- Matthias-Schleiden-Institut, AG Bakteriengenetik, Friedrich-Schiller-Universität Jena, Jena, Germany
In bacterial cells we find a variety of interacting macromolecules, among them RNAs and proteins. Not only small regulatory RNAs (sRNAs), but also small proteins have been increasingly recognized as regulators of bacterial gene expression. An average bacterial genome encodes between 200 and 300 sRNAs, but an unknown number of small proteins. sRNAs can be cis- or trans-encoded. Whereas cis-encoded sRNAs interact only with their single completely complementary mRNA target transcribed from the opposite DNA strand, trans-encoded sRNAs are only partially complementary to their numerous mRNA targets, resulting in huge regulatory networks. In addition to sRNAs, uncharged tRNAs can interact with mRNAs in T-box attenuation mechanisms. For a number of sRNA-mRNA interactions, the stability of sRNAs or translatability of mRNAs, RNA chaperones are required. In Gram-negative bacteria, the well-studied abundant RNA-chaperone Hfq fulfils this role, and recently another chaperone, ProQ, has been discovered and analyzed in this respect. By contrast, evidence for RNA chaperones or their role in Gram-positive bacteria is still scarce, but CsrA might be such a candidate. Other RNA-protein interactions involve tmRNA/SmpB, 6S RNA/RNA polymerase, the dual-function aconitase and protein-bound transcriptional terminators and antiterminators. Furthermore, small proteins, often missed in genome annotations and long ignored as potential regulators, can interact with individual regulatory proteins, large protein complexes, RNA or the membrane. Here, we review recent advances on biological role and regulatory principles of the currently known sRNA-mRNA interactions, sRNA-protein interactions and small protein-protein interactions in the Gram-positive model organism Bacillus subtilis. We do not discuss RNases, ribosomal proteins, RNA helicases or riboswitches.
Introduction
The first regulatory RNAs were identified and intensively studied as control elements in replication, conjugation and maintenance of bacterial plasmids as well as in transposition and transduction (rev. in Wagner and Romby, 2015). However, only after the publication of a variety of regulatory RNAs from intergenic regions of E. coli by two groups (Argaman et al., 2001; Wassarman et al., 2001), knowledge on bacterial regulatory RNAs started to expand. This was due to systematic bioinformatic approaches combined with experimental studies as well as RNA sequencing, which revealed that an average bacterial genome encodes between 200 and 300 small regulatory RNAs (sRNAs). The majority of sRNA targets are mRNAs. Therefore, sRNA-mRNA interactions play an important role in virtually all bacterial cells. Furthermore, uncharged tRNA can interact with the T-box in 5′ UTRs of various mRNAs encoding amino-acid related genes resulting in transcriptional read-through. Bacterial cells contain a number of RNA chaperones that stabilize sRNAs, modulate mRNA translation or promote mRNA-sRNA interactions. In general, Hfq (rev. in Kavita et al., 2018), ProQ (rev. in Olejniczak and Storz, 2017) and CsrA (rev. in Vakulskas et al., 2015) are known and have been investigated in great detail in this respect. Interestingly, Gram-positive bacteria do not encode ProQ, and Hfq does not seem to play a comparable role as in Gram-negative bacteria. Furthermore, CsrA has not been found to sequester small RNAs in Gram-positives. Other RNA-protein interactions involve tmRNA, the ubiquitous RNase P, 6S RNA that interacts with RNA polymerase, RNA antiterminators and terminators that are bound and regulated by proteins as well as the aconitase, a TCA enzyme that moonlights at iron limiting conditions as RNA binding protein. In addition, interactions between small proteins and larger proteins or protein complexes play an important role in gene expression control. Only a few interactions have been elucidated and investigated in some detail in this new field, e.g., modulation of the function of individual proteins or activation/inactivation of multiprotein complexes by small proteins, interactions of small proteins with membrane proteins or the membrane itself.
Here, we present an overview of recent advances in sRNA/tRNA-mRNA, RNA-protein and small protein-protein interactions in the Gram-positive model organism Bacillus subtilis. However, we will not include RNases, RNA helicases, ribosomal proteins or riboswitches.
RNA-RNA Interactions
Two main groups of interactions will be reviewed here: small regulatory RNAs (sRNAs) that interact with their target mRNAs and tRNAs that interact with the 5′ UTR of mRNAs in the so-called T-box riboswitches.
sRNAs can be classified in cis- and trans-encoded sRNAs. The first sRNAs discovered 35–40 years ago on plasmids and transposons are cis-encoded, i.e., transcribed convergently to their RNA targets from the complementary DNA strand and able to form complete duplexes with them (rev. in Brantl, 2012; Wagner and Romby, 2015). Therefore, each cis-encoded sRNA has only one target RNA. By contrast, trans-encoded sRNAs are encoded in another region of the genome and are only partially complementary to their broad variety of target RNAs. Whereas for the majority of the currently known ≈150 E. coli sRNAs targets have been identified and mechanisms of action elucidated, only a dozen of the 108 confirmed B. subtilis sRNAs (Rasmussen et al., 2009; Irnov et al., 2010) have been investigated in great detail. Here, we focus on sRNAs with known targets.
Cis-Encoded sRNAs
The largest group of currently known B. subtilis cis-encoded sRNAs are type I antitoxins that interact with their target toxin mRNAs either at their 5′ or 3′ end by a base-pairing mechanism. In addition, the targets for a few other cis-encoded sRNAs have been identified and characterized (see below). Table 1 provides an overview of all currently known cis-encoded sRNAs in B. subtilis.
Antitoxins in Type I Toxin-Antitoxin Systems
Type I toxin-antitoxin (TA) systems are composed of two elements, a hydrophobic toxin and an RNA antitoxin that neutralizes toxin action by interacting with the toxin mRNA to affect its stability and/or translation. Out of 14 predicted type I TA systems of B. subtilis (Durand et al., 2012a), four have been verified experimentally and analyzed in some detail: txpA/RatA (Silvaggi et al., 2005), bsrG/SR4 (Jahn et al., 2012), bsrE/SR5 (Meißner et al., 2015; Müller et al., 2016), and yonT/SR6 (Durand et al., 2012b; Reif et al., 2018). The majority of them are located on prophage elements or phage remnants in the chromosome.
The txpA/RatA module is encoded on the chromosomal skin element. In ratA knockout strains, the toxin TxpA (59 aa) causes cell lysis on agar plates after 5 days. The 220 nt long RatA overlaps the 3′ end of txpA mRNA by ∼120 nt. The interaction between both RNAs promotes the degradation of txpA mRNA by RNase III, which is required for the viability of B. subtilis and makes RNase III an essential enzyme in this bacterium (Durand et al., 2012b; Figure 1A). The secondary structures of RatA and txpA RNA as well as their complex have been experimentally determined. The SD sequence of txpA is sequestered in a 5 bp double-stranded region. RatA binding does not alter the txpA structure around the SD sequence, i.e., it has no influence on its accessibility to the ribosomal 30S SU. None of the interacting loops contains a U-turn motif (see below).
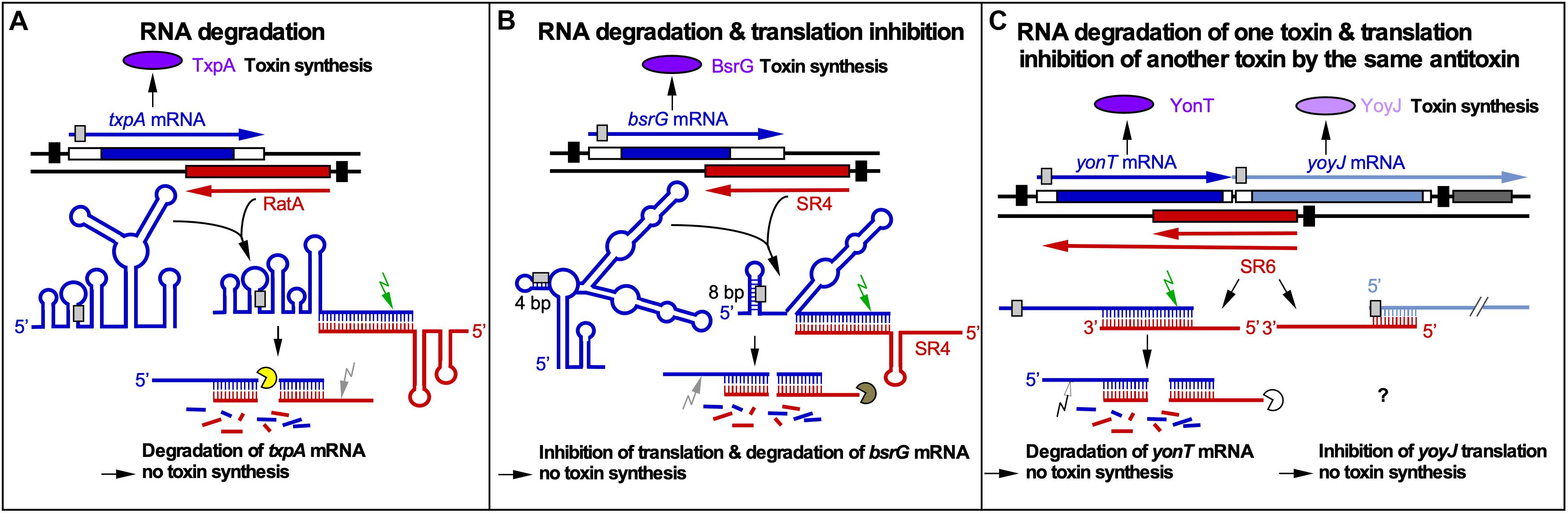
Figure 1. RNA-RNA interactions of cis-encoded sRNAs from B. subtilis. Black bars denote promoters. Toxins are drawn in purple or light purple, toxin mRNAs in blue or gray blue and antitoxins in red. Toxin ORFs are represented by blue and gray blue bars. Light gray boxes depict RBS. Arrows symbolize endoribonucleases (RNase III, green; RNase Y, gray; unknown RNase, white) and circular segments 3′-5′ exoribonucleases (PNPase, yellow; RNase R, brown; unknown RNase, white). (A) Promotion of RNA degradation. The antitoxin RatA and its txpA toxin mRNA base-pair at their 3′ ends. (B) RNA degradation and translation inhibition. The antitoxin SR4 and the corresponding bsrG toxin mRNA interact at their 3′ ends. SR4 binding to bsrG mRNA induces a conformational alteration that extends the region sequestering the SD sequence from 4 to 8 bp which inhibits bsrG translation. Additionally, the SR4/bsrG mRNA interaction facilitates toxin mRNA decay by an initial RNase III cleavage followed by subsequent RNase R and RNase Y degradation. (C) One antitoxin inhibits two toxins by different mechanisms. Antitoxin SR6 and yonT toxin mRNA base-pair at their 3′ ends, which promotes yonT mRNA decay by an initial RNase III cleavage that is followed by degradation by so far unidentified RNases. Furthermore, SR6 interacts with yoyJ toxin mRNA by base-pairing at the 5′ ends, which does not promote yoyJ mRNA degradation, but prevents yoyJ overexpression, possibly by translational inhibition. (Adapted with permission from Brantl and Müller, 2019).
The bsrG/SR4 module is located on the chromosomal SPβ prophage. In sr4 knockout strains, the hydrophobic toxin BsrG (38 aa) causes cell lysis on agar plates after overnight incubation at 37°C (Jahn et al., 2012, 2015). The 294 nt long bsrG mRNA and the 180 nt long antitoxin SR4 interact at their 3′ ends resulting in degradation of bsrG mRNA by RNase III 8 nt downstream from the stop codon. RNase III is, however, not involved in the degradation of either bsrG RNA or SR4 alone. Endoribonuclease Y and the 3′-5′ exoribonuclease R are responsible for further degradation of both RNAs. PNPase processes SR4 precursors into the mature RNA. In contrast to txpA/RatA, RNase III is not essential for the bsrG/SR4 system because a Δrnc strain neither lysed on agar plates nor had mutations in the bsrG ORF. Furthermore, the RNA chaperone Hfq is not required for the function of the bsrG/SR4 system, since a Δhfq strain does neither lyse on agar plates nor displays changed half-lives of SR4 or bsrG mRNA (Jahn et al., 2012). The secondary structures of SR4 and bsrG mRNA and their complex were experimentally determined (Jahn and Brantl, 2013). SR4 induces structural alterations around the SD sequence of bsrG by extending a 4 bp double-stranded region that makes the SD barely accessible into an 8 bp region which inhibits bsrG translation. SR4 is, therefore, the first dual-function type I antitoxin: It facilitates bsrG mRNA degradation and prevents bsrG translation by impairing ribosome access to the bsrG SD (Figure 1B).
Complex formation assays with bsrG RNA and SR4 yielded an apparent binding constant kapp of 6.5 × 105 M–1 s–1 (Jahn and Brantl, 2013). The elucidation of the binding pathway of bsrG mRNA and SR4 (Jahn and Brantl, 2013; Figure 2A) revealed that binding initiates with a single loop-loop contact between loop L3 of bsrG RNA and loop L4 of the SR4 terminator stem-loop. Subsequently, base-pairing progresses via the single-stranded region between L4 and L3 toward L3 of SR4, and, finally to L2 that pairs with the bsrG terminator-stem-loop. However, the latter interaction is not essential for efficient binding. A 5′ YUNR motif present in L3 of bsrG RNA might form a U-turn (see Heidrich and Brantl, 2003) to provide a scaffold for the efficient initial interaction with SR4. An excess of antitoxin over toxin mRNA is obtained by the 6- to 10-fold higher promoter strength of the sr4 promoter compared to the bsrG promoter (Jahn et al., 2012).
