- Department of Transfusion Medicine, Beijing Children’s Hospital, Capital Medical University, National Center for Children’s Health, Beijing, China
As an initiator of respiratory distress, transfusion-related acute lung injury (TRALI) is regarded as one of the rare complications associated with transfusion medicine. However, to date, the pathogenesis of TRALI is still unclear, and specific therapies are unavailable. Understanding the mechanisms of TRALI may promote the design of preventive and therapeutic strategies. The immune system plays vital roles in reproduction, development and homeostasis. Sterile tissue damage, such as physical trauma, ischemia, or reperfusion injury, induces an inflammatory reaction that results in wound healing and regenerative mechanisms. In other words, in addition to protecting against pathogens, the immune response may be strongly associated with TRALI prevention and treatment through a variety of immunomodulatory strategies to inhibit excessive immune system activation. Immunotherapy based on immune cells or immunological targets may eradicate complications. For example, IL-10 therapy is a promising therapeutic strategy to explore further. This review will focus on ultramodern advances in our understanding of the potential role of the immune system in TRALI prevention and treatment.
Introduction
Transfusion-related acute lung injury (TRALI) is the onset of respiratory distress and acute lung injury due to blood product transfusion (Semple et al., 2019), and it is a life-threatening complication characterized by the sudden onset of hypoxemic respiratory failure with non-cardiogenic pulmonary edema and bilateral lung infiltration that developed within 6 h of blood transfusion (Toy et al., 2005; Gokhale and Hendrickson, 2019). Although TRALI develops within 6 hrs of blood transfusion, most occurrences take place during transfusion or within the first 1 or 2 h. According to Voelker and Spieth (2019) TRALI is defined as more complex for cases of seeming TRALI, such as transfusion-associated circulatory overload (TACO), and further testing and diagnosis may be required. TRALI pathophysiology has been partially of elucidated. According to the 2004 Canadian Consensus Conference Panel (Kleinman et al., 2004) and TRALI redefinition (Vlaar et al., 2019), TRALI can be identified and diagnosed, and the terms TRALI I [without an acute respiratory distress syndrome (ARDS)] and TRALI II (accompanied by ARDS) have been proposed. However, there were some potential TRALI cases did not meet standard clinical definitions (Fuller et al., 2021).
Currently, TRALI is the leading cause of transfusion-related fatalities (Clifford et al., 2015), and the case fatality rate is 5–10% (Swanson et al., 2006). The incidence of TRALI in surgical transfusion patients is 1.3–1.4% (Clifford et al., 2015), and as many as 15% of patients with severe transfusions develop TRALI (Benson et al., 2010). A report from the US FDA showed that 34% of fatalities were due to TRALI from 2012 to 2016 (fatalities reported to FDA following blood collection and transfusion: annual summary for fiscal year 2016: US Food and Drug Administration), which constitutes one of the leading serious adverse reactions to transfusion. Lieberman et al. (2014) suggested that the incidence of TRALI in children is similar to that in adults. Although the numbers are low, there are no differences in outcomes or presentation between adults and children with TRALI.
Generally, the risk factors for blood transfusion can be divided into antibody-independent and antibody-dependent in the context of TRALI. The former is caused by the transfusion of aged red blood cells or platelets, which contain proinflammatory mediators, bioactive lipids, etc. (Mangalmurti et al., 2009; Vlaar et al., 2010; Peters et al., 2015a). The latter is caused by antibody infusions, which mainly contain human leukocyte antigen (HLA) I and II or human neutrophil antigen (HNA) antibodies (Peters et al., 2015b), are mainly obtained from female donors and cause human neutrophil activation (Popovsky et al., 1983). Antibody-dependent TRALI is the most prevalent type (Popovsky and Moore, 1985; Curtis and McFarland, 2006).
Currently, the two-hit model is used to describe the underlying mechanism of TRALI pathology (Peters et al., 2015b; Lorello and Alam, 2018). The predisposing factors of the patient are the first hit, and antibody or non-antibody factors are the second hit (Silliman et al., 1997; Silliman, 2006). Some clinical studies have shown that an inflammatory first hit almost always occurs before TRALI onset and have identified ARDS risk factors that may also be TRALI risk factors in transfusion recipients, including liver surgery, shock, increased peak airway pressure, chronic alcohol abuse and current smoking in the context of immune system balance and inflammation (Toy et al., 2012). Namely, the clinical condition of the patient induces the release of a large number of cytokines, which cause neutrophil activation, accumulation and adhesion to pulmonary microvascular endothelial cells. The second hit is mainly due to HLA or HNA antibodies from the blood products that directly or indirectly activate the recipient’s immune system. An increasing number of studies have shown that antibody-dependent TRALI is mediated by the activation of neutrophils, which results in the release of oxygen free radicals and proteases, causes endothelial damage, and increases capillary permeability, ultimately causing pulmonary edema, and inducing TRALI (Silliman, 2006).
According to the classic definition, the immune system is comprised of complement molecules, as well as immune cells and their products, including cytokines, chemokines, antibodies, and growth factors. The immune system is considered to be responsible for defending the host from invading pathogenic microorganisms. In fact, the immune system is an integral part of fundamental physiological processes and immune cells function beyond host defense (Sattler, 2017). Researchers in an expanding range of areas are beginning to recognize continuously the implications of the immune system in their respective fields. The immune system plays an essential role in reproduction both before and during pregnancy, and leukocytes are found in male and female reproductive tissues (Oakley et al., 2011; Care et al., 2013). In other words, the effects of the immune system are not limited to host defense but extend to tissue homeostasis, regeneration and the repair of tissues such as the liver (Meijer et al., 2000), kidney (Lin et al., 2010; Zhang et al., 2012), skin (Goren et al., 2009; Mirza et al., 2009), skeletal muscle, heart (Arnold et al., 2007; Nahrendorf et al., 2007; Frantz et al., 2012; Gallego-Colon et al., 2015; Tonkin et al., 2015; Sattler and Rosenthal, 2016), gut (Seno et al., 2009), and brain (Glod et al., 2006; London et al., 2013). Both evolutionary development and functional variety strongly support the idea of the immune system as an all-encompassing system to ensure systemic integrity.
More importantly, the immune response is closely associated with transfusion therapy (Passwater, 2018). Landers et al. (1996) showed the immunomodulatory effects of blood transfusion in 1996. The immune response is an important basis for the occurrence of TRALI (Tung, 2019). Specifically, before and after transfusion, TRALI patients exhibited elevated levels of IL-6 and IL-8, which are the main contributors to the development of TRALI (Roubinian et al., 2015). Fung et al. (2010) also suggested the importance of T cells in reducing the severity of antibody-dependent TRALI in a murine model. Furthermore, it is believed that TRALI is the result of imbalances in the body’s inflammatory response. During infectious diseases, a variety of immunomodulatory molecular mechanisms are activated, including the induction of regulatory T cells (Tregs), regulatory B cells, alternatively activated M2 macrophages or the anti-inflammatory cytokines IL-10 and TGF-β (Taylor et al., 2012; Ziegler et al., 2015; Xu et al., 2016; Loevenich et al., 2017; Soares et al., 2017), which affect all facets of the host immune response to ensure host survival. However, some immune factors, such as B cells, were shown to not play a significant role in the onset of murine antibody-mediated TRALI (Kapur et al., 2017a). Accordingly, in this report, we identified potential immunotherapeutic approaches for TRALI based on immune cells, cytokines and complement molecules by analyzing the literature.
Regulatory T Cells
Tregs are essential for maintaining immune homeostasis by regulating effector T cell responses, thus facilitating pathogen immune evasion (Velavan and Ojurongbe, 2011) and preventing potential pathogenic host pathogenic effects through a variety of mechanisms (Shevach, 2009; Maruyama et al., 2011; Gao et al., 2012). For example, Tregs express receptor molecules such as CTLA-4, PD-1 and GITR and directly contact target cells to regulate effector cell function, secrete IL-10, TGF-β and other inhibitory cytokines to exert immunosuppressive effects, and allow antigen-presenting cells to enter a non-response state; the expression of enzymes such as CD39 and CD73 indirectly affects the metabolism of target cells, and the secretion of granzymes A and B directly kills target cells and affects immune responses (Shevach, 2009; Josefowicz et al., 2012; Gubser et al., 2016; Rueda et al., 2016). Tregs represent an antigen-specific mechanism to inhibit potentially harmful autoreactive responses.
Although Tregs are defined as T cells with immunosuppressive activity, it has been documented that Treg populations remain diverse (Miyara and Sakaguchi, 2007). Tregs expressing CD25 and Foxp3 are naturally present in the immune system and are considered to be negative regulators of the T cell response. These natural Tregs originate during thymic development (Mold et al., 2008). In addition, other cells, such as IL-10-secreting CD4+CD25–Foxp3– (Tr1) cells and TGF-β-secreting CD4+CD25–Foxp3+ T cells, have also been shown to exert regulatory effects (Lan et al., 2005). While CD4+CD25–Foxp3+ T cells, which originate from CD4+CD25– T cells that develop in the periphery (Wen et al., 2012), CD4+CD25+Foxp3+, CD4+CD25–Foxp3+, and Tr1 Tregs can be activated in different microenvironments. Moreover, CD4–CD8– Tregs (double-negative Tregs, DNTregs) express the αβ TCR but do not express CD4, CD8, NK cell surface markers or Foxp3, and CD8+ Tregs have no specific surface markers (Lan et al., 2005; Sakaguchi et al., 2010; Murphy and Weaver, 2016). In recent years, a new subpopulation of Tregs (iTR35 cells) has been shown to mediate immunosuppression via IL-35 but not IL-10 or TGF-β and is independent of Foxp3 (Collison et al., 2010).
