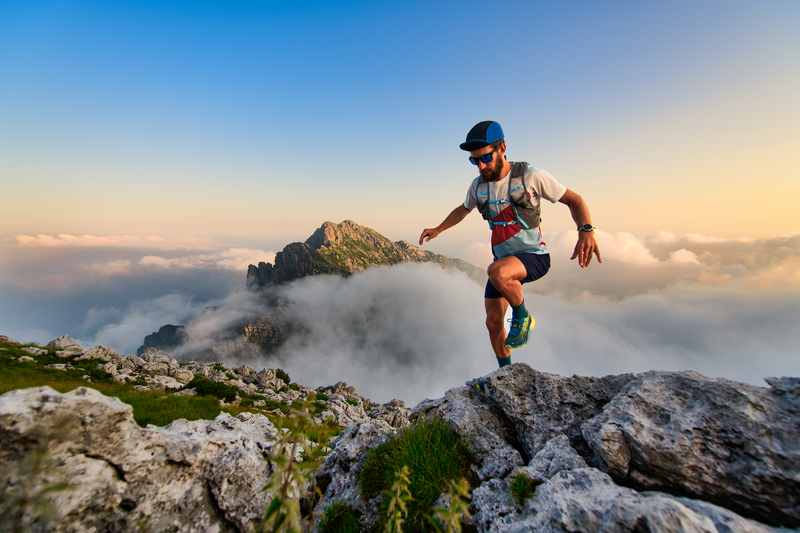
95% of researchers rate our articles as excellent or good
Learn more about the work of our research integrity team to safeguard the quality of each article we publish.
Find out more
REVIEW article
Front. Microbiol. , 03 February 2025
Sec. Antimicrobials, Resistance and Chemotherapy
Volume 16 - 2025 | https://doi.org/10.3389/fmicb.2025.1526250
The global crisis of antimicrobial resistance (AMR) is escalating due to the misuse and overuse of antibiotics, the slow development of new therapies, and the rise of multidrug-resistant (MDR) infections. Traditional antibiotic treatments face limitations, including the development of resistance, disruption of the microbiota, adverse side effects, and environmental impact, emphasizing the urgent need for innovative alternative antibacterial strategies. This review critically examines naturally derived biopolymers with intrinsic (essential feature) antibacterial properties as a sustainable, next-generation alternative to traditional antibiotics. These biopolymers may address bacterial resistance uniquely by disrupting bacterial membranes rather than cellular functions, potentially reducing microbiota interference. Through a comparative analysis of the mechanisms and applications of antibiotics and antibacterial naturally derived biopolymers, this review highlights the potential of such biopolymers to address AMR while supporting human and environmental health.
The World Health Organization (WHO) estimates that antimicrobial resistance (AMR) could cause up to 10 million deaths annually by 2050, with a severe impact on global healthcare costs and economic stability. Bacterial infections are among the most life-threatening healthcare challenges, accounting for approximately 13.6% of global mortality and affecting 1 in 8 individuals worldwide (Appanna, 2018). The rise of multidrug-resistant (MDR) bacteria further highlights an urgent need for alternative, next-generation antibacterial treatments. While antibiotics have historically revolutionized healthcare, their widespread use has led to substantial challenges, including disrupting human microbiota and the rise of antibiotic-resistant bacterial strains.
Beyond their pathogenic roles, bacteria are integral to human health, especially in the gut, contributing to immune modulation and digestion (Bull and Plummer, 2014; Yin et al., 2019; Arciola et al., 2018). Consequently, the modern healthcare system cannot fully eliminate infection risk, particularly in post-surgical settings (Oliva et al., 2021; Hedrick et al., 2006; van Seventer and Hochberg, 2017) and procedures involving biomaterials or medical devices (Arciola et al., 2018; Oliva et al., 2021; Hedrick et al., 2006). Infections occur when infectious agents enter human body tissues, multiply, and trigger host immune responses (van Seventer and Hochberg, 2017). Although infections cannot be entirely prevented due to inevitable interactions with environmental microbes, targeted measures can mitigate bacterial invasion and inhibit replication at potential infection sites.
The human microbiome, especially the gut microbiota, comprises numerous symbiotic bacterial species (e.g., Lactobacillus, Bacillus, Clostridium, Enterococcus, and Ruminococcus) that collectively represent approximately 90% of the gut flora (Rinninella et al., 2019; Martín et al., 2013; Eloe-Fadrosh and Rasko, 2013). These beneficial microorganisms are crucial in digestion, nutrient absorption, and immune defense. Importantly, they maintain a delicate balance, contributing to immune regulation and protecting against pathogens without causing harm to the host (Rinninella et al., 2019; Bogitsh et al., 2019; Hemarajata and Versalovic, 2013; Isolauri et al., 2001; Wieërs et al., 2020; Hills et al., 2019; Ding et al., 2019). However, the broad-spectrum use of antibiotics has disrupted this balance, weakening the microbiota’s natural protective functions and impairing the immune response, leading to gut dysbiosis—an environment conducive to antibiotic-resistant strains (Patangia et al., 2022).
While antibiotics effectively eliminate harmful pathogens, their indiscriminate targeting also affects beneficial bacteria, reducing microbial diversity and increasing the likelihood of antibiotic resistance (Lathakumari et al., 2024). Inappropriate antibiotic use across sectors, including clinical and animal health, has further escalated the global antibiotic resistance crisis, contributing to the emergence of MDR pathogens that resist multiple antibiotic classes (Yang et al., 2024).
The decreasing efficacy of antibiotics and the limited availability of alternative treatments underscore the urgent need for new classes of antibacterial therapeutics. Ideal alternatives would incorporate mechanisms of action that lower the risk of resistance. In this context, naturally derived biopolymers (NDBs) have attracted significant attention due to their unique antibacterial properties. Although long used in biomedical applications, interest in biopolymers as antibacterial agents has surged, with publications on their use rising by approximately 400% since 2015 (Web of Science, n.d.).
Approximately two decades ago, antibacterial biopolymers, e.g., those with intrinsic antibacterial activity, were first proposed as alternatives to antibiotics for treating bacterial infections (Muñoz-Bonilla and Fernández-García, 2012). Today, biopolymer-based strategies show potential for localized, non-antibiotic antibacterial applications that support the immune system and minimize impact on the natural microbiota. Such approaches could represent a sustainable innovation within modern healthcare.
Notably, NDBs disrupt bacterial membranes instead of targeting specific metabolic pathways, a mechanism less prone to resistance development (Bustamante-Torres et al., 2022; Kamaruzzaman et al., 2019). Numerous studies have documented the use of NDBs in biomedical devices, including drug delivery systems, contact lenses, and injectable cement, where they exhibit potent antibacterial activity and biocompatibility (Pahlevanzadeh et al., 2022; Sam et al., 2023; Coma, 2013). This review provides a comprehensive examination of the potential of antibacterial NDBs, analyzing recent literature to compare their effectiveness and applications with those of conventional antibiotics. By exploring the mechanisms, advantages, and limitations of NDBs, this review assesses whether these biopolymers could serve as reliable, antibiotic-free therapeutics capable of complementing or partially replacing traditional antibiotics in treating bacterial infections—or whether their promise remains largely theoretical.
In the pre-antibiotic era, more than half of deaths were attributable to infections (Aminov, 2010). Since the 20th century, antibiotics have revolutionized antibacterial therapeutics in the history of medicine, drastically changing modern medicine and extending the average human lifespan (Johnston and Badran, 2022; Cook and Wright, 2022; Sikdar et al., 2021). Several groups and generations of antibiotics have been discovered and developed with specific target mechanisms of action on bacterial cells (Kapoor et al., 2017; Coates et al., 2011) (Table 1). Conventionally, antibiotics are classified as cell wall inhibitors, protein synthesis inhibitors, nucleic acid synthesis inhibitors, antimetabolites, and cytoplasmic membrane inhibitors (Pancu et al., 2021; Ullah and Ali, 2017) (Figure 1).
Table 1. Classification of antibiotics based on the mechanism of action and chemical structure with characterization: action mechanisms, reported side effects, and bacterial resistance mechanisms.
Figure 1. Classification of antibiotics (created by Biorender).
Other classification principles of antibiotics rely on their origin, spectrum of action, administration strategies, chemical structure, and mechanism of action (Figure 1). Antibiotics can be administered through various routes, including oral, intravenous, intramuscular, and topical applications (Buonavoglia et al., 2021; Enenkel and Stille, 1988). The topical application is particularly relevant for localized infections, such as skin wounds or mucosal infections, where direct delivery to the affected area can enhance efficacy and minimize systemic side effects. The effectiveness of these administration strategies depends on various factors, including bioavailability, drug formulation, gastrointestinal conditions, and systemic distribution, which, if not optimized, could compromise therapeutic outcomes and limit the antibiotic’s efficacy against targeted infections (McCarthy and Avent, 2020; Vinarov et al., 2021). Moreover, antibiotics exhibit specific behavioral characteristics, including whether they are bactericidal or bacteriostatic, as well as their spectrum, which can be broad or narrow. Antibiotics with a wide spectrum and bactericidal action may impact the microbiota within organisms’ niches (Dubourg et al., 2014; Blaser, 2011; Yang et al., 2021), resulting in dysbacteriosis conditions post-therapy and an increasing risk of secondary disease. In addition, the systemic use of antibiotics has been documented to affect various organ systems, leading to heightened organism toxicity (Berry et al., 1995; Grill and Maganti, 2011) (Table 1).
Currently, bacteriophage therapy is the only alternative as effective as antibiotics. Phage therapy relies on using naturally occurring bacteriophages (viruses) to infect and lyse bacteria at the site of infection (Lin et al., 2017). However, phage therapy must still be licensed in the majority of countries or used under exceptional situations (Yang et al., 2023). Thus, antibiotics remain the primary treatment option in clinics to combat bacterial infections. However, the development of antibiotics has begun an endless race against pathogenic microorganisms. As a side problem, the overuse and misuse of these lifesaving drugs have developed the top global public health crisis named antibiotic resistance occurring worldwide (Akram et al., 2023). Antibiotic resistance arose from the evolutionary development of primary (antibiotic target site is not presented in bacteria strain) and secondary (genome-related and plasmid-related) resistance mechanisms in bacteria (Urban-Chmiel et al., 2022; Nilsson, 2019; Zhang and Cheng, 2022) (Table 1). The dramatic report by the WHO has shown that by 2050, drug resistance could catch up to cancer and sufficiently damage the economy if actions are not taken (World Health Organization, 2019). The uncontrolled use of antibiotics in agriculture and inappropriate therapeutic practices has provided an evolutionary advantage to bacteria, such as methicillin-resistant Staphylococcus aureus (MRSA), vancomycin-resistant Enterococcus (VRE), and others that have become resistant to one or multiple types of antibiotics, contributing to the dramatic situation in healthcare (Figure 2).
