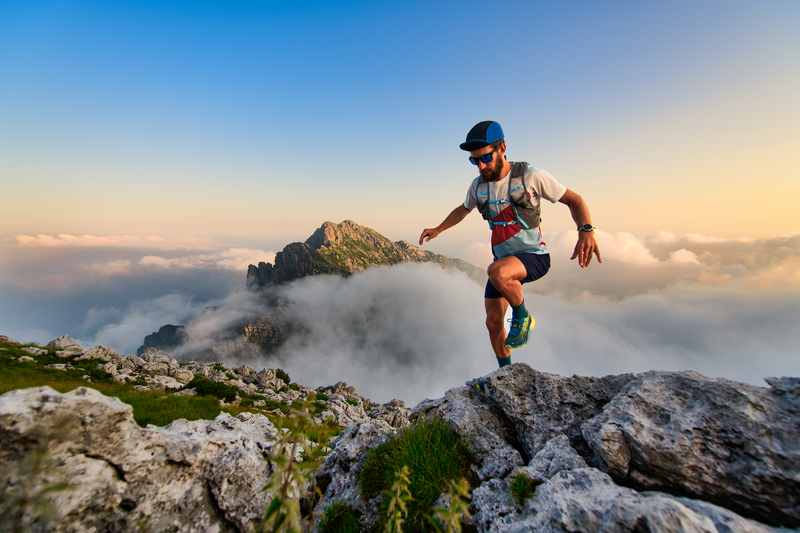
95% of researchers rate our articles as excellent or good
Learn more about the work of our research integrity team to safeguard the quality of each article we publish.
Find out more
MINI REVIEW article
Front. Mol. Biosci. , 28 May 2021
Sec. Molecular Diagnostics and Therapeutics
Volume 8 - 2021 | https://doi.org/10.3389/fmolb.2021.655584
The present review focuses on a dreaded vector-mediated leishmaniasis, with the existing therapeutic approaches including a variety of drugs along with their limitations, the treatment with natural compounds, and different types of metal/metal oxide nanoparticles (NPs). As evidenced, various metallic NPs, comprising silver, silver oxide, gold, zinc oxide, titanium, lead oxide, etc., played a curative role to treat leishmaniasis, are also presented. Keeping in view the advance success of vaccines against the prevalent dreaded diseases in the past and the present scenario, efforts are also being made to develop vaccines based on these NP formulations.
Neglected tropical diseases (NTDs) are contagious diseases that cause substantial illness in more than one billion people globally (Maheshwari and Bandyopadhyay, 2020). Various parasite-mediated diseases comprising giardiasis, Chagas disease, Babesiosis, toxoplasmosis, leishmaniasis, etc., befall in animals and further spread to human population (Oryan, 2015; Hotez et al., 2020). Leishmaniasis is one of the NTDs considered as imperative parasitic diseases, commonly caused by an etiologic agent Leishmania, a genus of trypanosomes. Leishmaniasis is located in the ninth place of the global burden of disease among individual infectious diseases. More than 22 species of infectious Leishmania have been reported (Maheshwari and Bandyopadhyay, 2020). Leishmania are transmitted to mammals through the bite of infected female sandflies belonging to Lutzomyia and Phlebotomus (Oryan and Akbari, 2016). It is endemic in 98 nations of the world, where more than 350 million people are at risk and more than 12 million cases of infection have been reported (Verma and Dey, 2004; Mcgwire and Satoskar, 2014). Based on the species and intensity of infection to the host, it has been classified into cutaneous leishmaniasis (CL), mucocutaneous leishmaniasis (MCL), and visceral leishmaniasis (VL) forms. Considering these classes, CL caused by L. aethiopica is most commonly found in human population and reported to infect 6,00,000 to one million people annually all around the world. It causes severe symptoms like ulcers, serious disabilities, and life-long marks (Surur et al., 2020). Previously, various chemical drugs including liposomal amphotericin B, amphotericin B, pentamidine, pentavalent antimonials, miltefosine, and paromomycin have been practiced against Leishmania. Among these drugs, pentavalent antimonials (sodium stibogluconate and meglumine) are existing chemical drugs, and they are a major therapeutic source to treat Leishmania infection. However, current treatment practices are associated with certain side effects like high toxicity, high cost, and most importantly, development of drug resistance. Hence, there is an instantaneous necessity to innovate new, harmless, and efficient prevention therapies to overcome these limitations (Mitropoulos et al., 2010). Currently, various approaches are involved to control the elevated level of infection, including nanoformulations and targeted drug delivery using nanocarriers as well as with the aid of particular bioactive compound obtained from plants (Javed et al., 2020; Santana et al., 2020). Inventions in nanoscience greatly contribute to overcome the problems allied with the treatment of infectious diseases. Owing to the small size of NPs (1–100 nm), the ability to penetrate easily into the cells, extensive circulation within the body, and the efficient targeted drug delivery system, they can be reflected as a better medication to treat endemic leishmaniasis (Ebrahimi et al., 2017). Further, plant-based nanoparticles have been reported as a successful approach for the preclusion of microbial infections as well as in the treatment of leishmaniasis (Alti et al., 2020). Owing to the green and eco-friendly nature, cost effectiveness, less hazardous nature, and involvement of phytoconstituents for capping and stabilization, plant-based metal oxide nanoparticles (NPs) (silver, zinc, nickel, iron, etc.) have been in use to cure leishmanial infection (Ismail et al., 2019; Alti et al., 2020). The present review focuses on the use of plant-based natural products, the phytosynthesized NPs, to cure Leishmania.
The life cycle of Leishmania parasite begins with the bite of infected female phlebotomine sandflies and is completed in two different morphological forms, that is, promastigote and amastigote. Flagellated metacyclic promastigote, formed in the infected sandflies firstly enter into the macrophages via phagocytosis and formed phagosome. Resultant phagosome enter into the stages of maturation (membrane transformation) and formed a new structure: the parasitophorous vacuole. In this organelle, promastigotes metamorphose into amastigote, followed by its multiplication until explosion of the cells of the macrophage system (4–6°days) spreads infection further. The parasite either initiates infection superficial cells or visceral cell depending on its tropism characteristics. The life cycle of Leishmania into the host is completed when another uninfected sandfly sucks blood as a source of meal. The sensitivity of infection is based on the sandfly species, ecology, epidemiology, and pathogenicity (Bañuls et al., 2007). Further, detailed description of its infection period and transmission is clearly shown in Figure 1.
