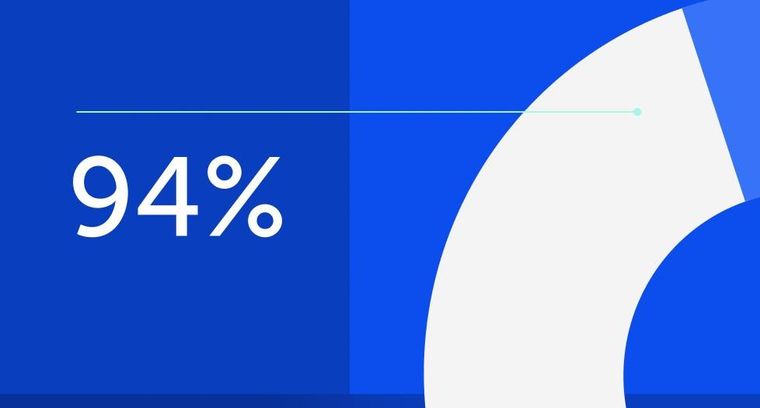
94% of researchers rate our articles as excellent or good
Learn more about the work of our research integrity team to safeguard the quality of each article we publish.
Find out more
REVIEW article
Front. Microbiomes, 16 January 2024
Sec. Host and Microbe Associations
Volume 2 - 2023 | https://doi.org/10.3389/frmbi.2023.1345330
This article is part of the Research TopicLive Biotherapeutic Products: Where Are We?View all 8 articles
Lactobacillus johnsonii is a commensal bacterium that has been isolated from vaginal and gastrointestinal (GI) tracts of vertebrate hosts, including humans, rodents, swine, and poultry. Lactobacillus-based probiotic supplements are popular because of the health advantages they offer. Species such as L. johnsonii are particularly interesting due to their potential health-promoting properties. Here, we reviewed the research on specific strains of L. johnsonii that have been studied in the context of health and disease and delved into the underlying mechanisms that aid in preserving host homeostasis. The utilization of L. johnsonii strains has been widely linked to numerous health benefits in the host. These include pathogen antagonism, control of mucosal and systemic immune responses, reduction of chronic inflammation, modulation of metabolic disorders, and enhanced epithelial barrier. These findings suggest that L. johnsonii plays a critical role in maintaining host homeostasis, highlighting its potential as a probiotic.
Lactobacillus johnsonii is a Gram-positive, homofermentative, non-spore-forming rod-shaped host-adapted bacterium (Zheng et al., 2020) with lactic acid being its predominant end product from sugar metabolism (Lebeer et al., 2008). Several strains of this species have been isolated from vaginal and gastrointestinal (GI) tracts of vertebrate hosts, including humans, rodents, swine, and poultry (Ravi et al., Pridmore et al., 2004; Leonard et al., 2014; Wu et al., 2016; Guerrero-Preston et al., 2017; Dec et al., 2018; Zhang et al., 2019; Ahire et al., 2021; Reed et al., 2022). The abundance of this bacterium in various niches is often influenced by external factors such as diet, antibiotic treatment, and invading microbes (Mason et al., 2012a; Mason et al., 2012b; Antonissen et al., 2016; Thompson et al., 2023). L. johnsonii, like other well-known Lactobacillus species, is of particular interest due to its potential health-promoting properties, which mark this specie as a probiotic candidate, defined by the FAO/WHO as ““live microorganisms which, when administered in adequate amounts, confer a health benefit on the host” (Hill et al., 2014).
As a commensal bacterium, L johnsonii needs to survive, colonize, multiply and exert its function in the acidic and high bile concentrated conditions in the gut (Stavropoulou and Bezirtzoglou, 2020). For these purposes it has developed resistance and tolerance mechanisms against stressors, while also competing with other indigenous microbes in this niche (O’Flaherty et al., 2018; Zhang et al., 2019; Stavropoulou and Bezirtzoglou, 2020; Bagon et al., 2021). L. johnsonii is surrounded by an outer packaged protein shell called S layer. In addition, extracellular peptidoglycan, teichoic acids, and capsular and exo-polysaccharides help to protect and keep cellular integrity and adherence to the host, while the mechanism for stress sensing and export systems complemented the stress resistance machinery (Lebeer et al., 2008). Furthermore, L. johnsonii can adapt to the host’s nutritional environment because its genome encodes a high number of the phosphotransferase system (PTS) and ATP-binding cassette (ABC) transporters as well as amino acid protease and peptidases that enable the uptake and utilization of a variety of sugars and amino acids available in the host GI tract microenvironment (Fujisawa et al., 1992; Lebeer et al., 2008; Zhang et al., 2019; Boucard et al., 2022). In vitro studies have shown that L. johnsonii L531 can produce higher levels of short-chain fatty acid (SCFA) (butyric acid, acetic acid) and lactic acid, having an impact on the metabolic profile and the gut resident microbiota (He et al., 2019). These metabolites are known to promote the maturation of the host immune system and regulate the onset and progression of inflammatory responses (Rooks and Garrett, 2016; Richards et al., 2016).
The inter-strain variations in carbohydrate utilization profile, as well as cell wall composition, determine L. johnsonii’s health-promoting and immunomodulatory properties (Fujisawa et al., 1992; Zhang et al., 2019; Schar-Zammaretti and Ubbink, 2003; Guinane et al., 2011). As a result, while L. johnsonii is a good probiotic candidate, the different strains of this specie must be independently investigated per the Food and Agriculture Organization of the United Nations (FAO), which guidelines demand to include the source of isolation, characterization, and a credible case presented for their health effects, to be called ‘probiotic’ (Hill et al., 2014).
L. johnsonii strains such as NCC 533 (also known as La1) is a commercially available probiotic. Several studies, including in vitro, animal models, and clinical trials have shown NCC 533 binding properties to host mucosal cells, as well as its ability to inhibit gut pathogens, stimulate the immune system and metabolic functions, enhance the mucosal barrier and improve human intestinal microbiota (Neeser et al., 2000; Granato et al., 2004; Pridmore et al., 2004; Bergonzelli et al., 2006; Yamano et al., 2006; Inoue et al., 2007; Denou et al., 2008). Several of these health-promoting activities are also observed in other L. johnsonii strains when administered to different animal models (La Ragione et al., 2004; Kingma et al., 2011; Fonseca et al., 2017; He et al., 2019; Charlet et al., 2020; Zou et al., 2020). Notably, the survival capacity and safety of L. johnsonii strains N6.2 and 456 supplementation have been studied in healthy human volunteers (Marcial et al., 2017; Davoren et al., 2019). L. johnsonii strain N6.2 is under clinical trials for its probiotic effect on Type I diabetes (T1D) onset in children, adolescents, and adults (Clinical Trial: NCT03961854, 2019-2023; Clinical Trial: NCT03961347, 2020-2026), while L. johnsonii strain MH-68 have shown promising results in the glycemic control and immunomodulation (Wang et al., 2022).
This review summarizes the existing scientific literature on the mechanisms by which L. johnsonii affects health and disease progression. Our goal is to comprehend the effects of L. johnsonii on various health outcomes.
Different regions of the GI tract, such as the mouth, stomach, small intestine, and colon, have unique environmental conditions, including variations in pH, nutrients availability, and oxygen levels. These variations created distinct niches for different microorganisms to thrive (Thursby and Juge, 2017). Scientists are studying how gut bacteria affect health and its potential role in treating gastrointestinal disorders (Bidell et al., 2022). L. johnsonii strains as probiotics have been shown to enhance gut health in humans and animals (Marcial et al., 2017; Yang et al., 2022b; Yang et al., 2022c). Microbes can colonize various regions of the GI tract and impact other microbial communities throughout the entire digestive system.
The intestinal epithelial barrier regulates immunity, nutrient absorption, digestion, and hormone production as well as metabolic processes (Lee et al., 2018). The tight junction (TJ) complex between epithelial cells maintains the intestinal barrier, regulates selective paracellular transit of ions, water, and solutes, and limits the transit of microorganisms, food allergens, and macromolecules (Lynch and Pedersen, 2016; Lee et al., 2018). Several studies have demonstrated the capacity of different L. johnsonii strains such as MG, L531, BS15, and 135-1-CHN to enhance the barrier function by upregulating TJ related genes (ZO-1, Occludin, and Claudin-1) (Xin et al., 2014; Liu et al., 2015; Mu et al., 2017; Chen et al., 2021; Lyu et al., 2023), as well as direct interaction with the Junctional Adhesion Molecule-2 (JAM-2) (Bai et al., 2022). Postnatal administration of L. johnsonii N6.2 to T1D-prone rats showed no morphological differences between groups in the structure of the villus however, an upregulated expression of claudin-1 and decreased expression of occludin was observed in the L. johnsonii-supplemented group, as well as decreased intestinal pro-inflammatory response, showing the ability of L. johnsonii N6.2 to ameliorate the intestinal barrier dysfunction (Valladares et al., 2010). The oral administration of L. johnsonii promoted the activation of the TLR1/2-STAT3 pathway and increased the number of anti-inflammatory macrophages, leading to IL-10 release and improvement of DSS-induced colitis in mice (Jia et al., 2022). In contrast, clinical studies evaluating the effect L. johnsonii NCC 533 supplementation in patients after intestinal resection for Chron’s disease reported that L. johnsonii NCC 533 failed to prevent endoscopic recurrence after six months (Marteau et al., 2006; Van Gossum et al., 2007). These studies demonstrated the potential benefits and limitations of L. johnsonii in improving intestinal barrier function and reducing epithelial inflammation (Figure 1).
Figure 1 Local health benefits conferred by L. johnsonii administration. (A) L. johnsonii secretes metabolites like lactic acid, hydrogen peroxide, antimicrobial peptides, and bile salt hydrolases (BSH) that facilitate pathogen inhibition and improved gut microbiome function. L. johnsonii also inhibits pathogen-induced activation NLRP3 inflammasome via inhibition of TLR4-mediated signaling and promotion of autophagy. It interacts with epithelial cells and repairs barrier function by increasing the expression of tight junction proteins like claudin and occludin. L. johnsonii also has immunomodulatory functions. For example, it stimulates dendritic cells (DC), resulting in downstream modulation of both pro-and anti-inflammatory cytokine secretion and thus mediating a Th1/Th2/Treg immune balance response. (B) L. jonhsonii colonize the vagina of healthy women were display its antifungal properties to promote a healthy vaginal microbiota. Created with BioRender.com.
Oral microbiota equilibrium can be affected by inflammatory conditions, such as periodontitis (Manos, 2022). It has been reported that oral pathobiont Porphyromonas gingivalis is highly expanded during chronic periodontitis and is associated with several inflammatory disorders, from atherosclerosis to colitis. It plays an important role in establishing and expanding gut pathobionts, highlighting the importance of the oral-gut axis in the development of GI tract pathologies (Kitamoto et al., 2020). Lactobacillus bacteria and specifically L. johnsonii strains, have been used as an alternative approach to the control of pathobionts associated with periodontitis and dental cavities because of their anti-biofilm activity, which alters the ability of pathobionts to colonize (Jaffar et al., 2016; Giordani et al., 2021). Controlling oral pathogens and oral inflammatory diseases could also impact individuals’ gut microbiota composition and overall health (Imai et al., 2021).
Extensive research on various L. johnsonii strains demonstrates the pathogen-inhibiting property of this bacterium in the GI tract, often via secretion of antimicrobial molecules, lowering the pH of the environment and competing for similar niches. The supplementation of L. johnsonii has modulated several intestinal pathogens, such as Helicobacter pylori, Salmonella spp., pathogenic Escherichia coli, and Clostridium perfringes (Figure 2).