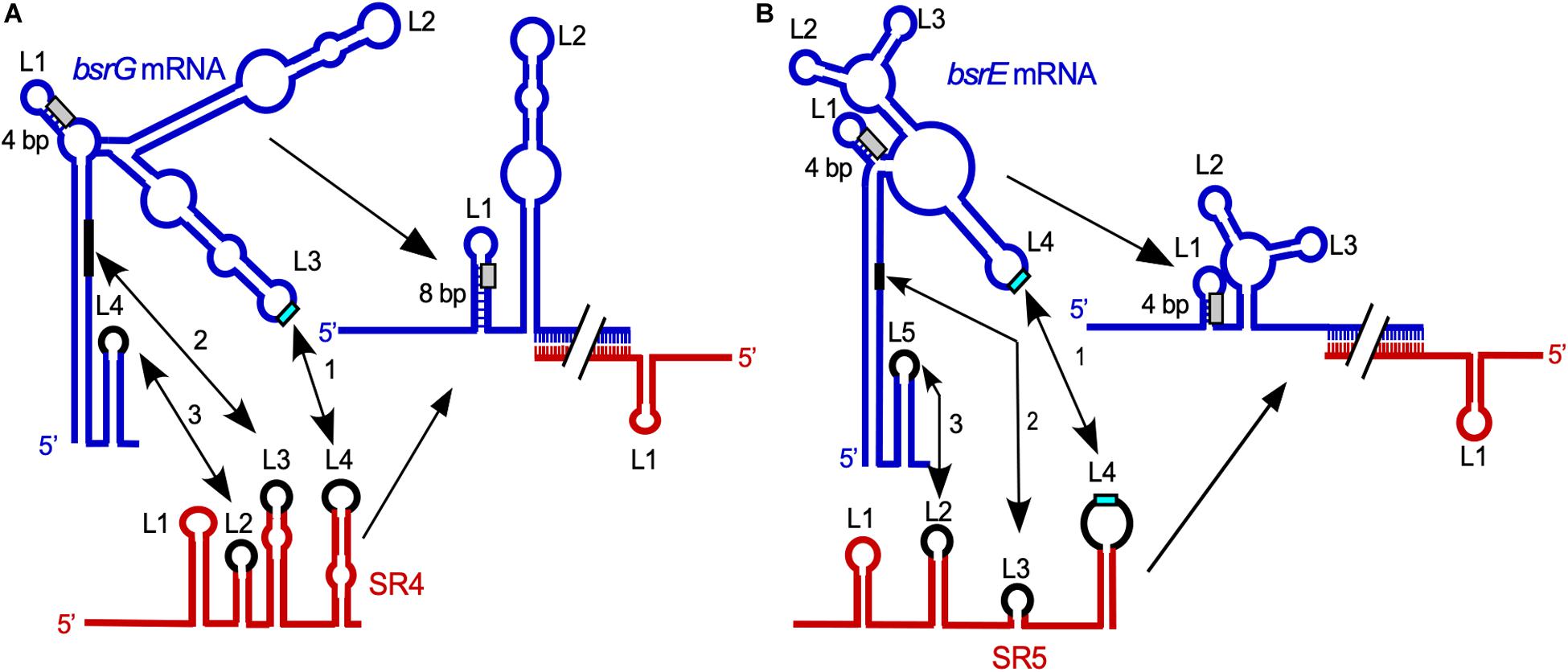
Figure 2. Comparison of the SR4/bsrG RNA (A) and SR5/bsrE RNA (B) interaction pathways. Blue, toxin mRNAs; red, RNA antitoxins. U-turn motifs are indicated in turquoise and RBS by light gray boxes. The interaction chronology is designated by 1–3. L, loop. (A) The initial contact between SR4 and bsrG RNA occurs between L4 of SR4 and L3 of bsrG RNA (1). It is followed by helix progression to an interaction between SR4 L3 and the 3′ part of helix P1 of bsrG RNA (2) and finally reaches L2 of SR4 that binds terminator loop L4 of bsrG RNA (3). The latter interaction is not essential. (B) The binding pathway of SR5 and bsrE RNA comprises three similar subsequent interactions. Schematic secondary structures are based on experimentally probed structures in Jahn and Brantl (2013) and Meißner et al. (2015). (Adapted with permission from Brantl and Jahn, 2015; Brantl and Müller, 2019).
The multistress-responsive bsrE/SR5 module is encoded on the prophage-like element P6. In contrast to txpA/RatA and bsrG/SR4, deletion of the sr5 promoter to prevent antitoxin expression does not lead to cell lysis on agar plates. Only bsrE overexpression from a multicopy plasmid inhibits cell growth and causes lysis on agar plates indicating that BsrE (30 aa) is a much weaker toxin than BsrG (Müller et al., 2016). SR5 (163 nt) and bsrE mRNA (256 nt) interact by 114 complementary bp at their 3′ ends, and this interaction causes degradation of the toxin mRNA by RNase III at all three bsrE stop codon positions (Meißner et al., 2015). The secondary structures of bsrE mRNA, the antitoxin SR5 and their complex were mapped and are similar to those of bsrG RNA and SR4 (Meißner et al., 2015). Complex formation between toxin mRNA and RNA antitoxin were studied and showed a similar kapp value of 1–3 × 106 M–1 s–1. Likewise, the SR5/bsrE mRNA interaction pathway (Figure 2B) closely resembles that of bsrG RNA and SR4 (see above). The main three differences between both pathways are: (i) the presence of only one U-turn motif in loop L3 of bsrG mRNA but two U-turn motifs, one in L4 of bsrE RNA and another in terminator loop L4 in SR5 which are engaged in the initial contact, (ii) two stem-loops of SR5, SL2 and SL4, are fundamental for formation of a stable duplex with bsrE mRNA, whereas only one stem-loop and a single stranded region of SR4 sufficed and (iii) SR5 binding did not trigger a structural change around the toxin mRNA RBS, whereas SR4 binding did. Therefore, the antitoxin SR5 is monofunctional: It promotes toxin mRNA degradation but does not inhibit bsrE translation directly. Interestingly, both bsrE mRNA and SR5 respond to different stress factors: Whereas bsrG mRNA is only heat-shock sensitive due to its refolding at 48–55°C which results in rapid degradation by RNases Y and J1 (Jahn and Brantl, 2016), bsrE mRNA is sensitive to heat-shock, ethanol stress and alkaline pH (Müller et al., 2016). SR5 amounts were influenced by iron limitation, acid, alkaline and anaerobic stress. Oxygen deficiency was the only stress that altered the SR5/bsrE mRNA ratio from 9: 1 to 0.5: 1, i.e., has the potential to induce cell lysis (Müller et al., 2016).
The multistress-responsive yonT/yoyJ/SR6 module is the second type I TA system encoded on the SPβ prophage. The peculiarity in this system is that antitoxin SR6 interacts with two toxin mRNAs encoding YonT (59 aa) and YoyJ (83 aa). The 3′ end of the 100 nt long SR6 interacts with the 3′ end of yonT mRNA to promote its degradation by RNase III. On the other hand, the 5′ end of SR6 binds to the 5′ end yoyJ mRNA, but neither affects the amount nor half-life of yoyJ RNA. Instead, it seems to inhibit yoyJ translation (Reif et al., 2018; Figure 1C). The latter interaction can only be postulated as there is only indirect evidence for YoyJ being a weak toxin: A yoyJ overexpression plasmid could only be established by transformation in a B. subtilis strain that carries the sr6 gene in the chromosome (Reif et al., 2018). In contrast to TxpA, BsrG or BsrE, YonT is a very strong toxin, as neither SR6 could be deleted from the chromosome nor yonT overexpressed from a medium copy plasmid in E. coli or B. subtilis. So far, no secondary structures were probed or complex formation studies performed. However, calculations of the yonT mRNA and SR6 amounts revealed only in minimal CSE medium with glucose at stationary phase an excess of yonT mRNA over SR6, i.e., under these conditions the toxin could be expressed.
Similarly to bsrE/SR5, yonT/yoyJ/SR6 responds to a number of stress factors: After ethanol stress, yonT mRNA disappeared as bsrE mRNA within 0.5 min, and heat-shock caused a 4-fold decrease of yonT RNA levels. However, in contrast to SR4 or SR5, SR6 levels also decreased about 4-fold after heat-shock. Vancomycin (cell-wall stress) treatment led to a decrease of both yonT mRNA and SR6.
Another potential type I TA system is bsrH/anti-bsrH (Durand et al., 2012a, b) located on the skin prophage. bsrH mRNA (285 nt) and anti-bsrH (200 nt) can interact at their 3′ ends and RNase J1 is involved in cleavage of the complex. But so far, it has not been shown that BsrH (29 aa) acts as a toxin in B. subtilis and that anti-bsrH neutralizes toxin action.
Other Cis-Encoded sRNAs in B. subtilis
At least five other cis-encoded sRNAs (antisense RNAs) have been reported: S25, gdpPas/S1559, S1326, S1136-1134 and S1290. S25 (≈1350 nt) is expressed under control of extracytoplasmic sigma factors σX and σM and regulates an autolysin encoded by yabE (Eiumphungporn and Helmann, 2009). S1559/gdpPas (Luo and Helmann, 2012) is transcribed under control of sD from the middle of the gdpP gene encoding the phosphodiesterase responsible for degradation of cyclic di-AMP. Neither sense nor antisense RNA were detectable in Northern blots. The presence of S1559/gdpPas reduced the level of FLAG-tagged GdpP about 2.5 to three-fold, but could not be associated with any phenotype which makes the biological role of this regulation elusive. Antisense RNA S1326 is transcribed from a σB dependent promoter located downstream of the cwlO gene that encodes a D,L-endopeptidase type autolysin (Noone et al., 2014). S1326 has a heterogenous length (between 700 and 2200 nt) and was detectable under phosphate stress. The largest S1326 transcript extends beyond the cwlO promoter. The cwlO mRNA is highly unstable due to an RNase Y cleavage in the leader region which seems to be decisive for regulation of this RNA. S1326 influenced weakly the exit of the cells from stationary phase.
The σB dependent sRNA S1136-1134 transcribed in cis to rpsD mRNA encoding the ribosomal primary binding protein S4 (22.7 kD) was reported to decrease the amount of rpsD mRNA and, consequently, the amount of the small ribosomal subunit ∼1.5 fold under ethanol-stress (Mars et al., 2015a). S1136-1134 could only act in cis, which suggests that its mechanism of action is transcriptional interference.
The same holds true for σB-dependent S1290 that is transiently transcribed in response to salt stress. S1290 acts solely in cis and independent of RNase III on its convergently transcribed target opuB mRNA encoding a choline transporter (Rath et al., 2020). It causes a time-delayed osmotic induction of opuB mRNA, since under acute salt stress, B. subtilis initially relies on the promiscuous OpuC transporter to import pre-formed compatible solutes as proline- or glycine betaine before employing OpuB to import choline that has to be converted into glycine betaine. In addition to S1290, opuB expression is dependent on the degree of the imposed osmotic stress.
For neither of these antisense RNAs, secondary structures or – in case they act through complex formation with their target RNAs – those of the antisenseRNA/target RNA complexes were determined.
Trans-Encoded sRNAs in B. subtilis
Currently, four trans-encoded sRNAs are known for which targets have been identified: SR1 (Licht et al., 2005; Heidrich et al., 2006), FsrA (Gaballa et al., 2008), RoxS (Durand et al., 2015, 2017), and RNAC (Mars et al., 2015b; Figure 3). Among them, SR1 is the only dual-function sRNA which acts as a base-pairing sRNA and as mRNA encoding a small peptide, SR1P (see below). Table 2 provides an overview of all currently known trans-encoded sRNAs in B. subtilis.
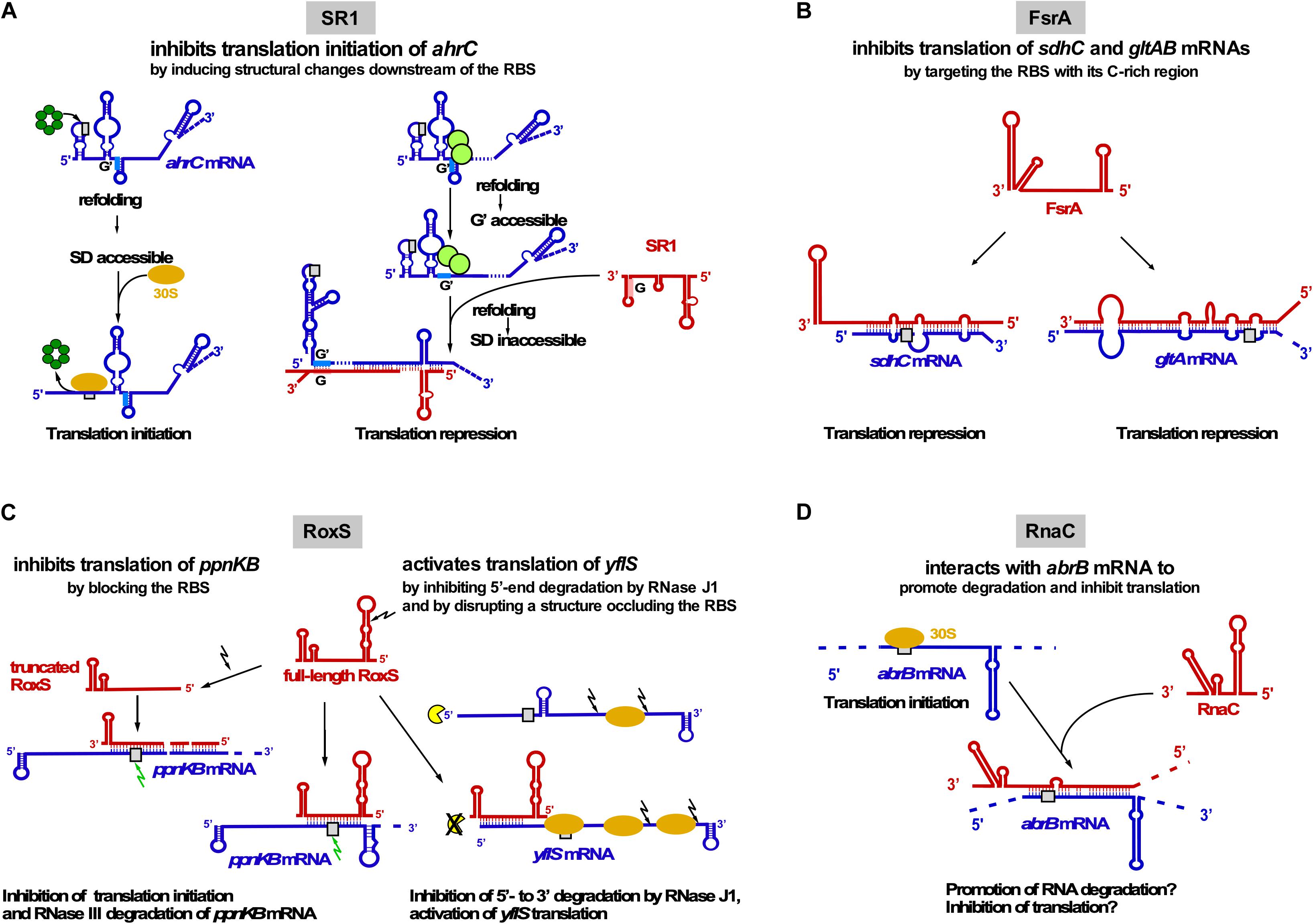
Figure 3. Interactions of trans-encoded sRNAs with their target mRNAs (A) SR1 interacts with ahrC mRNA about 100 nt downstream of the RBS which induces structural changes around the ahrC RBS that inhibit translation initiation. Left: Hfq (dark-green) binds immediately upstream of the ahrC RBS (gray rectangle) to make it accessible to the 30S SU. Right: By binding to GGA motives 1–3 of ahrC mRNA, CsrA (light-green) induces a slight structural change that makes region G′ accessible to complementary region G of SR1. SR1 binding induces a structural change that renders the ahrC RBS inaccessible to 30S binding, thereby inhibiting translation initiation (Brantl and Brückner, 2014). (B) Interaction of FsrA with sdhC and gltAB mRNAs. FsrA has numerous targets, but probably for all of them (see text) inhibits translation by targeting the RBS or an adjacent region. (C) Interaction of RoxS with two target mRNAs. Whereas RoxS and its truncated derivative repress ppnKB translation, full-length RoxS activates yflS translation, mainly by inhibiting RNase J1 degradation from the 5′ end. (D) Interaction of RNAC with abrB mRNA which might promote RNA degradation and inhibit abrB translation. Red, sRNAs; blue, target mRNAs; beige oval, 30S SU; gray box, RBS; green arrow, RNase III; black arrow, RNase Y; yellow, RNase J1.
SR1 (205 nt) has been discovered using a computational search for sRNAs in intergenic regions of the B. subtilis genome and later confirmed by Northern blotting (Licht et al., 2005). Its transcription is repressed under glycolytic conditions ≈20–30-Fold by CcpN binding upstream of and overlapping the sr1 promoter psr1 and, to a minor extent, by CcpA binding to a site ∼260 bp upstream of the transcription start site. CcpN needs ATP and a slightly acidic pH to exert its effect (Licht et al., 2008) and interacts with the α-subunit of the RNA polymerase to prevent promoter escape (Licht and Brantl, 2009). The first identified primary target of SR1 is ahrC mRNA (Heidrich et al., 2006) encoding the transcription activator of the arginine catabolic operons rocABC and rocDEF and the transcription repressor of the arginine biosynthesis genes (Czaplewski et al., 1992). In contrast to many other sRNAs, SR1 neither affects the half-life nor the amount of ahrC mRNA (Heidrich et al., 2006). Binding of SR1 and ahrC mRNA via their 7 complementary regions (A to G in SR1, A′ to G′ in ahrC mRNA) results in inhibition of ahrC translation initiation by a novel mechanism: induction of structural changes 20 to 40 nt downstream of the RBS, although SR1 binds ∼100 nt downstream of the ahrC RBS (Heidrich et al., 2007; Figure 3A). The interaction between SR1 and ahrC mRNA initiates at region G/G′ and is the crucial interaction, but the other complementary regions also contribute to complex formation and hence, translation inhibition (Heidrich et al., 2007). Recently, it was shown that the abundant RNA chaperone CsrA promotes the interaction between SR1 and ahrC mRNA: It binds both RNAs and induces a slight structural alteration in ahrC mRNA which liberates region G’ for a more efficient interaction with SR1 (Müller et al., 2019; Figure 3A).