Leishmaniasis is one of the most important NTDs coupled with various adverse as well as life-threating factors, including substantial morbidity, early death, and long-term infirmity. Treatment comprises control of disease spreading and use of existing parameters, while the currently used therapies including chemical drugs necessitate long-duration therapy and low efficiency with numerous toxic effects. Although no relevant therapies have been developed to prevent the infection which is extensively spread among human population, only few prevention methods are available (Gharirvand Eskandari et al., 2020). Among them, some kind of clinically approved drugs are found to treat this endemic disease, including meglumine antimoniate (glucatime), sodium stibogluconate (pentostan), amphotericin B, and miltefosine. However, the excessive use of these chemotherapeutic sources is associated with antagonistic effects (Ghobakhloo et al., 2016). This has led to the search of some natural methods to treat leishmaniasis.
Since last several years, various kinds of pharmaceutical drugs including amphotericin B, pentamidine, miltefosine, and paromomycin were involved in the treatment of leishmaniasis. None of the clinically approved drugs could be deliberated as the ultimate source of treatment due to their time-taking process and high toxicity combined with severe adversative effects. In addition, the most often used medicines do not eradicate the parasites entirely from all infected entities (De Menezes et al., 2015). Further, applications of some of these medicines with their limitations are described below:
• Pentavalent antimonials can be administrated by the intravenous, intramuscular, and intralymphatic routes with the optimum dosage of 20 mg/kg/day (28–30 days) and exhibited 35–95% potentiality. Continuous and excessive use of this drug causes toxicity like nephrotoxicity, hepatotoxicity, severe cardiotoxicity, and pancreatitis (De Menezes et al., 2015).
• Oral administration of miltefosine not only showed inhibitory effects on the growth of Leishmania but also affected adversely and created severe infecting symptoms comprising nephrotoxicity, teratogenicity, vomiting and diarrhea, and hepatotoxicity (Sundar et al., 2011).
• Paromomycin, also being used as a therapeutic agent to treat leishmaniasis, reported to show some toxic effects during its treatment phase, like severe nephrotoxicity, hepatotoxicity, and ototoxicity (Jhingran et al., 2009).
• Pentamidine with the prime dosage of 3 mg/kg/day can potentially involve in the retardation of Leishmania growth with some severe antagonistic effects such as hypotension, elevated rate of hyperglycemia, tachycardia, pancreatic damage, and electrocardiographic changes (De Menezes et al., 2015).
The existing chemotherapies have a list of short comings comprising high cost, higher toxicity, and acquired resistance toward parasitic strain, and other side effects during their prevention mechanism insisted scientists and medical practitioners to evolve a new therapeutic system to treat NTDs. During the last decades, green therapies involving plant extracts, bioactive compounds, and secondary metabolites derived from particular plant species and different kinds of NPs synthesized using plant extract become promising as well as safer prevention therapies.
From ancient times, plant-based traditional methods are being used in the therapeutics against various infectious ailments. Currently, plant extract and particular bioactive compound extracted from plants are either directly used as a therapeutic source or as derived herbal drugs for the treatment of leishmaniasis as well as other microbial infection (Oryan, 2015).
The consumption of herbal drugs derived from plants is being used from centuries as a prevention source for NTDs as well as other diseases including bacterial (and their vectors), helminth (and their vectors), fungal, ectoparasitic, protozoan (and their vectors), and viral infections (and their vectors). The use of medicinal plants becomes more advantageous over other chemotherapies due to their nontoxic, environment-friendly, and cost-effective properties. Further natural compounds obtained from plants are considered as a reliable therapeutic source to treat leishmaniasis (Cheuka et al., 2017).
Ageratum conyzoides, Bidens pilosa, and Eugenia uniflora showed efficient leishmanicidal effects. Bidens pilosa (root) has been reported for its antileishmanial properties (against L. amazonensis, promastigote) with the least IC50 value (1.5 μg/ml) as compared to other plant species (Table 1). Essential oils from Eugenia uniflorae potentially inhibit the growth of both the parasitic forms, that is, promastigote and amastigote, of L. amazonensis, and Ageratum conyzoides has been reported to treat infection caused by L. donovani (amastigote form) (Silveira et al., 2021). E-caryophyllene, the main component of Melampodium divaricatum and Casearia sylvestris essential oil, has been reported for its promising antileishmanial response against L. amazonensis (IC50 values of 10.7, 10.7, and 14.0 μg/ml) (Moreira et al., 2019). Moreover, 1,8-cineole, α-pinene, and p-cymene active constituents of Protium altsonii and P. hebetatum (Burseraceae) exhibited dose-dependent amastigote inhibition with IC50 of 48.4, 37, and 46 μg/ml, respectively (Santana et al., 2020). Butanol fraction of K. odoratissima with 154.1 μg/ml IC50 value showed antileishmanial properties against L. major promastigote and amastigote (Mirzaei et al., 2020).
Methods in controlling infectious diseases have modernized translational sciences to develop a better controlling method for infectious diseases. The field of nanomedicine has shown enormous potential in developing highly sensitive diagnostic tools with excellent drug delivery properties. Recently, nanoparticle-conjugated drugs have increasingly been studied as an alternative, cost-effective therapy with increased effectiveness. However, toxicity is a major barrier that needs to be encountered. Several reports have shown the effective antimicrobial activities of various metal/metal oxide nanoparticles as well as against the Leishmania causing organism through their wide surface area and unique properties.
Nanoparticles synthesized using crude as well as various solvent-fractionated extracts of medically important plants are considered as efficient agents for the delivery of specific phytoconstituents into the cells. Keeping in view the effective antimicrobial activities of silver metal, silver/silver oxide NPs have been synthesized using a variety of medicinally important plant species, including Mentha arvensis L., Ficus benghalensis, Cuminum cyminum, Moringa oleifera, Silybum marianum, and Sechium edule, at a dosage of 10, 300, 0.5, 246, and 51.88 μg/ml tested against L. tropica, L. donovani, L. tropica, L. major, L. tropica, and Leishmania donovani, respectively (Baranwal et al., 2018; El-Khadragy et al., 2018; Hameed et al., 2019; Ismail et al., 2019; Bagirova et al., 2020; Javed et al., 2020). Gold and silver bimetallic NPs synthesized by using medically important plants have also been reported to be used for the prevention of this disease (Alti et al., 2020). However, Cannabis sativa–based Au-NPs accomplished virtuous antileishmanial activity against amastigote forms (IC50: 171·00 ± 2·28 μg/ml) (Hameed et al., 2020). 7, 8-dihydroxyflavone, a type of flavonoid found abundantly in plants used to produce gold nanoparticles, has also been reported to preclude leishmaniasis (Prasanna et al., 2020).