Figure 2 Mechanism of interaction between L. johnsonii and commensal and pathogenic bacteria. As a host-associated species, L. johnsonii interacts with the resident microbiota as well as invading pathogens to establish stable colonization in the niche. This interaction is multifaceted and involves both secreted and cell surface molecules of L. johnsonii. L. johnsonii produces bacteriocins and bacteriolysis that target Lactobacillus and Enterococcal species in a strain-specific manner. Weak acids like lactic acid produced by L. johnsonii act together with hydrogen peroxide, inhibiting and killing enteric, vaginosis-associated, and uropathogenic pathogens like pathogenic E. coli, S. typhimurium and Gardnerella vaginalis. Several cell surface structures of L. johnsonii are also involved in antimicrobial activities. GroEL is a surface-associated protein that triggers aggregation of H. pylori upon secretion by L. johnsonii. This aggregation is hypothesized to cause the rapid exclusion of H. pylori from the GI tract upon L. johnsonii administration. Similarly, S- layer protein on L. johnsonii cell surface can inhibit S. sonnei growth. Other cell surface-associated structures like EPS, LTA, EF-t,u, and specific carbohydrate-binding receptors – all involved the in adhesion of L. johnsonii to epithelial cells and mucin – are hypothesized to play a role in competing with commensal microbes and pathogens for mucosal binding sites. L. johnsonii also inhibits the growth of other pathogens like S. enteritidis and reduces gut persistence of E. coli and C. perfringens through a yet unknown mechanism. Created with BioRender.com.
The ability of L. johnsonii to inhibit Helicobacter pylori infection has been widely studied. Supplementation of L. johnsonii in animal models infected with H. pylori, resulted in reduced pathogen load, mobility, and aggregation in the gastric mucosa (Sgouras et al., 2005; Isobe et al., 2012; Aiba et al., 2015; Aiba et al., 2019). L. johnsonii encode for and secretes a cell surface structure protein named GroEL, which triggers H. pylori aggregation under in vitro conditions, (Bergonzelli et al., 2006). This interaction could lead to the rapid exclusion of H. pylori observed in different in vivo studies (Sgouras et al., 2005; Isobe et al., 2012; Aiba et al., 2015; Aiba et al., 2019). A clinical trial using L. johnsonii Lj1 fermented milk in H. pylori-positive volunteers showed reduced antral gastritis, inflammatory score in the gastric mucosa, and decreased density of H. pylori (Pantoflickova et al., 2003). In contrast, oral supplementation with L. johnsonii NCC 533 supernatants did not control H. pylori persistence in humans (Michetti et al., 1999). However, heat killed/lyophilized as well as viable L. johnsonii No.1088. were shown to reduce gastrin-mediated acid production, by decreasing the number of gastrin-positive cells in mice stomach (Aiba et al., 2015) and its combination with anti-H. pylori urease immunoglobulin Y (IgY) significantly reduced H. pylori infection(Aiba et al., 2019). More studies are needed to determine the necessity of viable bacteria to report a positive effect of L johnsonii in H. pylori treatment.
In addition to pathogen exclusion, L. johnsonii supplemented mice resulted in reduced H. pylori-related inflammation by diminished gastric mucosa inflammatory leukocyte (neutrophils, lymphocytes, macrophages) infiltration and proinflammatory chemokine and cytokine expression (macrophage inflammatory protein 2, keratinocyte-derived cytokine) (Sgouras et al., 2005). Additional in vitro studies showed that the incubation of H. pylori-infected human adenocarcinoma AGS cell lines with L. johnsonii NCC 533 cultures supernatants reduced the expression of H. pylori-induced IL-8, without affecting the bacterial viability (Sgouras et al., 2005). These studies showed the immunomodulatory effect of L. johnsonii in the control of H. pylori-related inflammation.
Current therapies for H. pylori infection include antimicrobial agents and inhibitors of gastric acid secretion, such as proton pump inhibitors (PPI) and vonoprazan. In a mouse model, these drugs decreased the population ratio of L. johnsonii (Nadatani et al., 2019). Interestingly, L. johnsonii supplementation in a model of indomethacin-induced small intestinal damage in combination with PPI or vonopazan, protects mice from intestinal injury (Nadatani et al., 2019). These data illustrate the distinct characteristics of L. johnsonii and its potential used as part of therapeutic protocols to alleviate the adverse effects of medications and synergistically reduce detrimental bacterial growth and tissue inflammation.
Different studies suggest that L. johnsonii L531 has the potential to control other intestinal pathogens, such as Salmonella sp. (He et al., 2019; Xia et al., 2020; Yang et al., 2020; Chen et al., 2021; Yang et al., 2022b). Oral supplementation with L. johnsonii L531 to newly weaned piglets, one week before challenged with Salmonella enteric serovar Infantis, reduced diarrhea severity, intestinal inflammation, and tissue damage. The modulation of the inflammatory response led to epithelial protection and reduced abundance of Salmonella in the ileum mucosa (He et al., 2019; Yang et al., 2022b). The protective effects of L. johnsonii on Salmonella sp. immunopathogenesis, have been associated with the inhibition of the NOD pathway, the modulation of endoplasmic reticulum stress, and the promotion of autophagy degradation (Yang et al., 2020; Yang et al., 2022b); as well as the regulation of NLRC4 and NLRP3 inflammasome, proinflammatory cytokines expression via NFκB signaling and inhibition of mitochondrial damage (Xia et al., 2020; Chen et al., 2021).
In silico studies identified three potential gene products in L. johnsonii NCC 533 genome that may catalyze the known antimicrobial factor hydrogen peroxide (H2O2) synthesis. L. johnsonii NCC 533 and other L. johnsonii strains produced H2O2, which is hypothesized to play a role in the elimination of Salmonella enterica serovar Typhimurium SL1344 in vitro (Pridmore et al., 2008). Additionally, it has been suggested that H2O2 and lactic acid produced by L. johnsonii act co-operatively to kill enteric, vaginosis-associated, and uropathogenic pathogens, such as enteric pathogenic E. coli, S. typhimurium and Gardnerella vaginalis (Atassi and Servin, 2010). Acidification of the microenvironment is an anti-microbial mechanism employed by several lactic acid bacteria (LAB). Lactic acid and other weak acids produced by lactobacilli have been known to exhibit pathogen-inhibitory function by reducing the pH in the surrounding environment (Peter, 1993; Servin, 2004). Interestingly, L. johnsonii NCC 533 inhibits Salmonella enterica serovar Typhimurium SL1344 growth only at a low pH of 4.5, but not at pH 6.5 (Fayol-Messaoudi et al., 2005) (Figure 3).
Figure 3 Mechanisms of interaction between L. johnsonii and Candida sp. L. johnsonii antagonizes the growth of C. albicans both in vitro and in the GI tract via secreted and cell surface molecules. Chitinase and glucanase-like hydrolytic enzymes secreted by L. johnsonii can degrade the fungal cell wall, causing rapid decreases in Candida viability during co-culture. Acidification of the niche due to weak acids and other soluble metabolites produced by L. johnsonii can inhibit the formation of Candida biofilms and disrupt established C. albicans biofilm structure. Strains of L. johnsonii also encode the surfactin gene, a biosurfactant that prevents biofilm formation and inhibits C. albicans adhesion. Additionally, L. johnsonii biofilm structure and production of SCFAs like butyric acid can inhibit C. albicans hyphal morphogenesis, thereby affecting its pathogenicity. Finally, L. johnsonii and C. albicans co-aggregate in vitro, a characteristic hypothesized to interfere with adherence and trigger rapid exclusion. Created with BioRender.com.
L. johnsonii NCC 533 has been shown to control pathogens by producing bile-salt-hydrolase (BSH) (Travers et al., 2016). This enzyme hydrolyzes the amino bonds of conjugated bile salts to generate deconjugated bile salts (cholic, deoxycholic, and chenodeoxycholic acids) (Begley et al., 2006; Travers et al., 2016). It has been shown that BSH might play a role in antiparasitic activity against Giardia sp., a protozoan intestinal parasite that causes giardiasis, by inhibiting the proliferation of Giardia sp. trophozoites (Travers et al., 2016; Allain et al., 2017). The BSH present in the supernatants of L. johnsonii NCC 533 prevent Giardia sp. growth in vitro by converting bile’s non-toxic components into highly toxic components to Giardia sp. (Travers et al., 2016). Furthermore, mice treated with recombinant BSH during Giardia duodenalis infection presented decreased numbers of trophozoites in the small intestine, showing the antiparasitic effect of the BSH-L enzyme and suggesting that the mechanism by which L. johnsonii controls intestinal parasite infection is through the production of specific metabolic enzymes (Allain et al., 2017).
L. johnsonii can prevent the adhesion and cell invasion of several diarrheagenic bacteria, including enteropathogenic E. coli (EPEC), enterotoxigenic E. coli (ETEC), Yersinia pseudotuberculosis and Salmonella typhimurium, to intestinal epithelial cells (Bernet et al., 1994; Liu et al., 2015). This broad inhibitory effect of L. johnsonii strains was initially attributed to the non-specific steric interference of receptors needed for pathogen colonization. However, there is evidence suggesting the involvement of a more direct inhibitory mechanism by a recent work of Zang et al., which showed that the S-layer protein of L. johnsonii F0421 inhibited Shigella sonnei adhesion to HT-29 cells (Zhang et al., 2012). Thus, L. johnsonii strains can regulate the colonization of intestinal pathogens by controlling their adherence to the mucosal epithelium.
L. johnsonii has also been shown to provide protection against Citrobacter rodentium-induced colitis in an animal model by modulating the innate immune signaling pathways, as well as inflammatory responses and ER stress (Zhang et al., 2021). L. johnsonii administration in abiotic mice did not abrogate Campylobacter sp. jejune growth but reduced the expression of pro-inflammatory cytokines (such as IL-6, MCP1, and TNF) in the intestinal tract (Bereswill et al., 2017). L. johnsonii NJ3 supplementation of mice infected with enterohemorrhagic E. coli increased the diversity of the intestinal microbiota and improve the diarrhea index, body weight, and liver index (Hu et al., 2021).In vitro studies have shown that L. johnsonii L531 inhibit NLRP3 activity by promoting autophagy leading to reduced Escherichia coli-induced cell damage (Zou et al., 2020).
In the last decade, the number of antibiotic-resistant pathogenic bacteria and the search for alternative therapies to help control bacterial infections have increased. Probiotics, as well as fecal transplantation from healthy individuals, is an alternative therapy for the treatment of antibiotic-resistant bacteria and for re-establishing healthy gut microbiota in individuals with chronic diseases (Reyman et al., 2022). Studies by Ekmekciu et al. compared the efficacy of fecal microbiota transplantation (FMT) from healthy mice to oral supplementation with L. johnsonii in mice subjected to broad-spectrum antibiotic treatment for eight weeks. The antibiotic treatment diminished immune cell populations in the intestine, mesenteric lymph nodes, and spleen. In contrast, after antibiotic treatment, FMT and L. johnsonii supplementation increased CD4+, CD8+, and regulatory T cells (Tregs) cells in the small intestine and the spleen. Treatment with L. johnsonii also maintains colonic IL-10 production (Ekmekciu et al., 2017). This study showed the potential of L. johnsonii supplementation in individuals with dysbiosis caused by antibiotic treatment and its use as a therapeutic intervention for bacterial infection with an antibiotic-resistant phenotype.
