- 1Université Clermont Auvergne, Inserm, INRAE, M2iSH, Université Clermont Auvergne, Clermont–Ferrand, France
- 2Unité de Glycobiologie Structurale et Fonctionnelle (UGSF), Université de Lille, Lille, France
- 3Plateforme d’Exploration du Métabolisme, Composante Protéomique (PFEMcp), Theix, France
- 4UR 0370 Qualité des Produits Animaux (QuaPA), INRAE, Theix, France
- 5Université Clermont Auvergne, INRAE, Microbiologie Environnement Digestif Santé (MEDiS), Clermont–Ferrand, France
The Escherichia coli surfaceome consists mainly of the large surface organelles expressed by the organism to navigate and interact with the surrounding environment. The current study focuses on type I fimbriae and flagella. These large polymeric surface organelles are composed of hundreds to thousands of subunits, with their large size often preventing them from being studied in their native form. Recent studies are accumulating which demonstrate the glycosylation of surface proteins or virulence factors in pathogens, including E. coli. Using biochemical and glycobiological techniques, including biotin-hydrazide labeling of glycans and chemical and glycosidase treatments, we demonstrate (i) the presence of a well-defined and chemically resistant FimA oligomer in several strains of pathogenic and non-pathogenic E. coli, (ii) the major subunit of type I fimbriae, FimA, in pathogenic and laboratory strains is recognized by concanavalin A, (iii) standard methods to remove N-glycans (PNGase F) or a broad-specificity mannosidase fail to remove the glycan structure, despite the treatments resulting in altered migration in SDS-PAGE, (iv) PNGase F treatment results in a novel 32 kDa band recognized by anti-FliC antiserum. While the exact identity of the glycan(s) and their site of attachment currently elude detection by conventional glycomics/glycoproteomics, the current findings highlight a potential additional layer of complexity of the surface (glyco) proteome of the commensal or adhesive and invasive E. coli strains studied.
Introduction
Escherichia coli (E. coli) uses a range of virulence factors to promote their adhesion, colonization, and invasion of human eukaryotic epithelial cells as well as immune cells (Avalos Vizcarra et al., 2016). To facilitate adhesion to sites, such as the small intestine or urethra, pathogenic E. coli use adhesins most commonly in the form of type I fimbriae (also referred to as pili), among others (Kaper et al., 2004). Type I fimbriae have been extensively studied during the last 70 years (McMichael and Ou, 1979; Hanson et al., 1988; Hanson and Brinton, 1988; Brinton, 1965).
They are composed of 500–3000 FimA subunits, and are generally thought to be terminated by FimF, FimG, and FimH, the latter allowing interaction with host and immune cell structures primarily via the mannose glycan (Hanson and Brinton, 1988; Krogfelt et al., 1990). Subunit assembly is facilitated by donor strand complementation, with an extension of each subunit non-covalently inserted into a pocket of the preceding subunit in the assembly. While FimF, FimG, and FimH subunits are easily dissociated from the polymer via SDS treatment, FimA resists SDS treatment, chaotropic salts including guanidium chloride and urea, and trypsin (McMichael and Ou, 1979). Importantly, the terminal localization of FimF, FimG, and FimH has been questioned, with limited studies giving evidence for the potential distribution of the minor subunits along the pili shaft, creating potential weak points. Abraham et al. (1983) provided evidence for this phenomenon using confocal microscopy and immunogold labeling, demonstrating the presence of FimH along the fimbrial shaft, while Ponniah et al. (1991) demonstrated that freeze–thaw-induced pilus fragmentation leads to the emergence of high-affinity mannose-binding groups, proposed to be the exposure of non-terminal FimH. FimA subunits are readily dissociated following mild acid hydrolysis (< pH 2, 99°C, 5 min), enhancing protein detection and, in specific cases, enabling the detection of reducing sugars (McMichael and Ou, 1979). However, as type I fimbriae are large biopolymers, their study is intrinsically complicated, and little progress has been made in studying their intact structure in recent years (Eshdat et al., 1981).
Despite their widespread presence and conserved sequence homology between pathogenic and non-pathogenic E. coli strains, both fimbriae and flagella may contribute to strain differentiation (Abraham et al., 1983; Palacios Pelaez et al., 2002; Duncan et al., 2005), further reinforcing the interest for vaccine development. For example, with respect to Crohn’s disease-associated strains, defined as adhesive and invasive E. coli (AIEC), point mutations in the type I pili adhesive subunit, FimH, can be used to further categorize strains based on their increased ability to adhere to an intestinal ligand carrying the cognate sugar (mannose) of the pili adhesin lectin (Dreux et al., 2013). Additionally, Duncan et al. (2005) have demonstrated that FimH-binding to mannosylated structures is modulated by the host origin of the FimA shaft12 in which expression of a Klebsiella FimH on a pili shaft of E. coli origin altered the binding selectivity comparable to that of an E. coli fimbriae, thus highlighting the important contribution of the fimbrial shaft and not only the terminal lectin.
Until recently, little attention has been given to the possibility of post-translational modification of the fimbrial subunits and its potential impact. Originally, a summary of research on type I fimbriae carried out between 1951 and 1965 by Brinton (1965) stated that chemical analysis of type I fimbriae revealed no detectable amounts of carbohydrate. More recently, however, two studies have highlighted the potential glycosylation of pili - McMichael and Ou (1979), and a patent granted in 2002 (Palacios Pelaez et al., 2002). Later, another study identified FimH as a candidate glycoprotein interacting with the GlcNAc-binding lectin, WGA (Xiu et al., 2012). Recent findings indicate that glycan modifications of surface proteins may be associated with altered motility as well as interactions between bacteria and with host epithelial and immune cells (Neuhaus et al., 2020; Benz and Schmidt, 2001; Logan et al., 2009; Grass et al., 2010).
Forty years have passed since McMichael and Ou demonstrated the detection of reducing sugars present on purified fimbriae only after acid hydrolysis, with their additional findings proposing the presence of O-glycans and potentially other uncharacterized glycans (McMichael and Ou, 1979). The identity of E. coli fimbriae-associated glycans has been hypothesized based on the work of Palacios Pelaez et al. (2002) using lectin profiling, albeit limited to uropathogenic E. coli strains. The latter work implies the presence of mannose, N-acetylglucosamine, and sialic acid, but has not been further reported.
Despite indications of glycan post-translational modifications of fimbriae, E. coli lack the well-defined and characterized glycosylation systems currently identified among Gram-negative organisms, including Campylobacter, Neisseria, and others (Schirm et al., 2003; Horzempa et al., 2008; Hartley et al., 2011; Hopf et al., 2011; Lu et al., 2015). However, recent studies show promise in identifying E. coli glycosylation enzymes, with those of the LPS biosynthesis pathway playing a dual role. In particular, Benz and Schmidt demonstrated the modification of the adhesin responsible for diffuse adhesion (AIDA-I) with an average of 19 heptose molecules obtained from the pathway of LPS synthesis by AAH (autotransporter adhesin heptosyltransferase) (Benz and Schmidt, 2001). More recently, Thorsing et al. (2021) characterized the heptosylation of the ETEC H10407 protein, YghJ, with the non-glycosylated version of the protein expressed in the E. coli K-12 MG1655 strain lacking the heptosyltransferase gene, hldE, further underlining the dual nature of the LPS synthesis pathways as a source of both enzymes and substrate for glycosylation of E. coli proteins.
This study examines the large surface organelles of several bacterial strains in both their native and depolymerized states. Given the complex and challenging nature of studying type I fimbriae, this study seeks to explore their potential for post-translational glycan modifications and, in doing so, has identified novel properties and features associated with type I fimbriae and flagella of E. coli. The main results of this study highlight the existence of a predominant FimA-containing pili unit of around 90 kDa, in several pathogenic (AIEC) and non-pathogenic strains (E. coli K-12), which is resistant to SDS-mediated dissociation, and confirm the presence of FimA-associated glycosylation, which is resistant to several chemical and enzymatic treatments.
Results
Surface protein purification and general characterization
The major surface proteins of the panel of E. coli strains were initially extracted by mechanical shearing, as previously described (Eshdat et al., 1981). However, to limit the impact of mechanical stress on fimbria and flagella polymers, extraction was performed as per Barnich et al. (2003), via thermal treatment, demonstrating no significant effects on yields of extracted surface proteins (not shown).
Total surface protein extracts of a panel of strains were profiled by Ponceau S (Figure 1A), Alcian blue staining (Figure 1B) and biotin-hydrazide labeling of glycans (Figure 1C). Ponceau S staining demonstrates the various profiles of extracted surface proteins, while Alcian blue staining demonstrates the presence of acidic polysaccharides in the extracted samples appearing as well-defined bands. This is in contrast to smearing which is associated with migration of capsular polysaccharides. Of note, Alcian blue staining resulted in negative staining of the non-stained proteins. The majority of adherent and invasive strains stained for Alcian blue at ~72 kDa, with AIEC CEA218U (Figure 1B, lane 12) demonstrating the most intense and variable staining of these AIEC strains. The two AIEC strains not staining at 72 kDa (POP90-M0, POP135-M0) also demonstrate a lack of staining at this size in the Ponceau S image. Additionally, POP18-M0 (Figure 1B, lane 8) stains at 36 kDa, and POP179-M0 (Figure 1B, lane 11) at 38 kDa. E. coli K-12 MG1655 and E. coli K-12 C600 both stain at ~55 kDa, while E. coli HS (Figure 1B, lane 4) stains at ~38 kDa, with Alcian blue staining generally coinciding with the strongest bands in the Ponceau S staining.
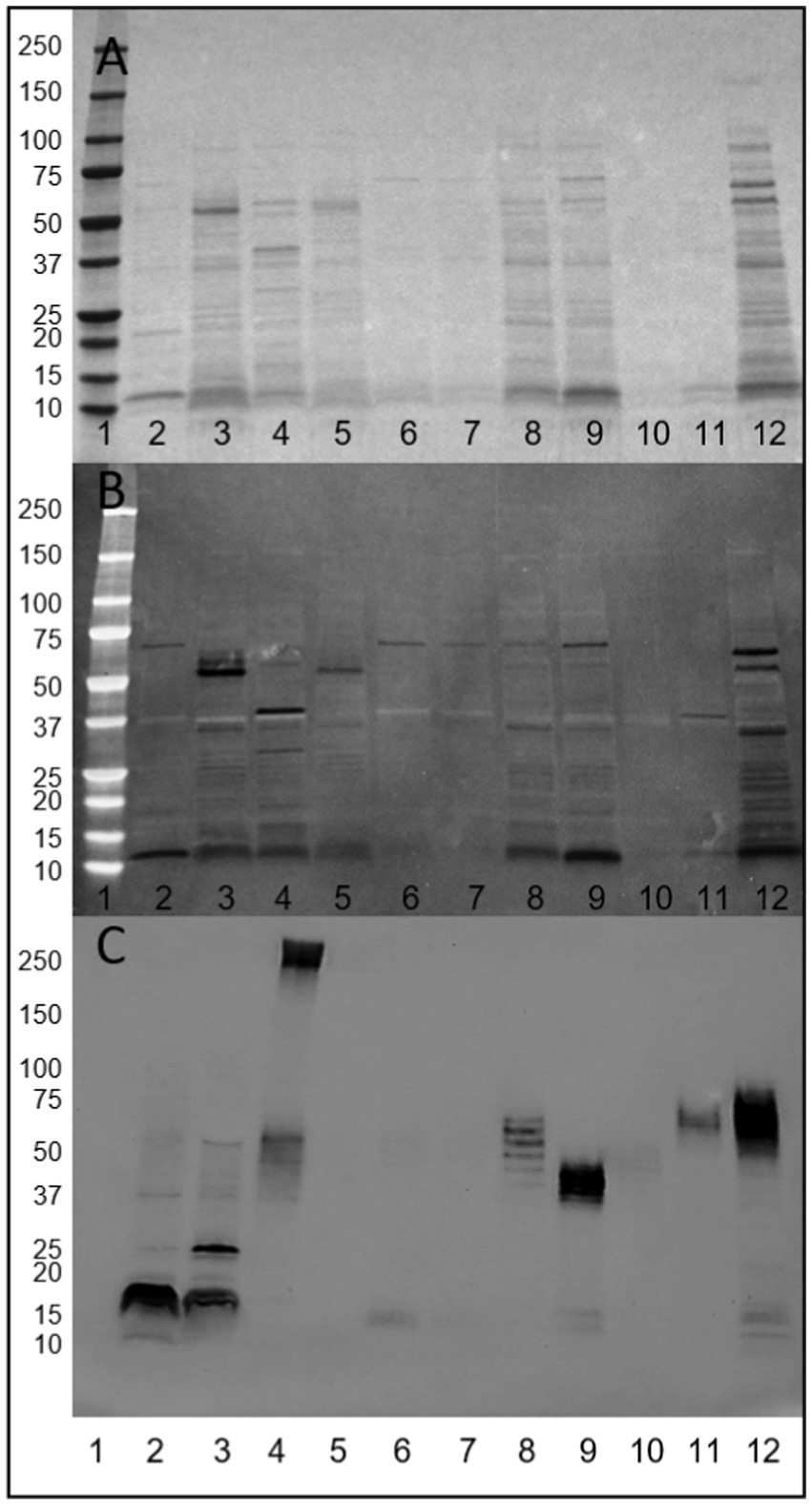
Figure 1. Visualization of released surface proteins of a panel of E. coli strains. (A) Ponceau S. (B) Alcian blue staining for acidic polysaccharides (dark bands). (C) Biotin-hydrazide labeling of glycans. Lanes 1. Molecular weight marker, 2. AIEC LF82, 3. E. coli K-12 MG1655, 4. E. coli HS, 5. E. coli K-12 C600, 6. AIEC LF82 ΔfimA, 7. AIEC LF82 ΔfimH, 8. POP18-M0, 9. POP90-M0, 10. POP135-M0, 11. POP179-M0, 12. CEA218U.
