- School of Biosciences and Technology, Vellore Institute of Technology SBST, Vellore, Tamil Nadu, India
The emergence and re-emergence of multi-drug-resistant (MDR) infectious diseases have once again posed a significant global health challenge, largely attributed to the development of bacterial resistance to conventional anti-microbial treatments. To mitigate the risk of drug resistance globally, both antibiotics and immunotherapy are essential. Antimicrobial peptides (AMPs), also referred to as host defense peptides (HDPs), present a promising therapeutic alternative for treating drug-resistant infections due to their various mechanisms of action, which encompass antimicrobial and immunomodulatory effects. Many eukaryotic organisms produce HDPs as a defense mechanism, for example Purothionin from Triticum aestivum plant, Defensins, Cathelicidins, and Histatins from humans and many such peptides are currently the focus of research because of their antibacterial, antiviral and anti-fungicidal properties. This article offers a comprehensive review of the immunomodulatory activities of HDPs derived from eukaryotic organisms including humans, plants, birds, amphibians, reptiles, and marine species along with their mechanisms of action and therapeutic benefits.
1 Introduction
Microbial evolution has given us many economically important microorganisms as well as pathogens. Evolution of antimicrobial resistance genes among the microbial strains has been taking place alarmingly over the past few years, leading to the emergence of multi-drug resistant (MDR) or extensively virulent and drug-resistant species such as the Enterococcus faecium, Staphylococcus aureus, Klebsiella pneumoniae, Acinetobacter baumannii, Pseudomonas aeruginosa, and Enterobacter spp., commonly termed the ESKAPE pathogens. This resulted in the discovery of next-generation alternative therapeutics known as host defense or antimicrobial peptides (AMPs) (Guryanova and Ovchinnikova, 2022). These peptides can be found in a wide variety of prokaryotic and eukaryotic organisms in nature. They are of short-length (~ 10 to 50 amino acids) peptides, mostly cationic with basic and hydrophobic amino acids (Huan et al., 2020). Many previous studies revealed that most of these cationic peptides were found to have microbicidal, cytotoxic and immunomodulatory activities against both harmful emerging and remerging pathogens like bacteria, protozoans, yeast, fungi and viruses (Luong et al., 2020; Pasupuleti et al., 2012).
In 1939, Gramicidin was the first AMP isolated from Bacillus species having bactericidal activity against S. pneumonia in mice. This led to the discovery of many AMPs in both prokaryotes and eukaryotes including bactericidal tyrocidine from Bacillus brevis, Purothionin with fungicidal and bactericidal properties from Triticum aestivum plant (Fernandez de Caleya et al., 1972). In 1956, first animal AMP defensin was isolated from leukocyte cells of rabbits, followed by lactoferrin from cow’s milk (Kühnle et al., 2019), cecropins from hemolymph of butterfly pupae Hyalophora cecropia (Wu et al., 2003) and in 1986 Magainins from mucous membrane of frog Xenopus laevis (Zasloff, 1987). AMPs were also found in lysosomes of human leukocytes and the human female reproductive tract (Sharma et al., 2011). To accommodate the increasing number of AMPs, an antimicrobial peptides database was built in 2011. To date, more than 3,200 peptides from various sources, including amphibians (28%), birds (22%), arthropods (11%), plants (10%), insects (7.9%), bacteria (7.4%), mammals (humans) (3.0%), Pisces (2.5%), viruses (1.2%), and fungus (0.4%), have been deposited in the database (Jhong et al., 2022; Guryanova and Ovchinnikova, 2022). CAMPR3, is another database used in the identification of natural AMPs based on structural and sequence analysis, which can be used in designing new and efficient AMPs (Waghu et al., 2016). Figure 1 depicts the chronological order of discovery starting from 1929 till date as antimicrobial drugs.
The immunomodulatory action of small peptides to protect the hosts from infections has been extensively investigated in recent years. These can stimulate or inhibit the host immune system by targeting immune cells such as leukocytes, macrophages, neutrophils and mast cells, thus leading to wound healing and angiogenesis (Lesiuk et al., 2022). Defensins and cathelicidins with immunomodulatory functions have been identified in a variety of sources, including both porcine and human samples (Dlozi et al., 2022). Most of these immunomodulatory peptides were found to be cost-effective, safe and their therapeutic applications are still under process of discovery. The present article provides an overview of natural and synthetic-derived peptides with immunomodulatory activity from various sources to understand their structural and therapeutic properties.
2 Immunomodulatory mechanism of action
The mechanism of immunomodulatory peptides mainly involves intracellular uptake of these peptides via membrane-bound G-protein receptors or localized translocation. They modulate signaling pathways by interacting intracellularly with signaling molecules or receptors (p62 and GAPDH) specifically targeting protein kinases to promote dendritic cell differentiation, recruitment of macrophages and mast cells inducing phagocytosis, stimulating secretion of anti-inflammatory cytokines, causing wound healing, apoptosis and lipopolysaccharide induced suppression of pro-inflammatory cytokines illustrated in Figure 2 (van der Does et al., 2019; Mookherjee et al., 2020; Barlow et al., 2010).