SR1 is not only a trans-encoded base-pairing sRNA, but also an mRNA encoding a 39 aa protein, SR1P (Gimpel et al., 2010). SR1P interacts with the glyceraldehyde-3P dehydrogenase GapA, thereby affecting RNA degradation (Gimpel and Brantl, 2016, 2017, see below and Figure 4). Both functions of SR1 are remarkably conserved over more than one billion years of evolution (Gimpel et al., 2012).
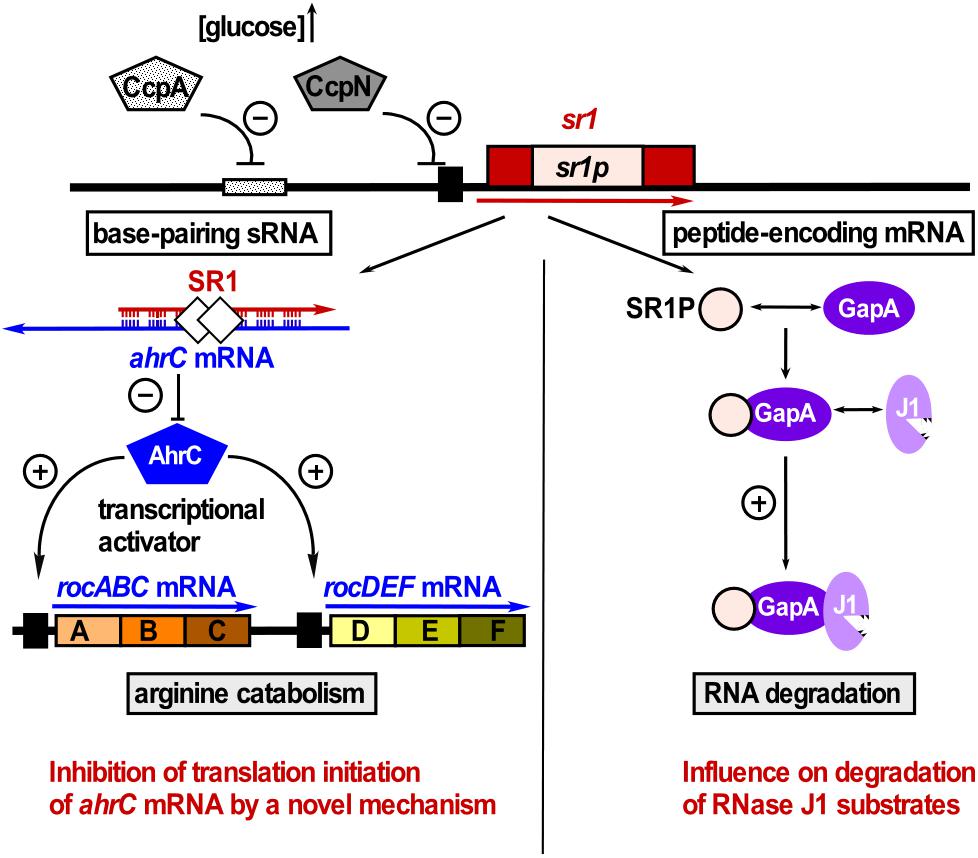
Figure 4. SR1 is a dual-function sRNA. Left: SR1 acts as base-pairing sRNA in arginine catabolism by inhibiting translation of ahrC mRNA encoding the transcription activator of the arginine catabolic operons. SR1 transcription is inhibited by repressors CcpA and CcpN. CsrA (white diamonds) promotes the SR1-ahrC mRNA interaction (Müller et al., 2019). Right: SR1 is an mRNA encoding SR1P that plays a role in RNA degradation. SR1P interacts with GapA thereby promoting the GapA-RNase J1 interaction and the enzymatic activity of RNase J1 (adapted with permission from Gimpel and Brantl, 2017).
In 2008, FsrA (84 nt), a functional homolog of E. coli RyhB, was discovered (Gaballa et al., 2008). FsrA is under transcriptional control of the iron-dependent Fur repressor. When iron is scarce, FsrA inhibits translation of target mRNAs involved in iron metabolism and storage, e.g., succinate dehydrogenase sdhCAB (Figure 3B), citB (aconitase) and lutABC (oxidases important for growth on lactate as sole C source). Subsequent studies revealed that FsrA is a global regulator with a broad variety of targets, among them gltAB encoding the iron-sulfur containing enzyme glutamate synthase, dctP (dicarboxylate transporter), resA and qcrA. By predicting complementary regions with RNAhybrid and M-fold the authors suggest that a C-rich single-stranded region of FsrA base-pairs with the RBS of its target mRNAs (Smaldone et al., 2012a). So far, in vivo base-pairing has only been confirmed between FsrA and the 5′ UTR of gltAB (Smaldone et al., 2012a; Figure 3B). In vitro base-pairing was demonstrated by EMSA for FsrA and sdhC mRNA, whereas for the other targets, only effects on mRNA and protein levels were reported (Gaballa et al., 2008). In contrast to the functionally related Fur-regulated sRNA RyhB from E. coli, which requires the RNA chaperone Hfq, FsrA cooperates with one, two or three Fur-regulated small basic proteins FbpA, FbpB, and FbpC suggested to be RNA chaperones. Under iron-deplete growth conditions, FsrA and FbpB (48 aa) inhibit lutABC to allow the direction of iron to higher-priority target proteins. Both target mRNA and protein levels were affected about two-fold by FsrA and FbpB with FbpB having a stronger effect on RNA levels. Whereas FsrA might directly inhibit translation, FbpB might facilitate FsrA/lutABC RNA pairing or recruit the RNA degradation machinery (Smaldone et al., 2012b). Currently, there is neither experimental evidence for FbpB binding RNA nor for its mechanism of action. Neither for sdhCAB mRNA nor for citB mRNA a contribution of one of the Fbp proteins to FsrA-mediated repression was found (Gaballa et al., 2008).
RoxS (originally termed RsaE, 115 nt) is the only base-pairing sRNA conserved between B. subtilis and S. aureus. RoxS transcription is activated by nitric oxide depending on the two-component system ResDE (Durand et al., 2015) and repressed by the NADH sensitive Rex (Durand et al., 2017). Repression is released by malate. RoxS helps to restore the NAD+/NADH balance by temporarily turning down part of the TCA cycle. Experimentally confirmed direct RoxS targets are ppnKB mRNA (NAD+/NADH kinase), sucCD mRNA (succinyl-CoA synthase) and yflS (one of four malate transporters; Durand et al., 2015, 2017). RoxS inhibits translation and promotes degradation of ppnKB and sucC mRNAs (Figure 3C). RNase III cleaves the RoxS/ppnKB RNA duplex and RNase Y cleaves ppnK mRNA both RoxS-dependently but also -independently. For translation regulation, one of four CCC rich regions of RoxS (CCR3) suffices. RoxS itself is also subject of RNase Y cleavage at nt + 20 yielding truncated RoxS(Y) which can efficiently regulate ppnKB and is required for translation inhibition of sucCD operon RNA. In contrast to ppnKB and sucCD, yflS is positively regulated: RoxS activates yflS translation by disrupting a structure at the 5′ UTR that impedes ribosome recruitment. In addition, RoxS stabilizes yflS mRNA by preventing 5′-3′ exonucleolytic degradation by RNase J1 (Figure 3C). Both effects are independent and require RoxS region CCR3. Hfq is not involved in RoxS-dependent regulation of ppnKB mRNA (Durand et al., 2015).
RNAC/S1022 was first identified in a microarray screen of B. subtilis intergenic regions, but has so far not been detected in Northern blots. It is transcribed exclusively under control of σD during logarithmic growth in LB medium (Schmalisch et al., 2010). RNAC modulates the cellular level of transition state regulator AbrB via base-pairing with abrB mRNA at the RBS and the first six codons (Mars et al., 2015b; Figure 3D). The Hfq-independent RNAC/abrB RNA interaction seems to promote degradation of abrB mRNA and might also inhibit its translation. However, only minimal alterations (≈33%) of mRNA and protein levels were observed in ΔrnaC strains. RNAC increases the cell-to-cell variation of the AbrB levels, which leads to growth rate heterogeneity of cells within one population during exponential phase. This heterogeneity is physiologically relevant, since slowly growing bacterial cells are less susceptible to antibiotics and environmental stress (Mars et al., 2015b).
The T-box Riboswitch
Another example of regulatory RNA-RNA interactions is the T-box riboswitch, first discovered in the B. subtilis tyrS gene (Henkin et al., 1992). It is an example for transcription attenuation mechanisms that are based on the ability of an mRNA leader region to fold into two mutually exclusive structures, a transcription terminator or an antiterminator, depending on ribosome movement, binding of proteins, an antisense RNA, small ligands or – in the T-box– the loading state of a tRNA (rev. in Brantl, 2004).
The T-box riboswitch is mainly found in Firmicutes to control the expression of amino acid related genes (aa biosynthesis or transport, aminoacyl-tRNA synthetases). Grundy et al. (1994) showed that a UAC to UUC mutation in the tyrS 5′ UTR suffices to induce expression under phenylalanine instead of tyrosine limiting conditions indicating that a tRNA was involved in regulation.
T-box leader RNAs are composed of stems I, II and III and a pseudoknot (Stem IIA/B) present upstream of the competing terminator and antiterminator helices (Figure 5). Stem I harbors an internal loop with the specifier sequence that base-pairs with the tRNA anticodon. The stem I distal region comprises conserved sequence motifs and a terminal loop which interact to form a contact surface with the tRNA D-/T-loops through stacking interactions (Grigg and Ke, 2013; Zhang and Ferré-D’Amaré, 2013). Both an E-loop and a pseudoknot directly downstream of size-variable stem II are required for efficient antitermination. Stem III upstream of the antiterminator varies significantly in sequence and length (rev. in Kreuzer and Henkin, 2018).
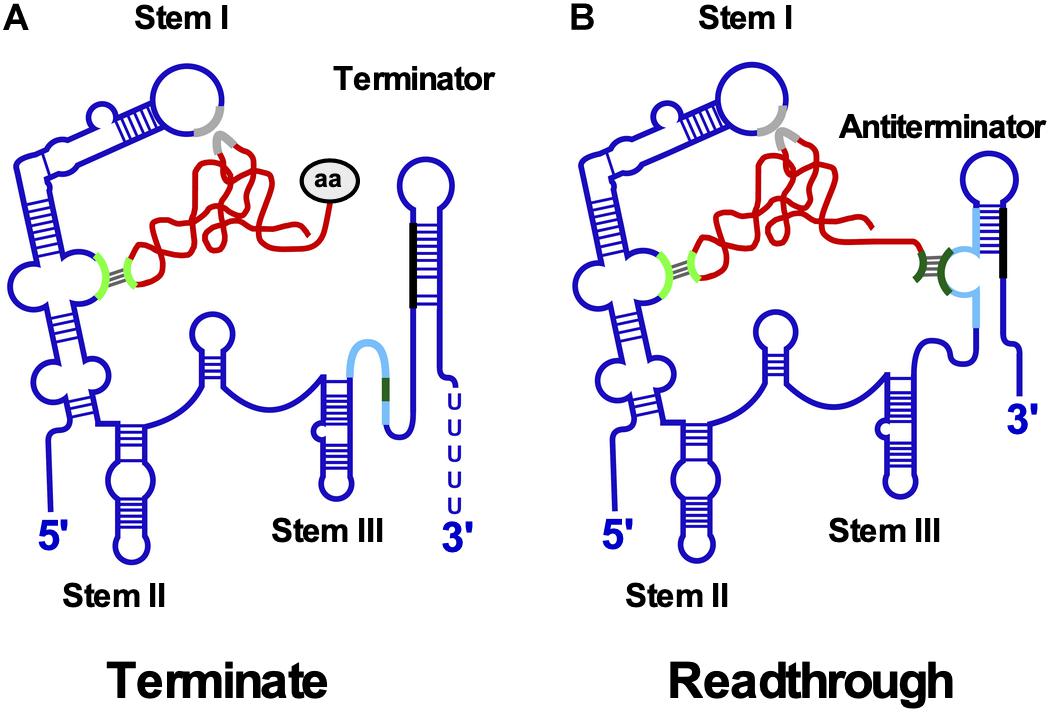
Figure 5. T-box riboswitch. (A) Interaction between T-box leader RNA and tRNA occurs at two positions. Aminoacylated tRNA binds the specifier loop (light green) in stem I through base-pairing and the stem I platform (gray) by stacking interactions. The aa at the 3′ end prevents binding of the acceptor arm to the terminator helix. The more stable terminator helix (black-blue) forms and transcription terminates. (B) Uncharged tRNA interacts with specifier loop and stem I platform. The free acceptor arm of tRNA (dark green) binds the antiterminator (light blue) and stabilizes it allowing transcription to read through the termination site into the downstream gene. Conserved structural domains including stems I-III and the mutually exclusive terminator and antiterminator helices are labeled. The tRNA is shown in red and the aa in gray.
Downstream gene expression depends on the ratio between charged and uncharged tRNAs (Figure 5): Both tRNAs bind to the specifier loop and stem I platform. However, the presence of the charged tRNA aa prevents the interaction of the acceptor end with the antiterminator bulge, facilitating formation of a more stable terminator helix causing premature transcription termination. By contrast, the acceptor arm of uncharged tRNA base-pairs with the antiterminator bulge thereby stabilizing the antiterminator, allowing read-through into the downstream ORF. Binding of uncharged tRNA induces structural changes throughout the leader RNA. An smFRET analysis of B. subtilis glyQS (Zhang et al., 2018) demonstrated a two-step T-box-tRNA interaction comprising the interaction with the anticodon followed by sensing of the 3′ NCCA end. The association rate constant for the first binding step was with 5.0 × 105 M–1 s–1 comparable to that of cis-encoded antisense RNAs (see above).
A cryo-EM structure of the B. subtilis T-box riboswitch-tRNA complex (Li et al., 2019) revealed a 66 nt functional unit – T-box discriminator – that selectively binds uncharged tRNA. It is formed of stem III with flanking purines and the adjacent antiterminator. The T-box adopts a U-shaped molecular vice that clamps the tRNA. The discriminator captures the tRNA 3′ end with nanomolar affinity and uses a steric filter fashioned from a G-U wobble bp to determine its aminoacylation state. When the tRNA is uncharged, the T-box clutches it and forms a continuously stacked central spine allowing transcriptional readthrough.
RNA-Protein Interaction
RNA Chaperones
RNA chaperones are proteins binding to RNAs and affecting their structure or stability and therefore influencing both translation of mRNAs and regulatory functions of sRNAs. B. subtilis encodes two major RNA chaperones: Hfq and CsrA, which are both well investigated in Gram-negative bacteria (rev. in Vakulskas et al., 2015; Kavita et al., 2018) and have a broad impact on gene regulation.