Identical samples were oxidized to create vicinal diols in any present glycans, and conjugated with biotin-hydrazide. Visualization with streptavidin-HRP demonstrates the presence of glycan structures present in the cell surface protein extracts of the majority of samples (results summarized in Table 1). Regarding AIEC LF82, glycans were detected at a molecular weight consistent with FimA monomers. Notably, glycans were absent in the E. coli K-12 C600 and the two AIEC LF82 isogenic mutant strains that do not express pili, suggesting an influence of the expression of surface pili on the presence of observed glycans in the released surface proteins. Interestingly, glycan-bearing structures were detected in E. coli K-12 MG1655 at ~25 kDa, and a high molecular weight structure (>250 kDa) present in the commensal strain, E. coli HS. The protocol employed for surface protein release is not likely sufficient to release LPS (not uniform across all samples), however, the current experiment did not exclude its presence. As such, in several samples there is a repeating pattern of bands, which may or may correspond to LPS, but will require further experiments to confirm.
To limit the potentially deleterious effects of trichloroacetic acid (TCA) on the released proteins and to aid in enrichment of type I fimbriae and flagella, as well as other present surface proteins (including OmpA and OmpC), released E. coli surface proteins were precipitated by 30% ammonium sulfate, collected, and resuspended in milli-Q water. The samples often remained cloudy and resistant to complete re-solubilization. In both, clarified and turbid samples, fimbria/FimA and FliC represent the primary proteins detected by Coomassie blue staining. However, removal of the insoluble material prior to Western blot analysis results in a significant decrease in detection of pili polymer fragments or acid-hydrolysis-released FimA subunits (Figure 2; lane 2) (non-clarified) versus lane 4 (centrifuged) as well as a reduction in galanthus nivalis (GNA) lectin staining. As the goal was to investigate the potential differences in polymer configurations or the presence of modifications, it was important to conserve the fimbrial fragments, avoiding removal of potentially important molecules by over-purification.
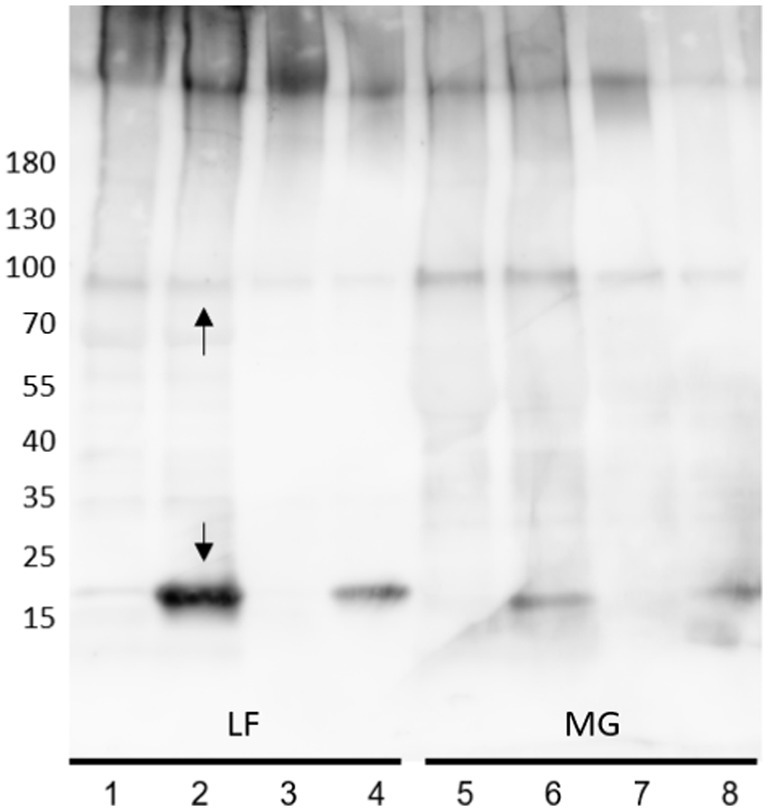
Figure 2. Anti-type I fimbria staining of released E. coli surface proteins. Lane 1, 2, 5, 6: Crude extracts; Lane 3, 4, 7, 8: Clarified supernatants. Samples non-acid-hydrolyzed: lanes 1, 3, 5, 7. Acid-hydrolyzed samples: lanes 2, 4, 6, 8. Upper arrow denotes the 90 kDa FimA oligomer, the lower arrow denotes the FimA subunit.
Narrowing the focus to type I fimbriae
Use of MgCl2 to further enrich the surface protein extracts for type I fimbria and flagella was carried out. Initially, the study of type I fimbria included analysis of intact fimbria employing typical SDS-PAGE conditions, and depolymerized FimA subunits by means of mild acid hydrolysis. Western blot of surface extracted proteins with anti-type I fimbria antiserum revealed the expected intense staining of FimA monomers at 18 kDa (Figure 2, lower arrow) for AIEC LF82 following mild acid hydrolysis. Interestingly, under non-hydrolyzed conditions, a distinct band of ~90 kDa stains for anti-type I fimbriae (Figure 2, upper arrow) indicating the presence of a conserved protein oligomer which is resistant to depolymerization by heat and SDS (Figures 2, 3– asterisk*). Accordingly, incubation with anti-FimF, anti-FimG, or anti-FimH did not stain the 90 kDa band (data not shown), confirming the expected absence of these fimbrial subunits in the high molecular weight polymers following preparation in Laemmli buffer containing SDS (as previously established by Jones et al., 1995). As such, the stained band likely represents a FimA homopentamer, corresponding to 5 × 18 kDa. This major band represents the minimal FimA oligomer unit to be resolved under SDS-PAGE conditions in these sample preparations, implying a minimum span of 5 FimA subunits separated by SDS/beta-mercaptoethanol-sensitive subunits. Given the absence of detection of a FimA subunit at 18 kDa, it is hypothesized that these stretches of FimA are likely separated by complexes of FimF, FimG, and FimH.
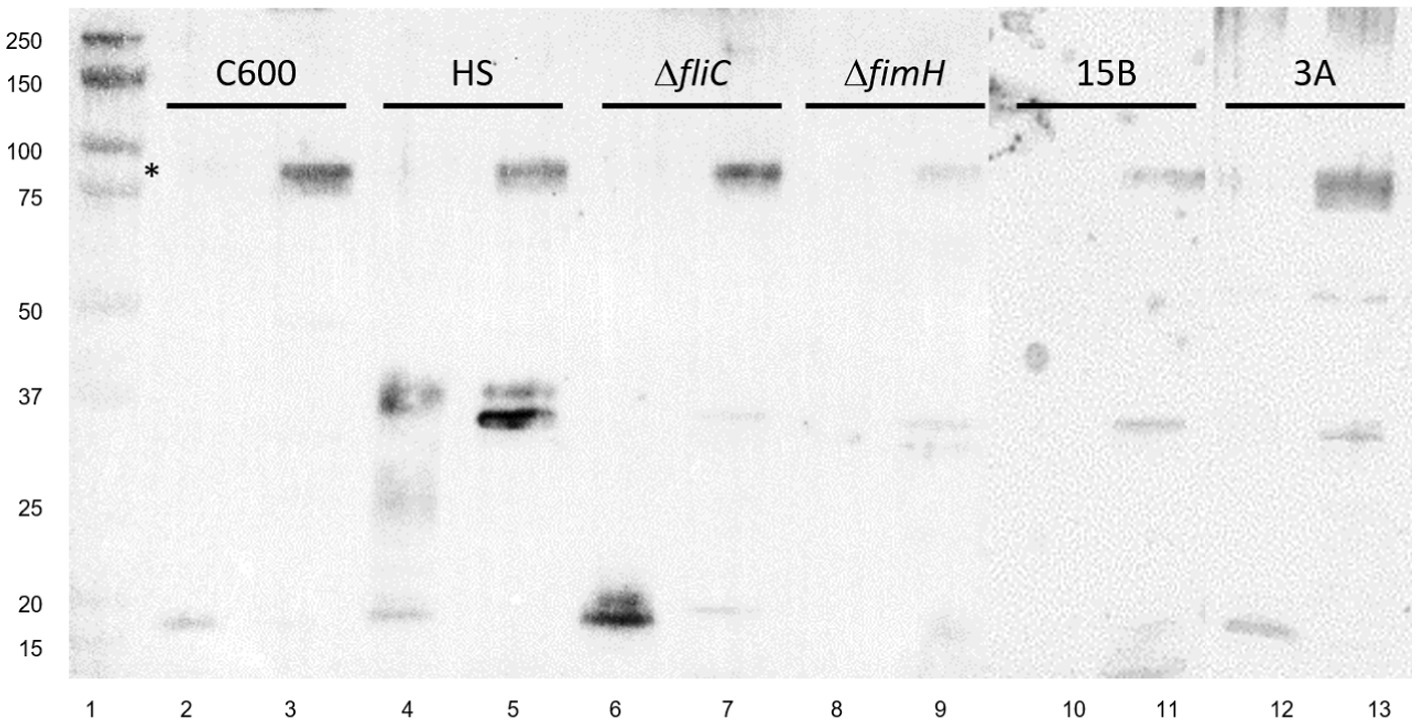
Figure 3. Anti-type I fimbria staining of panel of E. coli strains. Lane 1: Molecular weight ladder; lanes 2,3: E. coli K-12 C600; lanes 4,5: E. coli HS; lanes 6,7: LF82ΔfliC; lanes 8,9: LF82 ΔfimH; lanes 10,11: AIEC CEA614S (15B); lanes 12,13: AIEC CEA218U (3A). Splice of membranes treated identically. * FimA oligomer present under non-hydrolyzed conditions. Strains are shown in pairs as acid-hydrolyzed and non-hydrolyzed, respectively.
The 90 kDa band is present across all other strains tested from our collection following enrichment by MgCl2 treatment, including E. coli K-12 C600 and E. coli HS, and other strains of AIEC, including CEA218U and CEA614S (Figure 3), thus does not appear to be strain specific given the anti-type I fimbria serum used for staining.
Depolymerization of type I fimbria via chemical treatment
To facilitate purification and, later, interaction analysis of FimA monomers, two approaches were tested for the release of FimA subunits. First, reduction and alkylation were tested via incubation with dithiothreitol (DTT) and iodoacetamide (IAA), and secondly, incubation in saturated guanidine hydrochloride (GuHCl). These approaches were employed to avoid the potentially degradative effects of acid hydrolysis which is typically employed for FimA subunit release.
FimA possesses a cysteine bridge between residues 46–86. To open the tightly coiled fimbriae structure, reduction and alkylation of samples was carried out. As seen in Figure 4A, in both strains, AIEC LF82 and E. coli K-12 MG1655, the treatment enabled the detection of the FimA monomer (18 kDa and 15 kDa, respectively) by Coomassie blue staining, without the prior need of acid hydrolysis. In the case of GuHCl treatment of fimbria-enriched surface protein extracts, the release of FimA subunits is much more efficient than by reduction and alkylation alone, as determined by anti-type I fimbriae detection (Figure 4B). Additionally, reduction and alkylation results in the presence of FimA dimers, as revealed by Western blot. GuHCl treatment leaves the cysteine bridge intact, thus migrating further as a more compact structure, while reduction and alkylation breaks the disulfide fimbriae polymer. Treatment with beta-mercaptoethanol (β-ME) alone results in no change to FimA tetramers (~72 kDa), while FimA monomers are present, and due to the reduced disulfide bridge resulting in an opened structure, migrate at a larger perceived size (Figure 4B). To further probe the effects of reducing agents on the structural composition of FimA oligomers, we tested GuHCl-treated samples in the presence or absence of both reduction/alkylation and β-ME independently or together. Of note, the loading buffer contains SDS, which has been shown to release the FimF, FimG, and FimH subunits from the full-length fimbriae polymer. Treatment with β-ME alone results in no change to FimA tetramers (~72 kDa), while FimA monomers are present due to the initial GuHCl treatment (Figure 5, column 2: absence of β-ME and DTT/IAA versus column 3: presence of β-ME and absence of DTT/IAA). As mentioned above, reduction and alkylation results in FimA dimers and monomers with the disappearance of the FimA tetramers, implying the conversion of FimA tetramers to FimA dimers. Finally, addition of β-ME to reduced and alkylated samples results in only FimA monomers, disrupting the association between FimA dimers (Figure 5). Notably, addition of DTT to Laemmli buffer containing β-ME is not sufficient for complete dissociation (data not shown), indicating the requirement of either GuHCl treatment or alkylation, or both to achieve dissociation of tetramers and dimers.