Gut microbiota dysbiosis can exacerbate intestinal fungal infections, and Candida sp. is the most frequent cause of yeast infection (Charlet et al., 2020; Jawhara, 2022). L. johnsonii and Bacteroides thetaiotaomicron interact with Candida sp. and promote fungal cell wall degradation via chitinase-like and mannosidase-like activity, inhibiting fungal growth (Charlet et al., 2020). It has been shown that the administration of these two bacterial during DSS-induced colitis controlled the growth of pathogenic E. coli, Enterococcus faecalis, and Candida glabrata in the intestine, intestinal inflammation by downregulating intestinal IL-1β, TLR9, and NF-κB activation and upregulating IL-10 (Charlet et al., 2020). In a different approach, Bertolini et al. observed that changes in the microbial composition and function induced by dietary sucrose generated an increased abundance of Lactobacillus sp. and decreased Candida albicans burden in a murine model of oropharyngeal candidiasis during immunosuppression (Bertolini et al., 2021). The same authors showed that L. johnsonii MT-LB4 has an inhibitory effect on Enterococcus faecalis and planktonic Candida albicans growth in vitro (Bertolini et al., 2021). Furthermore, the production of oleic acid and palmitic acid by L. johnsonii during interaction with colonic epithelial cells has been associated with anti-inflammatory and antifungal properties in a DSS- induced colitis mice model (Charlet et al., 2022). Studies have shown that L. johnsonii MT4 exhibited pH-dependent and pH-independent antagonistic interactions with C. albicans, by inhibiting its growth and biofilm formation via nutrient competition and the production of metabolites with anticandidal activity with a similar sequence to antifungal compounds, such as Bacillomycin D, Surfactin, glucanase, and Msp1/p75 (Vazquez-Munoz et al., 2022). L. johnsonii JCM1022 inhibits C. albicans hyphal morphogenesis in vitro, via butyric acid production (Tang et al., 2010). Therefore, L. johnsonii in the GI tract can control the growth of fungal pathogens and hinder biofilm formation through L. johnsonii-derived metabolites, as well as anti-inflammatory and anti-fungal properties (Figure 3).
Dysbiosis of the gut microbiota has been hypothesized to promote autoimmune disorders, such as type 1 diabetes (TD1) (Chagwedera et al., 2019). T1D results from the destruction of insulin-producing β cells via autoreactive T cells, which affects the self-regulation of blood sugar in the body. Notably, T and B-cell-deficient rodents fail to develop T1D, even when carrying predisposing genetic mutations (Christianson et al., 1993). Evidence shows that the resident gut microbiota is involved in the progression of T1D and that altering the gut microbiota using probiotics can be a therapeutic tool to help manage T1D (Vaarala et al., 2008; Dovi et al., 2022).
It has been observed that rats which spontaneously develop TD1 due to genetic predisposition (BioBreeding diabetes-prone rats -BBDP), have increased susceptibility to infections (Roesch et al., 2009). One of the differences between BBDP rats and BioBreeding diabetes-resistant rats (BBDR) is their gut microbiota, specifically, Lactobacillus and Bifidobacterium abundance, which are dominant bacterial communities that negatively correlated with the onset of T1D (Lai et al., 2009; Roesch et al., 2009; Valladares et al., 2010). Interestingly, oral administration of L. johnsonii N6.2 to BBDP rats, decreased the incidence of diabetes by altering intestinal microbiota, decreasing the host intestinal oxidative stress response, and modifying the intestinal pro-inflammatory response, while Lactobacillus reuteri fails to mediate the resistance to T1D (Valladares et al., 2010). This was further accompanied with changes in dendritic cell phenotype that contributed to the Th17 lymphocyte’s immune polarization in mesenteric lymph nodes and spleen (Lau et al., 2011).In addition, it was described that L. johnsonii N6.2 derived lipids promoted a tolerogenic-migratory DC-like phenotype that could enhance regulatory T cells responses and prevent the initiation of the autoimmune process (Cuaycal et al., 2023). TLR9 activation seems to be implicated in this polarizing-tolerogenic mechanism (Kingma et al., 2011). In addition, to reshape the Treg/Th17 commitment, L. johnsonii N6.2 can modulate the assembly of the inflammasome, evidenced by lower levels of mature caspase-1 in BBDP rats (Teixeira et al., 2018). Immunoregulatory properties of L. johnsonii N6.2 derived H2O2 abolished the activity of the rate-limiting enzyme for tryptophan catabolism, indoleamine 2,3-dioxygenase (IDO) known by its capacity to induce the proinflammatory cytokine IFNγ (Valladares et al., 2013). A pilot clinical study with this strain supports the safety and tolerance of L. johnsonii N6.2 administration in healthy humans’ patients (Marcial et al., 2017). However, few clinical studies have supported the benefits of probiotic supplementation in patients with T1D (Dovi et al., 2022). In a clinical study, patients diagnosed with T1D (onset age 6 to 18 years old) were supplemented daily for 60 days with placebo or a capsule containing active probiotics including L. johnsonii MH-68. The probiotics mix had a positive impact on glycemic and glycated hemoglobin levels in the blood, increased the presence of beneficial bacteria species, such as Bifidobacterium animalis, Akkermansia muciniphila and Lactobacillus salivarius and reduced inflammatory cytokines in the serum of patients with TD1. Glycemic control and immunomodulation persisted 3 months after stopped probiotics intake (Wang et al., 2022). Although probiotics cannot cure T1D, they can help manage symptoms and be used as a supportive treatment for T1D and other autoimmune diseases.
Furthermore, it has been suggested that L. johnsonii can release bioactive molecules with immunomodulatory effects (Harrison et al., 2021). Microbial extracellular vesicles have been reported in feces, blood, and urine and show different patterns depending on the individual’s health status. There is an increasing interest in studying these microbial extracellular vesicles as possible biomarkers for disease assessment and as immunomodulators of disease over the use of live organism (Park et al., 2021; Diez-Sainz et al., 2022; Yang et al., 2022a). L. johnsonii N6.2-derived nanovesicles (NV10) are rich in glycerophosphoglycerols and contain several unique and differentially expressed proteins compared to the bacteria cellular membrane (Harrison et al., 2021). L. johnsonii N6.2 extracellular vesicles could upregulate IL-10 expression in macrophages, promoting the M2 tolerogenic phenotype through STAT3 activation, while in the human pancreatic cell line Blox5, it reduced cytokine-induced apoptosis (Teixeira et al., 2022). L. johnsonii N6.2 derived phospholipids modified bone marrow-derived dendritic cells (BMDCs) transcriptional signature, triggering the expression of anti-inflammatory cytokine Il10 (Cuaycal et al., 2023), suggesting that L. johnsonii N6.2 nanovesicles’ phospholipids components might have an immunomodulatory function. Interestingly, human pancreatic islets treated in vitro with L. johnsonii N6.2 extracellular vesicles showed significant upregulation of the expression of glucose transporter Solute Carrier Family 2, Member 6 (SLC2A6), also known as glucose transporter 6 (GLUT6), suggesting that L. johnsonii can induce glucose uptake by pancreatic islets under high glucose conditions, and increase insulin secretion (Teixeira et al., 2022). These studies showed possible mechanisms by which L. johnsonii generated changes at a location distant to the gut via extracellular vesicle and possible mechanisms of L. johnsonii N6.2 to attenuate the onset of T1D by immunoregulation (Figure 4).
Figure 4 Systemic health benefits conferred by L. johnsonii administration. L. johnsonii immunomodulatory properties are related to its capacity to alter microbiota composition and function, changing the bacterial communities’ metabolites profile. In addition, L. johnsonii express and produce substances and release vesicles with local and systemic anti-inflammatory and metabolic effects that modulate susceptibility to Th2 (allergic) responses and RSV infection in the airways and impacted onset and clinical manifestations of autoimmune and metabolic diseases. Created with BioRender.com.
It is important to investigate the effects of L. johnsonii-derived extracellular vesicles and live L. johnsonii to understand the different outcomes generated among them and the specific role of the extracellular vesicles in the modulation of inflammatory responses and autoimmune diseases.
Metabolic diseases due to poor diets and obesity also cause significant disease manifestations. Diet can affect gut microbiota composition and function, as well as metabolic processes that can lead to the development of metabolic syndrome, cardiovascular disease, and type 2 diabetes (Ginsberg and MacCallum, 2009; O’Toole and Shiels, 2020; Wicinski et al., 2020). Recent studies have evaluated the effect of L. johnsonii N6.2 supplementation in a high-fat diet (HFD) rat model to induce metabolic syndrome. The authors observed that L. johnsonii N6.2 in combination with phytophenols reduced mTORC1-activating phosphorylation of AKT and other genes expression downstream mTORC1 signaling pathway in HFD-fed females (Kling et al., 2018). mTOR and AKT functions are associated with glucose and lipid metabolism, which are involved in metabolic syndrome (Saxton and Sabatini, 2017), suggesting that L. johnsonii N6.2 supplementation may help to diminish fat deposition and could modulate the development of the metabolic syndrome.
The close relationship between the gut microbiome and obesity has been extensively studied (Wicinski et al., 2020). An elevated prevalence of obesity worldwide is associated with increased non-alcoholic fatty liver disease (NAFLD) (Wong and Ahmed, 2014). A study by Jinge et al. investigated the effect of L. johnsonii BS15 administration on the development of NAFLD in obese male mice. The authors observed that L. johnsonii BS15 supplementation protected mice from hepatic steatosis and hepatocyte apoptosis when exposed to a HFD. The protective effect was attributed to enhanced liver antioxidative defense, as well as inhibition of insulin resistance and decreased expression of acetyl-CoA carboxylase 1, fatty acid synthase, and peroxisome proliferator-activated receptor γ. Long-term alterations were observed in the gut microbiota of obese mice, with an increased abundance of Lactobacillus sp. and specifically L. johnsonii after 63 days of supplementation. After 119 days of probiotic supplementation with L. johnsonii, obese mice showed decreased serum LPS levels, and reduced intestinal permeability and pro-inflammatory response by downregulating TNFα expression (Xin et al., 2014). These studies confirm the crucial role of the microbiome in maintaining metabolic homeostasis in the host and reducing associated illnesses, as well as the long-term effect of L. johnsonii supplementation in the gut microbiota composition and functionof host metabolism and inflammatory status (Figure 4).
The vaginal microbiome is a dynamic ecosystem influenced by external or environmental stressors (sexual activity and personal hygiene) and intrinsic physiological conditions, such as hormonal changes, sexual development, pregnancy, and disease states. Lactobacillus sp. is the most abundant microorganism in the vaginal bacterial community and is a well-known pH acidifier (Greenbaum et al., 2019). Although recent data suggest that bacterial vaginosis (BV) results from polymicrobial disruption of the vaginal microbiota, the alkaline pH in BV patients has been related to decreased lactic acid production by Lactobacillus sp. (Greenbaum et al., 2019). One of the Lactobacillus specie that colonized the vagina and intestine of healthy women is L. Johnsonii (Dobrut et al., 2018). L. johnsonii UBLJ01, isolated from the vagina of healthy women, was found to inhibit the growth of Gardnerella vaginalis, Proteus mirabilis, and Candida albicans (Ahire et al., 2021). The therapeutic effects of L. johnsonii B-2178 and Lactobacillus acidophilus were tested in a rat model of vulvovaginal candidiasis and observed that both lactobacilli reduced C. albicans vaginal load and hyphae formation and significantly reduced proinflammatory cytokines IL-17 and IFNγ. Interestingly, only L. johnsonii B-2178 protected the vaginal mucosa epithelium from histopathological changes (Elfeky et al., 2023), suggesting that the presence of L. johnsonii in the reproductive tract may help to control the growth of pathogens and maintain a healthy environment (Figure 1).
Clinical studies during the prenatal, perinatal, and infant periods are relevant since infancy is a critical period when the human microbiome starts to establish, and alterations in the microbiome composition during early life can impact overall host homeostasis and promote the development of disease risk factors. In the last decade, there has been increased interest in studying the short- and long-term effects of pre- and post-natal microbiome alterations in mothers and newborns (Fujimura et al., 2016; Fonseca et al., 2017; Fonseca et al., 2021). The study of maternal and infant microbiomes is an opportunity to explore the critical role of L. johnsonii in physiological outcomes during pregnancy and the infant’s health.
