- 1The First Affiliated Hospital of Zhejiang Chinese Medical University (Zhejiang Provincial Hospital of Chinese Medicine), Hangzhou, China
- 2School of Life Sciences, Zhejiang Chinese Medical University, Hangzhou, China
Non-alcoholic fatty liver disease (NAFLD) is increasing worldwide and has become the greatest potential risk for cirrhosis and hepatocellular carcinoma. The metabolites produced by the gut microbiota act as signal molecules that mediate the interaction between microorganisms and the host and have biphasic effects on human health. The gut microbiota and its metabolites, short-chain fatty acids (SCFAs), have been discovered to ameliorate many prevalent liver diseases, including NAFLD. Currently, SCFAs have attracted widespread attention as potential therapeutic targets for NAFLD, but the mechanism of action has not been fully elucidated. This article summarizes the mechanisms of short-chain fatty acids of gut microbiota metabolites to regulate the metabolism of glucose and lipid, maintain the intestinal barrier, alleviate the inflammatory response, and improve the oxidative stress to improve NAFLD, in order to provide a reference for clinical application.
1 Introduction
The mechanism of NAFLD as a multifactorial metabolic disease remains unclear. Currently, generally recognized is the “multiple hit” theory, which uses insulin resistance (IR) and obesity the “first hit” to increase free fatty acids (FFAs) and make the liver more susceptible to hepatotoxic injury. NAFLD progresses when multiple factors cause liver damage, including oxidative stress, mitochondrial dysfunction, inflammatory cytokines, and intestinal dysbiosis (Gou et al., 2023). The exposure of the liver to the metabolites produced by the gut microbiota through the portal vein is the direct cause of the liver metabolic disorders and degeneration and necrosis (Singh et al., 2023), which is why the gut microbiota is considered as an important entry point for the treatment of NAFLD.
Gut microbiota is a complex ecosystem composed of trillions of microbiota, which is involved in regulating body immunity, maintaining intestinal barrier integrity, and defending against foreign pathogens (Fan and Pedersen, 2021). Changing gut microbiota composition can lead to ecological imbalance and cause many diseases, including NAFLD.90% of SCFAs are produced by dietary fiber fermentation under the action of gut microbiota, and the rest are produced by dietary intake and protein metabolism. It can enter the blood through the intestine and have a direct impact on human metabolism (Hsu et al., 2024). Deng et al. (2020) study on Methionine and Choline Deficient L-Amino Acid Diet (MCD) of mice injected with SCFA solution for treatment, found that significantly reduced serum levels of alanine aminotransferase (ALT) and aspartate aminotransferase (AST) in mouse liver, Number of lipid droplets and the levels of triglycerides (TG) and total cholesterol (TC), Also reduced macrophage hepatic aggregation and proinflammatory responses induced by MCD. This indicates that the abundance of SCFAs is closely correlated with NAFLD. A growing number of studies have found that it may be because SCFAs can intervene in the process of “multiple hits.”
In this paper, we outlined the involvement of SCFAs in the metabolism of gut microbiota and explored its mechanism of action in NAFLD, hoping to find new therapeutic target for clinical treatment.
2 The production and metabolism of SCFAs
The precursor of most SCFAs is pyruvate, produced from glycolysis by undigested dietary fiber in the presence of the gut microbiota (Chen et al., 2020), which consists of 1–6 carbon atoms. About 50–100 mmol SCFAs are produced in the normal intestine daily, mainly acetate, propionate and butyrate, which account for 95% of all SCFAs and are present at 3:1:1 in the gut (McMurdie et al., 2022; Anachad et al., 2023).
Acetate is mainly produced by Bifidobacteria, Lactobacilli, and other bacteria, such as Lachnospira, Roseburia, Akkermansia Muciniphila, and Parabacteroides (Anachad et al., 2023). Besides producing most of acetate via acetyl-CoA, pyruvate synthesizes acetate via the Wood-Ljungdahl pathway. This pathway reduces CO2 to CO and formate, which combines with methyl groups to form acetyl-CoA (Frampton et al., 2020).
The gut microbiota can produce propionate through succinate pathway, acrylate pathway, and propanediol pathway. Bacteroidetes and some Negativicutes bacteria such as Veillonella, Dialister, synthesize propionate through succinate pathway (Anachad et al., 2023), and some Negativicutes bacteria via acrylate pathway, like Megasphaera elsdenii and Lachnospiraceae (Schoenfeld and Wojtczak, 2016), to generate propionate. Other bacteria, including Escherichia coli and Lactobacillus reuteri (Louis and Flint, 2017), can degrade deoxy sugars by propanediol pathway.
Butyrate is produced after condensation of acetyl-CoA through butyrate kinase pathway. In addition, acetate can be converted to butyrate via the butyryl-CoA: acetate CoA-transferase pathway (Liu et al., 2021). Eubacterium and Roseburia are the main bacteria producing butyrate, and bacteria with the transferase genes also include Eubacterium hallii, Anaerostipes hadrus, Coprococcus catus (Louis and Flint, 2017).
SCFAs exist mainly in the intestine in the anionic (98%) and dissociated (2%) forms (Friederike, 2018). Most of the anionic form of the SCFAs are transported through the monocarboxylate transporter 1 (MCT1) and sodium-coupled monocarboxylate transporter 1 (SMCT1), whereas the dissociated form of SCFAs are absorbed via free diffusion (Sivaprakasam et al., 2017). After absorption, SCFAs enter the liver through the portal vein to play their role. Due to efficient extraction by the liver, only a limited amount of SCFAs enter the bloodstream. The liver filters almost 100% of the butyrate and supplies 70–90% of the energy to the colonic epithelial cells, so butyrate rarely enter the circulation (Boets et al., 2017).
3 Changes of gut microbiota and SCFAs in NAFLD
The composition of gut microbiota in NAFLD patients is different from that of normal people. In general, patients with NAFLD have significantly decreased diversity and altered composition of gut microbiota, such as decreased Coprococcus, Faecalibacterium, Megasphaera and Eubacterium, and increased Escherichia, Ruminococcaceaeand Adidaminococcus, compared to normal people (Cornejo-Pareja et al., 2024; Ha et al., 2024). Similarly, an increase in Firmicutes to Bacteroidetes (F/B) and a decrease in the abundance of Bifidobacteria, Lactobacillus, can also be monitored in the NAFLD rat model (Tanya et al., 2024). Among them, there are many bacteria associated with SCFA production that cause changes in SCFA levels.
Obesity is the major cause of NAFLD in children and adults, and obesity-induced ecological disturbances can lead to the development of NAFLD. The abundance of Bacteroidetes, Gemmiger, Prevotella and Oscillospira had decreased, and F/B ratio increased in obese NAFLD patients, which compared with obese youth without NAFLD (Monga Kravetz et al., 2020). In studies of obese children and adolescents, NAFLD patients have more less Bacteroidetes and no significant difference in Firmicutes. And Faecalibacterium prausnitzii, as the main butyrate-producing bacteria, reduced in obese NAFLD patients (Zhao et al., 2019). Of course, NAFLD not only occurs in obese people, but also high-fat diet, lack of exercise, and gender are the causes of NAFLD too (Tokuhara, 2021). In Wang’s study (Wang et al., 2016), non-obese NAFLD patients compared with healthy individuals showed a 20% increase in Bacteroidetes and a 24% decrease in Firmicutes. This is not consistent with the previous results, which suggests that the changes of gut microbiota is not single and also related to the status of NAFLD patients.