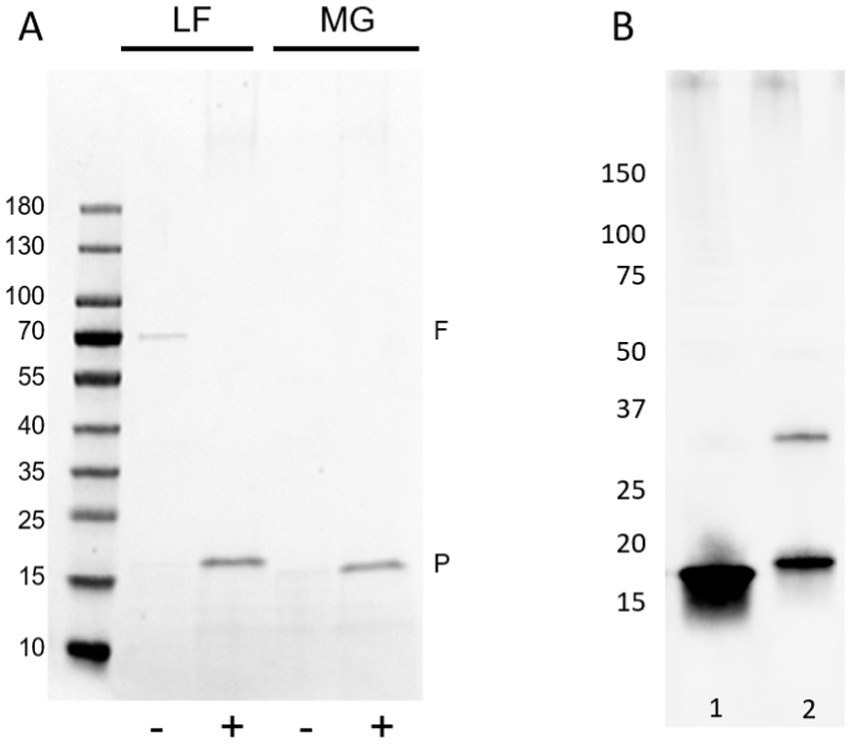
Figure 4. Influence of reduction and alkylation or guanidine hydrochloride treatment on FimA release and detection. (A) Coomassie blue staining of reduced and alkylated (+) or non-treated (−) fimbria-enriched surface protein extracts. Samples were not acid-hydrolyzed to aid the release and staining of FimA subunits. F = flagella (FliC), P = pili (fimbria; FimA), LF – AIEC LF82, MG – E. coli K-12 MG1655. (B) Guanidine HCl treatment versus reduction and alkylation of type I fimbria-enriched protein extracts from LF82. Type I fimbriae detection by anti-type I fimbria antiserum. Lane 1: GuHCl-treated, lane 2: reduced and alkylated.
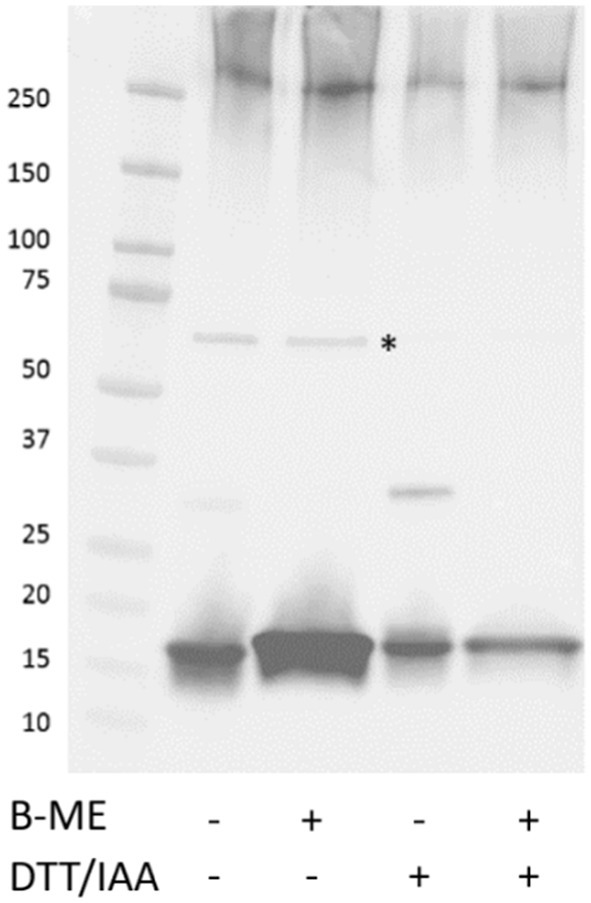
Figure 5. Effect of reducing agents on the depolymerization of FimA oligomers. GuHCl-treated LF82 surface extracted proteins in the absence or presence of varying reducing agents were resolved by SDS-PAGE followed by detection of type I fimbria. *Denotes FimA tetramer. B-ME, Beta-mercaptoethanol; DTT/IAA, reduction and alkylation.
Given the various steps involved in de-polymerization of type I fimbria and the eventual demonstration of the interaction between FimA and the lectin, concanavalin A (ConA), we propose a graphical summary of the evidence presented (Figure 6).
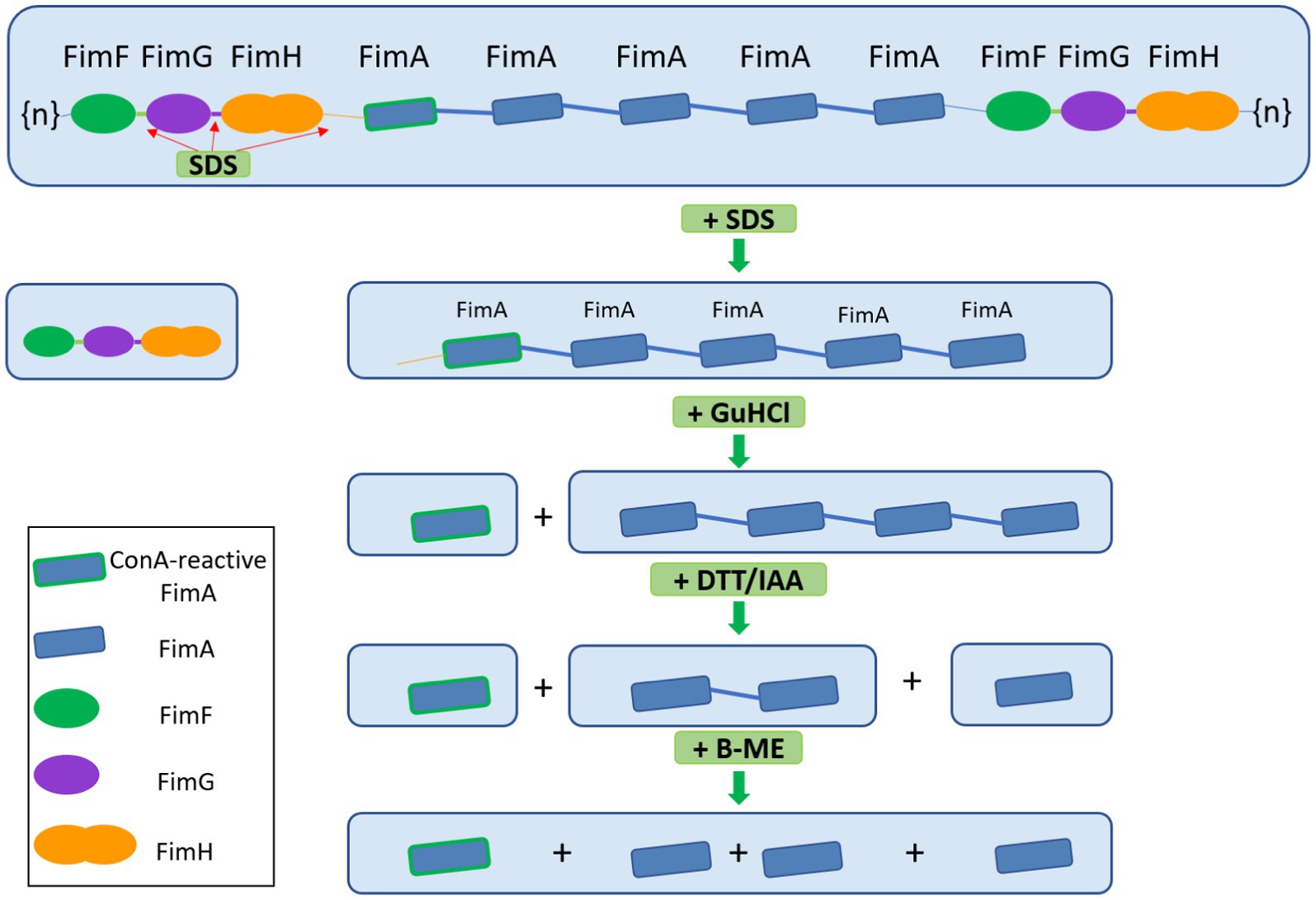
Figure 6. Graphical scheme of the depolymerization of type I fimbria following several stepwise chemical treatments. FimF, FimG, and FimH are removed by SDS and heat. GuHCl treatment further releases ConA-reactive FimA subunits. Reduction and alkylation (DTT/IAA) produces FimA dimers and monomers. Lastly, beta-mercaptoethanol dissociates the remaining FimA dimers into monomers. Beta-mercaptoethanol is able to release FimA subunits, however, the origin of these subunits does not appear to be the FimA tetramers, but likely the high molecular weight polymer migrating above 180 kDa.
Lectin staining
Concanavalin A (ConA)
E. coli surface protein extracts, either acid hydrolyzed (AH) to release FimA subunits or left untreated (NT) to analyze intact fimbriae, were stained with either ConA, GNA, or WGA. ConA strongly stains the released FimA subunits, but does not stain samples that were not acid-hydrolyzed (Figures 7A,C) implying the presence of a glycan embedded within the quaternary structure of the fimbriae. ConA and anti-type I fimbria staining overlap identically during the detection of released FimA subunits (Figures 7B,C). However, as ConA is reported as recognizing glucose, mannose, or L/D-glycero-manno-heptoses (Jaipuri et al., 2008), the identity of the attached glycan has not yet been confirmed. Sugar inhibition assays were carried out with glucose and mannose in an attempt to block ConA binding to FimA, however, this was unsuccessful, indicating the presence of an alternative glycan (results not shown). As further confirmation of the ConA staining of FimA subunits, lectin blotting of the wild-type AIEC LF82, along with isogenic mutants for the fimA and fliC genes was conducted, demonstrating an absence of ConA staining when the strain no longer expresses fimA, but not when fliC is deleted (Figure 8).
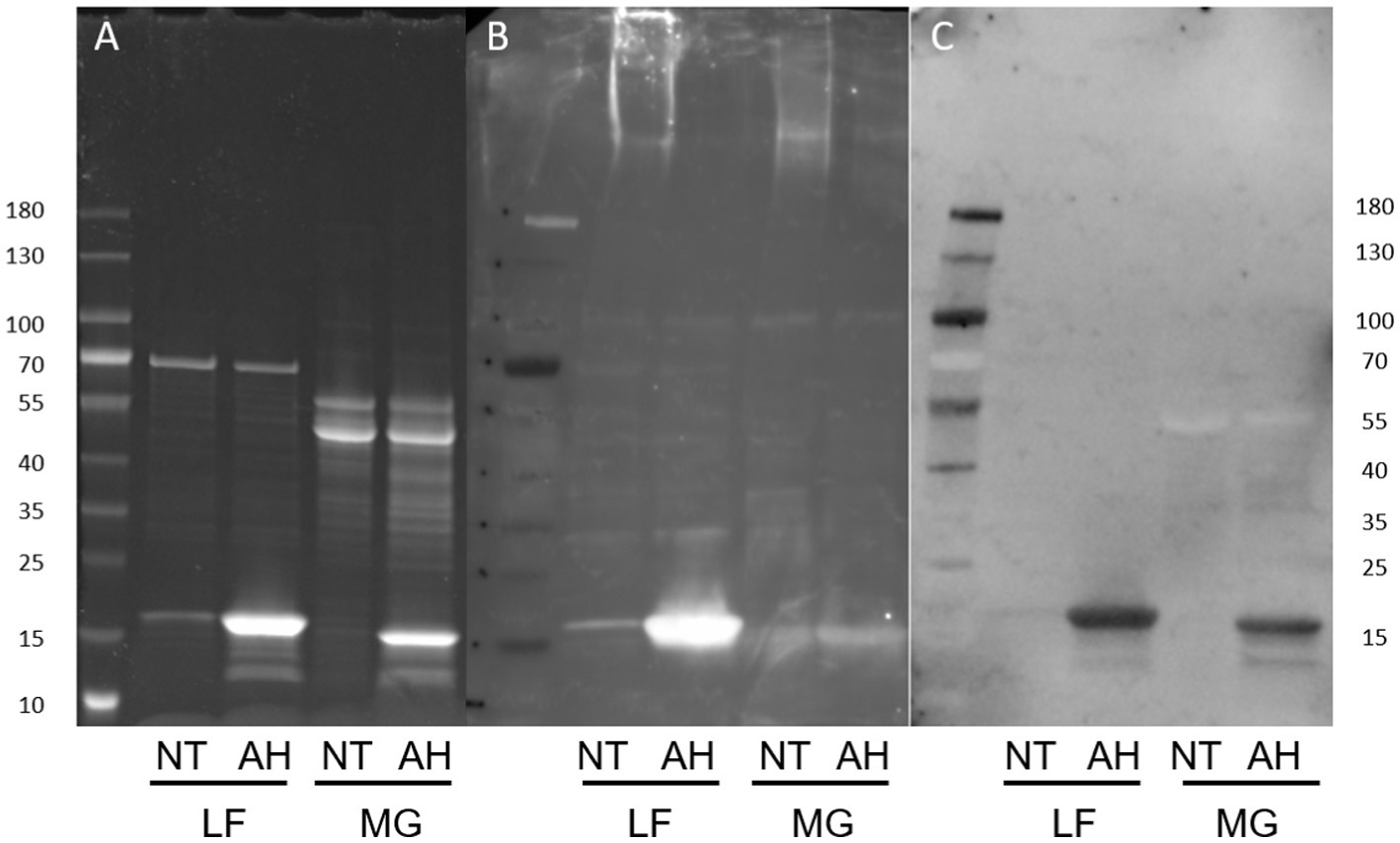
Figure 7. Protein and lectin staining of released E. coli surface proteins. NT, Non-treated; AH, Acid-hydrolyzed; LF, AIEC LF82. MG: E. coli K-12 MG1655. (A) Coomassie blue stain, (B) Anti-type I fimbriae-FITC staining, (C) ConA-HRP staining.