Figure 2. (A) Antibiotic discovery (title of antibiotics and relative point within timeline decade) and resistance reports timeline (resistance report against specific antibiotic with a title and relative point within timeline decade) (Dahal and Chaudhary, 2018). (B) Case map on three priority levels (I priority stands for critically high level; II priority – high level, and III – average level of resistance cases) resistant strains (carbapenem-resistant P. aeruginosa; methicillin-resistant S. aureus, and penicillin-resistant S. pneumoniae) in Europe, 2022, as well as a supporting table on resistant isolates’ case percentage and colorful indicator for priority, with green indicating low level and dark red indicating critically high level (Surveillance Atlas of Infectious Diseases, 2022). (C) Comparative graphs of the same three priority resistant strains with color indication in the top three most populated world countries: China, India, and the United States of America (USA) (ResistanceMap, n.d.) (created by Biorender).
The global threat of antimicrobial resistance (AMR) necessitates collaborative action to develop and implement effective strategies (Uchil et al., 2014). Several preventative measures have been established and continue to evolve, addressing AMR at international, national, community, hospital, and individual levels (Uchil et al., 2014). At the international level, efforts focus on enhancing collaboration among governments, non-governmental organizations, professional groups, and international agencies. Key initiatives include global networks for antimicrobial use and resistance surveillance, strategies to combat counterfeit antimicrobials, and programs to foster innovation in new drugs and vaccines. Strengthening global AMR control programs remains a priority. Nationally, dedicated committees and AMR policies have been introduced to monitor and manage AMR. These policies integrate geographical, social, and economic factors to provide tailored solutions. Educational initiatives, including training programs and certification courses, aim to equip healthcare professionals and the private sector with knowledge for the rational use of antibiotics. Regulatory controls to limit over-the-counter antibiotic sales further address misuse, a key driver of resistance. For example, a review revealed that non-prescription antibiotic use varies widely, from 3% in Northern Europe to 100% in some African regions (Uchil et al., 2014; Morgan et al., 2011). In addition, efforts are directed at improving standards in healthcare systems, microbiology laboratories, and pharmaceutical companies. Protecting existing antibiotic therapies remains critical, with ongoing research focused on developing new drugs to replace outdated ones and prolonging the effectiveness of current treatments. It has already been proven that synergy and drug combinations are a winning strategy in fighting multidrug-resistant bacteria and might help protect the existing drugs through antibiotic adjuvants. For instance, β-lactamase inhibitors have been used as adjuvants for penicillin group antibiotics as they block the resistance mechanism of bacteria against these antibiotics (see Table 1). Other examples include efflux pump inhibitors and outer membrane permeabilizers (Annunziato, 2019).
Naturally derived biopolymers (NDBs) are large macromolecules from living organisms such as plants and microorganisms. These polymers are formed through enzyme-catalyzed chain-growth polymerization processes of activated monomers (Sun et al., 2022). The molecular size of NDBs varies significantly based on their type and source, ranging from a few kilodaltons (kDa), as seen in polysaccharides such as chitosan (~10–50 kDa), to several megadaltons (MDa), such as cellulose and other structural polysaccharides (>1 MDa) (Moradali and Rehm, 2020; Yadav et al., 2015). This broad size range supports their diverse physicochemical properties and wide-ranging applications (Troy et al., 2021; Reddy et al., 2021).
The reason is that the source of these compounds is derived from living organisms through enzymatic polymerization, forming high molecular weight macromolecules. As a result, covalently bonded repetitive monomeric units form biodegradable compounds such as polysaccharides, polyamino acids, hydroxy fatty acids, polypeptides, and glycolipids (Moradali and Rehm, 2020; Yadav et al., 2015; Troy et al., 2021) (see Table 2). These compounds are classified as NDBs and have unique physical, chemical, and mechanical properties, which are exploited in biomedical applications. NDBs are commonly used in the development of drug delivery systems (Baranwal et al., 2022; Murali and Jayakumar, 2023; Atanase, 2021). In addition, NDBs such as collagen, gelatin, dextran, agarose/alginate, hyaluronic acid, cellulose, and fibrin are also being explored in various other biomedical applications, including open incision/wound suturing, fixing, adhesion, covering, occlusion, isolation, contact inhibition, cell proliferation, tissue guiding, and controlled drug administration (Baranwal et al., 2022). NDBs are of broad interest because of their potential to be used for developing environmentally friendly medical devices that perform high biocompatibility and serve as highly accurate biosensors, drug delivery systems, etc. (Manoukian et al., 2019). In addition to biocompatibility, biodegradation, bioadhesiveness, and biofunctionality of the NDBs, several drawbacks must be addressed, such as low stability, low melting point, high surface tension, structural complexity, and well-known immunological response from organisms (Ige et al., 2012; Jenkins et al., 1996; Reddy et al., 2021). Various NDBs such as chitosan, pectin, κ-carrageenan, alginate, ε-polylysine, and others have also been identified for their antibacterial activity (Muñoz-Bonilla et al., 2019; Li et al., 2021; Habeeb and Abdulkadhim, 2024; Hamidi et al., 2023) (see Table 2). Numerous studies have demonstrated the comparative effectiveness and potential advantages of NDBs over conventional antibiotics. For instance, Tin et al. (2009) reported that chitosan molecules with different molecular weights consistently exhibited a minimum inhibitory concentration (MIC) of 32 μg/mL against various strains of P. aeruginosa. In contrast, the MIC range for sulfamethoxazole was significantly broader, ranging from 64 to 2048 μg/mL (Tin et al., 2009). Similarly, Si et al. (2021) found that a chitosan derivative effectively inhibited Gram-negative and Gram-positive bacteria, with MIC values ranging from 8 to 32 μg/mL. Specifically, against A. baumannii, the chitosan derivative achieved an MIC of 32 μg/mL, compared to higher MIC values of 128 μg/mL for amikacin and tobramycin and 64 μg/mL for tazobactam. However, certain antibiotics outperformed NDBs in specific cases; for example, novobiocin demonstrated an MIC of 8 μg/mL, and carbenicillin and tobramycin were more effective against MRSA (Si et al., 2021). Another noteworthy example is ε-polylysine, which exhibited MIC values of 500, 800, 800, and 1,000 μg/mL against P. aeruginosa, K. pneumoniae, MSSA, and MRSA, respectively. Traditional antibiotics such as ampicillin, gentamicin, and tetracycline showed MIC values ranging from 35 to 250 μg/mL against the same bacterial strains (Sundaran et al., 2022). Importantly, combining NDBs with antibiotics has synergistic effects, significantly enhancing antibacterial activity and reducing the required antibiotic dosage (Si et al., 2021; Taheri-Araghi, 2024; Fayed et al., 2023; Baltimore et al., 1987; Khan et al., 2012; Kaur et al., 2022).
Considering the advantageous functionalities such as biodegradability, low immunogenicity, and non-toxicity of naturally derived biopolymer-based drug delivery systems, the antibacterial feature opens new horizons for developing local targeted antibacterial therapeutics based on antibacterial biopolymers. Countless reviews and studies have demonstrated the ability of NDBs to inhibit a broad spectrum of Gram-positive and Gram-negative bacteria, including bacterial strains currently being classified as “under urgent attention” due to their resistance to various antibiotics (Bustamante-Torres et al., 2022; Poznanski et al., 2023; Rofeal et al., 2022), as well as fungi (Poznanski et al., 2023; Ntow-Boahene et al., 2021) and viruses (Akbari et al., 2022; Bianculli et al., 2020). Several studies have highlighted that various naturally derived biopolymers (NDBs) exhibit notable antibiofilm activity (see Table 2). These antibiofilm mechanisms primarily involve disrupting biofilm exopolysaccharides (EPS), a critical component for biofilm stability. Such disruptions can lead to the detachment of bacterial cells or inhibit bacterial adhesion during the early stages of biofilm formation (Mishra et al., 2020; Melander et al., 2020). In addition, certain NDBs, such as lactoferrin-derived peptides, neutrophil peptides, and antimicrobial peptides (e.g., protegrin-1), have demonstrated antibacterial activity against intracellular pathogens, including Mycobacterium tuberculosis. These antibacterial effects are attributed to the disruption of the mycobacterial cell wall and enhanced membrane permeabilization (Khara et al., 2020; Jacobo-Delgado et al., 2023; Intorasoot et al., 2022). Despite these promising findings, significant challenges remain in translating NDBs into clinical applications. Key limitations include variability in their physicochemical and mechanical properties, which can impact reproducibility and reliability in therapeutic settings (Moradali and Rehm, 2020; Yadav et al., 2015). The limited physicochemical stability and difficulty tuning their biodegradation profiles further complicate their development as viable therapeutic solutions (Muñoz-Bonilla and Fernández-García, 2012; Pahlevanzadeh et al., 2022). In addition, the transition from laboratory-scale research to clinical application faces substantial barriers, including extensive preclinical testing to establish safety and efficacy, the complexities of large-scale manufacturing to ensure consistent quality, and the rigorous regulatory approval processes that demand considerable time and resources (Oliva et al., 2021; Murali and Jayakumar, 2023). Addressing these challenges will require interdisciplinary approaches and sustained efforts to optimize the properties of NDBs and streamline their development pipeline for clinical use (Arciola et al., 2018; Kong et al., 2023).
In further sections, the antibacterial potential of NDBs will be discussed to understand biopolymer interaction with bacterial cells, inhibition/bactericidal mechanism, and application specifics, to compare all these aspects with currently used conventional antibiotics, and to address the question posed in the title of this review.
Based on R&D reports, the most common local modes of delivery of antibacterial naturally derived biopolymers (aNDBs) to treat desired sites for various biomedical applications are summarized in Figure 3. Local application options and antibacterial activity are the main advantages reported in numerous studies for such biopolymers (Wu et al., 2022; Jarosz et al., 2023; Kong et al., 2023). Local delivery is preferable as it achieves the target site at the same concentration as it was prepared directly without passing through all the body barriers via the bloodstream and without losing biopolymer molecules. Such local delivery types include creams, ointments, and gels that are applied on the skin, burn or opened wounds, and surgery sites to prevent and treat infection (Zhao et al., 2023); intraoperative coatings and fillers (Ilić-Stojanović et al., 2023) are used for deeper surgical sites or dental sockets post-tooth extraction to avoid or combat already infected site; implant and catheters coating that allows preventing implant-and catheter-associated infections (Veiga and Schneider, 2013); and controlled delivery systems that achieve and bind to targeted site via different stimuli or due to specific conditions (temperature and pH), followed by antibacterial activity while providing controlled cell/ion/growth factor release from the matrix (Jacob et al., 2018) (Figure 3). This local delivery feature gives an advantage compared to conventional administration of antibiotics orally (pills and suspensions) or intravenously (in particular cases). While oral administration is convenient and suitable for at-home antibiotic therapy, it is associated with a decline in the concentration of the active compound upon reaching the infection site (Homayun et al., 2019; Kim and De Jesus, 2023). In addition, this strategy negatively impacts natural body microbiota, directly affecting the patient’s immune system response to continuous or new bacteria invasion (Konstantinidis et al., 2020). Nevertheless, it is worth acknowledging the efficacy of oral drug administration in systemic or severe infections at multiple sites (Cunha, 2006). However, various studies have reported that combined device development where aNDBs served as a drug delivery system with encapsulated antibiotics might have a promising synergistic effect to achieve the exact infection site (Liu et al., 2022; Khan et al., 2021; Meng et al., 2014; Hwang et al., 2023; Schrade et al., 2022).