Hfq
As in other Gram-positive bacteria, the role for Hfq in B. subtilis seems to be minor. Only around 150 transcripts could be identified to bind Hfq and even less were affected in their abundance (Dambach and Winkler, 2013; Hämmerle et al., 2014). The structure of B. subtilis Hfq is similar to that of E. coli Hfq, but it prefers binding to AG-repeats instead of ARN-repeats (Someya et al., 2012). So far, no case has been described where B. subtilis Hfq facilitates the interaction between two RNAs, a major task of E. coli and Salmonella Hfq with numerous examples and a pleiotropic phenotype in its absence. In contrast, in a huge screen with more than 2000 growth conditions no growth differences between a wild-type and an isogenic hfq-deficient B. subtilis strain were detected (Rochat et al., 2015). The only known phenotypes in the absence of hfq are a decreased long-term survival of cells in stationary phase, independent of sporulation (Rochat et al., 2015) and a drastically impaired motility and chemotaxis (Jagtap et al., 2016).
Remarkably some toxin mRNAs as well as the corresponding antitoxins of type I TA systems are bound by Hfq (Dambach and Winkler, 2013) and are less abundant in its absence (Hämmerle et al., 2014). However, this had no impact on growth or survival (Rochat et al., 2015). Similarly, Hfq had no effect on the type I TA system bsrE/SR5, although it stabilizes SR5 (Müller et al., 2016). The expression of hfq is upregulated in stationary phase and under several stress conditions (Dambach and Winkler, 2013; Rochat et al., 2015; Jagtap et al., 2016). Together this rather implies a role for Hfq as a general stationary phase fine-tuning regulator than as a globally acting RNA-RNA matchmaker in B. subtilis. Interestingly Hfq binds in vitro near the SD sequence of ahrC mRNA, refolds the 5′ UTR to allow access to the 30S SU and is required for ahrC translation in vivo, but dispensable for its inhibition by SR1 (Heidrich et al., 2007, see above). This confirms that Hfq is a chaperone in B. subtilis, but its role may be restricted to mRNAs.
CsrA
The second RNA chaperone, CsrA, also differs from its E. coli relative. Despite the highly similar general structure of the B. subtilis CsrA dimer (Altegoer et al., 2016) as well as the shared affinity for GGA motifs in single-stranded and looped-out RNA structures, only one major function in motility regulation in B. subtilis was described in detail (Yakhnin et al., 2007): The major flagellin protein Hag, the regulatory protein FliW, CsrA and the hag mRNA form a regulatory circuit to limit the intracellular Hag concentration. At high concentrations, Hag binds FliW, and CsrA is free to block Hag synthesis by hag mRNA binding, otherwise FliW sequesters CsrA and hag is expressed (Mukherjee et al., 2011). Nevertheless, the interaction with FliW does not exclude RNA binding by CsrA in general (Altegoer et al., 2016; Mukherjee et al., 2016), and further functions of CsrA are conceivable.
Only recently, a novel mechanism was discovered, where CsrA refolds the ahrC mRNA to facilitate binding of the regulatory sRNA SR1 (Müller et al., 2019, Figure 3A). This also raises the question of a more global role of CsrA in B. subtilis.
tmRNA
Trans-translation is an omnipresent bacterial mechanism to rescue ribosome stalling on mRNAs without stop-codon, to tag the nascent peptide and to initiate its degradation (rev. in Himeno et al., 2014, 2015). The functional complex comprises the tmRNA (ssrA) and the protein SmpB (ssrB). Together the complex mimics the structure of tRNAAla, is bound by EF-Tu-GTP and can be loaded onto stalled ribosomes. The globular domain of SmpB acts as a substitute of the anticodon-loop and occupies the decoding site, while the C-terminal tail of SmpB probes the vacant mRNA channel of the 30S SU (rev. in Miller and Buskirk, 2014). In contrast to normal tRNAs, the tmRNA has neither an anticodon-loop nor a D-loop. SmpB compensates for these structures and allows recognition by the ribosome and also fine-tunes the tmRNA structure (Miller and Buskirk, 2014). An additional looped out coding strand of tmRNA is used by the ribosome as mRNA template that encodes the species-specific tag sequence, in B. subtilis (A)GKTNSFNQNVALAA. Finally, the ribosome is released and the truncated and tagged protein degraded by Clp proteases that recognize the ALAA-motif (Wiegert and Schumann, 2001; Kolkman and Ferrari, 2004, Figure 6).
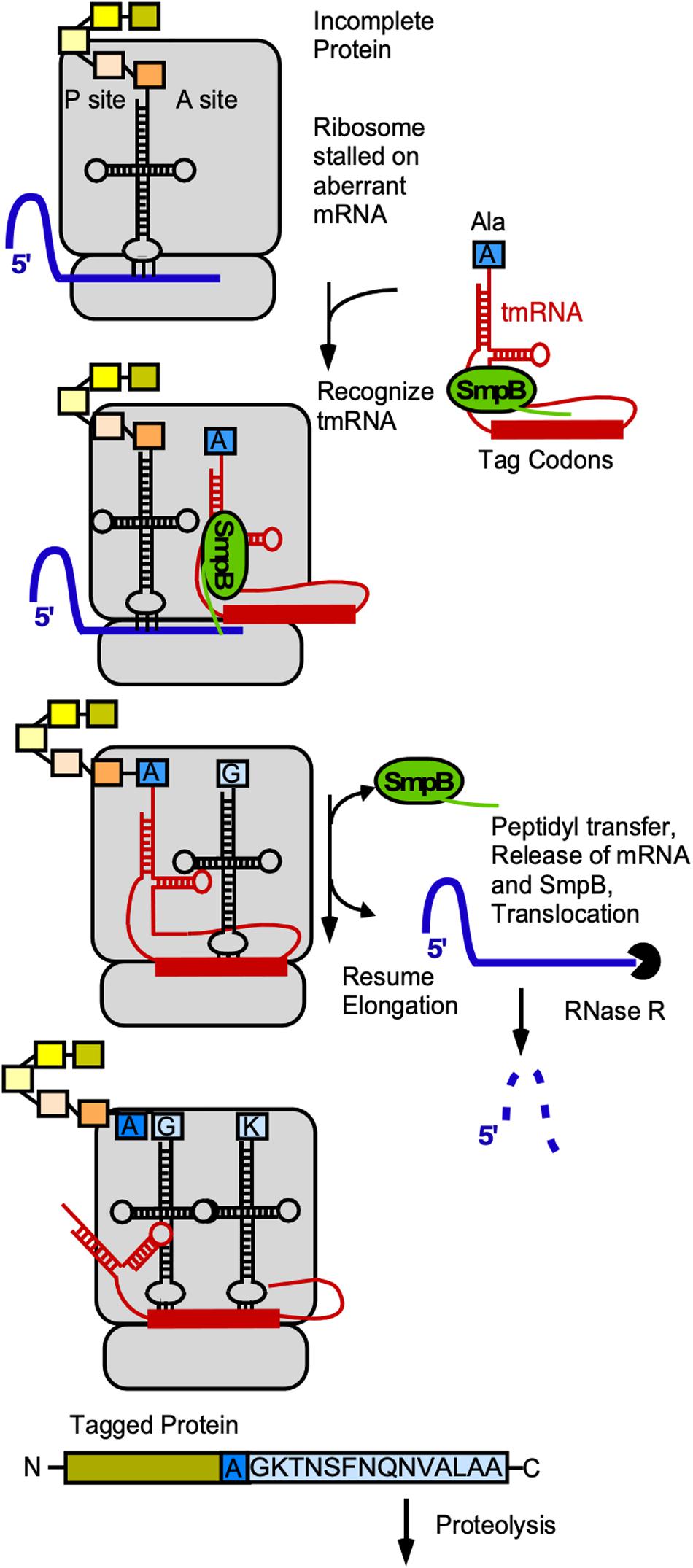
Figure 6. Role of tmRNA and SmpB in trans-translation. Gray, ribosome; blue, mRNA, red, tmRNA; yellow/orange-colored rectangles, aa in peptide chain associated with last tRNA; blue rectangle, alanine with which tRNA was charged by alanyl-tRNA synthetase; light-blue, tag encoded by tmRNA. For details see text.
Trans-translation is important under specific stress conditions to maintain error-free gene expression and rescue stalled ribosomes. In B. subtilis both ssrA and ssrB are important for growth at temperature extremes and under ethanol and heavy metal ion stress (Muto et al., 2000; Shin and Price, 2007). They are encoded in one operon – together with the gene for RNase R that degrades the aberrant mRNA – and upregulated under stress conditions by a σB-dependent promoter (Muto et al., 2000).
Surprisingly, trans-translation is not only involved in the prevention of defective expression under stress conditions, but also part of gene regulation in general (rev. in Keiler, 2015). In B. subtilis, the system is essential in the absence of a second ribosome rescue system, BrfA/PrfB, which uses a distinct mechanism and is directly downregulated by ssrA-smpB-dependent trans-translation (Shimokawa-Chiba et al., 2019). The expression of several genes depends on the tmRNA-SmpB-mechanism (Fujihara et al., 2002). It is also involved in carbon catabolite repression: CcpA binding to cre-sites causes a stall of transcription and subsequently translation, which is finally solved by trans-translation (Ujiie et al., 2008). Furthermore, trans-translation is important for efficient sporulation: In ΔssrA cells, the formation of sigK – coding for a sporulation dependent sigma factor – by a DNA rearrangement between spoIIIC and spoIVCB is impaired, leading to drastically reduced sporulation efficiency (Abe et al., 2008). It is also likely that other physiological processes could be affected by trans-translation in general.
6S RNA
The ≈200 nt long 6S RNA is ubiquitous in bacteria, and B. subtilis has even two 6S RNAs, 6S-1 and 6S-2. All 6S RNAs have a characteristic secondary structure with a central single-stranded loop flanked by two irregular double-stranded stems, which are interrupted by small bulges (Figure 7A). In 2000, it was discovered that 6S RNA forms a stable complex with E. coli RNA polymerase (RNAP) to regulate its activity (Wassarman and Storz, 2000). It interacts with the RNAP β/B’ subunit and σ70, but not with the stationary phase σS. Therefore, it can in stationary phase, when its amount increases to 10.000 molecules/cell, repress transcription at vegetative promoters (Figure 7B). Crystal structure analysis revealed that E. coli 6S RNA mimics B-form DNA (Chen et al., 2017) allowing it to mimic an open promoter thus interfering with the formation of transcription initiation complexes (Figure 7B). 6S RNA acts as template for the synthesis of 14–22 nt pRNAs that are required to relieve 6S-dependent transcription inhibition and thus, recovery of cells from stationary phase in B. subtilis and E. coli (Beckmann et al., 2011; Cavanagh et al., 2012). Both B. subtilis 6S-1 and 6S-2 are in vitro and in vivo templates for pRNA synthesis (Burenina et al., 2014; Hoch et al., 2016). 6S-1 is highest expressed in stationary phase, allows growth adaptation and prevents premature sporulation (Cavanagh and Wassarman, 2013) whereas 6S-2 is more abundant in logarithmic phase, and its function is still unknown. In 2012, the mechanism of action of B. subtilis 6S-1 RNA was elucidated (Beckmann et al., 2012; Figure 7C): As soon as the newly synthesized pRNA has formed a sufficiently stable duplex with 6S-1 RNA, it induces in cis a refolding of the 6S RNA that involves a base-pairing between the 5′ part of the central bulge and nucleotides that are available due to pRNA invasion. This rearrangement decreases the affinity of 6S-1 to the RNAP. Only 12–14 nt long pRNAs, but not shorter ones, are stable enough to induce a refolding. The ratio between the rate constants for polymerization (kpol), pRNA:6S RNA-dissociation (koff) and refolding (kconf) determines whether or not pRNAs dissociate or refold the 6S-1 RNA.
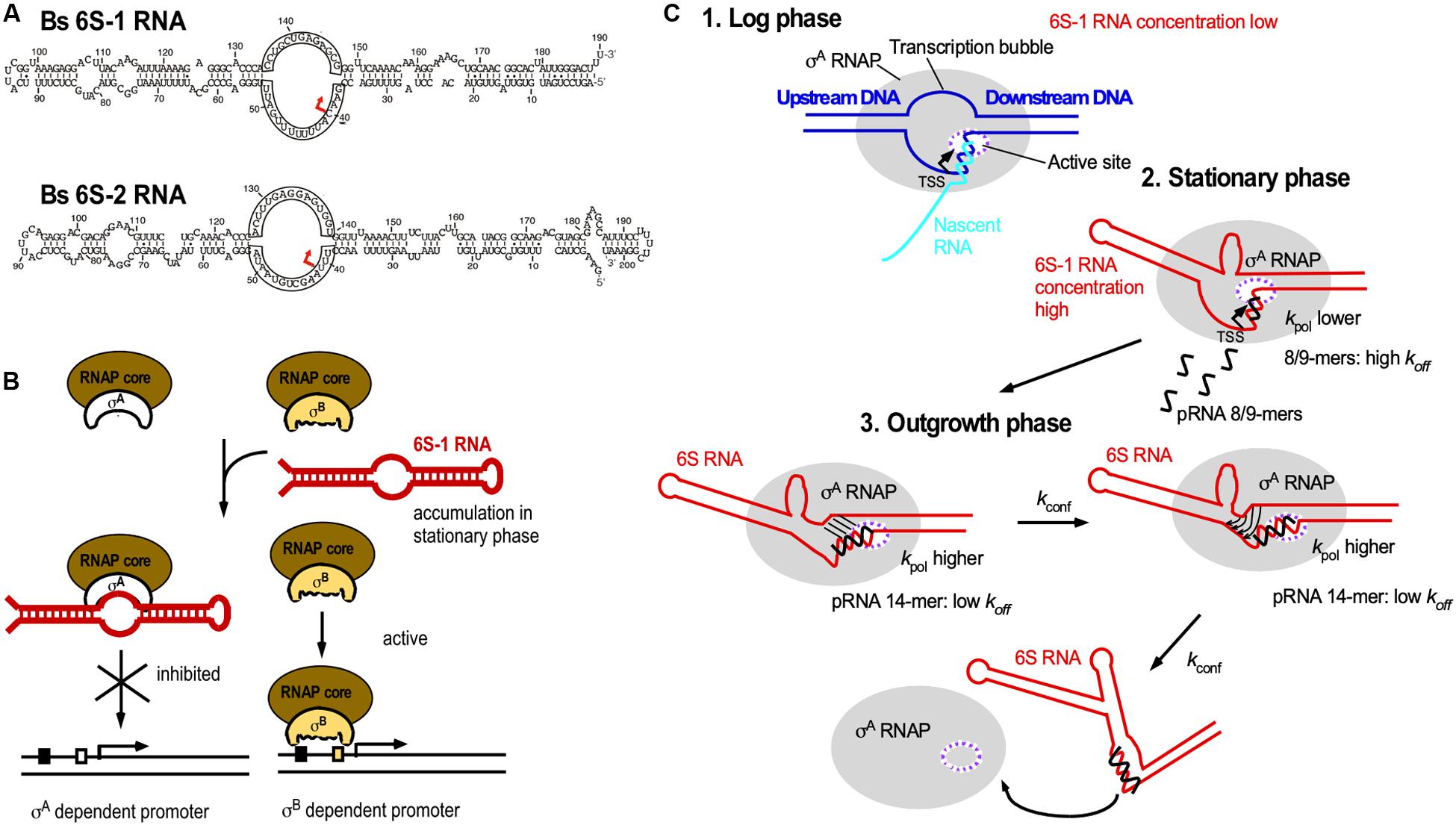
Figure 7. 6S RNA. (A) B. subtilis encodes two 6S RNAs, 6S-1 and 6S-2. Red arrow, TSS for pRNAs. (B) Biological function of 6S RNA. By mimicking an open promoter, 6S-1 RNA, which is abundant in stationary phase, can sequester σA-RNAP thereby preventing transcription initiation at σA-dependent promoters. σB-RNAP is not bound by 6S-1 RNA, thus allowing transcription from σB-dependent promoters. (C) Mechanism of action of pRNAs. Black, pRNA; kpol, rate constant of polymerization (synthesis) of pRNAs; koff, dissociation rate constant of pRNAs; kconf, rate constant for refolding of 6S RNA. The ratio between kpol, koff, and kconf determines whether or not pRNAs dissociate or 6S-1 RNA refolds. For detailed explanations see text. (C) is modified based on Beckmann et al., 2012, with permission.