SCFAs are the key metabolites of gut microbiota to prevent NAFLD. High-fat diet-induced NAFLD mice had increased abundance of Barnesiella, Anaerobacterium, Bacteroides, Parabacteroides, and Clostridium IV after pectin supplementation in a dose-dependent manner. The levels of total SCFA, acetate and propionate elevated, with positive effects on NAFLD, which could regulate lipids, suppress oxidative stress and inflammation (Li et al., 2018). Hao et al. (2024) found that Crataegus pinnatifida polysaccharide (CPP) effectively reduced hepatic steatosis in NAFLD mice by decreasing the F/B ratio and increasing the abundance of Akkermansia to promote the production of SCFA, especially acetate and butyrate. Studies like this reveal the crucial role of the gut microbiota and SCFAs in the development of NAFLD.
4 Mechanisms of SCFAs to regulate NAFLD
60–70% of the energy in the gut is provided by SCFAs, and the rest flow into the liver via blood, meeting 5 to 15% of the total energy needs (Zhang et al., 2021). Only a small proportion were excreted with the feces (Canfora et al., 2015). SCFAs involved in the physiological activities of the body mainly through three G protein-coupled receptors, GPR41 (FFAR3), GPR43 (FFAR2), and GPR109A, which are variably expressed in different cells, and interfered in the occurrence and development of NAFLD by regulating glucose and lipid metabolism, restoring the intestinal barrier, and improving oxidative stress (Ikeda et al., 2022). In addition, SCFAs also inhibit the activity of histone deacetylase (HDAC) and regulates gene expression to affect body health (He et al., 2020) (Figure 1).
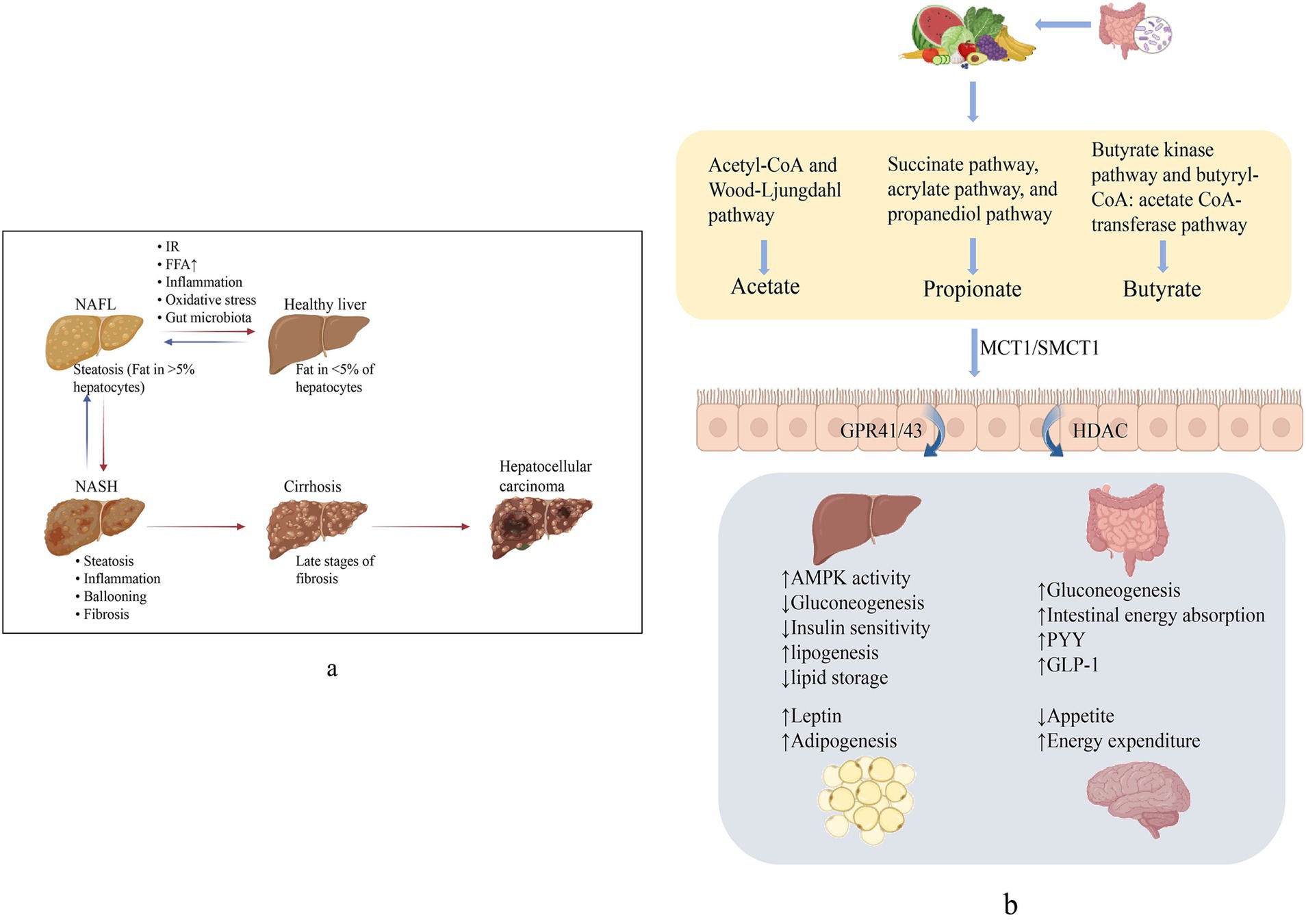
Figure 1. (a) Non-alcoholic fatty liver disease (NAFLD) spectrum. NAFL manifests as hepatic fat deposition >5% and can progress to NASH with hepatic inflammation and cellular necrosis. Broad fibrosis may lead to cirrhosis and ultimately to HCC. (b) Generation and role of SCFAs. SCFAs produced from dietary fiber, via active transport into the intestine mainly by SMCT1 and MCT1, activates GPCR, suppresses HDAC, and produces effects on multiple organs to perform the improvement of NAFLD.
4.1 SCFAs improve the glucose and lipid metabolism disorder
Sterol-regulatory element-binding protein 1c (SREBP-1c) is a nuclear transcription factor located in the liver and can regulate the expression of related enzymes such as TG, TC and fatty acids (Lazarević et al., 2019). ACC is the rate-limiting enzyme in fatty acid synthesis. AMPK stimulated by SCFAs will inhibit SREBP-1c and ACC to reduce the synthesis of fatty acid (He et al., 2020). Activation of AMPK increases the expression of peroxisome proliferator-activated receptor γ coactivator 1α (PGC-1α) in adipose tissue and skeletal muscle, which regulates the activity of various transcription factors including peroxisome proliferator-activated receptor α (PPARα) and peroxisome proliferator-activated receptor γ (PPARγ), and promotes the oxidation of fatty acid (Wang et al., 2020). Furthermore, AMPK regulates two major enzymes in lipolysis, hormone-sensitive lipase (HSL) and adipose triglyceride lipase (ATGL) (Tang et al., 2020).