Foxp3-expressing Tregs are known to be indispensable for the maintenance of immunological self-tolerance and immune homeostasis (Sakaguchi et al., 2006). IL-10, TGF-β and Foxp3 are functional and phenotypic markers of natural Tregs (nTregs) and are associated with inducible Tregs (iTregs) (Fontenot et al., 2003). Tregs expressing Foxp3 are able to suppress the activation, proliferation and effector functions (such as cytokine production) of CD4+ and CD8+ T cells, natural killer (NK) cells, NKT cells, B cells and antigen-presenting cells (APCs) in vitro and in vivo (Sakaguchi et al., 2008). Tregs can also be isolated and expanded in vitro; thus far, treatment with this cell product seems safe and well tolerated (Salas and Panes, 2015).
Notably, some researchers have shown that lymphocytes, especially Tregs, are recruited to the lung in response to lung injury. Tregs may play a key role in protecting against TRALI. Tregs can relieve inflammation by regulating immune responses, thereby alleviating lung injury in vivo and in vitro (D’Alessio et al., 2009). Venet et al. (2009) confirmed that Tregs reduced neutrophil recruitment and activation by producing IL-10 in lipopolysaccharide (LPS)-mediated acute lung injury. Furthermore, Kapur et al. (2017a) demonstrated for the first time that CD4+ CD25+ Foxp3+ Tregs are critical effectors that protect against antibody-dependent murine TRALI via IL-10. Two years later, He et al. (2019) showed that IL-2c and IL-2 derived from CD4+CD25+Foxp3+ Tregs increased IL-10 and decreased IL-17A, thereby prophylactically preventing antibody-dependent murine TRALI. Subsequently, different subtypes of Tregs, such as Tr1 and iTR35 cells, may play important roles in further studies.
Dendritic Cells
In general, dendritic cells (DCs) are highly specialized antigen-presenting cells that are important in not only initiating immune responses but also in tuning the quality of the immune response or inhibiting the response (Moll, 2003). DCs play a crucial role in this regulatory work, as these cells can regulate T cell-mediated effector responses by generating anti-inflammatory cytokines or inducing Tregs (Maldonado and von Andrian, 2010). Tolerogenic DCs, in particular, can regulate immune responses through various mechanisms (Steinman et al., 2003). Cell fate is predominantly controlled by cytokines in the microenvironment, and to some extent, by the strength of the interaction between the T cell receptor (such as CD45, CTLA-4, and PD-1) and the antigen (Boyton and Altmann, 2002). Tight regulation of effector T cell responses is required to effectively control infections and avoid autoimmune and immunopathological diseases. Aberrant T cell responses, especially those of Th1 and Th17 cells, may play key roles in organ-specific autoimmunity (Dardalhon et al., 2008) and immunopathology (Rutitzky et al., 2008) in the lung. Previously, Kapur R demonstrated that CD11c+ DCs protected against antibody-dependent TRALI via IL-10 in mice (Kapur et al., 2017a). The role of DCs may be essential for TRALI immunotherapy.
Macrophages
Currently, macrophage-targeted therapy has been used in patients (Patel and Janjic, 2015). Alveolar macrophages are central effector cells in the production of proinflammatory mediators. These cells are key in the initiation and resolution of lung inflammation in humans. In the future, the polarization of M1 macrophages may be essential in human TRALI. Lei W et al. (Wang et al., 2020) confirmed that α1-antitrypsin expression improved lung injury by regulating IL-6 production in alveolar macrophages and decreased the M1 macrophage polarization. Modulating macrophage polarization may serve as a potential treatment strategy for human TRALI. By establishing an anti-major histocompatibility complex (MHC) class I monoclonal antibody-induced mouse model of TRALI-like disease, Strait et al. (2011) showed that TRALI induction involves monocytes and macrophages in this murine model.
In addition, osteopontin, a recognized proinflammatory molecule that mediates diverse biological functions (Lund et al., 2009), is involved in various pulmonary disorders, such as fibrosis, inflammation, malignancies, and vascular lung disorders (O’Regan, 2003). Osteopontin is also an important protein involved in regulating the migration of immune cells (Lund et al., 2009). The osteopontin-mediated murine TRALI response is dependent on macrophages, which may be possibly the cellular source of osteopontin (Kapur et al., 2019). In summary, targeting macrophage function may be a critical strategy for TRALI treatment or prevention (Zeeuw van der Laan et al., 2020b).
Neutrophils
Neutrophils, which have diameters of 12–14 μm, are derived from hematopoietic stem cells. As the first line of cellular defense against invading pathogens, neutrophils can rapidly move across the blood-endothelial cell barrier and exert effector functions during inflammation. Neutrophils are the first responders and are recruited in large numbers to the inflammatory microenvironment by the accumulation of lipid mediators, cytokines, and chemokines, as well as changes in the vascular endothelium (Sadik and Luster, 2012; Mocsai et al., 2015). Neutrophil recruitment to inflamed tissues involves elements of neutrophil rolling and firm adhesion (Filippi, 2019). To capture extracellular pathogen-associated molecules and other stimuli, neutrophils release decondensed chromatin coated with granular proteins, which form neutrophil extracellular traps (NETs) (Branzk et al., 2014; Storisteanu et al., 2017; Watanabe and Petri, 2019). However, the secretion of NETs can also cause tissue damage at the expense of the host and has been shown to impair wound healing or facilitate lung injury in diabetes (Wong et al., 2015) and ventilator-induced lung injury (Yildiz et al., 2015). NETs play an essential role in TRALI. Caudrillier et al. (2012) demonstrated that platelets triggered NET formation in an LPS- or anti-MHC class I antibody-based TRALI mouse model. Thomas et al. (2012) showed that NET biomarkers were present in the serum of TRALI patients, as well as in the lungs of LPS- and anti-MHC class I antibody-induced TRALI mice. Treatment with DNase1 IV, which disrupts the formation of the extracellular chromatin web (Hakkim et al., 2010), was shown to have beneficial effects in these two studies. Although DNases are associated with cystic fibrosis in the lung (Thornby et al., 2014), and additional studies on NETs are necessary in the context of DNase therapy for human TRALI.
In addition, as the major producers of reactive oxygen species (ROS), neutrophils can damage the endothelium in antibody-dependent TRALI mice (Strait et al., 2011; Bayat et al., 2013) and in human pulmonary microvascular endothelial cells in vitro (Silliman et al., 2007). Other studies has shown neutrophil-mediated endothelial cell damage in antibody-independent TRALI (Xie et al., 2015) and antibody-dependent TRALI (Nishimura et al., 2006; Silliman et al., 2007; Khoy et al., 2017). Moreover, neutrophils and ROS were shown to be critical in antibody-mediated murine TRALI, as demonstrated by in vivo neutrophil depletion and the use of gp91phox-knockout mice, respectively (Kapur et al., 2017a).
Lipids in blood products also damage endothelial cells via neutrophils in an antibody-independent TRALI model, and in human pulmonary microvascular endothelial cells that were activated by LPS and cocultured with neutrophils (Wyman et al., 2002). Neutrophil-dependent endothelial cell damage also occurred in the presence of soluble CD40L (Khan et al., 2006). The Fcγ receptor on neutrophils can recognize the Fc-tail of IgG, which binds to pathogens via the IgG-Fab region (Vidarsson and van de Winkel, 1998; Hogarth and Pietersz, 2012; Kapur et al., 2014). A study by Looney MR (Looney et al., 2006) showed that neutrophils and their Fcγ receptors were essential in a TRALI mouse model. Moreover, researchers found that neutrophil Fcγ receptors were critically involved in TRALI, as adoptive transfer of wild-type neutrophils into TRALI-resistant Fcγ receptor-knockout mice ameliorated acute lung injury upon challenge with anti-MHC class I antibodies (Looney et al., 2006). Neutrophil depletion can protect against anti-HNA-3a antibody-mediated TRALI in mice, although TRALI was not completely prevented in this context (Bayat et al., 2013).
Neutrophils are key effectors in TRALI pathogenesis (Rebetz et al., 2018). Pulmonary neutrophil infiltration has been shown to occur in multiple murine TRALI models (Looney et al., 2006, 2009; Kelher et al., 2009; Fung et al., 2010; Tung et al., 2011; Semple et al., 2012; Bayat et al., 2013, 2015; McKenzie et al., 2014; Kapur et al., 2015, 2017a, 2018) and human TRALI patients (Dry et al., 1999; Silliman et al., 2005; Akagi et al., 2020). Currently, new evidence clearly points to the plasticity of neutrophils and may present a new strategy for the potential treatment of TRALI.
IL-10
IL-10 is a member of the IL-10 family of cytokines and a non-covalent homodimeric alpha helical cytokine with structural similarities to IFN-γ. The IL-10 receptor (IL-10R) is expressed on the surface of most hematopoietic cells, including T and B cells and macrophages. Genetically, the immunological and physiological functions of IL-10 were found to be non-redundant in engineered mouse models that lack IL-10 or IL-10R. IL-10 inhibits the proliferation of CD4+ T cells and the secretion of many kinds of cytokines (Fiorentino et al., 1989).