Supplementing with probiotics during pregnancy can alter the composition of the gut and vaginal microbiota, breastmilk microbes, impact mother and infant immunity, and types of molecules that can be passed to the newborn (Rautava et al., 2012; Kuang and Jiang, 2020; Fonseca et al., 2021; Lehtoranta et al., 2022). A study evaluating the effect of prenatal supplementation with L. johnsonii MR1 in mice observed changes in the gut microbiota and the systemic metabolic profile of supplemented mothers and their offspring. Offspring from L. johnsonii-supplemented mothers showed an expansion of bacteria belonging to Lachnospiraceae and Muribaculaceae families, similar to L. johnsonii-supplemented mothers. In addition, the systemic metabolic profile of mothers and offspring, as well as the mother’s breastmilk metabolic profile, displayed similarity in the decreased presence of inflammatory metabolites (9,10-dihydroxyoctadecenoic acid (DiHOME), linoleic acid metabolite, and guanosine) (Fonseca et al., 2021). Similar metabolite changes were found in clinical studies with birth cohorts and showed that increases in systemic metabolites, such as DiHOME were associated with severe allergic disease in children (Fujimura et al., 2016). Likewise, clinical studies have shown that prenatal probiotic supplementation prevents infection, preterm delivery during pregnancy, and the manifestation of GI disorders and allergic responses in newborns (Baldassarre et al., 2018; Navarro-Tapia et al., 2020) (Figure 4).
To our knowledge, there are no clinical studies evaluating the effects of L. johnsonii administration during pregnancy. However, the presence of Lactobacillus gasseri/Lactobacillus johnsonii in the vagina of pregnant women has been associated with a decreased risk of early preterm birth (Tabatabaei et al., 2019). These data from animal models and clinical studies emphasize the potential role of L. johnsonii in women’s reproductive health, including controlling pathogens and promoting healthy pregnancies. Additionally, early-life L. johnsonii exposure may be critical in establishing a healthy microbiome. Thus, the study of prenatal L. johnsonii supplementation represents an opportunity to assess the potential benefits in mothers and infants. Testing its use prenatally in mothers with vaginal dysbiosis and postnatally in infants born via C-section could be especially interesting.
The gut-lung axis concept postulates that alterations in the gut microbiota affect lung homeostasis. A correlation between the composition of the gut and lung microbiota from birth to adulthood suggests an interconnection. Altering the gut microbiome affects lung immunity and microbiota composition (Markey et al., 2018; Yagi et al., 2022). This could also be an effect generated by systemic microbiome-derived metabolites or even the previously described extracellular vesicles.
Exposure to environmental factors impacts the gut microbiome composition and has been associated with increased risk of asthma development (Fujimura et al., 2016; Yagi et al., 2022). Early-life exposure to livestock or pets significantly diversifies the gut microbiome and reduces allergy and asthma risk, highlighting the link between environment and microbiome composition and function (Ownby et al., 2002; von Mutius and Vercelli, 2010). House dust from dog owners was found to confer protection against ovalbumin and cockroach allergen-induced airway diseases when orally administered to mice (Fujimura et al., 2014). Notably, this protection in mice models was associated with an increased abundance of L. johnsonii MR1 in the gut (Fujimura et al., 2014; Ravi et al., 2023). Mice supplemented with L. johnsonii MR1 before an airway-allergen or respiratory syncytial virus (RSV) challenge presented reduced Th2-airway-related immune response and reduced mucus deposition in the airways (Fujimura et al., 2014; Fonseca et al., 2017). This effect was related to an attenuated proinflammatory phenotype in dendritic cells and increased pulmonary Treg cells due to altered systemic metabolic profile (Fujimura et al., 2014; Fonseca et al., 2017). Furthermore, maternal L. johnsonii MR1 supplementation protected the neonates from severe RSV immunopathology, presenting a significant decrease in airway mucus deposition, Th2 cytokines production, as well as reduced numbers of innate lymphocyte cells 2 (ILC2) and CD4+ T cells in the lung. Furthermore, offspring born from L. johnsonii-supplemented mothers maintain the immunomodulatory effect until adulthood. Adult offspring were infected with RSV and showed reduced RSV immunopathology, suggesting that prenatal L. johnsonii supplementation impacts mother and offspring gut microbiome composition and function and metabolic profiles that might alter long-term the mucosal and systemic immune response (Fonseca et al., 2021). This study emphasizes the importance of the mother’s microbiome and the transfer of gut microbiota and immune-modulatory metabolites from mother to offspring to control allergic disease and respiratory pathogens during infancy (Fonseca et al., 2021). Pre- and post-natal probiotics have been recommended for patients with a high risk of developing allergic diseases (Fiocchi et al., 2015). Overall, these studies emphasize the importance of the gut microbiota (gut-lung axis) in maintaining respiratory health by delivering metabolites, regulating metabolism, improving immune system maturation, and possibly lung development. L. johnsonii may improve lung health and modulate the immune response to pathogens. Clinical studies are needed to assess its potential use in controlling inflammation in the respiratory tract (Figure 4).
Similar to the gut, skin microorganisms play an essential role in educating the cutaneous innate and adaptive immune response, and skin microbiota dysbiosis has been associated with skin diseases (Byrd et al., 2018), suggesting that manipulation of skin microbiota could help control skin pathologies, such as atopic dermatitis (AD) and eczema. Interestingly, reshaping of the gut microbiota, metabolic functions, and immune responses by oral probiotic interventions has been proposed to positively impact the clinical manifestations of inflammatory skin disorders such as AD (Fang et al., 2021). However, AD patients have skin dysbiosis characterized by a high prevalence of Staphylococcus aureus (Brussow, 2016) and a lower presence of Lactobacillus species in the skin, as well as increased abundance of Clostridium difficile and bifidobacterial species in the gut (Melli et al., 2020). A connection between the gut microbiome and the skin microorganism community has been suggested, which could potentially impact the immune response of patients who have inflammatory skin conditions.
The benefits of altering skin microbiota by directly applying pre-and probiotics have been reviewed previously (Al-Ghazzewi and Tester, 2014). The microbe-microbe interactions and immunological action of a topical lotion containing heat-treated L. johnsonii NCC 533 were assessed in an in vitro reconstructed human epidermis (RHE) model. Non-replicative L. johnsonii NCC 533 reduced Staphylococcus aureus colonization and boosted cutaneous innate immunity by inducing the expression of antimicrobial peptides, such as cathelicidin and β-defensin (Rosignoli et al., 2018). In addition, the topical use of heat-killed L. johnsonii NCC 533 in 21 patients with AD and swab positive for Staphylococcus aureus, reduced S. aureus load and the AD overall score in an open-label, multicenter clinical study (Blanchet-Rethore et al., 2017). These studies showed an alternative use of L. johnsonii to control skin pathogens and boost the skin innate immune response. The authors pointed out the importance of non-replicating bacteria in this interaction with the host and argued that heat-killed L. johnsonii NCC 533 maintains its ability to stimulate cytokine production and induce the expression of antimicrobial peptides. It is possible that heat-killed L. johnsonii activates innate immune receptors by interacting directly with the skin epithelial cells in a TLR2-dependent but TLR4/MD-2-independent manner (Elson et al., 2007), helping to control Staphylococcus aureus growth. It is important to note that this intervention is not considered probiotic-mediated, as it does not contain live L. johnsonii. The interconnected nature of skin and gut microbiome interactions has not been thoroughly examined; however, they likely interact through their influence on local and systemic immune responses. Additional research is needed to better understand the potential skin health benefits of L. johnsonii, offering a valuable research opportunity.
Recent findings have highlighted the importance of probiotics for cancer treatment (Slizewska et al., 2021). The gut microbiome’s composition and function are linked to clinical response to immunotherapy for antitumor treatment (Weersma et al., 2020). Furthermore, a reproducible shift in bacterial richness and metabolic pathways has been consistently identified across different cohorts of individuals with colorectal cancer, which opens the possibility of using microbial signatures as biomarkers for intestinal cancer (Thomas et al., 2019). Microbiome-derived metabolites, such as short-chain fatty acids (SCFA), decreased inflammation and cancer cell proliferation (Ocadiz-Ruiz et al., 2017), and regulate the onset and progression of inflammatory responses (Richards et al., 2016). L. johnsonii is essential for influencing intestinal microbiota composition and metabolic activity, producing compounds with anticarcinogenic activity, stimulating the immune system, and modulating cell proliferation and apoptosis (Slizewska et al., 2021). Interestingly, in vitro and in vivo studies have shown that L. johnsonii L531 can produce high levels of SCFA, such as butyric, acetic, and lactic acids, affecting the metabolic profile and gut resident microbiota (He et al., 2019). Additionally, a comprehensive analysis of operational taxonomic units (OTU) in a mouse model of ataxia-telangiectasia, a genetic disorder associated with B cell lymphoma, showed that the less cancer-prone mouse colony had higher L. johnsonii colonization. Short-term restorative oral treatment with L. johnsonii RS-1 decreased systemic genotoxicity and inflammatory state in mice prone to developing cancer by diminishing hepatic T and NK cells, pro-inflammatory cytokines IL-1β and IFN-β levels, and elevated anti-inflammatory cytokines TGF-β and IL-10 (Yamamoto et al., 2013). This study shows the capacity of L. johnsonii strains to regulate the inflammatory response in cancer, like other beneficial bacteria that decrease inflammation and cancer cell proliferation and possibly modulate the efficacy of anticancer therapy (Lee et al., 2021). However, the mechanism by which each probiotic intervention exerts its anticarcinogenic activity must be clarified.
L. johnsonii is a commensal bacterium that has been isolated from vaginal and gastrointestinal (GI) tracts of vertebrate hosts, including humans, rodents, swine, and poultry. Lactobacillus-based probiotic supplements are popular because of the health advantages they offer and species such as L. johnsonii are of particular interest due to their potential health-promoting properties. L. johnsonii possesses exceptional properties that help it to maintain homeostasis in the host by controlling the expansion of pathogens, modulating metabolic pathways, and regulating the immune response systemically and locally. The modulation and restoration of healthy microbiota by L. johnsonii offer positive outcomes and represent an important tool to aid treatments and control specific pathologies’ development by directly modulating microbiota composition and function and, consequently, local and systemic immune responses. While several of these health-beneficial properties have been investigated in vitro settings and animal models (Table 1), there is still insufficient scientific evidence in humans to support these claims (Table 2). Studying the microbiomes of pregnant women and their infants presents an opportunity to investigate the significant role of L. johnsonii in impacting physiological outcomes and infant health. Hence, to validate the efficiency of L. johnsonii as a therapeutic probiotic, it is necessary to conduct more randomized clinical trials that encompass diverse populations, including individuals of different sexes, ages, and dietary habits. Other important parameters to consider include health status, underlying diseases or conditions, dosage, route and frequency of administration, the location of the study, and ensuring an adequate sample size for accuracy (Dronkers et al., 2020).
These trials should also adhere to intent-to-treat principles, conduct prospective evaluation, and use an adequate control group (Evans, 2010; Lim and In, 2019) to generate scientific evidence of the mechanism of action of L. johnsonii and validate its benefit during health and disease.
LA: Writing – original draft, Writing – review & editing. KR: Writing – original draft, Writing – review & editing. GH: Funding acquisition, Writing – original draft, Writing – review & editing. NL: Funding acquisition, Writing – original draft, Writing – review & editing. WF: Writing – original draft, Writing – review & editing.
The author(s) declare financial support was received for the research, authorship, and/or publication of this article. This work was funded by National Institutes of Health grants R01HL138013 (NWL), RO1AI138348 (NWL, GBH), and R35HL150682 (NWL). WF was supported by the Parker B. Francis Foundation Fellowship (21-PAF02113).
The authors declare that the research was conducted in the absence of any commercial or financial relationships that could be construed as a potential conflict of interest.
All claims expressed in this article are solely those of the authors and do not necessarily represent those of their affiliated organizations, or those of the publisher, the editors and the reviewers. Any product that may be evaluated in this article, or claim that may be made by its manufacturer, is not guaranteed or endorsed by the publisher.
