- 1Department of Biological Sciences, College of Biological Sciences and Biotechnology, Chungnam National University, Daejeon, South Korea
- 2State Key Laboratory of Agricultural Microbiology, College of Life Science and Technology, Huazhong Agricultural University, Wuhan, China
- 3Basic Research Laboratory, Center for Cancer Research, National Cancer Institute, Bethesda, MD, United States
In bacteria, mRNA decay is a major mechanism for regulating gene expression. In Escherichia coli, mRNA decay initiates with endonucleolytic cleavage by RNase E. Translating ribosomes impede RNase E cleavage, thus providing stability to mRNA. In transcripts containing multiple cistrons, the translation of each cistron initiates separately. The effect of internal translation initiations on the decay of polycistronic transcripts remains unknown, which we have investigated here using the four-cistron galETKM transcript. We find that RNase E cleaves a few nucleotides (14–36) upstream of the translation initiation site of each cistron, generating decay intermediates galTKM, galKM, and galM mRNA with fewer but full cistrons. Blocking translation initiation reduced stability, particularly of the mutated cistrons and when they were the 5′-most cistrons. This indicates that, together with translation failure, the location of the cistron is important for its elimination. The instability of the 5′-most cistron did not propagate to the downstream cistrons, possibly due to translation initiation there. Cistron elimination from the 5′ end was not always sequential, indicating that RNase E can also directly access a ribosome-free internal cistron. The finding in gal operon of mRNA decay by cistron elimination appears common in E. coli and Salmonella.
Introduction
In bacteria, mRNA concentration is modulated to cope with a rapidly changing environment. The modulation is mediated by varying the synthesis as well as the decay rates of mRNA. In Escherichia coli, mRNA decay is primarily initiated by the endoribonuclease RNase E (Ono and Kuwano, 1979; Mudd et al., 1990; Babitzke and Kushner, 1991; Melefors and von Gabain, 1991; Taraseviciene et al., 1991; Carpousis et al., 2009). RNase E can target mRNAs for the initial cleavage either by first sensing a 5′ monophosphate group (5′ end-dependent pathway) or by bypassing this requirement (direct access pathway) (Mackie, 2013; Hui et al., 2014).
The 5′ end-dependent pathway begins with RNase E binding to the mono-phosphorylated 5′ end of transcripts (Celesnik et al., 2007) and cleavage at an internal site rich in AU sequences (McDowall et al., 1994). The N-terminal sensor domain of RNase E allows it to bind preferentially to the mono-phosphorylated rather than to the tri- or di-phosphorylated 5′ end of mRNA (Mackie, 1998; Jiang and Belasco, 2004; Callaghan et al., 2005). Thus, the 5′ end-dependent access requires prior conversion of transcripts with 5′ tri- or di-phosphorylated end to mono-phosphorylated end by RNA pyrophosphohydrolase (RppH) (Celesnik et al., 2007; Deana et al., 2008). RNase E can also bypass the 5′ end binding and access a cleavage site internal to the message directly (Joyce and Dreyfus, 1998; Baker and Mackie, 2003). In either case, RNase E cleavage results in the generation of the 5′ portion of mRNA with a new 3′-OH end and the 3′ portion of mRNA with a new 5′ end. Generally, the 5′ portion mRNA is degraded immediately by 3′→ 5′ exonuclease digestion (Mott et al., 1985; Belasco et al., 1986; Hui et al., 2014; Wang et al., 2019). The 3′ portion mRNA could be subjected to decay by further rounds of cleavage by RNase E by either of the pathways (Joyce and Dreyfus, 1998; Spickler et al., 2001; Hui et al., 2014).
There are several mechanisms that modulate RNase E activity. Secondary structures at the 5′ end can extend the stability of mRNA because these structures interfere with RNase E initial cleavage (Bouvet and Belasco, 1992; Emory et al., 1992). Translation has a substantial effect on mRNA decay since translating ribosomes tend to inhibit RNase E cleavage (Braun et al., 1998; Deana and Belasco, 2005; Dreyfus, 2009). By the same token, translation initiation also promotes the stability of mRNA (Arnold et al., 1998; Joyce and Dreyfus, 1998; Baker and Mackie, 2003). In view of these modulating factors, it is unsurprising that polycistronic mRNAs show a differential decay of component cistrons (Belasco et al., 1985; Newbury et al., 1987; Adhya, 2003; Dar and Sorek, 2018).
Several models to account for the decay process of polycistronic mRNA in relation to translating ribosomes have been proposed (Alifano et al., 1994a). In polycistronic mRNA, the decay process is expected to be complex because, other than the secondary structures on RNA that interfere with RNase E and/or exoribonuclease activities, translation initiation at the internal start sites of each of the comprising cistrons could also be a compounding factor. Here we have addressed the decay process of a polycistronic mRNA in relation to translation initiation using the well-studied gal operon of E. coli. The operon harbors four genes–galE, galT, galK, and galM (about 1 kb each) – and produces the full-length mRNA galETKM of 4.3-kb size with the structure: 5′-galE–galT–galK–galM-terminator hairpin-3′ (Figure 1A; Adhya, 2003). The terminator hairpin causes Rho-independent transcription termination and blocks 3′→ 5′ exoribonuclease digestion initiated from the free 3′-OH end of the transcript, providing stability to the galETKM mRNA (Figure 1B; Wang et al., 2019). Interestingly, the operon also produces two other mRNA species, galETK and galTKM, both about 3.3 kb. The galETK mRNA, 5′-galE–galT–galK-3′, is generated by Rho-dependent transcription termination (Lee et al., 2008; Wang et al., 2014, 2015). How the galTKM mRNA, 5′-galT–galK–galM-3′, is generated is not clear.
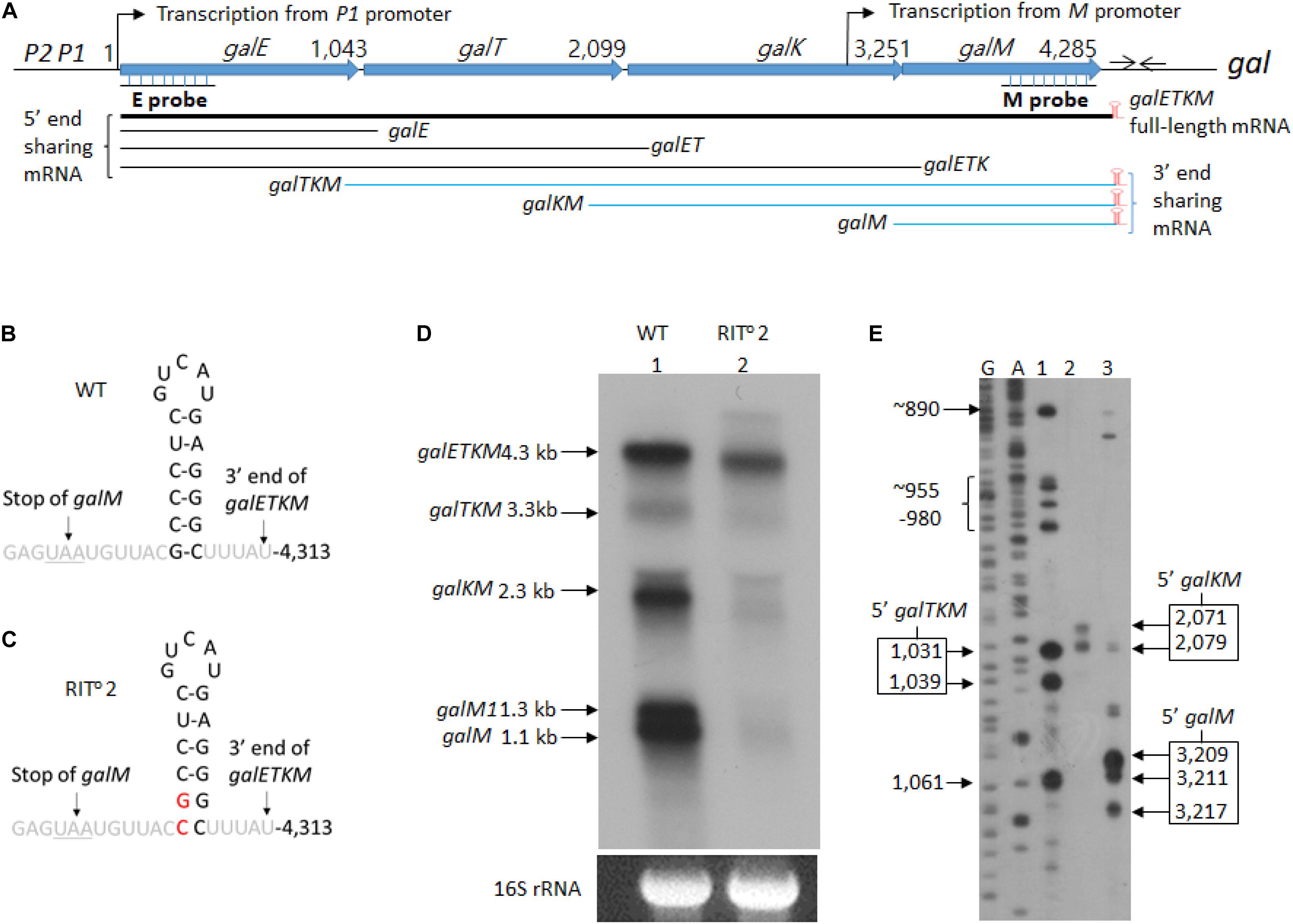
Figure 1. mRNA intermediates of the gal operon that share the 3′ end with the full-length gal mRNA. (A) Map of galETKM operon comprising the genes galE, galT, galK, and galM (Lewis and Adhya, 2015). Numbers indicate gal nucleotide residue coordinate, where 1 is the start of transcription from the galP1 promoter. The number at the end of each gene belongs to the last nucleotide of the stop codon of that gene. The gal operon transcription from the M promoter initiates at 3,073. At six nucleotides downstream of the stop codon of galM gene, there is a 17-nucleotide inverted repeat sequence (head-to-head arrows). It forms the terminator hairpin (red) that terminates transcription and protects galETKM mRNA from 3′→5′ exonuclease digestion (McLaren et al., 1991; Wang et al., 2019). Two probes, E and M (underlined), were used to identify intermediates that share the 5′ and the 3′ ends with the full-length galETKM mRNA. The 3′-end-sharing mRNA shares the same 3′ end at 4,313 and have 5′ ends at the beginning of each comprising gene. (B) Nucleotide sequence of the terminator hairpin. (C) Nucleotide sequence of the terminator hairpin in the RIT02 gal mutant. The nucleotide changes are indicated in red. (D) Northern blot of wild type (WT; lane 1) and RIT02 gal mutant (lane 2). gal mRNA prepared from cells grown up to OD600 of 0.6 in Luria broth with 0.5% galactose. The blot was probed with the M probe complementary to the 3′ half of the galM gene of the gal operon (A). (E) 5′RACE of WT gal mRNA prepared under the same condition as in (D). The lanes show the 5′ ends found near the beginning of either galT (lane 1) or galK (lane 2) or galM (lane 3) genes. DNA sequencing ladders used as molecular weight markers are in lanes marked G and A. Note that the 5′RACE procedure depends on a ligation step that requires a mono-phosphorylated 5′ end. Thus, 5′RACE detects specifically 5′ ends of mRNA that are mono-phosphorylated.
In E. coli, mRNA decay is generally believed to proceed in 5′ to 3′ direction without any known 5′ to 3′ exoribonuclease (Apirion, 1973). There are also a number of well-documented examples of mRNAs whose degradation begins with cleavage far from the 5′ end (von Gabain et al., 1983; Belasco et al., 1985; Newbury et al., 1987; Braun et al., 1998). In addition to galTKM mRNA, the gal operon also produces galKM (formerly known as mK2; Lee et al., 2008). Most intermediate gal mRNA species are stable enough to be detected by northern blot and harbor full open reading frames (ORF) of the remaining cistrons. In other words, gal operon produces mRNA species that are cistron(s)-shorter from the 5′ end of the full-length mRNA. Here we have addressed whether the intermediates galTKM and galKM mRNA are generated by cistron elimination of galETKM from the 5′ end.
In this study, using northern blot and 5′RACE assays, we found that the decay of polycistronic galETKM mRNA by and large proceeds by eliminating nearly full-length cistron(s) from the 5′ end, generating galTKM, galKM, and galM mRNA species as decay intermediates, but the elimination is not always sequential. Translation initiation at the newly generated 5′ end of the intermediate mRNA contributes to the stability of the decay intermediates. A sequence analysis of the 5′ end of mRNA generated as a result of RNase E cleavage (Chao et al., 2017) shows that eliminating cistrons from the 5′ end is the common theme in polycistronic mRNA decay in E. coli as well as in Salmonella.
Materials and Methods
Extraction of RNA From E. coli Cells
Total RNA was prepared from 2 × 108 E. coli cells grown to OD600 of 0.6 in Luria broth (LB) as described previously (Wang et al., 2014). The primers used in this study are listed in Supplementary Table S1.