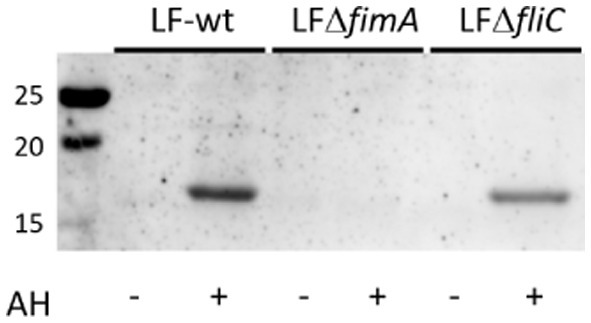
Figure 8. ConA staining of type I fimbriae-enriched LF82 surface protein extracts. ConA staining is negative in the absence of type I fimbria. AH, acid hydrolysis treatment.
Comparing the standard acid hydrolysis treatment against GuHCl treatment, acid hydrolysis appears to be less effective in releasing ConA-reactive FimA subunits (Figure 9), even when the GuHCl samples were prepared in the absence of beta-mercaptoethanol (β-ME). Additionally, acid hydrolysis produces a FimA monomer of the same size as that produced following reduction and alkylation, indicating that the treatment also disrupts the disulfide bridge of the monomer.
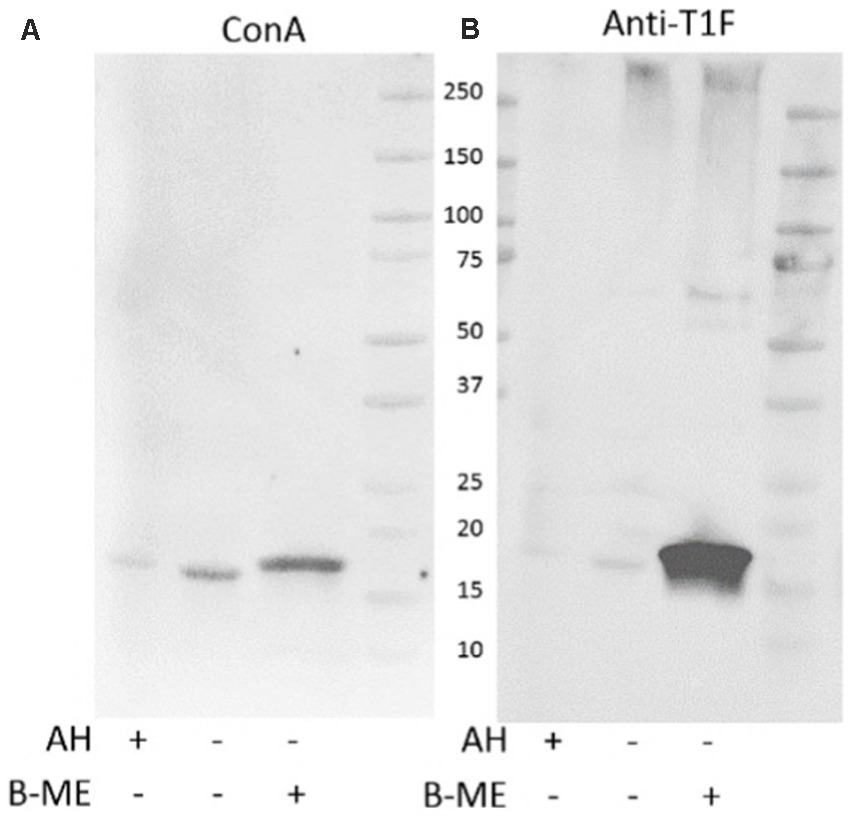
Figure 9. Guanidine HCl-treatment efficiently releases ConA-reactive FimA subunits. Extracted surface proteins of LF82 were treated with GuHCl and analyzed by SDS-PAGE and Western blot. Acid hydrolyzed (AH) sample was included as positive control for FimA subunits. GuHCl-treated samples were prepared in the presence or absence of beta-mercaptoethanol (B-ME). The blot was probed with ConA (A), followed by anti-type I fimbria antiserum (Anti-T1F) (B).
The addition of β-ME does not significantly increase the amount of ConA-reactive FimA, however, it greatly increases staining by anti-type I fimbria detection (Figure 9), ConA staining (left image) versus anti-T1F staining (right image). This leads to the hypothesis that: (1) reduction of the disulfide bond enables better antibody access, or (2) there exist at least two forms of FimA subunits—ConA-reactive, released by GuHCl, and those not recognized by ConA and released in the presence of β-ME. To confirm the efficacy of GuHCl treatment to release ConA-reactive FimA monomers, untreated and GuHCl-treated proteins were dot-blotted to a nitrocellulose membrane in the absence of beta-mercaptoethanol, DTT, and SDS and probed with ConA. The GuHCl-treated sample demonstrated ConA binding, while the untreated sample did not, despite equal loading of protein determined by Ponceau S staining (data not shown). Together, these results indicate a differential response of FimA oligomers in the presence of different reducing agents or conditions.
GNA and WGA staining
Galanthus nivalis (GNA; mannose) lectin staining was comparatively weak, requiring overnight lectin incubation and Clarity max ECL reagent for visualization, but consistently present in the region between the wells of the gel and ~75 kDa gel marker, running as a smear, and overlapping with the anti-type I fimbria staining of the poorly resolved non-hydrolyzed fimbrial polymer (Figure 10A). Wheat germ agglutinin (WGA; sialic acid and N-acetylglucosamine) staining was observed strongly in the E. coli K-12 MG1655 surface protein extract (Figure 10B), interacting with a lower molecular weight structure of between 12 and 14 kDa. This structure has yet to be identified, as antibody staining for FimA, FimF, or FimG were not superimposable with this staining.
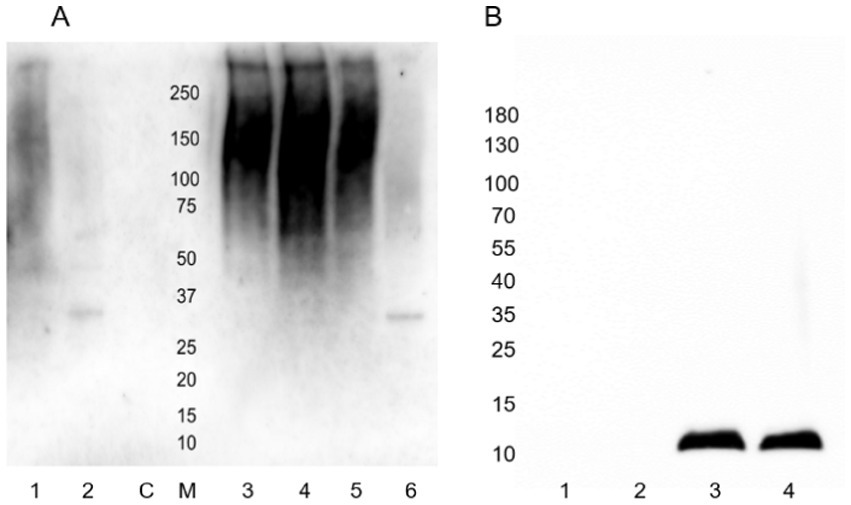
Figure 10. Lectin staining of released E. coli surface proteins. (A) GNA-HRP staining. Lane 1: Mock-treated AIEC LF82; Lane 2: PNGase F-treated AIEC LF82; Lane 3: Acid hydrolyzed E. coli K-12 MG1655; Lane 4: Untreated E. coli K-12 MG1655; Lane 5: Mock-treated E. coli K-12 MG1655; Lane 6: PNGase F-treated E. coli K-12 MG1655. C: PNGase F enzyme only control; M: Molecular weight ladder. (B) WGA-biotin staining. Lane 1: AIEC LF82 non-hydrolyzed; Lane 2: AIEC LF82 acid-hydrolyzed; Lane 3: E. coli K-12 MG1655 non-hydrolyzed; Lane 4: E. coli K-12 MG1655 acid-hydrolyzed.
Lectin-independent glycan detection
Lastly, to confirm the presence of glycosylated structures in the fimbria-enriched surface protein extracts, attached glycans were labeled with biotin-hydrazide, followed by detection with streptavidin-horseradish peroxidase/ECL detection. This staining of the resolved proteins on the PVDF blot demonstrates the presence of a glycan structure on the FimA subunit, when either acid hydrolyzed or depolymerized via GuHCl treatment, while the untreated sample does not demonstrate staining (Figures 11A,B). Additionally, negative controls, including soybean trypsin inhibitor, as well as the molecular weight ladder, were absent of staining. Interestingly, in the E. coli K-12 MG1655 strain fimbria-enriched surface protein extract, there is staining of the appropriate size for the FimA subunit (15 kDa, predominantly in the GuHCl-treated sample), but interestingly also staining of multiple bands in the ~36–80 kDa, in all sample treatments, including untreated and acid hydrolyzed, consistent with sizes above and below the predominant Ponceau S staining for the FliC protein. These results are in accordance with the ConA staining for AIEC LF82 FimA, wherein staining only occurs following either acid hydrolysis or GuHCl treatment, pointing to the presence of a sterically protected glycan. Regarding E. coli K-12 MG1655, the biotin-hydrazide staining confirms the ConA staining for the FimA subunit, as for LF82, but also lends evidence to the presence of additional distinct glycoproteins or a glycoprotein oligomer in the surface protein preparation.
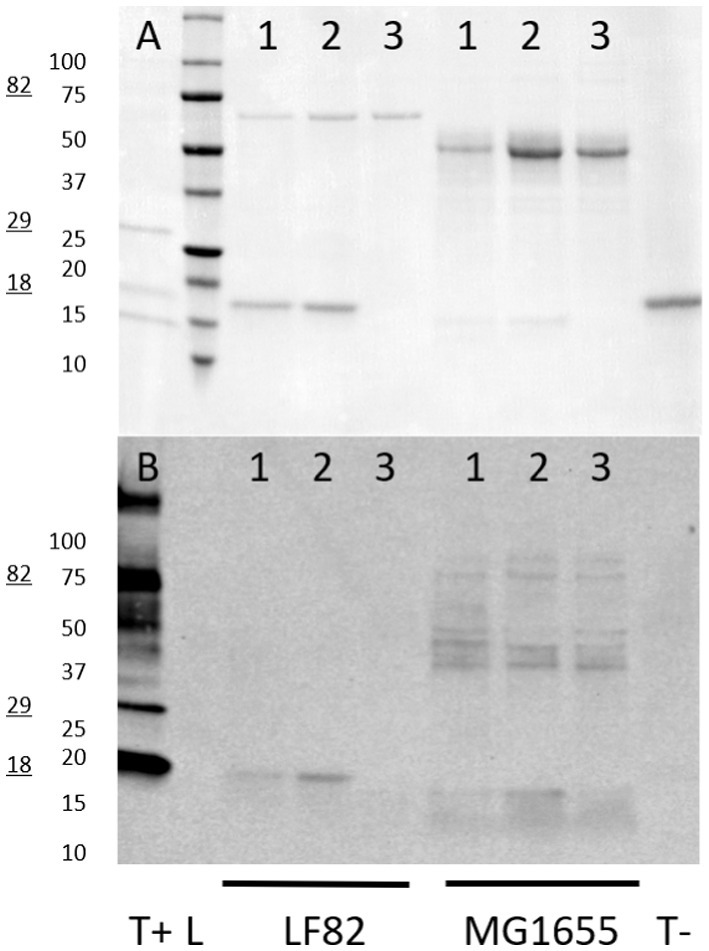
Figure 11. Biotin-hydrazide-labeling of glycoprotein sugars. Glycoproteins on PVDF blots were biotin-hydrazide labeled and detected by streptavidin-HRP and enhanced chemiluminescence. (A) Ponceau S staining; (B) Streptavidin-HRP visualization. Glycoprotein ladder is used as a positive control (T+, 18 kDa, 29 kDa, and 82 kDa glycoprotein bands), while soybean trypsin inhibitor is the negative control (T-). Samples loaded for each strain are: 1 – acid hydrolyzed protein extract, 2 – guanidine HCl-treated surface extract, 3 – untreated surface extract. LF82 – AIEC LF82, MG1655 – E. coli K-12 MG1655.
Further, fimbria-enriched surface protein extracts of AIEC LF82 and E. coli K-12 MG1655 underwent compositional analysis via acid hydrolysis and gas chromatography–mass spectrometric (GC–MS) analysis (Figure 12). The results indicate the presence of monosaccharides present in the LPS inner and outer core of both strains (including glucose - Glc, galactose – Gal, N-acetyl-glucosamine - GlcNAc, and ketodeoxyoctonic acid - KDO), however, the analysis demonstrated the presence of non-LPS sugars as well, including allose (All), talose (Tal), mannuronic acid (ManA), glucuronic acid (GlcA), arabinose (Ara), and fucose (Fuc). Thus, while we and others may have initially thought the samples to be contaminated with LPS, the presence of non-LPS sugars implies the presence of alternative glycans / glycoproteins. Further, comparison of the wild-type and the ΔfliC mutant extracts, certain sugars are no longer detected in the absence of FliC, namely arabinose, allose, talose, glucose, and glucuronic acid. Alternatively, the FliC-mutant was associated with increases in fucose, mannuronic acid, and galactose.