AD, atopic dermatitis; BV, bacterial vaginosis; BSH-L, bile-salt-hydrolase; BBDP, BioBreeding diabetes-prone rats; BBDR, BioBreeding diabetes-resistant rats; BMDC, bone marrow-derived dendritic cells; COPD, chronic obstructive pulmonary disease; DHA, docosahexaenoic acid; DSS, dextran Sulfate Sodium; EHEC, enterohemorrhagic Escherichia coli; FMT, fecal microbiota transplantation; HFD, high-fat diet; LAB, lactic acid bacteria; LPS, lipopolysaccharides; Msp1/p75, major secreted protein 1/p75; MCP1, monocyte chemoattractant protein-1; NAFLD, non-alcoholic fatty liver disease; OUT, operational taxonomic units; RHE, reconstructed human epidermis; RSV, respiratory syncytial virus; TD1, Type 1 diabetes; TJ, tight junction; Treg, regulatory T cells.
Ahire J. J., Sahoo S., Kashikar M. S., Heerekar A., Lakshmi S. G., Madempudi R. S. (2021). In vitro assessment of Lactobacillus crispatus UBLCp01, Lactobacillus gasseri UBLG36, and Lactobacillus johnsonii UBLJ01 as a potential vaginal probiotic candidate. Probiotics Antimicrob. Proteins. 15 (2), 275–286. doi: 10.1007/s12602-021-09838-9
Aiba Y., Nakano Y., Koga Y., Takahashi K., Komatsu Y. (2015). A highly acid-resistant novel strain of Lactobacillus johnsonii No. 1088 has antibacterial activity, including that against Helicobacter pylori, and inhibits gastrin-mediated acid production in mice. Microbiologyopen 4, 465–474. doi: 10.1002/mbo3.252
Aiba Y., Umeda K., Rahman S., Nguyen S. V., Komatsu Y. (2019). Synergistic effect of anti-Helicobacter pylori urease immunoglobulin Y from egg yolk of immunized hens and Lactobacillus johnsonii No.1088 to inhibit the growth of Helicobacter pylori in vitro and in vivo. Vaccine 37, 3106–3112. doi: 10.1016/j.vaccine.2019.04.045
Al-Ghazzewi F. H., Tester R. F. (2014). Impact of prebiotics and probiotics on skin health. Beneficial Microbes 5, 99–107. doi: 10.3920/BM2013.0040
Allain T., Chaouch S., Thomas M., Vallee I., Buret A. G., Langella P., et al. (2017). Bile-salt-hydrolases from the probiotic strain lactobacillus johnsonii la1 mediate anti-giardial activity in vitro and in vivo. Front. Microbiol. 8, 2707. doi: 10.3389/fmicb.2017.02707
Antonissen G., Eeckhaut V., Van Driessche K., Onrust L., Haesebrouck F., Ducatelle R., et al. (2016). Microbial shifts associated with necrotic enteritis. Avian Pathol. 45, 308–312. doi: 10.1080/03079457.2016.1152625
Atassi F., Servin A. L. (2010). Individual and co-operative roles of lactic acid and hydrogen peroxide in the killing activity of enteric strain Lactobacillus johnsonii NCC933 and vaginal strain Lactobacillus gasseri KS120.1 against enteric, uropathogenic and vaginosis-associated pathogens. FEMS Microbiol. Lett. 304, 29–38. doi: 10.1111/j.1574-6968.2009.01887.x
Bagon B. B., Valeriano V. D. V., Oh J. K., Pajarillo E. A. B., Lee J. Y., Kang D. K. (2021). Exoproteome perspective on the bile stress response of Lactobacillus johnsonii. Proteomes 9, 10. doi: 10.3390/proteomes9010010
Bai Y. Y., Lyu M. Y., Fukunaga M., Watanabe S., Iwatani S., Miyanaga K., et al. (2022). Lactobacillus johnsonii enhances the gut barrier integrity via the interaction between GAPDH and the mouse tight junction protein JAM-2. Food Funct. 13, 11021–11033. doi: 10.1039/D2FO00886F
Baldassarre M. E., Palladino V., Amoruso A., Pindinelli S., Mastromarino P., Fanelli M., et al. (2018). Rationale of probiotic supplementation during pregnancy and neonatal period. Nutrients 10 (11), 1693. doi: 10.3390/nu10111693
Begley M., Hill C., Gahan C. G. (2006). Bile salt hydrolase activity in probiotics. Appl. Environ. Microbiol. 72, 1729–1738. doi: 10.1128/AEM.72.3.1729-1738.2006
Bereswill S., Ekmekciu I., Escher U., Fiebiger U., Stingl K., Heimesaat M. M. (2017). Lactobacillus johnsonii ameliorates intestinal, extra-intestinal and systemic pro-inflammatory immune responses following murine Campylobacter jejuni infection. Sci. Rep. 7 (1), 2138. doi: 10.1038/s41598-017-02436-2
Bergonzelli G. E., Granato D., Pridmore R. D., Marvin-Guy L. F., Donnicola D., Corthesy-Theulaz I. E. (2006). GroEL of Lactobacillus johnsonii La1 (NCC 533) is cell surface associated: potential role in interactions with the host and the gastric pathogen Helicobacter pylori. Infect. Immun. 74, 425–434. doi: 10.1128/IAI.74.1.425-434.2006
Bernet M. F., Brassart D., Neeser J. R., Servin A. L. (1994). Lactobacillus acidophilus La 1 binds to cultured human intestinal-cell lines and inhibits cell attachment and cell invasion by enterovirulent bacteria. Gut 35, 483–489. doi: 10.1136/gut.35.4.483
Bertolini M., Vazquez Munoz R., Archambault L., Shah S., Souza J. G. S., Costa R. C., et al. (2021). Mucosal bacteria modulate candida albicans virulence in oropharyngeal candidiasis. mBio 12, e0193721. doi: 10.1128/mBio.01937-21
Bidell M. R., Hobbs A. L. V., Lodise T. P. (2022). Gut microbiome health and dysbiosis: A clinical primer. Pharmacotherapy 42, 849–857. doi: 10.1002/phar.2731
Blanchet-Rethore S., Bourdes V., Mercenier A., Haddar C. H., Verhoeven P. O., Andres P. (2017). Effect of a lotion containing the heat-treated probiotic strain Lactobacillus johnsonii NCC 533 on Staphylococcus aureus colonization in atopic dermatitis. Clin. Cosmet Investig. Dermatol. 10, 249–257. doi: 10.2147/CCID.S135529
Boucard A. S., Florent I., Polack B., Langella P., Bermudez-Humaran L. G. (2022). Genome sequence and assessment of safety and potential probiotic traits of Lactobacillus johnsonii CNCM I-4884. Microorganisms 10, 273. doi: 10.3390/microorganisms10020273
Brussow H. (2016). Turning the inside out: the microbiology of atopic dermatitis. Environ. Microbiol. 18, 2089–2102. doi: 10.1111/1462-2920.13050
Byrd A. L., Belkaid Y., Segre J. A. (2018). The human skin microbiome. Nat. Rev. Microbiol. 16, 143–155. doi: 10.1038/nrmicro.2017.157
Chagwedera D. N., Ang Q. Y., Bisanz J. E., Leong Y. A., Ganeshan K., Cai J., et al. (2019). Nutrient sensing in CD11c cells alters the gut microbiota to regulate food intake and body mass. Cell Metab. 30, 364–373.e7. doi: 10.1016/j.cmet.2019.05.002
Charlet R., Bortolus C., Sendid B., Jawhara S. (2020). Bacteroides thetaiotaomicron and Lactobacillus johnsonii modulate intestinal inflammation and eliminate fungi via enzymatic hydrolysis of the fungal cell wall. Sci. Rep. 10 (1), 11510. doi: 10.1038/s41598-020-68214-9
Charlet R., Le Danvic C., Sendid B., Nagnan-Le Meillour P., Jawhara S. (2022). Oleic Acid and Palmitic Acid from Bacteroides thetaiotaomicron and Lactobacillus johnsonii Exhibit Anti-Inflammatory and Antifungal Properties. Microorganisms 10. doi: 10.3390/microorganisms10091803
Chen S., Li Y., Chu B., Yuan L., Liu N., Zhu Y., et al. (2021). Lactobacillus johnsonii L531 Alleviates the Damage Caused by Salmonella Typhimurium via Inhibiting TLR4, NF-kappaB, and NLRP3 Inflammasome Signaling Pathways. Microorganisms 9 (9), 1983. doi: 10.3390/microorganisms9091983
Christianson S. W., Shultz L. D., Leiter E. H. (1993). Adoptive transfer of diabetes into immunodeficient NOD-scid/scid mice. Relative contributions of CD4+ and CD8+ T-cells from diabetic versus prediabetic NOD.NON-Thy-1a donors. Diabetes 42, 44–55. doi: 10.2337/diab.42.1.44
Cuaycal A. E., Teixeira L. D., Lorca G. L., Gonzalez C. F. (2023). Lactobacillus johnsonii N6.2 phospholipids induce immature-like dendritic cells with a migratory-regulatory-like transcriptional signature. Gut Microbes 15, 2252447. doi: 10.1080/19490976.2023.2252447
Davoren M. J., Liu J., Castellanos J., Rodriguez-Malave N. I., Schiestla R. H. (2019). A novel probiotic, Lactobacillus johnsonii 456, resists acid and can persist in the human gut beyond the initial ingestion period. Gut Microbes 10, 458–480. doi: 10.1080/19490976.2018.1547612
Dec M., Nowaczek A., Stepien-Pysniak D., Wawrzykowski J., Urban-Chmiel R. (2018). Identification and antibiotic susceptibility of lactobacilli isolated from Turkeys. BMC Microbiol. 18, 168. doi: 10.1186/s12866-018-1269-6
Denou E., Pridmore R. D., Berger B., Panoff J. M., Arigoni F., Brussow H. (2008). Identification of genes associated with the long-gut-persistence phenotype of the Probiotic Lactobacillus johnsonii strain NCC533 using a combination of Genomics and transcriptome analysis. J. Bacteriol. 190, 3161–3168. doi: 10.1128/JB.01637-07
Diez-Sainz E., Milagro F. I., Riezu-Boj J. I., Lorente-Cebrian S. (2022). Effects of gut microbiota-derived extracellular vesicles on obesity and diabetes and their potential modulation through diet. J. Physiol. Biochem. 78, 485–499. doi: 10.1007/s13105-021-00837-6
Dobrut A., Gosiewski T., Pabian W., Bodaszewska-Lubas M., Ochonska D., Bulanda M., et al. (2018). The dynamics of vaginal and rectal Lactobacillus spp. flora in subsequent trimesters of pregnancy in healthy Polish women, assessed using the Sanger sequencing method. BMC Pregnancy Childbirth 18, 350. doi: 10.1186/s12884-018-1987-7
Dovi K. S., Bajinka O., Conteh I. (2022). Evidence and possible mechanisms of probiotics in the management of type 1 diabetes mellitus. J. Diabetes Metab. Disord. 21, 1081–1094. doi: 10.1007/s40200-022-01006-2
Dronkers T. M. G., Ouwehand A. C., Rijkers G. T. (2020). Global analysis of clinical trials with probiotics. Heliyon 6, e04467. doi: 10.1016/j.heliyon.2020.e04467
Ekmekciu I., Von Klitzing E., Neumann C., Bacher P., Scheffold A., Bereswill S., et al. (2017). Fecal Microbiota Transplantation, Commensal Escherichia coli and Lactobacillus johnsonii Strains Differentially Restore Intestinal and Systemic Adaptive Immune Cell Populations Following Broad-spectrum Antibiotic Treatment. Front. Microbiol. 8, 2430. doi: 10.3389/fmicb.2017.02430
Elfeky D. S., Awad A. R., Shamseldeen A. M., Mowafy H. L., Hosny S. A. (2023). Comparing the therapeutic potentials of Lactobacillus johnsonii vs. Lactobacillus acidophilus against vulvovaginal candidiasis in female rats: an in vivo study. Front. Microbiol. 14, 1222503. doi: 10.3389/fmicb.2023.1222503
