chen, M., Wei, L., Law, C. T., Tsang, F. H. C., Shen, J., Cheng, C. L. H., et al. (2018). RNA N6-methyladenosine methyltransferase-like 3 promotes liver cancer progression through YTHDF2-dependent posttranscriptional silencing of SOCS2. Hepatology 67, 2254–2270. doi: 10.1002/hep.29683
Chen, S.-H., and Yu, X. (2018). Human DNA ligase IV is able to use NAD+ as an alternative adenylation donor for DNA ends ligation. Nucleic Acids Res. 47, 1321–1334. doi: 10.1093/nar/gky1202
Cohen, S., Puget, N., Lin, Y. L., Clouaire, T., Aguirrebengoa, M., Rocher, V., et al. (2018). Senataxin resolves RNA:DNA hybrids forming at DNA double-strand breaks to prevent translocations. Nat. Commun. 9:533. doi: 10.1038/s41467-018-02894-w
Costantino, L., and Koshland, D. (2018). Genome-wide Map of R-Loop-Induced damage reveals how a subset of r-loops contributes to genomic instability. Mol. Cell 71, 487.e3–497.e3. doi: 10.1016/j.molcel.2018.06.037
D’Alessandro, G., Whelan, D. R., Howard, S. M., Vitelli, V., Renaudin, X., Adamowicz, M., et al. (2018). BRCA2 controls DNA:RNA hybrid level at DSBs by mediating RNase H2 recruitment. Nat. Commun. 9:5376. doi: 10.1038/s41467-018-07799-2
Daley, J. M., Gaines, W. A., Kwon, Y., and Sung, P. (2014). Regulation of DNA pairing in homologous recombination. Cold Spring Harb. Perspect. Biol. 6:a017954. doi: 10.1101/cshperspect.a017954
Dango, S., Mosammaparast, N., Sowa, M. E., Xiong, L. J., Wu, F., Park, K., et al. (2011). DNA unwinding by ASCC3 helicase is coupled to ALKBH3-dependent DNA alkylation repair and cancer cell proliferation. Mol. Cell 44, 373–384. doi: 10.1016/j.molcel.2011.08.039
Davis, A. J. A., and Chen, D. D. J. (2013). DNA double strand break repair via non-homologous end-joining. Transl. Cancer Res. 2, 130–143. doi: 10.3978/j.issn.2218-676X.2013.04.02
Deveryshetty, J., Peterlini, T., Ryzhikov, M., Brahiti, N., Dellaire, G., Masson, J.-Y., et al. (2019). Novel RNA and DNA strand exchange activity of the PALB2 DNA binding domain and its critical role for DNA repair in cells. eLife 8:e44063. doi: 10.1101/495192
Domingo-Prim, J., Bonath, F., and Visa, N. (2020). RNA at DNA double-strand breaks: the challenge of dealing with DNA:RNA Hybrids. BioEssays 42, 1–7. doi: 10.1002/bies.201900225
Domingo-Prim, J., Endara-Coll, M., Bonath, F., Jimeno, S., Prados-Carvajal, R., Friedländer, M. R., et al. (2019). EXOSC10 is required for RPA assembly and controlled DNA end resection at DNA double-strand breaks. Nat. Commun. 10:2135. doi: 10.1038/s41467-019-10153-9
Esteller, M., and Pandolfi, P. P. (2017). The epitranscriptome of noncoding RNAs in cancer. Cancer Discov. 7, 359–368. doi: 10.1158/2159-8290.CD-16-1292
Francia, S., Cabrini, M., Matti, V., Oldani, A., and d’Adda di Fagagna, F. (2016). DICER, DROSHA and DNA damage response RNAs are necessary for the secondary recruitment of DNA damage response factors. J. Cell Sci. 129, 1468–1476. doi: 10.1242/jcs.182188
Francia, S., Michelini, F., Saxena, A., Tang, D., De Hoon, M., Anelli, V., et al. (2012). Site-specific DICER and DROSHA RNA products control the DNA-damage response. Nature 488, 231–235. doi: 10.1038/nature11179
Fu, D., Brophy, J. A. N., Chan, C. T. Y., Atmore, K. A., Begley, U., Paules, R. S., et al. (2010). Human AlkB Homolog ABH8 Is a tRNA methyltransferase required for wobble uridine modification and DNA damage survival. Mol. Cell. Biol. 30, 2449–2459. doi: 10.1128/mcb.01604-09
Gallo, A., Vukic, D., Michalík, D., O’Connell, M. A., and Keegan, L. P. (2017). ADAR RNA editing in human disease; more to it than meets the I. Hum. Genet. 136, 1265–1278. doi: 10.1007/s00439-017-1837-0