5′RACE
To determine the 5′ end of the gal mRNA, we ligated the 3′ end of the 5S rRNA in the RNA preparation from E. coli cells to 5′ ends of RNA in the same RNA preparation (Lee et al., 2008). Later, the gal mRNA of interest was amplified using RT-PCR. First, RNA ligation reaction was performed in a 15-μl volume containing 2.5 μg of total RNA, 5 U T4 RNA ligase (Ambion, United States), and 10 U rRNasin (Promega, United States) at 37°C for 3 h. One microgram of the ligated RNA was reverse-transcribed at 37°C for 2 h in a 20-μl reaction volume containing 4 U reverse transcriptase (Qiagen, Germany), 0.5 mM each of dNTP, 10 μM random hexamer primer (Takara, Japan), and 10 U rRNasin. Two microliters of the reverse transcription reaction was used as template for PCR amplification of the gal cDNA of interest in the next step in a total volume of 20 μl using 1 U of HotStar Taq DNA polymerase (Qiagen, Germany), with a forward primer complementary to the 3′ end of the E. coli 5S rRNA and a reverse primer specific to a gal cDNA of interest (Lee et al., 2008) (listed in Supplementary Table S1). The 5′ end of the gal cDNA (thus, gal mRNA) of interest was assayed by extending a 32P-labeled DNA primer bound to a specific region of the amplified gal cDNA of interest. This “primer extension” reaction was performed in a 20-μl volume containing 10 μl of amplified gal cDNA reaction, 0.75 μl 32P-labeled primer, 0.15 mM each of dNTP, and 1 U Taq polymerase with 25 cycles of amplification. The exact location of the 5′ ends of the gal mRNA was identified as follows: The extended-primer DNAs were resolved on 8% polyacrylamide–urea sequencing gel, and radioactive bands were visualized on an X-ray film. We measured the number of nucleotides of the extended-primer DNAs using the DNA sequencing ladder as a ruler. By subtracting the number of nucleotides in the primer DNA from the number of nucleotides of the extended-primer DNA, we located the exact position of the 5′ end of the gal mRNA in the nucleotide residue coordinates of the gal operon.
Northern Blot
Typically, 10 μg of total RNA (after staining with 1 μg/ml ethidium bromide) was resolved by electrophoresis using 1.2% (wt/vol) formaldehyde–agarose gel at 5 V/cm for 4 h. RNA on the gel was then transferred overnight to a positively charged nylon membrane (Ambion, United States) using a downward transfer system (TurboBlotter, Whatman, United Kingdom). The nylon membrane was baked at 80°C for 1 h. M3 probe was prepared by PCR using a pair of primers complementary to 3,751 and 4,285 in gal coordinates. Probe DNA was labeled with 32P. Hybridization procedures followed the manufacturer’s instructions (Ambion, United States). RNA bands were quantified using the software JMOL (NIH).
Results
mRNA Intermediates of the gal Operon That Share the 3′ End With the Full-Length gal mRNA
We performed northern blot of total RNA prepared from wild-type E. coli cells (MG1655) grown in LB supplemented with 0.5% galactose to OD600 of 0.6. The blots were analyzed using the M-probe, which hybridizes to the distal half of the last gene of the gal operon, galM (Figure 1A). We detected five mRNA species with sizes of 4.3, 3.3, 2.3, 1.3, and 1.1 kb (lane 1 in Figure 1D). We named these mRNA species galETKM, galTKM, galKM, galM1, and galM, respectively, as we show later that these mRNA species have their 5′ ends at the beginning of each cistron but have the same 3′ end, which is at the end of the operon (Figure 1A). We called these mRNA species “3′-end-sharing” mRNA.
The 1.3-kb mRNA named galM1 turned out to be the result of transcription initiation at an internal promoter in front of the galM gene, which terminated at the end of galM (Supplementary Figure S1). We designated the internal promoter as M promoter. We excluded the galM1 and galETKM mRNA species from the 3′-end-sharing group of decay intermediates because these are primary transcripts (see below).
To test if the 3′-end-sharing mRNA species harbor the terminator hairpin structure of the full-length galETKM 3′ end (Figures 1A,B; Wang et al., 2019), we performed northern blot on RNA prepared from the culture of MG1655Δgal strain, where the chromosomal gal operon is entirely deleted. The strain harbored a pBAC-derived low-copy-number plasmid that carries a mutant version of the gal operon, RITo2 (Wang et al., 2014). In the RITo2 mutant, two bases from the bottom of the stem of the terminator hairpin are substituted to their complementary bases (Figure 1C). In the RITo2 mutant, the function of the terminator hairpin in the blockage of 3′→ 5′ exoribonuclease was impaired (Wang et al., 2019). When RNA from the RITo2 mutant was analyzed using the M-probe, we confirmed that the mutations caused the shortening of the galETKM mRNA by about 100 nucleotides and also decreased the galETKM amount to about 40% of the wild type (WT; lane 2 in Figure 1D; Wang et al., 2019). The northern blot also showed that galTKM, galKM, and galM1 also got similarly shorter than their corresponding WT counterparts (lane 2, Figure 1D), and the amount of these mRNA species decreased to less than 10% of their amounts in the WT. The galM mRNA was hardly observed in the RITo2 mutant (lane 2, Figure 1D). These results demonstrate that the 3′-end-sharing mRNA species and the primary transcript galM1 harbor the terminator hairpin structure of the full-length galETKM.
To determine the 5′ end sequences of the 3′-end-sharing mRNA, we used 5′RACE. The assay showed that the 5′ ends of the galTKM mRNA were clustered around gal coordinates 1,031 and 1,039 (lane 1, Figure 1E), those of the galKM mRNA at 2,071 and 2,079 (lane 2, Figure 1E), and those of the galM mRNA at 3,209, 3,211, and 3,217 (lane 3, Figure 1E). The gal nucleotide coordinates, starting from the P1-transcription initiation site, are as in Figure 1A. These results indicate that the 5′ ends of the 3′-end-sharing mRNA are generated by endoribonucleolytic cleavages occurring near cistron junctions, mostly within the last few codons of the respective upstream ORF.
The 3′-End-Sharing mRNA Species Are the Intermediate Products of the RNase E-Mediated Decay of galETKM
RNase E is the major endoribonuclease in E. coli. To test whether this nuclease is responsible for cleavages near cistron junctions, generating the 3′-end-sharing mRNA species, we assayed for their presence in GW20 (ams1ts), a strain temperature-sensitive for RNase E activity (Wachi et al., 1997). We subjected the total RNA prepared from GW20 to northern blot analysis. Cells were grown to OD600 of 0.2 at 30°C in LB supplemented with 0.5% galactose, and the culture was divided into two halves. One half was shifted to 44°C, a non-permissive temperature, whereas the other half continued to grow at 30°C until they reached OD600 of 0.6. The northern blot results showed that, at non-permissive temperature, when GW20 cells were deprived of functional RNase E, galTKM, galKM, and galM mRNA species decreased drastically (Figure 2A). These results support the view that the 3′-end-sharing mRNA species, galTKM, galKM, and galM, are the products of RNase E cleavage.
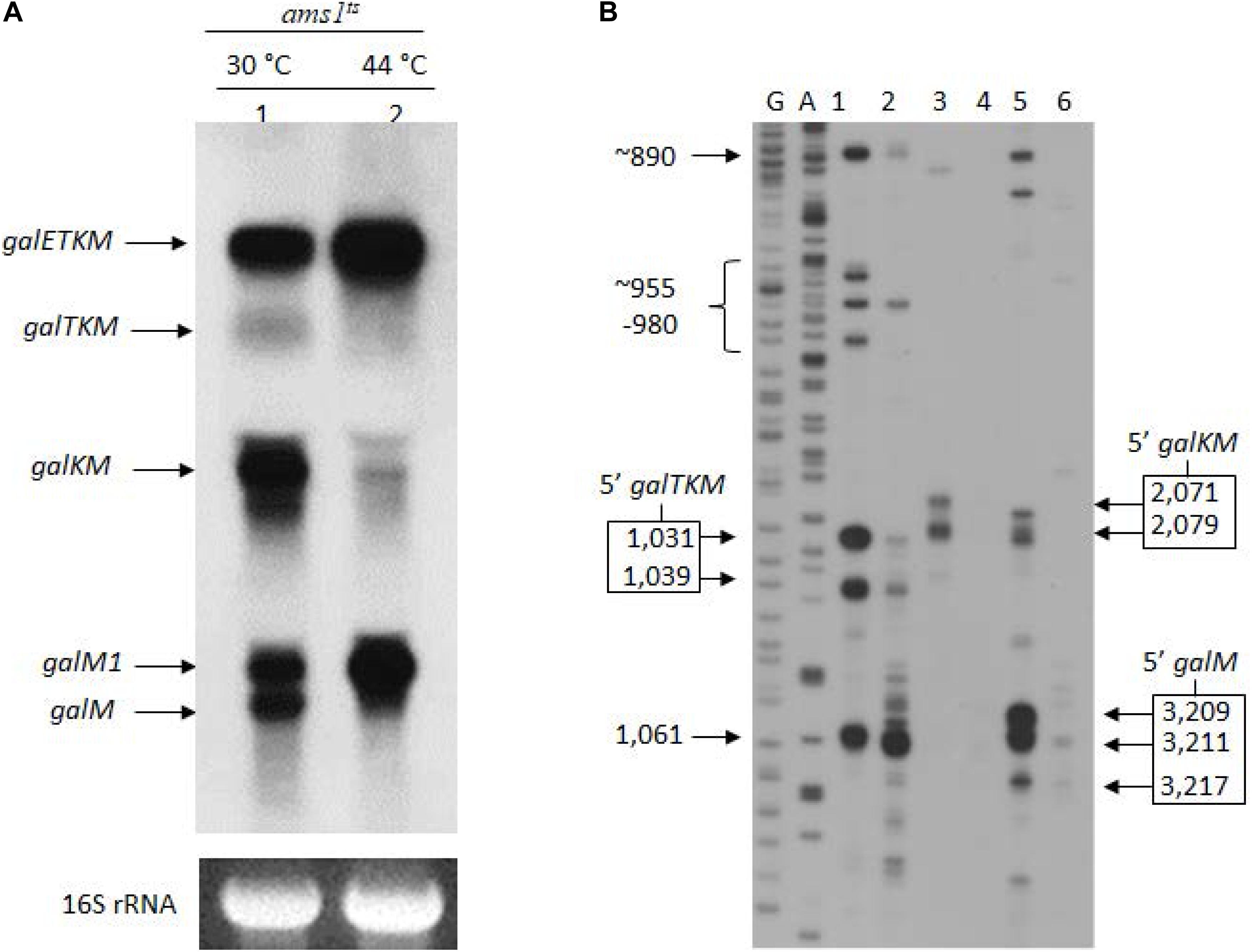
Figure 2. The 3′-end-sharing mRNA species are the intermediate products of the RNase E-mediated decay of galETKM. (A) Northern blot of the gal mRNA in GW20 (ams1ts) cells grown at either the permissive temperature of 30°C (lane 1) or the non-permissive temperature of 44°C (lane 2). The blot was probed with the M probe complementary to the 3′ half of the galM gene of the gal operon. galETKM and galM1 are the primary transcripts, and galTKM, galKM, and galM are the decay intermediates. (B) 5′RACE of gal mRNA prepared from GW20 (ams1ts) cells as in (A). The lanes show the 5′ ends of RNA at the permissive and the non-permissive temperatures found near the beginning of either the galT gene (lanes 1 and 2) or the galK gene (lanes 3 and 4) or the galM gene (lanes 5 and 6). DNA sequencing ladders used as molecular weight markers are in lanes marked G and A. An exception was the 5′ end at 1,061, which was unchanged at the two temperatures, indicating that this 5′ end was not generated by RNase E.
To confirm that the 5′ ends of the 3′-end-sharing mRNA (shown in Figure 1E) are generated by RNase E cleavage, we assayed the 5′ ends by 5′RACE on total RNA from GW20 cells grown at permissive and non-permissive temperatures. The results showed that, at the non-permissive temperature of 44°C, most 5′ ends of galTKM (lane 2, Figure 2B), galKM (lane 4, Figure 2B), and galM (lane 6, Figure 2B) were less than 10% of their amounts at the permissive temperature of 30°C (lanes 1, 3, and 5, Figure 2B). These results demonstrate that the 5′ ends of the 3′-end-sharing mRNA species are generated primarily by RNase E cleavage.
It is noteworthy that, in contrast to the decreasing amounts of 3′-end-sharing mRNA at the non-permissive temperature, the level of the primary transcripts, galETKM and galM1, increased by about twofold (lane 2, Figure 2A). The increased level of primary transcripts is to be expected if the 3′-end-sharing mRNA species are the RNase E-mediated decay products of galETKM.