2.1 Recruitment of leukocytes
One of the primary immunomodulatory functions of HDPs was the stimulation of chemokine secretion. Also, they function as chemokines at high concentrations, thereby enhancing chemotactic activity and leukocyte recruitment (Nijnik et al., 2010; Rivas-Santiago et al., 2013). The underlying mechanisms involve multiple cellular chemokine receptors, including G-coupled protein, CCR6, CCR2, and Toll-like receptors, as well as contact with intracellular signaling proteins like GAPDH and p62, which allows eradication of the infections thereby promoting faster wound healing (Hancock et al., 2016; Choi and Mookherjee, 2012).
2.2 Modulation of inflammatory response
HDPs can modulate the pro-inflammatory response by suppressing cytokines production, including interleukins such as IL-6, IL-8 and TNF-α, IL-6, and IL-8 in response to lipopolysaccharides (LPS). The LL-37 peptide was found to modulate cytokine TNF-α production produced in response to lipoteichoic acid and lipo-polysaccharides. They have effectively inhibited pro-inflammatory genes (Overhage et al., 2008; von Köckritz-Blickwede et al., 2008). Similarly, these peptides function as anti-inflammatory agents by preventing the binding of inflammatory stimulators to their target receptors or molecules. This is achieved either by neutralizing lipopolysaccharides (LPS) or by means of competitive inhibition of LPS and CD14 binding. Apart from these, they can suppress the release of interleukins or the expression of transcription factors (Luo and Song, 2021; Rajasekaran et al., 2019).
2.3 Neutrophil function modulation
HDPs can modulate neutrophil function either directly through chemotactic activity or indirectly by triggering the release of chemokines such as Gro-α and IL-8 to control infections (Hemshekhar et al., 2018; Zheng et al., 2007). In addition, neutrophil-derived extracellular traps (NET) containing DNA and HDPs stored in primary and secondary granules of neutrophils destroy biofilms and bacterial growth (de la Fuente-Núñez et al., 2014).
2.4 Enhancement of adaptive immunity
HDPs are capable of recruiting the antigen-presenting cells (APCs) to the infection site, thereby establishing a link between innate and adaptive immunity (Yu et al., 2007; Davidson et al., 2004). In addition to activating APCs, cationic HDPs (CHDPs) possess the ability to regulate the lymphocyte responses, which in turn impacts the adaptive immune response (Allaker, 2008). By boosting immunological activity, these peptides have the potential to cause phagocytic removal of microorganisms (Conlon, 2015).
3 Naturally occurring host defence peptides
The following section summarizes the HDPs from various eukaryotic sources, such as humans, avians, reptiles, amphibians and marine organisms. A detailed list of the peptides from various sources along with their mechanism of action was given in Table 1.
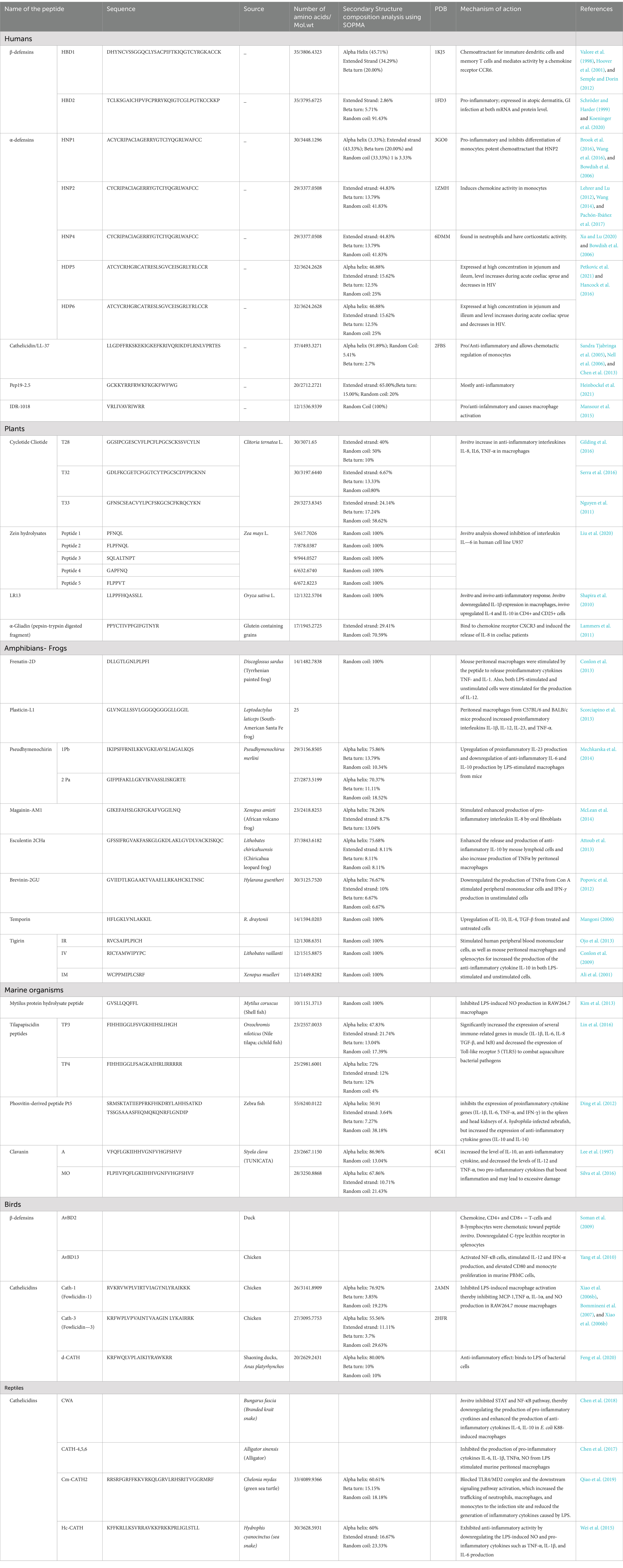
Table 1. List of host defence peptides from various sources along with their structural details and mechanism of action.