Gao, M., Wei, W., Li, M. M., Wu, Y. S., Ba, Z., Jin, K. X., et al. (2014). Ago2 facilitates Rad51 recruitment and DNA double-strand break repair by homologous recombination. Cell Res. 24, 532–541. doi: 10.1038/cr.2014.36
García-Muse, T., and Aguilera, A. (2019). R loops: from physiological to pathological roles. Cell 179, 604–618. doi: 10.1016/j.cell.2019.08.055
Greene, E. C. (2016). DNA sequence alignment during homologous recombination. J. Biol. Chem. 291, 11572–11580. doi: 10.1074/jbc.R116.724807
Hanahan, D., and Weinberg, R. A. (2011). Hallmarks of cancer: the next generation. Cell 144, 646–674. doi: 10.1016/j.cell.2011.02.013
Huang, H., Weng, H., Sun, W., Qin, X., Shi, H., Wu, H., et al. (2018). Recognition of RNA N 6 -methyladenosine by IGF2BP proteins enhances mRNA stability and translation. Nat. Cell Biol. 20, 285–295. doi: 10.1038/s41556-018-0045-z
Huertas, P. (2010). DNA resection in eukaryotes: deciding how to fix the break. Nat. Struct. Mol. Biol. 17, 11–16. doi: 10.1038/nsmb.1710
Ishizuka, J. J., Manguso, R. T., Cheruiyot, C. K., Bi, K., Panda, A., Iracheta-Vellve, A., et al. (2019). Loss of ADAR1 in tumours overcomes resistance to immune checkpoint blockade. Nature 565, 43–48. doi: 10.1038/s41586-018-0768-9
Jackson, S. P., and Bartek, J. (2009). The DNA-damage response in human biology and disease. Nature 461, 1071–1078. doi: 10.1038/nature08467
Jasin, M., and Rothstein, R. (2013). Repair of strand breaks by homologous recombination. Cold Spring Harb. Perspect. Biol. 5:a012740. doi: 10.1101/cshperspect.a012740
Jia, G., Fu, Y., Zhao, X., Dai, Q., Zheng, G., Yang, Y., et al. (2011). N6-Methyladenosine in nuclear RNA is a major substrate of the obesity-associated FTO. Nat. Chem. Biol. 7, 885–887. doi: 10.1038/nchembio.687
Jimeno, S., Prados-Carvajal, R., Fernandez-Avila, M. J., Silva, S., Silvestris, D. A., Endara-Coll, M., et al. (2021). ADAR2-mediated RNA editing of DNA:RNA hybrids is required for DNA double strand break repair. bioRxiv [Preprint]. doi: 10.1101/2021.03.24.436729
Jimeno, S., Prados-Carvajal, R., and Huertas, P. (2019). The role of RNA and RNA-related proteins in the regulation of DNA double strand break repair pathway choice. DNA Repair. 81:102662. doi: 10.1016/j.dnarep.2019.102662
Keskin, H., Shen, Y., Huang, F., Patel, M., Yang, T., Ashley, K., et al. (2014). Transcript-RNA-templated DNA recombination and repair. Nature 515, 436–439. doi: 10.1038/nature13682
Ketley, R. F., and Gullerova, M. (2020). Jack of all trades? The versatility of RNA in DNA double-strand break repair. Essays Biochem. 64, 721–735. doi: 10.1042/EBC20200008
Kim, J., Sturgill, D., Tran, A. D., Sinclair, D. A., and Oberdoerffer, P. (2015). Controlled DNA double-strand break induction in mice reveals post-damage transcriptome stability. Nucleic Acids Res. 44, 1–10. doi: 10.1093/nar/gkv1482
Li, X., Xiong, X., Wang, K., Wang, L., Shu, X., Ma, S., et al. (2016). Transcriptome-wide mapping reveals reversible and dynamic N1-methyladenosine methylome. Nat. Chem. Biol. 12, 311–316. doi: 10.1038/nchembio.2040
Liao, S., Sun, H., and Xu, C. (2018). YTH Domain: a family of N6-methyladenosine (m6A) Readers. Genomics, Proteomics Bioinforma 16, 99–107. doi: 10.1016/j.gpb.2018.04.002
Lin, S., Choe, J., Du, P., Triboulet, R., and Gregory, R. I. (2016). The m6A methyltransferase METTL3 promotes translation in human cancer Cells. Mol. Cell 62, 335–345. doi: 10.1016/j.molcel.2016.03.021
Liu, J., Yue, Y., Han, D., Wang, X., Fu, Y., Zhang, L., et al. (2014). A METTL3-METTL14 complex mediates mammalian nuclear RNA N6-adenosine methylation. Nat. Chem. Biol. 10, 93–95. doi: 10.1038/nchembio.1432
