- 1Department of Bioinformatics, State Key Laboratory of Plant Physiology and Biochemistry, College of Life Sciences, Zhejiang University, Hangzhou, China
- 2James D. Watson Institute of Genome Sciences, Zhejiang University, Hangzhou, China
CircRNAs are covalently closed-loop single-stranded RNA molecules ubiquitously expressing in eukaryotes. As an important member of the endogenous ncRNA family, circRNAs are associated with diverse biological processes and can regulate transcription, modulate alternative splicing, and interact with miRNAs or proteins. Compared to abundant advances in animals, studies of circRNAs in plants are rapidly emerging. The databases and analysis tools for plant circRNAs are constantly being developed. Large numbers of circRNAs have been identified and characterized in plants and proved to play regulatory roles in plant growth, development, and stress responses. Here, we review the biogenesis, characteristics, bioinformatics resources, and biological functions of plant circRNAs, and summarize the distinct circularization features and differentially expression patterns comparison with animal-related results.
Introduction
Circular RNAs (circRNAs) are a unique group of endogenous non-coding RNAs (ncRNAs) for their distinct closed-loop structures. CircRNAs are formed in the non-canonical “back-splicing” event, in which the 5′ and 3′ ends are attached by covalent bond (Jeck et al., 2013; Memczak et al., 2013; Jeck and Sharpless, 2014). Different from linear RNAs, circRNAs form closed-loop structures by covalent bond linking the 5′ and 3′ ends. Therefore, this type of ncRNAs is more stable than linear RNAs and not easily degraded by Ribonuclease R (RNase R) (Suzuki et al., 2006). Although circRNAs have been observed for decades, they are typically considered as by-products of aberrant RNA splicing (Nigro et al., 1991; Cocquerelle et al., 1993). In the recent decade, numerous circRNAs have been identified and annotated in diverse species as high-throughput sequencing technologies and bioinformatics tools advance. The published research exhibited that circRNAs are ubiquitous and abundant in all eukaryotes, such as mammals, worms, insects, fish, plants, fungi, and protists, and even in prokaryotic archaea (Danan et al., 2012; Jeck et al., 2013; Memczak et al., 2013; Wang et al., 2014; Westholm et al., 2014; Ivanov et al., 2015; Ye et al., 2015).
The majority of circRNAs are generated from protein-coding genes and consist of a single exon or multiple exons (Guo et al., 2014). Despite lack of 5′ caps and poly(A) tails, circRNAs produced in the nucleus are normally transported to the cytoplasm (Salzman et al., 2012), while a few circRNAs that are generated from excised intron lariats preferentially localize to the nucleus (Zhang et al., 2013). Although back-splicing is generally less efficient than linear splicing and their expression level are relatively low, circRNAs can accumulate in specific cell types or tissues in a temporally regulated manner (Rybak-Wolf et al., 2015; Veno et al., 2015) owing to their high stability (Jeck et al., 2013; Memczak et al., 2013). These results suggest the potential functions of circRNAs. Emerging evidence has shown that circRNAs are involved in the regulation of gene expression at transcriptional and post-transcriptional levels. They are reported to function as miRNA sponges (Hansen et al., 2013) or RNA-binding proteins (RBP) sponges (Ashwal-Fluss et al., 2014) and are ideal biomarkers (Lukiw, 2013). It is disclosed in the latest research that proteins and peptides could derive from circRNAs comprising internal ribosomal entry sites (IRESs) (Pamudurti et al., 2017; Yang et al., 2017).
The characterization of circRNAs in plants is comparatively less than the comprehensive systematic analysis in animals. Thousands of circRNAs have been identified in nearly 30 plant species, including the model plants, crops, and Chinese herbal medicines (Ye et al., 2015; Wang et al., 2016; Zuo et al., 2016; Dong et al., 2019). Plant circRNA information is available in various databases, such as PlantCircNet (Zhang et al., 2017) and PlantcircBase (Chu et al., 2018), which collect more than 200,000 circRNAs from different research groups in total. Studies have shown that there are several differences in the circularization mechanisms of circRNAs in plants and animals. Flanking intronic complementary sequences of circularized exons have been demonstrated to be significantly important for circRNA biogenesis in animals (Zhang et al., 2014). Nonetheless, enrichment of repetitive elements or reverse complementary sequences do not appear to be found in flanking sequences of identified plant circRNAs (Lu et al., 2015; Ye et al., 2015). Thus, the circularization of plant circRNAs may be regulated by alternative mechanisms that are yet to be found.
In this review, we outline the recent advances in the biogenesis and functions of circRNAs in plants. The distinct features and circularization mechanisms of plant circRNAs are summarized by comparing them with related studies in animals. Then available bioinformatics resources, expression patterns and diverse functions of plant circRNAs are described. Particularly, the putative roles of plant circRNAs in responses to biotic and abiotic stresses are well summarized in Table 2.
Biogenesis and Discovering of Plant CircRNAs
Although the mechanisms of circRNA biogenesis are not very clear, two well-known models have been proposed for circRNA formation. CircRNAs are usually circularized from canonical splicing sites (Jeck et al., 2013; Memczak et al., 2013), which means back-splicing requires canonical splicing machinery (Ashwal-Fluss et al., 2014; Starke et al., 2015). Moreover, back-splicing is normally mediated by base pairing between inverted repeat elements locating in upstream and downstream introns (Zhang et al., 2014; Ivanov et al., 2015), or by dimerization of RBPs that combine with specific motifs in the flanking introns (Figure 1A) (Conn et al., 2015). CircRNAs are also generated from lariat precursors during exon-skipping or from intron lariats that escape debranching (Figure 1B) (Kelly et al., 2015).
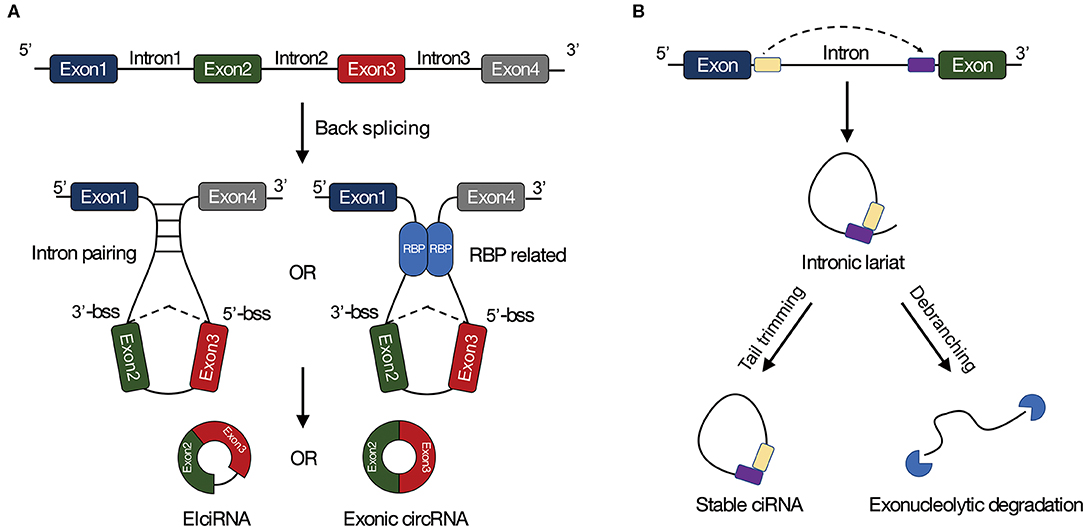
Figure 1. Biogenesis of circRNAs. (A) Long flanking introns, inverted repeat elements, and RNA binding proteins are facilitated to back-splicing. (B) Circular intronic RNAs (ciRNAs) can be generated from intronic lariat precursors that escape from debranching. Bss, back-splice site; EIciRNA, exon-intron circRNA; ciRNA, circular intronic RNA.
rRNA-depleted total RNA-seq is widely used in early genome-wide profiling studies of circRNAs. Previous studies have exploited this type of publicly available datasets for circRNA identification and characterization in plants (Ye et al., 2015). Considering circular structures and relatively low expression levels of circRNAs, an additional protocol that using linear RNase R for library preparation has become the preferred method for greatly enriching circRNAs. This method has been widely used in plant circRNA studies and yielded high confidence circRNAs in maize, soybean, bamboo, tomato, and grape (Zuo et al., 2016; Chen L. F. et al., 2018; Gao et al., 2019; Luo et al., 2019; Wang Y. S. et al., 2019).