Future investigations will reveal the function of 6S-2 and show which mechanisms mediate promoter-specific transcription regulation by both 6S RNAs.
RNA Binding Proteins Inducing Terminator or Antiterminator Formation
Examples for transcription attenuation (see above and Figure 5) depending on protein binding involve B. subtilis TRAP (trp RNA binding attenuation protein) and various antitermination proteins.
TRAP (Babitzke and Yanofsky, 1993; Otridge and Gollnick, 1993) is composed of 11 subunits stabilized through 11 intersubunit β-sheets to form a β-wheel with a large central hole of 80 Å diameter (Antson et al., 1999). L-tryptophan binding in clefts between adjacent β-sheets induces conformational changes. The unstructured trp operon 5′ UTR contains 11 U/GAG repeats spaced by 2–3 nt and forms a matching circle around Trp-bound TRAP using mostly specific protein-base interactions (Figure 8A) to cause transcription termination (rev. in Babitzke et al., 2019). When tryptophan is scarce, uncharged trptRNA allows expression of Anti-TRAP that binds TRAP to inhibit its activity. The RNA refolds into an antiterminator and transcription of trpEDCFBA continues (rev. in Babitzke et al., 2019; Figure 8A). Interestingly, TRAP-mediated termination is neither intrinsic nor Rho-dependent. Instead, a region around aa E60 which is not involved in Trp or RNA binding (McAdams et al., 2017) allows TRAP to induce forward translocation of the RNAP, which is required for efficient termination (Potter et al., 2011). Both the trpE and trpG genes are regulated by TRAP exclusively at translational level (rev. in Babitzke et al., 2019).
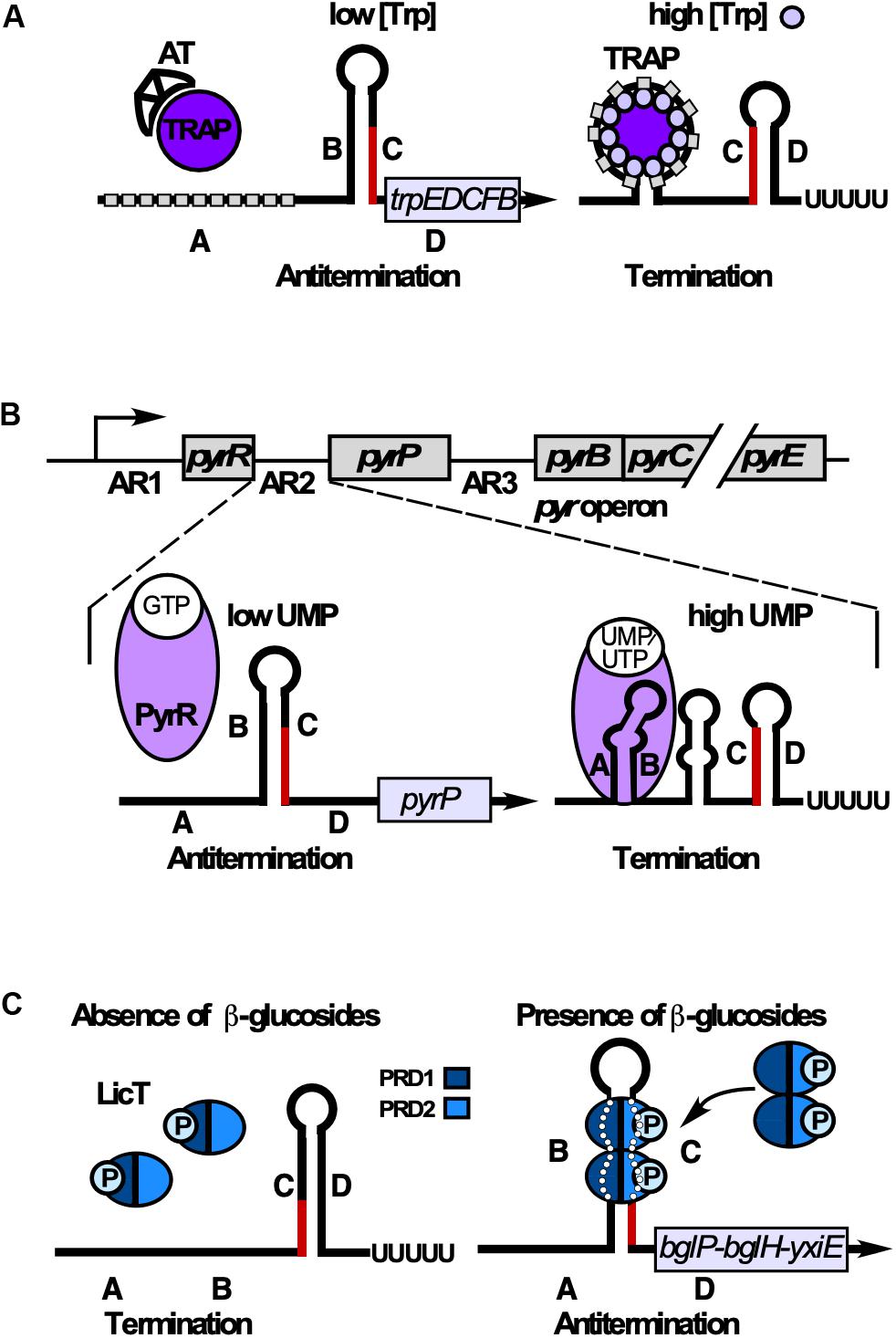
Figure 8. RNA-binding proteins that induce termination or antitermination. Alternative folding occurs by base-pairing between regions B and C (antiterminator) or C and D (terminator). B and C overlap. (A) Control of tryptophan (Trp) biosynthesis. When Trp is scarce, anti-TRAP (AT) is expressed and sequesters TRAP. Regions B and C base-pair preventing terminator formation. At excess Trp, 11-mer TRAP binds Trp enabling each subunit to bind one G(U)AG triplet (gray rectangle) through contacts with aa K37, K56, and R58. This results in RNA wrapping around TRAP in regions A and B. Therefore, B cannot base-pair with C, allowing terminator formation. (B) Top: The 5′ end of the pyr operon mRNA contains 3 attenuation regions (AR1-3) upstream of the pyrR, the pyrP and the pyrB ORF, respectively. Bottom: Control of UMP synthesis. At low UMP/UTP but high GMP levels, PyrR binds GTP and is unable to bind RNA. B and C base-pair preventing formation of the terminator and thus, termination of the pyr operon. At high UMP/UTP levels, PyrR binds UMP or UTP, but not GTP and stabilizes the A-B antianti-terminator, thus preventing of B-C-antiterminator formation. Instead, the terminator stem-loop forms resulting in premature transcription termination. (C) Control of utilization of β-glucans/β-glucosides. In the absence of salicin (β-glucoside), PRD1 is phosphorylated by BglP, causing LicT inactivation (monomers). In the presence of salicin, BglP dephosphorylates PRD1 and transfers the phosphate to incoming salicin, and HPr phosphorylates PRD2. This allows LicT to dimerize and bind/stabilize the otherwise unstable antiterminator.
The pyr operon contains 10 genes encoding all enzymes for UMP de novo synthesis under control of one constitutive promoter. PyrR, one of the two B. subtilis uracil-phosphoribosyltransferases, moonlights as RNA binding attenuation protein in regulation of this operon in three identical attenuation mechanisms (Turner et al., 1994, Figure 8B). An UMP-bound PyrR dimer stabilizes the anti-antiterminators comprising ARUCCAGAGAGGYU to allow formation of downstream terminators (rev. in Turnbough and Switzer, 2008; Turnbough, 2019). PyrR recognizes only conserved RNA sequences that are properly positioned in the correct secondary structure: terminal loop, top of the upper stem and a purine-rich internal bulge are crucial for efficient PyrR binding (Bonner et al., 2001) which involves a basic concave (Savacool and Switzer, 2002). The crystal structure of B. caldolyticus PyrR, which is active in B. subtilis pyr operon regulation (Chander et al., 2005) revealed an unexpected specific GMP binding antagonistic to RNA binding which suggests cross-regulation of the pyr operon by purines. Interestingly, in each of the three attenuation sites, NusA stabilizes in vitro pausing of the RNA polymerase at a major pause site to prevent formation of a complete antiterminator thus promoting formation of a PyrR binding loop (Zhang and Switzer, 2003). This might also play a role in termination of pyr transcription in vivo.
Antitermination proteins GlcT, SacY, SacT, and LicT contain an N-terminal RNA binding domain and regulatory domains PRD1 and PRD2 which are reversibly phosphorylated in response to the cognate carbon source (rev. in Stülke, 2002). In the absence of the carbon source, the cognate EII transporter of the phosphotransferase system phosphorylates PRD1 preventing dimerization. In its presence, EII dephosphorylates PRD1, while HPr phosphorylates PRD2, which allows dimerization and antiterminator binding (Figure 8C).
Crystal structures of inactive LicT revealed a wide swing movement of PRD2 causing a dimer opening that brings the phosphorylation sites to the protein surface (Graille et al., 2005). LicT interacts with two bulges in the antiterminator and the minor groove of the stem between them (Yang et al., 2002, Figure 8C). In GlcT, arrangement of the PRDs is under selective pressure to ensure a proper regulatory output (Himmel et al., 2012). For SacT, SacY, and LicT, specificity domains were identified that prevent a cross-talk between these systems (Hübner et al., 2011).
Glycerol-3P-bound GlpP binds the antiterminator and additionally stabilizes glpD mRNA (rev. in Stülke, 2002). Hexameric HutP (histidine utilization protein) prevents terminator formation in the hut operon. Coordination of L-histidine and Mg2+ activates HutP to bind two clusters of three NAG repeats spaced by 20 nt without undergoing further structural rearrangements (rev. in Kumarevel, 2007).
Aconitase CitB, a Metabolic Enzyme That Moonlights as RNA Binding Protein
Aconitase is an enzyme that operates in the tricarboxylic acid cycle (TCA) of all three kingdoms of life to convert citrate into isocitrate. For this activity, it needs a saturated iron-sulfur (FeS) cluster. Under iron limitation, the FeS cluster disassembles causing enzyme inactivation and, therefore, TCA shutdown. The inactive enzyme adopts an alternative conformation to bind at iron-response elements (IREs) located in the 5′ or 3′ UTR of mRNAs to repress translation or RNA degradation, respectively. The first bacterial aconitase discovered to bind RNA was B. subtilis CitB (Alén and Sonnenshein, 1999): Monomeric CitB binds in vitro at an IRE in the 3′ UTR of qoxD (cytochrome oxidase subunit) and another IRE between feuA and feuB (iron uptake). In addition, it binds at a stem-loop in the 3′ UTR of gerE mRNA encoding a transcription factor in sporulation, which might stabilize the RNA to ensure proper timing of spore coat formation (Serio et al., 2006). However, half-life measurements of qoxD, feuAB or gerE mRNAs have not yet been performed to confirm stabilization of these RNAs by CitB under iron limitation. Binding of CitB to the 5′ UTR of citZ mRNA resulted in destabilization of the three transcripts originating at the citZ promoter which is in line with prevention of translation (Pechter et al., 2013; Figure 9). Surprisingly, CitB bound in vitro both citZ mRNA and the negative control hag mRNA. To date, only the structure of the dual function human iron-regulatory protein 1 in complex with ferritin RNA has been solved (Walden et al., 2006). Under Fe2+ limitation, aconitase adopts a more open conformation with structural domains 3 and 4 extending perpendicularly from the central core composed of domains 1 and 2 (Figure 9). Direct contacts between the exposed IRE loop residues AGU and the domain 2/domain 3 interface provide specificity and stability to the interaction (Walden et al., 2006). Protein binding to the lower stem of the IRE is centered on a – sometimes bulged out – C nucleotide, which inserts into a pocket on the inner face of domain 4. Due to the high conservation of aconitase, similar structural changes most likely occur in B. subtilis CitB as well (Figure 9), although only in the case of feuAB, the IRE loop comprises an AGU motif.
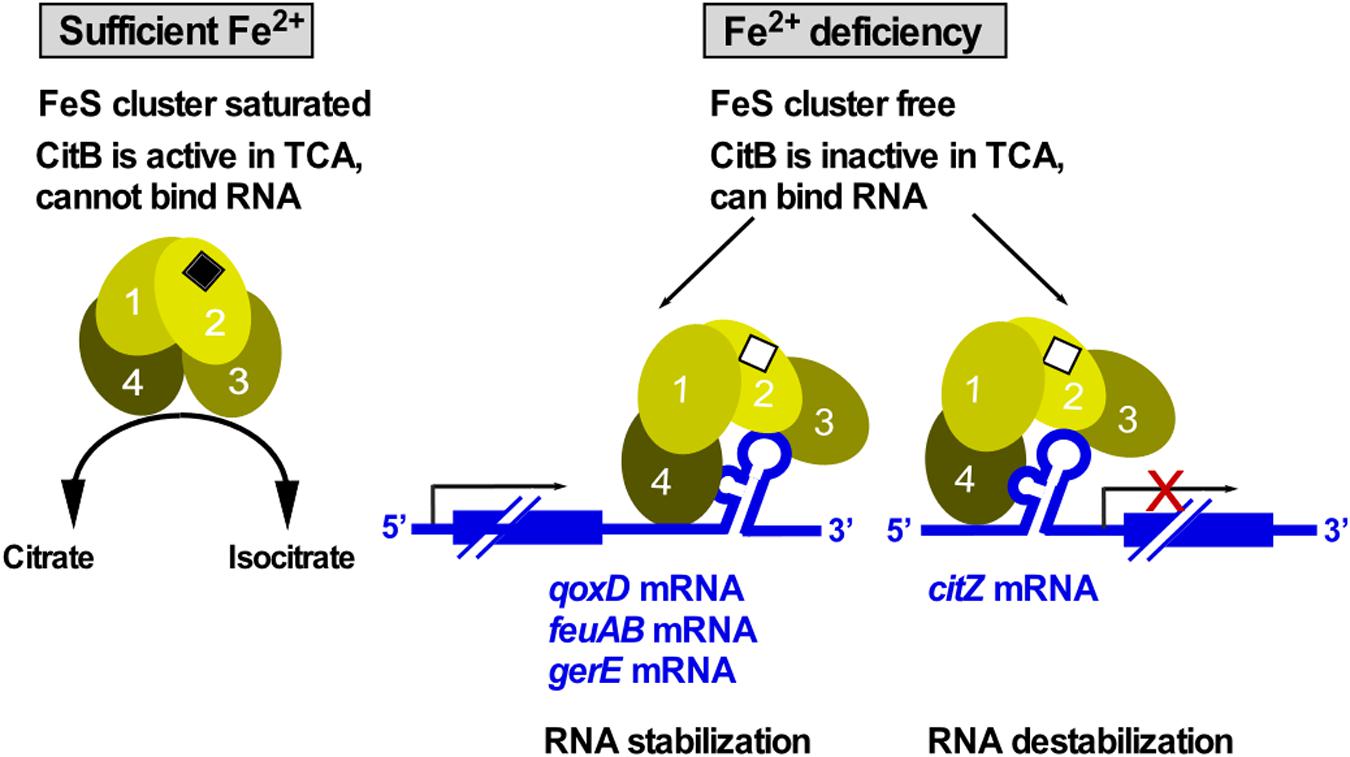
Figure 9. CitB – a dual-function enzyme that moonlights in RNA binding. Under iron-rich conditions, the FeS cluster of CitB is saturated allowing it to function as metabolic enzyme in the TCA. Under iron limitation, the FeS cluster is unsaturated resulting in a conformational change that allows CitB to bind RNA at iron-response elements (IREs) or stem-loops in 3′ UTRs to stabilize the corresponding RNAs or at the 5′ UTR to destabilize the RNA. Depicted conformational changes and domain numbers are based on the crystal structure of the human iron regulatory protein 1 complexed with ferritin IRE-RNA (see text, Walden et al., 2006).