Lu, W. T., Hawley, B. R., Skalka, G. L., Baldock, R. A., Smith, E. M., Bader, A. S., et al. (2018). Drosha drives the formation of DNA:RNA hybrids around DNA break sites to facilitate DNA repair. Nat. Commun. 9:532. doi: 10.1038/s41467-018-02893-x
Ma, H., Wang, X., Cai, J., Dai, Q., Natchiar, S. K., Lv, R., et al. (2019). N 6-Methyladenosine methyltransferase ZCCHC4 mediates ribosomal RNA methylation. Nat. Chem. Biol. 15, 88–94. doi: 10.1038/s41589-018-0184-3
Manfrini, N., Clerici, M., Wery, M., Colombo, C. V., Descrimes, M., Morillon, A., et al. (2015). Resection is responsible for loss of transcription around a double-strand break in Saccharomyces cerevisiae. eLife 4, 1–15. doi: 10.7554/eLife.08942
Mazina, O. M., Keskin, H., Hanamshet, K., Storici, F., and Mazin, A. V. (2017). Rad52 inverse strand exchange drives RNA-Templated DNA double-strand break repair. Mol. Cell 67, 19.e3–29.e3. doi: 10.1016/j.molcel.2017.05.019
McDevitt, S., Rusanov, T., Kent, T., Chandramouly, G., and Pomerantz, R. T. (2018). How RNA transcripts coordinate DNA recombination and repair. Nat. Commun. 9, 1–10. doi: 10.1038/s41467-018-03483-7
Meers, C., Keskin, H., Banyai, G., Mazina, O., Yang, T., Gombolay, A. L., et al. (2020). Genetic characterization of three distinct mechanisms supporting RNA-driven DNA repair and modification reveals major role of DNA polymerase ζ. Mol. Cell 79, 1037.e5–1050.e5. doi: 10.1016/j.molcel.2020.08.011
Michelini, F., Pitchiaya, S., Vitelli, V., Sharma, S., Gioia, U., Pessina, F., et al. (2017). Damage-induced lncRNAs control the DNA damage response through interaction with DDRNAs at individual double-strand breaks. Nat. Cell Biol. 19, 1400–1411. doi: 10.1038/ncb3643
Mohan, M., Akula, D., Dhillon, A., Goyal, A., and Anindya, R. (2019). Human RAD51 paralogue RAD51C fosters repair of alkylated DNA by interacting with the ALKBH3 demethylase. Nucleic Acids Res. 47, 11729–11745. doi: 10.1093/nar/gkz938
Munnur, D., Bartlett, E., Mikolčević, P., Kirby, I. T., Rack, J. G. M., Mikoč, A., et al. (2019). Reversible ADP-ribosylation of RNA. Nucleic Acids Res. 47, 5658–5669. doi: 10.1093/nar/gkz305
Ohle, C., Tesorero, R., Schermann, G., Dobrev, N., Sinning, I., and Fischer, T. (2016). Transient RNA-DNA hybrids are required for efficient double-strand break repair. Cell 167, 1001.e5–1013.e5. doi: 10.1016/j.cell.2016.10.001
Pankotai, T., Bonhomme, C., Chen, D., and Soutoglou, E. (2012). DNAPKcs-dependent arrest of RNA polymerase II transcription in the presence of DNA breaks. Nat. Struct. Mol. Biol. 19, 276–282. doi: 10.1038/nsmb.2224
Paull, T. T. (2019). RNA–DNA hybrids and the convergence with DNA repair. Crit. Rev. Biochem. Mol. Biol. 54, 371–384. doi: 10.1080/10409238.2019.1670131
Pendleton, K. E., Chen, B., Liu, K., Hunter, O. V., Xie, Y., Tu, B. P., et al. (2017). The U6 snRNA m6A Methyltransferase METTL16 regulates SAM synthetase intron retention. Cell 169, 824.e14–835.e14. doi: 10.1016/j.cell.2017.05.003
Pessina, F., Giavazzi, F., Yin, Y., Gioia, U., Vitelli, V., Galbiati, A., et al. (2019). Functional transcription promoters at DNA double-strand breaks mediate RNA-driven phase separation of damage-response factors. Nat. Cell Biol. 21, 1286–1299. doi: 10.1038/s41556-019-0392-4
Pryde, F., Khalili, S., Robertson, K., Selfridge, J., Ritchie, A., Melton, D. W., et al. (2005). 53BP1 exchanges slowly at the sites of DNA damage and appears to require RNA for its association with chromatin. J. Cell Sci. 118(Pt 9), 2043–2055. doi: 10.1242/jcs.02336