As mentioned, circRNAs are created through back-splicing where the downstream 3′ donor splice sites are joined to the upstream 5′ acceptor splice sites (Figure 1A). Two terminal sequences flanking the back-splice sites constitute the sequencing reads crossing back-splice junctions, which is normally ignored by mapping algorithms. These features have been exploited newly in developed bioinformatics tools to specifically detect circRNAs. These tools differ in the strategy of identifying circRNAs, so that they could be assigned to two categories (Chen et al., 2015). The first one is pseudo-reference-based approach, which relies entirely on the accurate genome annotation with all alternatively spliced mRNA isoforms, such as KNIFE (Szabo et al., 2016) and NCLscan (Chuang et al., 2016). Prior to reading mapping, all possible circRNA sequences are reconstructed based on genome annotation by shuffling exon-exon junctions (Szabo et al., 2016). The second group termed fragment-based approach, identify back-splice junctions from mapping information of multiple split alignments, including MapSplice (Wang et al., 2010), find_circ (Memczak et al., 2013), and CIRI (Gao et al., 2015). This approach has been extensively used in plant circRNA research, which infers all possible back-splicing events based on reads mapping information rather than the prior knowledge of gene annotation.
The locus-specific profiling of circRNAs that using genomic positions of back-splice sites, can be adapted for validating, quantitating, and investigating previously characterized circRNAs. For example, reverse transcription and PCR (RT-PCR) is more capable for circRNAs validation than northern blotting (Kristensen et al., 2019), droplet digital PCR (ddPCR) and reverse transcription quantitative PCR (RT-qPCR) are both available for circRNAs quantitation (Maheshwari et al., 2017; Li et al., 2018). However, genome-wide profiling and locus-specific methods could detect unique back-splice sites in circRNAs, while they cannot determine internal splicing patterns of circRNA formation.
Characterization of Plant CircRNAs
Circularization
CircRNAs can arise from a wide range of genomic positions and combinations, including exons, introns, or intergenic regions. The biogenesis of circRNAs basically depends on back-splicing of pre-mRNAs, which is conserved in eukaryotes. Significant enrichment of reverse complementary sequences comprising short repeat elements can be detected in flanking introns of circularized exons in animals, which are essential for circRNA circularization (Ashwal-Fluss et al., 2014; Liang and Wilusz, 2014). For example, circularized exons are bracketed by long introns comprising Arthrobacter luteus (Alu) elements in humans (Jeck et al., 2013). In addition, RNA secondary structures and RNA-binding proteins are associated with circRNA biogenesis, and the sequence length of the flanking introns of circularized exons significantly alter circularization efficiency (Conn et al., 2015; Kramer et al., 2015). Unlike animal circRNAs, comparatively few plant circRNAs contain reverse and repetitive complementary sequences in the intronic sequences flanking exons (Lu et al., 2015; Ye et al., 2015; Zhao T. et al., 2017; Zhu et al., 2019), suggesting that intron-pairing-driven circularization may not be the primary mechanism for plant circRNA biogenesis. It has been proved that transposons and their reverse-complement counterparts enrich in maize circRNA flanking regions, showing their affection to circRNA biogenesis (Chen L. et al., 2018).
CircRNAs are generated from back-splicing which covalently link spliced down-stream splice donors with upstream splice acceptors. In the main spliceosome for splicing of most introns of eukaryotes, GT and AG terminal dinucleotides locate in the 5′ and 3′ end, respectively (Szczesniak et al., 2013). However, the mechanisms for spliceosomes selection and splicing signals of circularization are poorly characterized. In plants, circRNAs have been shown to harbor non-GT/AG splicing signals, while the majority exonic circRNAs are spliced by canonical GT/AG signal in animals (Ye et al., 2017). In Arabidopsis, grape and cotton, the majority of identified circRNAs are spliced by canonical splicing signal (Sun et al., 2016; Zhao T. et al., 2017; Gao et al., 2019), which is disagreement with the results in rice. Hundreds of circRNAs are shared with non-canonical splicing signals in rice (Ye et al., 2017), such as GC/CG, CT/GC, and GC/GT, as well as in cucumber (Zhu et al., 2019) and chloroplast of A. thaliana (Liu et al., 2019). Interestingly, the circRNA identification methods (find_circ and CIRI2) only consider canonical GT/AG splicing in cucumber and chloroplast of A. thaliana. Recent studies have revealed that back-splice sites of circRNAs are flexible and the alternative splicing of circRNAs is prevalent and much of the alternative splicing of circRNAs occurred nearby canonical splicing sites (Starke et al., 2015; Chen et al., 2016; Szabo et al., 2016). Thus, the splice signal patterns of circRNA circularization need to be verified in more plant species.
Properties and Conservation
CircRNAs are derived from all the chromosomes, as well as mitochondrial and chloroplast genomes in plants. The length of circRNAs ranges from <200 bp to longer than 100 kb, normally <1 kb. In plants, circRNAs are mainly between 200 and 600 bp, only a few of them >2 kb (Ye et al., 2017). Consistent with animals, most plant circRNAs are generated from exons of a single gene, some of them from introns, intergenic regions, untranslated regions (UTRs) or more than one gene (Ye et al., 2015). The results of gene structure annotations demonstrated that most host genes prefer to produce one circRNA than produce more than one circRNA, and few of circRNAs are generated from different loci of the same gene. CircRNA isoforms can be derived from the same locus through alternative circularization. The expression of circRNAs are much lower than their linear counterparts and correlates with the expression of their parental gene transcripts. Though, the internal regulatory mechanism of these correlations needs further research. For example, the expression of Ac_ciRNA_04842 and its derived gene Achn372061 is positively correlated in kiwifruit (Wang et al., 2017); in rice, overexpressing Os08circ16564 significantly inhibits the expression of its parental gene AK064900 (Lu et al., 2015). CircRNAs are highly conserved in different plant species. More than 700 orthologous genes pairs that produce circRNAs were found between Arabidopsis and rice (Ye et al., 2015). Another study of eight plant species has shown that circRNA host orthologous genes accounting for nearly 20% of genes that produce circRNAs (Zhu et al., 2019).
Bioinformatics Resources for Plant CircRNAs
High-throughput sequencing technology enables millions of circRNA sequencing reads to be accumulated in a short time period. To deal with the large number of RNA-seq datasets and have deeper understanding of circRNA biogenesis, new algorithms for efficient and accurate identification of circRNA are constantly being developed, including find_circ (Memczak et al., 2013), CIRCexplorer (Zhang et al., 2014), KNIFE (Szabo et al., 2015), and CIRI (Gao et al., 2015). However, these tools have differential performance in terms of sensitivity, accuracy, and computational costs when detecting circRNAs from RNA-Seq datasets. Comparative analyses of circRNA identification tools revealed that CIRI, KNIFE, and CIRCexplorer had better performance in terms of balancing precision and sensitivity (Zeng et al., 2017) and combining different tools could achieve more reliable predictions (Hansen et al., 2016). These comparison results provide useful guidance for improving algorithms and using current tools by researchers. PcircRNA_finder is developed specifically for plant circRNA detection, which combined five different tools to provide a more comprehensive, precise, and sensitive prediction method (Table 1) (Chen et al., 2016). CircPro is developed for investigating the protein-coding ability of circRNAs, which is an automated analysis pipeline that integrates five tools (Table 1) (Meng et al., 2017).