The 5′ Portion mRNA Generated by RNase E Cleavage Is Degraded Rapidly
So far, we have analyzed only the 3′ portions of the galETKM mRNA after the RNase E cleavages at 1,031 and 1,039, 2,071 and 2,079, and 3,209, 3,211, and 3,217 that generated the 3′-end-sharing mRNA species galTKM, galKM, and galM, respectively. The 5′ portion of the galETKM mRNA after the RNase E cleavages should be in sizes of 1,031–1,039, 2,071–2,079, and 3,209–3,217 nucleotides long, respectively.
To detect these species, we performed northern blot of gal mRNA from wild-type and RITo2 mutant cells, this time using the E-probe (Figure 1A) that hybridizes to the first half of the galE gene (Figure 1A). The northern blot of the wild-type cells showed galETKM (4.3 kb), galETK (3.3 kb), galET (2.2 kb), galE1 (1.8 kb), and galE (1.2 kb) (Figure 3). These have been presented as “5′-end-sharing” mRNA in our previous reports (Figure 1A; Lee et al., 2008; Wang et al., 2014, 2015). In the RITo2 mutant, where the terminator hairpin is no longer functioning (Wang et al., 2019), galETKM is the only mRNA species affected; galETKM reduced in size by ∼100 nucleotides and decreased in amount to about 25% of the WT, but all the other mRNA species were present as much as they were in wild-type cells (Figure 3). These results demonstrate that the galETKM mRNA is not a precursor of all other gal mRNA species shown in Figure 3. These mRNA species are known to be generated as a result of premature transcription termination by Rho (Adhya, 2003; Wang et al., 2015). Indeed bicyclomycin (BCM), the Rho-inhibitor, inhibited the production of all the 5′-sharing mRNA species (Supplementary Figure S2).
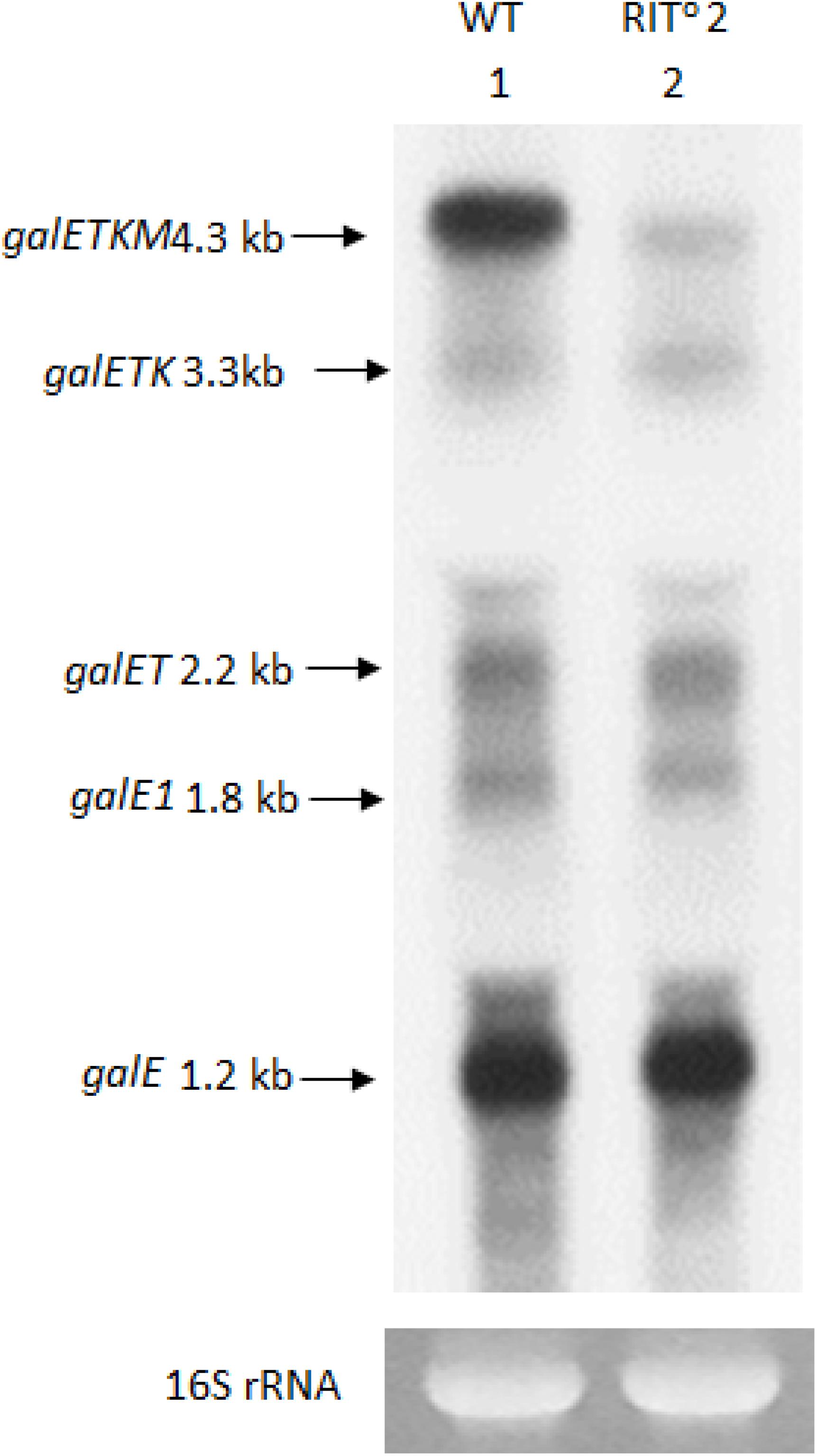
Figure 3. The 5′ portion mRNA generated by RNase E cleavage is degraded rapidly. Northern blot of gal mRNA probed with the E probe. gal mRNA was prepared from wild-type cells (lane 1), and the RIT02 gal mutant cells (lane 2) were grown up to OD600 of 0.6 in Luria broth with 0.5% galactose. The blot was probed with the E probe complementary to the 3′ half of the galE gene of the gal operon (Figure 1A).
These results thus suggest that the 5′ portion of the galETKM mRNA after the RNase E cleavages is removed immediately, possibly by 3′→5′ exoribonucleases, as has been demonstrated in many previous studies (Mott et al., 1985; Belasco et al., 1986; Hui et al., 2014; Wang et al., 2019).
Temporal Order of Cistron Elimination From the galETKM mRNA
To test whether the cistrons are eliminated one at a time from the 5′ end of the galETKM mRNA, we performed a “temperature shift-down” (TSD) experiment and followed the order of appearance of the decay intermediates with time. We used the GW20 (ams1ts) strain, expecting that RNase E would regain its function and initiate the decay process as the temperature of the GW20 culture is brought down from 44 to 30°C. If the cistrons are eliminated one at a time from the 5′ end of galETKM, we expect that the order of appearance of the intermediates would be galTKM, galKM, and finally galM.
We transferred a culture of GW20 grown to OD600 of 0.6 at 44 to 30°C and continued its growth. We took aliquots of the culture after 5, 10, and 20 min of TSD, prepared RNA, and subjected the RNA to northern blot analysis using the M-probe. The levels of decay intermediates 20 min after TSD (lane 5, Figure 4A), when the temperature of the culture reached 31°C, appeared as much as their levels when the GW20 culture was grown continuously to OD600 of 0.6 at 30°C (lane 1, Figure 4A). This indicates that, 20 min after, TSD suffices to restore the full function of RNase E. The same inference could also be made from the appearance of the 16S rRNA band presented as a loading control in Figure 4A. We observed that an RNA band with a molecular weight higher than that of the 16S rRNA appears at the non-permissive temperature (thick arrow, lane 2, Figure 4A). The intensity of this RNA band decreases and that of the 16S rRNA band increases during the TSD period. By 20 min, the ratio of the two bands became comparable to the ratio seen at the permissive temperature. Considering that the RNase E cleavage of the precursor 17S RNA generates 16S rRNA (Li et al., 1999), these results corroborate the abovementioned finding that the function of RNase E is restored by 20 min of TSD.
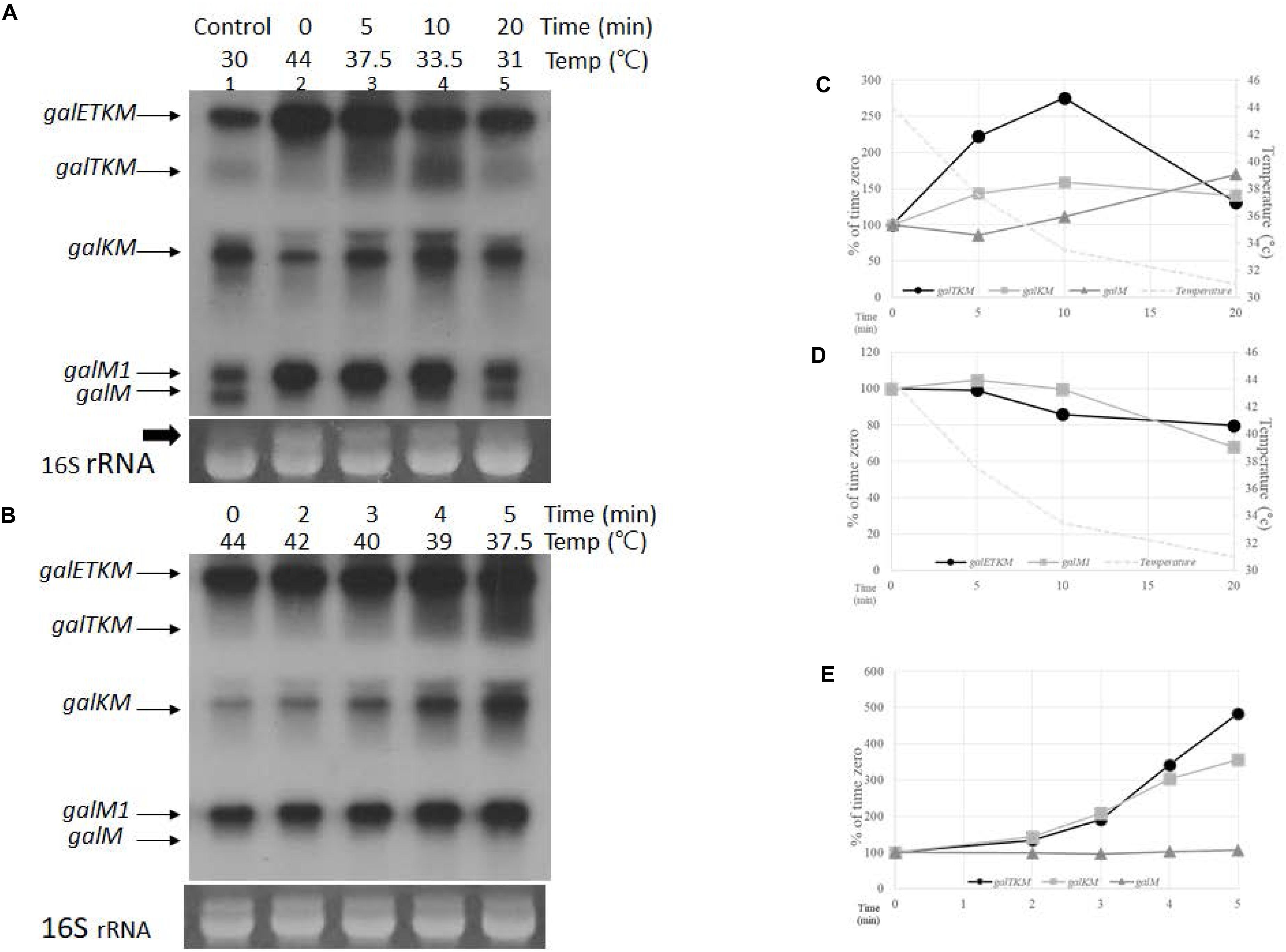
Figure 4. Temporal order of cistron elimination from the galETKM mRNA. (A) Northern blot (M-probed) of gal mRNA prepared from GW20 (ams1ts) cells taken at various times after temperature shift-down. The culture of GW20 cells grown up to A600 of 0.6 in Luria broth (LB) at 44°C was transferred to 30°C, and growth was allowed to continue. Aliquots of cells were taken at 0, 5, 10, and 20 min after transfer (lanes 2–5). The temperature of the culture at each time point is indicated. Lane 1 is gal mRNA from GW20 cells grown continuously to A600 of 0.6 in LB at 30°C. The arrow indicates the putative 17S rRNA, a precursor of 16S rRNA (Li et al., 1999), which were used here as loading controls. (B) Northern blot (M-probed) of gal mRNA from GW20 cells taken at 0, 2, 3, 4, and 5 min after temperature shift-down from 44 to 30°C. Temperature of the culture at each time point is indicated. (C) Changes in the amount of decay intermediate mRNA species after 5, 10, and 20 min of temperature shift-down. Amounts relative to that of time-zero were graphed; galTKM (dark circle), galKM (gray square), and galM (gray triangle). Temperature changes are indicated by a dotted line. (D) Changes in the amount of primary transcripts after 5, 10, and 20 min of temperature shift-down. Amounts relative to that of time-zero were graphed; galETKM (dark circle) and galM1 (gray square). Temperature changes are indicated by a dotted line. (E) Changes in the amount of decay intermediate mRNA species after 2, 3, 4, and 5 min of temperature shift-down. Amounts relative to that of time-zero were graphed; galTKM (dark circle), galKM (gray square), and galM (gray triangle).