Propionate is the most important GPR43 activator, while the most potent activators of GPR41 are propionate and butyrate (Horiuchi et al., 2020). Aragonès et al. (2019) found that it could promote the secretion of Peptide YY (PYY) and glucagon-like peptide-1 (GLP-1) in intestinal L cells through the activation of GPR41 and GPR43 to affect multiple tissues, including the pancreas and the brain. PYY can inhibit intestinal peristalsis, reduce appetite and increase satiety (Psichas et al., 2015); the main function of GLP-1 is to promote the proliferation of islet β cell, inhibit its apoptosis, protect hepatocytes from steatosis, and regulate blood glucose by improving insulin sensitivity (Ji and Guo, 2020).
Butyrate is a potent inhibitor of HDAC and is involved in epigenetic genetic regulation. Hong’s study (Hong et al., 2016) found that short-term oral administration of butyrate could improve high fat diet (HFD) induced obesity and IR. Further exploration proved that this is presumably due to butyrate inhibiting HDAC 1 expression which activate the adiponectin-mediated downstream pathway AMPK and stimulate mitochondrial function in skeletal muscle.
4.2 SCFAs improve insulin sensitivity
IR is a common feature of patients with NAFLD and refers to the decreased sensitivity of the body to insulin and the reduced ability of insulin to enhance glucose intake by peripheral tissues and suppress hepatic glucose export (Song L. et al., 2022; Song Q. et al., 2022). IR causes increased peripheral lipolysis, leading to disturbed hepatic fat metabolism and inducing NAFLD. NAFLD formation can also impair the antilipolytic effects of insulin, promoting excess FFAs production, causing hepatic lipid accumulation and leading to IR (Kim et al., 2022). The vicious cycle is the basic pathological characteristics that cause liver injury and lead to lipid metabolism disorders. Mariño et al. (2017) use the non-obese diabetic (NOD) mouse model found that feeds containing acetate and propionate effectively prevented diabetes. In sterile conditions, NOD mice are more likely to develop T1D.
Phosphoenolpyruvate carboxykinase (PEPCK) and glucose-6-phosphatase (G-6-pase)are two key enzymes of gluconeogenesis, and it was found that butyrate can directly enhance the expression of these two enzymes, stimulate intestinal gluconeogenesis through cAMP dependent mechanism, increase glucose release in the intestine, reduce hepatic gluconeogenesis, and maintain glucose homeostasis (De Vadder et al., 2014). Propionate can promote gluconeogenesis through the GPR41-mediated gut-brain neural pathway (Li et al., 2017). HDAC overexpression can hinder β cell differentiation and inhibit the transcription of insulin gene (Yamato, 2018). Li et al. (2013) found that butyrate as a natural antagonist of HDAC could inhibit β cell apoptosis, protect βcell function and promote insulin secretion.
Apart from insulin and glucagon, leptin also plays an important role in the regulation of blood glucose. Leptin is a hormone secreted by adipose tissue that acts on receptors located in the central nervous system (Zhou et al., 2023) to regulate negative feedback on human metabolism. SCFAs promote the secretion of leptin through GPR41 and GPR43 (Li et al., 2017), acting on insulin signaling and enhancing glucose uptake in brown adipose tissue.
4.3 SCFAs maintain the intestinal barrier and reduce inflammation
The intestinal barrier is composed of intestinal mucosa and epithelial cells, and intercellular junctions are important parts of the intestinal epithelial barrier, including tight junctions, adhesion junctions, and desmosomes, which can prevent bacteria from entering the mucosa and destroying the immune system (Wu et al., 2022). Increased inflammatory response of intestinal mucosa and damaged intestinal epithelial cells can cause microbial translocation and induce NAFLD. Tight junction proteins are the main functional proteins of the intestinal barrier, which will enlarge the intercellular space, increase intestinal mucosal permeability to increase translocation of bacteria such as Lipopolysaccharide (LPS) to cause inflammatory response (Horowitz et al., 2023).
SCFAs activate the GPR43 pathway to stimulate potassium efflux and hyperpolarization, consequently resulting in the activation of NOD-like receptor thermal protein domain associated protein 3 (NLRP3) inflammasome, which keeps the integrity of the intestinal barrier through reparation and cell survival under stress conditions (Li et al., 2024). Butyrate appears to be the most important SCFA to regulate tight junction proteins and has been shown to improve intestinal barrier function by increasing claudin-2 through the activation of AMPK (Caetano and Castelucci, 2022). In addition, as an inhibitor of HDAC, butyrate enhanced the transcriptional activity of HIF1α, leading to higher expression of tight junction proteins to maintain intestinal barrier integrity (Fachi et al., 2019).
T regulatory cells (Tregs) can be involved in immune tolerance, suppress inflammation and allergic reactions. Gurav et al. (2015) found that the treatment of Dendritic cells (DCs) with butyrate and propionate enhanced the ability of these cells to convert T cells into Tregs. In inflamed tissues, DCs present antigen to T cells and affect T cell polarization to T helper type 1 cells (Th1), Th2 or Th17 (Nastasi et al., 2015). Akhtar et al. (2022) proposed that butyrate regulates Th17 proliferation through HDAC and induces apoptosis, thereby regulating cytokine production and maintaining intestinal symbiosis and homeostasis. Moreover, Singh et al. (2010) found that Th17, which produce IL-17, were increased in GPR109A deficient mice. This signal transduction is activated by butyrate, implying that butyrate can inhibit the production of proinflammatory factors by activating the GPCR.
The above studies have fully demonstrated the positive effect of SCFA in fighting inflammation, but butyrate also seems to have a negative effect, inducing differentiation and apoptosis in transformed cells. In the Belcheva constructed mouse model of colorectal cancer, butyrate administration induced hyperproliferation of MSH2-deficient colon epithelial cells and promoted tumor formation (Belcheva et al., 2014). This suggests that SCFA has not only a positive role in intestinal health, and its complex molecular mechanisms need further elucidation.
4.4 SCFAs improve oxidative stress
Oxidative stress is a state of loss of balance between the oxidative and antioxidant systems of cells and tissues, and the process leads to the overproduction of oxidative free radicals and reactive oxygen species (ROS), destroying cellular proteins, lipids and nucleic acids, leading to cellular dysfunction (Rani et al., 2016). Oxidative stress can promote mitochondrial damage, FFAs oxidation and cytokine release, a large number of hepatocyte mitochondrial dysfunction, exposing the liver to high levels of ROS which promote lipid peroxidation and hepatitis aggravation (Ziolkowska et al., 2021), directly involved in the occurrence and development of NAFLD.
Domestic and foreign studies have shown that the improvement of hepatic oxidative stress by SCFAs involves the recovery of mitochondrial function. Hu et al. (2020) demonstrated that acetate and butyrate contributed to the recovery of mitochondrial respiratory function, reduced the produced ROS, and enhanced the antioxidant capacity of the pancreatic islet cells. Moreover, acetate is more efficient in inhibiting ROS, and butyrate can reduce oxidative stress by increasing the concentration of reduced glutathione (Li et al., 2021).
5 Potential therapeutic targets
Up to now, clinical studies targeting SCFAs to improve NAFLD are scarce, and the direction of drug development is mostly based on the promotion of SCFA-producing advantageous microbiota, such as increasing the abundance of Bacteroidetes, Ackermannia, Bifidobacterium, Lactobacillus, and Prevotella, and decreasing the ratios of Firmicutes and F/B (Castillo et al., 2021). Probiotics, prebiotics and Chinese herbs are representative of commonly used clinical treatments (Chen et al., 2023; Huang et al., 2024). In addition, exercise to improve obesity is also a way to regulate NAFLD. Aerobic exercise alone can cause changes in the composition and function of human gut microbiota, increase the concentration of SCFAs in feces and the ability of gut microbiota to produce SCFAs, especially in lean people with low BMI (Allen et al., 2018). Therefore, weight loss through exercise and diet also has the effect of regulating SCFA and improving NAFLD.