IL-10 expression is altered in patients with TRALI relative to that in control patients. Kapur et al. (2017b) showed reduced IL-10 levels in patients with TRALI. In all pulmonary transfusion reactions, the combination of clinical variables and cytokine measurements indicated optimal diagnostic performance, and the model comparing TACO and TRALI correctly classified 92% of cases relative to expert panel diagnoses (Roubinian et al., 2015). Low plasma IL-10 levels were associated with TRALI susceptibility in a mouse model, and IL-10-knockout mice were also hypersensitive to TRALI induction (Kapur et al., 2017a). These results suggested that low IL-10 levels were a risk factor for TRALI in humans (Kapur et al., 2017b). However, despite this beneficial effect, these data were in contrast to the findings in patients who suffered from other inflammatory/transfusion-associated pulmonary disorders, such as sepsis-associated acute lung injury (Kapur et al., 2017b) or transfusion-associated circulatory overload (Roubinian et al., 2015), in which IL-10 levels were often elevated. This finding may indicate that TRALI has a distinct mechanism of injury and may be uniquely characterized by impaired IL-10 production. However, two reports demonstrated that IL-10 levels were increased in TRALI patients (Looney et al., 2006, 2014). However, the study showed the changes that may indicate risk factors before blood transfusion (Looney et al., 2014) and may explain this discrepancy.
Regardless, IL-10 infusion may have a therapeutic effect in human TRALI and may completely prevent and protect against the development of antibody-dependent TRALI without any apparent side effects both prophylactically and therapeutically by ameliorating the TRALI reaction in mice (Kapur et al., 2017a). Furthermore, the administration of IL-10 to healthy volunteers was documented as a safe intervention, and only mild to moderate side effects, such as flu-like symptoms, were observed (Moore et al., 2001). We therefore hypothesize that IL-10 administration may be an attractive approach for alleviating TRALI, and clinical studies in humans are highly warranted within 6 h of transfusion for acute lung reactions. However, IL-10 may impair host immune system resistance to infection in clinical settings.
In summary, low plasma IL-10 levels were associated with TRALI susceptibility in a mouse model, and IL-10-knockout mice were also hypersensitive to TRALI induction (Kapur et al., 2017a). IL-10 levels are low in TRALI patients (Kapur et al., 2017b), and very importantly, IL-10 therapy both prophylactically and therapeutically alleviates TRALI in mice (Kapur et al., 2017a). Therefore, IL-10 therapy is a promising therapeutic strategy to explore further.
IL-8
IL-8 is a CXC chemokine that promotes neutrophil chemotaxis and degranulation downstream of CXC-chemokine receptor (CXCR) 1 and CXCR2, which are G protein–coupled receptors (Holmes et al., 1991; Hammond et al., 1995; Waugh and Wilson, 2008). A study by Roubinian NH demonstrated that IL-6 and IL-8 were elevated in patients with TRALI before and after transfusion relative to those in the control group (Roubinian et al., 2015). In H-2Kd/H-2Dd antibody-based severe combined immunodeficient TRALI models, it was demonstrated that the binding of H-2Kd/H-2Dd antibodies to monocytes increased the levels of macrophage inflammatory protein 2 (MIP-2), causing increased pulmonary neutrophil infiltration and subsequently inducing TRALI (McKenzie et al., 2014).
In vivo, peripheral blood monocyte depletion or chemokine blockade completely stopped TRALI induction using an MIP-2 receptor antagonist in severe combined immunodeficiency (SCID) mice (McKenzie et al., 2014). Roubinian et al. (2015) showed that IL-8 is a known risk factor for TRALI, and blocking the IL-8 receptor may also be sufficient to suppress neutrophil chemotaxis and degranulation, thereby counteracting TRALI induction. Moreover, in clinical trials, CXCR2 antagonists were evaluated in other pulmonary disorders, such as cystic fibrosis, asthma and chronic obstructive pulmonary disease (Chapman et al., 2009; O’Byrne et al., 2016), and may have potential efficacy in TRALI as well.
Complement Cascade
One hundred years ago, the complement system was defined based on its ability to “complement” the antibody-dependent and cell-dependent immune responses against pathogens in blood-based antimicrobial system to compared that of global regulators of immunity and tissue homeostasis. Today, many studies have focused on understanding the biology of complement and its mechanisms of action. Our understanding of innate immunity has substantially changed. Complement plays an essential role in modulating innate and adaptive immunity, supports innate immune responses and the initiation of general inflammatory reactions, contributes to tissue and organ development and promotes tissue repair after injury. In cooperation with other immune and physiological systems, the complement system is key in the recognition and elimination of invading pathogens and mediates the clearance of apoptotic cells, cellular debris and immune complexes to remove self-derived danger signals by integrating innate and adaptive immunity (Ricklin et al., 2010; Arbore et al., 2017).
Distinct mechanisms of the complement cascade are triggered by classic, lectin or alternative signals that converge at the third component (C) and lead to the generation of effectors that make up or supplement the ability of antibodies and phagocytes to clear microbial intruders via the opsonization of C3b, which promotes inflammation via anaphylatoxins C3a and C5a and lyses susceptible pathogens via the C5b-9 membrane attack complex (Ricklin et al., 2010). Complement C5a was shown to play an important role in an anti-MHC class I antibody–based TRALI model in BALB/c mice (Strait et al., 2011). Strait et al. (2011) confirmed that the anti-MHC class I antibody-induced murine TRALI model involved complement activation and C5a production (Strait et al., 2011). However, Looney et al. (2006) described the induction of TRALI 2 h after anti-MHC class I antibody injection in C5a receptor-deficient mice (Looney et al., 2006), and additional validation is needed on the role of C5a as a possible TRALI therapy before the use of C5 inhibitors (Nunn et al., 2005). This further research will shed light on the relevance of the complement system in TRALI, which may open up new therapeutic avenues to explore in combatting TRALI. Cleary SJ demonstrated that complement depletion protected the lungs in endothelial MHC I-deficient mice, and targeting complement cascade activation may be useful for TRALI treatment or prevention (Cleary et al., 2020). Zeeuw van der Laan et al. (2020a) further confirmed that TRALI-inducing murine antibodies have increased abilities to activate the complement system, especially Fc-mediated complement activation, compared to those of antibodies that do not cause TRALI. Complement activation by the endothelium initiates antibody-dependent acute lung injury. Neutrophil responses, including NET release, were intact in endothelial MHC I-deficient mice, whereas complement depletion reduced lung injury (Cleary et al., 2020).
In summary, the complement cascade eradicates invading microorganisms, apoptotic cells, and immune complexes. This evidence should significantly increase our understanding of the role of complement in TRALI and thereby potentially result in promising new treatment strategies. Additional systematic studies are needed to elucidate the role of complement in TRALI (Jongerius et al., 2019).
Other Factors
McKenzie et al. (2014) demonstrated that intact antibody-induced TRALI was abrogated after the depletion of peripheral blood monocytes. However, TRALI was restored upon purified monocyte restoration. Looney et al. (2009) showed that platelet depletion and aspirin treatment protected mice in an antibody-dependent TRALI model. Cognasse et al. (2020) further showed that although platelet depletion did not prevent the occurrence of TRALI in a mouse model, it did reduce TRALI severity and inhibit TRALI development, and recipient platelets are likely involved in TRALI (Semple and Kapur, 2020). The blockade of sphingomyelinase, extracellular vesicle elimination, or supplementation with sphingosine-1-phosphate during platelet storage may present promising new TRALI prevention strategies (McVey et al., 2021).
We should also note that C-reactive protein (CRP) enhances antibody-mediated TRALI induction in mice (Kapur et al., 2015) and that CRP levels are elevated in human TRALI patients (Kapur et al., 2016). Some studies have indicated that targeting the CD40-CD40L interaction could be an important method to prevent or protect against TRALI (Tariket et al., 2019; Hu et al., 2020). The importance of the gut flora in TRALI induction (Kapur et al., 2018) is continuously being studied and explored.
In addition, cytokines connect and interact with each other through a crosstalk network. Cytokines play important roles in regulating the immune response, differentiating and developing immune cells, and mediating the inflammatory response. The TRALI model shows that host inflammation develops, and the disease is the first blow, causing endothelial cells to secrete cytokines, which helps the lung attract neutrophils by increasing the surface expression of cell adhesion molecules. Lung endothelial cells release cytokines and chemokines to activate and induce neutrophils to accumulate in pulmonary capillaries, stimulating neutrophil-mediated endothelial cell interactions and mediating lung injury. Therefore, other than IL-10 and IL-8, other cytokines may be also potential research targets for TRALI prevention and treatment. For example, Qiao et al. (2020) preliminarily confirmed that IL-35 could prevent murine TRALI by inhibiting the activation of endothelial cells. The study of additional immunological factors in combination with previous research findings could shape the field of TRALI research. For example, IL-6, TNF-α, IL-1β and danger-associated molecular patterns (Land, 2013; Tolle and Standiford, 2013; Liu et al., 2017) are factors that induce ARDS.