Small Protein-Protein Interactions
Small proteins encoded by small ORFs comprise less than 50 aa and are involved in the regulation of a number of cellular functions including morphogenesis, cell division, enzymatic activities and stress response (rev. in Storz et al., 2014). Until recently, small ORFs had escaped the researchers’ attention as their detection was difficult. With the advent of new technologies, thousands of translated small ORFs have been recently identified in prokaryotes and eukaryotes. However, the identification and characterization of small proteins is still challenging. Here, we review B. subtilis small proteins that interact with larger proteins (Figure 10).
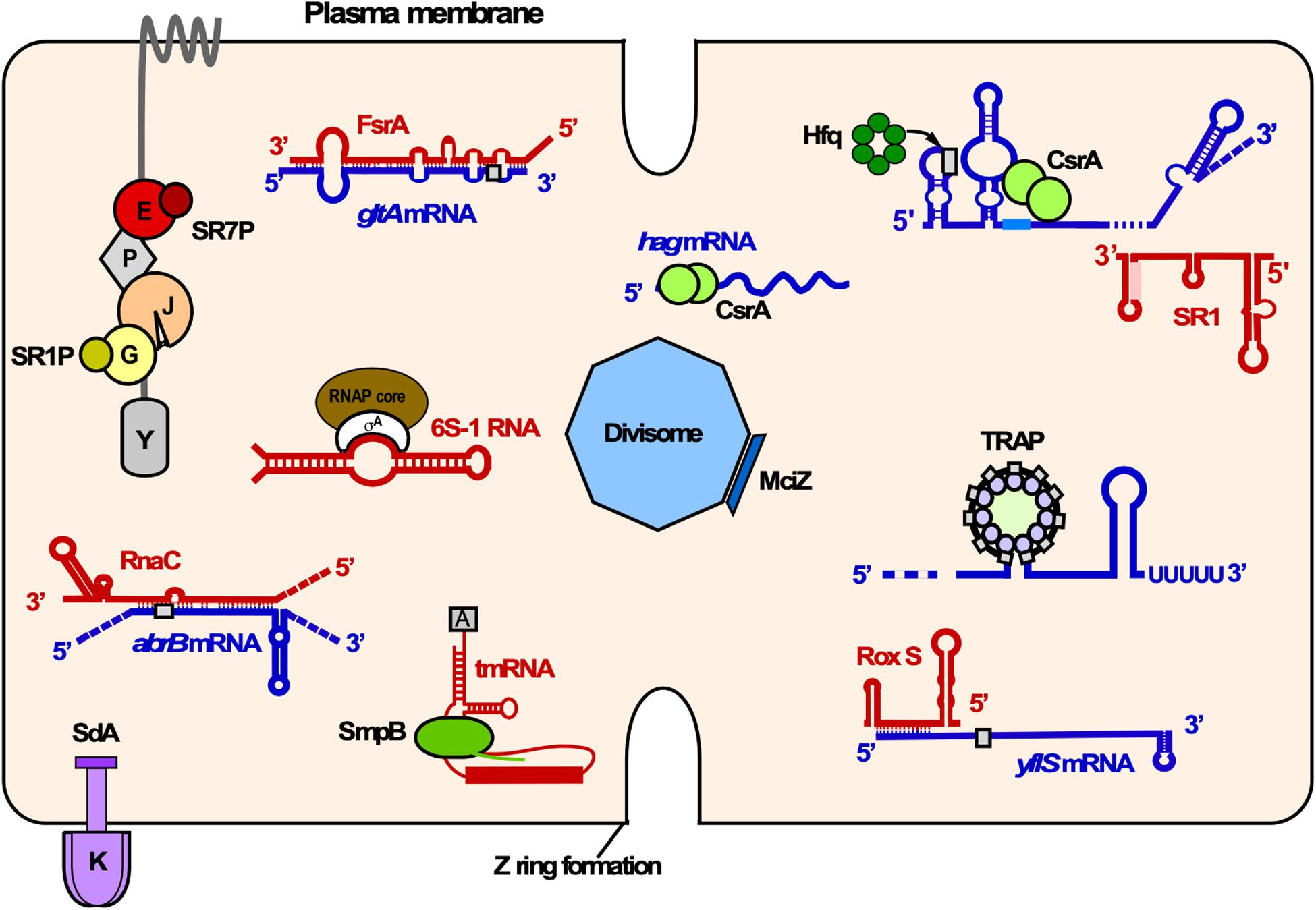
Figure 10. Overview of RNA-RNA, RNA-protein and small protein-protein interactions in B. subtilis. Shown is the cytosol of B. subtilis bounded by the plasma membrane. Proteins associated with various cellular functions are labeled as follows: K, KinA; E, enolase; P, PfkA; J, RNase J1; G, GapA. Small proteins are depicted as rectangles or solid circles. Only components of the degradosome mentioned in the text are illustrated. Red, sRNAs and other regulatory RNAs; blue, mRNAs; gray rectangles, RBS; different shades of green, RNA binding proteins. Other colors, protein-small protein interactions. Y, RNase Y.
Sda Interacts With KinA and Affects Signal Transduction
An example for small proteins localized at the inner membrane that interact with transmembrane sensor kinases is the 46 aa Sda. It binds to and inhibits KinA, the first histidine kinase in the phosphorelay that regulates B. subtilis sporulation. Upon starvation and stress, kinases KinA and KinB autophosphorylate and transfer their phosphates via Spo0F and Spo0B to the central regulator Spo0A. The sda gene was identified in a dnaA1 suppressor mutant which was able to sporulate. Its promoter region contains multiple DnaA binding sites, and mutations in these regions or in dnaA affected sda expression (Burkholder et al., 2001). Structural studies showed that the Sda monomer is composed of two α-helices held together by an interhelix loop forming a helical hairpin (Rowland et al., 2004). The Sda-KinA interaction surface was mapped: Sda uses a hydrophobic surface portion formed by L21 and F25 to interact with the KinA dimerization/phosphotransfer (DHp) domain (Rowland et al., 2004). Several mechanisms for KinA inhibition by Sda were proposed: Sda might prevent KinA autophosphorylation by interrupting the phosphate transfer between the ATP binding site in the catalytic and the DHp domain (Rowland et al., 2004). Sda could also induce conformational changes in the DHp domain which in turn interferes with the KinA-Spo0F-phosphotransfer (Whitten et al., 2007). A later study suggested that Sda directly blocks this phosphotransfer as its binding site on KinA overlaps with that of Spo0F (Cunningham and Burkholder, 2009). KinA regulation through Sda is advantageous for B. subtilis, as it contributes to modulate sporulation initiation at multiple levels in response to unfavorable conditions.
MciZ Regulates Cell Division
Bacterial cytokinesis is initiated when the cell division machinery, the so called divisome, assembles at midcell. The divisome comprises about ten core proteins which help in cell membrane attachment and constriction (Lutkenhaus et al., 2012). Among them is FtsZ, which polymerizes into the Z ring in a GTP-dependent manner and exerts the actual constriction force (Shapiro et al., 2009). A number of small proteins interacting with FtsZ or other divisome components were identified. They also include MciZ (mother cell inhibitor of FtsZ) which was discovered in 2008 in a yeast two-hybrid screening using FtsZ as a bait protein (Handler et al., 2008). MciZ (40 aa) is produced under control of σE during sporulation (Handler et al., 2008). It impedes Z-ring formation by inhibiting FtsZ polymerization under moderate to low concentration. The crystal structure of the FtsZ-MciZ complex revealed a binding pocket for MciZ on one of the polymerization surfaces at the FtsZ C-terminus. MciZ binding inhibits FtsZ polymerization by steric hindrance (Bisson-Filho et al., 2015). Whereas the N-terminus of free MciZ is unstructured, the interaction with FtsZ induces the formation of two β-sheets (Bisson-Filho et al., 2015). At high concentration, MciZ sequesters FtsZ, while at sub-stoichiometric concentration, it acts by filament capping. Moreover, it can also cause filamentation in vitro. A recent study showed that MciZ can affect B. subtilis sporulation: Excessive amounts of MciZ produced intracellularly or added exogenously can not only decrease spore formation efficiency but also inhibit spore germination (Araújo-Bazán et al., 2019).
Two Small Proteins Modulate the Degradosome
The proposed B. subtilis degradosome is composed of major endoribonuclease RNase Y, RNases J1 and J2, PNPase, helicase CshA, the glycolytic enzymes enolase and phosphofructokinase and under certain circumstances, GapA (Commichau et al., 2009; Gimpel and Brantl, 2016). So far, two small proteins – SR1P and SR7P – have been identified which interact with degradosome components.
SR1P is a 39 aa protein encoded by the dual-function sRNA SR1 (see above). It interacts with GapA, one of the two glyceraldehyde-3P-dehydrogenases (GAPDHs) in B. subtilis. SR1P stabilizes gapA mRNA by preventing its rapid degradation under gluconeogenesis (Gimpel et al., 2010). In addition to its role in glycolysis, GapA has a moonlighting property: It binds both RNases J1 and Y. SR1P promotes GapA binding to RNase J1 and enhances RNase J1 activity in vitro on at least two substrates, SR5 and threonyl-tRNA. In addition, it affects SR5 stability in vivo (Gimpel and Brantl, 2016). This means, SR1P modifies the moonlighting activity of GapA. Using peptide mutants in co-elution experiments complemented by SR1P functionality tests in Northern blotting, the SR1P-GapA interaction surface was determined. SR1P attaches to GapA using aa contacts in a binding pocket formed by the C-terminal GapA helix 14. In addition, SR1P contacts the N-terminal GapA helix 1 (Gimpel et al., 2017). Interestingly, SR1 is transcribed under gluconeogenic conditions, when GapB is active (Fillinger et al., 2000) and the glycolytic activity of GapA is not needed. Newman et al. proposed that RNA degradation is connected to the metabolic state of the cell (Newman et al., 2012). Similarly, it was hypothesized that under nutrient limitation, glycolytic enzymes like enolase, phosphofructokinase that are part of the B. subtilis degradosome as well as GapA might sense nutritional stress and transfer this signal to the RNA degradation machinery (Gimpel and Brantl, 2016, 2017). This could modulate the global RNA turnover rate to conserve energy for other important cellular functions.
SR7P (39 aa) previously known as S1136 (Mars et al., 2015a) is encoded by the dual-function antisense RNA SR7 (Ul Haq et al., 2020). The sr7 gene is located in the intergenic region between tyrS and rpsD and controlled by a σB-dependent promoter. SR7P is synthesized under five different stress conditions from the σB-dependent SR7 as well as constitutively from a tyrS mRNA processing product. SR7P interacts with enolase present in the degradosome. This interaction in turn improves enolase binding to RNase Y. The SR7P-Eno-RNase Y interaction is not bridged by RNA. The activity of RNase Y is significantly higher in the tri-component SR7P/Eno/RNase Y complex than in the Eno/RNase Y complex alone. This modulating effect of SR7P was demonstrated both in vivo and in vitro. In addition, SR7P impacts cell survival under selective stress conditions suggesting that it might play a specific role in stress response.
Conclusion and Perspectives
Although a variety of intermolecular interactions have been investigated in B. subtilis, major cavities in our knowledge still exist. Only for four of 108 trans-encoded sRNAs and for 8 small proteins, interaction partners have been identified and biological functions elucidated. Furthermore, a global approach is required to ascertain if CsrA plays a similar role in B. subtilis as Hfq and ProQ in Gram-negatives. The function of the putative RNA chaperones FbpA, FbpB and FbpC has to be unraveled. Targets of the multitude of sRNAs have to be identified and their – perhaps sometimes novel – mechanisms of action elucidated. A considerable increase in the number of dual-function sRNAs can be anticipated. sRNAs might be found that bind enzymes or act directly on the genome like some eukaryotic siRNAs. Structures of more antiterminator/protein complexes and CitB have to be solved and the function of 6S-2 RNA uncovered. The further study of small proteins which is still in its infancy, will allow insights into regulatory networks comprising not only small proteins modulating large proteins or the membrane but also those binding small ligands.
Author Contributions
SB wrote the sections on RNA-RNA interactions, 6S RNA, terminators/antiterminators, CitB., and designed Figures 1–4, 6–9. IU wrote the sections on T-box riboswitch, small protein-protein interactions, and designed Figure 5. PM wrote the sections on RNA chaperones and tmRNA. SB and IU designed Figure 10. All authors approved the final version of the manuscript.
Funding
Work on this topic was supported by the grants BR1552/6-1 to 6-3, 7-1, 7-2, 8-1, 10-1, and 11-1 from the Deutsche Forschungsgemeinschaft (to SB).
Conflict of Interest
The authors declare that the research was conducted in the absence of any commercial or financial relationships that could be construed as a potential conflict of interest.
Acknowledgments
We thank all former coworkers of the AG Bakteriengenetik for their substantial experimental contributions. Furthermore, we apologize for not having mentioned all valuable contributions from colleagues in the field due to space limitations.
Abbreviations
aa, amino acid; bp, base pair; MW, molecular weight; nt, nucleotide; RBS, ribosome binding site; sRNA, small RNA; SU, subunit; TA, toxin-antitoxin; TSS, transcription start site.
References
Abe, T., Sakaki, K., Fujihara, A., Ujiie, H., Ushida, C., Himeno, H., et al. (2008). tmRNA-dependent trans-translation is required for sporulation in Bacillus subtilis. Mol. Microbiol. 69, 1491–1498. doi: 10.1111/j.1365-2958.2008.06381.x
Alén, C., and Sonnenshein, A. L. (1999). Bacillus subtilis aconitase is an RNA-binding protein. Proc. Natl. Acad. Sci. U.S.A. 96, 10412–10417. doi: 10.1073/pnas.96.18.10412
Altegoer, F., Rensing, S. A., and Bange, G. (2016). Structural basis for the CsrA-dependent modulation of translation initiation by an ancient regulatory protein. Proc. Natl. Acad. Sci. U.S.A. 113, 10168–10173. doi: 10.1073/pnas.1602425113
Antson, A. A., Dodson, E. J., Dodson, G., Greaves, R. B., Chen, X., and Gollnick, P. (1999). Structure of the trp RNA-binding attenuation protein, TRAP, bound to RNA. Nature 401, 235–242. doi: 10.1038/45730
Araújo-Bazán, L., Huecas, S., Valle, J., Andreu, D., and Andreu, J. M. (2019). Synthetic developmental regulator MciZ targets FtsZ across Bacillus species and inhibits bacterial division. Mol. Microbiol. 111, 965–980. doi: 10.1111/mmi.14198
Argaman, L., Hershberg, R., Vogel, J., Bejerano, G., Wagner, E. G., Margalit, H., et al. (2001). Novel small RNA-encoding genes in the intergenic regions of Escherichia coli. Curr. Biol. 11, 941–950. doi: 10.1016/s0960-9822(01)00270-6
Babitzke, P., Lai, Y. J., Renda, A. J., and Romeo, T. (2019). Posttranscription initiation control of gene mediated by bacterial RNA-binding proteins. Annu. Rev. Microbiol. 73, 43–67. doi: 10.1146/annurev-micro-020518-115907
Babitzke, P., and Yanofsky, C. (1993). Reconstitution of Bacillus subtilis trp attenuation in vitro with TRAP, the trp RNA-binding attenuation protein. Proc. Natl. Acad. Sci. U.S.A. 90, 133–137. doi: 10.1073/pnas.90.1.133
Beckmann, B. M., Burenina, O. Y., Hoch, P. G., Kubareva, E. A., Sharma, C. M., and Hartmann, R. K. (2011). In vivo and in vitro analysis of 6S RNA-templated short transcripts in Bacillus subtilis. RNA Biol. 8, 839–849. doi: 10.4161/rna.8.5.16151
Beckmann, B. M., Philipp, G. H., Marz, M., Willkomm, D. K., Salas, M., and Hartmann, R. K. (2012). pRNA-induced structural rearrangements triggers 6S-1 RNA release from RNA polymerase in Bacillus subtilis. EMBO J. 31, 1727–1738. doi: 10.1038/emboj.2012.23
Bisson-Filho, A. W., Discola, K. F., Castellen, P., Blasios, V., Martins, A., Sforça, M. L., et al. (2015). FtsZ filament capping by MciZ, a developmental regulator of bacterial division. Proc. Natl. Acad. Sci. U.S.A. 12, 2130–2138.