Zno-NPs were also reported to show dose-dependent cytotoxicity against L. tropica (IC50: 8.30 μg/ml) (Iqbal et al., 2019). Rod-shaped zinc oxide NPs produced by using Lilium ledebourii tuber extract potentially inhibited the growth of L. major with the IC50 value of 0.001 mg ml−1 (Khatami et al., 2020). Saleh (2019) also concluded that green TiO2 nanoparticles have shown effective roles to counter noxiousness of Leishmania tropica in male rats. Hematite (Fe2O3) NPs fabricated with the Rhus punjabensis extract played an efficient role in the treatment of leishmaniasis (Naz et al., 2019). Khalil et al. (2020) prepared lead oxide NPs (PbO-NPs) by green route using aqueous leaf extracts of Sageretia thea. The experimental data showed that PbO-NPs were significantly active in arresting the growth of promastigote and amastigote forms of Leishmania tropica, with 14.7 μg/ml and 11.95 μg/ml IC50 values, respectively.
Plant-mediated (Trigonella foenum-graecum) iron oxide nanoparticles have been reported to exhibit significant inhibitory effects on L. tropica (Ain et al., 2019). Further, Abbasi et al. (2019) also stated the antileishmanial efficacy of Nio-NPs fabricated by using Geranium wallichianum against L. tropica.
Besides, the nanostructured drug delivery system was also reported in ameliorate NTDs including leishmaniasis. Furthermore, crude plant extracts and precise phytoconstituents obtained from plant which is involved in the prevention mechanism were also loaded in the nanostructured drug delivery system and used as a therapeutic source to cure leishmaniasis, and the mechanism is depicted below:
• Liposome NPs consisting of phospholipids are assisted as a transport system for the delivery of hydrophilic as well as lipophilic pharmaceutical drugs (Momeni et al., 2013). They provide improved pharmacokinetic assets along with target diligence which offers a foremost advantage (Kaye and Scott, 2011). Liposome can spear the macrophages through phagocytosis and offers direct delivery of the drugs at their targeted sites. Different drug formulations including AmB colloidal formulations, liposomal AmB, and AmB lipid network can overwhelm the toxic effects of conventional drugs (Moreno et al., 2015). Liposome-encapsulated Curcuma longa and Combretum leprosum extracts were also reported for their antileishmanial properties (Aditya et al., 2012; Barros et al., 2013).
• Beta-lapachone extracted from Lapacho tree with the use of lecithin-chitosan NP encapsulation method has been reported in the treatment of leishmaniasis (Moreno et al., 2015).
• 8-hydroxyquinoline with the polymeric micelle encapsulation method has been used to treat Leishmania (Duarte et al., 2016). Berberin, an isoquinoline alkaloid extracted from medicinal plants, has been reported to possess various biologic properties, including antileishmanial properties. A previous study addressed the preparation of BER-loaded liposomes with the aim to prevent its rapid liver metabolism and improve the drug selective delivery to the infected organs in visceral leishmaniasis (VL) (Calvo et al., 2020).
As per the literature survey, plant-based nanoparticles contribute efficient roles in the treatment of leishmaniasis as compared to other existing practices. Phytosynthesized NPs revealed an identical effect on the inhibition of parasitic growth at a comparatively lesser concentration than the prescribed dose of Amp B to cure this disease. Additionally, bimetallic nanoparticles including Au−Ag, Zn−Ag, and Ti−Ag were synthesized using the green approach and proficiently used as a therapeutic source to treat leishmaniasis (Alti et al., 2020). NPs are preferred over other therapeutic sources to treat this dreaded disease because of their nontoxic, harmless, and efficient delivery system for vaccine. Currently, with the advancement of nanosciences, there is a new method of synthesizing vaccines using NPs as carriers of antigen preparation. Solid lipid nanoparticles can assist as an effective tool to produce leishmanial vaccine (Saljoughian et al., 2013). However, any kind of NP-based vaccine is not accessible, and it needs more consideration.
Leishmania sp. are protozoal parasites which result in cutaneous and visceral leishmaniasis. Different clinical studies exhibit the development of self-curable to detrimental conditions, depending upon the immune responses triggered by the affected host (Noormehr et al., 2018). Chemotherapy with pentavalent antimonials (like sodium stibogluconate or meglumine antimoniate) and other antileishmanial drugs (amphotericin B, fluconazole, pentamidine, and miltefosine) are optimal for leishmanial therapy. However, due to adverse effects, high cost, difficult infusion routes, low cure, and increasing resistance are of significant concern in developing more efficient ways in leishmaniasis therapy. Moreover, the efficacy of the drug used in the treatment also varies for different leishmanial sp. (Noormehr et al., 2018; Alti et al., 2020; Calvo et al., 2020). In self-treatment, the innate immune cells (phagocytes) detect and engulf the causal agents, which induces Leishmania assassination by producing reactive oxygen species, nitric oxide, and tumor necrosis factors (Olekhnovitch et al., 2014). After innate immune responses, respective activation and production of CD8+, NK, and IFN cells by TH1 immunity results in killing of Leishmania parasites (Noormehr et al., 2018). In susceptible conditions, the defense system fails to overcome infections, and follows incorrect TH2 immune responses along with antibody response, which is the key factor to generate new ways of parasite elimination. Metal nanoparticles inhibit proliferation and viability of infected cells, which is contingent with the NP strength and time of exposure (Rosas-Hernández et al., 2009; Fanti et al., 2018).
Several in vitro as well as in vivo findings suggest leishmanicidal effects of bio–Ag-NPs by direct (exclusive of inflammatory mediators) or indirect (immunomodulatory) mechanisms (Fanti et al., 2018; Calvo et al., 2020). In the direct method, metal-NPs kill the parasitic cells by causing vacuolation inside parasites and damage to the cellular membrane without generating immunomodulatory intermediaries, that is, reactive oxygen species (ROS), nitric oxide (NO), and apoptotic and necrotic factors (Fanti et al., 2018). In situations when Leishmania parasites override the oxidative burst inside phagocytic cells and reside in phagolysosomes, nanoformulations assist site-specific delivery and accumulation of drugs, which is responsible for parasite killing (Shoaib Sarwar et al., 2020). According to Fanti et al. (2018), Ag-NPs after diffusion through the cellular membranes get oxidized due to acidic conditions within the phagolysosomes, and eventually, the release of free Ag + ions causes parasite assassination.