Increasing numbers of circRNA datasets have been generated and exploded in plants, several databases have been established to effectively organize and manage these datasets (Table 1). Comparing with animals, the plant circRNA databases are relatively insufficient in datasets and types. PlantcircBase (Chu et al., 2017, 2018) and PlantCircNet (Zhang et al., 2017) are comprehensive resources for plant circRNAs, containing published and new identified circRNAs information from different plant species, genome browsing, and putative mRNA-miRNA-circRNA interaction networks in corresponding species. Besides, PlantcircBase provides structure visualization of specific circRNA and validation information by PCR and sequencing. AtCircDB (Ye et al., 2019) is developed for analyzing the tissue specificity of circRNAs in Arabidopsis, while circRNA information in crops response to abiotic stress is summarized in CropCircDB (Wang K. et al., 2019). Though only two crops, maize and rice, are collected in the database. CircFunBase (Meng et al., 2019) provides circRNA information with experimentally validated and computationally predicted functions and visualized circRNA-miRNA interaction networks. ASmiR (Wang H. Y. et al., 2019) contains alternative splicing information form linear and circular RNAs in plants and their interaction information with miRNAs. However, plant species, information of phylogenetic conservation, cell-type, tissue or development stage expression, functional annotation, and interaction with other molecules are still insufficient in various databases, which will greatly promote circRNA research in plants.
Function of Plant CircRNAs
CircRNAs Act as miRNA Sponges
Due to previous studies in animals, the most striking function of circRNAs is to act as miRNA sponges or participate in miRNA-related pathways to regulate gene expression (Figure 2IV). The transcripts that contain multiple miRNA-binding sites and inhibit miRNA activity are called miRNA sponges (Ebert et al., 2007), also called competing endogenous RNAs (ceRNAs) in animals or target mimicry in plants (Franco-Zorrilla et al., 2007). These transcripts could be used to explore the function of miRNAs. Due to their loop structures, circRNAs are resistant to RNA exonucleases. These high stabilities make circRNAs have an advantage as miRNA sponges, with half-lives more than 48 h, while the half-lives of their linear counterparts are <20 h (Jeck et al., 2013). The circRNA CiRS-7 is a canonical miRNA sponge playing roles in inhibiting miR-7. In humans, ciRS-7 (also known as CDR1as) contains more than 70 conserved miR-7 binding sites and can strongly suppress miR-7 activity, resulting in increased levels of miR-7 targets (Hansen et al., 2013). Some studies have shown that circRNAs are putative miRNA sponges in plants, but few direct experimental evidence has been proposed. Moreover, compared with animals, plant circRNAs acting as miRNA sponges account for a smaller proportion of the total circRNAs and have lesser miRNA-binding sites (Ye et al., 2015; Zuo et al., 2016).
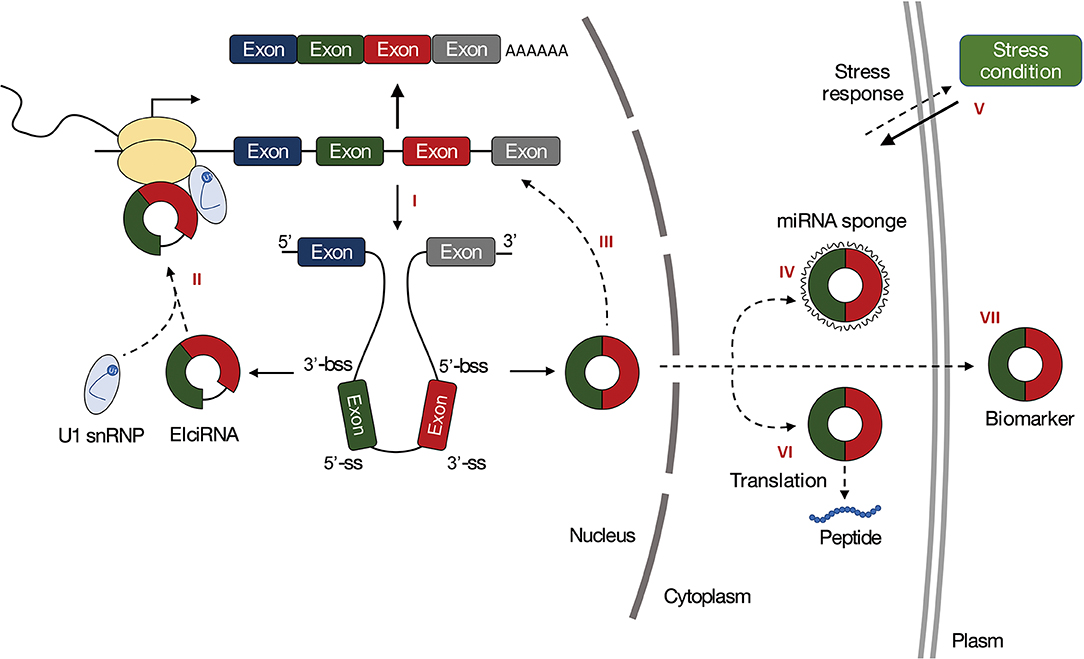
Figure 2. Functions of circRNAs in plants. (I) The processing of circRNAs can affect the splicing of their linear counterparts. (II) CircRNAs can regulate transcription of their parental genes. (III) CircRNAs can regulate the splicing of their linear cognates. (IV) CircRNAs can act as miRNA sponges. (V) CircRNAs can regulate gene expression in response to biotic or abiotic stresses. (VI) CircRNAs can be translated. (VII) CircRNAs are promising biomarkers.
It is reported that only 6.6 and 5.0% of circRNAs contained putative miRNA-binding sites in rice and Arabidopsis, respectively (Ye et al., 2015). Another study in rice has been shown that 31 circRNAs harbors two or more putative miRNA-binding sites, while in total 235 circRNAs have miRNA-binding sites (Lu et al., 2015). Besides, 53 sea buckthorn circRNAs, 30 cucumber circRNAs, 25 chinese cabbage circRNAs, and 9 pepper circRNAs are predicted to act as miRNA sponges (Wang H. Y. et al., 2019; Zhang G. Y. et al., 2019; Zhu et al., 2019; Zuo et al., 2019), which required further experimental validation. In Arabidopsis thaliana, five circRNAs derived from flowers may function as miRNA sponges while one of them has been experimentally validated (Frydrych Capelari et al., 2019). Based on the hypothesis that circRNAs and mRNAs are targeted by the same miRNA, the putative ceRNA networks have been investigated in Arabidopsis leaves, which suggests the regulatory roles of circRNAs in leaf senescence base on differential expression patterns of mRNAs, circRNAs, and miRNAs (Meng et al., 2018). By interacting with miRNA, circRNAs may play regulatory roles in a variety of processes, including metabolic processes, developmental processes, reproductive processes, abiotic, and bionic stress response. Nevertheless, their authenticity requires further experimental validation and crosslinking immunoprecipitation and high-throughput sequencing (CLIP-seq) can help for it (Chi et al., 2009).
CircRNAs in Stress Response
Previous studies have demonstrated that plant circRNAs exhibit specific cell-type, tissue, or developmental stage expression patterns and circRNAs expression is usually induced under various environmental stresses (Figure 2V; Table 2), including drought, chilling, heat, nutrient deficiency, or pathogen invasion. These suggest that circRNAs may like other ncRNAs, such as miRNAs and lncRNAs, which are crucial to plant growth and development, as well as biotic or abiotic stresses response. Evidence has shown that those differentially expressed circRNAs could regulate plant in responses to stresses by interacting with miRNAs and regulating the expression of stimulus-responsive genes. The regulatory roles and potential functions of circRNAs could be inferred and experimentally verified by establishing the circRNA-mediated ceRNA network under stress conditions.