Puget, N., Miller, K. M., and Legube, G. (2019). Non-canonical DNA/RNA structures during transcription-coupled double-strand break repair: roadblocks or Bona fide repair intermediates? DNA Repair. 81:102661. doi: 10.1016/j.dnarep.2019.102661
Radhakrishnan, S. K., Jette, N., and Lees-Miller, S. P. (2014). Non-homologous end joining: emerging themes and unanswered questions. DNA Repair. 17, 2–8. doi: 10.1016/j.dnarep.2014.01.009
Roundtree, I. A., Evans, M. E., Pan, T., and He, C. (2017). Dynamic RNA modifications in gene expression regulation. Cell 169, 1187–1200. doi: 10.1016/j.cell.2017.05.045
Shanbhag, N. M., Rafalska-Metcalf, I. U., Balane-Bolivar, C., Janicki, S. M., and Greenberg, R. A. (2010). ATM-dependent chromatin changes silence transcription in cis to DNA double-strand breaks. Cell 141, 970–981. doi: 10.1016/j.cell.2010.04.038
Sharma, S., Anand, R., Zhang, X., Francia, S., Michelini, F., Galbiati, A., et al. (2021). MRE11-RAD50-NBS1 complex is sufficient to promote transcription by RNA Polymerase II at double-strand breaks by melting DNA ends. Cell Rep. 34:108565. doi: 10.1016/j.celrep.2020.108565
Shelton, S. B., Reinsborough, C., and Xhemalce, B. (2016). Who watches the watchmen: roles of RNA modifications in the RNA interference pathway. PLoS Genet. 12:e1006139. doi: 10.1371/journal.pgen.1006139
Shostak, K., Jiang, Z., Charloteaux, B., Mayer, A., Habraken, Y., Tharun, L., et al. (2020). The X-linked trichothiodystrophy-causing gene RNF113A links the spliceosome to cell survival upon DNA damage. Nat. Commun. 11, 1–21. doi: 10.1038/s41467-020-15003-7
Svobodová Kovaříková, A., Stixová, L., Kovařík, A., Komůrková, D., Legartová, S., Fagherazzi, P., et al. (2020). N6-Adenosine methylation in RNA and a reduced m3G/TMG level in non-coding RNAs appear at microirradiation-induced DNA Lesions. Cells 9:360. doi: 10.3390/cells9020360
Symington, L. S., Bizard, A. H., Hickson, I. D., Schiller, C. B., Seifert, F. U., and Linke-winnebeck, C. (2014). End resection at double-strand breaks: mechanism and regulation end resection at double-strand breaks: mechanism and regulation. Cold Spring Harb. Perspect. 6:a016436. doi: 10.1101/cshperspect.a016436
Thapar, R. (2018). Regulation of DNA double-strand break repair by non-coding RNAs. Molecules 23:2789. doi: 10.3390/molecules23112789
Tomaselli, S., Galeano, F., Massimi, L., Di Rocco, C., Lauriola, L., Mastronuzzi, A., et al. (2013). ADAR2 editing activity in newly diagnosed versus relapsed pediatric high-grade astrocytomas. BMC Cancer 13:255. doi: 10.1186/1471-2407-13-255
van Tran, N., Ernst, F. G. M., Hawley, B. R., Zorbas, C., Ulryck, N., Hackert, P., et al. (2019). The human 18S rRNA m6A methyltransferase METTL5 is stabilized by TRMT112. Nucleic Acids Res. 47, 7719–7733. doi: 10.1016/B978-0-323-60984-5.00062-7
Vítor, A. C., Sridhara, S. C., Sabino, J. C., Afonso, A. I., Grosso, A. R., Martin, R. M., et al. (2019). Single-molecule imaging of transcription at damaged chromatin. Sci. Adv. 5:eaau1249. doi: 10.1126/sciadv.aau1249
Vu, L. P., Pickering, B. F., Cheng, Y., Zaccara, S., Nguyen, D., Minuesa, G., et al. (2017). The N 6 -methyladenosine (m 6 A)-forming enzyme METTL3 controls myeloid differentiation of normal hematopoietic and leukemia cells. Nat. Med. 23, 1369–1376. doi: 10.1038/nm.4416
Wahba, L., Gore, S. K., and Koshland, D. (2013). The homologous recombination machinery modulates the formation of RNA-DNA hybrids and associated chromosome instability. eLife 2013, 1–20. doi: 10.7554/eLife.00505