Bonner, E. R., D’Elia, J. N., Billips, B. K., and Switzer, R. L. (2001). Molecular recognition of pyr mRNA by the Bacillus subtilis attenuation regulatory protein PyrR. Nucleic Acids Res. 29, 4851–4165. doi: 10.1093/nar/29.23.4851
Brantl, S. (2004). Bacterial gene regulation: from transcription attenuation to riboswitches and ribozymes. Trends Microbiol. 12, 473–475. doi: 10.1016/j.tim.2004.09.008
Brantl, S. (2012). Bacterial type I toxin-antitoxin systems. RNA Biol. 9, 1488–1490. doi: 10.4161/rna.23045
Brantl, S. (2012). Acting antisense: plasmid- and chromosome-encoded sRNAs from Gram-positive bacteria. Future Microbiol. 7, 853–871. doi: 10.2217/fmb.12.59
Brantl, S., and Brückner, R. (2014). Small regulatory RNAs from low-GC Gram-positive bacteria. RNA Biol. 11, 443–456. doi: 10.4161/rna.28036
Brantl, S., and Jahn, N. (2015). sRNAs in bacterial type I and type III toxin/antitoxin systems. FEMS Microbiol. Rev. 39, 413–427. doi: 10.1093/femsre/fuv003
Brantl, S., and Müller, P. (2019). Toxin antitoxin systems in Bacillus subtilis. Toxins (Basel) 11:E262.
Burenina, O. Y., Hoch, P. G., Damm, K., Salas, M., Zatsepin, T. S., Lechner, M., et al. (2014). Mechanistic comparison of Bacillus subtilis 6S-1 and 6S-2 RNAs – commonalities and differences. RNA 20, 348–359. doi: 10.1261/rna.042077.113
Burkholder, W. F., Kurtser, I., and Grossman, A. D. (2001). Replication initiation proteins regulate a developmental checkpoint in Bacillus subtilis. Cell 104, 269–279. doi: 10.1016/s0092-8674(01)00211-2
Cavanagh, A. T., Sperger, J. M., and Wassarman, K. M. (2012). Regulation of 6S RNA by pRNA synthesis is required for efficient recovery from stationary phase in E. coli and B. subtilis. Nucleic Acids Res. 40, 2234–2246. doi: 10.1093/nar/gkr1003
Cavanagh, A. T., and Wassarman, K. M. (2013). 6S-1 RNA function leads to a delay in sporulation in Bacillus subtilis. J. Bacteriol. 195, 2079–2086. doi: 10.1128/jb.00050-13
Chander, P., Halbig, K. M., Miller, J. K., Fields, C. J., Bonner, H. K. S., Grabner, G. K., et al. (2005). Structure of the nucleotide complex of PyrR, the Pyr attenuation protein from Bacillus caldolyticus, suggests dual regulation by pyrimidine and purine nucleotides. J. Bacteriol. 187, 1773–1782. doi: 10.1128/jb.187.5.1773-1782.2005
Chen, J., Wassarman, K. M., Feng, S., Leon, K., Feklistov, A., Winkelman, J. T., et al. (2017). 6S RNA mimics B-form DNA to regulate Escherichia coli RNA polymerase. Mol. Cell 68, 388–397.
Commichau, F. M., Rothe, F. M., Herzberg, C., Wagner, E., Hellwig, D., Lehnik-Habrink, M., et al. (2009). Novel activities of glycolytic enzymes in Bacillus subtilis: interactions with essential proteins involved in mRNA processing. Mol. Cell Proteomics. 8, 1350–1360. doi: 10.1074/mcp.m800546-mcp200
Cunningham, K. A., and Burkholder, W. F. (2009). The histidine kinase inhibitor Sda binds near the site of autophosphorylation and may sterically hinder autophosphorylation and phosphotransfer to Spo0F. Mol. Microbiol. 71, 659–677. doi: 10.1111/j.1365-2958.2008.06554.x
Czaplewski, L. G., North, A. I., Smith, M. C., Baumberg, S., and Stockley, P. G. (1992). Purification and initial characterization of AhrC: the regulator of arginine metabolism genes in Bacillus subtilis. Mol. Microbiol. 6, 267–275. doi: 10.1111/j.1365-2958.1992.tb02008.x
Dambach, M. C., and Winkler, W. C. (2013). Association of RNAs with B. subtilis Hfq. PLoS One 8:e55156. doi: 10.1371/journal.pone.0055156
Durand, S., Braun, F., Helfer, A. C., Romby, P., and Condon, C. (2017). sRNA-mediated activation of gene expression by inhibiton of 5’-3’ exonulceolytic mRNA degradation. Elife 17:e23602.
Durand, S., Braun, F., Lioliou, E., Romilly, C., Helfer, A. C., Kuhn, L., et al. (2015). A nitric oxide regulated small RNA controls expression of genes involved in redox homeostasis in Bacillus subtilis. PLoS Genet. 11:e1004957. doi: 10.1371/journal.pgen.1004957
Durand, S., Gilet, L., and Condon, C. (2012a). The essential function of B. subtilis RNase III is to silence foreign toxin genes. PLoS Genet. 8:e1003181. doi: 10.1371/journal.pgen.1003181
Durand, S., Jahn, N., Condon, C., and Brantl, S. (2012b). Type I toxin-antitoxin systems in Bacillus subtilis. RNA Biol. 9, 1491–1497. doi: 10.4161/rna.22358
Eiumphungporn, W., and Helmann, J. D. (2009). Extracytoplasmic function sigma factors regulate expression of the Bacillus subtilis yabE gene via a cis-acting antisense RNA. J. Bacteriol. 191, 1101–1105. doi: 10.1128/jb.01530-08
Fillinger, S., Boschi-Muller, S., Azza, S., Dervyn, E., Branlant, G., and Aymerich, S. (2000). Two glyceraldehyde-3-phosphate dehydrogenases with opposite physiological roles in a nonphotosynthetic bacterium. J. Biol. Chem. 275, 14031–14037. doi: 10.1074/jbc.275.19.14031
Fujihara, A., Tomatsu, H., Inagaki, S., Tadaki, T., Ushida, C., Himeno, H., et al. (2002). Detection of tmRNA-mediated trans-translation products in Bacillus subtilis. Genes Cells 7, 343–350. doi: 10.1046/j.1365-2443.2002.00523.x
Gaballa, A., Antelmann, H., Aguilar, C., Khakh, S. K., Song, K. B., Smaldone, G. T., et al. (2008). The Bacillus subtilis iron-sparing response is mediated by a Fur-regulated small RNA and three small, basic proteins. Proc. Natl. Acad. Sci. U.S.A. 105, 11927–11932. doi: 10.1073/pnas.0711752105
Gimpel, M., and Brantl, S. (2016). Dual-function sRNA encoded peptide SR1P modulates moonlighting activity of B. subtilis GapA. RNA Biol. 13, 916–926. doi: 10.1080/15476286.2016.1208894
Gimpel, M., and Brantl, S. (2017). Dual-function small regulatory RNAs in bacteria. Mol. Microbiol. 103, 387–397. doi: 10.1111/mmi.13558
Gimpel, M., Heidrich, N., Mäder, U., Krügel, H., and Brantl, S. (2010). A dual-function sRNA from Bacillus subtilis: SR1 acts as a peptide-encoding mRNA on the gapA operon. Mol. Microbiol. 76, 990–1009. doi: 10.1111/j.1365-2958.2010.07158.x
Gimpel, M., Maiwald, C., Wiedemann, C., Görlach, M., and Brantl, S. (2017). Characterization of the interaction between the small RNA-encoded peptide SR1P and GapA from Bacillus subtilis. Microbiology 163, 1248–1259. doi: 10.1099/mic.0.000505
Gimpel, M., Preis, H., Barth, E., Gramzow, L., and Brantl, S. (2012). SR1 – a small RNA with two remarkably conserved functions. Nucleic Acids Res. 40, 11659–11672. doi: 10.1093/nar/gks895
Graille, M., Zhou, C. Z., Brechot, V. R., Collinet, B., Declerck, N., and van Tilbeurgh, H. (2005). Activation of the LicT transcriptional antiterminator involves a domain swing/lock mechanism provoking massive structural changes. J. Biol. Chem. 280, 14780–14789. doi: 10.1074/jbc.m414642200
Grigg, J. C., and Ke, A. (2013). Structural determinants for geometry and information decoding of tRNA by T box leader RNA. Cell 21, 2025–2032. doi: 10.1016/j.str.2013.09.001
Grundy, F. J., Rollins, S. M., and Henkin, T. M. (1994). Interaction between the acceptor end of tRNA and the T box stimulates antitermination in the Bacillus subtilis tyrS gene: a new role for the discriminator base. J. Bacteriol. 176, 4518–4526. doi: 10.1128/jb.176.15.4518-4526.1994
Hämmerle, H., Amman, F., Veèerek, B., Stülke, J., Hofacker, I., and Bläsi, U. (2014). Impact of Hfq on the Bacillus subtilis transcriptome. PLoS One 9:e98661. doi: 10.1371/journal.pone.0098661
Handler, A. A., Lim, J. E., and Losick, R. (2008). Peptide inhibitor of cytokinesis during sporulation in Bacillus subtilis. Mol. Microbiol. 68, 588–599. doi: 10.1111/j.1365-2958.2008.06173.x
Heidrich, N., and Brantl, S. (2003). Antisense-RNA mediated transcriptional attenuation: importance of a U-turn loop structure in the target RNA of plasmid pIP501 for inhibition by the antisense RNA. J. Mol. Biol. 153, 420–427. doi: 10.1099/mic.0.2006/002329-0
Heidrich, N., Chinali, A., Gerth, U., and Brantl, S. (2006). The small untranslated RNA SR1 from the B. subtilis genome is involved in the regulation of arginine catabolism. Mol. Microbiol. 62, 520–536. doi: 10.1111/j.1365-2958.2006.05384.x
Heidrich, N., Moll, I., and Brantl, S. (2007). In vitro analysis of the interaction between the small RNA SR1 and its primary target ahrC mRNA. Nucleic Acids Res. 35, 4331–4346. doi: 10.1093/nar/gkm439
Henkin, T. M., Glass, B. L., and Grundy, F. J. (1992). Analysis of the Bacillus subtilis tyrS gene: conservation of a regulatory sequence in multiple tRNA synthetase genes. J. Bacteriol. 174, 1299–1306. doi: 10.1128/jb.174.4.1299-1306.1992
Himeno, H., Kurita, D., and Muto, A. (2014). tmRNA-mediated trans-translation as the major ribosome rescue system in a bacterial cell. Front. Genet. 5:66.
Himeno, H., Nameki, N., Kurita, D., Muto, A., and Abo, T. (2015). Ribosome rescue systems in bacteria. Biochimie 114, 102–112. doi: 10.1016/j.biochi.2014.11.014
Himmel, S., Zschiedrich, C. P., Becker, S., Hsiao, H. H., Wolff, S., Diethmaier, C., et al. (2012). Determinants of interaction specificity of the Bacillus subtilis GlcT antitermination protein: functionality and phosphorylation specificity depend on the arrangement of the regulatory domains. J. Biol. Chem. 287, 27731–27742. doi: 10.1074/jbc.m112.388850
Hoch, P. G., Schlereth, J., Lechner, M., and Hartmann, R. K. (2016). Bacillus subtilis 6S-2 RNA serves as a template for short transcripts in vivo. RNA 22, 614–622. doi: 10.1261/rna.055616.115
Hübner, S., Declerck, N., Diethmaier, C., Le Coq, D., Aymerich, S., and Stülke, J. (2011). Prevention of cross-talk in conserved regulatory systems: identification of specificity determinants in RNA-binding anti-termination proteins of the BglG family. Nucleic Acids Res. 39, 4360–4372. doi: 10.1093/nar/gkr021
Irnov, I., Sharma, C. M., Vogel, J., and Winkler, W. C. (2010). Identification of regulatory RNAs in Bacillus subtilis. Nucleic Acids Res. 38, 6637–6651. doi: 10.1093/nar/gkq454
Jagtap, C. B., Kumar, P., and Rao, K. K. (2016). Bacillus subtilis Hfq: a role in chemotaxis and motility. J. Biosci. 41, 347–358. doi: 10.1007/s12038-016-9618-9
Jahn, N., and Brantl, S. (2013). One antitoxin – two functions: SR4 controls toxin mRNA decay and translation. Nucleic Acids Res. 41, 9870–9880. doi: 10.1093/nar/gkt735
Jahn, N., and Brantl, S. (2016). Heat shock induced refolding entails rapid degradation of bsrG toxin mRNA by RNases Y and J1. Microbiology 162, 590–599. doi: 10.1099/mic.0.000247
Jahn, N., Brantl, S., and Strahl, H. (2015). Against the mainstream: the membrane associated type I toxin BsrG from Bacillus subtilis interferes with cell envelope biosynthesis without increasing membrane permeability. Mol. Microbiol. 98, 651–666. doi: 10.1111/mmi.13146
Jahn, N., Preis, H., Wiedemann, C., and Brantl, S. (2012). BsrG/SR4 from Bacillus subtilis – the first temperature-dependent type I toxin-antitoxin system. Mol. Microbiol. 83, 579–598. doi: 10.1111/j.1365-2958.2011.07952.x
Kavita, K., de Mets, F., and Gottesman, S. (2018). New aspects of RNA-based regulation by Hfq and its partner sRNAs. Curr. Opin. Microbiol. 42, 53–61. doi: 10.1016/j.mib.2017.10.014
Kolkman, M. A. B., and Ferrari, E. (2004). The fate of extracellular proteins tagged by the SsrA system of Bacillus subtilis. Microbiology 150, 427–436. doi: 10.1099/mic.0.26388-0
Kreuzer, K. D., and Henkin, T. M. (2018). The T-Box riboswitch: tRNA as an effector to modulate gene regulation. Microbiol. Spectr. 6:1128.