The sequence of appearance of the decay intermediates during TSD period could be sequential from the 5′ end since, at the earliest time point (5 min) after TSD, the amount of galTKM increased the most, followed by the amounts of galKM and galM, the increase in the latter being hardly detectable (Figure 4A). This suggests that galTKM and galKM are generated before galM. At 10 min after TSD, the amounts of galTKM and galKM increased 2.7- and 1.6-fold, respectively, relative to their amounts at time-zero (Figure 4C). A slight increase in galM amount (about 1.1-fold) was also detected. At 20 min after TSD, the galM amount increased 1.8-fold, while the amounts of the other two intermediates, galTKM and galKM, decreased (Figure 4C). These results further show that galM is formed subsequent to the formation of galTKM and galKM, and their decrease suggests that these longer intermediates could be serving as precursors of galM.
During the TSD period, the amounts of both primary transcripts galETKM and galM1 decreased (Figure 4D). Considering that the function of RNase E restores gradually during the TSD period, the gradual decrease in the amounts of galETKM mRNA is consistent with the notion that RNase E cleavage of the galETKM gives rise to the decay intermediates. The decrease in galM1 indicates that it is also a substrate of RNase E.
To test if there is a difference in the timing of appearance between galTKM and galKM, we analyzed the decay intermediates every minute during the first 5-min period after TSD. Temperature gradually fell from 44 to 37°C during the first 5 min (Figure 4B). galTKM and galKM appeared essentially simultaneously, while the galM mRNA amount hardly changed (Figures 4B,E). The simultaneous appearance of galTKM and galKM indicates that the latter is not produced from the former and that both are independently produced by the RNase E cleavage of galETKM. Thus, the appearance of the decay intermediates may not be strictly sequential.
RNase E Also Cleaves Upstream of the Terminator Hairpin of the gal Operon
All the decay intermediates and the primary transcripts galETKM and galM1 have the same terminator hairpin stem-loop structure at the 3′ end (Figure 1B). In the RITo2 mutant, where the function of the stem-loop is impaired, production of these mRNA species was greatly impaired (Figure 1D). The 3′ terminal stem-loop is known to enhance the half-life of mRNA by serving as a road-block to the 3′→5′ exoribonuclease digestion (McLaren et al., 1991; Regnier and Hajnsdorf, 1991; Carpousis et al., 2009). We argued that, to complete the decay of the galETKM mRNA, RNase E should cleave upstream of the terminator hairpin of galM mRNA to allow 3′→ 5′ exoribonuclease access for digestion of galM mRNA.
To test if there are any RNase E-generated 5′ ends upstream of the terminator hairpin, we performed 5′RACE around the terminator hairpin in GW20 (ams1ts) cells. We found two clusters of 5′ ends at permissive temperature. In one cluster, the ends were at coordinates 4,273, 4,269, and 4,266, and in the other, the ends were at 4,241, 4,237, and 4227 (lane 1, Figure 5A). These 5′ end products became nearly undetectable at non-permissive temperature, except for the product ending at 4,227 (lane 2, Figure 5A). We infer that, barring the end at 4,227, the other five ends were generated by RNase E. The RNase E cleavage sites were at 20–40 nucleotides upstream of the hairpin, which should allow exoribonuclease processing unencumbered by the hairpin (Figure 5B). The origin of the 5′ end at 4,227 remains to be studied.
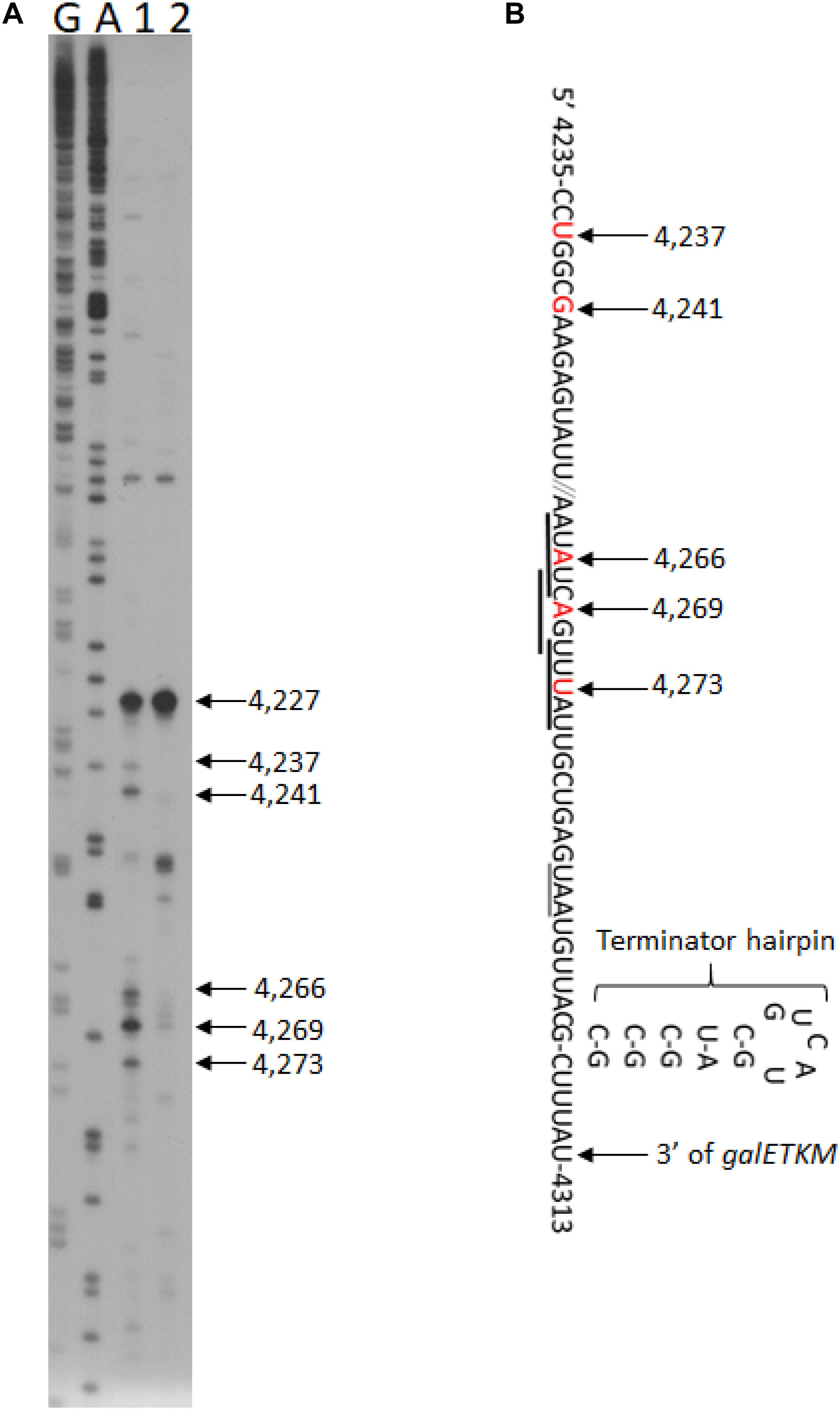
Figure 5. RNase E cleavage upstream of the terminator hairpin of the gal operon. (A) 5′RACE of RNA in front of the terminator hairpin of gal operon isolated from GW20 (ams1ts) cells grown either at the permissive temperature of 30°C (lane 1) or the non-permissive temperature of 44°C (lane 2). The 5′ end at 4,227 is not generated by RNase E cleavage since its intensity did not decrease at the non-permissive temperature. Lanes G and A are DNA sequencing ladders, as in Figure 1E. We note that the 5′ ends located between 4,273 and 4,266 also fall within the consensus sequence for the RNase E cleavage (Belasco, 2017; Chao et al., 2017), but the ends located at 4,241–4,237 seem to deviate from it. Nevertheless, both cleavage clusters would serve the purpose of removing the hairpin, allowing 3′ to 5′ exoribonuclease digestion. (B) Nucleotide sequence of mRNA at the end of galM presented together with their gal coordinates. The nucleotide residue and its gal coordinate that becomes the 5′ end after RNase E cleavage are indicated in red. The RNase E cleavage is indicated with a downward arrow. Matches to the consensus sequence for RNase E cleavage are underlined with thick horizontal lines. The translation stop codon of the galM gene is also underlined (thin line). Also shown is the terminator hairpin. The cytosine residue at 4,313 is the last residue of the full-length mRNA, galETKM (Wang et al., 2019).
Effect of Translation Initiation on the Production of mRNA Intermediates
RNase E cleavage is known to have moderate sequence specificity with a degenerate consensus sequence 5′-R(A/G)NW(A/U)UU-3′ (R = A or G, N = any, W = A or U) (Belasco, 2017; Chao et al., 2017). In this sequence, RNase E cleaves between the N and the W residues, leaving the W residue at the 5′ end (Belasco, 2017; Chao et al., 2017). We found that all the 5′ ends of decay intermediates indeed had the W residue (downward arrows) of the consensus RNase E cleavage sequence (underlined) (Figures 6A–C). The 5′ ends of galTKM mRNA at 1,031 and 1,039 are 13 and 5 nucleotides upstream from the putative Shine–Dalgarno (SD) sequence of the galT gene (Figure 6A). Similarly, in the galKM mRNA, the 5′ end is 12 or 20 nucleotides upstream from the putative SD sequence of the galK gene (Figure 6B), and in the galM mRNA, the 5′ end is 16, 22, or 24 nucleotides upstream from the putative SD sequence of the galM gene (Figure 6C).
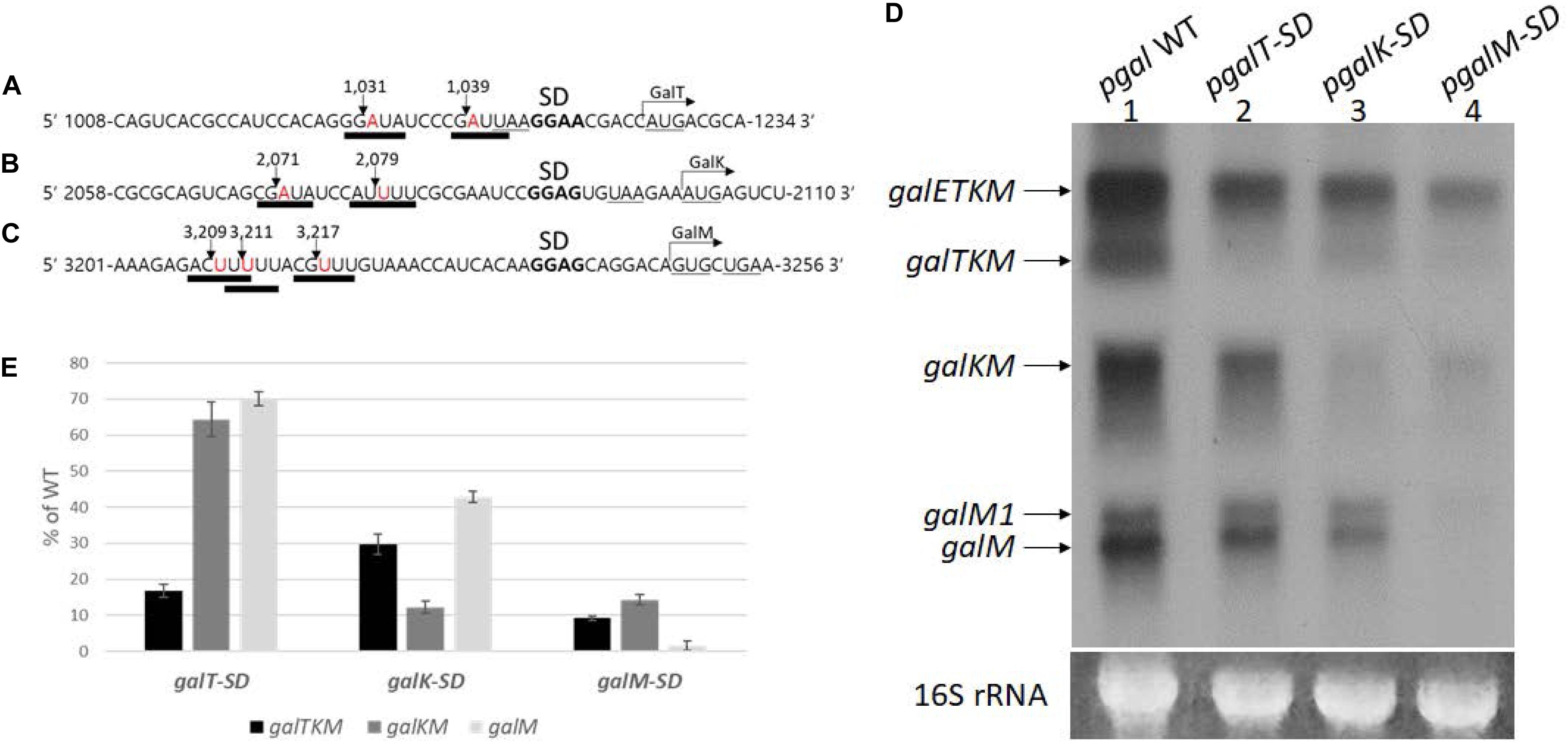
Figure 6. Effect of translation initiation on the production of mRNA intermediates. (A–C) Sequence features at cistron boundaries in gal mRNA, showing the correspondence of the cleavage positions (inverted arrows) of the samples in Figure 2A to the RNase E consensus cleavage sites (thick underlines). Also highlighted are the putative Shine–Dalgarno sequence (in bold) and initiation (AUG or GUG) and termination (UAA or UGA) codons (underlined). (D) Northern blot of gal mRNA prepared from MG1655Δgal cells harboring pgal (lane 1) or its mutant derivative plasmids, pgalT-SD (lane 2), pgalK-SD (lane 3), and pgalM-SD (lane 4). The blot was probed with the M probe. (E) Quantification of each decay intermediate mRNA from (D) using the software ImageJ (NCBI, NIH). Note that when mRNA was extracted from cells with pgalT-SD (lane 2 in D), of the three intermediates, galTKM, galKM, and galM, galTKM mRNA decreased the most, compared to their levels in cells with the wild-type plasmid pgal. Similarly, when the plasmid was pgalK-SD (lane 3 in D), the galKM mRNA decreased the most, and the same trend was seen when the plasmid was pgalM-SD (lane 4 in D).