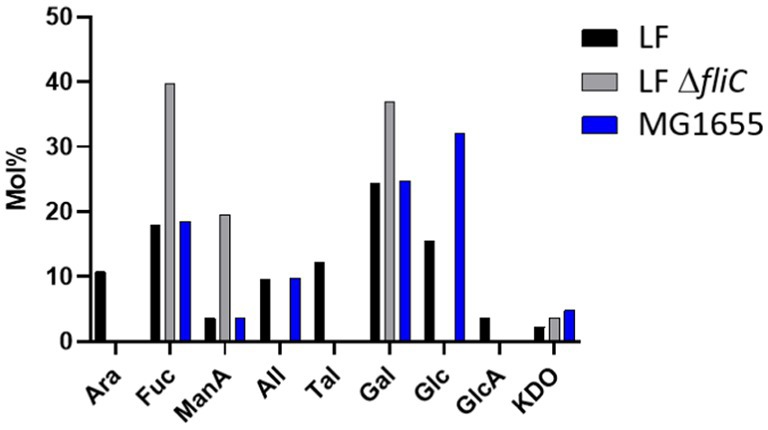
Figure 12. Compositional analysis of flagella/pili-enriched surface protein extracts. LF – AIEC LF82, LF ΔfliC – LF82 ΔfliC, MG1655 – E. coli K-12 MG1655. Ara, arabinose; Fuc, fucose; ManA, mannuronic acid; All, allose; Tal, talose; Gal, galactose; Glc, glucose; GlcA, glucuronic acid; KDO, ketodeoxyoctonic acid. Highlighted sugars are those not found in O83 antigen.
As GuHCl treatment results in the most efficient depolymerization of FimA oligomers, having the highest detection by interaction with ConA, this approach was employed to prepare FimA for binding affinity determination by thermal shift. The interaction of a dilution series of FimA with a fixed concentration of NHS-RED-tagged ConA led to the determination of the KD of interaction of ~40 μM, indicating an intermediary strength of interaction.
Enzymatic treatments
PNGase F or mannosidase
Based on the previous staining by lectins recognizing mannose, more information was sought on the identity or nature of the glycan linkage. Enzymatic treatments using glycosidases were implemented in an attempt to cleave the attached glycan. Both PNGase F and mannosidase treatments did not result in a demonstrable change in ConA staining of the FimA subunit (Figures 13A–C).
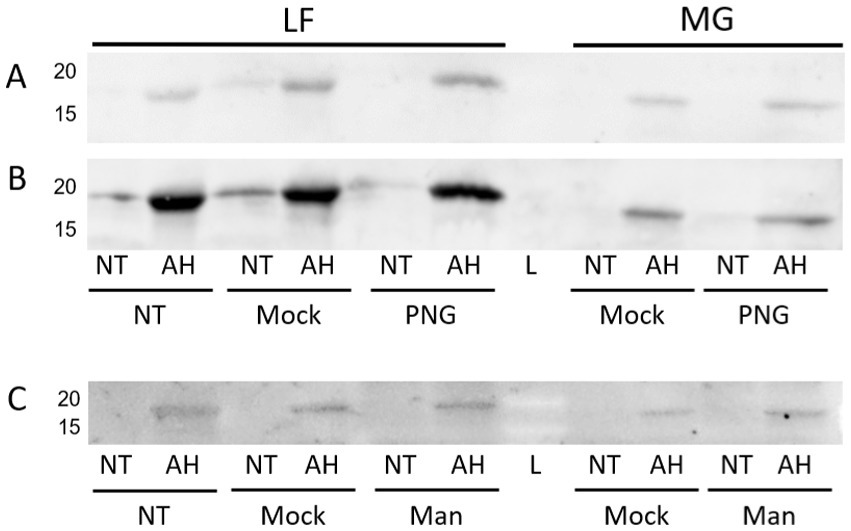
Figure 13. ConA lectin probing following PNGase or mannosidase treatment of released E. coli surface proteins. LF: AIEC LF82, MG: E. coli K-12 MG1655. NT, Non-acid hydrolyzed; AH, Acid hydrolyzed; L, Molecular weight ladder; Mock, Mock-treated sample; Man, Mannosidase-treated sample. (A) PNGase F treatment probed with ConA. (B) Anti-type I fimbria staining of membrane (A). (C) Mannosidase treatment probed with ConA.
However, interestingly, incubation with either of these enzymes resulted in a significant increase in the detection of type I fimbria by Western blot in comparison to the mock-treated samples (Figure 14). The exact mechanism has not yet been identified. While treatment with these enzymes did not manage to diminish the ConA binding, it has demonstrated that the treatments have an effect on the behavior of the fimbrial polymers, by increasing the amount of fimbrial polymer to enter into the SDS-PAGE gel. In samples treated with PNGase F, novel bands were detected by means of the stain-free technology in the Bio-Rad TGX SDS-PAGE gels (Figure 15). However, while bands were excised and analyzed by proteomics, the polymeric nature of FimA/pili or flagella/FliC resulted in the primary result in each band being FimA or FliC, while neither protein is typically detected by the stain-free system owing to their lack of tryptophan residues. Notably, following PNGase F treatment, GNA staining was significantly reduced, nearly eliminating the smear and was associated with the appearance of a GNA-stained band of ~31 kDa (Figure 10A, lanes 2 and 6), however, the identity of this protein has not yet been determined, nor has a PNGase F-released glycan been identified by standard glycomics analyses.
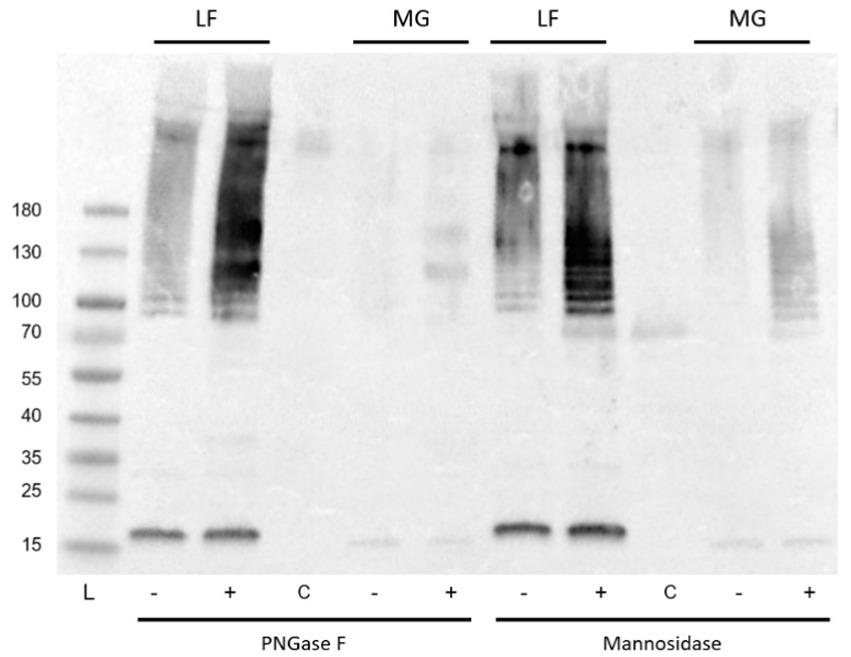
Figure 14. Anti-type I fimbria staining following PNGase F or mannosidase treatment of released E. coli surface proteins. PNGase F or mannosidase treatment of released E. coli surface proteins increased anti-type I pili detection. L: PageRule protein ladder; LF: AIEC LF82; MG: E. coli K-12 MG1655; − denotes mock-treated, + denotes enzyme treated, C denotes enzyme-only control.
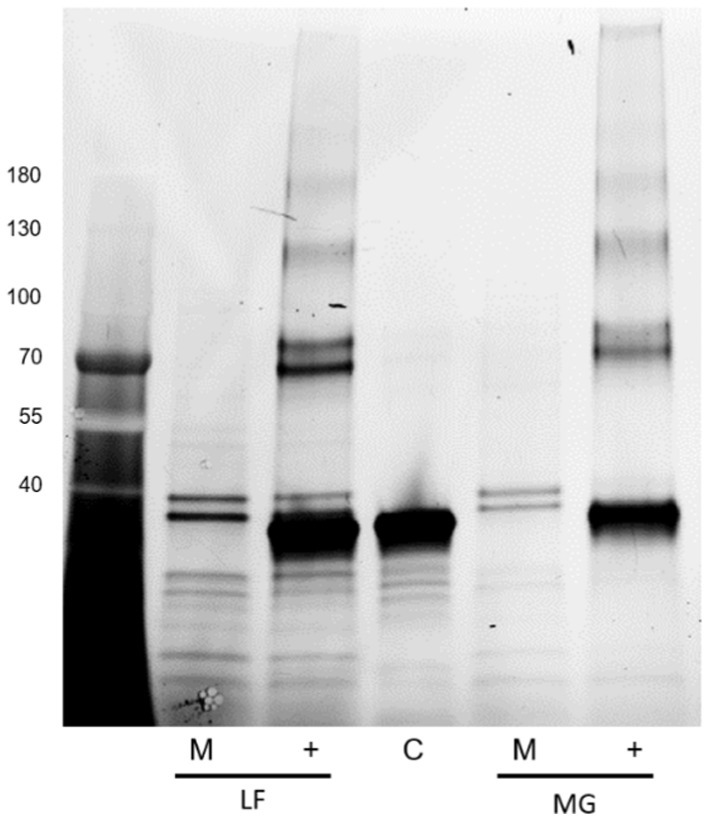
Figure 15. Stain-free detection following PNGase F treatment of released E. coli surface proteins. Far left: Molecular weight ladder. LF: AIEC LF82, MG: E. coli K-12 MG1655, M denotes mock-treated sample, + denotes PNGase F-treated sample, and C denotes the enzyme only control.
While PNGase F treatment of samples results in a generally altered behavior of type I fimbriae, Western blot of identically treated samples with anti-FliC antibody results in a novel ~32 kDa band, implying a susceptibility of the FliC protein to degradation by PNGase F (Figure 16).
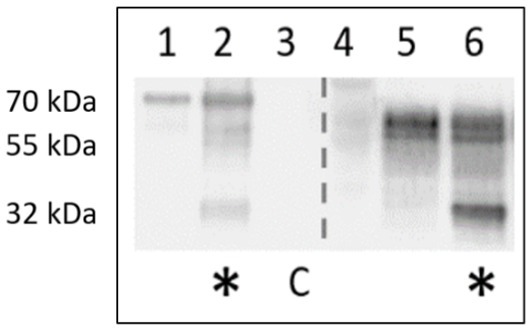
Figure 16. Anti-FliC detection in PNGase F-treated surface protein extracts of AIEC LF82 and E. coli K-12 MG1655. Lanes 1,5 – mock treatment with denaturation buffer, enzyme replaced by H2O. Lanes 2, 6 – PNGase-F-treated (*). C denotes PNGase F enzyme-only control.
Chemical treatments
Alkaline treatment was next tested on samples of surface proteins of LF82 for its ability to release O-linked glycans. Following overnight incubation in alkaline conditions (pH >10) at 45°C, samples were assayed by lectin blot with ConA to determine if the treatment would reduce the intensity of the binding. However, the opposite finding was observed, and following alkaline incubation staining with ConA of FimA subunits was actually increased (not shown) likely indicating a more efficient release of FimA monomers for detection. Furthermore, while it was considered that the FimA monomers would be robust enough to survive beta-elimination, treatment results in complete degradation of the FimA protein, hindering the determination of whether the glycan is O-linked by SDS-PAGE mobility shift or lectin staining.
Discussion
To our knowledge, this represents the first study demonstrating the presence of a glycan on the FimA subunit of Escherichia coli type I fimbriae via Western blot. Previous studies have indicated the presence of reducing sugars (McMichael and Ou, 1979) and speculated on their identities (Palacios Pelaez et al., 2002) in preparations of purified pilin, but have not gone so far as to demonstrate the interaction of a specific lectin with FimA subunits, and to explore means by which to remove the associated glycan.
Type I fimbriae, along with flagella, are surface proteins extending into the external environment (~1 μm and 5–10 μm, respectively) (Namba et al., 1989; Miller et al., 2006) much further than that of LPS (3–5 nm or 17–37 nm, depending on the presence of O-antigen) (Strauss et al., 2009) and their expression can be modulated by contact with host structures (Sheikh et al., 2017). As such, fimbriae represent an initial point of contact of the bacterium with many structures, including other surrounding bacteria, the intestinal epithelium, the overlying mucus barrier, as well as host immune cells (Avalos Vizcarra et al., 2016). Unfortunately, the study and characterization of type I fimbriae is inherently difficult.
Firstly, type I fimbriae are polymeric structures, which impedes the resolution of the involved subunits. In fimbrial preparations, the minor subunits, FimF and FimG, are reported to be present at a ratio of <1:100 to the major subunit, FimA, with quantities of FimH even more scarce (Krogfelt and Klemm, 1988). As such, their study is limited by either the requirement for large batch cultures (often >5 L) (Jones et al., 1995), or their heterologous expression (Alonso-Caballero et al., 2018), which risks artificial or non-natural conditions. Secondly, the unique property of the fimbrial FimA subunit assembly hinders detachment and purification of the major subunit. In turn, FimA subunit release often requires acid hydrolysis, further hindering the study of the ensemble of subunits at once. To overcome this obstacle, studies have relied on deletion mutants or the overexpression of specific subunits (Alonso-Caballero et al., 2018). As studies have focused on the role of individual subunits, the behavior and composition of intact polymers have been largely overlooked, despite early interest primarily around the 1970s (Ponniah et al., 1991; Eshdat et al., 1981; Abraham et al., 1987).