Kumarevel, T. (2007). Structural insights of HutP-mediated regulation of transcription of the hut operon in Bacillus subtilis. Biophys. Chem. 128, 1–12. doi: 10.1016/j.bpc.2007.03.003
Li, S., Su, Z., Lehmann, J., Stamatopoulou, V., Giarimoglou, N., Henderson, F. E., et al. (2019). Structural basis of amino acid surveillance by higher-order tRNA-mRNA interactions. Nat. Struct. Mol. Biol. 26, 1094–1105. doi: 10.1038/s41594-019-0326-7
Licht, A., and Brantl, S. (2009). The transcriptional repressor CcpN from B. subtilis uses different repression mechanisms at different promoters. J. Biol. Chem. 284, 30032–30038. doi: 10.1074/jbc.m109.033076
Licht, A., Golbik, R., and Brantl, S. (2008). Identification of ligands affecting the activity of the transcriptional repressor CcpN from Bacillus subitlis. J. Mol. Biol. 380, 17–30. doi: 10.1016/j.jmb.2008.05.002
Licht, A., Preis, S., and Brantl, S. (2005). Implication of CcpN in the regulation of a novel untranslated RNA (SR1) in B. subtilis. Mol. Microbiol. 58, 189–206. doi: 10.1111/j.1365-2958.2005.04810.x
Luo, Y., and Helmann, J. D. (2012). A sigma D-dependent antisense transcript modulates expression of the cyclic-di-AMP hydrolase GdpP in Bacillus subtilis. Microbiology 158, 2732–2741. doi: 10.1099/mic.0.062174-0
Lutkenhaus, J., Pichoff, S., and Du, S. (2012). Bacterial cytokinesis: from Z ring to divisome. Cytoskeleton 69, 778–790. doi: 10.1002/cm.21054
Mars, R. A., Mendonça, K., Denham, E. L., and van Dijl, J. M. (2015a). The reduction in small ribosomal subunit abundance in ethanol-stressed cells of Bacillus subtilis is mediated by a SigB-dependent antisense RNA. Biochim. Biophys. Acta 1853, 2553–2559. doi: 10.1016/j.bbamcr.2015.06.009
Mars, R. A., Nicolas, P., Ciccolini, M., Reilman, E., Reder, A., Schaffer, M., et al. (2015b). Small regulatory RNA-induced growth rate heterogeneity of Bacillus subtilis. PLoS Genet. 11:e1005046. doi: 10.1371/journal.pgen.1005046
McAdams, N. M., Patterson, A., and Gollnick, P. (2017). Identification of a residue (Glu60) in TRAP required for inducing efficient transcription termination at the trp attenuator independent of binding tryptophan and RNA. J. Bacteriol. 199, e710–e716.
Meißner, C., Jahn, N., and Brantl, S. (2015). In vitro characterization of the type I toxin-antitoxin system bsrE/SR5 from Bacillus subtilis. J. Biol. Chem. 291, 560–571. doi: 10.1074/jbc.m115.697524
Miller, M. R., and Buskirk, A. R. (2014). The SmpB C-terminal tail helps tmRNA to recognize and enter stalled ribosomes. Front. Microbiol. 5:462.
Mukherjee, S., Oshiro, R. T., Yakhnin, H., Babitzke, P., and Kearns, D. B. (2016). FliW antagonizes CsrA RNA binding by a noncompetitive allosteric mechanism. Proc. Natl. Acad. Sci. U.S.A. 113, 9870–9875. doi: 10.1073/pnas.1602455113
Mukherjee, S., Yakhnin, H., Kysela, D., Sokoloski, J., Babitzke, P., and Kearns, D. B. (2011). CsrA-FliW interaction governs flagellin homeostasis and a checkpoint on flagellar morphogenesis in Bacillus subtilis. Mol. Microbiol. 82, 447–461.
Müller, P., Gimpel, M., Wildenhain, T., and Brantl, S. (2019). A new role for CsrA: promotion of complex formation between an sRNA and its mRNA target in Bacillus subtilis. RNA Biol. 16, 972–987. doi: 10.1080/15476286.2019.1605811
Müller, P., Jahn, N., Ring, C., Maiwald, C., Neubert, R., Meißner, C., et al. (2016). A multistress responsive type I toxin-antitoxin system: bsrE/SR5 from the B. subtilis chromosome. RNA Biol. 13, 511–523. doi: 10.1080/15476286.2016.1156288
Muto, A., Fujihara, A., Ito, K. I., Matsuno, J., Ushida, C., and Himeno, H. (2000). Requirement of transfer-messenger RNA for the growth of Bacillus subtilis under stresses. Genes Cells. 5, 627–635. doi: 10.1046/j.1365-2443.2000.00356.x
Newman, J. A., Hewitt, L., Rodrigues, C., Solovyova, A. S., Harwood, C. R., and Lewis, R. J. (2012). Dissection of the network of interactions that links RNA processing with glycolysis in the Bacillus subtilis degradosome. J Mol. Biol. 416, 121–136. doi: 10.1016/j.jmb.2011.12.024
Noone, D., Salzberg, L. I., Botella, E., Basell, K., Becher, D., Antelmann, H., et al. (2014). A highly unstable transcript makes CwlO D,L endopeptidase expression responsive to growth conditions in Bacillus subtilis. J. Bacteriol. 196, 237–247. doi: 10.1128/jb.00986-13
Olejniczak, M., and Storz, G. (2017). ProQ/FinO-domain proteins: another ubiquitous family of RNA matchmakers. Mol. Microbiol. 104, 905–915. doi: 10.1111/mmi.13679
Otridge, J., and Gollnick, P. (1993). MtrB from Bacillus subtilis binds specifically to trp leader RNA in a tryptophan dependent manner. Proc. Natl. Acad. Sci. U.S.A. 90, 128–132. doi: 10.1073/pnas.90.1.128
Pechter, K. B., Meyer, F. M., Serio, A. W., Stülke, J., and Sonenshein, A. L. (2013). Two roles for aconitase in the regulation of tricarboxylic acid branch gene expression in Bacillus subtilis. J. Bacteriol. 195, 1525–1537. doi: 10.1128/jb.01690-12
Potter, K., Merlino, N. M., Jacobs, T., and Gollnick, P. (2011). TRAP binding to the Bacillus subtilis trp leader region RNA causes efficient transcription termination at a weak intrinsic terminator. Nucleic Acids Res. 39, 2092–2102. doi: 10.1093/nar/gkq965
Rasmussen, S., Nielsen, H. B., and Jarmer, H. (2009). Transcriptionally active regions in the genome of Bacillus subtilis. Mol. Microbiol. 73, 1043–1057. doi: 10.1111/j.1365-2958.2009.06830.x
Rath, H., Reder, A., Hoffmann, T., Hammer, E., Seubert, A., Bremer, E., et al. (2020). Management of osmoprotectant uptake hierarchy in Bacillus subtilis via a SigB-dependent antisense RNA. Front. Microbiol. 11:622.
Reif, C., Löser, C., and Brantl, S. (2018). Bacillus subtilis type I antitoxin SR6 promotes degradation of toxin yonT mRNA and is required to prevent toxin yoyJ overexpression. Toxins (Basel) 10:E74.
Rochat, T., Delumeau, O., Figueroa-Bossi, N., Noirot, P., Bossi, L., Dervyn, E., et al. (2015). Tracking the elusive function of Bacillus subtilis Hfq. PLoS One 10:e0124977. doi: 10.1371/journal.pone.0124977
Rowland, S. L., Burkholder, W. F., Cunningham, K. A., Maciejewski, M. W., Grossman, A. D., and King, G. F. (2004). Structure and mechanism of action of Sda, an inhibitor of the histidine kinases that regulate initiation of sporulation in Bacillus subtilis. Mol. Cell 13, 689–701. doi: 10.1016/s1097-2765(04)00084-x
Savacool, H. K., and Switzer, R. L. (2002). Characterization of the interaction of Bacillus subtilis PyrR with pyr mRNA by site-directed mutagenesis of the protein. J. Bacteriol. 184, 2521–2528. doi: 10.1128/jb.184.9.2521-2528.2002
Schmalisch, M., Maiques, E., Nikolov, L., Camp, A. H., Chevreux, B., Muffler, A., et al. (2010). Small genes under sporulation control in the Bacillus subtilis genome. J. Bacteriol. 192, 5402–5412. doi: 10.1128/jb.00534-10
Serio, A. W., Pechter, K. B., and Sonenshein, A. L. (2006). Bacillus subtilis aconitase is required for efficient late-sporulation gene expression. J. Bacteriol. 188, 6396–6405. doi: 10.1128/jb.00249-06
Shapiro, L., McAdams, H. H., and Losick, R. (2009). Why and how bacteria localize proteins. Science 326, 1225–1228. doi: 10.1126/science.1175685
Shimokawa-Chiba, N., Müller, C., Fujiwara, K., Beckert, B., Ito, K., Wilson, D. N., et al. (2019). Release factor-dependent ribosome rescue by BrfA in the Gram-positive bacterium Bacillus subtilis. Nat. Commun. 10:5397.
Shin, J. H., and Price, C. W. (2007). The SsrA-SmpB ribosome rescue system is important for growth of Bacillus subtilis at low and high temperatures. J. Bacteriol. 189, 3729–3737. doi: 10.1128/jb.00062-07
Silvaggi, J. M., Perkins, J. B., and Losick, R. (2005). Small untranslated RNA antitoxin in Bacillus subtilis. J. Bacteriol. 187, 6641–6650. doi: 10.1128/jb.187.19.6641-6650.2005
Smaldone, G. T., Revelles, O., Gaballa, A., Sauer, U., Antelmann, H., and Helmann, J. D. (2012a). A global investigation of the Bacillus subtilis iron-sparing response identifies major changes in metabolism. J. Bacteriol. 194, 2594–2605. doi: 10.1128/jb.05990-11
Smaldone, G. T., Antelmann, H., Gaballa, A., and Helmann, J. D. (2012b). The FsrA sRNA and FbpB protein mediate the iron-dependent induction of the Bacillus subtilis lutABC iron-sulfur-containing oxidases. J. Bacteriol. 194, 2586–2593. doi: 10.1128/jb.05567-11
Someya, T., Baba, S., Fujimoto, M., Kawai, G., Kumasaka, T., and Nakamura, K. (2012). Crystal structure of Hfq from Bacillus subtilis in complex with SELEX-derived RNA aptamer: insight into RNA-binding properties of bacterial Hfq. Nucleic Acids Res. 40, 1856–1867. doi: 10.1093/nar/gkr892
Storz, G., Wolf, Y. I., and Ramamurthi, K. S. (2014). Small proteins can no longer be ignored. Annu. Rev. Biochem. 83, 753–777. doi: 10.1146/annurev-biochem-070611-102400
Stülke, J. (2002). Control of transcription termination in bacteria by RNA-binding proteins that modulate RNA structures. Arch. Microbiol. 177, 433–440.
Turnbough, C. L. Jr. (2019). Regulation of bacterial gene expression by transcription attenuation. Microbiol. Mol. Biol. Rev. 83:e00019-19.
Turnbough, C. L. Jr., and Switzer, R. L. (2008). Regulation of pyrimidine biosynthetic gene expression bacteria: repression without repressors. Microbiol. Mol. Biol. Rev. 72, 266–300. doi: 10.1128/mmbr.00001-08
Turner, R. J., Lu, Y., and Switzer, R. L. (1994). Regulation of the Bacillus subtilis pyrimidine biosynthetic (pyr) gene cluster by an autogenous transcriptional attenuation mechanism. J. Bacteriol. 176, 3708–3722. doi: 10.1128/jb.176.12.3708-3722.1994
Ujiie, H., Matsutani, T., Tomatsu, H., Fujihara, A., Ushida, C., Miwa, Y., et al. (2008). Trans-translation is involved in the CcpA-dependent tagging and degradation of TreP in Bacillus subtilis. J Biochem. 145, 59–66. doi: 10.1093/jb/mvn143
Ul Haq, I., Müller, P., and Brantl, S. (2020). SR7–a dual function antisense RNA from Bacillus subtilis. RNA Biol. 5, 1–14. doi: 10.1080/15476286.2020.1798110
Vakulskas, C. A., Potts, A. H., Babitzke, P., Ahmer, B. M., and Romeo, T. (2015). Regulation of bacterial virulence by Csr (Rsm) systems. Microbiol. Mol. Biol. Rev. 79, 193–224. doi: 10.1128/mmbr.00052-14
Wagner, E. G., and Romby, P. (2015). Small RNAs in bacteria and archaea: who they are, what they do, and how they do it. Adv. Genet. 909, 133–208.
Walden, W. E., Selezneva, A. I., Dupuy, J., Volbeda, A., Fontecilla-Camps, J. C., Theil, E., et al. (2006). Structure of dual function iron regulatory protein 1 complexed with ferritin IRE-RNA. Science 314, 1903–1908. doi: 10.1126/science.1133116
Wassarman, K. M., Repoila, F., Rosenow, C., Storz, G., and Gottesman, S. (2001). Identification of novel small RNAs using comparative genomics and microarrays. Genes Dev. 15, 1637–1651. doi: 10.1101/gad.901001
Wassarman, K. M., and Storz, G. (2000). 6S RNA regulates E. coli RNA polymerase activity. Cell 101, 613–623. doi: 10.1016/s0092-8674(00)80873-9
Whitten, A. E., Jacques, D. A., Hammouda, B., Hanley, T., King, G. F., Guss, J. M., et al. (2007). The structure of the KinA-Sda complex suggests an allosteric mechanism of histidine kinase inhibition. J. Mol. Biol. 368, 407–420. doi: 10.1016/j.jmb.2007.01.064
Wiegert, T., and Schumann, W. (2001). SsrA-mediated tagging in Bacillus subtilis. J. Bacteriol. 183, 3885–3889. doi: 10.1128/jb.183.13.3885-3889.2001
Yakhnin, H., Pandit, P., Petty, T. J., Baker, C. S., Romeo, T., and Babitzke, P. (2007). CsrA of Bacillus subtilis regulates translation initiation of the gene encoding the flagellin protein (hag) by blocking ribosome binding. Mol. Microbiol. 64, 1605–1620. doi: 10.1111/j.1365-2958.2007.05765.x
Yang, Y., Declerck, N., Manival, X., Aymerich, S., and Kochoyan, M. (2002). Solution structure of the LicT RNA antitermination complex: CAT clamping RAT. EMBO J. 21, 1987–1997. doi: 10.1093/emboj/21.8.1987
Zhang, H., and Switzer, R. L. (2003). Transcriptional pausing in the Bacillus subtilis pyr operon in vitro: a role in transcriptional attenuation? J. Bacteriol. 185, 4764–4771. doi: 10.1128/JB.185.16.4764-4771.2003
Zhang, J., Chetnani, B., Cormack, E. D., Alonso, D., Liu, W., Mondragón, A., et al. (2018). Specific structural elements of the T-box riboswitch drive the two-step binding of the tRNA ligand. eLife 7:e39518.
Keywords: sRNA, small regulatory RNA, Bacillus subtilis, type I toxin-antitoxin system, T-box riboswitch, RNA chaperones, small proteins, antiterminators
Citation: Ul Haq I, Müller P and Brantl S (2020) Intermolecular Communication in Bacillus subtilis: RNA-RNA, RNA-Protein and Small Protein-Protein Interactions. Front. Mol. Biosci. 7:178. doi: 10.3389/fmolb.2020.00178
Received: 28 April 2020; Accepted: 09 July 2020;
Published: 07 August 2020.
Edited by:
Manuel Espinosa, Margarita Salas Center for Biological Research (CSIC), SpainReviewed by:
Jörg Stülke, University of Göttingen, GermanyElena Evguenieva-Hackenberg, University of Giessen, Germany
Copyright © 2020 Ul Haq, Müller and Brantl. This is an open-access article distributed under the terms of the Creative Commons Attribution License (CC BY). The use, distribution or reproduction in other forums is permitted, provided the original author(s) and the copyright owner(s) are credited and that the original publication in this journal is cited, in accordance with accepted academic practice. No use, distribution or reproduction is permitted which does not comply with these terms.
*Correspondence: Sabine Brantl, c2FiaW5lLmJyYW50bEB1bmktamVuYS5kZQ==