These results suggest that translation initiation at the beginning of each cistron in galETKM could be responsible for the location of the observed RNase E cleavage sites. To test the effect of translation initiation on the observed RNase E cleavage sites, we mutated the putative SD sequences of galT, galK, and galM (bold letters) to their complementary sequences (Figures 6A–C), one SD sequence at a time, and assayed the production of decay intermediates as well as the primary transcripts. These SD mutations were generated in plasmid, pgal. The resulting mutant plasmids were called pgalT-SD, pgalK-SD, and pgalM-SD, respectively. We performed northern blot of gal mRNA from the three SD mutant plasmid-carrying cells using the M-probe.
Results showed that the primary transcript galETKM production decreased gradually, farther down where the SD mutations were in the operon (Figure 6D). The galETKM was 60, 55, and 17% of the WT in galT-SD, galK-SD, and galM-SD mutants, respectively. It has been well documented that disruption of the transcription–translation coupling leads to premature Rho-dependent transcription termination (Adhya and Gottesman, 1978). This notion has been rigorously supported in a recent study (Zhu et al., 2019). We reasoned that the premature Rho-dependent transcription termination caused by the SD mutations was responsible for the decreased production of galETKM. To test, we performed northern blot of RNA from the SD mutant plasmid-carrying cells that have been treated with the Rho-inhibitor BCM (20 μg/ml for 10 min). The results showed that, upon BCM treatment, galETKM increased 20% in cells with pgalT-SD, 50% in cells with pgalK-SD, and 250% in cells with pgalM-SD (Supplementary Figure S3). Thus, in the presence of BCM, SD mutants produced more galETKM, suggesting that Rho-dependent transcription termination could be primarily responsible for the decreased production of galETKM.
The northern blot of RNA from the SD mutant plasmid-carrying cells (Figure 6D) additionally showed that:
In pgalT-SD-harboring cells (lane 2, Figure 6D), galTKM mRNA decreased to less than 20% of the WT level, while the other two intermediate mRNAs, galKM and galM, decreased to about 60–70% of the WT level (Figure 6E).
In pgalK-SD-harboring cells (lane 3, Figure 6D), galKM mRNA decreased to 12% of the WT level, while the other two intermediate mRNAs, galTKM and galM, decreased to about 30–40% of the WT level (Figure 6E).
In pgalM-SD-harboring cells (lane 4, Figure 6D), galM mRNA decreased less than 5% of the WT level, while the other two intermediate mRNAs, galTKM and galKM, decreased to about 10% of the WT level (Figure 6E).
These results show that, when an intermediate mRNA harbors SD mutation at its 5′ end, its amount is reduced the most than the other two intermediate mRNAs in every case (Figure 6E). The level of decrease of the other two intermediates appeared roughly similar to that of galETKM in all of the SD mutants, suggesting that the SD mutations, by decreasing the galETKM amount indirectly, caused an overall decrease of the intermediates. We have not correlated mRNA level with protein level, which remains an important task for the future.
Translation Initiation at the 5′ End of Decay Intermediates Promotes Their Stability
To test the effect of blocking translation initiation further, we measured the half-lives of intermediate mRNA species from cells with the WT or the SD-mutated pgal plasmids. MG1655Δgal cells harboring WT or the mutant pgal plasmids were grown in LB to OD600 of 0.6, at which time rifampicin was added at 100 μg/ml to block new RNA synthesis. Cells were harvested every minute or two, and total RNA was subjected to northern blot analysis using the M-probe. From these blots, we measured the half-lives of the intermediates as well as the full-length galETKM mRNA (Figure 7 and Table 1).
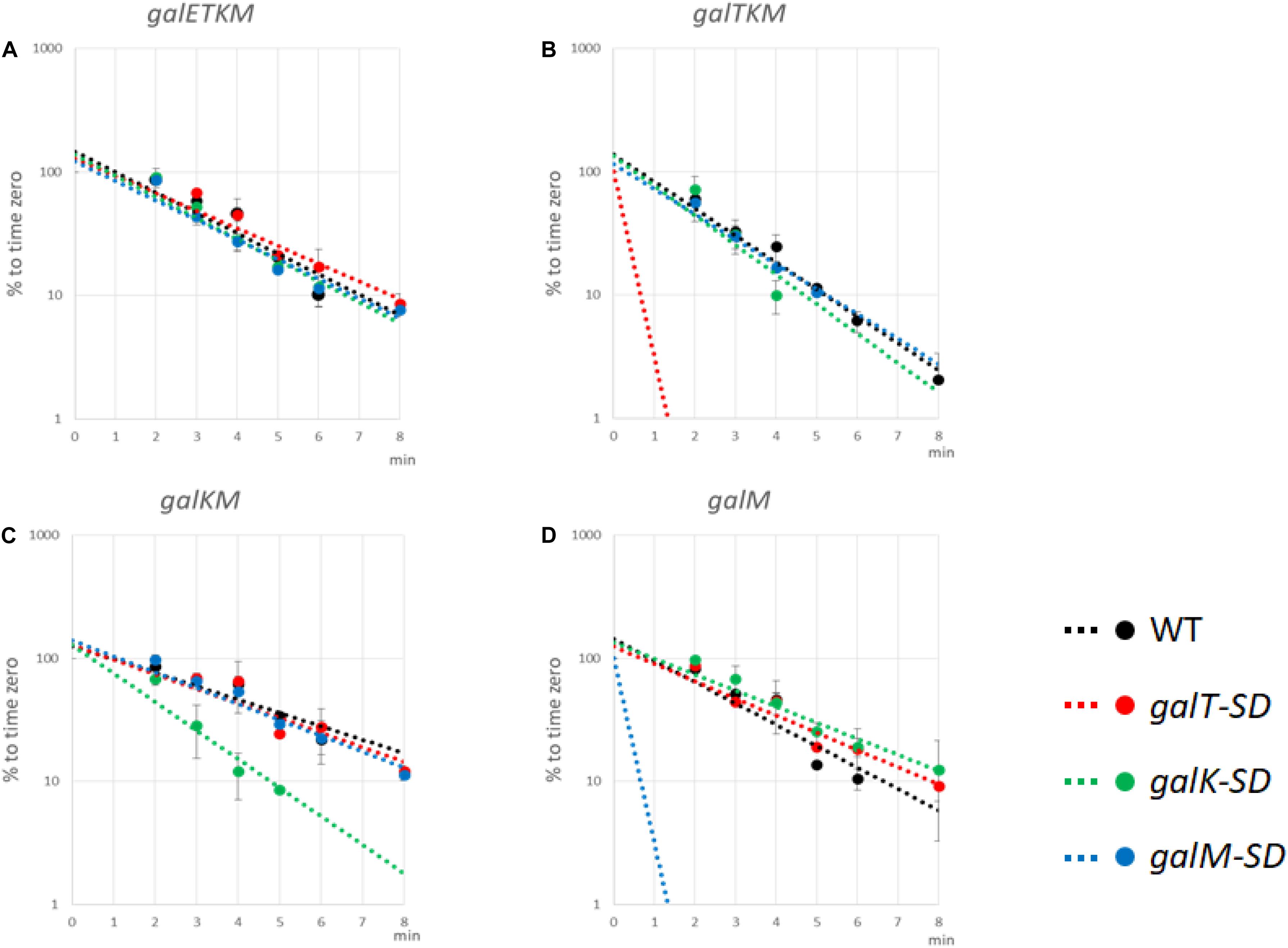
Figure 7. Translation initiation at 5′ end of decay intermediates promotes their stability. The Shine–Dalgarno (SD) mutants as well as wild-type (WT) cells were grown in Luria broth with 0.5% galactose up to OD600 of 0.6. Rifampicin (100 μg/ml, final concentration) was added, and thereafter aliquots of the culture were taken at times indicated in the abscissa of the graphs. RNA prepared from the culture samples were subjected to northern blot and probed with M-probe. RNA bands were quantified and plotted against the time of their sampling. Northern blot analyses were repeated at least two times. The decay rate of galETKM (A), galTKM (B), galKM (C), and galM (D) in WT (black), galT-SD (red), galK-SD (green), and galM-SD (blue) is presented. Note that the decay rate of the primary transcript, galETKM, did not change in any of the SD mutants, but the decay rate of an intermediate mRNA decreased significantly only when it harbored an SD mutation at its 5′ end. The dotted lines for galTKM in the galT-SD mutant [red in (B)] and for galM in the galM-SD mutant [blue in (D)] without any experimental points indicate that those RNAs were undetectable even at the earliest sampling time (2 min).
The results are as follows:
galETKM: Its half-life in all the SD mutant plasmid-carrying cells was nearly the same as in WT pgal-carrying cells, which was 1.82 ± 0.11 min (Figure 7A and Table 1).
galTKM: Its half-life in pgalK-SD- and pgalM-SD-carrying cells was nearly the same as in WT pgal-carrying cells, which was 1.50 ± 0.08 min (Figure 7B and Table 1). However, when the SD mutation was in galT, the half-life of galTKM decreased to an unmeasurably low value.
galKM: Its half-life in pgalT-SD- and pgalM-SD-carrying cells was nearly the same as in the WT pgal-carrying cells, which was 2.82 ± 0.14 min (Figure 7C and Table 1). However, when the SD mutation was in galK, the half-life of galKM decreased to 1.31 ± 0.07, significantly below its value in WT pgal-carrying cells of 2.82 ± 0.14 (Table 1).
galM: Its half-life in pgalT-SD- and pgalK-SD-carrying cells was nearly the same as in the WT pgal-carrying cells, which was 1.86 ± 0.37 min (Figure 7D and Table 1). However, when the SD mutation was in galM, the half-life of galM decreased to an unmeasurably low value.
Since the galETKM mRNA half-life did not change significantly when the RNA had a defective translation initiation signal in any one of its internal cistrons, galT, galK, or galM (Figure 7A), this suggests that internal translation initiation does not affect RNase E cleavage significantly and thus the decay process of galETKM.
Studies with the mutant plasmids revealed that, unlike the SD mutations internal to the operon, when the mutations are present at the 5′ end of an intermediate mRNA, its half-life is specifically reduced (Figures 7B,D). These results suggest that translation initiation at the 5′ end of an intermediate mRNA could be one of the factors that confer stability to the intermediate mRNA. It is also clear that the instability due to SD mutations does not propagate to downstream cistrons, consistent with the view that translation initiation in those cistrons confers stability to them.
RNase E Cleavage That Results in galTKM Generation Requires 5′ Mono-Phosphate
Di-phosphorylated mRNA 5′ ends are abundant in E. coli. RppH removes the β-phosphate from di-phosphorylated mRNA 5′ ends and generates mono-phosphorylated mRNA 5′ ends (Luciano et al., 2017). Thus, in the absence of RppH, the percentage of full-length primary transcripts that are monophosphorylated drops to almost zero as measured by 5′RACE (Deana et al., 2008). To see the generation of the decay intermediates in a situation where most galETKM 5′ ends are tri- or di-phosphorylated, we performed northern blot using the M-probe of MG1655ΔrppH cells (where the gene for RppH is deleted from the chromosome) grown to OD600 of 0.6. The result showed that, while galETKM decreased to only 90% of its level in WT, galTKM and galKM dropped to undetectable and 40% of their level in WT, respectively. The results also showed that galM decreased to 80% of WT, but galM1 hardly at all (Figures 8A,C). Thus, in a situation where most galETKM and galM1 5′ ends are tri- or di-phosphorylated, mainly the generation of galTKM was affected.