In the current study, we identified a common fimbrial behavior in which intact fimbrial polymers migrate in gradient SDS-PAGE gels. This was detected both in the presence and absence of the high molecular weight smear of fimbrial polymer, as a well-defined band, pointing to a minimal unit size of FimA oligomer of ~90 kDa. This structure, likely representing a pentameric assembly, resists SDS, beta-mercaptoethanol, and heat treatment, but can be further depolymerized with harsher treatments to release FimA subunits. As the fimbrial subunits FimF, FimG, and FimH readily dissociate from the structure following SDS treatment at elevated temperatures (Hanson and Brinton, 1988), it could be proposed that each or a combination of FimF, FimG, or FimH is located along the pilus (Ponniah et al., 1991; Abraham et al., 1987) and once released by SDS results in a minimal FimA homopolymer size of ~90 kDa, present as a common minimal size of type I fimbrial structure maintained across several laboratory and pathobiont strains studied here. Further evidence of smaller, interspersed spans of FimA is put forth by Eshdat et al. (1981) in which they determine that their GuHCl fimbrial preparations contain the lectin component, FimH, at 1%. For a 28 kDa protein, this extrapolates to 1 FimH subunit in 2800 kDa, or 1 FimH subunit per ~165 FimA subunits, given a size of 17 kDa and ignoring the presence of the other two subunits, FimF and FimG (Eshdat et al., 1981). At present, there is mounting evidence of the distribution of FimF, FimG, and FimH along the fimbrial shaft, yet most models incorporate these subunits solely at the fimbrial tip.
Earlier papers studying type I fimbriae are often focused on fimbrial subunits, and accordingly the SDS-PAGE gels are restricted or cropped to <100 kDa in most cases (Hanson and Brinton, 1988), and as such the presence of the fimbrial smear and the size at which it stops is not discussed (Krogfelt and Klemm, 1988). Type I fimbria purification typically includes 3 rounds of MgCl2 precipitation, aiding in the removal of fimbrial fragments, as well as other contaminants. This possibly explains the absence of the FimA-pentamer in most papers investigating type I fimbria, as it may bias the purification to the larger, intact structures. Of note, we observed that the amount of time permitted for re-solubilization of MgCl2 precipitated fimbria could influence the presence of the high molecular weight type I fimbria polymer smear (higher degree of solubility), or favor the presence of the 90 kDa FimA oligomer, as well as FimH presence in the pellet that required an extended period to re-solubilize (unpublished results). Further of note is that Abraham et al. (1983) produced monoclonal antibodies, recognizing either a specific FimA polymeric quaternary structure, but not the dissociated FimA subunits, or solely the dissociated FimA subunits, but not the fully assembled structure. This indicates both, the recognition of larger structures (repeating intervals, in a spiral motif), or hidden antigens, only detected upon dissociation of fimbriae (Abraham et al., 1983). In the current study, the anti-type I fimbria serum was produced in a rabbit host, lacking the specificity of monoclonal antibodies, but allowing the detection of various forms of FimA-bearing fimbrial constructs and the individual FimA subunits. The specificity of the antibody is not limited to a specific strain of E. coli, given its interaction with >10 strains in the current study, but the binding has been shown to be specific to either FimA monomers, or polymerized pili, evidenced by a lack of binding to FimF or FimG (not shown), or FliC in isogenic mutants for FimA/pili.
Following the observation of ConA staining of released FimA subunits, but not of the non-hydrolyzed type I fimbria samples, it was hypothesized that the glycan may be inaccessible due to either steric hindrance or internal localization in the tight helical fimbrial structure. This is in agreement with the findings of Abraham et al. (1983) in which glycerol treatment to uncoil fimbriae or guanidine hydrochloride treatment to induce dissociation of type I fimbriae permitted antibody recognition of previously hidden epitopes (Abraham et al., 1983; Abraham et al., 1992). Accordingly, we hypothesized given that acid hydrolysis and release of FimA subunits from the polymeric fimbriae is required to expose the attached glycan to enable lectin binding, acid hydrolysis should be included before enzymatic treatment, with the aim to remove the newly exposed glycan. However, pre-treatment by acid hydrolysis before PNGase F enzymatic treatment failed to decrease ConA recognition of the FimA subunit, implying either (i) the enzymatic treatments were not successful despite the exposure of the FimA glycan, or (ii) re-neutralization of samples prior to treatment results in re-folding of the FimA subunit and protection of the associated glycan, although acid hydrolysis is generally considered irreversible.
The exact role of the glycans linked to FimA has not yet been elucidated, but based on chemical and enzymatic treatments to remove sugars unexpectedly increasing the quantity of fimbriae migrating into SDS-PAGE gels, it is hypothesized that the currently unidentified sugars may play a role in E. coli type I pilus and flagellar stability. Glycans are typically vulnerable to acid hydrolysis, yet McMichael and Ou demonstrated that the detection of reducing sugars on type I fimbriae requires acid hydrolysis (McMichael and Ou, 1979). Additionally, their study implied the presence of a non-O-linked glycan present on fimbriae, possibly representing an alternative glycan moiety, proposed to be an N-glycan, based on their alkaline hydrolysis results. However, our results following digestion with PNGase F or mannosidase indicate that the FimA-associated glycan is either not an N-glycan, or a non-classical N-glycan resisting PNGase F cleavage. Further, regarding PNGase F activity, following incubation in surface protein extracts (AIEC LF82 and E. coli K-12 MG1655), both type I pili oligomers and FliC detection are significantly increased, despite no increase in FimA monomer. This change in migration implies an effect of PNGase F on the structure, organization, or interaction among these surface organelles. Moreover, we have demonstrated the susceptibility of FliC to be degraded following incubation with PNGase F. This results in a band of ~32 kDa staining with anti-FliC antibodies. At present it has not been determined if it is a degradation product, or has been released by disruption of an unidentified interaction. Sequence analysis of the FliC protein demonstrates the potential presence of an N-glycan attachment site based on the bacterial N-glycosylation sequon D/E-X-N-X-S/T (X ≠ Pro), where Asn (N) is the attachment site (Kowarik et al., 2006). Cleavage of the protein at this site would produce an appropriately sized fragment, but this remains to be confirmed, as PNGase F is not commonly associated with protein cleavage. Follow-up PNGase F digestion experiments have been reproduced using a mannose-binding protein conjugate of PNGase F, to exploit the size difference between the enzyme and the novel band (PNGase F-MBP: 41 versus 32 kDa novel band; not shown). However, despite anti-FliC detection of the novel bands following digestion with a different version of the enzyme, the resolution did not prove sufficient for further analyses. Future experiments will exploit the presence of the conjugated mannose-binding protein to remove the enzyme and facilitate extraction of the novel band.
Evidence is accumulating regarding the glycosylation of FliC and its implication for motility and virulence (Scott et al., 2011). In the current study, lectin blotting with GNA, ConA, or WGA was unable to identify an associated glycan with the FliC protein. However, in TCA precipitated surface proteins of a panel of E. coli strains, untreated samples, (without acid hydrolysis or GuHCl de-polymerization), demonstrated a trend of Alcian Blue staining, corresponding with the approximate size of FliC in the respective strains. This finding indicates the presence of a charged molecule, often demonstrated to be either sulphate or an acidic carbohydrate, but remains to be confirmed in future experiments.
Treatment with biotin-hydrazide indicated the presence of glycans or glycan-bearing structures of various sizes approximately overlapping with that of the predicted size of FliC. While Alcian blue staining was associated with a single band, biotin-hydrazide detection revealed a greater range of structures. These observations are promising, but require further testing to conclusively rule out the presence of LPS.
Despite lectin staining of surface protein extracts enriched for fimbriae and flagella indicating the presence of mannose or glucose, treatment with a mannosidase did not reduce the lectin staining of ConA (GNA staining confounded by staining of mannosidase enzyme), nor did lectin inhibition with alpha-methylmannose or glucose (100 mM). Interestingly, PNGase F or mannosidase treatment increased the staining of type I fimbriae in treated samples compared with mock-treated controls.
To rule out the potential for aberrant behavior of the lectins, a methodology which directly conjugated biotin to an oxidized glycan was employed. The results indicate the distinct staining of the FimA subunits in the LF82 samples, but not FliC. Conversely, the E. coli K-12 MG1655 sample demonstrated staining for FimA subunits, as well as separate bands varying between 30 and 80 kDa. Secondly, compositional analysis revealed the presence of mannuronic acid in the enriched fimbria sample of LF82, which was augmented in the flagella-deficient mutant. Given the potential for acidic saccharides, Alcian blue staining was employed, first staining positive for dot blots for pili-enriched samples, and later on total protein blots across several strains, revealing conserved staining of a ~ 36 kDa band, as well as strain specific staining. This staining implies the presence of acidic- or muco-polysaccharides associated with surface proteins in the panel of strains screened. The exact identities and function require further research.
As E. coli do not possess a characteristically typical glycosylation pathway, the origin of the glycan is hypothesized to come from a non-specialized synthetic pathway, potentially associated with the assembly of LPS. Thorsing et al. (2021) have investigated the presence of heptose on the YghJ protein secreted by E. coli H10407, and in doing so have inactivated the hldE gene in E. coli K-12 MG1655, used as the protein-expression strain. The previous works of Boysen et al. (2016) demonstrated that the pathogenic strain was more likely to glycosylate surface proteins of the H10407 strain. However, it draws attention to the fact that it was deemed necessary to express the YghJ protein in the E. coli K-12 MG1655 ΔhldE strain, inferring the ability of the common E. coli K-12 MG1655 strain to modify proteins with heptose.
Isogenic mutants for the genes involved in the LPS synthesis pathway are often associated with an absence or reduction in the expression of surface organelles, including flagella and fimbriae. More specifically, this involves the genes required for the synthesis or addition of the LPS inner core saccharides. While knockout of those genes involved in the attachment of 3-deoxy-D-manno-oct-2-ulosonic acids (Kdo) to lipid A are lethal, those involved in the attachment of the subsequent heptoses produce a phenotype referred to as “deep rough” linked to colony appearance (Raetz and Whitfield, 2002; Nakao et al., 2012). The first gene involved in heptose addition, hldE (formerly waaE or rfaE) encodes the HepI transferase (Desroy et al., 2009). HldE mutants in ETEC H10407 are non-flagellated and display altered piliation (Maigaard Hermansen et al., 2018). Recently, HldE has demonstrated the capacity to glycosylate non-LPS structures, including YghJ, which is a secreted metalloprotease able to degrade the intestinal mucin layer, thus facilitating colonization. As LPS synthesis and expression and presence of fimbria and flagella are closely interconnected, the investigation of the potential activity of LPS enzymes in their modification via isogenic mutants is hindered.
The limitations of our study lie in the fact that classic glycomics and glycoproteomics analyses have thus far failed to identify a fimbrial or FimA-associated glycan. However, this may be explained, at least partly, following the enzymatic and chemical treatments in the current study, and that FimA is inherently resistant to trypsin digestion (Abraham et al., 1992). As it was not possible to directly confirm the presence of a fimbria-associated glycan(s), indirect biochemical means were employed. Of note, based on the findings of Abraham et al. (1992), sample preparation for glycoproteomics may benefit from the inclusion of glycerol, in order to augment the fimbriae subunits susceptibility to trypsin digestion, however, necessitating later removal of the glycerol from the digested peptides.
Additionally, bacterial surface protein glycan modifications are often in lower abundance than those of eukaryotic cells, and require enrichment steps for their detection. As an example, in the study by Boysen et al. (2016) in which glycopeptides were enriched, the YghJ protein was originally found to possess 4 amino acid residues with O-linked heptose, while in a subsequent study it was found to be hyper-heptosylated bearing 54 occupied sites. Notably, despite the previous efforts of groups to identify E. coli glycoproteins (Xiu et al., 2012), the use of lectins specific to either more standard N-glycans or O-glycans will have missed those proteins modified with heptose.
Despite the exact identity of the FimA subunit modification remaining currently unknown, the study has cast light on the varying behavior of the FimA subunits, while they are typically regarded as a homopolymer of identical subunits. However, the current study demonstrates the presence of a pentameric FimA structure, which when treated with guanidine hydrochloride results in a FimA tetramer which demonstrates varying susceptibility reducing agents. Accordingly, this sequence of treatments highlights the differences in resistance of FimA oligomers, as well as novel means to selectively dissociate FimA oligomers to distinct sizes.
In summary, the results of the current study demonstrate the presence of post-translational modification of type I fimbriae and highlight further areas of research interest regarding the surface proteins of E. coli. Future research will focus on determining the exact identity of the FimA-associated glycan, as well as its importance to bacterial survival, colonization, or infection.
Materials and methods
Materials
Magnesium chloride, dithiothreitol (DTT), iodoacetamide (IAA), biotinylated wheat germ agglutinin (WGA) lectin, FITC-conjugated anti-rabbit IgG antibody, and biotin- and HRP-conjugated concanavalin A (ConA) lectin were obtained from Merck Sigma (France). HRP-conjugated galanthus nivalis lectin (GNA) was obtained from USBiological (CliniSciences, Nanterre; G1044-25). HRP-conjugated anti-rabbit antibody was obtained from Cell Signaling Technology via Ozyme (France). Primary rabbit anti-FliC antibody (ab93713) was obtained from Abcam, and the anti-type I fimbria serum was a kind gift from Dr. Annie Brée (Université de Tours, France).
Bacterial strains and growth conditions
Ampicillin-erythromycin–resistant E. coli strain LF82, isolated from a chronic ileal lesion of a CD patient (Darfeuille-Michaud et al., 1998), was used as the AIEC reference strain in the current study. The clinical AIEC strains CEA816S, CEA614S (15B), CEA218U (3A), CEA 814S, CEA224S were isolated from the ileal lesions of CD patients from the CEALIVE cohort (Buisson et al., 2021), while the strains POP18-M0, POP90-M0, POP135-M0, and POP179-M0 were isolated during the study of post-operative (POP) recurrence in the REMIND cohort (Buisson et al., 2023). The AIEC strain LF82 and the commensal strain E. coli K-12 MG1655 were routinely cultured 48 h in lysogeny broth (LB) broth at 37°C under static conditions to promote fimbriae expression. Briefly, glycerol stocks were streaked for individual colonies, which was then used for inoculation of the starter culture. Cultures were diluted 1,000-fold and grown for 48 h to enhance type I fimbrial production. E. coli strains were grown under static conditions in large Erlenmeyer flasks (culture volume was ≤20% of maximal volume) to ensure a larger air:liquid interface for the culture. Additional E. coli K-12, deletion mutants, and AIEC strains used in the screening experiment are part of the M2iSH laboratory strain collection and are found in Table 2.