On the other hand, the indirect method involves immunomodulatory response generation at infection sites. Other ways to provide leishmanicidal effects are through activating immune response mediators in which the cell viability and proliferation get declined as an effect of metallic nanoparticles. NPs basically induce different morphological abrasions such as distorted membranal integrity, cytotoxicity, mitochondrial destruction, cell cycle arrest (G1), increased/decreased ROS and NO generation, affected enzymatic activities, and release of apoptotic or necrotic factors (Park et al., 2010; Kruszewski et al., 2011; Zahir et al., 2015). As a result of mitochondrial disintegration, ATP generation gets influenced, which causes cytotoxic effects, and ultimately affects the infection growth (AshaRani et al., 2009). Moreover, NP exposure exhibits decreased parasitic load and reduction in an essential parasitic enzyme trypanothione reductase system (Fanti et al., 2018).
Chemotherapy due to lack of effective therapies till date has become the only choice in treating leishmaniasis, as these therapies exhibit higher toxicity levels, treatment cost, and resistance development against leishmanial parasites, and encourage other side effects. In addition, it is evident that the efficiency of drugs varies from species to species due to leishmanial antigen variants and different immunological responses against the drug. To overcome these challenges, biogenic nanomaterials being nontoxic, bio-compatible, cost effective, and having high targeted drug-loading potentials have been indicated as beneficial alternatives to formulate nanovaccines. Targeted drug delivery barriers can be conquered by using nanoformulations for enhanced parasiticidal proficiencies. Also, various studies have demonstrated leishmanicidal activities of plant-derived natural compounds (such as berberine, 7, 8-dihydroxyflavone, E-caryophyllene, essential oil constituents, α-terpineol, glycosides, tannins, and anthraquinone flavonoids), which can further integrate beneficial outcomes. Besides, most of the studies conducted on leishmanicidal activities revealed only the basic outcomes like assessment of the effect of test drugs (crude extract, isolated bioactive compounds, essential oil, and purified fraction) on the parasite growth. Few of them identify the proper formulation as well as the effect on the promastigote stage, found in the sandflies (vector). As widely conferred in the literature, plants possess a variety of bioactive compounds, and most of them have been reported for their pharmaceutical properties. Thus, the standardization may conclude the identification of particular compound responsible for leishmanicidal activities. Biosynthesized nanoparticles majorly eliminate the infection either by triggering the immunomodulatory response of the host or sometimes directly by resulting in vacuolization of parasitic cells, leading to parasite killing. Nanovaccines are a relatively new concept in treating Leishmania although no vaccine is yet available, but studies are ongoing to find efficient nanovaccines. Although nanotechnology has provided a hope toward improved and successful eradication of neglected tropical diseases, the accurate molecular mechanism responsible still needs thorough transparency to bring utmost benefits.
LS: writing original draft and editing; MD: writing, review, and editing; AS: conceptualization and validation; MS: supervision, conceptualization, and validation.
The authors declare that the research was conducted in the absence of any commercial or financial relationships that could be construed as a potential conflict of interest.
The work supported by Manipal University Jaipur is gratefully acknowledged. Authors are obliged to Manipal University Jaipur for necessary support and facilities.
The Supplementary Material for this article can be found online at: https://www.frontiersin.org/articles/10.3389/fmolb.2021.655584/full#supplementary-material.
Abbasi, B. A., Iqbal, J., Mahmood, T., Ahmad, R., Kanwal, S., and Afridi, S. (2019). Plant-mediated Synthesis of Nickel Oxide Nanoparticles (NiO) via Geranium Wallichianum: Characterization and Different Biological Applications. Mater. Res. Express 6, 0850a7. doi:10.1088/2053-1591/ab23e1
Aditya, N. P., Chimote, G., Gunalan, K., Banerjee, R., Patankar, S., and Madhusudhan, B. (2012). Curcuminoids-loaded Liposomes in Combination with Arteether Protects against Plasmodium Berghei Infection in Mice. Exp. Parasitol. 131, 292–299. doi:10.1016/j.exppara.2012.04.010
Ain, Q. U., Islam, A., Nadhman, A., and Yasinzai, M. (2019). Comparative Analysis of Chemically and Biologically Synthesized Iron Oxide Nanoparticles against Leishmania Tropica. bioRxiv. doi:10.1101/829408
Alkhaldi, A. A. M., Musa, A., Mostafa, E. M., Amin, E., and De Koning, H. P. (2020). Docking Studies and Antiprotozoal Activity of Secondary Metabolites Isolated from Scrophularia Syriaca Benth. Growing in Saudi Arabia. Rec.Nat.Prod. 14, 23–30. doi:10.25135/rnp.19.03.1224
Alti, D., Veeramohan Rao, M., Rao, D. N., Maurya, R., and Kalangi, S. K. (2020). Gold-Silver Bimetallic Nanoparticles Reduced with Herbal Leaf Extracts Induce ROS-Mediated Death in Both Promastigote and Amastigote Stages of Leishmania Donovani. ACS Omega 5, 16238–16245. doi:10.1021/acsomega.0c02032
Amin, A., Tuenter, E., Exarchou, V., Upadhyay, A., Cos, P., Maes, L., et al. (2016). Phytochemical and Pharmacological Investigations onNymphoides indicaLeaf Extracts. Phytother. Res. 30, 1624–1633. doi:10.1002/ptr.5663
Amoa-Bosompem, M., Ohashi, M., Mosore, M.-T., Agyapong, J., Tung, N. H., Kwofie, K. D., et al. (2016). In Vitro anti-leishmania Activity of Tetracyclic Iridoids from Morinda Lucida, Benth. Trop. Med. Health 44. 25. doi:10.1186/s41182-016-0026-5