Discussion
Overall TRALI remains a significant clinical problem. The development of TRALI animal models is highly important in dissecting the disease pathology because factors other than volume appear to play a significant role in TRALI. Based on previously studies, these immune approaches may significantly contribute to combating these life-threatening complications of blood transfusion. Cell-based therapies, such as those based on Treg or DC administration, seem to be safe and well tolerated. Cytokines or secreted factors have increasingly been utilized to validate risk factors for TRALI, and cytokine concentrations are related to the pathogenesis of TRALI. As Semple et al. (2018, 2019) proposed, the most promising therapeutic strategies to explore are interleukin-10 therapy, down-modulating C-reactive protein levels, targeting reactive oxygen species, or blocking the interleukin-8 receptor. Innate immune molecules, such as complement, are also important. The immune cells or molecules involvement in TRALI are summarized in Figure 1.
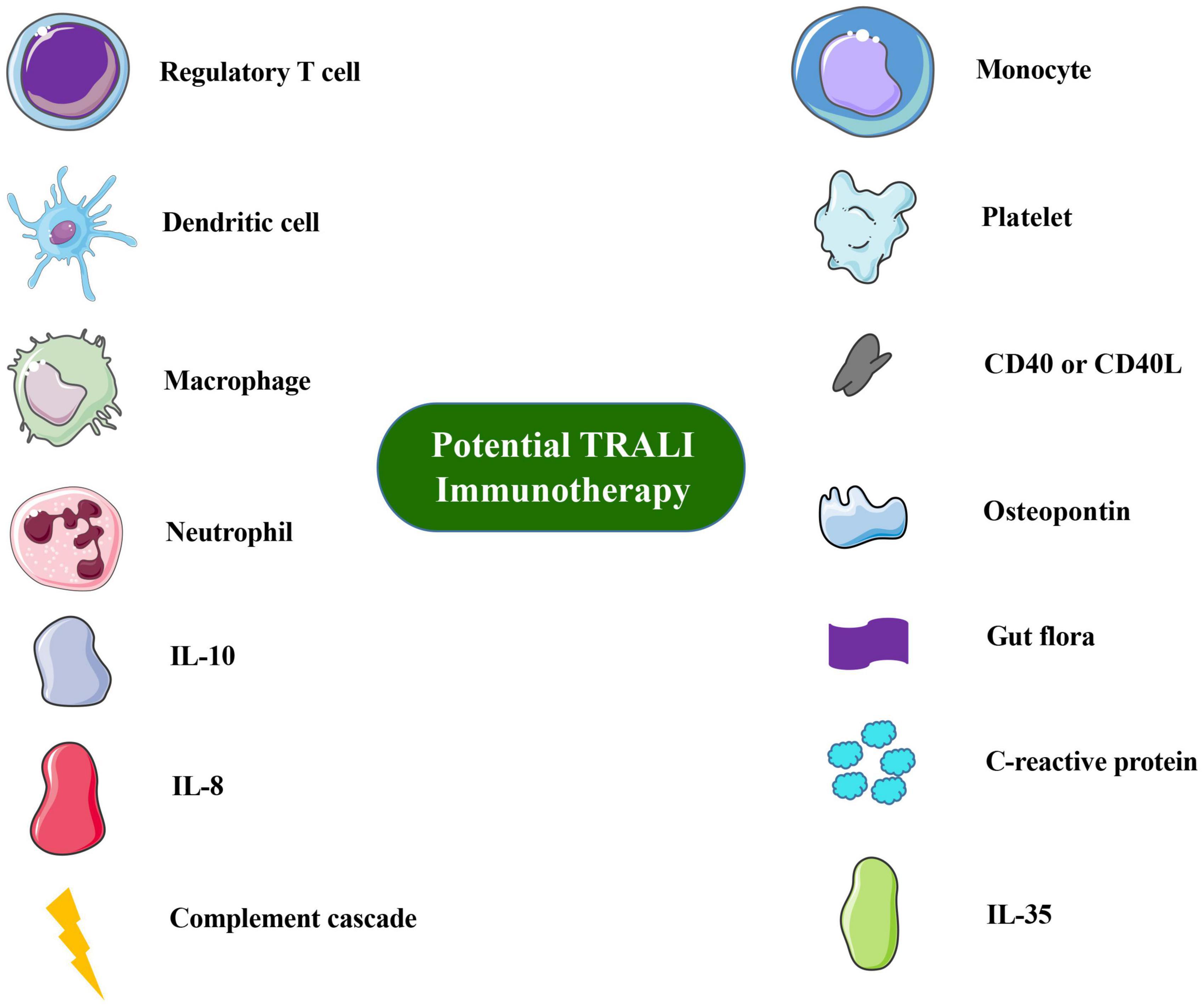
Figure 1. Overview of immune cells or molecules involved in TRALI immunotherapy or prevention. Images of cells and molecules were in part produced or modified using the Smart Servier Medical Art (https://smart.servier.com/), which is licensed under a Creative Commons Attribution 3.0 Unported License (https://screativecommons.org/licenses/by/3.0/).
These studies focused on the immune system and may provide potential new therapeutic strategies for TRALI. It will be important to expand the investigation of immune-mediated treatments for the clinical diagnosis of and decision making regarding TRALI patients.
Author Contributions
KG and SM conceptualized the review. Both authors wrote the review, prepared the figure, and edited and approved the final manuscript.
Funding
This work was supported by grants from Cultivation Fund of Capital Medical University (No. PYZ19033), Cultivation Fund Project of the National Natural Science Foundation in Beijing Children’s Hospital, Capital Medical University (No. GPY201802), and the Children’s Medicine Research Project of Beijing Children’s Hospital, Capital Medical University (Nos. YZQN202003 and YZYB202004).
Conflict of Interest
The authors declare that the research was conducted in the absence of any commercial or financial relationships that could be construed as a potential conflict of interest.
Acknowledgments
We thank our colleagues from the Department of Transfusion Medicine, Beijing Children’s Hospital, Capital Medical University for all their invaluable efforts.
References
Akagi, Y., Murata, S., Yamashita, Y., Tanaka, K., Hiroi, T., Mushino, T., et al. (2020). Two episodes of Transfusion-related Acute Lung Injury (TRALI) occurring within a short period. Intern. Med. 59, 2577–2581. doi: 10.2169/internalmedicine.4700-20
Arbore, G., Kemper, C., and Kolev, M. (2017). Intracellular complement - the complosome - in immune cell regulation. Mol. Immunol. 89, 2–9. doi: 10.1016/j.molimm.2017.05.012
Arnold, L., Henry, A., Poron, F., Baba-Amer, Y., van Rooijen, N., Plonquet, A., et al. (2007). Inflammatory monocytes recruited after skeletal muscle injury switch into antiinflammatory macrophages to support myogenesis. J. Exp. Med. 204, 1057–1069. doi: 10.1084/jem.20070075
Bayat, B., Tjahjono, Y., Berghofer, H., Werth, S., Deckmyn, H., De Meyer, S. F., et al. (2015). Choline transporter-like protein-2: new von willebrand factor-binding partner involved in antibody-mediated neutrophil activation and transfusion-related acute lung injury. Arterioscler. Thromb. Vasc. Biol. 35, 1616–1622. doi: 10.1161/ATVBAHA.115.305259
Bayat, B., Tjahjono, Y., Sydykov, A., Werth, S., Hippenstiel, S., Weissmann, N., et al. (2013). Anti-human neutrophil antigen-3a induced transfusion-related acute lung injury in mice by direct disturbance of lung endothelial cells. Arterioscler. Thromb. Vasc. Biol. 33, 2538–2548. doi: 10.1161/ATVBAHA.113.301206
Benson, A. B., Austin, G. L., Berg, M., McFann, K. K., Thomas, S., Ramirez, G., et al. (2010). Transfusion-related acute lung injury in ICU patients admitted with gastrointestinal bleeding. Intens. Care Med. 36, 1710–1717. doi: 10.1007/s00134-010-1954-x
Boyton, R. J., and Altmann, D. M. (2002). Is selection for TCR affinity a factor in cytokine polarization? Trends Immunol. 23, 526–529. doi: 10.1016/s1471-4906(02)02319-0
Branzk, N., Lubojemska, A., Hardison, S. E., Wang, Q., Gutierrez, M. G., Brown, G. D., et al. (2014). Neutrophils sense microbe size and selectively release neutrophil extracellular traps in response to large pathogens. Nat. Immunol. 15, 1017–1025. doi: 10.1038/ni.2987
Care, A. S., Diener, K. R., Jasper, M. J., Brown, H. M., Ingman, W. V., and Robertson, S. A. (2013). Macrophages regulate corpus luteum development during embryo implantation in mice. J. Clin. Invest. 123, 3472–3487. doi: 10.1172/JCI60561
Caudrillier, A., Kessenbrock, K., Gilliss, B. M., Nguyen, J. X., Marques, M. B., Monestier, M., et al. (2012). Platelets induce neutrophil extracellular traps in transfusion-related acute lung injury. J. Clin. Invest. 122, 2661–2671. doi: 10.1172/JCI61303
Chapman, R. W., Phillips, J. E., Hipkin, R. W., Curran, A. K., Lundell, D., and Fine, J. S. (2009). CXCR2 antagonists for the treatment of pulmonary disease. Pharmacol. Ther. 121, 55–68. doi: 10.1016/j.pharmthera.2008.10.005
Cleary, S. J., Kwaan, N., Tian, J. J., Calabrese, D. R., Mallavia, B., Magnen, M., et al. (2020). Complement activation on endothelium initiates antibody-mediated acute lung injury. J. Clin. Invest. 130, 5909–5923. doi: 10.1172/JCI138136
Clifford, L., Jia, Q., Subramanian, A., Yadav, H., Wilson, G. A., Murphy, S. P., et al. (2015). Characterizing the epidemiology of postoperative transfusion-related acute lung injury. Anesthesiology 122, 12–20. doi: 10.1097/ALN.0000000000000514
Cognasse, F., Tariket, S., Hamzeh-Cognasse, H., Arthaud, C. A., Eyraud, M. A., Bourlet, T., et al. (2020). Platelet depletion limits the severity but does not prevent the occurrence of experimental transfusion-related acute lung injury. Transfusion 60, 713–723. doi: 10.1111/trf.15738
Collison, L. W., Chaturvedi, V., Henderson, A. L., Giacomin, P. R., Guy, C., Bankoti, J., et al. (2010). IL-35-mediated induction of a potent regulatory T cell population. Nat. Immunol. 11, 1093–1101. doi: 10.1038/ni.1952
Curtis, B. R., and McFarland, J. G. (2006). Mechanisms of transfusion-related acute lung injury (TRALI): anti-leukocyte antibodies. Crit. Care Med. 34, S118–S123. doi: 10.1097/01.CCM.0000214293.72918.D8
D’Alessio, F. R., Tsushima, K., Aggarwal, N. R., West, E. E., Willett, M. H., Britos, M. F., et al. (2009). CD4+CD25+Foxp3+ Tregs resolve experimental lung injury in mice and are present in humans with acute lung injury. J. Clin. Invest. 119, 2898–2913. doi: 10.1172/JCI36498
Dardalhon, V., Korn, T., Kuchroo, V. K., and Anderson, A. C. (2008). Role of Th1 and Th17 cells in organ-specific autoimmunity. J. Autoimmun. 31, 252–256. doi: 10.1016/j.jaut.2008.04.017
Dry, S. M., Bechard, K. M., Milford, E. L., Churchill, W. H., and Benjamin, R. J. (1999). The pathology of transfusion-related acute lung injury. Am. J. Clin. Pathol. 112, 216–221. doi: 10.1093/ajcp/112.2.216
Filippi, M.-D. (2019). Neutrophil transendothelial migration: updates and new perspectives. Blood 133, 2149–2158. doi: 10.1182/blood-2018-12-844605
Fiorentino, D. F., Bond, M. W., and Mosmann, T. R. (1989). Two types of mouse T helper cell. IV. Th2 clones secrete a factor that inhibits cytokine production by Th1 clones. J. Exp. Med. 170, 2081–2095.