CircRNAs were firstly identified under the biotic stress condition was in Arabidopsis leaves under pathogenic interaction (Sun et al., 2016). In kiwifruit, circRNAs were also identified differentially expressed under pathogen invasion (Wang et al., 2017). In total, 584 circRNAs have been shown differential expression patterns during Pseudomonas syringae pv. actinidiae (Psa) infection and their expression are related to the stage of infection (Wang et al., 2017). Besides, a list of circRNAs related to plant defense response have been identified by network analysis (Wang et al., 2017). Later studies indicate that circRNAs can function as negative regulators of tomato yellow leaf curl virus (TYLCV) interaction in tomato (Wang J. Y. et al., 2018), play regulatory roles in the Verticillium wilt response in cotton (Xiang et al., 2018) and are response to maize Iranian mosaic virus (MIMV) infection in maize (Ghorbani et al., 2018).
CircRNAs have been shown to be differentially expressed under abiotic stresses, including nutrient depletion, high light, heat, chilling, drought, or salt. However, the regulatory mechanism and specific biological significance of plant circRNAs during these conditions remain to be elucidated. In the samples from Oryza sativa roots with phosphate-starvation condition and A. thaliana leaves with light treatment, circRNAs were firstly identified in plants, and 27 circRNAs in rice were found to display stress-specific expression patterns under phosphate deficiency condition, of which 6 were up-regulated and 21 were down-regulated (Ye et al., 2015). These results indicated the potential roles of plant circRNAs in response to stress, furthermore, the stress-specific expression patterns are also found in other plant species with different biotic stress conditions. Analyses have shown that 36 and 163 differentially expressed circRNAs were identified in chilled bell pepper (Zuo et al., 2018) and chilled tomato fruit (Zuo et al., 2016) respectively, 475 differentially expressed circRNAs were identified in grape leaves under cold stress (Gao et al., 2019). The grape Vv-circATS1, derived from glycerol-3-P acyltransferase, has been found to improve cold tolerance in Arabidopsis by regulating the expression of stimulus-responsive genes, such as CSD2, PRXCA, PME41, LOX3, and WRKY48. Under dehydration-stressed conditions, differentially expressed circRNAs have been detected in wheat (Wang et al., 2016), pear (Wang J. et al., 2018), maize, and Arabidopsis (Zhang P. et al., 2019). Moreover, the similar patterns of expressional changes were observed in crops under nutrient depletion (Darbani et al., 2016; Wang W. H. et al., 2019; Lv et al., 2020), metal ion toxicity (Fu et al., 2019) or salt (Zhu et al., 2019). In addition, researchers found that the stress condition could alter lengths of circRNAs, numbers of circularized exons and alternative circularization events in Arabidopsis (Pan et al., 2018).
CircRNAs Regulate Gene Expression
Accumulating evidence has proved that circRNAs are involved in the regulation of gene expression. The processing of circRNAs can affect the splicing of their linear counterparts (Figure 2I), and regulate the transcription of their parental genes (Figure 2II). In human, exon-intron circRNAs (EIciRNAs) enhance transcription of circRNA host genes through association with U1 snRNP. EIciRNAs have been identified in plants and implicated in gene regulation in biotic stress response (Zhao W. et al., 2017). In Arabidopsis, CircSEP3 that derived from SEPALLATA3 (SEP3) gene has been shown to regulate transcription and splicing of their linear counterparts (Figure 2III). CircSEP3 can bind strongly to its cognate DNA locus and form an RNA:DNA hybrid, whereas the linear RNA with the same sequence bind to the DNA much more weakly. The circRNA:DNA formation would result in transcriptional pausing and leading to the formation of alternatively spliced SEP3 mRNA with exon skipping (Conn et al., 2017). These together suggest that circRNAs can modulate gene expression at both transcription and splicing levels. In addition, the correlations between circRNAs and their parental genes have been indicated in studies of plant circRNAs, the mechanistic basis of these correlations needs further experimental validation.
CircRNAs Can Be Translated
Due to the lack of key components for the canonical cap-dependent translation, such as 5′ caps or poly(A) tails, circRNAs are considered untranslatable. However, recent research in mammals revealed that circRNAs translation could be driven by IRESs (Abe et al., 2015) and promoted by N6-Methyladenosine (m6A) RNA modification (Meyer et al., 2015; Yang et al., 2017) (Figure 2VI). Although a number of circRNAs have been predicted to contain putative open reading frames (ORFs) with IRESs, a few circRNAs have proven to act as protein templates, and functions of peptides derived from circRNAs remain to be explored (Legnini et al., 2017; Pamudurti et al., 2017; Zhang et al., 2018). For example, peptides FBXW-185aa that are translated from circ-FBXW7 may inhibit tumorigenesis of brain cancer (Yang et al., 2018). FBXW-185aa has been shown to interact with the deubiquitinating enzyme USP28, protecting USP28 from binding to a key regulator of tumorigenesis, FBXW7α. Therefore, FBXW-185aa prevents FBXW7α-induced degradation by antagonizing USP28-induced c-Myc stabilization. Moreover, circRNAs that contain large ORFs and m6A-modified sites in junction sequences have been detected to encode hundreds of peptides (Tang et al., 2020). For example, m6A-modified circE7 could be translated to produce E7 oncoprotein, which is directly responsible for HPV-induced carcinogenesis (Zhao et al., 2019). The latest evidence also shows that m6A modified circRNA could inhibit innate immunity (Chen et al., 2019). However, there is no circRNA in plants has been reported to be translated. The studies of m6A modification in Arabidopsis have been reported (Zhou et al., 2017), translatable circRNAs and their biological functions would be proposed in plants as research progresses.
CircRNAs Act as Biomarkers
It has been illustrated by previous research that circRNAs are universal in diverse cell types, conserved in different species and exhibit specific expression patterns, which makes them potential biomarkers (Figure 2VII). Moreover, circRNAs have been shown to participate in diverse physiological and pathophysiological processes, including cancers (Li et al., 2015; Qin et al., 2016; Zhu et al., 2017), cardiovascular disease (Satoh et al., 2013), neurological disease (Grapp et al., 2013) and diabetes (Zhao Z. et al., 2017), suggesting that circRNAs are becoming the emerging biomarkers for diagnosis and treatment of human diseases. CircRNAs are also regarded as aging biomarkers in Drosophila research (Westholm et al., 2014). Biomarkers have been investigated and used in breeding applications for a couple of years in plants (Yang et al., 2011). Due to their characteristics, circRNAs are emerging biomarkers in plants. In Arabidopsis, circRNAs have been shown to act as bona fide biomarkers of alternative splicing variants (Conn et al., 2017). The study in maize and Arabidopsis suggests that circRNAs play essential roles in plant drought response, and can be used as effective biomarkers in genetic improvement of crop drought tolerance (Zhang P. et al., 2019). Hence, the biomarker is an interesting research topic in plant circRNAs.
Conclusions and Future Perspectives
With large numbers of circRNAs being identified, more insights have been given into circRNAs, which has become a promising research hotspot. CircRNAs usually exhibit specific expression patterns and are also induced by stress conditions, suggesting that circRNAs may be a new regulatory factor at the transcriptional and post-transcriptional levels. Evidence indicates that circRNAs participate in diverse biological processes, they can act as miRNA sponges, interact with many different RBPs, be translated into peptides or act as promising biomarkers. Compared with related studies in animals, the research of plant circRNAs are relatively less, and lack of experimental and functional verification. The formation mechanism of plant circRNAs is based on bioinformatics analysis rather than convincing experimental evidence, which is still in the theoretical stage. The characterization and function of plant circRNA have been proposed and verified, but there are still quite a few prediction results, especially the interactions between circRNAs and miRNAs. In addition, the coding ability of circRNAs and their individual function roles in plant growth and developments, such as leaf senescence, flower development, fruit maturation, and response to biotic and abiotic stresses, have not been investigated so far, which may account for primary research topics of circRNAs in plants. We still need more observation and validated results about the biogenesis mechanism, specific regulatory roles, and functions of these molecules, as well as large-scale quantification, full-length sequencing and functional verification of circRNAs. In addition, the differences between plant and animal circRNAs will also be an interesting topic.