Araújo, I. A. C., de Paula, R. C., Alves, C. L., Faria, K. F., Oliveira, M. M. d., Mendes, G. G., et al. (2019). Efficacy of Lapachol on Treatment of Cutaneous and Visceral Leishmaniasis. Exp. Parasitol. 199, 67–73. doi:10.1016/j.exppara.2019.02.013
AshaRani, P. V., Low Kah Mun, G., Hande, M. P., and Valiyaveettil, S. (2009). Cytotoxicity and Genotoxicity of Silver Nanoparticles in Human Cells. ACS Nano 3, 279–290. doi:10.1021/nn800596w
Badirzadeh, A., Heidari-Kharaji, M., Fallah-Omrani, V., Dabiri, H., Araghi, A., and Salimi Chirani, A. (2020). Antileishmanial Activity of Urtica Dioica Extract against Zoonotic Cutaneous Leishmaniasis. Plos Negl. Trop. Dis. 14, e0007843. doi:10.1371/journal.pntd.0007843
Bagirova, M., Dinparvar, S., Allahverdiyev, A. M., Unal, K., Abamor, E. S., and Novruzova, M. (2020). Investigation of Antileshmanial Activities of Cuminum Cyminum Based green Silver Nanoparticles on L. Tropica Promastigotes and Amastigotes In Vitro. Acta Tropica 208, 105498. doi:10.1016/j.actatropica.2020.105498
Baker, B. J., Witowski, C. G., Maschek, J. A., Vesely, B., and Kyle, D. E. (2016). Diterpenoide Membranolide Compounds Having Anti-leishmania Acitivity and Uses Thereof. US 20160030388
Bañuls, A. L., Hide, M., and Prugnolle, F. (2007). Leishmania and the Leishmaniases: A Parasite Genetic Update and Advances in Taxonomy, Epidemiology and Pathogenicity in Humans. Adv. Parasitol. 64, 1–458. doi:10.1016/S0065-308X(06)64001-3
Baranwal, A., Chiranjivi, A. K., Kumar, A., Dubey, V. K., and Chandra, P. (2018). Design of Commercially Comparable Nanotherapeutic Agent against Human Disease-Causing Parasite, Leishmania. Sci. Rep. 8. 8814. doi:10.1038/s41598-018-27170-1
Barros, N. B., Migliaccio, V., Facundo, V. A., Ciancaglini, P., Stábeli, R. G., Nicolete, R., et al. (2013). Liposomal-lupane System as Alternative Chemotherapy against Cutaneous Leishmaniasis: Macrophage as Target Cell. Exp. Parasitol. 135, 337–343. doi:10.1016/j.exppara.2013.07.022
Bello, O., Ogbesejana, A., and Osibemhe, M. (2018). Iridoid Glucosides from Vitex Grandifolia Displayed Anti-inflammatory and Antileishmania Effects and Structure Activity Relationship. J. Appl. Sci. Environ. Manag. 22, 373. doi:10.4314/jasem.v22i3.14
Calvo, A., Moreno, E., Larrea, E., Sanmartín, C., Irache, J. M., and Espuelas, S. (2020). Berberine-loaded Liposomes for the Treatment of Leishmania Infantum-Infected Balb/c Mice. Pharmaceutics 12, 858. doi:10.3390/pharmaceutics12090858
Cheuka, P., Mayoka, G., Mutai, P., and Chibale, K. (2017). The Role of Natural Products in Drug Discovery and Development against Neglected Tropical Diseases. Molecules 22, 58. doi:10.3390/molecules22010058
Chowdhury, S. R., Kumar, A., Godinho, J. L. P., De Macedo Silva, S. T., Zuma, A. A., Saha, S., et al. (2017). Voacamine Alters Leishmania Ultrastructure and Kills Parasite by Poisoning Unusual Bi-subunit Topoisomerase IB. Biochem. Pharmacol. 138, 19–30. doi:10.1016/j.bcp.2017.05.002
Dagnino, A. P. A., Mesquita, C. S., Dorneles, G. P., Teixeira, V. D. O. N., De Barros, F. M. C., Vidal Ccana-Ccapatinta, G., et al. (2018). Phloroglucinol Derivatives from hypericum Species Trigger Mitochondrial Dysfunction in Leishmania Amazonensis. Parasitology 145, 1199–1209. doi:10.1017/S0031182018000203
Dagnino, A. P., Barros, F. M. C. d., Ccana-Ccapatinta, G. V., Prophiro, J. S., Poser, G. L. v., and Romão, P. R. T. (2015). Leishmanicidal Activity of Lipophilic Extracts of Some Hypericum Species. Phytomedicine 22, 71–76. doi:10.1016/j.phymed.2014.10.004
De Menezes, J. P. B., Guedes, C. E. S., Petersen, A. L. d. O. A., Fraga, D. B. M., and Veras, P. S. T. (2015). Advances in Development of New Treatment for Leishmaniasis. Biomed. Res. Int. 2015, 1–11. doi:10.1155/2015/815023
Duarte, M. C., Lagedos, L. M. d. R. R., Lage, D. P., Martins, V. T., Carvalho, A. M. R. S., Roatt, B. M., et al. (2016). Treatment of Murine Visceral Leishmaniasis Using an 8-Hydroxyquinoline-Containing Polymeric Micelle System. Parasitol. Int. 65, 728–736. doi:10.1016/j.parint.2016.07.005
Ebrahimi, K., Shiravand, S., and Mahmoudvand, H. (2017). Biosynthesis of Copper Nanoparticles Using Aqueous Extract of Capparis Spinosa Fruit and Investigation of its Antibacterial Activity. mpj 21, 866–871. doi:10.12991/mpj.2017.31
El-Khadragy, M., Alolayan, E., Metwally, D., El-Din, M., Alobud, S., Alsultan, N., et al. (2018). Clinical Efficacy Associated with Enhanced Antioxidant Enzyme Activities of Silver Nanoparticles Biosynthesized Using Moringa Oleifera Leaf Extract, against Cutaneous Leishmaniasis in a Murine Model of Leishmania Major. Ijerph 15, 1037. doi:10.3390/ijerph15051037
Fanti, J. R., Tomiotto-Pellissier, F., Miranda-Sapla, M. M., Cataneo, A. H. D., Andrade, C. G. T. d. J., Panis, C., et al. (2018). Biogenic Silver Nanoparticles Inducing Leishmania Amazonensis Promastigote and Amastigote Death In Vitro. Acta Tropica 178, 46–54. doi:10.1016/j.actatropica.2017.10.027
Ghaffarifar, F., Esavand Heydari, F., Dalimi, A., Hassan, Z. M., Delavari, M., and Mikaeiloo, H. (2015). Evaluation of Apoptotic and Antileishmanial Activities of Artemisinin on Promastigotes and BALB/C Mice Infected with Leishmania Major. Iran. J. Parasitol.
Gharirvand Eskandari, E., Setorki, M., and Doudi, M. (2020). Medicinal Plants with Antileishmanial Properties: A Review Study. Pbr 6(11), 1–16. doi:10.18502/pbr.v6i1.3422
Ghobakhloo, N., Motazedian, M. H., and Fardaei, M. (2016). Expression Analysis of Multiple Genes May Involve in Antimony Resistance Among Leishmania Major Clinical Isolates from Fars Province, Central Iran. Iran. J. Parasitol. 11(2), 168–176.