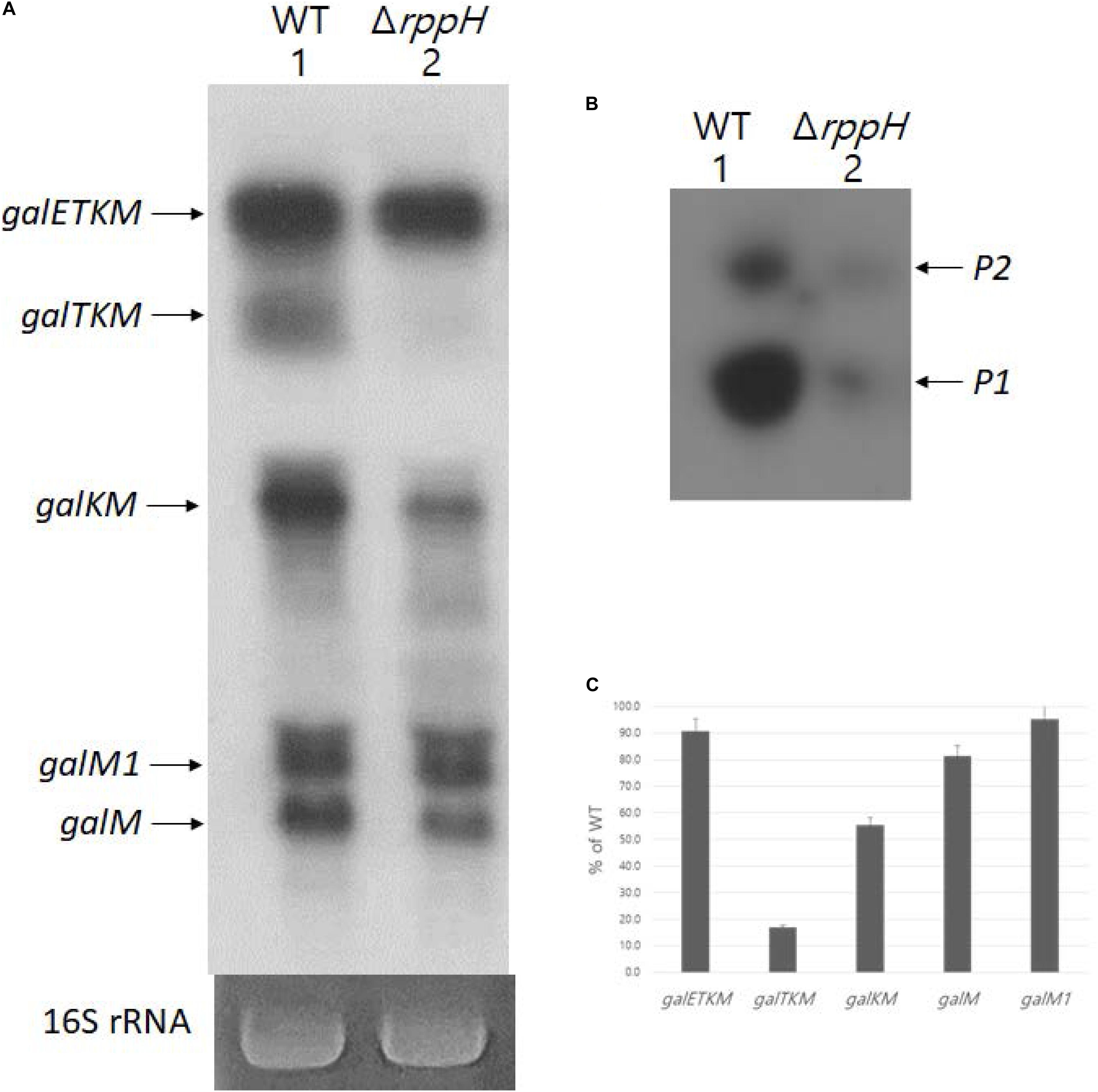
Figure 8. RNase E cleavage that results in galTKMgeneration requires 5’ mono-phosphate. (A) Northern blot (M-probed). (B) 5’RACE of galmRNA prepared from MG1655ΔrppHcells grown at OD600 of 0.6. (C) Quantification of each decay intermediate mRNA from (A) using the software ImageJ (NCBI, NIH).
Using 5′RACE, we assayed the 5′ end of the gal transcript initiated from the two promoters of gal, P1 and P2 (Adhya and Miller, 1979), in MG1655ΔrppH. The 5′RACE results showed that the 5′ ends of gal transcripts that are monophosphorylated drastically reduces in MG1655ΔrppH compared to their levels in the WT (Figure 8B). Since the 5′RACE assay requires mono-phosphorylated mRNA 5′ end, the 5′RACE assay results indicate that most galETKM in MG1655ΔrppH are tri- or di-phosphorylated at the 5′ end. Taken together, these results suggest that the reason for the lack of generation of galTKM and, to a significant extent, galKM is because of the lack of mono-phosphorylation of the 5′ end of galETKM. In other words, the 5′ mono-phosphorylated galETKM appears to be the precursor of galTKM and galKM, as was suggested by the results of the TSD experiments (Figure 4). Since galKM is still generated in ΔrppH cells, albeit in reduced amounts, this indicates that there is a second pathway of generating them which does not depend on a mono-phosphorylated 5′ end.
Discussion
The gal Operon Produces Two Classes of mRNA: 5′-End-Sharing and 3′-End-Sharing
Northern blot analyses revealed that the gal operon produces two kinds of mRNA species that are 5′-end-sharing or 3′-end-sharing, as depicted in Figure 1A. Northern blot of WT E. coli cells probed with the E-probe, which hybridizes to the first half of the first gene galE, shows the 5′-end-sharing mRNA (lane 1 in Figure 3). However, if the same blot is probed with the M-probe, which hybridizes to the second half of the last gene galM, it shows the 3′-end-sharing mRNA (lane 1 in Figure 1D). We investigated how the 5′-end-sharing mRNA species are generated in all of our previous studies (Lee et al., 2008; Wang et al., 2014, 2015, 2019). In this study, we focused on how the 3′-end-sharing mRNA species are generated.
RNase E Cleavage at Cistron Boundaries
RNase E cleavage at cistron boundaries has been reported in many different operons. Representative examples are in between dnaG and rpoD (Burton et al., 1983) in the polycistronic rxc mRNA (Belasco et al., 1985), in between papB and papA in the pap operon (Baga et al., 1988), and between cistrons of the his operon (Alifano et al., 1994b). Recently, using transcriptome analysis, Dar and Sorek demonstrated that RNase E cleavage at the cistron boundaries occur in many different operons in E. coli (Dar and Sorek, 2018).
Using the sequence information downstream of the 5′ end of 22,000 RNA generated by RNase E cleavage in Salmonella (Chao et al., 2017), we searched for Salmonella operons harboring the RNase E-generated 5′ end sequences 40 nucleotides upstream from the initiator codon of internal cistrons. The results showed that 182 operons (out of 881 operons investigated) have RNase E-cleaved 5′ end at cistron boundaries, a few codons upstream from the putative SD sequence of the downstream genes (Supplementary Figure S4). Many operons have RNase E-cleaved 5′ end at multiple cistron boundaries in the same operon (Supplementary Figure S4). Thus, the RNase E cleavages at the cistron boundaries shown in this study appear to be a common practice of mRNA decay of polycistronic mRNA in E. coli as well as in Salmonella. Of the two activities of RNase E, processing and degradation, generation of stable decay intermediates can also be thought of as a processing event rather than a destructive event.
Polycistronic galETKM mRNA Decays Preferentially Through Cistron Elimination From the 5′ End
Since the initial proposal by Apirion (1973) that mRNA decay in E. coli proceeds in 5′→3′ direction, in spite of the lack of any 5′ to 3′ exoribonucleases in E. coli (Deutscher, 1985), it is generally believed that mRNA decay in E. coli initiates from the 5′ end and proceeds toward the 3′ end (Mackie, 2013; Hui et al., 2014; Mohanty and Kushner, 2016). This is because RNase E has a preference to initiate decay by binding first to the 5′ mono-phosphorylated end. From our limited kinetic analysis of precursor-to-product formation, most of the data could be interpreted as sequential elimination of cistrons from the 5′ end of galETKM. A clear exception was also found: About 40% of galKM that was dependent on RNase E, but not on 5′ monophosphate formation, was produced (Figures 2A, 4E, 8A). It appears that RNase E can also directly access internal sites within a polycistronic message. As we elaborate below, although overall there is a preference for elimination of cistron from the 5′ end, the process may not be always sequential.
Use of Two Modes by RNase E in the Elimination of Cistrons From galETKM mRNA
Based on the data presented in this study, we propose the following model for the decay of galETKM mRNA (Figure 9).
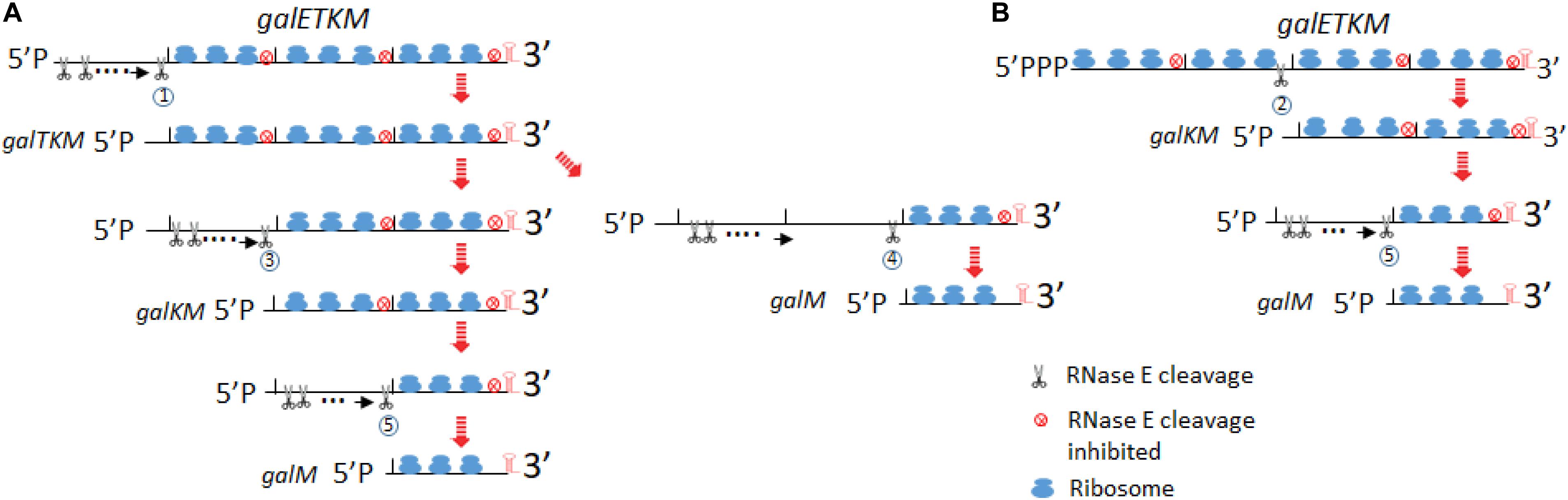
Figure 9. Model for decay of the polycistronic mRNA, galETKM. The model depicts two different ways to eliminate a cistron(s) from the 5′ end of galETKM. In (A), the galE gene is progressively eliminated from the galETKM mRNA by the 5′ end-dependent pathway. We propose that RNase E binds to the mono-phosphorylated 5′ end of the galETKM mRNA. The RNase E binding causes a cascade of events: (1) inhibition of translation initiation of galETKM, (2) receding of already initiated ribosomes from the 5′ end, which would reveal RNase E cleavage sites in the ribosome-free regions of mRNA, and (3) cleavage. Because the newly generated 5′ ends will be mono-phosphorylated, RNase E can again bind and cleave, and the cycle can continue until near the end of the cistron at 1,031 or 1,039, generating galTKM by RNase E cleavage labeled 1. By the same process, galKM and galM (RNase E cleavage labeled 3 and 4, respectively) could be generated. galM could also be generated from galKM by RNase E cleavage labeled 5. RNase E cleavage 6 would complete the decay of galETKM. In (B), we depict the simultaneous elimination of galE and galT from the galETKM mRNA by the direct access pathway. We also propose that the tri-phosphorylated galETKM mRNA can stochastically become ribosome-free in the internal cistrons. For example, when the galE–galT part becomes ribosome-free, RNase E cleavage labeled 2 at 2,071 or 2,079 directly generates galKM. The results in this study suggested that galKM could be generated from galTKM by RNase E cleavage 3. galM could be generated by RNase E cleavage 4 and 5 from galTKM and galKM, respectively.