Currently, a variety of probiotics, prebiotics and synbiotics containing both have been developed to improve blood lipid, reduce inflammation, enhance IR in NAFLD patients and change the composition of gut microbiota (Castillo et al., 2021). Cristofori et al. (2021) observed that commercial probiotics such as Lactobacillus and Bifidobacterium, as SCFA-producing bacteria, can promote anti-inflammation, facilitate the growth and survival of intestinal epithelial cells, and oppose pathogens by modulating the immune system and host defense. As a prebiotic, Inulin increased the abundance of Bifidobacterium, Phascolarctobacterium and Blautia while inhibited the growth of pathogenic bacteria such as Fusobacterium and Corynebacterium_1 to promote the production of SCFAs, especially propionate and butyrate. The supplementation of inulin restored the integrity and function of the intestinal barrier by upregulating the expression of tight junction proteins, and effectively ameliorated the hepatic steatosis and inflammation induced by a high sucrose diet (Yang et al., 2023). Moreover, treatment of synbiotic increased the content of Bifidobacterium and Faecalibacterium, and decreased Oscillibacter and Alistipes (Scorletti et al., 2020). Ekhlasi et al. (2016) also found that synbiotic reduced serum liver enzyme levels, fasting blood glucose (FBG) and insulin levels.
Forsythoside A (FTA) is the main active ingredient isolated from Forsythiae Fructus and has prominent bioactivity. FTA can restore normal levels of Firmicutes and Bacteroidetes, elevate the abundance of prevotellaceae_UCG-001 and Ruminococcus_1, and decrease the abundance of Lactobacillus and mucispirillum. With the increase of SCFA-producing bacteria, there was an increase of SCFA levels and a decrease of serum inflammatory factors lever (Fu et al., 2022).
Genistein is the main active ingredient in isoflavones and is present in almost all legumes (Jaiswal et al., 2019). Hou et al. (2023) reported that Genistein-fed mice had increased Lactobacillus, Alloprevotella, Lachnospiraceae, and Bifidobacterium content and significantly enhanced SCFA synthesis. This experiment also found that genistein inhibited the inflammatory response, reduced IL-6 and TNF-α, promoted the intestinal epithelial renewal in elderly animals, and effectively improved the intestinal barrier function in mice.
Oluf Pedersen (Forslund et al., 2015)’ team sequenced the gut microbiota of patients with abnormal glucose metabolism and found that metformin could significantly increase the abundance of SCFAs-producing bacteria. It have also demonstrated that under the mediation of metformin, patients have increased Butyrivibrio, Megasphaera and Prevotella levels, promoting the production of SCFAs to alter gut microbiota composition. They also found a higher abundance of Akkermansia muciniphila, Bifidobacterium bifidum. Akkermansia muciniphila as a beneficial bacterium breaks down mucins to produce SCFAs (Zheng et al., 2024) and also adheres to undifferentiated and mature enterocytes to enhance epithelial integrity (Reunanen et al., 2015). This suggests that the beneficial effects of metformin may be due to the strengthening of the intestinal mucosal barrier (de la Cuesta-Zuluaga et al., 2017).
Traditional Chinese medicine (TCM) monomer is one of the main components of TCM. As a bioactive macromolecule in many Chinese herbal medicines, polysaccharides can increase the generation of SCFAs to improve glucose and lipid metabolism by regulating gut microbiota. The Astragalus membranaceus polysaccharides (AMP) extracted from Astragalus can participate in hypoglycemic effects by improving the disturbed gut microbiota. In db/db mice treated with AMP, ratio of Bacteroidota to Firmicutes is higher, and Allobaculum, Faecalibaculum, Akkermansia, Bifidobacterium and Romboutsia positively correlated with the levels of SCFAs. It has a hypoglycemic effect and improves intestinal integrity by increasing the expression of GPR41/43 and Occludin ZO-1 (Song L. et al., 2022; Song Q. et al., 2022). Ma et al. (2022) found that Lycium barbarum polysaccharide (LBP) reversed the abundance of Bacteroides, Ruminococcaceae_UCG-014, Mucispirillum and Intestinimonas in HFD induced diabetic mice, and SCFAs levels were increased significantly in LBP treated mice, which corresponded to the increase in the beneficial genus, alleviating hyperglycemia and hyperlipemia. Yao et al. (2020) found that Ruminococcus_bromii, Clostridium_methylpentosum, Roseburia_intestinalis, Clostridium_asparagiforme and Oscillibacter_valericigenes increased after Cyclocarya paliurus polysaccharide (CCPP) treatment. They also found that CCPP can promote the production of SCFAs both in vitro and in vivo to control blood glucose and blood lipid. Fucoidan has been proved to increase the relative abundance of Bacteroidetes, Ruminococcus, Prevotella, and Oscillospira and to decrease the relative abundance of Firmicutes and Actinobacterium. Meanwhile, fucoidan improved blood lipids and reduced hepatic steatosis and LPS levels in dyslipidaemic rats (Chen et al., 2019).
Gegen Qinlian Decoction (GQD) is a TCM compound commonly used for the treatment of metabolic diseases, consisting of Puerariae Lobatae Radix, Coptidis Rhizoma, Scutellariae Radix, Glycyrrhizae Radix et Rhizoma Praeparata cum Melle (Lu et al., 2021). Xu et al. (2020) found that GQD can increase the expression of several bacteria, including Faecalibacterium, Clostridium XIVa, Ruminococcus2, Butyricicoccus, and Coprococcus, which are well-known butyrate producers. Therefore, they also detected increased butyrate levels in the feces. The experimental observation that GOD treatment enhances glucose clearance in rats and ameliorates systemic inflammation suggests that GOD affects host metabolism by regulating altered gut microbiota.
Intervention of diet is also one of the effective ways to improve NAFLD. High dietary fiber diets, such as those rich in fruit and legume fiber (Simpson and Campbell, 2015), are involved in the remodeling process of microbial diversity, mediating the production of the dominant microflora and delaying the progression of NAFLD development. The pectin from Citrus unshiu Marc. corrected the metabolic disturbances of SCFAs in db/db mice and reduced levels of FBG, glycated serum protein (GSP), TG, TC and low density lipoprotein cholesterol (LDL-C), while increasing levels of high density lipoprotein cholesterol (HDL-C). Ren also observed the increasing of Firmicutes/Bacteroidetes and the abundance of Ligilactobacillus, Lactobacillus, Limosilactobacillus (Yanming et al., 2023). Comparing Western-type diet (WD) and fiber-enriched Mediterranean diet (FMD), the abundance of Firmicutes decreased, Bacteroidetes and Proteobacteria levels increased, and SCFA content increased, especially propionate and butyrate after FMD (Soldán et al., 2024).