Glaser, J., Schultheis, M., Moll, H., Hazra, B., and Holzgrabe, U. (2015). Antileishmanial and Cytotoxic Compounds from Valeriana Wallichii and Identification of a Novel Nepetolactone Derivative. Molecules 20, 5740–5753. doi:10.3390/molecules20045740
Hajaji, S., Sifaoui, I., López-Arencibia, A., Reyes-Batlle, M., Jiménez, I. A., Bazzocchi, I. L., et al. (2018). Leishmanicidal Activity of α-bisabolol from Tunisian Chamomile Essential Oil. Parasitol. Res. 117, 2855–2867. doi:10.1007/s00436-018-5975-7
Hameed, S., Ali Shah, S., Iqbal, J., Numan, M., Muhammad, W., Junaid, M., et al. (2020). Cannabis Sativa-Mediated Synthesis of Gold Nanoparticles and Their Biomedical Properties. Bioinspired, Biomimetic and Nanobiomaterials 9, 95–102. doi:10.1680/jbibn.19.00023
Hameed, S., Khalil, A. T., Ali, M., Numan, M., Khamlich, S., Shinwari, Z. K., et al. (2019). Greener Synthesis of ZnO and Ag-ZnO Nanoparticles Using Silybum marianum for Diverse Biomedical Applications. Nanomedicine 14, 655–673. doi:10.2217/nnm-2018-0279
Hotez, P. J., Aksoy, S., Brindley, P. J., and Kamhawi, S. (2020). World Neglected Tropical Diseases Day. Plos Negl. Trop. Dis. 14, e0007999–4. doi:10.1371/JOURNAL.PNTD.0007999
Iqbal, J., Abbasi, B. A., Mahmood, T., Kanwal, S., Ahmad, R., and Ashraf, M. (2019). Plant-extract Mediated green Approach for the Synthesis of ZnONPs: Characterization and Evaluation of Cytotoxic, Antimicrobial and Antioxidant Potentials. J. Mol. Struct. 1189, 315–327. doi:10.1016/j.molstruc.2019.04.060
Ismail, H. H., Hasoon, S. A., and Saheb, E. J. (2019). The Anti- Leishmaniasis Activity of green Synthesis Silver Oxide Nanoparticles. Ann. Trop. Med. Public Heal 22, 28–38. doi:10.36295/ASRO.2019.22044
Jesus, J. A., Fragoso, T. N., Yamamoto, E. S., Laurenti, M. D., Silva, M. S., Ferreira, A. F., et al. (2017). Therapeutic Effect of Ursolic Acid in Experimental Visceral Leishmaniasis. Int. J. Parasitol. Drugs Drug Resist 7 (11), 1–11.
Javed, B., Raja, N. I., Nadhman, A., and Mashwani, Z.-u. -R. (2020). Understanding the Potential of Bio-Fabricated Non-oxidative Silver Nanoparticles to Eradicate Leishmania and Plant Bacterial Pathogens. Appl. Nanosci. 10, 2057–2067. doi:10.1007/s13204-020-01355-5
Jhingran, A., Chawla, B., Saxena, S., Barrett, M. P., and Madhubala, R. (2009). Paromomycin: Uptake and Resistance in Leishmania Donovani. Mol. Biochem. Parasitol. 164, 111–117. doi:10.1016/j.molbiopara.2008.12.007
KarimiPourSaryazdi, A., Ghaffarifar, F., Dalimi, A., and Dayer, M. S. (2020). In-vitro and In-Vivo Comparative Effects of the spring and Autumn-Harvested Artemisia Aucheri Bioss Extracts on Leishmania Major. J. Ethnopharmacology 257, 112910. doi:10.1016/j.jep.2020.112910
Kaye, P., and Scott, P. (2011). Leishmaniasis: Complexity at the Host-Pathogen Interface. Nat. Rev. Microbiol. 9, 604–615. doi:10.1038/nrmicro2608
Khalil, A. T., Ovais, M., Ullah, I., Ali, M., Jan, S. A., Shinwari, Z. K., et al. (2020). Bioinspired Synthesis of Pure Massicot Phase lead Oxide Nanoparticles and Assessment of Their Biocompatibility, Cytotoxicity and In-Vitro Biological Properties. Arabian J. Chem. 13, 916–931. doi:10.1016/j.arabjc.2017.08.009
Khatami, M., Khatami, S., Mosazade, F., Raisi, M., Haghighat, M., Sabaghan, M., et al. (2020). Greener Synthesis of Rod Shaped Zinc Oxide NPs Using Lilium Ledebourii Tuber and Evaluation of Their Leishmanicidal Activity. Iranj. Biotechnol. 18. e2196. doi:10.30498/ijb.2020.119481.2196
Kruszewski, M., Brzoska, K., Brunborg, G., Asare, N., Dobrzyńska, M., Dušinská, M., et al. (2011). “Toxicity of Silver Nanomaterials in Higher Eukaryotes”. Advances in Molecular Toxicology, 5, 179–218. doi:10.1016/B978-0-444-53864-2.00005-0
Kyriazis, I. D., Koutsoni, O. S., Aligiannis, N., Karampetsou, K., Skaltsounis, A.-L., and Dotsika, E. (2016). The Leishmanicidal Activity of Oleuropein Is Selectively Regulated through Inflammation- and Oxidative Stress-Related Genes. Parasites Vectors 9. 441. doi:10.1186/s13071-016-1701-4
Lage, P. S., Chávez-Fumagalli, M. A., Mesquita, J. T., Mata, L. M., Fernandes, S. O. A., et al. (2015). Antileishmanial activity and evaluation of the mechanism of action of strychnobiflavone flavonoid isolated from Strychnos pseudoquina against Leishmania infantum. Parasitol. Res. 114 (12), 4625-4635. doi:10.1007/s00436-015-4708-4
Macêdo, C. G., Fonseca, M. Y. N., Caldeira, A. D., Castro, S. P., Pacienza-Lima, W., Borsodi, M. P. G., et al. (2020). Leishmanicidal Activity of Piper Marginatum Jacq. From Santarém-PA against Leishmania Amazonensis. Exp. Parasitol. 210, 107847. doi:10.1016/j.exppara.2020.107847
Maheshwari, K. K., and Bandyopadhyay, D. (2021). Heterocycles in the Treatment of Neglected Tropical Diseases. Cmc 28, 472–495. doi:10.2174/0929867327666200219141652
Maina, E. N. M., Njau, V. N., and Gavamukulya, Y. (2020). Phytochemical Analysis and Antileishmanial Activity of Clerodendrum Myricoides and Salvadora Persica Plant Extracts against Leishmania Major. Jocamr 9(11), 29–44. doi:10.9734/jocamr/2020/v9i130134
Maurya, R., and Chandrasekaran, S. (2017). A Novel Synergistic Composition for the Treatment of Visceral Leishmaniasis. WO 2017046778.