Figure 3. Overview of naturally derived biopolymers for local delivery applications (Biswas et al., 2022; Dimri et al., 2023; Ibrahim et al., 2019) (created by Biorender).
Regarding aNDBs, it is crucial to understand that these biopolymer molecules, unlike the previously described antibiotics, do not target specific synthesis pathways or molecules. First, it is worth mentioning that bacterial cell wall outer structures serve as adhesion and pathogenicity factors; for example, lipopolysaccharides and phospholipids of Gram-negative bacteria and teichoic and lipoteichoic acids of Gram-positive bacteria are negatively charged. Second, aNDBs consist of positively charged molecules (chondroitin sulfate, o-pullulan, alginate, ε-polylysine, chitosan, magainin-2, etc.), containing cationic groups such as quaternary ammonium, quaternary phosphonium, guanidinium, or tertiary sulfonium (Santos et al., 2016), which have a positive charge. As a result, the interaction between biopolymers and bacteria begins with mutual attachment caused by electrostatic forces (Haktaniyan and Bradley, 2022). As a result, if aNDB molecules and bacteria cells are close enough, oppositely charged molecules attract each other, leading to physical binding (Figure 4). Another essential fact is that not all cationic molecules are lethal to bacteria. The electrostatic interaction represents just the first step toward the bactericidal effect of aNDBs. Second, a specific concentration of the cationicity of aNDBs must be achieved to reach a multivalence effect (Smola-Dmochowska et al., 2023) that results in the simultaneous binding of aNDB molecules to the bacterial cell structures and moving to the next step.
Figure 4. Biopolymer (aNDBs)—bacterial cell interaction (left) and bactericidal mechanisms (right) (created by Biorender).
In the next step, aNDB mechanisms of action on bacterial cell walls are divided into pore-forming and micelle-forming mechanisms (Qiu et al., 2020; Zhou et al., 2023). The pore-forming mechanism can be further categorized into two models: barrel-stave pore and toroidal pore (Kumar et al., 2018; Pastore et al., 2020; Mihajlovic and Lazaridis, 2010). Within the barrel-stave model, the aNDB molecules are initially oriented parallel to the membrane but eventually inserted perpendicularly in the lipid bilayer (Hegde et al., 2022) (Figure 4). This promotes lateral peptide–peptide interactions such as membrane protein ion channels. Hydrophobic regions interact with membrane lipids, and hydrophilic residues form the lumen of the channels (Brogden, 2005). On the other hand, in the toroidal pore model, peptides are also inserted perpendicularly in the lipid bilayer, but specific peptide–peptide interactions are not present (Wimley, 2010). Instead, the peptides induce a local curvature of the lipid bilayer, with the pores partly formed by peptides and partly by the phospholipid head group. The dynamic and transient lipid–peptide supramolecule is the “toroidal pore.” The key distinguishing feature of this model, compared to the barrel-stave pore, is the net arrangement of the bilayer. In the barrel-stave pore, the hydrophobic and hydrophilic arrangement of the lipids is maintained, whereas, in toroidal pores, the hydrophobic and hydrophilic arrangement of the bilayer is disrupted. This provides alternate surfaces for interacting with the lipid tail and head group. As the pores are transient upon disintegration, some peptides translocate to the inner cytoplasmic leaflet, entering the cytoplasm and potentially targeting intracellular components (Kumar et al., 2018). Other features of the toroidal pores include ion selectivity and discrete size (Yeaman and Yount, 2003). Due to pore formation, joint cell wall integrity and permeability are disrupted, resulting in bacterial cell lysis.
The micelle-forming mechanism is usually called the “Carpet-like” model (Wimley, 2010; Huan et al., 2020; Shai, 2002). In this case, the aNDBs adsorb parallel to the lipid bilayer and reach a threshold concentration to cover the surface of the membrane, thereby forming a “carpet” (Figure 4). This leads to unfavorable interactions on the membrane surface. Consequently, membrane integrity is lost, producing a detergent-like effect, which eventually disintegrates the membrane by forming micelles, followed by bacterial cell death (Qiu et al., 2020; Zhou et al., 2023; Kumar et al., 2018).
Another crucial consideration lies in the potential for bacteria to develop resistance to antibiotics. As previously highlighted, different bacterial strains develop resistance to commonly used antibiotics. Resistance mechanisms are unique and depend on the antibiotic group and mechanism of action specifics. Still, overall mechanisms involve specific enzyme production, loss of targeted molecules, efflux pumps, mutation of the target site, increased cell permeability, etc. (Reygaert, 2018; Munita and Arias, 2016; Peterson and Kaur, 2018; Abushaheen et al., 2020). It has been conventionally assumed that this propensity for resistance is exclusive to antibiotics, and theoretically, bacteria cannot develop resistance to aNDBs. On the one hand, electrostatic attraction between aNDBs and bacterial outer structures seems inevitable. In addition, the aNDB mechanism of action is not explicitly targeted. Even after entering the inner environment, aNDBs could enter many metabolic pathways. Based on that, it is more likely that bacteria encounter challenges in impeding electrostatic interaction and developing resistance, given the biological expense associated with such a complex process.
Although thought to be improbable, alteration of bacterial membranes has been shown as a mechanism of resistance (Epand et al., 1858; Nawrocki et al., 2014). Such alterations include incorporating components with reduced anionic charge, which leads to the inability of peptides to aggregate on bacterial membranes and prevents them from entering the cell (Baltzer and Brown, 2011). For instance, studies have shown that Staphylococcus aureus modifies the anionic phospholipids in the cytoplasmic membrane with L-lysine, resulting in a reduction of the net negative charge of the bacterial membrane and leading to the repulsion and subsequent resistance to aNDBs (Peschel et al., 2001). Similarly, modification of Gram-negative bacteria’s lipopolysaccharides (LPS) is another bacterial mechanism contributing to resistance (Gunn, 2001; Guo et al., 1998). These modifications include incorporating fatty acids, thereby reducing the permeability of the outer membrane and increasing membrane structural stability (Peschel, 2002). Furthermore, bacteria can change the permeability of the cell wall, as is widely reported in the case of tetracyclines (Chmit et al., 2014); in addition, such non-specific structures as efflux pumps are also responsible for pumping out unfavorable molecules, and they are evidenced to work correctly against macrolides (Zhong and Shortridge, 2000).
The highlighted findings in our review confirm that naturally occurring biopolymers with intrinsic antibacterial performance can be considered high-performance, sustainable, next-generation materials for biomedical field applications. Various studies have shown that hydrogels, biosensors, drug delivery systems, and implant coatings based on natural antibacterial biopolymers have promising physicochemical features. They possess excellent biocompatibility and are naturally derived, thus making them environmentally friendly. However, the main focus of this review was to elucidate the potential of naturally derived antibacterial biopolymers toward a specific aim—antibacterial therapy against bacterial infection in the human body—and second, to understand whether aNDBs are a future or a failure in replacing antibiotics. Multiple studies have reported these biopolymers in vitro; their antibacterial potential revealed action mechanisms. In addition, aNDBs have demonstrated substantial inhibitory effects against antibiotic-resistant bacteria strains. Based on the results, aNDBs could emerge as a novel weapon against bacterial infections to replace currently used antibiotics and antibiotic use approaches. Unique antibacterial action and the possibility of loading directly to the infection site (locally) open new horizons for this type of material.
However, learning from the past must be taken properly; many years ago, antibiotics were in the same situation. What is known for sure is that antibacterial biopolymers exhibit remarkable potential for combating bacteria and possess unique qualities. This material class is confined to research studies and is exclusively utilized for scientific purposes under controlled conditions. At the same time, antibiotics have already deserved the trust of medical doctors and have been proven effective antibacterial therapy in clinical care. Bacteria possess the biological mechanisms necessary for potential evolution, raising questions about the likelihood of encountering analogous issues. The problem associated with antibiotic resistance has emerged due to prolonged global exposure to antibiotics in the medical sector, inappropriate drug misuse or overuse, and the usage of antibiotics in agriculture. It is still being determined if antibacterial biopolymers will be opened to the world as much as antibiotics and undergo the same conditions. Will we face the same problem as now? Bacteria possess the biological mechanisms necessary for potential evolution, raising questions about the likelihood of encountering similar issues. In summary, antibacterial biopolymers are promising materials with many advantages, including their antibacterial potential. However, in light of various considerations and experiences, numerous questions must be answered, particularly considering the development of bacterial resistance. It is not merely a matter of substituting one for the other but a nuanced exploration of the complexities involved.
AS: Conceptualization, Resources, Writing – original draft, Writing – review & editing. IS: Writing – review & editing. MC: Writing – review & editing. JK: Supervision, Writing – review & editing. KS-A: Conceptualization, Funding acquisition, Supervision, Writing – review & editing.
The author(s) declare that financial support was received for the research, authorship, and/or publication of this article. The authors acknowledge financial support for granting Open Access from the European Union’s Horizon 2020 research and innovation program under grant agreement No. 857287 (BBCE—Baltic Biomaterials Centre of Excellence) and from the C316 Implementation of Consolidation and Governance Changes at Riga Technical University, LiepU, RAT, LMA, and LMC to Advance Excellence in Higher Education, Science, and Innovation program under the grant agreement C4835.Dok.1025 No. 5.2.1.1.i.0/2/24/I/CFLA/003.
The authors declare that the research was conducted in the absence of any commercial or financial relationships that could be construed as a potential conflict of interest.
The authors declare that no Generative AI was used in the creation of this manuscript.
All claims expressed in this article are solely those of the authors and do not necessarily represent those of their affiliated organizations, or those of the publisher, the editors and the reviewers. Any product that may be evaluated in this article, or claim that may be made by its manufacturer, is not guaranteed or endorsed by the publisher.
Abushaheen, M. A., Muzaheed, A. J., Fatani, M., Alosaimi, W., Mansy, M., George, S., et al. (2020). Antimicrobial resistance, mechanisms and its clinical significance. Dis. Mon. 66:100971. doi: 10.1016/J.DISAMONTH.2020.100971
Akbari, A., Bigham, A., Rahimkhoei, V., Sharifi, S., and Jabbari, E. (2022). Antiviral polymers: a review. Polym. 2022:1634. doi: 10.3390/POLYM14091634
Akram, F., Imtiaz, M., and ul Haq, I. (2023). Emergent crisis of antibiotic resistance: a silent pandemic threat to 21st century. Microb. Pathog. 174:105923. doi: 10.1016/J.MICPATH.2022.105923
Aminov, R. I. (2010). A brief history of the antibiotic era: lessons learned and challenges for the future. Front. Microbiol. 1:8040. doi: 10.3389/FMICB.2010.00134
Annunziato, G. (2019). Strategies to overcome antimicrobial resistance (AMR) making use of non-essential target inhibitors: a review. Int. J. Mol. Sci. 20:5844. doi: 10.3390/IJMS20235844
Antibiotics Review, (2010). Available at: https://errolozdalga.com/medicine/pages/OtherPages/AntibioticReview.ChanuRhee.html (Accessed April 2, 2024).
Appanna, V. D. (2018). Human microbes-the Power within, vol. 2018. 1st Edn: Singapore: Springer Singapore, Springer Nature Singapore Pte Ltd.