Elson G., Dunn-Siegrist I., Daubeuf B., Pugin J. (2007). Contribution of Toll-like receptors to the innate immune response to Gram-negative and Gram-positive bacteria. Blood 109, 1574–1583. doi: 10.1182/blood-2006-06-032961
Evans S. R. (2010). Fundamentals of clinical trial design. J. Exp. Stroke Transl. Med. 3, 19–27. doi: 10.6030/1939-067X-3.1.19
Fang Z. F., Li L. Z., Zhang H., Zhao J. X., Lu W. W., Chen W. (2021). Gut microbiota, probiotics, and their interactions in prevention and treatment of atopic dermatitis: a review. Front. Immunol. 12. doi: 10.3389/fimmu.2021.720393
Fayol-Messaoudi D., Berger C. N., Coconnier-Polter M. H., Lievin-Le Moal V., Servin A. L. (2005). pH-, Lactic acid-, and non-lactic acid-dependent activities of probiotic Lactobacilli against Salmonella enterica serovar Typhimurium. Appl. Environ. Microbiol. 71, 6008–6013. doi: 10.1128/AEM.71.10.6008-6013.2005
Fiocchi A., Pawankar R., Cuello-Garcia C., Ahn K., Al-Hammadi S., Agarwal A., et al. (2015). World allergy organization-mcMaster university guidelines for allergic disease prevention (GLAD-P): probiotics. World Allergy Organ J. 8, 4. doi: 10.1186/s40413-015-0055-2
Fonseca W., Lucey K., Jang S., Fujimura K. E., Rasky A., Ting H. A., et al. (2017). Lactobacillus johnsonii supplementation attenuates respiratory viral infection via metabolic reprogramming and immune cell modulation. Mucosal Immunol. 10, 1569–1580. doi: 10.1038/mi.2017.13
Fonseca W., Malinczak C. A., Fujimura K., Li D., Mccauley K., Li J., et al. (2021). Maternal gut microbiome regulates immunity to RSV infection in offspring. J. Exp. Med. 218 (11), e20210235. doi: 10.1084/jem.20210235
Fujimura K. E., Demoor T., Rauch M., Faruqi A. A., Jang S., Johnson C. C., et al. (2014). House dust exposure mediates gut microbiome Lactobacillus enrichment and airway immune defense against allergens and virus infection. Proc. Natl. Acad. Sci. U.S.A. 111, 805–810. doi: 10.1073/pnas.1310750111
Fujimura K. E., Sitarik A. R., Havstad S., Lin D. L., Levan S., Fadrosh D., et al. (2016). Neonatal gut microbiota associates with childhood multisensitized atopy and T cell differentiation. Nat. Med. 22, 1187–1191. doi: 10.1038/nm.4176
Fujisawa T., Benno Y., Yaeshima T., Mitsuoka T. (1992). Taxonomic study of the Lactobacillus acidophilus group, with recognition of Lactobacillus gallinarum sp. nov. and Lactobacillus johnsonii sp. nov. and synonymy of Lactobacillus Acidophilus group A3 (Johnson et al. 1980) with the type strain of Lactobacillus amylovorus (Nakamura 1981). Int. J. Syst. Bacteriol. 42, 487–491. doi: 10.1099/00207713-42-3-487
Ginsberg H. N., MacCallum P. R. (2009). The obesity, metabolic syndrome, and type 2 diabetes mellitus pandemic: Part I. Increased cardiovascular disease risk and the importance of atherogenic dyslipidemia in persons with the metabolic syndrome and type 2 diabetes mellitus. J. Cardiometab Syndr. 4, 113–119. doi: 10.1111/j.1559-4572.2008.00044.x
Giordani B., Parolin C., Vitali B. (2021). Lactobacilli as anti-biofilm strategy in oral infectious diseases: a mini-review. Front. Med. Technol. 3, 769172. doi: 10.3389/fmedt.2021.769172
Granato D., Bergonzelli G. E., Pridmore R. D., Marvin L., Rouvet M., Corthesy-Theulaz I. E. (2004). Cell surface-associated elongation factor Tu mediates the attachment of Lactobacillus johnsonii NCC533 (La1) to human intestinal cells and mucins. Infect. Immun. 72, 2160–2169. doi: 10.1128/IAI.72.4.2160-2169.2004
Greenbaum S., Greenbaum G., Moran-Gilad J., Weintraub A. Y. (2019). Ecological dynamics of the vaginal microbiome in relation to health and disease. Am. J. Obstet. Gynecol. 220, 324–335. doi: 10.1016/j.ajog.2018.11.1089
Guerrero-Preston R., White J. R., Godoy-Vitorino F., Rodriguez-Hilario A., Navarro K., Gonzalez H., et al. (2017). High-resolution microbiome profiling uncovers Fusobacterium nucleatum, Lactobacillus gasseri/johnsonii, and Lactobacillus vaginalis associated to oral and oropharyngeal cancer in saliva from HPV positive and HPV negative patients treated with surgery and chemo-radiation. Oncotarget 8, 110931–110948. doi: 10.18632/oncotarget.20677
Guinane C. M., Kent R. M., Norberg S., Hill C., Fitzgerald G. F., Stanton C., et al. (2011). Host specific diversity in Lactobacillus johnsonii as evidenced by a major chromosomal inversion and phage resistance mechanisms. PloS One 6, e18740.
Harrison N. A., Gardner C. L., Da Silva D. R., Gonzalez C. F., Lorca G. L. (2021). Identification of biomarkers for systemic distribution of nanovesicles from lactobacillus johnsonii N6.2. Front. Immunol. 12, 723433. doi: 10.3389/fimmu.2021.723433
He T., Zhu Y. H., Yu J., Xia B., Liu X., Yang G. Y., et al. (2019). Lactobacillus johnsonii L531 reduces pathogen load and helps maintain short-chain fatty acid levels in the intestines of pigs challenged with Salmonella enterica Infantis. Vet. Microbiol. 230, 187–194. doi: 10.1016/j.vetmic.2019.02.003
Hill C., Guarner F., Reid G., Gibson G. R., Merenstein D. J., Pot B., et al. (2014). Expert consensus document. The International Scientific Association for Probiotics and Prebiotics consensus statement on the scope and appropriate use of the term probiotic. Nat. Rev. Gastroenterol. Hepatol. 11, 506–514. doi: 10.1038/nrgastro.2014.66
Hsieh P. S., Tsai Y. C., Chen Y. C., Teh S. F., Ou C. M., King V. A. (2012). Eradication of Helicobacter pylori infection by the probiotic strains Lactobacillus johnsonii MH-68 and L. salivarius ssp. salicinius AP-32. Helicobacter 17, 466–477. doi: 10.1111/j.1523-5378.2012.00992.x
Hu Y., Zhao M., Lu Z., Lv F., Zhao H., Bie X. (2021). L. johnsonii, L. plantarum and L. rhamnosus alleviated Enterohaemorrhagic Escherichia coli-induced diarrhoea in mice by regulating gut microbiota. Microb. Pathog. 154, 104856. doi: 10.1016/j.micpath.2021.104856
Imai J., Ichikawa H., Kitamoto S., Golob J. L., Kaneko M., Nagata J., et al. (2021). A potential pathogenic association between periodontal disease and Crohn's disease. JCI Insight 6 (23), e148543. doi: 10.1172/jci.insight.148543
Inoue R., Nishio A., Fukushima Y., Ushida K. (2007). Oral treatment with probiotic Lactobacillus johnsonii NCC533 (La1) for a specific part of the weaning period prevents the development of atopic dermatitis induced after maturation in model mice, NC/Nga. Br. J. Dermatol. 156, 499–509. doi: 10.1111/j.1365-2133.2006.07695.x
Isobe H., Nishiyama A., Takano T., Higuchi W., Nakagawa S., Taneike I., et al. (2012). Reduction of overall Helicobacter pylori colonization levels in the stomach of Mongolian gerbil by Lactobacillus johnsonii La1 (LC1) and its in vitro activities against H. pylori motility and adherence. Biosci. Biotechnol. Biochem. 76, 850–852. doi: 10.1271/bbb.110921
Jaffar N., Ishikawa Y., Mizuno K., Okinaga T., Maeda T. (2016). Mature Biofilm Degradation by Potential Probiotics: aggregatibacter actinomycetemcomitans versus Lactobacillus spp. PloS One 11, e0159466. doi: 10.1371/journal.pone.0159466
Jawhara S. (2022). How Gut Bacterial Dysbiosis Can Promote Candida albicans Overgrowth during Colonic Inflammation. Microorganisms 10 (5), 1014. doi: 10.3390/microorganisms10051014
Jia D. J., Wang Q. W., Hu Y. Y., He J. M., Ge Q. W., Qi Y. D., et al. (2022). Lactobacillus johnsonii alleviates colitis by TLR1/2-STAT3 mediated CD206(+) macrophages(IL-10) activation. Gut Microbes 14, 2145843. doi: 10.1080/19490976.2022.2145843
Kingma S. D., Li N., Sun F., Valladares R. B., Neu J., Lorca G. L. (2011). Lactobacillus johnsonii N6.2 stimulates the innate immune response through Toll-like receptor 9 in Caco-2 cells and increases intestinal crypt Paneth cell number in biobreeding diabetes-prone rats. J. Nutr. 141, 1023–1028. doi: 10.3945/jn.110.135517
Kitamoto S., Nagao-Kitamoto H., Jiao Y., Gillilland M. G. 3rd, Hayashi A., Imai J., et al. (2020). The intermucosal connection between the mouth and gut in commensal pathobiont-driven colitis. Cell 182, 447–462.e14. doi: 10.1016/j.cell.2020.05.048
Kling D. N., Debose-Scarlett E. M., Teixeira L. D., Gezan S. A., Lorca G. L., Gonzalez C. F. (2018). Sex Modulates Lactobacillus johnsonii N6.2 and Phytophenol Effectiveness in Reducing High Fat Diet Induced mTOR Activation in Sprague-Dawley Rats. Front. Microbiol. 9, 2649. doi: 10.3389/fmicb.2018.02649
Kuang L., Jiang Y. (2020). Effect of probiotic supplementation in pregnant women: a meta-analysis of randomised controlled trials. Br. J. Nutr. 123, 870–880. doi: 10.1017/S0007114519003374
La Ragione R. M., Narbad A., Gasson M. J., Woodward M. J. (2004). In vivo characterization of Lactobacillus johnsonii FI9785 for use as a defined competitive exclusion agent against bacterial pathogens in poultry. Lett. Appl. Microbiol. 38, 197–205. doi: 10.1111/j.1472-765X.2004.01474.x
Lai K. K., Lorca G. L., Gonzalez C. F. (2009). Biochemical properties of two cinnamoyl esterases purified from a Lactobacillus johnsonii strain isolated from stool samples of diabetes-resistant rats. Appl. Environ. Microbiol. 75, 5018–5024. doi: 10.1128/AEM.02837-08
Lau K., Benitez P., Ardissone A., Wilson T. D., Collins E. L., Lorca G., et al. (2011). Inhibition of type 1 diabetes correlated to a Lactobacillus johnsonii N6.2-mediated Th17 bias. J. Immunol. 186, 3538–3546. doi: 10.4049/jimmunol.1001864
Lebeer S., Vanderleyden J., De Keersmaecker S. C. (2008). Genes and molecules of lactobacilli supporting probiotic action. Microbiol. Mol. Biol. Rev. 72, 728–764. doi: 10.1128/MMBR.00017-08
Lee B., Moon K. M., Kim C. Y. (2018). Tight junction in the intestinal epithelium: its association with diseases and regulation by phytochemicals. J. Immunol. Res. 2018, 2645465. doi: 10.1155/2018/2645465
Lee K. A., Luong M. K., Shaw H., Nathan P., Bataille V., Spector T. D. (2021). The gut microbiome: what the oncologist ought to know. Br. J. Cancer 125, 1197–1209. doi: 10.1038/s41416-021-01467-x