Fontenot, J. D., Gavin, M. A., and Rudensky, A. Y. (2003). Foxp3 programs the development and function of CD4+CD25+ regulatory T cells. Nat. Immunol. 4, 330–336. doi: 10.1038/ni904
Frantz, S., Hofmann, U., Fraccarollo, D., Schäfer, A., Kranepuhl, S., Hagedorn, I., et al. (2012). Monocytes/macrophages prevent healing defects and left ventricular thrombus formation after myocardial infarction. FASEB J. 27, 871–881. doi: 10.1096/fj.12-214049
Fuller, C. C., Nambudiri, V. E., Spencer-Smith, C., Curtis, L. H., Shinde, M., Cosgrove, A., et al. (2021). Medical chart validation of inpatient diagnosis codes for transfusion-related acute lung injury 2013-2015. Transfusion doi: 10.1111/trf.16251 [Epub ahead of print].
Fung, Y. L., Kim, M., Tabuchi, A., Aslam, R., Speck, E. R., Chow, L., et al. (2010). Recipient T lymphocytes modulate the severity of antibody-mediated transfusion-related acute lung injury. Blood 116, 3073–3079. doi: 10.1182/blood-2010-05-284570
Gallego-Colon, E., Sampson, R. D., Sattler, S., Schneider, M. D., Rosenthal, N., and Tonkin, J. (2015). Cardiac-restricted IGF-1Ea overexpression reduces the early accumulation of inflammatory myeloid cells and mediates expression of extracellular matrix remodelling genes after myocardial infarction. Med. Inflamm. 2015:484357. doi: 10.1155/2015/484357
Gao, Y., Lin, F., Su, J., Gao, Z., Li, Y., Yang, J., et al. (2012). Molecular mechanisms underlying the regulation and functional plasticity of FOXP3(+) regulatory T cells. Genes Immun. 13, 1–13. doi: 10.1038/gene.2011.77
Glod, J., Kobiler, D., Noel, M., Koneru, R., Lehrer, S., Medina, D., et al. (2006). Monocytes form a vascular barrier and participate in vessel repair after brain injury. Blood 107, 940–946. doi: 10.1182/blood-2004-11-4403
Gokhale, A., Hendrickson, J. E. (2019). “Chapter 66 – Transfusion-Related Acute Lung Injury,” in Transfusion Medicine and Hemostasis (Third Edition), eds B. H. Shaz, C. D. Hillyer and G. M. Reyes (Elsevier), 405–408. doi: 10.1016/B978-0-12-813726-0.00066-0
Goren, I., Allmann, N., Yogev, N., Schurmann, C., Linke, A., Holdener, M., et al. (2009). A transgenic mouse model of inducible macrophage depletion: effects of diphtheria toxin-driven lysozyme M-specific cell lineage ablation on wound inflammatory, angiogenic, and contractive processes. Am. J. Pathol. 175, 132–147. doi: 10.2353/ajpath.2009.081002
Gubser, C., Schmaler, M., Rossi, S. W., and Palmer, E. (2016). Monoclonal regulatory T cells provide insights into T cell suppression. Sci. Rep. 6:25758. doi: 10.1038/srep25758
Hakkim, A., Furnrohr, B. G., Amann, K., Laube, B., Abed, U. A., Brinkmann, V., et al. (2010). Impairment of neutrophil extracellular trap degradation is associated with lupus nephritis. Proc. Natl. Acad. Sci. U.S.A. 107, 9813–9818. doi: 10.1073/pnas.0909927107
Hammond, M. E., Lapointe, G. R., Feucht, P. H., Hilt, S., Gallegos, C. A., Gordon, C. A., et al. (1995). IL-8 induces neutrophil chemotaxis predominantly via type I IL-8 receptors. J. Immunol. 155, 1428–1433.
He, R., Li, L., Kong, Y., Tian, L., Tian, X., Fang, P., et al. (2019). Preventing murine transfusion-related acute lung injury by expansion of CD4(+) CD25(+) FoxP3(+) Tregs using IL-2/anti-IL-2 complexes. Transfusion 59, 534–544. doi: 10.1111/trf.15064
Hogarth, P. M., and Pietersz, G. A. (2012). Fc receptor-targeted therapies for the treatment of inflammation, cancer and beyond. Nat. Rev. Drug Discov. 11, 311–331. doi: 10.1038/nrd2909
Holmes, W. E., Lee, J., Kuang, W. J., Rice, G. C., and Wood, W. I. (1991). Structure and functional expression of a human interleukin-8 receptor. Science 253, 1278–1280. doi: 10.1126/science.1840701
Hu, A., Chen, W., Wu, S., Pan, B., Zhu, A., Yu, X., et al. (2020). An animal model of transfusion-related acute lung injury and the role of soluble CD40 ligand. Vox Sang. 115, 303–313. doi: 10.1111/vox.12895
Jongerius, I., Porcelijn, L., van Beek, A. E., Semple, J. W., van der Schoot, C. E., Vlaar, A. P. J., et al. (2019). The role of complement in transfusion-related acute lung injury. Transfus. Med. Rev. 33, 236–242. doi: 10.1016/j.tmrv.2019.09.002
Josefowicz, S. Z., Lu, L. F., and Rudensky, A. Y. (2012). Regulatory T cells: mechanisms of differentiation and function. Annu. Rev. Immunol. 30, 531–564. doi: 10.1146/annurev.immunol.25.022106.141623
Kapur, R., Einarsdottir, H. K., and Vidarsson, G. (2014). IgG-effector functions: “the good, the bad and the ugly”. Immunol. Lett. 160, 139–144. doi: 10.1016/j.imlet.2014.01.015
Kapur, R., Kasetty, G., Rebetz, J., Egesten, A., and Semple, J. W. (2019). Osteopontin mediates murine transfusion-related acute lung injury via stimulation of pulmonary neutrophil accumulation. Blood 134, 74–84. doi: 10.1182/blood.2019000972
Kapur, R., Kim, M., Aslam, R., McVey, M. J., Tabuchi, A., Luo, A., et al. (2017a). T regulatory cells and dendritic cells protect against transfusion-related acute lung injury via IL-10. Blood 129, 2557–2569. doi: 10.1182/blood-2016-12-758185
Kapur, R., Kim, M., Rebetz, J., Rondina, M. T., Porcelijn, L., and Semple, J. W. (2017b). Low levels of interleukin-10 in patients with transfusion-related acute lung injury. Ann. Transl. Med. 5:339. doi: 10.21037/atm.2017.04.37
Kapur, R., Kim, M., Rebetz, J., Hallstrom, B., Bjorkman, J. T., Takabe-French, A., et al. (2018). Gastrointestinal microbiota contributes to the development of murine transfusion-related acute lung injury. Blood Adv. 2, 1651–1663. doi: 10.1182/bloodadvances.2018018903
Kapur, R., Kim, M., Rondina, M. T., Porcelijn, L., and Semple, J. W. (2016). Elevation of C-reactive protein levels in patients with transfusion-related acute lung injury. Oncotarget 7, 78048–78054. doi: 10.18632/oncotarget.12872
Kapur, R., Kim, M., Shanmugabhavananthan, S., Liu, J., Li, Y., and Semple, J. W. (2015). C-reactive protein enhances murine antibody-mediated transfusion-related acute lung injury. Blood 126, 2747–2751. doi: 10.1182/blood-2015-09-672592
Kelher, M. R., Masuno, T., Moore, E. E., Damle, S., Meng, X., Song, Y., et al. (2009). Plasma from stored packed red blood cells and MHC class I antibodies causes acute lung injury in a 2-event in vivo rat model. Blood 113, 2079–2087. doi: 10.1182/blood-2008-09-177857