Arciola, C. R., Campoccia, D., and Montanaro, L. (2018). Implant infections: adhesion, biofilm formation and immune evasion. Nat. Rev. Microbiol. 2018, 397–409. doi: 10.1038/s41579-018-0019-y
Arer, V., and Kar, D. (2023). Biochemical exploration of β-lactamase inhibitors. Front. Genet. 13:1060736. doi: 10.3389/FGENE.2022.1060736
Asadpoor, M., Ithakisiou, G. N., van Putten, J. P. M., Pieters, R. J., Folkerts, G., and Braber, S. (2021). Antimicrobial activities of alginate and chitosan oligosaccharides against Staphylococcus aureus and group B Streptococcus. Front. Microbiol. 12:700605. doi: 10.3389/FMICB.2021.700605
Atanase, L. I. (2021). Micellar drug delivery systems based on natural biopolymers. Polym. 2021:477. doi: 10.3390/POLYM13030477
Baldwin, A., and Booth, B. W. (2022). Biomedical applications of tannic acid. J. Biomater. Appl. 36, 1503–1523. doi: 10.1177/08853282211058099
Baltimore, R. S., Cross, A. S., and Dobek, A. S. (1987). The inhibitory effect of sodium alginate on antibiotic activity against mucoid and non-mucoid strains of Pseudomonas aeruginosa. J. Antimicrob. Chemother. 20, 815–823. doi: 10.1093/JAC/20.6.815
Baltzer, S. A., and Brown, M. H. (2011). Antimicrobial peptides: promising alternatives to conventional antibiotics. J. Mol. Microbiol. Biotechnol. 20, 228–235. doi: 10.1159/000331009
Baranwal, J., Barse, B., Fais, A., Delogu, G. L., and Kumar, A. (2022). Biopolymer: a sustainable material for food and medical applications. Polym. 14:983. doi: 10.3390/POLYM14050983
Benhabiles, M. S., Salah, R., Lounici, H., Drouiche, N., Goosen, M. F. A., and Mameri, N. (2012). Antibacterial activity of chitin, chitosan and its oligomers prepared from shrimp shell waste. Food Hydrocoll. 29, 48–56. doi: 10.1016/J.FOODHYD.2012.02.013
Berry, M., Gurung, A., and Easty, D. L. (1995). Toxicity of antibiotics and antifungals on cultured human corneal cells: effect of mixing, exposure and concentration. Eye 9, 110–115. doi: 10.1038/eye.1995.17
Bhattacharjee, M. K. (2016). Antibiotics that inhibit nucleic acid synthesis. Chem. Antibiot. Relat. Drugs, 109–128. doi: 10.1007/978-3-319-40746-3_5
Bianculli, R. H., Mase, J. D., and Schulz, M. D. (2020). Antiviral polymers: past approaches and future possibilities. Macromolecules 53, 9158–9186. doi: 10.1021/ACS.MACROMOL.0C01273
Biswas, M. C., Jony, B., Nandy, P. K., Chowdhury, R. A., Halder, S., Kumar, D., et al. (2022). Recent advancement of biopolymers and their potential biomedical applications. J. Polym. Environ. 30, 51–74. doi: 10.1007/s10924-021-02199-y
Blaser, M. (2011). Stop the killing of beneficial bacteria. Nature. 476, 393–394. doi: 10.1038/476393a
Bogitsh, B. J., Carter, C. E., and Oeltmann, T. N. (2019). Symbiosis and parasitism. Hum. Parasitol., 1–14. doi: 10.1016/B978-0-12-813712-3.00001-1
Brogden, K. A. (2005). Antimicrobial peptides: pore formers or metabolic inhibitors in bacteria? Nat. Rev. Microbiol. 3, 238–250. doi: 10.1038/nrmicro1098
Bull, M. J., and Plummer, N. T. (2014). Part 1: the human gut microbiome in health and disease. Integr. Med. 13, 17–22.
Buonavoglia, A., Leone, P., Solimando, A. G., Fasano, R., Malerba, E., Prete, M., et al. (2021). Antibiotics or no antibiotics, That is the question: An update on efficient and effective use of antibiotics in dental practice, vol. 10. Basel, Switzerland: Antibiot.
Bush, K., and Bradford, P. A. (2016). β-Lactams and β-lactamase inhibitors: an overview. Cold Spring Harb. Perspect. Med. 6:5247. doi: 10.1101/CSHPERSPECT.A025247
Bustamante-Torres, M., Arcentales-Vera, B., Estrella-Nuñez, J., Yánez-Vega, H., and Bucio, E. (2022). Antimicrobial activity of composites-based on biopolymers. Macromolecules 2022, 258–283. doi: 10.3390/MACROMOL2030018
Castle, S. S. (2007). Beta-lactamase inhibitors. In: xPharm: The Comprehensive Pharmacology Reference, eds. S. J. Enna and B. David. (Netherlands: Bylund, Elsevier publisher) pp. 1–3.
Cell Membrane Inhibitors. Cell Membrane Inhibitors: Examples, Inhibition, Resistance, (2023). Available at: https://microbenotes.com/cell-membrane-inhibitors/ (Accessed December 18, 2024).
Chamidah, A., and Hardoko, A. A. P. (2017). Antibacterial activities of β-glucan (laminaran) against gram-negative and gram-positive bacteria. AIP Conf. Proc. 1844:20011. doi: 10.1063/1.4983422/748336
Chmit, M., Kanaan, H., Habib, J., Abbass, M., Mcheik, A., and Chokr, A. (2014). Antibacterial and antibiofilm activities of polysaccharides, essential oil, and fatty oil extracted from Laurus nobilis growing in Lebanon, Asian Pac. J. Trop. Med. 7S1, S546–S552. doi: 10.1016/S1995-7645(14)60288-1
Chortkoff, B., and Stenehjem, D. (2019). Chemotherapy, immunosuppression, and anesthesia. In: Pharmacology and Physiology for Anesthesia, (Second Edition), eds. H. C. Hemmings Jr and T. D. Egan. (Netherlands: Elsevier publisher) pp. 753–768.
Coates, A. R., Halls, G., and Hu, Y. (2011). Novel classes of antibiotics or more of the same? Br. J. Pharmacol. 163, 184–194. doi: 10.1111/J.1476-5381.2011.01250.X
Collin, F., Karkare, S., and Maxwell, A. (2011). Exploiting bacterial DNA gyrase as a drug target: current state and perspectives. Appl. Microbiol. Biotechnol. 92, 479–497. doi: 10.1007/S00253-011-3557-Z
Coma, V. (2013). Polysaccharide-based biomaterials with antimicrobial and antioxidant properties. Polimeros 20, 287–297. doi: 10.4322/POLIMEROS020OV002
Cook, M. A., and Wright, G. D. (2022). The past, present, and future of antibiotics. Sci. Transl. Med. 14:7793. doi: 10.1126/SCITRANSLMED.ABO7793
Cunha, B. A. (2006). Oral antibiotic therapy of serious systemic infections. Med. Clin. North Am. 90, 1197–1222. doi: 10.1016/J.MCNA.2006.07.009
Dahal, R. H., and Chaudhary, D. K. (2018). Microbial infections and antimicrobial resistance in Nepal: current trends and recommendations. Open Microbiol. J. 12, 230–242. doi: 10.2174/1874285801812010230
Daoud, Z., Sura, M., Abdel-Massih, R. M., Daoud, Z., Sura, M., and Abdel-Massih, R. M. (2013). Pectin shows antibacterial activity against Helicobacter pylori. Adv. Biosci. Biotechnol. 4, 273–277. doi: 10.4236/ABB.2013.42A037
Dimri, R., Mall, S., Sinha, S., Joshi, N. C., Bhatnagar, P., Sharma, R., et al. (2023). Role of microalgae as a sustainable alternative of biopolymers and its application in industries. Plant Sci. Today 10, 8–18. doi: 10.14719/PST.2460
Ding, R.-X., Goh, W. R., Wu, R.-N., Yue, X.-Q., Luo, X., Khine, W. W. T., et al. (2019). Revisit gut microbiota and its impact on human health and disease. J. Food Drug Anal. 27, 623–631. doi: 10.1016/J.JFDA.2018.12.012
Ding, Q., Mo, Z., Wang, X., Chen, M., Zhou, F., Liu, Z., et al. (2024). The antibacterial and hemostatic curdlan hydrogel–loading epigallocatechin gallate for facilitating the infected wound healing. Int. J. Biol. Macromol. 266:131257. doi: 10.1016/J.IJBIOMAC.2024.131257
Dong, Q., Guo, X., Li, L., Yu, C., Nie, L., Tian, W., et al. (2020). Understanding hyaluronic acid induced variation of water structure by near-infrared spectroscopy. Sci. Rep. 10, 1–8. doi: 10.1038/s41598-020-58417-5
Dönmez, İ. E., and Önem, E. (2024). Chemical composition and in vitro antibacterial activity of bark extractives and suberin monomers from Pinus brutia and Pinus nigra. Eur. J. Wood Wood Prod. 82, 231–240. doi: 10.1007/S00107-023-02004-8
Dubourg, G., Lagier, J. C., Robert, C., Armougom, F., Hugon, P., Metidji, S., et al. (2014). Culturomics and pyrosequencing evidence of the reduction in gut microbiota diversity in patients with broad-spectrum antibiotics. Int. J. Antimicrob. Agents 44, 117–124. doi: 10.1016/J.IJANTIMICAG.2014.04.020
Dunn, M. J., and Zambraski, E. J. (1980). Renal effects of drugs that inhibit prostaglandin synthesis. Kidney Int. 18, 609–622. doi: 10.1038/KI.1980.179
Egle, K., Dohle, E., Hoffmann, V., Salma, I., Al-Maawi, S., Ghanaati, S., et al. (2024). Fucoidan/chitosan hydrogels as carrier for sustained delivery of platelet-rich fibrin containing bioactive molecules. Int. J. Biol. Macromol. 262:129651. doi: 10.1016/J.IJBIOMAC.2024.129651
El-Fawal, G. (2014). Preparation, characterization and antibacterial activity of biodegradable films prepared from carrageenan. J. Food Sci. Technol. 51, 2234–2239. doi: 10.1007/S13197-013-1255-9
Eloe-Fadrosh, E. A., and Rasko, D. A. (2013). The human microbiome: from symbiosis to pathogenesis. Annu. Rev. Med. 64, 145–163. doi: 10.1146/ANNUREV-MED-010312-133513
Enenkel, S., and Stille, W. (1988). Administration of AntiBiotics. In: Antibiotics in the Tropics., ed. Springer Berlin. Heidelberg (Springer-Varlag, Germany), 39–40.