3.1 Host defence peptides from mammals: humans
Defensins, Cathelicidins, and Histatins are three categories of peptides endowed with antimicrobial as well as immunomodulatory functions (Guryanova and Ovchinnikova, 2022). Defensins are coded by genes present on chromosome 8 and are made of 30 amino acid residues held together with 3 cysteine disulfide bonds (Bowdish et al., 2006). Based on the type of disulfide bond, defensins are further classified into alpha-defensins and beta-defensins. Both α and β defensins are constitutively synthesized by lymphocytes, neutrophils, and epithelial cells of the mucous membrane and skin (Jarczak et al., 2013).
α-defensins (xCxCRxCxExGxCxGxCCx) are 2 to 6 kDa micropeptides abundant in azurophilic granules present in neutrophils. To date, six distinct α-defensins have been identified, including HNP-1, HNP-2, HNP-3, and HNP-4 (Xu and Lu, 2020) and enteric α-defensins-HD5 and HD6 secreted by Paneth cells of the gastrointestinal tract (Bevins and Salzman, 2011). α-defensins released from necrotic neutrophils can inhibit cytokines (TNFα, IL-6, IL-8, and IL-1β) that are secreted from macrophages, demonstrating anti-inflammatory activities (Miles et al., 2009). Human α-defensins also stimulate pro-inflammatory cytokines (IFN-γ, TNF-α) secretions, thereby stimulating the macrophages to enhance the phagocytotic activity (Soehnlein et al., 2008; Chaly et al., 2000). HNP1 and HNP3 defensins were found to inhibit monocyte differentiation (Droin et al., 2010). Enteric defensins (HD5, HD6) play a critical role in enhancing innate and adaptive immunity. They bind to toll-like receptors via MAP kinase pathway to transmit signals for the transcription of immune response genes, thereby initiating inflammation, wound healing and angiogenesis (Foureau et al., 2010; Eckmann, 2004). Human α-defensins increase the expression of the pro-inflammatory cytokines TNF-α and IL-1 in human monocytes (Chaly et al., 2000).
β-Defensins cluster present on chromosome 8, are released from epithelial cells and shield mucosal membrane from microbial invasions (Schutte et al., 2002). They are promiscuous in nature and can bind or interact with many receptors. β-Defensins (hBD1, hBD2) are chemotactic for immature dendritic and memory T cells (CD4+) (Yang et al., 1999) whereas hBD3 and 4 are chemotactic to monocytes (Wu et al., 2003). When combined with lipoteichoic acid cancer therapy, these peptides via the TLR2/NF-B signaling cascade increase the production of the chemokines (CCL20, CCL22, and CXL8) and cytokines (IL-1, IL-6, and IL-12) in human prostate cancer cells (Kim et al., 2015). Recent studies on the mechanism of hBD3-induced proinflammatory cytokine secretion revealed that hbD3 through TLR1/2 pathway elevates IL-1, IL-6, and IL-8 in human monocytes (Funderburg et al., 2011). There is a dearth of literature concerning the in vivo activity of β-Defensins. To date, only hBD-3 showed immunosuppressive activity under an in vivo setup (Semple et al., 2010).
Cathelicidin LL-37 is an α-helical peptide with 37 amino acid residues and the only cathelicidin synthesized in the human body. This peptide can trigger the synthesis of cytokines IL-6, IL-8, IL-10 and CCL2 either individually or in concert with IL-1 (Yu et al., 2007). Additionally, cathelicidin LL-37 promotes α-defensin production, thereby intensifying the inflammatory process (Zheng et al., 2007).