Khan, S. Y., Kelher, M. R., Heal, J. M., Blumberg, N., Boshkov, L. K., Phipps, R., et al. (2006). Soluble CD40 ligand accumulates in stored blood components, primes neutrophils through CD40, and is a potential cofactor in the development of transfusion-related acute lung injury. Blood 108, 2455–2462. doi: 10.1182/blood-2006-04-017251
Khoy, K., Nguyen, M. V. C., Masson, D., Bardy, B., Drouet, C., and Paclet, M. H. (2017). Transfusion-related acute lung injury: critical neutrophil activation by anti-HLA-A2 antibodies for endothelial permeability. Transfusion 57, 1699–1708. doi: 10.1111/trf.14134
Kleinman, S., Caulfield, T., Chan, P., Davenport, R., McFarland, J., McPhedran, S., et al. (2004). Toward an understanding of transfusion-related acute lung injury: statement of a consensus panel. Transfusion 44, 1774–1789. doi: 10.1111/j.0041-1132.2004.04347.x
Lan, R. Y., Ansari, A. A., Lian, Z. X., and Gershwin, M. E. (2005). Regulatory T cells: development, function and role in autoimmunity. Autoimmun. Rev. 4, 351–363. doi: 10.1016/j.autrev.2005.01.007
Land, W. G. (2013). Transfusion-related acute lung injury: the work of DAMPs. Transfus. Med. Hemother. 40, 3–13. doi: 10.1159/000345688
Landers, D. F., Hill, G. E., Wong, K. C., and Fox, I. J. (1996). Blood transfusion-induced immunomodulation. Anesth. Analg. 82, 187–204. doi: 10.1097/00000539-199601000-00035
Lieberman, L., Petraszko, T., Yi, Q. L., Hannach, B., and Skeate, R. (2014). Transfusion-related lung injury in children: a case series and review of the literature. Transfusion 54, 57–64. doi: 10.1111/trf.12249
Lin, S. L., Li, B., Rao, S., Yeo, E. J., Hudson, T. E., Nowlin, B. T., et al. (2010). Macrophage Wnt7b is critical for kidney repair and regeneration. Proc. Natl. Acad. Sci. U.S.A. 107, 4194–4199. doi: 10.1073/pnas.0912228107
Liu, X., Cao, H., Li, J., Wang, B., Zhang, P., Dong Zhang, X., et al. (2017). Autophagy induced by DAMPs facilitates the inflammation response in lungs undergoing ischemia-reperfusion injury through promoting TRAF6 ubiquitination. Cell Death Differ. 24, 683–693. doi: 10.1038/cdd.2017.1
Loevenich, K., Ueffing, K., Abel, S., Hose, M., Matuschewski, K., Westendorf, A. M., et al. (2017). DC-Derived IL-10 modulates pro-inflammatory cytokine production and promotes induction of CD4(+)IL-10(+) regulatory t cells during Plasmodium yoelii infection. Front. Immunol. 8:152. doi: 10.3389/fimmu.2017.00152
London, A., Cohen, M., and Schwartz, M. (2013). Microglia and monocyte-derived macrophages: functionally distinct populations that act in concert in CNS plasticity and repair. Front. Cell Neurosci. 7:34. doi: 10.3389/fncel.2013.00034
Looney, M. R., Nguyen, J. X., Hu, Y., Van Ziffle, J. A., Lowell, C. A., and Matthay, M. A. (2009). Platelet depletion and aspirin treatment protect mice in a two-event model of transfusion-related acute lung injury. J. Clin. Invest. 119, 3450–3461. doi: 10.1172/JCI38432
Looney, M. R., Roubinian, N., Gajic, O., Gropper, M. A., Hubmayr, R. D., Lowell, C. A., et al. (2014). Prospective study on the clinical course and outcomes in transfusion-related acute lung injury. Crit. Care Med. 42, 1676–1687. doi: 10.1097/CCM.0000000000000323
Looney, M. R., Su, X., Van Ziffle, J. A., Lowell, C. A., and Matthay, M. A. (2006). Neutrophils and their Fc gamma receptors are essential in a mouse model of transfusion-related acute lung injury. J. Clin. Invest. 116, 1615–1623. doi: 10.1172/JCI27238
Lorello, G. R., and Alam, A. (2018). Perioperative transfusion-related acute lung injury. Int. Anesthesiol. Clin. 56, 47–67. doi: 10.1097/AIA.0000000000000178
Lund, S. A., Giachelli, C. M., and Scatena, M. (2009). The role of osteopontin in inflammatory processes. J. Cell Commun. Signal. 3, 311–322. doi: 10.1007/s12079-009-0068-0
Maldonado, R. A., and von Andrian, U. H. (2010). How tolerogenic dendritic cells induce regulatory T cells. Adv Immunol. 108, 111–165. doi: 10.1016/S0065-2776(10)08004_1
Mangalmurti, N. S., Xiong, Z., Hulver, M., Ranganathan, M., Liu, X. H., Oriss, T., et al. (2009). Loss of red cell chemokine scavenging promotes transfusion-related lung inflammation. Blood 113, 1158–1166. doi: 10.1182/blood-2008-07-166264
Maruyama, T., Konkel, J. E., Zamarron, B. F., and Chen, W. (2011). The molecular mechanisms of Foxp3 gene regulation. Semin. Immunol. 23, 418–423. doi: 10.1016/j.smim.2011.06.005
McKenzie, C. G., Kim, M., Singh, T. K., Milev, Y., Freedman, J., and Semple, J. W. (2014). Peripheral blood monocyte-derived chemokine blockade prevents murine transfusion-related acute lung injury (TRALI). Blood 123, 3496–3503. doi: 10.1182/blood-2013-11-536755
McVey, M. J., Weidenfeld, S., Maishan, M., Spring, C., Kim, M., Tabuchi, A., et al. (2021). Platelet extracellular vesicles mediate transfusion-related acute lung injury by imbalancing the sphingolipid rheostat. Blood 137, 690–701. doi: 10.1182/blood.2020005985
Meijer, C., Wiezer, M. J., Diehl, A. M., Schouten, H. J., Schouten, H. J., Meijer, S., et al. (2000). Kupffer cell depletion by CI2MDP-liposomes alters hepatic cytokine expression and delays liver regeneration after partial hepatectomy. Liver 20, 66–77. doi: 10.1034/j.1600-0676.2000.020001066.x
Mirza, R., DiPietro, L. A., and Koh, T. J. (2009). Selective and specific macrophage ablation is detrimental to wound healing in mice. Am. J. Pathol. 175, 2454–2462. doi: 10.2353/ajpath.2009.090248
Miyara, M., and Sakaguchi, S. (2007). Natural regulatory T cells: mechanisms of suppression. Trends Mol. Med. 13, 108–116. doi: 10.1016/j.molmed.2007.01.003
Mocsai, A., Walzog, B., and Lowell, C. A. (2015). Intracellular signalling during neutrophil recruitment. Cardiovasc. Res. 107, 373–385. doi: 10.1093/cvr/cvv159
Mold, J. E., Michaelsson, J., Burt, T. D., Muench, M. O., Beckerman, K. P., Busch, M. P., et al. (2008). Maternal alloantigens promote the development of tolerogenic fetal regulatory T cells in utero. Science 322, 1562–1565. doi: 10.1126/science.1164511
Moll, H. (2003). Dendritic cells and host resistance to infection. Cell Microbiol. 5, 493–500. doi: 10.1046/j.1462-5822.2003.00291.x
Moore, K. W., de Waal Malefyt, R., Coffman, R. L., and O’Garra, A. (2001). Interleukin-10 and the interleukin-10 receptor. Annu. Rev. Immunol. 19, 683–765. doi: 10.1146/annurev.immunol.19.1.683
Murphy, K., and Weaver, C. (2016). Janeway’s Immunobiology: Garland Science, 9th Edn, New York, NY: W.W. Norton & Company.