Author Contributions
PZ and MC wrote and revised the manuscript. SL prepared the figures and revised the manuscript. All authors read and approved the final manuscript.
Funding
This work was supported by National Key Research and Development Program of China (Nos. 2016YFA0501704 and 2018YFC0310602), National Natural Science Foundation of China (Nos. 31771477, 31571366, and 31450110068), the Fundamental Research Funds for the Central Universities, Jiangsu Collaborative Innovation Center for Modern Crop Production, and 2018 Zhejiang University Academic Award for Outstanding Doctoral Candidates.
Conflict of Interest
The authors declare that the research was conducted in the absence of any commercial or financial relationships that could be construed as a potential conflict of interest.
Acknowledgments
The authors are grateful to Hongjun Chen and the other members of MC's laboratory for helpful discussions and valuable comments.
References
Abe, N., Matsumoto, K., Nishihara, M., Nakano, Y., Shibata, A., Maruyama, H., et al. (2015). Rolling circle translation of circular RNA in living human cells. Sci. Rep. 5:16435. doi: 10.1038/srep16435
Ashwal-Fluss, R., Meyer, M., Pamudurti, N. R., Ivanov, A., Bartok, O., Hanan, M., et al. (2014). circRNA biogenesis competes with Pre-mRNA splicing. Mol. Cell 56, 55–66. doi: 10.1016/j.molcel.2014.08.019
Chen, I., Chen, C. Y., and Chuang, T. J. (2015). Biogenesis, identification, and function of exonic circular RNAs. Wiley Interdiscip. Rev. RNA 6, 563–579. doi: 10.1002/wrna.1294
Chen, L., Yu, Y., Zhang, X., Liu, C., Ye, C., and Fan, L. (2016). PcircRNA_finder: a software for circRNA prediction in plants. Bioinformatics 32, 3528–3529. doi: 10.1093/bioinformatics/btw496
Chen, L., Zhang, P., Fan, Y., Lu, Q., Li, Q., Yan, J. B., et al. (2018). Circular RNAs mediated by transposons are associated with transcriptomic and phenotypic variation in maize. N. Phytol. 217, 1292–1306. doi: 10.1111/nph.14901
Chen, L. F., Ding, X. L., Zhang, H., He, T. T., Li, Y. W., Wang, T. L., et al. (2018). Comparative analysis of circular RNAs between soybean cytoplasmic male-sterile line NJCMS1A and its maintainer NJCMS1B by high-throughput sequencing. BMC Genomics 19:663. doi: 10.1186/s12864-018-5054-6
Chen, Y. G., Chen, R., Ahmad, S., Verma, R., Kasturi, S. P., Amaya, L., et al. (2019). N6-methyladenosine modification controls circular RNA immunity. Mol. Cell 76:e109.e9. doi: 10.1016/j.molcel.2019.07.016
Chi, S. W., Zang, J. B., Mele, A., and Darnell, R. B. (2009). Argonaute HITS-CLIP decodes microRNA-mRNA interaction maps. Nature 460, 479–486. doi: 10.1038/nature08170
Chu, Q., Bai, P., Zhu, X., Zhang, X., Mao, L., Zhu, Q. H., et al. (2018). Characteristics of plant circular RNAs. Brief. Bioinform. 21, 135–143. doi: 10.1093/bib/bby111
Chu, Q., Zhang, X., Zhu, X., Liu, C., Mao, L., Ye, C., et al. (2017). PlantcircBase: a database for plant circular RNAs. Mol. Plant 10, 1126–1128. doi: 10.1016/j.molp.2017.03.003
Chuang, T. J., Wu, C. S., Chen, C. Y., Hung, L. Y., Chiang, T. W., and Yang, M. Y. (2016). NCLscan: accurate identification of non-co-linear transcripts (fusion, trans-splicing and circular RNA) with a good balance between sensitivity and precision. Nucleic Acids Res. 44:e29. doi: 10.1093/nar/gkv1013
Cocquerelle, C., Mascrez, B., Hetuin, D., and Bailleul, B. (1993). Mis-splicing yields circular RNA molecules. FASEB J. 7, 155–160. doi: 10.1096/fasebj.7.1.7678559
Conn, S. J., Pillman, K. A., Toubia, J., Conn, V. M., Salmanidis, M., Phillips, C. A., et al. (2015). The RNA binding protein quaking regulates formation of circRNAs. Cell 160, 1125–1134. doi: 10.1016/j.cell.2015.02.014
Conn, V. M., Hugouvieux, V., Nayak, A., Conos, S. A., Capovilla, G., Cildir, G., et al. (2017). A circRNA from SEPALLATA3 regulates splicing of its cognate mRNA through R-loop formation. Nat. Plants 3:17053. doi: 10.1038/nplants.2017.53
Danan, M., Schwartz, S., Edelheit, S., and Sorek, R. (2012). Transcriptome-wide discovery of circular RNAs in Archaea. Nucleic Acids Res. 40, 3131–3142. doi: 10.1093/nar/gkr1009
Darbani, B., Noeparvar, S., and Borg, S. (2016). Identification of circular RNAs from the parental genes involved in multiple aspects of cellular metabolism in Barley. Front. Plant Sci. 7:776. doi: 10.3389/fpls.2016.00776
Dong, Y., Chen, H. W., Gao, J. L., Liu, Y. M., Li, J., and Wang, J. (2019). Bioactive ingredients in Chinese herbal medicines that target non-coding RNAs: promising new choices for disease treatment. Front. Pharmacol. 10:515. doi: 10.3389/fphar.2019.00515
Ebert, M. S., Neilson, J. R., and Sharp, P. A. (2007). MicroRNA sponges: competitive inhibitors of small RNAs in mammalian cells. Nat. Methods 4, 721–726. doi: 10.1038/nmeth1079
Franco-Zorrilla, J. M., Valli, A., Todesco, M., Mateos, I., Puga, M. I., Rubio-Somoza, I., et al. (2007). Target mimicry provides a new mechanism for regulation of microRNA activity. Nat. Genet. 39, 1033–1037. doi: 10.1038/ng2079
Frydrych Capelari, E., da Fonseca, G. C., Guzman, F., and Margis, R. (2019). Circular and micro RNAs from Arabidopsis thaliana flowers are simultaneously isolated from AGO-IP libraries. Plants 8:302. doi: 10.3390/plants8090302
Fu, X. Z., Zhang, X. Y., Qiu, J. Y., Zhou, X., Yuan, M., He, Y. Z., et al. (2019). Whole-transcriptome RNA sequencing reveals the global molecular responses and ceRNA regulatory network of mRNAs, lncRNAs, miRNAs and circRNAs in response to copper toxicity in Ziyang Xiangcheng (Citrus junos Sieb. Ex Tanaka). BMC Plant Biol. 19:509. doi: 10.1186/s12870-019-2087-1
Gao, Y., Wang, J., and Zhao, F. (2015). CIRI: an efficient and unbiased algorithm for de novo circular RNA identification. Genome Biol. 16:4. doi: 10.1186/s13059-014-0571-3
Gao, Z., Li, J., Luo, M., Li, H., Chen, Q. J., Wang, L., et al. (2019). Characterization and cloning of grape circular RNAs identified the cold resistance-related Vv-circATS1. Plant Physiol. 180, 966–985. doi: 10.1104/pp.18.01331
Ghorbani, A., Izadpanah, K., Peters, J. R., Dietzgen, R. G., and Mitter, N. (2018). Detection and profiling of circular RNAs in uninfected and maize Iranian mosaic virus-infected maize. Plant Sci. 274, 402–409. doi: 10.1016/j.plantsci.2018.06.016