Lehtoranta L., Ala-Jaakkola R., Laitila A., Maukonen J. (2022). Healthy vaginal microbiota and influence of probiotics across the female life span. Front. Microbiol. 13, 819958. doi: 10.3389/fmicb.2022.819958
Leonard M. T., Valladares R. B., Ardissone A., Gonzalez C. F., Lorca G. L., Triplett E. W. (2014). Complete Genome Sequences of Lactobacillus johnsonii Strain N6.2 and Lactobacillus reuteri Strain TD1. Genome Announcements 2 (3), e00397-14. doi: 10.1128/genomeA.00397-14
Lim C. Y., In J. (2019). Randomization in clinical studies. Korean J. Anesthesiol 72, 221–232. doi: 10.4097/kja.19049
Liu H.-Y., Roos S., Jonsson H., Ahl D., Dicksved J., Lindberg J. E., et al. (2015). Effects of Lactobacillus johnsonii and Lactobacillus reuteri on gut barrier function and heat shock proteins in intestinal porcine epithelial cells. Physiol. Rep. 3, e12355. doi: 10.14814/phy2.12355
Lynch S. V., Pedersen O. (2016). The human intestinal microbiome in health and disease. N. Engl. J. Med. 375, 2369–2379. doi: 10.1056/NEJMra1600266
Lyu M., Bai Y., Orihara K., Miyanaga K., Yamamoto N. (2023). GAPDH released from lactobacillus johnsonii MG enhances barrier function by upregulating genes associated with tight junctions. Microorganisms 11 (6), 1393. doi: 10.3390/microorganisms11061393
Manos J. (2022). The human microbiome in disease and pathology. APMIS 130, 690–705. doi: 10.1111/apm.13225
Marcial G. E., Ford A. L., Haller M. J., Gezan S. A., Harrison N. A., Cai D., et al. (2017). Lactobacillus johnsonii N6.2 modulates the host immune responses: a double-blind, randomized trial in healthy adults. Front. Immunol. 8, 655. doi: 10.3389/fimmu.2017.00655
Markey L., Shaban L., Green E. R., Lemon K. P., Mecsas J., Kumamoto C. A. (2018). Pre-colonization with the commensal fungus Candida albicans reduces murine susceptibility to Clostridium difficile infection. Gut Microbes 9, 497–509. doi: 10.1080/19490976.2018.1465158
Marteau P., Lemann M., Seksik P., Laharie D., Colombel J. F., Bouhnik Y., et al. (2006). Ineffectiveness of Lactobacillus johnsonii LA1 for prophylaxis of postoperative recurrence in Crohn's disease: a randomised, double blind, placebo controlled GETAID trial. Gut 55, 842–847. doi: 10.1136/gut.2005.076604
Mason K. L., Downward J. R. E., Falkowski N. R., Young V. B., Kao J. Y., Huffnagle G. B. (2012a). Interplay between the gastric bacterial microbiota and Candida albicans during postantibiotic recolonization and gastritis. Infect. Immun. 80, 150–158. doi: 10.1128/IAI.05162-11
Mason K. L., Downward J. R. E., Mason K. D., Falkowski N. R., Eaton K. A., Kao J. Y., et al. (2012b). Candida albicans and bacterial microbiota interactions in the cecum during recolonization following broad-spectrum antibiotic therapy. Infect. Immun. 80, 3371–3380. doi: 10.1128/IAI.00449-12
Melli L. C. F. L., Do Carmo-Rodrigues M. S., Araujo H. B., Mello C. S., Tahan S., Pignatari A. C. C., et al. (2020). Gut microbiota of children with atopic dermatitis: Controlled study in the metropolitan region of Sao Paulo, Brazil. Allergologia Et Immunopathologia 48, 107–115. doi: 10.1016/j.aller.2019.08.004
Michetti P., Dorta G., Wiesel P. H., Brassart D., Verdu E., Herranz M., et al. (1999). Effect of whey-based culture supernatant of Lactobacillus acidophilus (johnsonii) La1 on Helicobacter pylori infection in humans. Digestion 60, 203–209. doi: 10.1159/000007660
Mu Q., Zhang H., Liao X., Lin K., Liu H., Edwards M. R., et al. (2017). Control of lupus nephritis by changes of gut microbiota. Microbiome 5, 73. doi: 10.1186/s40168-017-0300-8
Nadatani Y., Watanabe T., Suda W., Nakata A., Matsumoto Y., Kosaka S., et al. (2019). Gastric acid inhibitor aggravates indomethacin-induced small intestinal injury via reducing Lactobacillus johnsonii. Sci. Rep. 9, 17490. doi: 10.1038/s41598-019-53559-7
Navarro-Tapia E., Sebastiani G., Sailer S., Toledano L. A., Serra-Delgado M., Garcia-Algar O., et al. (2020). Probiotic supplementation during the perinatal and infant period: effects on gut dysbiosis and disease. Nutrients 12 (8), 2243. doi: 10.3390/nu12082243
Neeser J.-R., Granato D., Rouvet M., Servin A., Teneberg S., Karlsson K.-A. (2000). Lactobacillus johnsonii La1 shares carbohydrate-binding specificities with several enteropathogenic bacteria. Glycobiology 10, 1193–1199. doi: 10.1093/glycob/10.11.1193
O’Toole P. W., Shiels P. G. (2020). The role of the microbiota in sedentary lifestyle disorders and ageing: lessons from the animal kingdom. J. Intern. Med. 287, 271–282. doi: 10.1111/joim.13021
Ocadiz-Ruiz R., Photenhauer A. L., Hayes M. M., Ding L., Fearon E. R., Merchant J. L. (2017). ZBP-89 function in colonic stem cells and during butyrate-induced senescence. Oncotarget 8, 94330–94344. doi: 10.18632/oncotarget.21698
O’Flaherty S., Briner Crawley A., Theriot C. M., Barrangou R. (2018). The Lactobacillus bile salt hydrolase repertoire reveals niche-specific adaptation. mSphere 3, e00140-18. doi: 10.1128/mSphere.00140-18
Ownby D. R., Johnson C. C., Peterson E. L. (2002). Exposure to dogs and cats in the first year of life and risk of allergic sensitization at 6 to 7 years of age. JAMA 288, 963–972. doi: 10.1001/jama.288.8.963
Pantoflickova D., Corthesy-Theulaz I., Dorta G., Stolte M., Isler P., Rochat F., et al. (2003). Favourable effect of regular intake of fermented milk containing Lactobacillus johnsonii on Helicobacter pylori associated gastritis. Aliment Pharmacol. Ther. 18, 805–813. doi: 10.1046/j.1365-2036.2003.01675.x
Park J., Kim N. E., Yoon H., Shin C. M., Kim N., Lee D. H., et al. (2021). Fecal microbiota and gut microbe-derived extracellular vesicles in colorectal cancer. Front. Oncol. 11, 650026. doi: 10.3389/fonc.2021.650026
Peter A. V. (1993). Lactic acid bacteria, their metabolic products and interference with microbial growth. FEMS Microbiol. Rev. 12, 221–237. doi: 10.1111/j.1574-6976.1993.tb00020.x
Pridmore R. D., Berger B., Desiere F., Vilanova D., Barretto C., Pittet A. C., et al. (2004). The genome sequence of the probiotic intestinal bacterium Lactobacillus johnsonii NCC 533. Proc. Natl. Acad. Sci. U.S.A. 101, 2512–2517. doi: 10.1073/pnas.0307327101
Pridmore R. D., Pittet A. C., Praplan F., Cavadini C. (2008). Hydrogen peroxide production by Lactobacillus johnsonii NCC 533 and its role in anti-Salmonella activity. FEMS Microbiol. Lett. 283, 210–215. doi: 10.1111/j.1574-6968.2008.01176.x
Rautava S., Luoto R., Salminen S., Isolauri E. (2012). Microbial contact during pregnancy, intestinal colonization and human disease. Nat. Rev. Gastroenterol. Hepatol. 9, 565–576. doi: 10.1038/nrgastro.2012.144
Ravi K., Rauch M., Lynch S. V., Lukacs N. W., Huffnagle G. B. (2023). Complete genome sequence of lactobacillus johnsonii MR1, isolated from a BALB/c mouse cecum. Microbiol. Resour. Announc. 12, e0107822. doi: 10.1128/mra.01078-22
Reed A., Olszewska M. A., Mann A., Novoa Rama E., Thippareddi H., Singh M., et al. (2022). Draft genome sequences of two Lactobacillus johnsonii and three Ligilactobacillus salivarius strains isolated from intestinal microbiomes of chickens. Microbiol. Resour. Announc. 11, e0092521. doi: 10.1128/mra.00925-21
Reyman M., Van Houten M. A., Watson R. L., Chu M., Arp K., De Waal W. J., et al. (2022). Effects of early-life antibiotics on the developing infant gut microbiome and resistome: a randomized trial. Nat. Commun. 13, 893. doi: 10.1038/s41467-022-28525-z
Richards J. L., Yap Y. A., Mcleod K. H., Mackay C. R., Marino E. (2016). Dietary metabolites and the gut microbiota: an alternative approach to control inflammatory and autoimmune diseases. Clin. Trans. Immunol. 5 (5), e82. doi: 10.1038/cti.2016.29
Roesch L. F., Lorca G. L., Casella G., Giongo A., Naranjo A., Pionzio A. M., et al. (2009). Culture-independent identification of gut bacteria correlated with the onset of diabetes in a rat model. ISME J. 3, 536–548. doi: 10.1038/ismej.2009.5
Rooks M. G., Garrett W. S. (2016). Gut microbiota, metabolites and host immunity. Nat. Rev. Immunol. 16, 341–352.
Rosignoli C., Thibaut De Menonville S., Orfila D., Beal M., Bertino B., Aubert J., et al. (2018). A topical treatment containing heat-treated Lactobacillus johnsonii NCC 533 reduces Staphylococcus aureus adhesion and induces antimicrobial peptide expression in an in vitro reconstructed human epidermis model. Exp. Dermatol. 27, 358–365. doi: 10.1111/exd.13504
Saxton R. A., Sabatini D. M. (2017). mTOR signaling in growth, metabolism, and disease. Cell 169, 361–371. doi: 10.1016/j.cell.2017.03.035
Schar-zammaretti P., Ubbink J. (2003). The cell wall of lactic acid bacteria: surface constituents and macromolecular conformations. Biophys. J. 85, 4076–4092.
Servin A. L. (2004). Antagonistic activities of lactobacilli and bifidobacteria against microbial pathogens. FEMS Microbiol. Rev. 28, 405–440. doi: 10.1016/j.femsre.2004.01.003
Sgouras D. N., Panayotopoulou E. G., Martinez-Gonzalez B., Petraki K., Michopoulos S., Mentis A. (2005). Lactobacillus johnsonii La1 attenuates Helicobacter pylori-associated gastritis and reduces levels of proinflammatory chemokines in C57BL/6 mice. Clin. Diagn. Lab. Immunol. 12, 1378–1386. doi: 10.1128/CDLI.12.12.1378-1386.2005
Slizewska K., Markowiak-Kopec P., Slizewska W. (2021). The role of probiotics in cancer prevention. Cancers 13 (1), 20. doi: 10.3390/cancers13010020
Stavropoulou E., Bezirtzoglou E. (2020). Probiotics in medicine: a long debate. Front. Immunol. 11. doi: 10.3389/fimmu.2020.02192
Tabatabaei N., Eren A. M., Barreiro L. B., Yotova V., Dumaine A., Allard C., et al. (2019). Vaginal microbiome in early pregnancy and subsequent risk of spontaneous preterm birth: a case-control study. BJOG 126, 349–358. doi: 10.1111/1471-0528.15299
Tang H., Ren J., Yuan J., Zeng B., Wei H. (2010). An in vitro assessment of inhibitory effect of 16 strains of probiotics on the germination of Candida albicans. Afr. J. Microbiol. Res. 4, 1251–1256.