Nahrendorf, M., Swirski, F. K., Aikawa, E., Stangenberg, L., Wurdinger, T., Figueiredo, J. L., et al. (2007). The healing myocardium sequentially mobilizes two monocyte subsets with divergent and complementary functions. J. Exp. Med. 204, 3037–3047. doi: 10.1084/jem.20070885
Nishimura, M., Takanashi, M., Okazaki, H., and Satake, M. (2006). Lung microvascular endothelial cell injury caused by treatment with polymorphonuclear neutrophils and low-IgM serum: a model of transfusion-related acute lung injury. Lung 184, 25–32. doi: 10.1007/s00408-005-2559-y
Nunn, M. A., Sharma, A., Paesen, G. C., Adamson, S., Lissina, O., Willis, A. C., et al. (2005). Complement inhibitor of C5 activation from the soft tick Ornithodoros moubata. J. Immunol. 174, 2084–2091. doi: 10.4049/jimmunol.174.4.2084
Oakley, O. R., Frazer, M. L., and Ko, C. (2011). Pituitary-ovary-spleen axis in ovulation. Trends Endocrinol. Metab. 22, 345–352. doi: 10.1016/j.tem.2011.04.005
O’Byrne, P. M., Metev, H., Puu, M., Richter, K., Keen, C., Uddin, M., et al. (2016). Efficacy and safety of a CXCR2 antagonist, AZD5069, in patients with uncontrolled persistent asthma: a randomised, double-blind, placebo-controlled trial. Lancet Respir. Med. 4, 797–806. doi: 10.1016/S2213-2600(16)30227-2
O’Regan, A. (2003). The role of osteopontin in lung disease. Cytokine Growth Fact. Rev. 14, 479–488. doi: 10.1016/s1359-6101(03)00055-8
Passwater, M. (2018). Antibody formation in transfusion therapy. J. Infus. Nurs. 41, 87–95. doi: 10.1097/NAN.0000000000000264
Patel, S. K., and Janjic, J. M. (2015). Macrophage targeted theranostics as personalized nanomedicine strategies for inflammatory diseases. Theranostics 5, 150–172. doi: 10.7150/thno.9476
Peters, A. L., van Hezel, M. E., Juffermans, N. P., and Vlaar, A. P. (2015a). Pathogenesis of non-antibody mediated transfusion-related acute lung injury from bench to bedside. Blood Rev. 29, 51–61. doi: 10.1016/j.blre.2014.09.007
Peters, A. L., van Stein, D., and Vlaar, A. P. (2015b). Antibody-mediated transfusion-related acute lung injury; from discovery to prevention. Br. J. Haematol. 170, 597–614. doi: 10.1111/bjh.13459
Popovsky, M. A., Abel, M. D., and Moore, S. B. (1983). Transfusion-related acute lung injury associated with passive transfer of antileukocyte antibodies. Am. Rev. Respir. Dis. 128, 185–189. doi: 10.1164/arrd.1983.128.1.185
Popovsky, M. A., and Moore, S. B. (1985). Diagnostic and pathogenetic considerations in transfusion-related acute lung injury. Transfusion 25, 573–577.
Qiao, J., He, R., Yin, Y., Tian, L., Li, L., Lian, Z., et al. (2020). rIL-35 prevents murine transfusion-related acute lung injury by inhibiting the activation of endothelial cells. Transfusion 60, 1434–1442. doi: 10.1111/trf.15805
Rebetz, J., Semple, J. W., and Kapur, R. (2018). The pathogenic involvement of neutrophils in acute respiratory distress syndrome and transfusion-related acute lung injury. Transfus. Med. Hemother. 45, 290–298. doi: 10.1159/000492950
Ricklin, D., Hajishengallis, G., Yang, K., and Lambris, J. D. (2010). Complement: a key system for immune surveillance and homeostasis. Nat. Immunol. 11, 785–797. doi: 10.1038/ni.1923
Roubinian, N. H., Looney, M. R., Kor, D. J., Lowell, C. A., Gajic, O., Hubmayr, R. D., et al. (2015). Cytokines and clinical predictors in distinguishing pulmonary transfusion reactions. Transfusion 55, 1838–1846. doi: 10.1111/trf.13021
Rueda, C. M., Jackson, C. M., and Chougnet, C. A. (2016). Regulatory T-cell-mediated suppression of conventional T-cells and dendritic cells by different cAMP intracellular pathways. Front. Immunol. 7:216. doi: 10.3389/fimmu.2016.00216
Rutitzky, L. I., Bazzone, L., Shainheit, M. G., Joyce-Shaikh, B., Cua, D. J., and Stadecker, M. J. (2008). IL-23 is required for the development of severe egg-induced immunopathology in schistosomiasis and for lesional expression of IL-17. J. Immunol. 180, 2486–2495.
Sadik, C. D., and Luster, A. D. (2012). Lipid-cytokine-chemokine cascades orchestrate leukocyte recruitment in inflammation. J. Leukoc. Biol. 91, 207–215. doi: 10.1189/jlb.0811402
Sakaguchi, S., Miyara, M., Costantino, C. M., and Hafler, D. A. (2010). FOXP3+ regulatory T cells in the human immune system. Nat. Rev. Immunol. 10, 490–500. doi: 10.1038/nri2785
Sakaguchi, S., Ono, M., Setoguchi, R., Yagi, H., Hori, S., Fehervari, Z., et al. (2006). Foxp3+ CD25+ CD4+ natural regulatory T cells in dominant self-tolerance and autoimmune disease. Immunol. Rev. 212, 8–27. doi: 10.1111/j.0105-2896.2006.00427.x
Sakaguchi, S., Yamaguchi, T., Nomura, T., and Ono, M. (2008). Regulatory T cells and immune tolerance. Cell 133, 775–787. doi: 10.1016/j.cell.2008.05.009
Salas, A., and Panes, J. (2015). IBD: regulatory T cells for treatment of Crohn’s disease. Nat. Rev. Gastroenterol. Hepatol. 12, 315–316. doi: 10.1038/nrgastro.2015.68
Sattler, S. (2017). The role of the immune system beyond the fight against infection. Adv. Exp. Med. Biol. 1003, 3–14. doi: 10.1007/978-3-319-57613-8_1
Sattler, S., and Rosenthal, N. (2016). The neonate versus adult mammalian immune system in cardiac repair and regeneration. Biochim. Biophys. Acta 1863, 1813–1821. doi: 10.1016/j.bbamcr.2016.01.011
Semple, J. W., and Kapur, R. (2020). The contribution of recipient platelets in TRALI: has the jury reached a verdict? Transfusion 60, 886–888. doi: 10.1111/trf.15814
Semple, J. W., Kim, M., Hou, J., McVey, M., Lee, Y. J., Tabuchi, A., et al. (2012). Intravenous immunoglobulin prevents murine antibody-mediated acute lung injury at the level of neutrophil reactive oxygen species (ROS) production. PLoS One 7:e31357. doi: 10.1371/journal.pone.0031357
Semple, J. W., McVey, M. J., Kim, M., Rebetz, J., Kuebler, W. M., and Kapur, R. (2018). Targeting transfusion-related acute lung injury: the journey from basic science to novel therapies. Crit. Care Med. 46, e452–e458. doi: 10.1097/CCM.0000000000002989
Semple, J. W., Rebetz, J., and Kapur, R. (2019). Transfusion-associated circulatory overload and transfusion-related acute lung injury. Blood 133, 1840–1853. doi: 10.1182/blood-2018-10-860809
Seno, H., Miyoshi, H., Brown, S. L., Geske, M. J., Colonna, M., and Stappenbeck, T. S. (2009). Efficient colonic mucosal wound repair requires Trem2 signaling. Proc. Natl. Acad. Sci. U.S.A. 106, 256–261. doi: 10.1073/pnas.0803343106
Shevach, E. M. (2009). Mechanisms of foxp3+ T regulatory cell-mediated suppression. Immunity 30, 636–645. doi: 10.1016/j.immuni.2009.04.010
Silliman, C. C. (2006). The two-event model of transfusion-related acute lung injury. Crit. Care Med. 34, S124–S131. doi: 10.1097/01.CCM.0000214292.62276.8E
Silliman, C. C., Ambruso, D. R., and Boshkov, L. K. (2005). Transfusion-related acute lung injury. Blood 105, 2266–2273. doi: 10.1182/blood-2004-07-2929
Silliman, C. C., Curtis, B. R., Kopko, P. M., Khan, S. Y., Kelher, M. R., Schuller, R. M., et al. (2007). Donor antibodies to HNA-3a implicated in TRALI reactions prime neutrophils and cause PMN-mediated damage to human pulmonary microvascular endothelial cells in a two-event in vitro model. Blood 109, 1752–1755. doi: 10.1182/blood-2006-05-025106
Silliman, C. C., Paterson, A. J., Dickey, W. O., Stroneck, D. F., Popovsky, M. A., Caldwell, S. A., et al. (1997). The association of biologically active lipids with the development of transfusion-related acute lung injury: a retrospective study. Transfusion 37, 719–726. doi: 10.1046/j.1537-2995.1997.37797369448.x
Soares, R. R., Antinarelli, L. M. R., Abramo, C., Macedo, G. C., Coimbra, E. S., and Scopel, K. K. G. (2017). What do we know about the role of regulatory B cells (Breg) during the course of infection of two major parasitic diseases, malaria and leishmaniasis? Pathog. Glob. Health 111, 107–115. doi: 10.1080/20477724.2017.1308902
Steinman, R. M., Hawiger, D., and Nussenzweig, M. C. (2003). Tolerogenic dendritic cells. Annu. Rev. Immunol. 21, 685–711. doi: 10.1146/annurev.immunol.21.120601.141040
Storisteanu, D. M., Pocock, J. M., Cowburn, A. S., Juss, J. K., Nadesalingam, A., Nizet, V., et al. (2017). Evasion of neutrophil extracellular traps by respiratory pathogens. Am. J. Respir. Cell Mol. Biol. 56, 423–431. doi: 10.1165/rcmb.2016-0193PS
Strait, R. T., Hicks, W., Barasa, N., Mahler, A., Khodoun, M., Kohl, J., et al. (2011). MHC class I-specific antibody binding to nonhematopoietic cells drives complement activation to induce transfusion-related acute lung injury in mice. J. Exp. Med. 208, 2525–2544. doi: 10.1084/jem.20110159
Swanson, K., Dwyre, D. M., Krochmal, J., and Raife, T. J. (2006). Transfusion-related acute lung injury (TRALI): current clinical and pathophysiologic considerations. Lung 184, 177–185. doi: 10.1007/s00408-005-2578-8
Tariket, S., Hamzeh-Cognasse, H., Laradi, S., Arthaud, C. A., Eyraud, M. A., Bourlet, T., et al. (2019). Evidence of CD40L/CD40 pathway involvement in experimental transfusion-related acute lung injury. Sci. Rep. 9:12536. doi: 10.1038/s41598-019-49040-0
Taylor, M. D., van der Werf, N., and Maizels, R. M. (2012). T cells in helminth infection: the regulators and the regulated. Trends Immunol. 33, 181–189. doi: 10.1016/j.it.2012.01.001
Thomas, G. M., Carbo, C., Curtis, B. R., Martinod, K., Mazo, I. B., Schatzberg, D., et al. (2012). Extracellular DNA traps are associated with the pathogenesis of TRALI in humans and mice. Blood 119, 6335–6343. doi: 10.1182/blood-2012-01-405183
Thornby, K. A., Johnson, A., and Axtell, S. (2014). Dornase alfa for non-cystic fibrosis pediatric pulmonary Atelectasis. Ann. Pharmacother. 48, 1040–1049. doi: 10.1177/1060028014535199
Tolle, L. B., and Standiford, T. J. (2013). Danger-associated molecular patterns (DAMPs) in acute lung injury. J. Pathol. 229, 145–156. doi: 10.1002/path.4124
Tonkin, J., Temmerman, L., Sampson, R. D., Gallego-Colon, E., Barberi, L., Bilbao, D., et al. (2015). Monocyte/macrophage-derived IGF-1 orchestrates murine skeletal muscle regeneration and modulates autocrine polarization. Mol. Ther. 23, 1189–1200. doi: 10.1038/mt.2015.66
Toy, P., Gajic, O., Bacchetti, P., Looney, M. R., Gropper, M. A., Hubmayr, R., et al. (2012). Transfusion-related acute lung injury: incidence and risk factors. Blood 119, 1757–1767. doi: 10.1182/blood-2011-08-370932
Toy, P., Popovsky, M. A., Abraham, E., Ambruso, D. R., Holness, L. G., Kopko, P. M., et al. (2005). Transfusion-related acute lung injury: definition and review. Crit. Care Med. 33, 721–726. doi: 10.1097/01.ccm.0000159849.94750.51
Tung, J.-P. (2019). Transfusion-related acute lung injury (Trali): pathogenesis and diagnosis. Pathology 51:S44.