Protein purification
Bacterial cultures were grown under static conditions for 48 h at 37°C to promote fimbriae expression. Following growth, cultures were incubated at 4°C for 30 min, and then harvested by centrifugation at 10,000 x g, 4°C for 10 min. Bacterial pellets were then washed with cold PBS, and finally resuspended at a final volume of 45 mL PBS / 500 mL culture. Bacterial surface proteins were sheared by mechanical force using an Ultraturrax blender with 5 pulses at 16000 rpm of 1 min on ice, with 1 min rest periods between pulses. Intact bacteria and debris were then pelleted by centrifugation at 10000 x g, for 15 min at 4°C, and the supernatant containing released proteins was conserved. Alternatively, to limit or avoid potential fragmentation of released flagella and pili, extraction of surface proteins was implemented as per Barnich et al. (2003). Briefly, instead of mechanical shearing, collected and washed bacteria were resuspended in PBS and heated at 60°C for 20 min with vigorous shaking. This extraction method was found to yield equivalent amounts of flagella and type I fimbriae relative to mechanical shearing, while enabling higher throughput of samples.
When global analysis of released proteins was required, released surface proteins were precipitated with trichloroacetic acid (TCA; 10% final concentration). Following 30 min at 4°C, samples were collected by centrifugation at 15,000 x g for 15 min, washed twice in 100% ice cold acetone, and resuspended in 1/1000 volume milli-Q water and stored at 4°C until analyzed, as freeze–thaw has been demonstrated to fragment pili (Ponniah et al., 1991). Relative enrichment of flagella and type I fimbriae was carried out by precipitation in 30% ammonium sulphate, with overnight incubation under gentle agitation at 4°C, and collection by centrifugation as above. More strict purification of pili and flagella was achieved by 2 rounds of precipitation in 100 mM MgCl2 for ≥2 h at 4°C, recovery of precipitated proteins by centrifugation, and resuspension in milli-Q water.
SDS-PAGE and western blotting
E. coli surface protein extracts were characterized by SDS-PAGE. 4–20% stain-free TGX gradient gels were used to allow the detection of both non-acid-hydrolyzed and acid hydrolyzed samples simultaneously. For analysis, 9 μL of surface extract was mixed with 4x Laemmli buffer, and heated at 99°C for 5 min prior to loading. Acid hydrolysis of samples was performed by the addition of 0.2 μL of 3.7% HCl to the 12 μL sample, followed by heating at 99°C for 5 min. Samples were then neutralized with ~0.6 μL of 1 M NaOH, and heated at 99°C for 5 min prior to loading. Stain-free protein detection was performed according to Bio-Rad instructions. Western blot analysis of samples was performed with the TurboBlot system, transferring the SDS-PAGE gel to PVDF using the high molecular weight program. Following transfer, blots were dried under a stream of warm air and then re-activated in methanol prior to blocking and detection. Blots were blocked in protein-free TBS buffer (Thermo Scientific) for ≥1 h. To visualize FimA or FliC, blots were incubated with either antibodies raised against E. coli type I fimbria or FliC (diluted 1:10000 in protein-free tris-buffered saline (TBS) blocking solution (Fisher Scientific, France)) for 1 h at room temperature, respectively. Blots were then washed 3 times in TBS + 0.1% Tween 20 (TBST), and incubated for 1 h with anti-rabbit-HRP or anti-rabbit-IgG-FITC (1:10,000; Sigma, France) in protein-free TBS blocking buffer. Blots were washed 4 × 10 min in TBST, following by direct visualization of FITC, or enhanced chemiluminescence via Clarity (Bio-rad) in Bio-rad MP chemidoc. Lectin staining was performed on membranes blocked as for antibody detection. Galanthus nivalis lectin (GNA), Concanavalin A (ConA) or wheat germ agglutinin lectin (WGA) conjugated with horseradish peroxidase (HRP) were diluted to 10 μg/mL in protein-free TBS blocking solution and incubated for ≥1 h at room temperature. Blots were washed 4 × 10 min with TBST and then visualized by enhanced chemiluminescence using the Clarity Max (Bio-Rad) substrate.
Alcian blue staining was performed following protein transfer to nitrocellulose membranes. Membranes were washed with deionized water, then incubated in 1% (w/v) Alcian blue in 3% (v/v) acetic acid, pH 2.5 for 30 min. Membranes were then extensively washed in deionized water to remove unbound dye.
In experiments involving enzymatic (PNGase F) or chemical treatments (reduction and alkylation, or presence/absence of beta-mercaptoethanol), the extracted proteins were aliquoted from the same tube, serving as an internal loading control to account for potential differences in detection.
Enzymatic and chemical treatment of E. coli surface proteins
To further probe the nature of the attached glycans, protein aliquots were subjected to PNGase F or a broad range mannosidase (α1,2/3/6) (New England Biolabs, France) digestion according to the manufacturer’s specifications, with the exception that incubations were carried out overnight for 18 h. Mock treatments were identical, maintaining the initial denaturation step, but substituting water for enzyme. Following incubation, if necessary, samples were concentrated by means of chloroform:methanol precipitation and resuspended in 12 μL H2O for SDS-PAGE analysis. Where acid hydrolysis was performed before and after enzymatic treatments, with the aim of facilitating enzyme access to potential glycans, chloroform:methanol precipitation was used to precipitate samples and adjust sample volumes. To do so, samples were precipitated, acid hydrolyzed, neutralized, precipitated, and resuspended in the original volume of H2O.
Alkaline treatment was carried out, as per McMichael and Ou (1979), on samples to investigate whether the attached glycan(s) were susceptible to alkaline hydrolysis. Surface protein extracts were adjusted to pH > 10 and incubated 16 h at 45°C. Control samples remained neutral, but were treated 16 h at 45°C.
Further, β-elimination to remove O-glycans was carried out similarly to the alkaline treatment, however, including 1 M sodium borohydride as reductant in the 100 mM NaOH, pH 12 solution. This treatment is typically very harsh on proteins, but pili and FimA subunits display unusually highly resistant properties. The resulting solution was neutralized with 5% acetic acid dropwise until the fizzing stopped. The solution was then dialyzed against water (MWCO 3,000 Da) overnight with 3 changes in order to remove salts.
For reduction and alkylation, samples were incubated in 10 mM dithiothreitol at 56°C for 30 min, followed by incubation with 30 mM iodoacetamide at room temperature for 30 min. Samples were then precipitated by the chloroform:methanol method to remove the chemical reagents and resuspended in the original volume.
Guanidine hydrochloride treatment was performed on chloroform:methanol precipitated samples resuspended in saturated GuHCl (~8.6 M) in water for 2 h at 37°C. The guanidine solution is first heated to >37°C to allow saturation, as a saturated solution at 20°C corresponds to ~6 M, rather than 8.6 M at 37°C. Samples were dialyzed extensively against water to remove GuHCl and then concentrated prior to further use.
Following enzymatic or chemical treatments, samples were analyzed by antibody and lectin blotting, to determine the impact on the presence/detection of pili, FimA subunits, or glycan staining.
Nanotemper Monolith × interaction analysis of FimA monomers and ConA
Following optimization of FimA monomer release, the FimA-ConA interaction was characterized by spectral shift using capillary tubes. PBS, pH 6.8 was used as reaction buffer. FimA was prepared at 10 μM, and the NHS-red-tag kit (Nanotemper, Germany) was used to label ConA (Sigma, France), which was diluted according to manufacturer’s instructions.
Hydrazine-biotin tagging of PVDF immobilized proteins
PVDF membranes obtained using the above methodology were washed in milli-Q water followed by glycan oxidation via incubation in 10 mM sodium metaperiodate in 100 mM sodium acetate buffer, pH 5.5 for 20 min in the dark at 4°C with gentle agitation. Membranes were washed extensively with water to remove residual periodate, followed by incubation with 5 mM biotin-hydrazide (Sigma, France) in acetate buffer for 60 min, at ambient temperature with agitation and protected from light. Membranes were blocked with 3% non-fat milk for 1.5 h followed by probing with streptavidin-HRP (1:1000) for 1 h. Labeled glycans were visualized with Clarity ECL (Bio-Rad) and imaged with a Bio-Rad MP Chemidoc. A glycoprotein ladder (Candy Cane ladder; Invitrogen) served as positive control, and soybean trypsin inhibitor, as well as other molecular weight marker proteins (Bio-Rad precision plus blue) as negative controls.
Monosaccharide compositional analysis
Compositional analysis of monosaccharides was conducted on surface protein preparations enriched for type I fimbriae and flagella. Lyophilized extracts of surface proteins underwent methanolysis in 500 μL of 0.5 M HCl in anhydrous methanol at 80°C for 16 h. Methanolic HCl was evaporated and the resulting products were per-acetylated in 200 μL of acetic anhydride and 50 μL of pyridine overnight at room temperature. Reagents were evaporated and the sample was dissolved in chloroform prior to GC–MS analysis.
Data availability statement
The original contributions presented in the study are included in the article/supplementary material, further inquiries can be directed to the corresponding authors.
Author contributions
DK: Conceptualization, Formal analysis, Investigation, Methodology, Visualization, Writing – original draft, Writing – review & editing, Funding acquisition. AS: Conceptualization, Methodology, Writing – review & editing. YR: Formal analysis, Investigation, Methodology, Writing – review & editing. ZC: Data curation, Investigation, Methodology, Writing – review & editing. CC: Formal analysis, Investigation, Writing – review & editing. LB: Writing – review & editing, Methodology, Investigation. MB: Writing – review & editing, Project administration, Funding acquisition, Resources. MH: Writing – review & editing. YG: Methodology, Writing – review & editing. HN: Funding acquisition, Project administration, Supervision, Writing – review & editing, Conceptualization, Resources. NB: Funding acquisition, Project administration, Resources, Supervision, Writing – review & editing, Conceptualization.
Funding
The author(s) declare that financial support was received for the research, authorship, and/or publication of this article. This study was made possible through the generous financing of a Université Clermont Auvergne grant, encouraging the mobility of international researchers, received by Devon Kavanaugh. This work was also supported by the “Ministère de l’Enseignement Supérieur, de la Recherche et de l’Innovation (MESRI), Inserm (Institut national de la santé et de la recherche médicale; UMR1071), INRAE (Institut national de recherche en agriculture, alimentation et environnement; USC 1382), the Agence Nationale de la Recherche of the French government through the program “Investissements d’Avenir” I-SITE CAP 20–25 CLERMONT (Projects HAPPYCROHN, RUNNINGUT and EXOCROHN to HN), the ANR (French National Research Agency; Project AAPG2021_SIALOBACTER to AS, project AAPG2021_RESTOGUT to HN and project AAPG2022_TOPIC to NB and HN), the “Région Auvergne-Rhône-Alpes” (CPER Auvergne 2019, Défi EPICURE), and the National Program “Microbiote” Inserm.
Acknowledgments
We would like to thank the kind gift of anti-FimF-C and anti-FimG-C antibodies from David Thanassi of Stony Brook University, Stony Brook, NY, USA. We would also like to acknowledge the extensive advice and conversations with Stephanie Archer-Hartmann and Parastoo Azadi of the Complex Carbohydrate Research Centre, Georgia, USA, as well as Dimitris Latousakis of the Quadram Institute, Norwich, UK, and Priscilla Branchu of INRAE, Toulouse, France. Finally, we thank Aurélien Birer of the French National Reference Center for Antibiotic Resistance, along with Emilie Vazeille, Michael Rodrigues, Anthony Buisson, and Caroline Chevarin (M2iSH, U1071 Inserm, Inrae 1382, Université Clermont Auvergne) for the isolation and curation of the AIEC strains.
Conflict of interest
The authors declare that the research was conducted in the absence of any commercial or financial relationships that could be construed as a potential conflict of interest.
Generative AI statement
The author(s) declare that no Gen AI was used in the creation of this manuscript.
Publisher’s note
All claims expressed in this article are solely those of the authors and do not necessarily represent those of their affiliated organizations, or those of the publisher, the editors and the reviewers. Any product that may be evaluated in this article, or claim that may be made by its manufacturer, is not guaranteed or endorsed by the publisher.