Grapp, M., Wrede, A., Schweizer, M., Huwel, S., Galla, H. J., Snaidero, N., et al. (2013). Choroid plexus transcytosis and exosome shuttling deliver folate into brain parenchyma. Nat. Commun. 4:2123. doi: 10.1038/ncomms3123
Guo, J. U., Agarwal, V., Guo, H. L., and Bartel, D. P. (2014). Expanded identification and characterization of mammalian circular RNAs. Genome Biol. 15:409. doi: 10.1186/s13059-014-0409-z
Hansen, T. B., Jensen, T. I., Clausen, B. H., Bramsen, J. B., Finsen, B., Damgaard, C. K., et al. (2013). Natural RNA circles function as efficient microRNA sponges. Nature 495, 384–388. doi: 10.1038/nature11993
Hansen, T. B., Veno, M. T., Damgaard, C. K., and Kjems, J. (2016). Comparison of circular RNA prediction tools. Nucleic Acids Res. 44:e58. doi: 10.1093/nar/gkv1458
He, X., Guo, S., Wang, Y., Wang, L., Shu, S., and Sun, J. (2019). Systematic identification and analysis of heat-stress-responsive lncRNAs, circRNAs and miRNAs with associated co-expression and ceRNA networks in cucumber (Cucumis sativus L.). Physiol. Plant. 168, 736-54. doi: 10.1111/ppl.12997
Ivanov, A., Memczak, S., Wyler, E., Torti, F., Porath, H. T., Orejuela, M. R., et al. (2015). Analysis of intron sequences reveals hallmarks of circular RNA biogenesis in animals. Cell Rep. 10, 170–177. doi: 10.1016/j.celrep.2014.12.019
Jeck, W. R., and Sharpless, N. E. (2014). Detecting and characterizing circular RNAs. Nat. Biotechnol. 32, 453–461. doi: 10.1038/nbt.2890
Jeck, W. R., Sorrentino, J. A., Wang, K., Slevin, M. K., Burd, C. E., Liu, J. Z., et al. (2013). Circular RNAs are abundant, conserved, and associated with ALU repeats. RNA 19, 141–157. doi: 10.1261/rna.035667.112
Kelly, S., Greenman, C., Cook, P. R., and Papantonis, A. (2015). Exon skipping is correlated with exon circularization. J. Mol. Biol. 427, 2414–2417. doi: 10.1016/j.jmb.2015.02.018
Kramer, M. C., Liang, D. M., Tatomer, D. C., Gold, B., March, Z. M., Cherry, S., et al. (2015). Combinatorial control of Drosophila circular RNA expression by intronic repeats, hnRNPs, and SR proteins. Genes Dev. 29, 2168–2182. doi: 10.1101/gad.270421.115
Kristensen, L. S., Andersen, M. S., Stagsted, L. V. W., Ebbesen, K. K., Hansen, T. B., and Kjems, J. (2019). The biogenesis, biology and characterization of circular RNAs. Nat. Rev. Genet. 20, 675–691. doi: 10.1038/s41576-019-0158-7
Legnini, I., Di Timoteo, G., Rossi, F., Morlando, M., Briganti, F., Sthandier, O., et al. (2017). Circ-ZNF609 is a circular RNA that can be translated and functions in myogenesis. Mol. Cell 66, 22–37e.29. doi: 10.1016/j.molcel.2017.02.017
Li, P. F., Chen, S. C., Chen, H. L., Mo, X. Y., Li, T. W., Shao, Y. F., et al. (2015). Using circular RNA as a novel type of biomarker in the screening of gastric cancer. Clin. Chim. Acta 444, 132–136. doi: 10.1016/j.cca.2015.02.018
Li, T. W., Shao, Y. F., Fu, L. Y., Xie, Y., Zhu, L. W., Sun, W. L., et al. (2018). Plasma circular RNA profiling of patients with gastric cancer and their droplet digital RT-PCR detection. J. Mol. Med. 96, 85–96. doi: 10.1007/s00109-017-1600-y
Liang, D. M., and Wilusz, J. E. (2014). Short intronic repeat sequences facilitate circular RNA production. Genes Dev. 28, 2233–2247. doi: 10.1101/gad.251926.114
Liu, S., Wang, Q. J., Li, X. Y., Wang, G. B., and Wan, Y. L. (2019). Detecting of chloroplast circular RNAs in Arabidopsis thaliana. Plant Signal Behav. 14:1621088. doi: 10.1080/15592324.2019.1621088
Lu, T. T., Cui, L. L., Zhou, Y., Zhu, C. R., Fan, D. L., Gong, H., et al. (2015). Transcriptome-wide investigation of circular RNAs in rice. RNA 21, 2076–2087. doi: 10.1261/rna.052282.115
Lukiw, W. J. (2013). Circular RNA (circRNA) in Alzheimer's disease (AD). Front. Genet. 4:307. doi: 10.3389/fgene.2013.00307
Luo, Z., Han, L. Q., Qian, J., and Li, L. (2019). Circular RNAs exhibit extensive intraspecific variation in maize. Planta 250, 69–78. doi: 10.1007/s00425-019-03145-y
Lv, L., Yu, K., Lu, H., Zhang, X., Liu, X., Sun, C., et al. (2020). Transcriptome-wide identification of novel circular RNAs in soybean in response to low-phosphorus stress. PLoS ONE 15:e0227243. doi: 10.1371/journal.pone.0227243
Maheshwari, Y., Selvaraj, V., Hajeri, S., and Yokomi, R. (2017). Application of droplet digital PCR for quantitative detection of Spiroplasma citri in comparison with real time PCR. PLoS One 12:e0184751. doi: 10.1371/journal.pone.0184751
Memczak, S., Jens, M., Elefsinioti, A., Torti, F., Krueger, J., Rybak, A., et al. (2013). Circular RNAs are a large class of animal RNAs with regulatory potency. Nature 495, 333–338. doi: 10.1038/nature11928
Meng, X., Chen, Q., Zhang, P., and Chen, M. (2017). CircPro: an integrated tool for the identification of circRNAs with protein-coding potential. Bioinformatics 33, 3314–3316. doi: 10.1093/bioinformatics/btx446
Meng, X., Hu, D., Zhang, P., Chen, Q., and Chen, M. (2019). CircFunBase: a database for functional circular RNAs. Database 2019:baz003. doi: 10.1093/database/baz003
Meng, X. W., Zhang, P. J., Chen, Q., Wang, J. J., and Chen, M. (2018). Identification and characterization of ncRNA-associated ceRNA networks in Arabidopsis leaf development. BMC Genom. 19:607. doi: 10.1186/s12864-018-4993-2
Meyer, K. D., Patil, D. P., Zhou, J., Zinoviev, A., Skabkin, M. A., Elemento, O., et al. (2015). 5' UTR m(6)a promotes cap-independent translation. Cell 163, 999–1010. doi: 10.1016/j.cell.2015.10.012
Nigro, J. M., Cho, K. R., Fearon, E. R., Kern, S. E., Ruppert, J. M., Oliner, J. D., et al. (1991). Scrambled exons. Cell 64, 607–613. doi: 10.1016/0092-8674(91)90244-S
Pamudurti, N. R., Bartok, O., Jens, M., Ashwal-Fluss, R., Stottmeister, C., Ruhe, L., et al. (2017). Translation of circRNAs. Mol Cell 66:e27. doi: 10.1016/j.molcel.2017.02.021
Pan, T., Sun, X. Q., Liu, Y. X., Li, H., Deng, G. B., Lin, H. H., et al. (2018). Heat stress alters genome-wide profiles of circular RNAs in Arabidopsis. Plant Mol. Biol. 96, 217–229. doi: 10.1007/s11103-017-0684-7