3.2 Host defence peptides from plants
Although plants have a very complex immune system. The bioactive peptides isolated from wheat, rice, maize, and soybean, have long been valued for their ability to control infections. These peptides have also been intensively explored for their immunomodulatory activities (Pavlicevic et al., 2022). Cationic defensins rich in cysteine amino acids bind to receptors activating neutrophils and macrophages to enhance innate and adaptive immunity. PEP1 and LR13 from Oryza sativa L (Rice) exhibited anti-inflammatory activity in both in vitro and in vivo conditions. The peptides were able to increase CD4+ and CD8+, thereby enhancing anti-inflammatory cytokines (IL-4, IL-10) and suppressing secretion of proinflammatory cytokines (IL-17, IFN-γ) (Shapira et al., 2010). Cyclolinopeptides D and G from Linum usitatissimum have been identified as modulators of proinflammatory responses, associated with increased secretion of IL-1β and TNF-α, while reducing IL-10 secretion in macrophages (Morita et al., 1999; Matsumoto et al., 2001). The second type of HDPs are less homogenous cryptic peptides produced in plants in response to antigens. Through the stimulation of natural killer cells, they can impact innate immunity (Lyapina et al., 2019). The GmSubPep peptide isolated from soybean leaves and synthesized by the extracellular subtilisin-like protease, can bind to membrane receptors and initiate the MAPK signaling cascade (Pearce et al., 2010). Also, the tomato compound CAP-derived peptide 1 (CAPE1) modifies protein–protein interactions and increases the transcription of antioxidative defence genes (Chen et al., 2014).
3.3 Host defence peptides from amphibians: frogs
Frogs are the largest reservoir of AMPs, which play a significant role in their defense mechanism. The skin secretions of the Pipidae frog family, including the genera Silurana, Xenopus, Hymenochirus, and Pseudhymenochirus are a rich source of AMPs with potent antimicrobial, immunomodulatory and anticancer activity (Conlon and Mechkarska, 2014; Patocka et al., 2019). Frog HDPs are produced in high concentrations and stored in the skin’s granular glands which are released immediately in retaliation to stress or tissue damage. These naturally occurring peptides are typically 8 to 48 amino acids in length and lack any conserved regions that are necessary for their therapeutic or biological activity. Most of them are cationic with hydrophobic amino acids and have shown therapeutic activity on mammalian cell lines (Conlon and Mechkarska, 2014). Frenatin 2D and Plasticin-L1 isolated from Alytidae and Leptodactylidae family of frogs did not show any anti-microbial activity but were found to stimulate the release of proinflammatory cytokines TNF-α, IL-1β, IL-12 from macrophages of mouse (Conlon et al., 2013; Scorciapino et al., 2013). Also, Plasticin-L1 enhanced IL-6 production but had no impact on anti-inflammatory IL-10 secretion (Scorciapino et al., 2013). The Tigerinins family of short, cyclic, cationic peptides with α -amidated C-terminus (present only in a few peptides) were isolated from the Dicroglossidae, Ranidae, and Pipidae families, demonstrated anti-inflammatory activity without hemolytic or antibacterial activity (Pantic et al., 2017). In both LPS-stimulated and unstimulated cells, they discovered that they could promote the production of the anti-inflammatory cytokine IL-10 by macrophages, splenocytes, and blood mononuclear cells (Pantic et al., 2014). Furthermore, tigerinin-1 V increased IL-6 production in LPS-triggered macrophages in mice. Tigerinin-1 M and -1 V significantly decreased IFN production in mononuclear cells isolated from mouse spleen, but had no impact on IL-17 release (Pantic et al., 2014). Studies have demonstrated that a number of HDPs, including the African clawed frog Xenopus laevis’s Magainin 1 and 2, Caerulein precursor fragment (CPF-AM1), and peptide glycine leucine amide (PGLa-AM1) stimulate the release of the immunomodulatory molecule glucagon-like peptide 1 (GLP-1), which reduces the immune system’s response to infection (Ojo et al., 2013; Insuela and Carvalho, 2017). Additionally, structurally distinct frog skin peptides, such as Esculentin-2CHa, Alyteserin-2a, and Pseudohymenochirins-1Pb and -2 Pa exhibited antibacterial and immunostimulatory properties (Pantic et al., 2017). However, no frog peptides have yet been used in clinical use as anti-infective or anti-inflammatory medicines. Further studies are underway, to understand HDPs interactions with immune cells and their impact on signaling pathways.