References
Abraham, S. N., Goguen, J. D., Sun, D., Klemm, P., and Beachey, E. H. (1987). Identification of two ancillary subunits of Escherichia coli type 1 fimbriae by using antibodies against synthetic oligopeptides of fim gene products. J. Bacteriol. 169, 5530–5536. doi: 10.1128/jb.169.12.5530-5536.1987
Abraham, S. N., Hasty, D. L., Simpson, W. A., and Beachey, E. H. (1983). Antiadhesive properties of a quaternary structure-specific hybridoma antibody against type 1 fimbriae of Escherichia coli. J. Exp. Med. 158, 1114–1128. doi: 10.1084/jem.158.4.1114
Abraham, S. N., Land, M., Ponniah, S., Endres, R., Hasty, D. L., and Babu, J. P. (1992). Glycerol-induced unraveling of the tight helical conformation of Escherichia coli type 1 fimbriae. J. Bacteriol. 174, 5145–5148. doi: 10.1128/jb.174.15.5145-5148.1992
Alonso-Caballero, A., Schönfelder, J., Poly, S., Corsetti, F., de Sancho, D., Artacho, E., et al. (2018). Mechanical architecture and folding of E. coli type 1 pilus domains. Nat. Commun. 9:2758. doi: 10.1038/s41467-018-05107-6
Avalos Vizcarra, I., Hosseini, V., Kollmannsberger, P., Meier, S., Weber, S. S., Arnoldini, M., et al. (2016). How type 1 fimbriae help Escherichia coli to evade extracellular antibiotics. Sci. Rep. 6:18109. doi: 10.1038/srep18109
Barnich, N., Boudeau, J., Claret, L., and Darfeuille-Michaud, A. (2003). Regulatory and functional co-operation of flagella and type 1 pili in adhesive and invasive abilities of AIEC strain LF82 isolated from a patient with Crohn’s disease: flagella and adherent-invasive E. coli. Mol. Microbiol. 48, 781–794. doi: 10.1046/j.1365-2958.2003.03468.x
Benz, I., and Schmidt, M. A. (2001). Glycosylation with heptose residues mediated by the aah gene product is essential for adherence of the AIDA-I adhesin. Mol. Microbiol. 40, 1403–1413. doi: 10.1046/j.1365-2958.2001.02487.x
Boysen, A., Palmisano, G., Krogh, T. J., Duggin, I. G., Larsen, M. R., and Møller-Jensen, J. (2016). A novel mass spectrometric strategy “BEMAP” reveals extensive O-linked protein glycosylation in Enterotoxigenic Escherichia coli. Sci. Rep. 6:32016. doi: 10.1038/srep32016
Brinton, C. C. (1965). The structure, function, synthesis and genetic control of bacterial pili and a molecular model for DNA and RNA transport in gram negative bacteria. Trans. N. Y. Acad. Sci. 27, 1003–1054. doi: 10.1111/j.2164-0947.1965.tb02342.x
Buisson, A., Sokol, H., Hammoudi, N., Nancey, S., Treton, X., Nachury, M., et al. (2023). Role of adherent and invasive Escherichia coli in Crohn’s disease: lessons from the postoperative recurrence model. Gut 72, 39–48. doi: 10.1136/gutjnl-2021-325971
Buisson, A., Vazeille, E., Fumery, M., Pariente, B., Nancey, S., Seksik, P., et al. (2021). Faster and less invasive tools to identify patients with ileal colonization by adherent-invasive E. coli in Crohn’s disease. United Eur. Gastroenterol. J. 9, 1007–1018. doi: 10.1002/ueg2.12161
Darfeuille-Michaud, A., Neut, C., Barnich, N., Lederman, E., di Martino, P., Desreumaux, P., et al. (1998). Presence of adherent Escherichia coli strains in ileal mucosa of patients with Crohn’s disease. Gastroenterology 115, 1405–1413. doi: 10.1016/s0016-5085(98)70019-8
Desroy, N., Moreau, F., Briet, S., Fralliec, G. L., Floquet, S., Durant, L., et al. (2009). Towards gram-negative antivirulence drugs: new inhibitors of HldE kinase. Bioorg. Med. Chem. 17, 1276–1289. doi: 10.1016/j.bmc.2008.12.021
Dreux, N., Denizot, J., Martinez-Medina, M., Mellmann, A., Billig, M., Kisiela, D., et al. (2013). Point mutations in FimH adhesin of Crohn’s disease-associated adherent-invasive Escherichia coli enhance intestinal inflammatory response. PLoS Pathog. 9:e1003141. doi: 10.1371/journal.ppat.1003141
Duncan, M. J., Mann, E. L., Cohen, M. S., Ofek, I., Sharon, N., and Abraham, S. N. (2005). The distinct binding specificities exhibited by Enterobacterial type 1 fimbriae are determined by their Fimbrial shafts*. J. Biol. Chem. 280, 37707–37716. doi: 10.1074/jbc.M501249200
Eshdat, Y., Silverblatt, F. J., and Sharon, N. (1981). Dissociation and reassembly of Escherichia coli type 1 pili. J. Bacteriol. 148, 308–314. doi: 10.1128/jb.148.1.308-314.1981
Grass, S., Lichti, C. F., Townsend, R. R., Gross, J., and St Geme, J. W. (2010). The Haemophilus influenzae HMW1C protein is a glycosyltransferase that transfers hexose residues to asparagine sites in the HMW1 Adhesin. Stebbins CE. PLoS Pathog. 6:e1000919. doi: 10.1371/journal.ppat.1000919
Hanson, M. S., and Brinton, C. C. (1988). Identification and characterization of E. coli type-1 pilus tip adhesion protein. Nature 332, 265–268. doi: 10.1038/332265a0
Hanson, M. S., Hempel, J., and Brinton, J. (1988). Purification of the Escherichia coli type 1 pilin and minor pilus proteins and partial characterization of the adhesin protein. J Bacteriol. 170, 3350–3358. doi: 10.1128/jb.170.8.3350-3358.1988
Hartley, M. D., Morrison, M. J., Aas, F. E., Børud, B., Koomey, M., and Imperiali, B. (2011). Biochemical characterization of the O-linked glycosylation pathway in Neisseria gonorrhoeae responsible for biosynthesis of protein Glycans containing N′-Diacetylbacillosamine. Biochemistry 50, 4936–4948. doi: 10.1021/bi2003372
Hopf, P. S., Ford, R. S., Zebian, N., Merkx-Jacques, A., Vijayakumar, S., Ratnayake, D., et al. (2011). Protein glycosylation in Helicobacter pylori: beyond the Flagellins? PLoS One 6:e25722. doi: 10.1371/journal.pone.0025722
Horzempa, J., Held, T. K., Cross, A. S., Furst, D., Qutyan, M., Neely, A. N., et al. (2008). Immunization with a Pseudomonas aeruginosa 1244 pilin provides O-antigen-specific protection. Clin. Vaccine Immunol. 15, 590–597. doi: 10.1128/CVI.00476-07
Jaipuri, F. A., Collet, B. Y. M., and Pohl, N. L. (2008). Synthesis and quantitative evaluation of Glycero-D-manno-heptose binding to Concanavalin a by Fluorous-tag assistance. Angew. Chem. 120, 1731–1734. doi: 10.1002/ange.200704262
Jones, C. H., Pinkner, J. S., Roth, R., Heuser, J., Nicholes, A. V., Abraham, S. N., et al. (1995). FimH adhesin of type 1 pili is assembled into a fibrillar tip structure in the Enterobacteriaceae. Proc. Natl. Acad. Sci. USA 92, 2081–2085. doi: 10.1073/pnas.92.6.2081
Kaper, J. B., Nataro, J. P., and Mobley, H. L. T. (2004). Pathogenic Escherichia coli. Nat. Rev. Microbiol. 2, 123–140. doi: 10.1038/nrmicro818
Kowarik, M., Young, N. M., Numao, S., Schulz, B. L., Hug, I., Callewaert, N., et al. (2006). Definition of the bacterial N-glycosylation site consensus sequence. EMBO J. 25, 1957–1966. doi: 10.1038/sj.emboj.7601087
Krogfelt, K. A., Bergmans, H., and Klemm, P. (1990). Direct evidence that the FimH protein is the mannose-specific adhesin of Escherichia coli type 1 fimbriae. Infect. Immun. 58, 1995–1998. doi: 10.1128/iai.58.6.1995-1998.1990
Krogfelt, K. A., and Klemm, P. (1988). Investigation of minor components of Escherichia coli type 1 fimbriae: protein chemical and immunological aspects. Microb. Pathog. 4, 231–238. doi: 10.1016/0882-4010(88)90073-3
Logan, S. M., Hui, J. P. M., Vinogradov, E., Aubry, A. J., Melanson, J. E., Kelly, J. F., et al. (2009). Identification of novel carbohydrate modifications on Campylobacter jejuni 11168 flagellin using metabolomics-based approaches: Flagellin glycosylation in C. jejuni. FEBS J. 276, 1014–1023. doi: 10.1111/j.1742-4658.2008.06840.x
Lu, Q., Li, S., and Shao, F. (2015). Sweet talk: protein glycosylation in bacterial interaction with the host. Trends Microbiol. 23, 630–641. doi: 10.1016/j.tim.2015.07.003
Maigaard Hermansen, G. M., Boysen, A., Krogh, T. J., Nawrocki, A., Jelsbak, L., and Møller-Jensen, J. (2018). HldE is important for virulence phenotypes in Enterotoxigenic Escherichia coli. Front. Cell. Infect. Microbiol. 8:253. doi: 10.3389/fcimb.2018.00253
McMichael, J. C., and Ou, J. T. (1979). Structure of common pili from Escherichia coli. J. Bacteriol. 138, 969–975. doi: 10.1128/jb.138.3.969-975.1979
Miller, E., Garcia, T., Hultgren, S., and Oberhauser, A. F. (2006). The mechanical properties of E. coli type 1 pili measured by atomic force microscopy techniques. Biophys. J. 91, 3848–3856. doi: 10.1529/biophysj.106.088989
Nakao, R., Ramstedt, M., Wai, S. N., and Uhlin, B. E. (2012). Enhanced biofilm formation by Escherichia coli LPS mutants defective in Hep biosynthesis. PLoS One 7:e51241. doi: 10.1371/journal.pone.0051241
Namba, K., Yamashita, I., and Vonderviszt, F. (1989). Structure of the core and central channel of bacterial flagella. Nature 342, 648–654. doi: 10.1038/342648a0
Neuhaus, A., Selvaraj, M., Salzer, R., Langer, J. D., Kruse, K., Kirchner, L., et al. (2020). Cryo-electron microscopy reveals two distinct type IV pili assembled by the same bacterium. Nat. Commun. 11:2231. doi: 10.1038/s41467-020-15650-w
Palacios Pelaez, R., Martinez Garate, A., and Martinez, Q. J. (2002). Type 1 and type p fimbriae-adhesins isolated from novel e. coli strains, process for their preparation and uses thereof. Available at: https://patents.google.com/patent/US6471966B1/en (Accessed October 29, 2002).
Ponniah, S., Endres, R. O., Hasty, D. L., and Abraham, S. N. (1991). Fragmentation of Escherichia coli type 1 fimbriae exposes cryptic D-mannose-binding sites. J. Bacteriol. 173, 4195–4202. doi: 10.1128/jb.173.13.4195-4202.1991
Raetz, C. R. H., and Whitfield, C. (2002). Lipopolysaccharide Endotoxins. Annu. Rev. Biochem. 71, 635–700. doi: 10.1146/annurev.biochem.71.110601.135414
Schirm, M., Soo, E. C., Aubry, A. J., Austin, J., Thibault, P., and Logan, S. M. (2003). Structural, genetic and functional characterization of the flagellin glycosylation process in Helicobacter pylori: glycosylation of Helicobacter flagellin. Mol. Microbiol. 48, 1579–1592. doi: 10.1046/j.1365-2958.2003.03527.x
Scott, A. E., Twine, S. M., Fulton, K. M., Titball, R. W., Essex-Lopresti, A. E., Atkins, T. P., et al. (2011). Flagellar glycosylation in Burkholderia pseudomallei and Burkholderia thailandensis. J. Bacteriol. 193, 3577–3587. doi: 10.1128/JB.01385-10
Sheikh, A., Rashu, R., Begum, Y. A., Kuhlman, F. M., Ciorba, M. A., Hultgren, S. J., et al. (2017). Highly conserved type 1 pili promote enterotoxigenic E. coli pathogen-host interactions. PLoS Negl. Trop. Dis. 11:e0005586. doi: 10.1371/journal.pntd.0005586
Strauss, J., Burnham, N. A., and Camesano, T. A. (2009). Atomic force microscopy study of the role of LPS O-antigen on adhesion of E. coli: AFM STUDY OF E. coli LPS. J. Mol. Recognit. 22, 347–355. doi: 10.1002/jmr.955
Thorsing, M., Krogh, T. J., Vitved, L., Nawrocki, A., Jakobsen, R., Larsen, M. R., et al. (2021). Linking inherent O-linked protein glycosylation of YghJ to increased antigen potential. Front. Cell. Infect. Microbiol. 11:705468. doi: 10.3389/fcimb.2021.705468
Keywords: type I fimbriae, flagella, post-translational modification, pathobiont, glycobiology, structural characterization, Escherichia coli
Citation: Kavanaugh DW, Sivignon A, Rossez Y, Chouit Z, Chambon C, Béal L, Bonnet M, Hébraud M, Guérardel Y, Nguyen HTT and Barnich N (2025) Biochemical characterization of the Escherichia coli surfaceome: a focus on type I fimbriae and flagella. Front. Microbiol. 16:1507286. doi: 10.3389/fmicb.2025.1507286
Edited by:
Phillip E. Klebba, Kansas State University, United StatesReviewed by:
Avishek Mitra, Oklahoma State University, United StatesSupriyo Ray, Bowie State University, United States
Copyright © 2025 Kavanaugh, Sivignon, Rossez, Chouit, Chambon, Béal, Bonnet, Hébraud, Guérardel, Nguyen and Barnich. This is an open-access article distributed under the terms of the Creative Commons Attribution License (CC BY). The use, distribution or reproduction in other forums is permitted, provided the original author(s) and the copyright owner(s) are credited and that the original publication in this journal is cited, in accordance with accepted academic practice. No use, distribution or reproduction is permitted which does not comply with these terms.
*Correspondence: Devon W. Kavanaugh, ZGV2b24ua2F2YW5hdWdoQHVjYS5mcg==Hang Thi Thu Nguyen, aGFuZy5uZ3V5ZW5AdWNhLmZy
†These authors have contributed equally to this work