Progressive Elimination of galE From galETKM That Generates galTKM
RNase E is a large tetramer and forms a multi-protein complex called the degradosome (Py et al., 1996; Callaghan et al., 2005). Its binding to the 5′ end of mRNA causes steric hindrance to nearby translation initiation (Deana and Belasco, 2005). Inhibition of new translation initiation will make the already initiated ribosomes to retreat away from the 5′ end and expose RNase E cleavage sites which were otherwise to be occluded by translating ribosomes (Figure 9A). When RNase E cleaves the first time, it would generate a new 5′mono-phosphate end, to which RNase E can bind. This cleavage and binding can repeat until the cleavage site approaches the galT translation initiation site. The RNase E cleavage labeled as circle 1 would generate galTKM (Figure 9A).
In our temperature shift-down experiments, galTKM appears to be produced by the gradual shortening of the full-length galETKM mRNA during the 5- to 20-min time period (Figure 4A). The trailing of the galTKM band (the material between the bands labeled galETKM and galTKM) is most conspicuous in lane 3 of Figure 4A. The results of 5′RACE indicate that there are two major 5′ ends of galTKM located at 1,031 and 1,039, but there are also longer-length species located at ∼890 and 955–980 (Figure 1E), which can be interpreted to represent progressive RNase E cleavage on the galE part of galETKM during the galTKM formation process. We did find a consensus of RNase E cleavage sequences near 890, but none at 955–980. This mechanism is analogous to RNase E scanning activity on RNA from the 5′ end before it cleaves the message (Richards and Belasco, 2019). The generation of galTKM was also drastically reduced in ΔrppH mutant (Figure 8) in support of the 5′ end-dependent pathway. Thus, the 5′ end-dependent pathway appears to be used exclusively to generate galTKM from galETKM (Figure 9A).
One-Step Elimination of galET From 5′-(P)PP–galETKM Generates galKM
In comparison to galTKM, the galKM band was less smeary in northern blots (Figure 4A). Only two closely spaced ends of galKM at 2,071 or 2,079 were also revealed by 5′RACE (Figure 1E). The appearance of galTKM and galKM at the same time and the RppH-independent accumulation of galKM through direct-entry processing of a triphosphorylated galETKM precursor suggest that RNase E generates galKM directly from full-length galETKM and not from intermediate length precursors, as observed for galTKM. Since galTKM and galKM appear at the same time, they are most likely produced independently from different galETKM RNAs. Alternatively, it is also possible that, once it has formed, galTKM is rapidly cleaved to produce galKM in a step that is not rate limiting.
Since a significant amount of galKM is generated in ΔrppH cells (Figure 8), it indicates that the direct access pathway was responsible for the generation. Since the level of production was different in the WT and ΔrppH cells, this indicates that both the direct access (by RNase E cleavage labeled as circle 2 in Figure 9B) and the 5′ end-dependent (by the circle 3 RNase E cleavage in Figure 9A) pathways are employed to generate galKM. Note that, in E. coli, ∼35–50% of each mRNA is di-phosphorylated, and the rest is mono-phosphorylated at the 5′ end (Luciano et al., 2017), and the direct access pathway of mRNA decay has been demonstrated as a major pathway for mRNA decay in E. coli (Clarke et al., 2014).
Elimination of a Cistron(s) to Generate galM
From the temperature shift-down experiment, we propose the following pathways for galM mRNA generation. By the sequence of appearance, galM could be generated either by the simultaneous removal of the galTK part from galTKM (the RNase E cleavage labeled as circle 4 in Figure 9A) or by removing the galK part from galKM (the cleavage labeled as circle 5 in Figure 9A). The decrease of galTKM and the increase of galM from 10 to 20 min time points in one hand and relatively no change in galKM amount on the other may suggest that galM is derived directly from galTKM by the cleavage 4, but the alternate pathway, indicated by the cleavage 5, such that galM is primarily derived from galKM, which is derived from galTKM, cannot be eliminated (Figure 9A). galKM amounts are not expected to change if the rate of decay of galTKM to galKM and galKM to galM is similar. The generation of galM in ΔrppH cells does not necessarily indicate that it is produced by direct access if the precursors are galTKM and/or galKM, which have a mono-phosphorylated 5′ end. This indicates that galM is derived from the 5′ end-dependent pathway. The smearing RNA below the galKM band in the first 5 min (Figure 4B) or at 10 min (Figure 4A) of TSD suggests that the galK part of the galKM mRNA could be progressively removed from the 5′ end (as indicated in the cleavage 5). However, the smearing does not extend all the way down to the galM band (Figure 4B), suggesting that the progressive elimination of galK proceeds to a certain point of galK before RNase E cleaves at 3,209–3,217 (Figure 2B) to generate galM.
Elimination of galM
Elimination of galM is the last step in the sequence of cistron elimination, completing the decay process of galETKM mRNA. The galM band also smears to lower-size species to some extent, indicating that it decays progressively (Figure 4B). However, RNase E could also directly access the cleavage sites at 4,266–4,273.
Failure in Translation Initiation at the 5′ End of Polycistronic mRNA Initiates mRNA Decay
Our results here demonstrate that interfering with translation initiation at the 5′ end of each intermediate mRNA greatly increases the message decay rate (Figure 7 and Table 1). Because translating ribosomes inhibit RNase E cleavage (Deana and Belasco, 2005) and RNase E cleaves mRNA regions free of ribosome (Iost and Dreyfus, 1995), the “absence of ribosome” seems obligatory for the cleavage. Inhibiting translation initiation seems the most reasonable way to generate ribosome-free regions within a message. Creating ribosome-free regions within an ORF undergoing translation other than by inhibiting translation initiation would lead to the production of truncated proteins, which would be wasteful (Keiler et al., 1996; Muto et al., 1996; Hayes et al., 2002). Thus, RNase E cleavage on galTKM or galKM to generate galM is most likely preceded by inhibition of translation initiation at the 5′ end.
The same decay strategy could be applied to the primary (full-length) polycistronic mRNA. Considering that both of the 5′ end-dependent and direct access pathways work on the primary mRNA, galETKM, it is likely that the phosphorylation status of 5′ end influences which pathway RNase E would take to initiate the cleavage. Aside from occasional stochastic failure to initiate translation, there could be formation of a stem-loop structure as in the 5′ UTR (Arnold et al., 1998), other secondary structures, or sRNA binding that could inhibit translation initiation at the 5′ end of many mRNAs. For example, sRNA Spot 42 binding to the 5′ end of one of the intermediate gal mRNA, galKM (mK2), expedites the decay of galKM (Wang et al., 2015). Even proteins such as RNase E itself could inhibit translation initiation (Deana and Belasco, 2005). We propose that it is these translation initiation-inhibiting factors at the 5′ end of polycistronic mRNA that induce cleavage by making the RNase E cleavage sites ribosome-free. Our experiments also revealed that the cistron elimination did not proceed to the downstream cistrons possibly because of their translation initiation signals.
In all the cartoons of Figure 9, a cistron was eliminated when it was the 5′-most cistron and ribosome-free. This was evident in experiments of Figure 6 where translation initiation signals were mutated. For example, in the case of the galT-SD mutant, whatever pathway generates galTKM from galETKM, the intermediate is eliminated selectively if it did not have a translation initiation signal of galT. However, galTKM was not preferentially eliminated, although it was the 5′-most cistron when it had the galK-SD mutation (Figure 6). Direct entry of RNase E into the ribosome-free galK region should have eliminated the galTKM intermediate together with galKM, but apparently that did not happen. In other words, the absence of translation, although necessary, is not sufficient for decay.
In sum, even in a four-cistron mRNA, there can be progressive and one-step elimination of cistrons and no elimination of internal cistrons even in the absence of translation. Although the picture is complex, vulnerability of the 5′-most cistrons appears to be the most consistent theme of the present study, in line with the general thinking that decay proceeds in the 5′→3′ direction.
Future Studies on gal mRNA Decay
Intermediates of gal mRNA other than those described here are also produced by agents such as Spot 42 small RNA and transcription terminator factor. For example, in MG1655Δspf cells, where the gene for Spot 42 is deleted from the chromosome, the production of galKM mRNA is reduced compared to that seen in the WT, and this is because Spot 42 expedites the decay of the galKM mRNA (Wang et al., 2015). Based on these results, we propose that Spot 42 binding might expedite the decay of those gal mRNA species, such as galETKM, galTKM, and galKM, that harbor the Spot 42 binding site at the galT–galK cistron junction. We are currently testing this prediction. We are also currently investigating whether in the generation of the 5′ end of galKM there is a relationship between the RNase P cleavage at 1,764 and 1,777 (Wang et al., 2014) and the RNase E cleavage at 2,071 and 2,079 (Figure 1E).
Data Availability Statement
All datasets presented in this study are included in the article/Supplementary Material.
Author Contributions
HL designed the research. HJ, CK, MN, and YL performed the research. HJ, CK, MN, YL, XW, DC, and HL analyzed the data. DC and HL wrote the manuscript. All authors contributed to the article and approved the submitted version.
Funding
This study was supported by a grant (2017-1400-01) of the Basic Science Research Program of the National Research Foundation of Korea (NRF). This work was partially supported by the Intramural Research Program of the Center for Cancer Research, NCI, NIH.
Conflict of Interest
The authors declare that the research was conducted in the absence of any commercial or financial relationships that could be construed as a potential conflict of interest.
Acknowledgments
The authors would like to thank Dr. Taeok Bae (Indiana University, United States) for his critical reading of the manuscript and editorial suggestions.
Supplementary Material
The Supplementary Material for this article can be found online at: https://www.frontiersin.org/articles/10.3389/fmolb.2020.586413/full#supplementary-material
Supplementary Figure 1 | Newly found internal promoter M and the transcript galM1.
Supplementary Figure 2 | Effect of bicyclomycin, the Rho-inhibitor, on the production of gal mRNA.
Supplementary Figure 3 | Production of galETKM in the Shine–Dalgarno mutants in the presence of bicyclomycin.
Supplementary Figure 4 | Search of Salmonella operon harboring the 5′ end of mRNA at cistron boundaries.
Supplementary Table 1 | A list of primers used in this study.
References
Adhya, S., and Gottesman, M. (1978). Control of transcription termination. Annu. Rev. Biochem. 47, 967–996. doi: 10.1146/annurev.bi.47.070178.004535
Adhya, S., and Miller, W. (1979). Modulation of the two promoters of the galactose operon of Escherichia coli. Nature 279, 492–494. doi: 10.1038/279492a0
Alifano, P., Bruni, C. B., and Carlomagno, M. S. (1994a). Control of mRNA processing and decay in prokaryotes. Genetica 94, 157–172. doi: 10.1007/bf01443430
Alifano, P., Rivellini, F., Piscitelli, C., Arraiano, C. M., Bruni, C. B., and Carlomagno, M. S. (1994b). Ribonuclease E provides substrates for ribonuclease P-dependent processing of a polycistronic mRNA. Genes Dev. 8, 3021–3031. doi: 10.1101/gad.8.24.3021
Apirion, D. (1973). Degradation of RNA in Escherichia coli. A hypothesis. Mol. Gen. Genet. 122, 313–322. doi: 10.1007/bf00269431
Arnold, T. E., Yu, J., and Belasco, J. G. (1998). mRNA stabilization by the ompA 5′ untranslated region: two protective elements hinder distinct pathways for mRNA degradation. RNA 4, 319–330.