3.4 Host defence peptides from marine organisms
Peptides from marine organisms including fish, oyster, red algae, and mollusk demonstrate enhanced innate and adaptive immunity in host organisms. For example, Phosvitin-derived peptide Pt5 from Danio rerio increased the longevity rate of zebrafish infected with Aeromonas hydrophila by decreasing the expression of IL-1, IL-6, TNF-α, and IFN-γ secretions, while increasing the expression of IL-10 and IL-14 in spleen and kidney (Ding et al., 2012). In mice, Clavanin A and Clavanin-MO from Styela clava (Tunicate) altered cytokine synthesis by suppressing IL-12 and TNF-α and enhancing IL-1 (Lee et al., 1997; Silva et al., 2016). Shellfish Mytilus protein hydrolysate inhibited lipopolysaccharide stimulated nitrous oxide production in RAW 264.7 macrophages (Kim et al., 2013). Shark-derived protein hydrolysate (PeptiBal™) on oral administration enhanced intestinal cytokines (IL-6 and TNF-α) and immunoglobulin IgA production thereby leading to increase in TGF-β and IL-10. Thus indirectly decreasing the E.coli infection induced inflammation in the gut (Mallet et al., 2014). Although many HDPs from marine organisms were studied and formulated with biological enzymes and most of the peptides were tested only on animals. Clinical studies on humans still need to be conducted.
3.5 Host defence peptides from reptiles: snakes, crocodiles, lizards, turtles
In-silico analysis of reptile genomes (turtles, tortoise, snakes, lizards, crocodiles) was carried out to predict defensins and cathelicidins like peptides. In a lizard genome (Anole carolinensis or green anole) 32 β-defensin-like genes have been identified (Dalla Valle et al., 2012). First, in vivo role of β-defensins in wound healing and regeneration of lost tail was identified in the Anole lizard (Alibardi, 2013; Alibardi, 2014). Most of the β-defensin-like peptides found in lizards and snakes were expressed in heterophilic, azurophilic, and basophilic granulocytes whereas β-defensin (TBD-1) from turtles was found in leukocytes (Stegemann et al., 2009). However, no α-defensins have been identified. The second class of HDPs found in reptiles are cathelicidin-like peptides. Blast analysis with human cathelicidin revealed high similarity with cathelicidin-like peptides found in pit snakes, eastern brown snakes and elapid snakes (van Hoek, 2014; Zhao et al., 2008; Schmidt et al., 1992). Cathelicidin-like peptide genes have been also identified in Cobra king snake, Anole lizard, turtles, and crocodiles (van Hoek, 2014). However, no in vitro or in vivo studies were conducted to understand their immunomodulatory activity.
3.6 Host defence peptides from avians-birds
Like reptiles, only β-defensins were identified in birds. 14 chicken β-defensins cluster was identified on chromosome 3 (Hellgren and Ekblom, 2010) and three chicken Cathelicidin gene clusters were identified at a proximal end of chromosome 2 (Xiao et al., 2006a). The first avian defensin was isolated from leukocytes followed by the respiratory tract (AvBD 1, 2, 6, 10), reproductive system (testis- AvBD 1, 2, 4, 6, 10; ovary and oviduct AvBD 6, 10, 12) and spleen (AvBD 13). In vivo studies showed that duck AvBD2 has chemotactic activity toward CD4+, CD8+ T-cells and B-lymphocytes. This peptide was found to induce IFN-γ and IL-12 in mouse monocytes and enhanced CD3+, CD4+ and CD8+ T-cell proliferation (Cuperus et al., 2013). Cathelicidin especially Cath 1, 2, 3 are expressed in many tissues including lungs, tonsils, bone marrow, gastrointestinal tract, respiratory tract and lymphoid organs (Achanta et al., 2012). Cath 1 and 2 (fowlicidins) were found to inhibit the production of IL-1α, nitrous oxide, TNF-α and MCM-1 in mouse macrophages. They also inhibited lipopolysaccharide- induced macrophage activation (Bommineni et al., 2007; Xiao et al., 2006a; Xiao et al., 2006b).
4 The interaction between immunomodulatory HDPs and disease outcomes
Many of the diseases or disorders are associated with the immune system. As discussed in previous sections, HDPs directly or indirectly modulate immune cell secretions and release. These peptides play a critical role in disease progression and recovery. For example, Cathelicidin LL-37 was found to enhance increased uptake of CpG-oligonucleotide ligand by immune cells (CD4+ and CD8+ cells, B cells, neutrophils and macrophages), thereby enhancing the immunostimulatory and anti-tumor activity in ovarian cancer (Chuang et al., 2009). Human keratinocytes treated with Esculentin 1a (1–21), isolated from frog Rana esculenta, had enhanced STAT3 phosphorylation, thereby stimulating the transcription of downstream genes involved in wound healing (Di Grazia et al., 2015). By significantly altering the bovine neutrophil host defense peptide bactenecin, a small synthetic peptide known as innate defense regulator (IDR-)1018 was developed. This peptide acts as an immunoregulator, capable of suppressing the pro-inflammatory response by enhancing the production of selective chemokines and promoting cellular differentiation. It was found to enhance wound healing, anti-biofilm activity, cystic fibrosis and treatment of inflammatory diseases (neuronal damage and cerebral malaria) (Mansour et al., 2015). In both Type I and Type II diabetic Miletus, dysregulation in the HDP synthesis enhanced IFN-α synthesis leading to the progression of the disease (Sun et al., 2015). Also, low concentrations of HDPs were observed to enhance the pro-inflammatory responses thereby leading to multiple autoimmune disorders including rheumatoid arthritis, psoriasis, and systemic lupus erythematosus (SLE) (Kahlenberg and Kaplan, 2013).