Fecal microbiota transplantation (FMT) can reconstruct the composition of the gut microbiota and maintain the dynamic balance of beneficial bacteria. The concentrations of the major SCFAs, including acetate, propionate, and butyrate, were increased in patients undergoing FMT (Seekatz et al., 2018). Since most patients struggle to maintain healthy lifestyle habits, NAFLD often worsen. In recent years, FMT has become a promising treatment modality for NAFLD. In the HFD-fed mouse model built by Zhou et al. (2017), the disturbed gut microbiota was corrected after FMT, with increased abundance of beneficial bacteria Christensenellaceae and Lactobacillus and decreased Oscillibacter. The results of this study showed that FMT can inhibit the release of IL-6 and TNF-α, protect the liver, and alleviate the steatohepatitis induced by HFD (Table 1).
6 Summary
Currently, the role of gut microbiota in the development of NAFLD has become a research hotspot, and the regulation of gut microbiota is a novel target for the treatment of NAFLD. The gut microbiota metabolite SCFAs are one of the effective targets for ameliorating NAFLD. Although SCFA are seldom used directly in clinical trials, there have numerous researches showing the correlation between SCFAs and NAFLD in recent years. This review summarizes the therapeutic role of SCFAs for NAFLD by regulating glucose and lipid metabolism, improving IR, anti-inflammation, anti-oxidative stress, and improving intestinal barrier function. Increasing the content of beneficial flora and metabolite SCFAs through special diet or probiotics to restore liver metabolism is an effective method and a new idea for the treatment of NAFLD. However, the role of SCFAs is still controversial. Its effect mechanism is complex, and it will harm the human body when the concentration is too high. But in general, the impact on the host is more beneficial than harmful. Therefore, the research on the relationship between SCFAs and the human body still needs to be continued to achieve precise treatment.
Author contributions
XQ: Writing – original draft. MC: Writing – original draft. BH: Writing – review & editing. YC: Writing – review & editing. YZ: Writing – review & editing.
Funding
The author(s) declare that financial support was received for the research and/or publication of this article. This study was supported by Key Discipline Construction Project of Traditional Chinese Medicine of Zhejiang Province (Subject number: 2024-XK-25), the Zhejiang Provincial Natural Science Foundation of China (LGF22H290001), the Fund of State Administration of Traditional Chinese Medicine of Zhejiang Province (2023ZL419 and 2021ZB096).
Conflict of interest
The authors declare that the research was conducted in the absence of any commercial or financial relationships that could be construed as a potential conflict of interest.
Generative AI statement
The authors declare that no Gen AI was used in the creation of this manuscript.
Publisher’s note
All claims expressed in this article are solely those of the authors and do not necessarily represent those of their affiliated organizations, or those of the publisher, the editors and the reviewers. Any product that may be evaluated in this article, or claim that may be made by its manufacturer, is not guaranteed or endorsed by the publisher.
References
Ahn, S. B., Jun, D. W., Kang, B. K., Lim, J. H., Lim, S., and Chung, M. J. (2019). Randomized, double-blind, placebo-controlled study of a multispecies probiotic mixture in nonalcoholic fatty liver disease. Sci. Rep. 9:5688. doi: 10.1038/s41598-019-42059-3
Akhtar, M., Chen, Y., Ma, Z., Zhang, X., Shi, D., Jawaria, A. K., et al. (2022). Gut microbiota-derived short chain fatty acids are potential mediators in gut inflammation. Anim. Nutr. 8, 350–360. doi: 10.1016/j.aninu.2021.11.005
Allen, J. M., Mailing, L. J., Niemiro, G. M., Moore, R., Cook, M. D., White, B. A., et al. (2018). Exercise alters gut microbiota composition and function in lean and obese humans. Med. Sci. Sports Exerc. 50, 747–757. doi: 10.1249/MSS.0000000000001495
Anachad, O., Taouil, A., Taha, W., Bennis, F., and Chegdani, F. (2023). The implication of short-chain fatty acids in obesity and diabetes. Microbiol. Insights 16:11786361231162720. doi: 10.1177/11786361231162720
Aragonès, G., González-García, S., Aguilar, C., Richart, C., and Auguet, T. (2019). Gut microbiota-derived mediators as potential markers in nonalcoholic fatty liver disease. Biomed. Res. Int. 2019, 8507583–8507510. doi: 10.1155/2019/8507583
Barber, C., Mego, M., Sabater, C., Vallejo, F., Bendezu, R. A., Masihy, M., et al. (2021). Differential effects of Western and Mediterranean-type diets on gut microbiota: a metagenomics and metabolomics approach. Nutrients 13:2638. doi: 10.3390/nu13082638
Belcheva, A., Irrazabal, T., Robertson, S. J., Streutker, C., Maughan, H., Rubino, S., et al. (2014). Gut microbial metabolism drives transformation of MSH2-deficient colon epithelial cells. Cell 158, 288–299. doi: 10.1016/j.cell.2014.04.051
Boets, E., Gomand, S. V., Deroover, L., Preston, T., Vermeulen, K., De Preter, V., et al. (2017). Systemic availability and metabolism of colonic-derived short-chain fatty acids in healthy subjects: a stable isotope study. J. Physiol. Lond. 595, 541–555. doi: 10.1113/JP272613
Caetano, M., and Castelucci, P. (2022). Role of short chain fatty acids in gut health and possible therapeutic approaches in inflammatory bowel diseases. World J. Clin. Cases 10, 9985–10003. doi: 10.12998/wjcc.v10.i28.9985
Canfora, E. E., Jocken, J. W., and Blaak, E. E. (2015). Short-chain fatty acids in control of body weight and insulin sensitivity. Nat. Rev. Endocrinol. 11, 577–591. doi: 10.1038/nrendo.2015.128
Castillo, V., Figueroa, F., González-Pizarro, K., Jopia, P., and Ibacache-Quiroga, C. (2021). Probiotics and prebiotics as a strategy for non-alcoholic fatty liver disease, a narrative review. Food Secur. 10:1719. doi: 10.3390/foods10081719
Chen, X. F., Chen, X., and Tang, X. (2020). Short-chain fatty acid, acylation and cardiovascular diseases. Clin. Sci. (Lond.) 134, 657–676. doi: 10.1042/CS20200128
Chen, C., Gao, K., Chen, Z., Zhang, Q., Ke, X., Mao, B., et al. (2023). The supplementation of the multi-strain probiotics WHHPRO™ alleviates high-fat diet-induced metabolic symptoms in rats via gut-liver axis. Front. Nutr. 10:1324691. doi: 10.3389/fnut.2023.1324691
Chen, Q., Liu, M., Zhang, P., Fan, S., Huang, J., Yu, S., et al. (2019). Fucoidan and galactooligosaccharides ameliorate high-fat diet-induced dyslipidemia in rats by modulating the gut microbiota and bile acid metabolism. Nutrition 65, 50–59. doi: 10.1016/j.nut.2019.03.001
Cornejo-Pareja, I., Amiar, M. R., Ocaña-Wilhelmi, L., Soler-Humanes, R., Arranz-Salas, I., Garrido-Sánchez, L., et al. (2024). Non-alcoholic fatty liver disease in patients with morbid obesity: the gut microbiota axis as a potential pathophysiology mechanism. J. Gastroenterol. 59, 329–341. doi: 10.1007/s00535-023-02075-7
Cristofori, F., Dargenio, V. N., Dargenio, C., Miniello, V. L., Barone, M., and Francavilla, R. (2021). Anti-inflammatory and immunomodulatory effects of probiotics in gut inflammation: a door to the body. Front. Immunol. 12:578386. doi: 10.3389/fimmu.2021.578386