Teixeira L. D., Harrison N. A., Da Silva D. R., Mathews C. E., Gonzalez C. F., Lorca G. L. (2022). Nanovesicles from lactobacillus johnsonii N6.2 reduce apoptosis in human beta cells by promoting AHR translocation and IL10 secretion. Front. Immunol. 13, 899413. doi: 10.3389/fimmu.2022.899413
Teixeira L. D., Kling D. N., Lorca G. L., Gonzalez C. F. (2018). Lactobacillus johnsonii N6.2 diminishes caspase-1 maturation in the gastrointestinal system of diabetes prone rats. Beneficial Microbes 9, 527–539. doi: 10.3920/BM2017.0120
Thomas A. M., Manghi P., Asnicar F., Pasolli E., Armanini F., Zolfo M., et al. (2019). Metagenomic analysis of colorectal cancer datasets identifies cross-cohort microbial diagnostic signatures and a link with choline degradation. Nat. Med. 25, 667–678. doi: 10.1038/s41591-019-0405-7
Thompson S. C., Ford A. L., Moothedan E. J., Stafford L. S., Garrett T. J., Dahl W. J., et al. (2023). Identification of food and nutrient components as predictors of Lactobacillus colonization. Front. Nutr. 10. doi: 10.3389/fnut.2023.1118679
Thursby E., Juge N. (2017). Introduction to the human gut microbiota. Biochem. J. 474, 1823–1836. doi: 10.1042/BCJ20160510
Travers M. A., Sow C., Zirah S., Deregnaucourt C., Chaouch S., Queiroz R. M., et al. (2016). Deconjugated Bile Salts Produced by Extracellular Bile-Salt Hydrolase-Like Activities from the Probiotic Lactobacillus johnsonii La1 Inhibit Giardia duodenalis In vitro Growth. Front. Microbiol. 7, 1453. doi: 10.3389/fmicb.2016.01453
Vaarala O., Atkinson M. A., Neu J. (2008). The "perfect storm" for type 1 diabetes: the complex interplay between intestinal microbiota, gut permeability, and mucosal immunity. Diabetes 57, 2555–2562. doi: 10.2337/db08-0331
Valladares R., Bojilova L., Potts A. H., Cameron E., Gardner C., Lorca G., et al. (2013). Lactobacillus johnsonii inhibits indoleamine 2,3-dioxygenase and alters tryptophan metabolite levels in BioBreeding rats. FASEB J. 27, 1711–1720. doi: 10.1096/fj.12-223339
Valladares R., Sankar D., Li N., Williams E., Lai K.-K., Abdelgeliel A. S., et al. (2010). Lactobacillus johnsonii N6.2 mitigates the development of type 1 diabetes in BB-DP rats. PloS One 5, e10507. doi: 10.1371/journal.pone.0010507
Van Gossum A., Dewit O., Louis E., De Hertogh G., Baert F., Fontaine F., et al. (2007). Multicenter randomized-controlled clinical trial of probiotics (Lactobacillus johnsonii, LA1) on early endoscopic recurrence of Crohn's disease after lleo-caecal resection. Inflamm. Bowel Dis. 13, 135–142. doi: 10.1002/ibd.20063
Vazquez-Munoz R., Thompson A., Russell J. T., Sobue T., Zhou Y., Dongari-Bagtzoglou A. (2022). Insights from the Lactobacillus johnsonii genome suggest the production of metabolites with antibiofilm activity against the pathobiont Candida albicans. Front. Microbiol. 13, 853762. doi: 10.3389/fmicb.2022.853762
von Mutius E., Vercelli D. (2010). Farm living: effects on childhood asthma and allergy. Nat. Rev. Immunol. 10, 861–868. doi: 10.1038/nri2871
Wang C. H., Yen H. R., Lu W. L., Ho H. H., Lin W. Y., Kuo Y. W., et al. (2022). Adjuvant Probiotics of Lactobacillus salivarius subsp. salicinius AP-32, L. johnsonii MH-68, and Bifidobacterium animalis subsp. lactis CP-9 Attenuate Glycemic Levels and Inflammatory Cytokines in Patients With Type 1 Diabetes Mellitus. Front. Endocrinol. (Lausanne) 13, 754401. doi: 10.3389/fendo.2022.754401
Weersma R. K., Zhernakova A., Fu J. Y. (2020). Interaction between drugs and the gut microbiome. Gut 69, 1510–1519. doi: 10.1136/gutjnl-2019-320204
Wicinski M., Gebalski J., Golebiewski J., Malinowski B. (2020). Probiotics for the treatment of overweight and obesity in humans-A review of clinical trials. Microorganisms 8 (8), 1148. doi: 10.3390/microorganisms8081148
Wong R. J., Ahmed A. (2014). Obesity and non-alcoholic fatty liver disease: Disparate associations among Asian populations. World J. Hepatol. 6, 263–273. doi: 10.4254/wjh.v6.i5.263
Wu X., Zhao C., Guo Z., Hao Y., Li J., Shi H., et al. (2016). Genome sequence of Lactobacillus johnsonii strain W1, Isolated from mice. Genome Announc 4, e00561. doi: 10.1128/genomeA.00561-16
Xia B., Yu J., He T., Liu X., Su J., Wang M., et al. (2020). Lactobacillus johnsonii L531 ameliorates enteritis via elimination of damaged mitochondria and suppression of SQSTM1-dependent mitophagy in a Salmonella infantis model of piglet diarrhea. FASEB J. 34, 2821–2839. doi: 10.1096/fj.201901445RRR
Xin J., Zeng D., Wang H., Ni X., Yi D., Pan K., et al. (2014). Preventing non-alcoholic fatty liver disease through Lactobacillus johnsonii BS15 by attenuating inflammation and mitochondrial injury and improving gut environment in obese mice. Appl. Microbiol. Biotechnol. 98, 6817–6829. doi: 10.1007/s00253-014-5752-1
Yagi K., Asai N., Huffnagle G. B., Lukacs N. W., Fonseca W. (2022). Early-life lung and gut microbiota development and respiratory syncytial virus infection. Front. Immunol. 13, 877771. doi: 10.3389/fimmu.2022.877771
Yamamoto M. L., Maier I., Dang A. T., Berry D., Liu J., Ruegger P. M., et al. (2013). Intestinal bacteria modify lymphoma incidence and latency by affecting systemic inflammatory state, oxidative stress, and leukocyte genotoxicity. Cancer Res. 73, 4222–4232. doi: 10.1158/0008-5472.CAN-13-0022
Yamano T., Iino H., Takada M., Blum S., Rochat F., Fukushima Y. (2006). Improvement of the human intestinal flora by ingestion of the probiotic strainLactobacillus johnsoniiLa1. Br. J. Nutr. 95, 303–312. doi: 10.1079/BJN20051507
Yang G. Y., Xia B., Su J. H., He T., Liu X., Guo L., et al. (2020). Anti-inflammatory effects of Lactobacillus johnsonii L531 in a pig model of Salmonella Infantis infection involves modulation of CCR6(+) T cell responses and ER stress. Vet. Res. 51, 26. doi: 10.1186/s13567-020-00754-4
Yang J., Shin T. S., Kim J. S., Jee Y. K., Kim Y. K. (2022a). A new horizon of precision medicine: combination of the microbiome and extracellular vesicles. Exp. Mol. Med. 54, 466–482. doi: 10.1038/s12276-022-00748-6
Yang L., Wang J. F., Liu N., Wang X., Wang J., Yang G. H., et al. (2022b). Lactobacillusjohnsonii L531 protects against salmonella infantis-induced intestinal damage by regulating the NOD activation, endoplasmic reticulum stress, and autophagy. Int. J. Mol. Sci. 23 (18), 10395. doi: 10.3390/ijms231810395
Yang S., Liu Y., Yang N., Lan Y., Lan W., Feng J., et al. (2022c). The gut microbiome and antibiotic resistome of chronic diarrhea rhesus macaques (Macaca mulatta) and its similarity to the human gut microbiome. Microbiome 10, 29. doi: 10.1186/s40168-021-01218-3
Zhang W., Wang J., Zhang D., Liu H., Wang S., Wang Y., et al. (2019). Complete genome sequencing and comparative genome characterization of Lactobacillus johnsonii ZLJ010, a potential probiotic with health-promoting properties. Front. Genet. 10, 812. doi: 10.3389/fgene.2019.00812
Zhang Y., Mu T., Yang Y., Zhang J., Ren F., Wu Z. (2021). Lactobacillus johnsonii attenuates Citrobacter rodentium-induced colitis by regulating inflammatory responses and endoplasmic reticulum stress in mice. J. Nutr. 151, 3391–3399. doi: 10.1093/jn/nxab250
Zhang Y. C., Zhang L. W., Ma W., Yi H. X., Yang X., Du M., et al. (2012). Screening of probiotic lactobacilli for inhibition of Shigella sonnei and the macromolecules involved in inhibition. Anaerobe 18, 498–503. doi: 10.1016/j.anaerobe.2012.08.007
Zheng J., Wittouck S., Salvetti E., Franz C., Harris H. M. B., Mattarelli P., et al. (2020). A taxonomic note on the genus Lactobacillus: Description of 23 novel genera, emended description of the genus Lactobacillus Beijerinck 1901, and union of Lactobacillaceae and Leuconostocaceae. Int. J. Syst. Evol. Microbiol. 70, 2782–2858. doi: 10.1099/ijsem.0.004107
Zou Y. J., Xu J. J., Wang X., Zhu Y. H., Wu Q., Wang J. F. (2020). Lactobacillus johnsonii L531 ameliorates Escherichia coli-induced cell damage via Inhibiting NLRP3 inflammasome activity and promoting ATG5/ATG16L1-mediated autophagy in porcine mammary epithelial cells. Vet. Sci. 7, 112. doi: 10.3390/vetsci7030112
Keywords: Lactobacillus johnsonii, gut microbiota, gut-lung axis, probiotics, microbiota metabolites
Citation: Arzola-Martínez L, Ravi K, Huffnagle GB, Lukacs NW and Fonseca W (2024) Lactobacillus johnsonii and host communication: insight into modulatory mechanisms during health and disease. Front. Microbiomes 2:1345330. doi: 10.3389/frmbi.2023.1345330
Received: 27 November 2023; Accepted: 29 December 2023;
Published: 16 January 2024.
Edited by:
Ana Gomes, Universidade Católica Portuguesa, PortugalReviewed by:
José Carlos Andrade, University Institute of Health Sciences, PortugalCopyright © 2024 Arzola-Martínez, Ravi, Huffnagle, Lukacs and Fonseca. This is an open-access article distributed under the terms of the Creative Commons Attribution License (CC BY). The use, distribution or reproduction in other forums is permitted, provided the original author(s) and the copyright owner(s) are credited and that the original publication in this journal is cited, in accordance with accepted academic practice. No use, distribution or reproduction is permitted which does not comply with these terms.
*Correspondence: Wendy Fonseca, d2ZhZ3VpbGFAdW1pY2guZWR1
Disclaimer: All claims expressed in this article are solely those of the authors and do not necessarily represent those of their affiliated organizations, or those of the publisher, the editors and the reviewers. Any product that may be evaluated in this article or claim that may be made by its manufacturer is not guaranteed or endorsed by the publisher.
Research integrity at Frontiers
Learn more about the work of our research integrity team to safeguard the quality of each article we publish.