Mcgwire, B. S., and Satoskar, A. R. (2014). Leishmaniasis: Clinical Syndromes and Treatment. QJM 107, 7–14. doi:10.1093/qjmed/hct116
Metwally, D. M., Al-Olayan, E. M., El-Khadragy, M. F., and Alkathiri, B. (2016). Anti-leishmanial Activity (In Vitro and In Vivo) of Allicin and Allicin Cream Using Leishmania Major (Sub-strain Zymowme LON4) and Balb/c Mice. PLoS One 11, e0161296. doi:10.1371/journal.pone.0161296
Mirzaei, F., Norouzi, R., Siyadatpanah, A., Mitsuwan, W., Nilforoushzadeh, M., Maleksabet, A., et al. (2020). Butanol Fraction of Kelussia Odoratissima Mozaff Inhibits the Growth of Leishmania Major Promastigote and Amastigote. jwpr 10, 254–259. doi:10.36380/scil.2020.wvj33
Mitropoulos, P., Konidas, P., and Durkin-Konidas, M. (2010). New World Cutaneous Leishmaniasis: Updated Review of Current and Future Diagnosis and Treatment. J. Am. Acad. Dermatol. 63, 309–322. doi:10.1016/j.jaad.2009.06.088
Moawad, A., Hassan, H., and Amin, E. (2016). Biologically-Guided Isolation of Leishmanicidal Secondary Metabolites from Euphorbia Peplus L. Planta Med. 82, PC47. doi:10.1055/s-0036-1578749
Momeni, A., Rasoolian, M., Momeni, A., Navaei, A., Emami, S., Shaker, Z., et al. (2013). Development of Liposomes Loaded with Anti-leishmanial Drugs for the Treatment of Cutaneous Leishmaniasis. J. Liposome Res. 23, 134–144. doi:10.3109/08982104.2012.762519
Moreira, R. R. D., SantosDos, A. G. d., Carvalho, F. A., Perego, C. H., Crevelin, E. J., Crotti, A. E. M., et al. (2019). Antileishmanial Activity of Melampodium Divaricatum and Casearia Sylvestris Essential Oils on Leishmania Amazonensis. Rev. Inst. Med. Trop. S. Paulo 61. e33. doi:10.1590/s1678-9946201961033
Moreno, E., Schwartz, J., Larrea, E., Conde, I., Font, M., Sanmartín, C., et al. (2015). Assessment of β-lapachone Loaded in Lecithin-Chitosan Nanoparticles for the Topical Treatment of Cutaneous Leishmaniasis in L. Major Infected BALB/c Mice. Nanomedicine: Nanotechnology, Biol. Med. 11, 2003–2012. doi:10.1016/j.nano.2015.07.011
Mukherjee, D., Singh, C. B., Dey, S., Mandal, S., Ghosh, J., Mallick, S., et al. (2016). Induction of Apoptosis by Zerumbone Isolated from Zingiber Zerumbet (L.) Smith in Protozoan Parasite Leishmania Donovani Due to Oxidative Stress. Braz. J. Infect. Dis. 20, 48–55. doi:10.1016/j.bjid.2015.10.002
Naz, S., Islam, M., Tabassum, S., Fernandes, N. F., Carcache de Blanco, E. J., and Zia, M. (2019). Green Synthesis of Hematite (α-Fe2O3) Nanoparticles Using Rhus Punjabensis Extract and Their Biomedical prospect in Pathogenic Diseases and Cancer. J. Mol. Struct. 1185, 1–7. doi:10.1016/j.molstruc.2019.02.088
Noormehr, H., Zavaran Hosseini, A., Soudi, S., and Beyzay, F. (2018). Enhancement of Th1 Immune Response against Leishmania Cysteine Peptidase A, B by PLGA Nanoparticle. Int. Immunopharmacology 59, 97–105. doi:10.1016/j.intimp.2018.03.012
Olekhnovitch, R., Ryffel, B., Müller, A. J., and Bousso, P. (2014). Collective Nitric Oxide Production Provides Tissue-wide Immunity during Leishmania Infection. J. Clin. Invest. 124, 1711–1722. doi:10.1172/JCI72058
Oryan, A., and Akbari, M. (2016). Worldwide Risk Factors in Leishmaniasis. Asian Pac. J. Trop. Med. 9, 925–932. doi:10.1016/j.apjtm.2016.06.021
Oryan, A. (2015). Plant-derived Compounds in Treatment of Leishmaniasis. Iranj. Vet. Res. 16(1), 1–19. doi:10.22099/ijvr.2015.2917
Park, E.-J., Yi, J., Kim, Y., Choi, K., and Park, K. (2010). Silver Nanoparticles Induce Cytotoxicity by a Trojan-Horse Type Mechanism. Toxicol. Vitro 24, 872–878. doi:10.1016/j.tiv.2009.12.001
Pereira, K. L. G., Vasconcelos, N. B. R., Braz, J. V. C., Inácio, J. D. F., Estevam, C. S., Correa, C. B., et al. (2020). Ethanolic Extract of Croton Blanchetianus ball Induces Mitochondrial Defects in Leishmania Amazonensis Promastigotes. Acad. Bras. Ciênc. 92. e20180968. doi:10.1590/0001-3765202020180968
Prasanna, P., Kumar, P., Mandal, S., Patyal, T., Patyal, T., Kumar, S., Das, P., et al. (2020). Synthesis of 7, 8–dihydroxyflavone Functionalized Gold Nanoparticles and its Mechanism of Action against Leishmania Donovani. Res. Square 1–29. doi:10.21203/rs.3.rs-37873/v3
Robledo, S. M., Cardona, W., Ligardo, K., Henao, J., Arbeláez, N., Montoya, A., et al. (2015). Antileishmanial Effect of 5,3′-Hydroxy-7,4′-Dimethoxyflavanone ofPicramnia gracilisTul. (Picramniaceae) Fruit:In VitroandIn VivoStudies. Adv. Pharmacol. Sci. 2015, 1–8. doi:10.1155/2015/978379
Rosas-Hernández, H., Jiménez-Badillo, S., Martínez-Cuevas, P. P., Gracia-Espino, E., Terrones, H., Terrones, M., et al. (2009). Effects of 45-nm Silver Nanoparticles on Coronary Endothelial Cells and Isolated Rat Aortic Rings. Toxicol. Lett. 191, 305–313. doi:10.1016/j.toxlet.2009.09.014
Rossi, D., Ahmed, K., Gaggeri, R., Della Volpe, S., Maggi, L., Mazzeo, G., et al. (2017). (R)-(−)-Aloesaponol III 8-Methyl Ether from Eremurus Persicus: A Novel Compound against Leishmaniosis. Molecules 22, 519. doi:10.3390/molecules22040519
Salama, I. C., Arrais-Lima, C., and Arrais-Silva, W. W. (2017). Evaluation of Boldine Activity against Intracellular Amastigotes of Leishmania Amazonensis. Korean J. Parasitol. 55, 337–340. doi:10.3347/kjp.2017.55.3.337
Saleh, A. H. (2019). Potential Role of Titanium Dioxide (TiO2) Nanoparticles against the Toxicity of Leishmania Tropica in Adult Albino Male Rats. JGPT 11 (3), 453–457.