5 The role of host defense peptides on immunomodulation in infectious disease management
Antimicrobial peptides are produced as key modulators of the innate immune system from various prokaryotic and eukaryotic organisms. Nowadays, with the alarming rise in infectious diseases, and bacterial resistance to traditional antibiotics, researchers are more inclined toward antimicrobial peptide-based treatment (Xuan et al., 2023). As we have previously discussed in the above sections, most of the AMPs from reptiles, amphibians and plants are studied on animal models and very less on human disease models. Among the very few peptides that have been studied, LL-37, a human cathelicidin, exhibits immunomodulatory activity as well as antimicrobial activity against E. coli and Staphylococcus aureus (Bhattacharjya et al., 2024). LL-37 promotes dendritic cell function contributing to efficient antigen presentation and activation of T cells in response to bacterial infections. Histatins, peptides obtained from human saliva, possess anti-bacterial and anti-fungal activity, by interfering with biofilm formation as well as activating host immune response (Kavanagh and Dowd, 2004). Protegrin-1, from porcine neutrophils, have immunosuppressive effect in chronic inflammatory diseases like sepsis (Javed et al., 2024). To tackle the SARS-CoV-2 pandemic, researchers developed models to incorporate defensins with T-cell and B-cell epitopes for developing vaccines against SARS-CoV-2. They also found that the binding of spike, nucleocapsid and membrane proteins with hBD-2 and hBD-3 escalates the immunogenic properties of the vaccine (Rahmani et al., 2022; Kumar et al., 2021; Guryanova and Ovchinnikova, 2022).
6 Prospects of peptides as therapeutics
Immunomodulatory host defense peptides affect a wide range of immune cells, including T-cells, B-cells, non-killer cells, macrophages, monocytes, CD4+ and CD8+ T cells. These peptides mainly act as ligands binding to Toll-like receptors transmitting signals via MAPK or TLR1/2 pathways enhancing activation of macrophages, stimulation of phagocytosis, an increase in leukocytes, increased production of immunoglobulins, and regulation of cytokines secretions, thereby modulating the innate and adaptive immunity in the host organisms. Due to these immunomodulatory activities, HDPs could be considered potent alternatives to antibiotics in the control of infections.
Clinical trials for many of these HDPs and their synthetic analogs are currently in various stages. Brilacidin, a synthetic peptide, has been effectively evaluated in phase II clinical trials for the treatment of acute bacterial skin infections. It has also been demonstrated to have antiviral activity against the SARS-CoV-2 virus (Hu et al., 2022). The Phase III clinical trials for pexiganan (MSI-78), an analog of magainin obtained from the African clawed frog Xenopus laevis, as a topical cream for diabetic foot ulcer treatment have been completed (Gomes et al., 2020). IDR-1 (Bactenecin), a synthetic peptide, is currently in phase I clinical trials to control inflammation, bacterial infection, and sepsis (Price et al., 2019). Some of the examples of synthetic peptides with immunomodulatory activity in phase II and III clinical trials were given in Table 2.
However, the development of these naturally occurring or synthetic analogs as therapeutics is quite challenging and limited. The cost of producing synthetic peptides is approximately 50 to 400 USD per gram (Afacan et al., 2012). Some of the peptides in clinical trials were found to stimulate histamine production from mast cells, which can be toxic to host cells (Izumiya et al., 1981). The third major concern is their instability. Studies show simple HDPs without disulfide bonds are highly susceptible to proteolytic cleavage by host cell proteases (Kim et al., 2014).
7 Conclusion
The present review summarizes antimicrobial peptides as immunomodulatory agents. Most of the studies have shown that these peptides are effective in alleviating innate and adaptive immunity. These peptides are considered alternative therapeutics for the treatment of microbial infections, wound healing, inflammation control, diabetic care, cancer and auto-immune diseases. However, most of the studies are animal-based studies and not involving human patients. Only a few of them are in clinical trials for further commercial therapeutic applications. To comprehend their structural complexity, ligand-receptor interactions, and mechanism of action, more research is required. Furthermore, the safety and biocompatibility of host defense peptides must be explored to be developed as potent therapeutic agents.
Author contributions
DC: Writing – original draft, Methodology. KS: Conceptualization, Formal analysis, Supervision, Validation, Writing – review & editing.
Funding
The author(s) declare that no financial support was received for the research, authorship, and/or publication of this article.
Conflict of interest
The authors declare that the research was conducted in the absence of any commercial or financial relationships that could be construed as a potential conflict of interest.
Generative AI statement
The author(s) declare that no Generative AI was used in the creation of this manuscript.