Babitzke, P., and Kushner, S. R. (1991). The Ams (altered mRNA stability) protein and ribonuclease E are encoded by the same structural gene of Escherichia coli. Proc. Natl. Acad. Sci. U.S.A. 88, 1–5. doi: 10.1073/pnas.88.1.1
Baga, M., Goransson, M., Normark, S., and Uhlin, B. E. (1988). Processed mRNA with differential stability in the regulation of E. coli pilin gene expression. Cell 52, 197–206. doi: 10.1016/0092-8674(88)90508-9
Baker, K. E., and Mackie, G. A. (2003). Ectopic RNase E sites promote bypass of 5′-end-dependent mRNA decay in Escherichia coli. Mol. Microbiol. 47, 75–88. doi: 10.1046/j.1365-2958.2003.03292.x
Belasco, J. G. (2017). Ribonuclease E: chopping knife and sculpting tool. Mol. Cell 65, 3–4. doi: 10.1016/j.molcel.2016.12.015
Belasco, J. G., Beatty, J. T., Adams, C. W., Von Gabain, A., and Cohen, S. N. (1985). Differential expression of photosynthesis genes in R. capsulata results from segmental differences in stability within the polycistronic rxcA transcript. Cell 40, 171–181. doi: 10.1016/0092-8674(85)90320-4
Belasco, J. G., Nilsson, G., Von Gabain, A., and Cohen, S. N. (1986). The stability of E. coli gene transcripts is dependent on determinants localized to specific mRNA segments. Cell 46, 245–251. doi: 10.1016/0092-8674(86)90741-5
Bouvet, P., and Belasco, J. G. (1992). Control of RNase E-mediated RNA degradation by 5′-terminal base pairing in E. coli. Nature 360, 488–491. doi: 10.1038/360488a0
Braun, F., Le Derout, J., and Regnier, P. (1998). Ribosomes inhibit an RNase E cleavage which induces the decay of the rpsO mRNA of Escherichia coli. EMBO J. 17, 4790–4797. doi: 10.1093/emboj/17.16.4790
Burton, Z. F., Gross, C. A., Watanabe, K. K., and Burgess, R. R. (1983). The operon that encodes the sigma subunit of RNA polymerase also encodes ribosomal protein S21 and DNA primase in E. coli K12. Cell 32, 335–349. doi: 10.1016/0092-8674(83)90453-1
Callaghan, A. J., Marcaida, M. J., Stead, J. A., Mcdowall, K. J., Scott, W. G., and Luisi, B. F. (2005). Structure of Escherichia coli RNase E catalytic domain and implications for RNA turnover. Nature 437, 1187–1191. doi: 10.1038/nature04084
Carpousis, A. J., Luisi, B. F., and Mcdowall, K. J. (2009). Endonucleolytic initiation of mRNA decay in Escherichia coli. Prog. Mol. Biol. Transl. Sci. 85, 91–135. doi: 10.1016/s0079-6603(08)00803-9
Celesnik, H., Deana, A., and Belasco, J. G. (2007). Initiation of RNA decay in Escherichia coli by 5′ pyrophosphate removal. Mol. Cell 27, 79–90. doi: 10.1016/j.molcel.2007.05.038
Chao, Y., Li, L., Girodat, D., Forstner, K. U., Said, N., Corcoran, C., et al. (2017). In vivo cleavage map illuminates the central role of RNase E in coding and non-coding RNA pathways. Mol. Cell 65, 39–51. doi: 10.1016/j.molcel.2016.11.002
Clarke, J. E., Kime, L., Romero, A. D., and Mcdowall, K. J. (2014). Direct entry by RNase E is a major pathway for the degradation and processing of RNA in Escherichia coli. Nucleic Acids Res. 42, 11733–11751. doi: 10.1093/nar/gku808
Dar, D., and Sorek, R. (2018). Extensive reshaping of bacterial operons by programmed mRNA decay. PLoS Genet 14:e1007354. doi: 10.1371/journal.pgen.1007354
Deana, A., and Belasco, J. G. (2005). Lost in translation: the influence of ribosomes on bacterial mRNA decay. Genes Dev. 19, 2526–2533. doi: 10.1101/gad.1348805
Deana, A., Celesnik, H., and Belasco, J. G. (2008). The bacterial enzyme RppH triggers messenger RNA degradation by 5′ pyrophosphate removal. Nature 451, 355–358. doi: 10.1038/nature06475
Deutscher, M. P. (1985). E. coli RNases: making sense of alphabet soup. Cell 40, 731–732. doi: 10.1016/0092-8674(85)90330-7
Dreyfus, M. (2009). Killer and protective ribosomes. Prog Mol Biol Transl Sci 85, 423–466. doi: 10.1016/s0079-6603(08)00811-8
Emory, S. A., Bouvet, P., and Belasco, J. G. (1992). A 5′-terminal stem-loop structure can stabilize mRNA in Escherichia coli. Genes Dev. 6, 135–148. doi: 10.1101/gad.6.1.135
Hayes, C. S., Bose, B., and Sauer, R. T. (2002). Stop codons preceded by rare arginine codons are efficient determinants of SsrA tagging in Escherichia coli. Proc. Natl. Acad. Sci. U.S.A. 99, 3440–3445. doi: 10.1073/pnas.052707199
Hui, M. P., Foley, P. L., and Belasco, J. G. (2014). Messenger RNA degradation in bacterial cells. Annu. Rev. Genet 48, 537–559. doi: 10.1146/annurev-genet-120213-092340
Iost, I., and Dreyfus, M. (1995). The stability of Escherichia coli lacZ mRNA depends upon the simultaneity of its synthesis and translation. EMBO J. 14, 3252–3261. doi: 10.1002/j.1460-2075.1995.tb07328.x
Jiang, X., and Belasco, J. G. (2004). Catalytic activation of multimeric RNase E and RNase G by 5′-monophosphorylated RNA. Proc. Natl. Acad. Sci. U.S.A. 101, 9211–9216. doi: 10.1073/pnas.0401382101
Joyce, S. A., and Dreyfus, M. (1998). In the absence of translation, RNase E can bypass 5′ mRNA stabilizers in Escherichia coli. J. Mol. Biol. 282, 241–254. doi: 10.1006/jmbi.1998.2027
Keiler, K. C., Waller, P. R., and Sauer, R. T. (1996). Role of a peptide tagging system in degradation of proteins synthesized from damaged messenger RNA. Science 271, 990–993. doi: 10.1126/science.271.5251.990
Lee, H. J., Jeon, H. J., Ji, S. C., Yun, S. H., and Lim, H. M. (2008). Establishment of an mRNA gradient depends on the promoter: an investigation of polarity in gene expression. J. Mol. Biol. 378, 318–327. doi: 10.1016/j.jmb.2008.02.067
Lewis, D. E., and Adhya, S. (2015). Molecular mechanisms of transcription initiation at gal promoters and their multi-level regulation by GalR, CRP and DNA Loop. Biomolecules 5, 2782–2807. doi: 10.3390/biom5042782
Li, Z., Pandit, S., and Deutscher, M. P. (1999). RNase G (CafA protein) and RNase E are both required for the 5′ maturation of 16S ribosomal RNA. EMBO J. 18, 2878–2885. doi: 10.1093/emboj/18.10.2878
Luciano, D. J., Vasilyev, N., Richards, J., Serganov, A., and Belasco, J. G. (2017). A Novel RNA Phosphorylation State Enables 5′ End-Dependent Degradation in Escherichia coli. Mol. Cell 67, 44–54e46.
Mackie, G. A. (1998). Ribonuclease E is a 5′-end-dependent endonuclease. Nature 395, 720–723. doi: 10.1038/27246
Mackie, G. A. (2013). RNase E: at the interface of bacterial RNA processing and decay. Nat. Rev. Microbiol. 11, 45–57. doi: 10.1038/nrmicro2930
McDowall, K. J., Lin-Chao, S., and Cohen, S. N. (1994). A+U content rather than a particular nucleotide order determines the specificity of RNase E cleavage. J. Biol. Chem. 269, 10790–10796.
McLaren, R. S., Newbury, S. F., Dance, G. S., Causton, H. C., and Higgins, C. F. (1991). mRNA degradation by processive 3′-5′ exoribonucleases in vitro and the implications for prokaryotic mRNA decay in vivo. J. Mol. Biol. 221, 81–95. doi: 10.1016/0022-2836(91)90806-h
Melefors, O., and von Gabain, A. (1991). Genetic studies of cleavage-initiated mRNA decay and processing of ribosomal 9S RNA show that the Escherichia coli ams and rne loci are the same. Mol. Microbiol. 5, 857–864. doi: 10.1111/j.1365-2958.1991.tb00759.x
Mohanty, B. K., and Kushner, S. R. (2016). Regulation of mRNA decay in bacteria. Annu. Rev. Microbiol. 70, 25–44. doi: 10.1146/annurev-micro-091014-104515
Mott, J. E., Galloway, J. L., and Platt, T. (1985). Maturation of Escherichia coli tryptophan operon mRNA: evidence for 3′ exonucleolytic processing after rho-dependent termination. EMBO J. 4, 1887–1891. doi: 10.1002/j.1460-2075.1985.tb03865.x
Mudd, E. A., Krisch, H. M., and Higgins, C. F. (1990). RNase E, an endoribonuclease, has a general role in the chemical decay of Escherichia coli mRNA: evidence that rne and ams are the same genetic locus. Mol. Microbiol. 4, 2127–2135. doi: 10.1111/j.1365-2958.1990.tb00574.x
Muto, A., Sato, M., Tadaki, T., Fukushima, M., Ushida, C., and Himeno, H. (1996). Structure and function of 10Sa RNA: trans-translation system. Biochimie 78, 985–991. doi: 10.1016/s0300-9084(97)86721-1
Newbury, S. F., Smith, N. H., and Higgins, C. F. (1987). Differential mRNA stability controls relative gene expression within a polycistronic operon. Cell 51, 1131–1143. doi: 10.1016/0092-8674(87)90599-x
Ono, M., and Kuwano, M. (1979). A conditional lethal mutation in an Escherichia coli strain with a longer chemical lifetime of messenger RNA. J. Mol. Biol. 129, 343–357. doi: 10.1016/0022-2836(79)90500-x
Py, B., Higgins, C. F., Krisch, H. M., and Carpousis, A. J. (1996). A DEAD-box RNA helicase in the Escherichia coli RNA degradosome. Nature 381, 169–172. doi: 10.1038/381169a0
Regnier, P., and Hajnsdorf, E. (1991). Decay of mRNA encoding ribosomal protein S15 of Escherichia coli is initiated by an RNase E-dependent endonucleolytic cleavage that removes the 3′ stabilizing stem and loop structure. J. Mol. Biol. 217, 283–292. doi: 10.1016/0022-2836(91)90542-e
Richards, J., and Belasco, J. G. (2019). Obstacles to scanning by RNase E govern bacterial mRNA lifetimes by hindering access to distal cleavage sites. Mol. Cell 74, 284–295e285.
Spickler, C., Stronge, V., and Mackie, G. A. (2001). Preferential cleavage of degradative intermediates of rpsT mRNA by the Escherichia coli RNA degradosome. J. Bacteriol. 183, 1106–1109. doi: 10.1128/jb.183.3.1106-1109.2001
Taraseviciene, L., Miczak, A., and Apirion, D. (1991). The gene specifying RNase E (rne) and a gene affecting mRNA stability (ams) are the same gene. Mol. Microbiol. 5, 851–855. doi: 10.1111/j.1365-2958.1991.tb00758.x
von Gabain, A., Belasco, J. G., Schottel, J. L., Chang, A. C., and Cohen, S. N. (1983). Decay of mRNA in Escherichia coli: investigation of the fate of specific segments of transcripts. Proc. Natl. Acad. Sci. U.S.A. 80, 653–657. doi: 10.1073/pnas.80.3.653
Wachi, M., Umitsuki, G., and Nagai, K. (1997). Functional relationship between Escherichia coli RNase E and the CafA protein. Mol. Gen. Genet 253, 515–519. doi: 10.1007/s004380050352
Wang, X., Ji, S. C., Jeon, H. J., Lee, Y., and Lim, H. M. (2015). Two-level inhibition of galK expression by Spot 42: degradation of mRNA mK2 and enhanced transcription termination before the galK gene. Proc. Natl. Acad. Sci. U.S.A. 112, 7581–7586. doi: 10.1073/pnas.1424683112
Wang, X., Ji, S. C., Yun, S. H., Jeon, H. J., Kim, S. W., and Lim, H. M. (2014). Expression of each cistron in the gal operon can be regulated by transcription termination and generation of a galk-specific mRNA, mK2. J. Bacteriol. 196, 2598–2606. doi: 10.1128/jb.01577-14
Wang, X., Monford Paul Abishek, N., Jeon, H. J., Lee, Y., He, J., Adhya, S., et al. (2019). Processing generates 3′ ends of RNA masking transcription termination events in prokaryotes. Proc. Natl. Acad. Sci. U.S.A. 116, 4440–4445. doi: 10.1073/pnas.1813181116
Keywords: mRNA decay, RNase E, mRNA stability, translation initiation, polycistronic gal mRNA
Citation: Jeon HJ, Kang C, N MPA, Lee Y, Wang X, Chattoraj DK and Lim HM (2020) Translation Initiation Control of RNase E-Mediated Decay of Polycistronic gal mRNA. Front. Mol. Biosci. 7:586413. doi: 10.3389/fmolb.2020.586413
Received: 23 July 2020; Accepted: 28 August 2020;
Published: 06 November 2020.
Edited by:
Chew Chieng Yeo, Sultan Zainal Abidin University, MalaysiaReviewed by:
Miguel Angel Cevallos, National Autonomous University of Mexico, MexicoXiongfeng Dai, Central China Normal University, China
Copyright © 2020 Jeon, Kang, N, Lee, Wang, Chattoraj and Lim. This is an open-access article distributed under the terms of the Creative Commons Attribution License (CC BY). The use, distribution or reproduction in other forums is permitted, provided the original author(s) and the copyright owner(s) are credited and that the original publication in this journal is cited, in accordance with accepted academic practice. No use, distribution or reproduction is permitted which does not comply with these terms.
*Correspondence: Heon M. Lim, aG1saW1AY251LmFjLmty
†Present address: Heung Jin Jeon, Infection Control Convergence Research Center, College of Medicine, Chungnam National University, Daejeon, South Korea