de la Cuesta-Zuluaga, J., Mueller, N. T., Corrales-Agudelo, V., Velásquez-Mejía, E. P., Carmona, J. A., Abad, J. M., et al. (2017). Metformin is associated with higher relative abundance of mucin-degrading Akkermansia muciniphila and several short-chain fatty acid-producing microbiota in the gut. Diabetes Care 40, 54–62. doi: 10.2337/dc16-1324
De Vadder, F., Kovatcheva-Datchary, P., Goncalves, D., Vinera, J., Zitoun, C., Duchampt, A., et al. (2014). Microbiota-generated metabolites promote metabolic benefits via gut-brain neural circuits. Cell 156, 84–96. doi: 10.1016/j.cell.2013.12.016
Deng, M., Qu, F., Chen, L., Liu, C., Zhang, M., Ren, F., et al. (2020). SCFAs alleviated steatosis and inflammation in mice with NASH induced by MCD. J. Endocrinol. 245, 425–437. doi: 10.1530/JOE-20-0018
Ekhlasi, G., Kolahdouz Mohammadi, R., Agah, S., Zarrati, M., Hosseini, A. F., Arabshahi, S. S., et al. (2016). Do symbiotic and vitamin E supplementation have favorite effects in nonalcoholic fatty liver disease? A randomized, double-blind, placebo-controlled trial. J. Res. Med. Sci. 21:106. doi: 10.4103/1735-1995.193178
Fachi, J. L., Felipe, J. S., Pral, L. P., da Silva, B. K., Corrêa, R. O., de Andrade, M., et al. (2019). Butyrate protects mice from Clostridium difficile-induced colitis through an HIF-1-dependent mechanism. Cell Rep. 27, 750–761.e7. doi: 10.1016/j.celrep.2019.03.054
Fan, Y., and Pedersen, O. (2021). Gut microbiota in human metabolic health and disease. Nat. Rev. Microbiol. 19, 55–71. doi: 10.1038/s41579-020-0433-9
Forslund, K., Hildebrand, F., Nielsen, T., Falony, G., Le Chatelier, E., Sunagawa, S., et al. (2015). Disentangling type 2 diabetes and metformin treatment signatures in the human gut microbiota. Nature 528, 262–266. doi: 10.1038/nature15766
Frampton, J., Murphy, K. G., Frost, G., and Chambers, E. S. (2020). Short-chain fatty acids as potential regulators of skeletal muscle metabolism and function. Nat. Metab. 2, 840–848. doi: 10.1038/s42255-020-0188-7
Friederike, S. (2018). A look at the smelly side of physiology: transport of short chain fatty acids. Pflugers Arch. 470, 571–598. doi: 10.1007/s00424-017-2105-9
Fu, K., Ma, C., Wang, C., Zhou, H., Gong, L., Zhang, Y., et al. (2022). Forsythiaside a alleviated carbon tetrachloride-induced liver fibrosis by modulating gut microbiota composition to increase short-chain fatty acids and restoring bile acids metabolism disorder. Biomed. Pharmacother. 151:113185. doi: 10.1016/j.biopha.2022.113185
Gou, X., Qin, L., Wu, D., Xie, J., Lu, Y., Zhang, Q., et al. (2023). Research Progress of Takeda G protein-coupled receptor 5 in metabolic syndrome. Molecules 28:5870. doi: 10.3390/molecules28155870
Gurav, A., Sivaprakasam, S., Bhutia, Y. D., Boettger, T., Singh, N., and Ganapathy, V. (2015). Slc5a8, a Na+−coupled high-affinity transporter for short-chain fatty acids, is a conditional tumour suppressor in colon that protects against colitis and colon cancer under low-fibre dietary conditions. Biochem. J. 469, 267–278. doi: 10.1042/BJ20150242
Ha, S., Wong, V., Zhang, X., and Yu, J. (2024). Interplay between gut microbiome, host genetic and epigenetic modifications in MASLD and MASLD-related hepatocellular carcinoma. Gut 74, 141–152. doi: 10.1136/gutjnl-2024-332398
Hao, P., Yang, X. N., Yin, W., Wang, X. Y., Ling, Y., Zhu, M. Y., et al. (2024). A study on the treatment effects of Crataegus pinnatifida polysaccharide on non-alcoholic fatty liver in mice by modulating gut microbiota. Front. Vet. Sci. 11:1383801. doi: 10.3389/fvets.2024.1383801
He, J., Zhang, P., Shen, L., Niu, L., Tan, Y., Chen, L., et al. (2020). Short-chain fatty acids and their association with Signalling pathways in inflammation, glucose and lipid metabolism. Int. J. Mol. Sci. 21:6356. doi: 10.3390/ijms21176356
Hong, J., Jia, Y., Pan, S., Jia, L., Li, H., Han, Z., et al. (2016). Butyrate alleviates high fat diet-induced obesity through activation of adiponectin-mediated pathway and stimulation of mitochondrial function in the skeletal muscle of mice. Oncotarget 7, 56071–56082. doi: 10.18632/oncotarget.11267
Horiuchi, H., Kamikado, K., Aoki, R., Suganuma, N., Nishijima, T., Nakatani, A., et al. (2020). Bifidobacterium animalis subsp. lactis GCL2505 modulates host energy metabolism via the short-chain fatty acid receptor GPR43. Sci. Rep. 10:4158. doi: 10.1038/s41598-020-60984-6
Horowitz, A., Chanez-Paredes, S. D., Haest, X., and Turner, J. R. (2023). Paracellular permeability and tight junction regulation in gut health and disease. Nat. Rev. Gastroenterol. Hepatol. 20, 417–432. doi: 10.1038/s41575-023-00766-3
Hou, Q., Huang, J., Zhao, L., Pan, X., Liao, C., Jiang, Q., et al. (2023). Dietary genistein increases microbiota-derived short chain fatty acid levels, modulates homeostasis of the aging gut, and extends healthspan and lifespan. Pharmacol. Res. 188:106676. doi: 10.1016/j.phrs.2023.106676
Hsu, C. Y., Khachatryan, L. G., Younis, N. K., Mustafa, M. A., Ahmad, N., Athab, Z. H., et al. (2024). Microbiota-derived short chain fatty acids in pediatric health and diseases: from gut development to neuroprotection. Front. Microbiol. 15:1456793. doi: 10.3389/fmicb.2024.1456793
Hu, S., Kuwabara, R., de Haan, B. J., Smink, A. M., and de Vos, P. (2020). Acetate and butyrate improve β-cell metabolism and mitochondrial respiration under oxidative stress. Int. J. Mol. Sci. 21:1542. doi: 10.3390/ijms21041542
Huang, W., Wang, J., Xiao, Z., Lin, J., Tan, Z., and Sun, G. (2024). Lingguizhugan decoction alleviates obesity in rats on a high-fat diet through the regulation of lipid metabolism and intestinal microbiota. Front. Microbiol. 15:1462173. doi: 10.3389/fmicb.2024.1462173
Ikeda, T., Nishida, A., Yamano, M., and Kimura, I. (2022). Short-chain fatty acid receptors and gut microbiota as therapeutic targets in metabolic, immune, and neurological diseases. Pharmacol. Ther. 239:108273. doi: 10.1016/j.pharmthera.2022.108273
Jaiswal, N., Akhtar, J., Singh, S. P., and Ahsan, F. (2019). An overview on Genistein and its various formulations. Drug Res. (Stuttg) 69, 305–313. doi: 10.1055/a-0797-3657
Ji, L., and Guo, L. (2020). Protective effects of glucagon-like peptide-1 on cardiovascular system. Chin. J. Diabetes 12, 654–660. doi: 10.3760/cma.j.cn115791-20200326-00179
Kim, H., Zhang, D., Song, Z., Tong, X., and Zhang, K. (2022). Analysis of insulin resistance in nonalcoholic Steatohepatitis. Methods Mol. Biol. 2455, 233–241. doi: 10.1007/978-1-0716-2128-8_18
Lazarević, S., Đanić, M., Goločorbin-Kon, S., Al-Salami, H., and Mikov, M. (2019). Semisynthetic bile acids: a new therapeutic option for metabolic syndrome. Pharmacol. Res. 146:104333. doi: 10.1016/j.phrs.2019.104333
Li, D., Bai, X., Jiang, Y., and Cheng, Y. (2021). Butyrate alleviates PTZ-induced mitochondrial dysfunction, oxidative stress and neuron apoptosis in mice via Keap1/Nrf2/HO-1 pathway. Brain Res. Bull. 168, 25–35. doi: 10.1016/j.brainresbull.2020.12.009
Li, H. P., Chen, X., and Li, M. Q. (2013). Butyrate alleviates metabolic impairments and protects pancreatic β cell function in pregnant mice with obesity. Int. J. Clin. Exp. Pathol. 6, 1574–1584.