Wei, L., Nakajima, S., Böhm, S., Bernstein, K. A., Shen, Z., Tsang, M., et al. (2015). DNA damage during the G0/G1 phase triggers RNA-templated, Cockayne syndrome B-dependent homologous recombination. Proc. Natl. Acad. Sci. U.S.A. 112, E3495–E3504. doi: 10.1073/pnas.1507105112
Welty, S., Teng, Y., Liang, Z., Zhao, W., Sanders, L. H., Greenamyre, J. T., et al. (2018). RAD52 is required for RNA-templated recombination repair in post-mitotic neurons. J. Biol. Chem. 293, 1353–1362. doi: 10.1074/jbc.M117.808402
Wu, R., Li, A., Sun, B., Sun, J. G., Zhang, J., Zhang, T., et al. (2019). A novel m6A reader Prrc2a controls oligodendroglial specification and myelination. Cell Res. 29, 23–41. doi: 10.1038/s41422-018-0113-8
Xiang, J. F., Yang, Q., Liu, C. X., Wu, M., Chen, L. L., and Yang, L. (2018). N6-methyladenosines modulate A-to-I RNA editing. Mol. Cell 69, 126.e6–135.e6. doi: 10.1016/j.molcel.2017.12.006
Xiang, Y., Laurent, B., Hsu, C. H., Nachtergaele, S., Lu, Z., Sheng, W., et al. (2017). RNA m6 A methylation regulates the ultraviolet-induced DNA damage response. Nature 543, 573–576. doi: 10.1038/nature21671
Yang, X., Liu, Q. L., Xu, W., Zhang, Y. C., Yang, Y., Ju, L. F., et al. (2019). m6A promotes R-loop formation to facilitate transcription termination. Cell Res. 29, 1035–1038. doi: 10.1038/s41422-019-0235-7
Yasuhara, T., Kato, R., Hagiwara, Y., Shiotani, B., Yamauchi, M., Nakada, S., et al. (2018). Human Rad52 Promotes XPG-Mediated R-loop processing to initiate transcription-associated homologous recombination repair. Cell 175, 558.e11–570.e11. doi: 10.1016/j.cell.2018.08.056
Zdzalik, D., Vågbø, C. B., Kirpekar, F., Davydova, E., Puścian, A., Maciejewska, A. M., et al. (2014). Protozoan ALKBH8 oxygenases display both DNA repair and tRNA modification activities. PLoS One 9:e0098729. doi: 10.1371/journal.pone.0098729
Zhang, C., Chen, L., Peng, D., Jiang, A., He, Y., Zeng, Y., et al. (2020a). METTL3 and N6-methyladenosine promote homologous recombination-mediated repair of DSBs by modulating DNA-RNA Hybrid accumulation. Mol. Cell 79, 425.e7–442.e7. doi: 10.1016/j.molcel.2020.06.017
Zhang, L. H., Zhang, X. Y., Hu, T., Chen, X. Y., Li, J. J., Raida, M., et al. (2020b). The SUMOylated METTL8 Induces R-loop and Tumorigenesis via m3C. iScience 23:100968. doi: 10.1016/j.isci.2020.100968
Zhao, B. S., Roundtree, I. A., and He, C. (2016). Post-transcriptional gene regulation by mRNA modifications. Nat. Rev. Mol. Cell Biol. 18, 31–42. doi: 10.1038/nrm.2016.132
Keywords: RNA modification, DNA repair, homologous recombination, DNA resection, R-loop
Citation: Jimeno S, Balestra FR and Huertas P (2021) The Emerging Role of RNA Modifications in DNA Double-Strand Break Repair. Front. Mol. Biosci. 8:664872. doi: 10.3389/fmolb.2021.664872
Received: 06 February 2021; Accepted: 08 April 2021;
Published: 29 April 2021.
Edited by:
Wenyi Feng, Upstate Medical University, United StatesReviewed by:
Atsushi Shibata, Gunma University, JapanMichael Hendzel, University of Alberta, Canada
Aline Marnef, FR3743 Centre de Biologie Intégrative (CBI), France
Copyright © 2021 Jimeno, Balestra and Huertas. This is an open-access article distributed under the terms of the Creative Commons Attribution License (CC BY). The use, distribution or reproduction in other forums is permitted, provided the original author(s) and the copyright owner(s) are credited and that the original publication in this journal is cited, in accordance with accepted academic practice. No use, distribution or reproduction is permitted which does not comply with these terms.
*Correspondence: Pablo Huertas, cGFibG8uaHVlcnRhc0BjYWJpbWVyLmVz