Tung, J. P., Fung, Y. L., Nataatmadja, M., Colebourne, K. I., Esmaeel, H. M., Wilson, K., et al. (2011). A novel in vivo ovine model of transfusion-related acute lung injury (TRALI). Vox Sang. 100, 219–230. doi: 10.1111/j.1423-0410.2010.01381.x
Velavan, T. P., and Ojurongbe, O. (2011). Regulatory T cells and parasites. J. Biomed. Biotechnol. 2011:520940. doi: 10.1155/2011/520940
Venet, F., Chung, C. S., Huang, X., Lomas-Neira, J., Chen, Y., and Ayala, A. (2009). Lymphocytes in the development of lung inflammation: a role for regulatory CD4+ T cells in indirect pulmonary lung injury. J. Immunol. 183, 3472–3480. doi: 10.4049/jimmunol.0804119
Vidarsson, G., and van de Winkel, J. G. (1998). Fc receptor and complement receptor-mediated phagocytosis in host defence. Curr. Opin. Infect. Dis. 11, 271–278. doi: 10.1097/00001432-199806000-00002
Vlaar, A. P., Hofstra, J. J., Kulik, W., van Lenthe, H., Nieuwland, R., Schultz, M. J., et al. (2010). Supernatant of stored platelets causes lung inflammation and coagulopathy in a novel in vivo transfusion model. Blood 116, 1360–1368. doi: 10.1182/blood-2009-10-248732
Vlaar, A. P. J., Toy, P., Fung, M., Looney, M. R., Juffermans, N. P., Bux, J., et al. (2019). A consensus redefinition of transfusion-related acute lung injury. Transfusion 59, 2465–2476. doi: 10.1111/trf.15311
Voelker, M. T., and Spieth, P. (2019). Blood transfusion associated lung injury. J. Thorac. Dis. 11, 3609–3615. doi: 10.21037/jtd.2019.06.61
Wang, L., Wu, T., Yan, S., Wang, Y., An, J., Wu, C., et al. (2020). M1-polarized alveolar macrophages are crucial in a mouse model of transfusion-related acute lung injury. Transfusion 60, 303–316. doi: 10.1111/trf.15609
Watanabe, K., and Petri, W. A. (2019). Learning from the research on amebiasis and gut microbiome: is stimulation by gut flora essential for effective neutrophil mediated protection from external pathogens? Gut Microb. 10, 100–104. doi: 10.1080/19490976.2018.1479626
Waugh, D. J., and Wilson, C. (2008). The interleukin-8 pathway in cancer. Clin. Cancer Res. 14, 6735–6741. doi: 10.1158/1078-0432.CCR-07-4843
Wen, K., Li, G., Yang, X., Bui, T., Bai, M., Liu, F., et al. (2012). CD4+ CD25- FoxP3+ regulatory cells are the predominant responding regulatory T cells after human rotavirus infection or vaccination in gnotobiotic pigs. Immunology 137, 160–171. doi: 10.1111/j.1365-2567.2012.03617.x
Wong, S. L., Demers, M., Martinod, K., Gallant, M., Wang, Y., Goldfine, A. B., et al. (2015). Diabetes primes neutrophils to undergo NETosis, which impairs wound healing. Nat. Med. 21, 815–819. doi: 10.1038/nm.3887
Wyman, T. H., Bjornsen, A. J., Elzi, D. J., Smith, C. W., England, K. M., Kelher, M., et al. (2002). A two-insult in vitro model of PMN-mediated pulmonary endothelial damage: requirements for adherence and chemokine release. Am. J. Physiol. Cell Physiol. 283, C1592–C1603. doi: 10.1152/ajpcell.00540.2001
Xie, R. F., Hu, P., Wang, Z. C., Yang, J., Yang, Y. M., Gao, L., et al. (2015). Platelet-derived microparticles induce polymorphonuclear leukocyte-mediated damage of human pulmonary microvascular endothelial cells. Transfusion 55, 1051–1057. doi: 10.1111/trf.12952
Xu, A., Liu, Y., Chen, W., Wang, J., Xue, Y., Huang, F., et al. (2016). TGF-beta-induced regulatory T cells directly suppress B cell responses through a noncytotoxic mechanism. J. Immunol. 196, 3631–3641. doi: 10.4049/jimmunol.1501740
Yildiz, C., Palaniyar, N., Otulakowski, G., Khan, M. A., Post, M., Kuebler, W. M., et al. (2015). Mechanical ventilation induces neutrophil extracellular trap formation. Anesthesiology 122, 864–875. doi: 10.1097/ALN.0000000000000605
Zeeuw van der Laan, E. A. N., van der Velden, S., Bentlage, A. E. H., Larsen, M. D., van Osch, T. L. J., Mok, J. Y., et al. (2020a). Biological and structural characterization of murine TRALI antibody reveals increased Fc-mediated complement activation. Blood Adv. 4, 3875–3885. doi: 10.1182/bloodadvances.2020002291
Zeeuw van der Laan, E. A. N., van der Velden, S., Porcelijn, L., Semple, J. W., van der Schoot, C. E., and Kapur, R. (2020b). Update on the pathophysiology of transfusion-related acute lung injury. Curr. Opin. Hematol. 27, 386–391. doi: 10.1097/MOH.0000000000000607
Zhang, M. Z., Yao, B., Yang, S., Jiang, L., Wang, S., Fan, X., et al. (2012). CSF-1 signaling mediates recovery from acute kidney injury. J. Clin. Invest. 122, 4519–4532. doi: 10.1172/JCI60363
Keywords: transfusion-related acute lung injury, immune system, immune molecule, immunotherapy, prevention
Citation: Guo K and Ma S (2021) The Immune System in Transfusion-Related Acute Lung Injury Prevention and Therapy: Update and Perspective. Front. Mol. Biosci. 8:639976. doi: 10.3389/fmolb.2021.639976
Received: 10 December 2020; Accepted: 05 March 2021;
Published: 24 March 2021.
Edited by:
Wen Li, Zhejiang University, ChinaReviewed by:
Ajay Dixit, University of Minnesota Twin Cities, United StatesJohn W. Semple, Lund University, Sweden
Copyright © 2021 Guo and Ma. This is an open-access article distributed under the terms of the Creative Commons Attribution License (CC BY). The use, distribution or reproduction in other forums is permitted, provided the original author(s) and the copyright owner(s) are credited and that the original publication in this journal is cited, in accordance with accepted academic practice. No use, distribution or reproduction is permitted which does not comply with these terms.
*Correspondence: Kai Guo, Z3Vva2FpMjIzQG91dGxvb2suY29t; Mjg1NjQ3MTUyQHFxLmNvbQ==; Shuxuan Ma, bWFzeGZ3eXlAc2luYS5jb20=