Epand, R. M., Walker, C., Epand, R. F., and Magarvey, N. A. (1858). Molecular mechanisms of membrane targeting antibiotics. Biochim. Biophys. Acta-Biomembr. 1858, 980–987. doi: 10.1016/J.BBAMEM.2015.10.018
Ersanli, C., Tzora, A., Skoufos, I., Voidarou, C., and Zeugolis, D. I. (2023). Recent advances in collagen antimicrobial biomaterials for tissue engineering applications: a review. Int. J. Mol. Sci. 24:7808. doi: 10.3390/IJMS24097808
Farha, A. K., Yang, Q. Q., Kim, G., Bin Li, H., Zhu, F., Liu, H. Y., et al. (2020). Tannins as an alternative to antibiotics. Food Biosci. 38:100751. doi: 10.1016/J.FBIO.2020.100751
Fayed, B., Jagal, J., Cagliani, R., Kedia, R. A., Elsherbeny, A., Bayraktutan, H., et al. (2023). Co-administration of amoxicillin-loaded chitosan nanoparticles and inulin: a novel strategy for mitigating antibiotic resistance and preserving microbiota balance in Helicobacter pylori treatment. Int. J. Biol. Macromol. 253:126706. doi: 10.1016/J.IJBIOMAC.2023.126706
Ghalei, S., and Handa, H. (2022). A review on antibacterial silk fibroin-based biomaterials: current state and prospects. Mater. Today Chem. 23:100673. doi: 10.1016/J.MTCHEM.2021.100673
Gómez, M. A., Bonilla, J. M., Coronel, M. A., Martínez, J., Morán-Trujillo, L., Orellana, S. L., et al. (2018). Antibacterial activity against Staphylococcus aureus of chitosan/chondroitin sulfate nanocomplex aerogels alone and enriched with erythromycin and elephant garlic (Allium ampeloprasum L. var. ampeloprasum) extract. Pure Appl. Chem. 90, 885–900. doi: 10.1515/pac-2016-1112
González-Restrepo, D., Zuluaga-Vélez, A., Orozco, L. M., and Sepúlveda-Arias, J. C. (2024). Silk fibroin-based dressings with antibacterial and anti-inflammatory properties. Eur. J. Pharm. Sci. 195:106710. doi: 10.1016/J.EJPS.2024.106710
Grill, M. F., and Maganti, R. K. (2011). Neurotoxic effects associated with antibiotic use: management considerations. Br. J. Clin. Pharmacol. 72, 381–393. doi: 10.1111/J.1365-2125.2011.03991.X
Gunn, J. S. (2001). Bacterial modification of LPS and resistance to antimicrobial peptides, sage jour. Innate Imunity 7, 57–62. doi: 10.1177/09680519010070011001
Guo, L., Lim, K. B., Poduje, C. M., Daniel, M., Gunn, J. S., Hackett, M., et al. (1998). Lipid a acylation and bacterial resistance against vertebrate antimicrobial peptides. Cell 95, 189–198. doi: 10.1016/S0092-8674(00)81750-X
Guo, F., Pan, F., Zhang, W., Liu, T., Zuber, F., Zhang, X., et al. (2022). Robust antibacterial activity of xanthan-gum-stabilized and patterned CeO2-x-TiO2Antifog films. ACS Appl. Mater. Interfaces 14, 44158–44172. doi: 10.1021/ACSAMI.2C11968
Habeeb, S. A., and Abdulkadhim, M. K. (2024). Natural biopolymer-hydrogel nanofibers for antibacterial applications. J. Eng. Mater. Technol. 146:3329. doi: 10.1115/1.4063329
Habibi, M., Golmakani, M. T., Eskandari, M. H., and Hosseini, S. M. H. (2024). Potential prebiotic and antibacterial activities of fucoidan from Laminaria japonica. Int. J. Biol. Macromol. 268:131776. doi: 10.1016/J.IJBIOMAC.2024.131776
Haktaniyan, M., and Bradley, M. (2022). Polymers showing intrinsic antimicrobial activity. Chem. Soc. Rev. 51, 8584–8611. doi: 10.1039/D2CS00558A
Hamidi, S., Monajjemzadeh, F., Siahi-Shadbad, M., Khatibi, S. A., and Farjami, A. (2023). Antibacterial activity of natural polymer gels and potential applications without synthetic antibiotics. Polym. Eng. Sci. 63, 5–21. doi: 10.1002/PEN.26184
Hanlon, J. T., Perera, S., Drinka, P. J., Crnich, C. J., Schweon, S. J., Klein-Fedyshin, M., et al. (2019). The IOU consensus recommendations for empirical therapy of cystitis in nursing home residents. J. Am. Geriatr. Soc. 67, 539–545. doi: 10.1111/JGS.15726
Hassan, E. A., Abou Elseoud, W. S., Abo-Elfadl, M. T., and Hassan, M. L. (2021). New pectin derivatives with antimicrobial and emulsification properties via complexation with metal-terpyridines. Carbohydr. Polym. 268:118230. doi: 10.1016/J.CARBPOL.2021.118230
Hedrick, T. L., Adams, J. D., and Sawyer, R. G. (2006). Implant-associated infections: an overview. J. Long-Term Eff. Med. Implants 16, 83–99. doi: 10.1615/jlongtermeffmedimplants.v16.i1.90
Hegde, V., Uthappa, U. T., Altalhi, T., Jung, H. Y., Han, S. S., and Kurkuri, M. D. (2022). Alginate based polymeric systems for drug delivery, antibacterial/microbial, and wound dressing applications. Mater. Today Commun. 33:104813. doi: 10.1016/J.MTCOMM.2022.104813
Hemarajata, P., and Versalovic, J. (2013). Effects of probiotics on gut microbiota: mechanisms of intestinal immunomodulation and neuromodulation. Ther. Adv. Gastroenterol. 6, 39–51. doi: 10.1177/1756283X12459294
Hernandez-Montelongo, J., Nicastro, G. G., Pereira, T. O., Zavarize, M., Beppu, M. M., Macedo, W. A. A., et al. (2021). Antibacterial effect of hyaluronan/chitosan nanofilm in the initial adhesion of Pseudomonas aeruginosa wild type, and IV pili and LPS mutant strains. Surfac. Interfac. 26:101415. doi: 10.1016/J.SURFIN.2021.101415
Hills, R. D., Pontefract, B. A., Mishcon, H. R., Black, C. A., Sutton, S. C., and Theberge, C. R. (2019). Gut microbiome: profound implications for diet and disease. Nutrients 2019:1613. doi: 10.3390/NU11071613
Homayun, B., Lin, X., and Choi, H. J. (2019). Challenges and recent Progress in Oral drug delivery Systems for Biopharmaceuticals. Pharm. 11:129. doi: 10.3390/PHARMACEUTICS11030129
Hou, X., Wang, H., Shi, Y., and Yue, Z. (2023). Recent advances of antibacterial starch-based materials. Carbohydr. Polym. 302:120392. doi: 10.1016/J.CARBPOL.2022.120392
Huan, Y., Kong, Q., Mou, H., and Yi, H. (2020). Antimicrobial peptides: classification, design, application and research Progress in multiple fields. Front. Microbiol. 11:582779. doi: 10.3389/FMICB.2020.582779
Hwang, J., Huang, H., Sullivan, M. O., and Kiick, K. L. (2023). Controlled delivery of vancomycin from collagen-tethered peptide vehicles for the treatment of wound infections. Mol. Pharm. 20, 1696–1708. doi: 10.1021/ACS.MOLPHARMACEUT.2C00898
Ibrahim, S., Riahi, O., Said, S. M., Sabri, M. F. M., and Rozali, S. (2019). Biopolymers from crop plants. Ref. Modul. Mater. Sci. Mater. Eng. doi: 10.1016/B978-0-12-803581-8.11573-5
Ige, O. O., Umoru, L. E., and Aribo, S. (2012). Natural products: a minefield of biomaterials. ISRN Mater. Sci. 2012, 1–20. doi: 10.5402/2012/983062
Ilić-Stojanović, S., Nikolić, L., and Cakić, S. (2023). A review of patents and innovative biopolymer-based hydrogels. Gels. 9:556. doi: 10.3390/gels9070556
Intorasoot, S., Intorasoot, A., Tawteamwong, A., Butr-Indr, B., Phunpae, P., Tharinjaroen, C. S., et al. (2022). In vitro antimycobacterial activity of human Lactoferrin-derived peptide, D-hLF 1-11, against susceptible and drug-resistant mycobacterium tuberculosis and its synergistic effect with rifampicin. Antibiot. 11:1785. doi: 10.3390/ANTIBIOTICS11121785
Isolauri, E., Sütas, Y., Kankaanpää, P., Arvilommi, H., and Salminen, S. (2001). Probiotics: effects on immunity. Am. J. Clin. Nutr. 73, 444s–450s. doi: 10.1093/AJCN/73.2.444S
Iuliano, S., Senn, L., Moi, L., Muller, Y. D., Ribi, C., Buss, G., et al. (2022). Management of beta-lactam antibiotics allergy: a real-life study. Front. Allergy 3:853587. doi: 10.3389/FALGY.2022.853587
Jacob, J., Haponiuk, J. T., Thomas, S., and Gopi, S. (2018). Biopolymer based nanomaterials in drug delivery systems: a review. Mater. Today Chem. 9, 43–55. doi: 10.1016/J.MTCHEM.2018.05.002
Jacobo-Delgado, Y. M., Rodríguez-Carlos, A., Serrano, C. J., and Rivas-Santiago, B. (2023). Mycobacterium tuberculosis cell-wall and antimicrobial peptides: a mission impossible? Front. Immunol. 14:1194923. doi: 10.3389/FIMMU.2023.1194923
Jarosz, K., Stadnik, D. A., Giannakoudakis, B., Barczyński, B., Barczak, M., and Kapusta, O. (2023). Antimicrobial natural hydrogels in biomedicine: properties, applications, and challenges—a concise review. Int. J. Mol. Sci. 24:2191. doi: 10.3390/IJMS24032191
Jenkins, A. D., Stepto, R. F. T., Kratochvíl, P., and Suter, U. W. (1996). Glossary of basic terms in polymer science (IUPAC recommendations 1996). Pure Appl. Chem. 68, 2287–2311. doi: 10.1351/PAC199668122287
Jenssen, H., and Hancock, R. E. W. (2009). Antimicrobial properties of lactoferrin. Biochimie 91, 19–29. doi: 10.1016/J.BIOCHI.2008.05.015
Johnston, C. W., and Badran, A. H. (2022). Natural and engineered precision antibiotics in the context of resistance. Curr. Opin. Chem. Biol. 69:102160. doi: 10.1016/J.CBPA.2022.102160
Kamaruzzaman, N. F., Tan, L. P., Hamdan, R. H., Choong, S. S., Wong, W. K., Gibson, A. J., et al. (2019). Antimicrobial polymers: the potential replacement of existing antibiotics? Int. J. Mol. Sci. 2019:2747. doi: 10.3390/IJMS20112747
Kapoor, G., Saigal, S., and Elongavan, A. (2017). Action and resistance mechanisms of antibiotics: a guide for clinicians. J. Anaesthesiol. Clin. Pharmacol. 33, 300–305. doi: 10.4103/JOACP.JOACP_349_15
Kaur, M., Cohen, Y., Poverenov, E., and Eltzov, E. (2022). Synergistic antimicrobial effect of the combination of beta-lactam antibiotics and chitosan derivative on multidrug-resistant bacteria. Int. J. Biol. Macromol. 223, 1107–1114. doi: 10.1016/J.IJBIOMAC.2022.11.132
Kaur, J., Rajkhowa, R., Afrin, T., Tsuzuki, T., and Wang, X. (2014). Facts and myths of antibacterial properties of silk. Biopolymers 101, 237–245. doi: 10.1002/BIP.22323
Khan, Y. A., Ozaltin, K., Bernal-Ballen, A., and Di Martino, A. (2021). Chitosan-alginate hydrogels for simultaneous and sustained releases of ciprofloxacin, amoxicillin and vancomycin for combination therapy. J. Drug Deliv. Sci. Technol. 61:102126. doi: 10.1016/J.JDDST.2020.102126
Khan, S., Tøndervik, A., Sletta, H., Klinkenberg, G., Emanuel, C., Onsøyen, E., et al. (2012). Overcoming drug resistance with alginate oligosaccharides able to potentiate the action of selected antibiotics. Antimicrob. Agents Chemother. 56, 5134–5141. doi: 10.1128/AAC.00525-12
Khara, J. S., Mojsoska, B., Mukherjee, D., Langford, P. R., Robertson, B. D., Jenssen, H., et al. (2020). Ultra-short antimicrobial Peptoids show propensity for membrane activity against multi-drug resistant Mycobacterium tuberculosis. Front. Microbiol. 11:515317. doi: 10.3389/FMICB.2020.00417
Khorshidian, N., Khanniri, E., Koushki, M. R., Sohrabvandi, S., and Yousefi, M. (2022). An overview of antimicrobial activity of lysozyme and its functionality in cheese. Front. Nutr. 9:833618. doi: 10.3389/FNUT.2022.833618
Kim, J., and De Jesus, O., Medication routes of administration, StatPearls (2023). Available at: https://www.ncbi.nlm.nih.gov/books/NBK568677/ (accessed April 2, 2024).