Li, X., He, M., Yi, X., Lu, X., Zhu, M., Xue, M., et al. (2024). Short-chain fatty acids in nonalcoholic fatty liver disease: new prospects for short-chain fatty acids as therapeutic targets. Heliyon 10:e26991. doi: 10.1016/j.heliyon.2024.e26991
Li, X., Shimizu, Y., and Kimura, I. (2017). Gut microbial metabolite short-chain fatty acids and obesity. Biosci. Microbiota Food Health 36, 135–140. doi: 10.12938/bmfh.17-010
Li, W., Zhang, K., and Yang, H. (2018). Pectin alleviates high fat (lard) diet-induced nonalcoholic fatty liver disease in mice: possible role of short-chain fatty acids and gut microbiota regulated by pectin. J. Agric. Food Chem. 66, 8015–8025. doi: 10.1021/acs.jafc.8b02979
Liu, P., Wang, Y., Yang, G., Zhang, Q., Meng, L., Xin, Y., et al. (2021). The role of short-chain fatty acids in intestinal barrier function, inflammation, oxidative stress, and colonic carcinogenesis. Pharmacol. Res. 165:105420. doi: 10.1016/j.phrs.2021.105420
Louis, P., and Flint, H. J. (2017). Formation of propionate and butyrate by the human colonic microbiota. Environ. Microbiol. 19, 29–41. doi: 10.1111/1462-2920.13589
Lu, J. Z., Ye, D., and Ma, B. L. (2021). Constituents, pharmacokinetics, and pharmacology of Gegen-Qinlian decoction. Front. Pharmacol. 12:668418. doi: 10.3389/fphar.2021.668418
Ma, Q., Zhai, R., Xie, X., Chen, T., Zhang, Z., Liu, H., et al. (2022). Hypoglycemic effects of Lycium barbarum polysaccharide in type 2 diabetes mellitus mice via modulating gut microbiota. Front. Nutr. 9:916271. doi: 10.3389/fnut.2022.916271
Mariño, E., Richards, J. L., McLeod, K. H., Stanley, D., Yap, Y. A., Knight, J., et al. (2017). Gut microbial metabolites limit the frequency of autoimmune T cells and protect against type 1 diabetes. Nat. Immunol. 18, 552–562. doi: 10.1038/ni.3713
McMurdie, P. J., Stoeva, M. K., Justice, N., Nemchek, M., Sieber, C., Tyagi, S., et al. (2022). Increased circulating butyrate and ursodeoxycholate during probiotic intervention in humans with type 2 diabetes. BMC Microbiol. 22:19. doi: 10.1186/s12866-021-02415-8
Monga Kravetz, A., Testerman, T., Galuppo, B., Graf, J., Pierpont, B., Siebel, S., et al. (2020). Effect of gut microbiota and PNPLA3 rs738409 variant on nonalcoholic fatty liver disease (NAFLD) in obese youth. J. Clin. Endocrinol. Metab. 105, e3575–e3585. doi: 10.1210/clinem/dgaa382
Nastasi, C., Candela, M., Bonefeld, C. M., Geisler, C., Hansen, M., Krejsgaard, T., et al. (2015). The effect of short-chain fatty acids on human monocyte-derived dendritic cells. Sci. Rep. 5:16148. doi: 10.1038/srep16148
Psichas, A., Sleeth, M. L., Murphy, K. G., Brooks, L., Bewick, G. A., Hanyaloglu, A. C., et al. (2015). The short chain fatty acid propionate stimulates GLP-1 and PYY secretion via free fatty acid receptor 2 in rodents. Int. J. Obes. 39, 424–429. doi: 10.1038/ijo.2014.153
Rani, V., Deep, G., Singh, R. K., Palle, K., and Yadav, U. C. (2016). Oxidative stress and metabolic disorders: pathogenesis and therapeutic strategies. Life Sci. 148, 183–193. doi: 10.1016/j.lfs.2016.02.002
Reunanen, J., Kainulainen, V., Huuskonen, L., Ottman, N., Belzer, C., Huhtinen, H., et al. (2015). Akkermansia muciniphila adheres to enterocytes and strengthens the integrity of the epithelial cell layer. Appl. Environ. Microbiol. 81, 3655–3662. doi: 10.1128/AEM.04050-14
Schoenfeld, P., and Wojtczak, L. (2016). Short- and medium-chain fatty acids in energy metabolism: the cellular perspective. J. Lipid Res. 57, 943–954. doi: 10.1194/jlr.R067629
Scorletti, E., Afolabi, P. R., Miles, E. A., Smith, D. E., Almehmadi, A., Alshathry, A., et al. (2020). Synbiotics Alter fecal microbiomes, but not liver fat or fibrosis, in a randomized trial of patients with nonalcoholic fatty liver disease. Gastroenterology 158, 1597–1610.e7. doi: 10.1053/j.gastro.2020.01.031
Seekatz, A. M., Theriot, C. M., Rao, K., Chang, Y. M., Freeman, A. E., Kao, J. Y., et al. (2018). Restoration of short chain fatty acid and bile acid metabolism following fecal microbiota transplantation in patients with recurrent Clostridium difficile infection. Anaerobe 53, 64–73. doi: 10.1016/j.anaerobe.2018.04.001
Simpson, H. L., and Campbell, B. J. (2015). Review article: dietary fibre-microbiota interactions. Aliment. Pharmacol. Ther. 42, 158–179. doi: 10.1111/apt.13248
Singh, T. P., Kadyan, S., Devi, H., Park, G., and Nagpal, R. (2023). Gut microbiome as a therapeutic target for liver diseases. Life Sci. 322:121685. doi: 10.1016/j.lfs.2023.121685
Singh, N., Thangaraju, M., Prasad, P. D., Martin, P. M., Lambert, N. A., Boettger, T., et al. (2010). Blockade of dendritic cell development by bacterial fermentation products butyrate and propionate through a transporter (Slc5a8)-dependent inhibition of histone deacetylases. J. Biol. Chem. 285, 27601–27608. doi: 10.1074/jbc.M110.102947
Sivaprakasam, S., Bhutia, Y. D., Yang, S., and Ganapathy, V. (2017). Short-chain fatty acid transporters: role in colonic homeostasis. Compr. Physiol. 8, 299–314. doi: 10.1002/cphy.c170014
Soldán, M., Argalášová, Ľ., Hadvinová, L., Galileo, B., and Babjaková, J. (2024). The effect of dietary types on gut microbiota composition and development of non-communicable diseases: a narrative review. Nutrients 16:3134. doi: 10.3390/nu16183134