Qin, M. L., Liu, G., Huo, X. S., Tao, X. M., Sun, X. M., Ge, Z. H., et al. (2016). Hsa_circ_0001649: a circular RNA and potential novel biomarker for hepatocellular carcinoma. Cancer Biomark. 16, 161–169. doi: 10.3233/CBM-150552
Ren, Y. Z., Yue, H. F., Li, L., Xu, Y. H., Wang, Z. Q., Xin, Z. Y., et al. (2018). Identification and characterization of circRNAs involved in the regulation of low nitrogen-promoted root growth in hexaploid wheat. Biol. Res. 51:43. doi: 10.1186/s40659-018-0194-3
Rybak-Wolf, A., Stottmeister, C., Glazar, P., Jens, M., Pino, N., Giusti, S., et al. (2015). Circular RNAs in the mammalian rain are highly abundant, conserved, and dynamically expressed. Mol. Cell 58, 870–885. doi: 10.1016/j.molcel.2015.03.027
Salzman, J., Gawad, C., Wang, P. L., Lacayo, N., and Brown, P. O. (2012). Circular RNAs are the predominant transcript isoform from hundreds of human genes in diverse cell types. PLoS ONE 7:e30733. doi: 10.1371/journal.pone.0030733
Satoh, K., Fukumoto, Y., Sugimura, K., Miura, Y., Aoki, T., Nochioka, K., et al. (2013). Plasma cyclophilin A is a novel biomarker for coronary artery disease. Circ. J. 77, 447–455. doi: 10.1253/circj.CJ-12-0805
Starke, S., Jost, I., Rossbach, O., Schneider, T., Schreiner, S., Hung, L. H., et al. (2015). Exon circularization requires canonical splice signals. Cell Rep. 10, 103–111. doi: 10.1016/j.celrep.2014.12.002
Sun, X. Y., Wang, L., Ding, J. C., Wang, Y. R., Wang, J. S., Zhang, X. Y., et al. (2016). Integrative analysis of Arabidopsis thaliana transcriptomics reveals intuitive splicing mechanism for circular RNA. FEBS Lett. 590, 3510–3516. doi: 10.1002/1873-3468.12440
Suzuki, H., Zuo, Y., Wang, J., Zhang, M. Q., Malhotra, A., and Mayeda, A. (2006). Characterization of RNase R-digested cellular RNA source that consists of lariat and circular RNAs from pre-mRNA splicing. Nucleic Acids Res. 34:e63. doi: 10.1093/nar/gkl151
Szabo, L., Morey, R., Palpant, N. J., Wang, P. L., Afari, N., Jiang, C., et al. (2015). Statistically based splicing detection reveals neural enrichment and tissue-specific induction of circular RNA during human fetal development. Genome Biol. 16:126. doi: 10.1186/s13059-015-0690-5
Szabo, L., Morey, R., Palpant, N. J., Wang, P. L., Afari, N., Jiang, C., et al. (2016). Statistically based splicing detection reveals neural enrichment and tissue-specific induction of circular RNA during human fetal development (vol 16, 126, 2016). Genome Biol. 17:126. doi: 10.1186/s13059-016-1123-9
Szczesniak, M. W., Kabza, M., Pokrzywa, R., Gudys, A., and Makalowska, I. (2013). ERISdb: a database of plant splice sites and splicing signals. Plant Cell Physiol. 54:e10. doi: 10.1093/pcp/pct001
Tang, C., Xie, Y., Yu, T., Liu, N., Wang, Z., Woolsey, R. J., et al. (2020). m(6)A-dependent biogenesis of circular RNAs in male germ cells. Cell Res. 30, 211–228. doi: 10.1038/s41422-020-0279-8
Veno, M. T., Hansen, T. B., Veno, S. T., Clausen, B. H., Grebing, M., Finsen, B., et al. (2015). Spatio-temporal regulation of circular RNA expression during porcine embryonic brain development. Genome Biol. 16:245. doi: 10.1186/s13059-015-0801-3
Wang, H. Y., Wang, H. H., Zhang, H. X., Liu, S., Wang, Y. S., Gao, Y. B., et al. (2019). The interplay between microRNA and alternative splicing of linear and circular RNAs in eleven plant species. Bioinformatics 35, 3119–3126. doi: 10.1093/bioinformatics/btz038
Wang, J., Lin, J., Wang, H., Li, X., Yang, Q., Li, H., et al. (2018). Identification and characterization of circRNAs in Pyrus betulifolia bunge under drought stress. PLoS ONE 13:e0200692. doi: 10.1371/journal.pone.0200692
Wang, J. Y., Yang, Y. W., Jin, L. M., Ling, X. T., Liu, T. L., Chen, T. Z., et al. (2018). Re-analysis of long non-coding RNAs and prediction of circRNAs reveal their novel roles in susceptible tomato following TYLCV infection. BMC Plant Biol. 18:104. doi: 10.1186/s12870-018-1332-3
Wang, K., Singh, D., Zeng, Z., Coleman, S. J., Huang, Y., Savich, G. L., et al. (2010). MapSplice: accurate mapping of RNA-seq reads for splice junction discovery. Nucleic Acids Res. 38:e178. doi: 10.1093/nar/gkq622
Wang, K., Wang, C., Guo, B., Song, K., Shi, C., Jiang, X., et al. (2019). CropCircDB: a comprehensive circular RNA resource for crops in response to abiotic stress. Database 2019:baz053. doi: 10.1093/database/baz053
Wang, P. L., Bao, Y., Yee, M. C., Barrett, S. P., Hogan, G. J., Olsen, M. N., et al. (2014). Circular RNA is expressed across the Eukaryotic tree of life. PLoS ONE 9:90859. doi: 10.1371/journal.pone.0090859
Wang, W. H., Wang, J. L., Wei, Q. Z., Li, B. Y., Zhong, X. M., Hu, T. H., et al. (2019). Transcriptome-wide identification and characterization of circular RNAs in leaves of Chinese cabbage (Brassica rapa L. ssp. pekinensis) in response to calcium deficiency-induced tip-burn. Sci. Rep. 9:14544. doi: 10.1038/s41598-019-51190-0
Wang, Y., Yang, M., Wei, S., Qin, F., Zhao, H., and Suo, B. (2016). Identification of circular RNAs and their targets in leaves of Triticum aestivum L. under dehydration stress. Front. Plant Sci. 7:2024. doi: 10.3389/fpls.2016.02024
Wang, Y. S., Gao, Y. B., Zhang, H. X., Wang, H. H., Liu, X. Q., Xu, X., et al. (2019). Genome-wide profiling of circular RNAs in the rapidly growing shoots of moso bamboo (Phyllostachys edulis). Plant Cell Physiol. 60, 1354–1373. doi: 10.1093/pcp/pcz043
Wang, Z. P., Liu, Y. F., Li, D. W., Li, L., Zhang, Q., Wang, S. B., et al. (2017). Identification of circular RNAs in kiwifruit and their species-specific response to bacterial canker pathogen invasion. Front. Plant Sci. 8:413. doi: 10.3389/fpls.2017.00413
Westholm, J. O., Miura, P., Olson, S., Shenker, S., Joseph, B., Sanfilippo, P., et al. (2014). Genome-wide analysis of Drosophila circular RNAs reveals their structural and sequence properties and age-dependent neural accumulation. Cell Rep. 9, 1966–1980. doi: 10.1016/j.celrep.2014.10.062
Xiang, L. X., Cai, C. W., Cheng, J. R., Wang, L., Wu, C. F., Shi, Y. Z., et al. (2018). Identification of circularRNAs and their targets in Gossypium under Verticillium wilt stress based on RNA-seq. PeerJ 6:e4500. doi: 10.7717/peerj.4500