Kim, M. K., Kang, N., Ko, S. J., Park, J., Park, E., Shin, D. W., et al. (2018). Antibacterial and Antibiofilm activity and mode of action of Magainin 2 against drug-resistant Acinetobacter baumannii. Int. J. Mol. Sci. 19:3041. doi: 10.3390/IJMS19103041
Kong, B., Liu, R., Cheng, Y., Cai, X., Liu, J., Zhang, D., et al. (2023). Natural biopolymers derived hydrogels with injectable, self-healing, and tissue adhesive abilities for wound healing. Nano Res. 16, 2798–2807. doi: 10.1007/S12274-022-4936-8
Konstantinidis, T., Tsigalou, C., Karvelas, A., Stavropoulou, E., Voidarou, C., and Bezirtzoglou, E. (2020). Effects of antibiotics upon the gut microbiome: a review of the literature. Biomed. 8:502. doi: 10.3390/BIOMEDICINES8110502
Kucharska, M., Sikora, M., Brzoza-Malczewska, K., and Owczarek, M. (2019). Antimicrobial properties of chitin and chitosan, chitin chitosan prop. In: Chitin and Chitosan: Properties and Applications., eds. L. A. M. van den and C. G. Broek Boeriu. (United States of America: John Wiley & Sons Ltd) pp.169–187.
Kumar, P., Kizhakkedathu, J. N., and Straus, S. K. (2018). Antimicrobial peptides: diversity, mechanism of action and strategies to improve the activity and biocompatibility in vivo. Biomolecules 8:4. doi: 10.3390/BIOM8010004
Lathakumari, R. H., Vajravelu, L. K., Satheesan, A., Ravi, S., and Thulukanam, J. (2024). Antibiotics and the gut microbiome: understanding the impact on human health. Med. Microecol. 20:100106. doi: 10.1016/J.MEDMIC.2024.100106
Li, Q., Montalban-Lopez, M., and Kuipers, O. P. (2018). Increasing the antimicrobial activity of nisinbased lantibiotics against gram-negative pathogens. Appl. Environ. Microbiol. 84:e00052-18. doi: 10.1128/AEM.00052-18
Li, J., Tian, X., Hua, T., Fu, J., Koo, M., Chan, W., et al. (2021). Chitosan natural polymer material for improving antibacterial properties of textiles. ACS Appl. Bio Mater. 4, 4014–4038. doi: 10.1021/ACSABM.1C00078
Liakos, I. L., Menager, C., Guigo, N., Holban, A. M., Iordache, F., Pignatelli, F., et al. (2019). Suberin/trans-cinnamaldehyde oil nanoparticles with antimicrobial activity and anticancer properties when loaded with paclitaxel. ACS Appl. Bio Mater. 2, 3484–3497. doi: 10.1021/ACSABM.9B00408
Lin, D. M., Koskella, B., and Lin, H. C. (2017). Phage therapy: an alternative to antibiotics in the age of multi-drug resistance. World J. Gastrointest. Pharmacol. Ther. 8, 162–173. doi: 10.4292/WJGPT.V8.I3.162
Liu, Y., Li, X., and Liang, A. (2022). Current research progress of local drug delivery systems based on biodegradable polymers in treating chronic osteomyelitis. Front. Bioeng. Biotechnol. 10:1042128. doi: 10.3389/FBIOE.2022.1042128
Majiduddin, F. K., Materon, I. C., and Palzkill, T. G. (2002). Molecular analysis of beta-lactamase structure and function. Int. J. Med. Microbiol. 292, 127–137. doi: 10.1078/1438-4221-00198
Manoukian, O. S., Sardashti, N., Stedman, T., Gailiunas, K., Ojha, A., Penalosa, A., et al. (2019). Biomaterials for tissue engineering and regenerative medicine, Encycl. Biomed. Eng. 1–3, 462–482. doi: 10.1016/B978-0-12-801238-3.64098-9
Martín, R., Miquel, S., Ulmer, J., Kechaou, N., Langella, P., and Bermúdez-Humarán, L. G. (2013). Role of commensal and probiotic bacteria in human health: a focus on inflammatory bowel disease. Microb. Cell Factories 12:71. doi: 10.1186/1475-2859-12-71
McCarthy, K., and Avent, M. (2020). Oral or intravenous antibiotics? Aust. Prescr. 43, 45–48. doi: 10.18773/AUSTPRESCR.2020.008
McGee, M., Brienesse, S., Chong, B., Levendel, A., and Lai, K. (2018). Tropheryma whipplei endocarditis: case presentation and review of the literature. Open Forum Infect. Dis. 6:330. doi: 10.1093/OFID/OFY330
Melander, R. J., Basak, A. K., and Melander, C. (2020). Natural products as inspiration for the development of bacterial antibiofilm agents. Nat. Prod. Rep. 37, 1454–1477. doi: 10.1039/D0NP00022A
Meng, G., He, J., Wu, Y., Wu, F., and Gu, Z. (2014). Antibiotic-loaded chitosan hydrogel with superior dual functions: antibacterial efficacy and osteoblastic cell responses. ACS Appl. Mater. Interfaces 6, 10005–10013. doi: 10.1021/AM502537K
Mihajlovic, M., and Lazaridis, T. (2010). Antimicrobial peptides in toroidal and cylindrical pores. Biochim. Biophys. Acta 1798, 1485–1493. doi: 10.1016/J.BBAMEM.2010.04.004
Mishra, R., Panda, A. K., De Mandal, S., Shakeel, M., Bisht, S. S., and Khan, J. (2020). Natural anti-biofilm agents: strategies to control biofilm-forming pathogens. Front. Microbiol. 11:566325. doi: 10.3389/FMICB.2020.566325
Moradali, M. F., and Rehm, B. H. A. (2020). Bacterial biopolymers: from pathogenesis to advanced materials. Nat. Rev. Microbiol. 18, 195–210. doi: 10.1038/s41579-019-0313-3
Moraschini, V., Miron, R. J., Mourão, C. F. A. B., Louro, R. S., Sculean, A., Fonseca, L. A. M., et al. (2024). Antimicrobial effect of platelet-rich fibrin: a systematic review of in vitro evidence-based studies. Periodontol. 94, 131–142. doi: 10.1111/PRD.12529
Morgan, D. J., Okeke, I. N., Laxminarayan, R., Perencevich, E. N., and Weisenberg, S. (2011). Non-prescription antimicrobial use worldwide: a systematic review. Lancet Infect. Dis. 11, 692–701. doi: 10.1016/S1473-3099(11)70054-8
Munita, J. M., and Arias, C. A. (2016). Mechanisms of antibiotic resistance. Microbiol. Spectr. 4. doi: 10.1128/MICROBIOLSPEC.VMBF-0016-2015
Muñoz-Bonilla, A., Echeverria, C., Sonseca, Á., Arrieta, M. P., and Fernández-García, M. (2019). Bio-based polymers with antimicrobial properties towards sustainable development. Mater. 12:641. doi: 10.3390/MA12040641
Muñoz-Bonilla, A., and Fernández-García, M. (2012). Polymeric materials with antimicrobial activity. Prog. Polym. Sci. 37, 281–339. doi: 10.1016/j.progpolymsci.2011.08.005
Murali, V. P., and Jayakumar, R. (eds). (2023). Natural biopolymers in drug delivery—role, challenges and clinical applications. In: Natural Biopolymers in Drug Delivery and Tissue Engineering, (United Kingdom: Woodhead Publishing) pp. 3–23.
Nawrocki, K. L., Crispell, E. K., and McBride, S. M. (2014). Antimicrobial peptide resistance mechanisms of gram-positive Bacteria. Antibiot. 3, 461–492. doi: 10.3390/ANTIBIOTICS3040461
Nemeş, N. S., Ardean, C., Davidescu, C. M., Negrea, A., Ciopec, M., Duţeanu, N., et al. (2022). Antimicrobial activity of cellulose based materials. Polym. 14:735. doi: 10.3390/POLYM14040735
Nilsson, A. S. (2019). Pharmacological limitations of phage therapy. Ups. J. Med. Sci. 124, 218–227. doi: 10.1080/03009734.2019.1688433
Ntow-Boahene, W., Cook, D., and Good, L. (2021). Antifungal polymeric materials and nanocomposites. Front. Bioeng. Biotechnol. 9:780328. doi: 10.3389/FBIOE.2021.780328
Oliva, A., Miele, M. C., Al Ismail, D., Di Timoteo, F., De Angelis, M., Rosa, L., et al. (2021). Challenges in the microbiological diagnosis of implant-associated infections: a summary of the current knowledge. Front. Microbiol. 12:750460. doi: 10.3389/FMICB.2021.750460
Pahlevanzadeh, F., Setayeshmehr, M., Bakhsheshi-Rad, H. R., Emadi, R., Kharaziha, M., Poursamar, S. A., et al. (2022). A review on antibacterial biomaterials in biomedical applications: from materials perspective to bioinks design. Polym. 2022:2238. doi: 10.3390/POLYM14112238
Pancu, D. F., Scurtu, A., Macasoi, I. G., Marti, D., Mioc, M., Soica, C., et al. (2021). Antibiotics: conventional therapy and natural compounds with antibacterial activity—a Pharmaco-toxicological screening. Antibiotics 10:401. doi: 10.3390/ANTIBIOTICS10040401
Pastore, A., Raimondi, F., Rajendran, L., and Temussi, P. A. (2020). Why does the Aβ peptide of Alzheimer share structural similarity with antimicrobial peptides? Commun. Biol. 3, 1–7. doi: 10.1038/s42003-020-0865-9
Patangia, D. V., Anthony Ryan, C., Dempsey, E., Paul Ross, R., and Stanton, C. (2022). Impact of antibiotics on the human microbiome and consequences for host health. Microbiology 11:e1260. doi: 10.1002/MBO3.1260
Peschel, A. (2002). How do bacteria resist human antimicrobial peptides? Trends Microbiol. 10, 179–186. doi: 10.1016/S0966-842X(02)02333-8
Peschel, A., Jack, R. W., Otto, M., Collins, L. V., Staubitz, P., Nicholson, G., et al. (2001). Staphylococcus aureus resistance to human defensins and evasion of neutrophil killing via the novel virulence factor MprF is based on modification of membrane lipids with l-lysine. J. Exp. Med. 193, 1067–1076. doi: 10.1084/JEM.193.9.1067
Peterson, E., and Kaur, P. (2018). Antibiotic resistance mechanisms in bacteria: relationships between resistance determinants of antibiotic producers, environmental bacteria, and clinical pathogens. Front. Microbiol. 9:426686. doi: 10.3389/FMICB.2018.02928
Pino, P., Pellegrino, G., Ronchetti, S., Mollea, C., Bosco, F., and Onida, B. (2023). Antibacterial β-glucan/zinc oxide nanocomposite films for wound healing. BioNanoScience 13, 426–435. doi: 10.1007/S12668-023-01079-0
Poznanski, P., Hameed, A., and Orczyk, W. (2023). Chitosan and chitosan nanoparticles: parameters enhancing antifungal activity. Molecules 28:2996. doi: 10.3390/MOLECULES28072996
Protein Synthesis Inhibitors-Definition. Protein Synthesis Inhibitors-Definition, Examples, Inhibition, Resistance, (2023). Available at: https://microbenotes.com/protein-synthesis-inhibitors/ (Accessed December 18, 2024).