Song, Q., Cheng, S. W., Li, D., Cheng, H., Lai, Y. S., Han, Q., et al. (2022). Gut microbiota mediated hypoglycemic effect of Astragalus membranaceus polysaccharides in db/db mice. Front. Pharmacol. 13:1043527. doi: 10.3389/fphar.2022.1043527
Song, L., Hu, Y., Xu, Y., and Guo, L. (2022). Re-conceptualization of insulin resistance. Chin. J. Diabetes 14, 1341–1347. doi: 10.3760/cma.j.cn115791-20220921-00485
Tang, T., Song, J., Li, J., Wang, H., Zhang, Y., and Suo, H. (2020). A synbiotic consisting of Lactobacillus plantarum S58 and hull-less barley β-glucan ameliorates lipid accumulation in mice fed with a high-fat diet by activating AMPK signaling and modulating the gut microbiota. Carbohydr. Polym. 243:116398. doi: 10.1016/j.carbpol.2020.116398
Tanya, R., Shahnawaz, A., Zoya, S., Abdulsalam, A., Vidhu, A., Mohd, A., et al. (2024). Exploring the therapeutic potential of silymarin-based herbal remedy (prebiotic) and probiotic blend in a mouse model of NAFLD: insights into gut microbiota modulation and liver health. Heliyon 10:e33505. doi: 10.1016/j.heliyon.2024.e33505
Tokuhara, D. (2021). Role of the gut microbiota in regulating non-alcoholic fatty liver disease in children and adolescents. Front. Nutr. 8:700058. doi: 10.3389/fnut.2021.700058
Wang, B., Jiang, X., Cao, M., Ge, J., Bao, Q., Tang, L., et al. (2016). Altered fecal microbiota correlates with liver biochemistry in nonobese patients with non-alcoholic fatty liver disease. Sci. Rep. 6:32002. doi: 10.1038/srep32002
Wang, H., Zhang, Y., Xu, M., Zhang, W., Liu, C., and Chen, S. (2020). Research progresses on PGC-1α, a key energy metabolic regulator. Acta Physiol. Sin. 72, 804–816. doi: 10.13294/j.aps.2020.0046
Wu, Z., Liu, X., Huang, S., Li, T., Zhang, X., Pang, J., et al. (2022). Milk fat globule membrane attenuates acute colitis and secondary liver injury by improving the mucus barrier and regulating the gut microbiota. Front. Immunol. 13:865273. doi: 10.3389/fimmu.2022.865273
Xu, X., Gao, Z., Yang, F., Yang, Y., Chen, L., Han, L., et al. (2020). Antidiabetic effects of Gegen Qinlian decoction via the gut microbiota are attributable to its key ingredient Berberine. Genomics Proteomics Bioinformatics 18, 721–736. doi: 10.1016/j.gpb.2019.09.007
Yamato, E. (2018). High dose of histone deacetylase inhibitors affects insulin secretory mechanism of pancreatic beta cell line. Endocr. Regul. 52, 21–26. doi: 10.2478/enr-2018-0004
Yang, Z., Su, H., Lv, Y., Tao, H., Jiang, Y., Ni, Z., et al. (2023). Inulin intervention attenuates hepatic steatosis in rats via modulating gut microbiota and maintaining intestinal barrier function. Food Res. Int. 163:112309. doi: 10.1016/j.foodres.2022.112309
Yanming, R., Shuifang, M., Yujun, Z., Shiguo, C., Jinhu, T., and Xingqian, Y. (2023). Pectin from Citrus unshiu Marc. Alleviates glucose and lipid metabolism by regulating the gut microbiota and metabolites. Foods 12:4094. doi: 10.3390/foods12224094
Yao, Y., Yan, L., Chen, H., Wu, N., Wang, W., and Wang, D. (2020). Cyclocarya paliurus polysaccharides alleviate type 2 diabetic symptoms by modulating gut microbiota and short-chain fatty acids. Phytomedicine 77:153268. doi: 10.1016/j.phymed.2020.153268
Zhang, S., Zhao, J., Xie, F., He, H., Johnston, L. J., Dai, X., et al. (2021). Dietary fiber-derived short-chain fatty acids: a potential therapeutic target to alleviate obesity-related nonalcoholic fatty liver disease. Obes. Rev. 22:e13316. doi: 10.1111/obr.13316
Zhao, Y., Zhou, J., Liu, J., Wang, Z., Chen, M., and Zhou, S. (2019). Metagenome of gut microbiota of children with nonalcoholic fatty liver disease. Front. Pediatr. 7:518. doi: 10.3389/fped.2019.00518
Zheng, L. T., Wang, Z., Chen, Y. C., Liu, S. S., Xie, Y. S., Li, C. Y., et al. (2024). Role of Akkermansia muciniphila in nonalcoholic fatty liver disease. J. Clin. Hepatol. 40, 594–599. doi: 10.12449/JCH240326
Zhou, D., Pan, Q., Shen, F., Cao, H. X., Ding, W. J., Chen, Y. W., et al. (2017). Total fecal microbiota transplantation alleviates high-fat diet-induced steatohepatitis in mice via beneficial regulation of gut microbiota. Sci. Rep. 7:1529. doi: 10.1038/s41598-017-01751-y
Zhou, X., Wu, D., Mi, T., and Li, W. (2023). Developments on the biological role of leptin and its related mechanisms. Med. Res. Educ. 40, 1–10. doi: 10.3969/j.issn.1674-490X.2023.04.001
Keywords: non-alcoholic fatty liver disease, short-chain fatty acids, gut microbiota, glycolipid metabolism, therapy
Citation: Qin X, Chen M, He B, Chen Y and Zheng Y (2025) Role of short-chain fatty acids in non-alcoholic fatty liver disease and potential therapeutic targets. Front. Microbiol. 16:1539972. doi: 10.3389/fmicb.2025.1539972
Edited by:
Yongli Ye, Jiangnan University, ChinaReviewed by:
Szymon Suwala, Nicolaus Copernicus University in Toruń, PolandCopyright © 2025 Qin, Chen, He, Chen and Zheng. This is an open-access article distributed under the terms of the Creative Commons Attribution License (CC BY). The use, distribution or reproduction in other forums is permitted, provided the original author(s) and the copyright owner(s) are credited and that the original publication in this journal is cited, in accordance with accepted academic practice. No use, distribution or reproduction is permitted which does not comply with these terms.
*Correspondence: Yuelin Zheng, NDE4NTYwNDcyQHFxLmNvbQ==
†These authors have contributed equally to this work and share first authorship