Yang, X. S., Wu, J., Ziegler, T. E., Yang, X., Zayed, A., Rajani, M. S., et al. (2011). Gene expression biomarkers provide sensitive indicators of in planta nitrogen status in maize. Plant Physiol. 157, 1841–1852. doi: 10.1104/pp.111.187898
Yang, Y., Fan, X., Mao, M., Song, X., Wu, P., Zhang, Y., et al. (2017). Extensive translation of circular RNAs driven by N(6)-methyladenosine. Cell Res. 27, 626–641. doi: 10.1038/cr.2017.31
Yang, Y. B., Gao, X. Y., Zhang, M. L., Yan, S., Sun, C. J., Xiao, F. Z., et al. (2018). Novel role of FBXW7 circular RNA in repressing glioma tumorigenesis. J. Natl Cancer Inst. 110, 304–315. doi: 10.1093/jnci/djx166
Yang, Z., Li, W., Su, X., Ge, P., Zhou, Y., Hao, Y., et al. (2019). Early response of radish to heat stress by strand-specific transcriptome and miRNA analysis. Int. J. Mol. Sci. 20:3321. doi: 10.3390/ijms20133321
Ye, C. Y., Chen, L., Liu, C., Zhu, Q. H., and Fan, L. J. (2015). Widespread noncoding circular RNAs in plants. N. Phytol. 208, 88–95. doi: 10.1111/nph.13585
Ye, C. Y., Zhang, X. C., Chu, Q. J., Liu, C., Yu, Y. Y., Jiang, W. Q., et al. (2017). Full-length sequence assembly reveals circular RNAs with diverse non-GT/AG splicing signals in rice. RNA Biol. 14, 1055–1063. doi: 10.1080/15476286.2016.1245268
Ye, J., Wang, L., Li, S., Zhang, Q., Zhang, Q., Tang, W., et al. (2019). AtCircDB: a tissue-specific database for Arabidopsis circular RNAs. Brief. Bioinform. 20, 58–65. doi: 10.1093/bib/bbx089
Zeng, X., Lin, W., Guo, M., and Zou, Q. (2017). A comprehensive overview and evaluation of circular RNA detection tools. PLoS Comput. Biol. 13:e1005420. doi: 10.1371/journal.pcbi.1005420
Zhang, G. Y., Diao, S. F., Zhang, T., Chen, D. G., He, C. Y., and Zhang, J. G. (2019). Identification and characterization of circular RNAs during the sea buckthorn fruit development. RNA Biol. 16, 354–361. doi: 10.1080/15476286.2019.1574162
Zhang, M., Huang, N., Yang, X., Luo, J., Yan, S., Xiao, F., et al. (2018). A novel protein encoded by the circular form of the SHPRH gene suppresses glioma tumorigenesis. Oncogene 37, 1805–1814. doi: 10.1038/s41388-017-0019-9
Zhang, P., Fan, Y., Sun, X. P., Chen, L., Terzaghi, W., Bucher, E., et al. (2019). A large-scale circular RNA profiling reveals universal molecular mechanisms responsive to drought stress in maize and Arabidopsis. Plant J. 98, 697–713. doi: 10.1111/tpj.14267
Zhang, P., Meng, X., Chen, H., Liu, Y., Xue, J., Zhou, Y., et al. (2017). PlantCircNet: a database for plant circRNA-miRNA-mRNA regulatory networks. Database 2017:bax089. doi: 10.1093/database/bax089
Zhang, X. O., Wang, H. B., Zhang, Y., Lu, X., Chen, L. L., and Yang, L. (2014). Complementary sequence-mediated exon circularization. Cell 159, 134–147. doi: 10.1016/j.cell.2014.09.001
Zhang, Y., Zhang, X. O., Chen, T., Xiang, J. F., Yin, Q. F., Xing, Y. H., et al. (2013). Circular intronic long noncoding RNAs. Mol. Cell 51, 792–806. doi: 10.1016/j.molcel.2013.08.017
Zhao, J., Lee, E. E., Kim, J., Yang, R., Chamseddin, B., Ni, C., et al. (2019). Transforming activity of an oncoprotein-encoding circular RNA from human papillomavirus. Nat. Commun. 10:2300. doi: 10.1038/s41467-019-10246-5
Zhao, T., Wang, L. Y., Li, S., Xu, M., Guan, X. Y., and Zhou, B. L. (2017). Characterization of conserved circular RNA in polyploid gossypium species and their ancestors. FEBS Lett. 591, 3660–3669. doi: 10.1002/1873-3468.12868
Zhao, W., Cheng, Y. H., Zhang, C., You, Q. B., Shen, X. J., Guo, W., et al. (2017). Genome-wide identification and characterization of circular RNAs by high throughput sequencing in soybean. Sci. Rep. 7:5636. doi: 10.1038/s41598-017-05922-9
Zhao, Z., Li, X., Jian, D., Hao, P., Rao, L., and Li, M. (2017). Hsa_circ_0054633 in peripheral blood can be used as a diagnostic biomarker of pre-diabetes and type 2 diabetes mellitus. Acta Diabetol. 54, 237–245. doi: 10.1007/s00592-016-0943-0
Zhou, C., Molinie, B., Daneshvar, K., Pondick, J. V., Wang, J. K., Van Wittenberghe, N., et al. (2017). Genome-wide maps of m6A circRNAs identify widespread and cell-type-specific methylation patterns that are distinct from mRNAs. Cell Rep. 20, 2262–2276. doi: 10.1016/j.celrep.2017.08.027
Zhu, X. L., Wang, X. Y., Wei, S. Z., Chen, Y., Chen, Y., Fan, X. B., et al. (2017). hsa_circ_0013958: a circular RNA and potential novel biomarker for lung adenocarcinoma. Febs J. 284, 2170–2182. doi: 10.1111/febs.14132
Zhu, Y. X., Jia, J. H., Yang, L., Xia, Y. C., Zhang, H. L., Jia, J. B., et al. (2019). Identification of cucumber circular RNAs responsive to salt stress. BMC Plant Biol. 19:164. doi: 10.1186/s12870-019-1712-3
Zuo, J., Wang, Y., Zhu, B., Luo, Y., Wang, Q., and Gao, L. (2018). Analysis of the coding and non-coding RNA transcriptomes in response to bell pepper chilling. Int. J. Mol. Sci. 19:2001. doi: 10.3390/ijms19072001
Zuo, J. H., Wang, Q., Zhu, B. Z., Luo, Y. B., and Gao, L. P. (2016). Deciphering the roles of circRNAs on chilling injury in tomato. Biochem. Biophys. Res. Commun. 479, 132–138. doi: 10.1016/j.bbrc.2016.07.032
Keywords: circRNA, plant, circularization, characterization, regulation, stress response, bioinformatics
Citation: Zhang P, Li S and Chen M (2020) Characterization and Function of Circular RNAs in Plants. Front. Mol. Biosci. 7:91. doi: 10.3389/fmolb.2020.00091
Received: 02 March 2020; Accepted: 22 April 2020;
Published: 19 May 2020.
Edited by:
Florian Karreth, Moffitt Cancer Center, United StatesReviewed by:
Alfredo Berzal-Herranz, Instituto de Parasitología y Biomedicina López-Neyra (IPBLN), SpainSunny Sharma, Harvard Medical School, United States
Copyright © 2020 Zhang, Li and Chen. This is an open-access article distributed under the terms of the Creative Commons Attribution License (CC BY). The use, distribution or reproduction in other forums is permitted, provided the original author(s) and the copyright owner(s) are credited and that the original publication in this journal is cited, in accordance with accepted academic practice. No use, distribution or reproduction is permitted which does not comply with these terms.
*Correspondence: Ming Chen, bWNoZW5Aemp1LmVkdS5jbg==