Qiu, H., Si, Z., Luo, Y., Feng, P., Wu, X., Hou, W., et al. (2020). The mechanisms and the applications of antibacterial polymers in surface modification on medical devices. Front. Bioeng. Biotechnol. 8:571162. doi: 10.3389/FBIOE.2020.00910
Rai, M., Wypij, M., Ingle, A. P., Trzcińska-Wencel, J., and Golińska, P. (2021). Emerging trends in pullulan-based antimicrobial systems for various applications. Int. J. Mol. Sci. 22:13596. doi: 10.3390/IJMS222413596
Ramappa, V., and Aithal, G. P. (2013). Hepatotoxicity related to anti-tuberculosis drugs: mechanisms and management. J. Clin. Exp. Hepatol. 3, 37–49. doi: 10.1016/J.JCEH.2012.12.001
Ranjbar, H. H., Hosseini-Abari, A., Ghasemi, S. M., and Birgani, Z. H. (2023). Antibacterial activity of epsilon-poly-l-lysine produced by Stenotrophomonas maltophilia HS4 and Paenibacillus polymyxa HS5, alone and in combination with bacteriophages. Microbiol. 169:001363. doi: 10.1099/MIC.0.001363
Razak, N. S., and Mohamed, R. (2021). Antimicrobial sustainable biopolymers for biomedical plastics applications – an overview. Polimery 66, 574–583. doi: 10.14314/POLIMERY.2021.11.2
Reddy, M. S. B., Ponnamma, D., Choudhary, R., and Sadasivuni, K. K. (2021). A comparative review of natural and synthetic biopolymer composite scaffolds. Polym. 13:1105. doi: 10.3390/POLYM13071105
Ren, Z., Xiao, W., Song, H., and Zhou, L. (2024). Development of quantum computational model for analysis of drug delivery: loading of human beta-defensin 3 and mitoxantrone onto composite polymers. J. Mol. Liq. 399:124383. doi: 10.1016/J.MOLLIQ.2024.124383
ResistanceMap, (n.d.). https://resistancemap.onehealthtrust.org/Countries.php (Accessed December 18, 2024).
Reygaert, W. C. (2018). An overview of the antimicrobial resistance mechanisms of bacteria. AIMS Microbiol. 4, 482–501. doi: 10.3934/MICROBIOL.2018.3.482
Ridyard, K. E., and Overhage, J. (2021). The potential of human peptide LL-37 as an antimicrobial and anti-biofilm agent. Antibiot. 10:650. doi: 10.3390/ANTIBIOTICS10060650
Rinninella, E., Raoul, P., Cintoni, M., Franceschi, F., Miggiano, G. A. D., Gasbarrini, A., et al. (2019). What is the healthy gut microbiota composition? A changing ecosystem across age, environment, diet, and diseases. Microorg 7:14. doi: 10.3390/MICROORGANISMS7010014
Rofeal, M., Abdelmalek, F., and Steinbüchel, A. (2022). Naturally-sourced antibacterial polymeric nanomaterials with special reference to modified polymer variants. Int. J. Mol. Sci. 23:4101. doi: 10.3390/IJMS23084101
Romano, A., Mondino, C., Viola, M., and Montuschi, P. (2003). Immediate allergic reactions to β-lactams: diagnosis and therapy, Int J Immunopathol Pharmacol. 16, 19–23. doi: 10.1177/039463200301600103
Roy, S., Halder, M., Ramprasad, P., Dasgupta, S., Singh, Y., and Pal, D. (2023). Oxidized pullulan exhibits potent antibacterial activity against S. aureus by disrupting its membrane integrity. Int. J. Biol. Macromol. 249:126049. doi: 10.1016/J.IJBIOMAC.2023.126049
Sam, S., Joseph, B., and Thomas, S. (2023). Exploring the antimicrobial features of biomaterials for biomedical applications. Results Eng. 17:100979. doi: 10.1016/J.RINENG.2023.100979
Santos, M. R. E., Fonseca, A. C., Mendonça, P. V., Branco, R., Serra, A. C., Morais, P. V., et al. (2016). Recent developments in antimicrobial polymers: a review. Mater. 9:599. doi: 10.3390/MA9070599
Sceglovs, A., Wychowaniec, J. K., Skadins, I., Reinis, A., Edwards-Gayle, C. J. C., D’Este, M., et al. (2023). Effect of steam sterilisation on physico-chemical properties of antibacterial covalently cross-linked ε-polylysine/hyaluronic acid hydrogels. Carbohydr. Polym. Technol. Appl. 6:100363. doi: 10.1016/J.CARPTA.2023.100363
Schrade, S., Ritschl, L., Süss, R., Schilling, P., and Seidenstuecker, M. (2022). Gelatin nanoparticles for targeted dual drug release out of alginate-di-aldehyde-gelatin gels. Gels. 8:365. doi: 10.3390/gels8060365
Schwartz, B., and Vetvicka, V. (2021). Review: β-glucans as effective antibiotic alternatives in poultry. Mol. 26:3560. doi: 10.3390/MOLECULES26123560
Shai, Y. (2002). Mode of action of membrane active antimicrobial peptides. Pept. Sci. 66, 236–248. doi: 10.1002/BIP.10260
Shanmugasundaram, O. L., and Ramkumar, M. (2018). Characterization and study of physical properties and antibacterial activities of human hair keratin–silver nanoparticles and keratin–gold nanoparticles coated cotton gauze fabric. J. Ind. Text. 47, 798–814. doi: 10.1177/1528083716674904
Shin, J. M., Gwak, J. W., Kamarajan, P., Fenno, J. C., Rickard, A. H., and Kapila, Y. L. (2016). Biomedical applications of nisin. J. Appl. Microbiol. 120, 1449–1465. doi: 10.1111/JAM.13033
Si, Z., Hou, Z., Vikhe, Y. S., Thappeta, K. R. V., Marimuthu, K., De, P. P., et al. (2021). Antimicrobial effect of a novel chitosan derivative and its synergistic effect with antibiotics. ACS Appl. Mater. Interfaces 13, 3237–3245. doi: 10.1021/ACSAMI.0C20881
Sikdar, P., Uddin, M. M., Dip, T. M., Islam, S., Hoque, M. S., Dhar, A. K., et al. (2021). Recent advances in the synthesis of smart hydrogels. Mater. Adv. 2, 4532–4573. doi: 10.1039/D1MA00193K
Silvestro, L., Weiser, J. N., and Axelsen, P. H. (2000). Antibacterial and antimembrane activities of cecropin a in Escherichia coli. Antimicrob. Agents Chemother. 44, 602–607. doi: 10.1128/AAC.44.3.602-607.2000
Smola-Dmochowska, A., Lewicka, K., Macyk, A., Rychter, P., Pamuła, E., and Dobrzyński, P. (2023). Biodegradable polymers and polymer composites with antibacterial properties. Int. J. Mol. Sci. 24:7473. doi: 10.3390/IJMS24087473
Solensky, R. (2003). Hypersensitivity reactions to beta-lactam antibiotics. Clin. Rev. Allergy Immunol. 24, 201–220. doi: 10.1385/CRIAI:24:3:201
Song, J., Chen, H., Wei, Y., and Liu, J. (2020). Synthesis of carboxymethylated β-glucan from naked barley bran and its antibacterial activity and mechanism against Staphylococcus aureus. Carbohydr. Polym. 242:116418. doi: 10.1016/J.CARBPOL.2020.116418
Suflet, D. M., Popescu, I., Stanciu, M. C., and Rimbu, C. M. (2024). Antimicrobial hydrogels based on cationic Curdlan derivatives for biomedical applications. Gels 10:424. doi: 10.3390/gels10070424
Sun, Y., Bai, Y., Yang, W., Bu, K., Tanveer, S. K., and Hai, J. (2022). Global trends in natural biopolymers in the 21st century: a scientometric review. Front. Chem. 10:915648. doi: 10.3389/FCHEM.2022.915648
Sun, C., Liu, W., Wang, L., Meng, R., Deng, J., Qing, R., et al. (2023). Photopolymerized keratin-PGLa hydrogels for antibiotic resistance reversal and enhancement of infectious wound healing. Mater. Today Bio 23:100807. doi: 10.1016/J.MTBIO.2023.100807
Sundaran, S., Kok, L. C., and Chang, H. Y. (2022). Combination effect of epsilon-poly-L-lysine and antibiotics against common bacterial pathogens. J. Antibiot. 75, 354–359. doi: 10.1038/s41429-022-00523-9
Surveillance Atlas of Infectious Diseases. (2022). Available at: https://atlas.ecdc.europa.eu/public/index.aspx?Dataset=27&HealthTopic=4 (Accessed April 2, 2024).