Publisher’s note
All claims expressed in this article are solely those of the authors and do not necessarily represent those of their affiliated organizations, or those of the publisher, the editors and the reviewers. Any product that may be evaluated in this article, or claim that may be made by its manufacturer, is not guaranteed or endorsed by the publisher.
Supplementary material
The Supplementary material for this article can be found online at: https://www.frontiersin.org/articles/10.3389/fmicb.2024.1505571/full#supplementary-material
References
Achanta, M., Sunkara, L. T., Dai, G., Bommineni, Y. R., Jiang, W., and Zhang, G. (2012). Tissue expression and developmental regulation of chicken cathelicidin antimicrobial peptides. J. Anim. Sci. Biotechnol. 3:15. doi: 10.1186/2049-1891-3-15
Afacan, J., Yeung, A. T. Y., Pena, O. M., and Hancock, R. E. W. (2012). Therapeutic potential of host defense peptides in antibiotic-resistant infections. Curr. Pharm. Des. 18, 807–819. doi: 10.2174/138161212799277617
Ali, M. F., Soto, A., Knoop, F. C., and Conlon, J. M. (2001). Antimicrobial peptides isolated from skin secretions of the diploid frog, Xenopus tropicalis (Pipidae). Biochim. Biophys. Acta Protein Struct. Mol. Enzymol. 1550, 81–89. doi: 10.1016/S0167-4838(01)00272-2
Alibardi, L. (2013). Ultrastructural immunolocalization of beta-defensin-27 in granulocytes of the dermis and wound epidermis of lizard suggests they contribute to the anti-microbial skin barrier. Anat. Cell Biol. 46:246. doi: 10.5115/acb.2013.46.4.246
Alibardi, L. (2014). Histochemical, biochemical and cell biological aspects of tail regeneration in lizard, an amniote model for studies on tissue regeneration. Prog. Histochem. Cytochem. 48, 143–244. doi: 10.1016/j.proghi.2013.12.001
Allaker, R. P. (2008). Host defence peptides—A bridge between the innate and adaptive immune responses. Trans. R. Soc. Trop. Med. Hyg. 102, 3–4. doi: 10.1016/j.trstmh.2007.07.005
Attoub, S., Mechkarska, M., Sonnevend, A., Radosavljevic, G., Jovanovic, I., Lukic, M. L., et al. (2013). Esculentin-2CHa: A host-defense peptide with differential cytotoxicity against bacteria, erythrocytes and tumor cells. Peptides 39, 95–102. doi: 10.1016/j.peptides.2012.11.004
Barlow, P. G., Beaumont, P. E., Cosseau, C., Mackellar, A., Wilkinson, T. S., Hancock, R. E. W., et al. (2010). The human cathelicidin LL-37 preferentially promotes apoptosis of infected airway epithelium. Am. J. Respir. Cell Mol. Biol. 43, 692–702. doi: 10.1165/rcmb.2009-0250OC
Bevins, C. L., and Salzman, N. H. (2011). Paneth cells, antimicrobial peptides and maintenance of intestinal homeostasis. Nat. Rev. Microbiol. 9, 356–368. doi: 10.1038/nrmicro2546
Bhattacharjya, S., Zhang, Z., and Ramamoorthy, A. (2024). LL-37: structures, antimicrobial activity, and influence on amyloid-related diseases. Biomol. Ther. 14:320. doi: 10.3390/biom14030320
Bommineni, Y. R., Dai, H., Gong, Y.-X., Soulages, J. L., Fernando, S. C., DeSilva, U., et al. (2007). Fowlicidin-3 is an α-helical cationic host defense peptide with potent antibacterial and lipopolysaccharide-neutralizing activities: structure and functions of fowlicidin-3. FEBS J. 274, 418–428. doi: 10.1111/j.1742-4658.2006.05589.x
Bowdish, D. M. E., Davidson, D. J., and Hancock, R. E. W. (2006). “Immunomodulatory properties of Defensins and Cathelicidins” in Antimicrobial peptides and human disease. ed. W. M. Shafer, vol. 306 (Heidelberg: Springer), 27–66.
Brook, M., Tomlinson, G. H., Miles, K., Smith, R. W. P., Rossi, A. G., Hiemstra, P. S., et al. (2016). Neutrophil-derived alpha defensins control inflammation by inhibiting macrophage mRNA translation. Proc. Natl. Acad. Sci. 113, 4350–4355. doi: 10.1073/pnas.1601831113
Chaly, Y. V., Paleolog, E. M., Kolesnikova, T. S., Tikhonov, I. I., Petratchenko, E. V., and Voitenok, N. N. (2000). Neutrophil alpha-defensin human neutrophil peptide modulates cytokine production in human monocytes and adhesion molecule expression in endothelial cells. Eur. Cytokine Netw. 11, 257–266.