Saljoughian, N., Zahedifard, F., Doroud, D., Doustdari, F., Vasei, M., Papadopoulou, B., et al. (2013). Cationic Solid-Lipid Nanoparticles Are as Efficient as Electroporation in DNA Vaccination against Visceral Leishmaniasis in Mice. Parasite Immunol. 35, 397–408. doi:10.1111/pim.12042
Santana, R. C., Rosados, A. d. S. S., Mateus, M. H. d. S., Soares, D. C., Atella, G., Guimarães, A. C., et al. (2020). In Vitro leishmanicidal Activity of Monoterpenes Present in Two Species of Protium (Burseraceae) on Leishmania Amazonensis. J. Ethnopharmacology 259, 112981. doi:10.1016/j.jep.2020.112981
Shah, S., Ullah, F., Ayaz, M., Sadiq, A., Hussain, S., Ali Shah, A.-U. -H., et al. (2019). Benzoic Acid Derivatives of Ifloga Spicata (Forssk.) Sch.Bip. As Potential Anti-leishmanial against Leishmania Tropica. Processes, 7, 208. doi:10.3390/pr7040208
Shaheen, N., Qureshi, N. A., Ashraf, A., Hamid, A., Iqbal, A., and Fatima, H. (2020). In Vitro anti-leishmanial Activity of Prunus Armeniaca Fractions on Leishmania Tropica and Molecular Docking Studies. J. Photochem. Photobiol. B: Biol. 213, 112077. doi:10.1016/j.jphotobiol.2020.112077
Sharma, R., Gupta, V., Chawla, V., and Chawla, P. (2019). Exploring the Possible Role of Nature in Curing Leishmaniasis. Jamps, 20(3), 1–21. doi:10.9734/jamps/2019/v20i330114
Shoaib Sarwar, H., Varikuti, S., Farhan Sohail, M., Sarwar, M., Akhtar, S., Satoskar, A. R., et al. (2020). Oral Delivery and Enhanced Efficacy of Antimonal Drug through Macrophage-Guided Multifunctional Nanocargoes against Visceral Leishmaniasis. Eur. J. Pharmaceutics Biopharmaceutics 152, 307–317. doi:10.1016/j.ejpb.2020.05.029
Silveira, E. S., Castro Rodrigues, N. L. D., Machado, N. J., Marciano Fonseca, F. R., Teixeira, M. J., and Almeida Moreira Leal, L. K. (2021). Medicinal Plants Containing Coumarin or Essential Oils from the Brazilian Biome May Be New Option for Treating Leishmaniasis?. Phrev 14, 53–61. doi:10.5530/phrev.2020.14.9
Sundar, S., Sinha, P. K., Rai, M., Verma, D. K., Nawin, K., Alam, S., et al. (2011). Comparison of Short-Course Multidrug Treatment with Standard Therapy for Visceral Leishmaniasis in India: An Open-Label, Non-inferiority, Randomised Controlled Trial. Lancet 377(9764), 477–486. doi:10.1016/S0140-6736(10)62050-8
Surur, A. S., Fekadu, A., Makonnen, E., and Hailu, A. (2020). Challenges and Opportunities for Drug Discovery in Developing Countries: The Example of Cutaneous Leishmaniasis. ACS Med. Chem. Lett. 11, 2058–2062. doi:10.1021/acsmedchemlett.0c00446
Vendruscolo, M. H., das Neves, G. M., Kagami, L. P., Rodrigues Junior, L. C., Nunes Diehl, M. L., Gnoatto, S. C. B., et al. (2019). In Vitro and In Silico Activity of Iridoids against Leishmania Amazonensis. Cddt 16, 173–183. doi:10.2174/1570163814666171002102058
Verma, N. K., and Dey, C. S. (2004). Possible Mechanism of Miltefosine-Mediated Death of Leishmania Donovani. Aac 48, 3010–3015. doi:10.1128/AAC.48.8.3010-3015.2004
Zahir, A. A., Chauhan, I. S., Bagavan, A., Kamaraj, C., Elango, G., Shankar, J., et al. (2015). Green Synthesis of Silver and Titanium Dioxide Nanoparticles Using Euphorbia Prostrata Extract Shows Shift from Apoptosis to G0/G1Arrest Followed by Necrotic Cell Death in Leishmania Donovani. Antimicrob. Agents Chemother. 59, 4782–4799. doi:10.1128/AAC.00098-15
Keywords: leishmaniasis, natural sources, nanobiotech, green route, nanoformulations
Citation: Sharma L, Dhiman M, Singh A and Sharma MM (2021) Green Approach: ‘‘A Forwarding Step for Curing Leishmaniasis—A Neglected Tropical Disease’’. Front. Mol. Biosci. 8:655584. doi: 10.3389/fmolb.2021.655584
Received: 19 January 2021; Accepted: 04 May 2021;
Published: 28 May 2021.
Edited by:
Diptiman Choudhury, Thapar Institute of Engineering and Technology, IndiaReviewed by:
Patrick Valere Tsouh Fokou, The University of Bamenda, CameroonCopyright © 2021 Sharma, Dhiman, Singh and Sharma. This is an open-access article distributed under the terms of the Creative Commons Attribution License (CC BY). The use, distribution or reproduction in other forums is permitted, provided the original author(s) and the copyright owner(s) are credited and that the original publication in this journal is cited, in accordance with accepted academic practice. No use, distribution or reproduction is permitted which does not comply with these terms.
*Correspondence: M. M. Sharma, bWFkYW5tb2hhbi5zaGFybWFAamFpcHVyLm1hbmlwYWwuZWR1
Disclaimer: All claims expressed in this article are solely those of the authors and do not necessarily represent those of their affiliated organizations, or those of the publisher, the editors and the reviewers. Any product that may be evaluated in this article or claim that may be made by its manufacturer is not guaranteed or endorsed by the publisher.
Research integrity at Frontiers
Learn more about the work of our research integrity team to safeguard the quality of each article we publish.