Syaban, M. F. R., Erwan, N. E., Syamsuddin, M. R. R., Zahra, F. A., and Sabila, F. L. (2022). Insilico study and analysis antibacterial activity of Beta-glucan against Beta-lactamase and protein binding penicillin-2A. Res. J. Pharm. Technol. 15, 1948–1952. doi: 10.52711/0974-360X.2022.00324
Syryamina, V. N., Aisenbrey, C., Kardash, M., Dzuba, S. A., and Bechinger, B. (2024). Self-assembly of spin-labeled antimicrobial peptides magainin 2 and PGLa in lipid bilayers. Biophys. Chem. 310:107251. doi: 10.1016/J.BPC.2024.107251
Taheri-Araghi, S. (2024). Synergistic action of antimicrobial peptides and antibiotics: current understanding and future directions. Front. Microbiol. 15:1390765. doi: 10.3389/FMICB.2024.1390765
Tehrani, K. H. M. E., and Martin, N. I. (2018). β-Lactam/β-lactamase inhibitor combinations: an update. Medchemcomm 9, 1439–1456. doi: 10.1039/C8MD00342D
Tin, S., Sakharkar, K. R., Lim, C. S., and Sakharkar, M. K. (2009). Activity of Chitosans in combination with antibiotics in Pseudomonas aeruginosa. Int. J. Biol. Sci. 5, 153–160. doi: 10.7150/IJBS.5.153
Tripathi, S., and Mishra, S. (2021). Antioxidant, antibacterial analysis of pectin isolated from Banana Peel and its application in edible coating of freshly made mozzarella cheese. Asian Food Sci. J. 20, 82–92. doi: 10.9734/AFSJ/2021/V20I730324
Troy, E., Tilbury, M. A., Power, A. M., and Wall, J. G. (2021). Nature-based biomaterials and their application in biomedicine. Polym. 13:3321. doi: 10.3390/POLYM13193321
Uchil, R. R., Kohli, G. S., Katekhaye, V. M., and Swami, O. C. (2014). Strategies to combat antimicrobial resistance. J. Clin. Diagn. Res. 8, ME01–ME04. doi: 10.7860/JCDR/2014/8925.4529
Ullah, H., and Ali, S. (2017). Classification of anti-bacterial agents and their functions. Antibact. Agents. doi: 10.5772/INTECHOPEN.68695
Unver, T., Erenler, A. S., Bingul, M., and Boga, M. (2023). Comparative analysis of antioxidant, anticholinesterase, and antibacterial activity of microbial chondroitin sulfate and commercial chondroitin sulfate. Chem. Biodivers. 20:e202300924. doi: 10.1002/CBDV.202300924
Urban-Chmiel, R., Marek, A., Stępień-Pyśniak, D., Wieczorek, K., Dec, M., Nowaczek, A., et al. (2022). Antibiotic resistance in Bacteria—a review. Antibiotics 11:1079. doi: 10.3390/antibiotics11081079
Valenzuela-Rojo, R. D., López-Cervantes, J., Sánchez-Machado, D. I., Escárcega-Galaz, A. A., and Martínez-Macias, M. R. (2020). Antibacterial, mechanical and physical properties of collagen – chitosan sponges from aquatic source. Sustain. Chem. Pharm. 15:100218. doi: 10.1016/J.SCP.2020.100218
van den Heuvel, D. B., Stawski, T. M., Tobler, D. J., Wirth, R., Peacock, C. L., and Benning, L. G. (2018). Formation of silica-lysozyme composites through co-precipitation and adsorption. Front. Mater. 5:331384. doi: 10.3389/FMATS.2018.00019
van Seventer, J. M., and Hochberg, N. S. (2017). Principles of infectious diseases: transmission, diagnosis, prevention, and control. In: International Encyclopedia of Public Health. (Second Edition). ed. S. R. Quah. (Singapore: Duke-NUS Medical School) 22–39.
Veiga, A. S., and Schneider, J. P. (2013). Antimicrobial hydrogels for the treatment of infection. Biopolymers. 100, 637–644. doi: 10.1002/bip.22412
Vinarov, Z., Abdallah, M., Agundez, J. A. G., Allegaert, K., Basit, A. W., Braeckmans, M., et al. (2021). Impact of gastrointestinal tract variability on oral drug absorption and pharmacokinetics: an UNGAP review. Eur. J. Pharm. Sci. 162:105812. doi: 10.1016/J.EJPS.2021.105812
Waley, S. G. (1992). ß-lactamase: mechanism of action. In: The Chemistry of β-Lactams., ed. M. I. Page. (Netherlands: Springer).
Wang, K., Sun, H., Cui, Z., Wang, J., Hou, J., Lu, F., et al. (2024). Lactoferrin-chitosan composite hydrogels induced by microbial transglutaminase: potential delivery systems for thermosensitive bioactive substances. J. Agric. Food Chem. 72, 14302–14314. doi: 10.1021/ACS.JAFC.4C01551
Wang, Z., Yang, Q., Wang, X., Li, R., Qiao, H., Ma, P., et al. (2020). Antibacterial activity of xanthan-oligosaccharide against Staphylococcus aureus via targeting biofilm and cell membrane. Int. J. Biol. Macromol. 153, 539–544. doi: 10.1016/J.IJBIOMAC.2020.03.044
Wathoni, N., Suhandi, C., Purnama, M. F. G., Mutmainnah, A., Nurbaniyah, N. S., Syafra, D. W., et al. (2024). Alginate and chitosan-based hydrogel enhance antibacterial agent activity on topical application. Infect. Drug Resist. 17, 791–805. doi: 10.2147/IDR.S456403
Web of Science. Journal articles on antimicrobial natural bio-polymers. (n.d.). Available at: https://www.webofscience.com/wos/woscc/summary/6ed8dab8-5b3c-4a8f-a385-af59f647f6ff-ac09097b/relevance/1 (accessed April 4, 2024)
Wieërs, G., Belkhir, L., Enaud, R., Leclercq, S., Philippart de Foy, J. M., Dequenne, I., et al. (2020). How probiotics affect the microbiota. Front. Cell. Infect. Microbiol. 9:454. doi: 10.3389/FCIMB.2019.00454
Wimley, W. C. (2010). Describing the mechanism of antimicrobial peptide action with the interfacial activity model. ACS Chem. Biol. 5, 905–917. doi: 10.1021/CB1001558
World Health Organization. New report calls for urgent action to avert antimicrobial resistance crisis, (2019). https://www.who.int/news/item/29-04-2019-new-report-calls-for-urgent-action-to-avert-antimicrobial-resistance-crisis (Accessed April 2, 2024).
Wu, G., Ma, F., Xue, Y., Peng, Y., Hu, L., Kang, X., et al. (2022). Chondroitin sulfate zinc with antibacterial properties and anti-inflammatory effects for skin wound healing. Carbohydr. Polym. 278:118996. doi: 10.1016/J.CARBPOL.2021.118996
Wu, J., Shaidani, S., Theodossiou, S. K., Hartzell, E. J., and Kaplan, D. L. (2022). Localized, on-demand, sustained drug delivery from biopolymer-based materials. Expert Opin. Drug Deliv. 19, 1317–1335. doi: 10.1080/17425247.2022.2110582
Yadav, P., Yadav, H., Shah, V. G., Shah, G., and Dhaka, G. (2015). Biomedical biopolymers, their origin and evolution in biomedical sciences: a systematic review. J. Clin. Diagn. Res. 9, ZE21–ZE25. doi: 10.7860/JCDR/2015/13907.6565
Yan, R., Liu, M., Zeng, X., Du, Q., Wu, Z., Guo, Y., et al. (2024). Preparation of modified chitosan-based nano-TiO2–nisin composite packaging film and preservation mechanism applied to chilled pork. Int. J. Biol. Macromol. 269:131873. doi: 10.1016/J.IJBIOMAC.2024.131873
Yang, L., Bajinka, O., Jarju, P. O., Tan, Y., Taal, A. M., and Ozdemir, G. (2021). The varying effects of antibiotics on gut microbiota. AMB Express 11, 116–113. doi: 10.1186/S13568-021-01274-W
Yang, Q., Le, S., Zhu, T., and Wu, N. (2023). Regulations of phage therapy across the world. Front. Microbiol. 14:1250848. doi: 10.3389/FMICB.2023.1250848
Yang, S., Qiao, J., Zhang, M., Kwok, L. Y., Matijašić, B. B., Zhang, H., et al. (2024). Prevention and treatment of antibiotics-associated adverse effects through the use of probiotics: a review. J. Adv. Res. [in Press]. doi: 10.1016/J.JARE.2024.06.006
Yeaman, M. R., and Yount, N. Y. (2003). Mechanisms of antimicrobial peptide action and resistance. Pharmacol. Rev. 55, 27–55. doi: 10.1124/PR.55.1.2
Yin, R., Kuo, H. C., Hudlikar, R., Sargsyan, D., Li, S., Wang, L., et al. (2019). Gut microbiota, dietary phytochemicals, and benefits to human health. Curr. Pharmacol. Rep. 5, 332–344. doi: 10.1007/S40495-019-00196-3
Zamboni, F., Wong, C. K., and Collins, M. N. (2023). Hyaluronic acid association with bacterial, fungal and viral infections: can hyaluronic acid be used as an antimicrobial polymer for biomedical and pharmaceutical applications? Bioact. Mater. 19, 458–473. doi: 10.1016/J.BIOACTMAT.2022.04.023
Zhang, F., and Cheng, W. (2022). The mechanism of bacterial resistance and potential bacteriostatic strategies. Antibiot. 2022:1215. doi: 10.3390/ANTIBIOTICS11091215
Zhao, Y., Wang, X., Qi, R., and Yuan, H. (2023). Recent advances of natural-polymer-based hydrogels for wound antibacterial therapeutics. Polym. 15:3305. doi: 10.3390/POLYM15153305
Zhong, P., and Shortridge, V. D. (2000). The role of efflux in macrolide resistance. Drug Resist. Updat. 3, 325–329. doi: 10.1054/DRUP.2000.0175
Zhou, X., Liao, F., Ye, Z., Cheng, J., Zhu, J., Chen, X., et al. (2023). Discovery and engineering of a novel peptide, Temporin-WY2, from frog skin secretion, with enhanced in vitro and in vivo antimicrobial efficacy against multi-drug resistant bacteria. Sci Rep. 14:18769. doi: 10.21203/RS.3.RS-3705341/V1
Keywords: antibacterial naturally derived biopolymers, antibiotics, antibiotic resistance, bacterial infections, mechanism of action
Citation: Sceglovs A, Skadins I, Chitto M, Kroica J and Salma-Ancane K (2025) Failure or future? Exploring alternative antibacterials: a comparative analysis of antibiotics and naturally derived biopolymers. Front. Microbiol. 16:1526250. doi: 10.3389/fmicb.2025.1526250
Received: 11 November 2024; Accepted: 13 January 2025;
Published: 03 February 2025.
Edited by:
Sebastian Guenther, University of Greifswald, GermanyReviewed by:
Eugene A. Rogozhin, Institute of Bioorganic Chemistry (RAS), RussiaCopyright © 2025 Sceglovs, Skadins, Chitto, Kroica and Salma-Ancane. This is an open-access article distributed under the terms of the Creative Commons Attribution License (CC BY). The use, distribution or reproduction in other forums is permitted, provided the original author(s) and the copyright owner(s) are credited and that the original publication in this journal is cited, in accordance with accepted academic practice. No use, distribution or reproduction is permitted which does not comply with these terms.
*Correspondence: Kristine Salma-Ancane, a3Jpc3RpbmUuc2FsbWEtYW5jYW5lQHJ0dS5sdg==
Disclaimer: All claims expressed in this article are solely those of the authors and do not necessarily represent those of their affiliated organizations, or those of the publisher, the editors and the reviewers. Any product that may be evaluated in this article or claim that may be made by its manufacturer is not guaranteed or endorsed by the publisher.
Research integrity at Frontiers
Learn more about the work of our research integrity team to safeguard the quality of each article we publish.