Chen, Y., Cai, S., Qiao, X., Wu, M., Guo, Z., Wang, R., et al. (2017). As-CATH1–6, novel cathelicidins with potent antimicrobial and immunomodulatory properties from Alligator sinensis, play pivotal roles in host antimicrobial immune responses. Biochem. J. 474, 2861–2885. doi: 10.1042/BCJ20170334
Chen, Y.-L., Lee, C.-Y., Cheng, K.-T., Chang, W.-H., Huang, R.-N., Nam, H. G., et al. (2014). Quantitative Peptidomics study reveals that a wound-induced peptide from PR-1 regulates immune signaling in tomato. Plant Cell 26, 4135–4148. doi: 10.1105/tpc.114.131185
Chen, S., Lu, Z., Wang, F., and Wang, Y. (2018). Cathelicidin-WA polarizes E. coli K88-induced M1 macrophage to M2-like macrophage in RAW264.7 cells. Int. Immunopharmacol. 54, 52–59. doi: 10.1016/j.intimp.2017.10.013
Chen, X., Takai, T., Xie, Y., Niyonsaba, F., Okumura, K., and Ogawa, H. (2013). Human antimicrobial peptide LL-37 modulates proinflammatory responses induced by cytokine milieus and double-stranded RNA in human keratinocytes. Biochem. Biophys. Res. Commun. 433, 532–537. doi: 10.1016/j.bbrc.2013.03.024
Choi, K.-Y., and Mookherjee, N. (2012). Multiple immune-modulatory functions of cathelicidin host defense peptides. Front. Immunol. 3:149. doi: 10.3389/fimmu.2012.00149
Chuang, C.-M., Monie, A., Wu, A., Mao, C.-P., and Hung, C.-F. (2009). Treatment with LL-37 peptide enhances antitumor effects induced by CpG oligodeoxynucleotides against ovarian cancer. Hum. Gene Ther. 20, 303–313. doi: 10.1089/hum.2008.124
Conlon, J. M. (2015). Host-defense peptides of the skin with therapeutic potential: from hagfish to human. Peptides 67, 29–38. doi: 10.1016/j.peptides.2015.03.005
Conlon, J. M., and Mechkarska, M. (2014). Host-defense peptides with therapeutic potential from skin secretions of frogs from the family Pipidae. Pharmaceuticals 7, 58–77. doi: 10.3390/ph7010058
Conlon, J. M., Mechkarska, M., Pantic, J. M., Lukic, M. L., Coquet, L., Leprince, J., et al. (2013). An immunomodulatory peptide related to Frenatin 2 from skin secretions of the Tyrrhenian painted frog Discoglossus sardus (Alytidae). Peptides 40, 65–71. doi: 10.1016/j.peptides.2012.12.012
Conlon, J. M., Raza, H., Coquet, L., Jouenne, T., Leprince, J., Vaudry, H., et al. (2009). Purification of peptides with differential cytolytic activities from the skin secretions of the central American frog, Lithobates vaillanti (Ranidae). Comparat. Biochem. Physiol. Part C 150, 150–154. doi: 10.1016/j.cbpc.2009.04.003
Cuperus, T., Coorens, M., van Dijk, A., and Haagsman, H. P. (2013). Avian host defense peptides. Dev. Compar. Immunol. 41, 352–369. doi: 10.1016/j.dci.2013.04.019
Dalla Valle, L., Benato, F., Maistro, S., Quinzani, S., and Alibardi, L. (2012). Bioinformatic and molecular characterization of beta-defensins-like peptides isolated from the green lizard Anolis carolinensis. Dev. Compar. Immunol. 36, 222–229. doi: 10.1016/j.dci.2011.05.004
Davidson, D. J., Currie, A. J., Reid, G. S. D., Bowdish, D. M. E., MacDonald, K. L., Ma, R. C., et al. (2004). The cationic antimicrobial peptide LL-37 modulates dendritic cell differentiation and dendritic cell-induced T cell polarization. J. Immunol. 172, 1146–1156. doi: 10.4049/jimmunol.172.2.1146
de la Fuente-Núñez, C., Reffuveille, F., Haney, E. F., Straus, S. K., and Hancock, R. E. W. (2014). Broad-Spectrum anti-biofilm peptide that targets a cellular stress response. PLoS Pathog. 10:e1004152. doi: 10.1371/journal.ppat.1004152
Di Grazia, A., Cappiello, F., Imanishi, A., Mastrofrancesco, A., Picardo, M., Paus, R., et al. (2015). The frog skin-derived antimicrobial peptide Esculentin-1a(1-21)NH2 promotes the migration of human HaCaT keratinocytes in an EGF receptor-dependent manner: A novel promoter of human skin wound healing? PLoS One 10:e0128663. doi: 10.1371/journal.pone.0128663
Ding, Y., Liu, X., Bu, L., Li, H., and Zhang, S. (2012). Antimicrobial-immunomodulatory activities of zebrafish phosvitin-derived peptide Pt5. Peptides 37, 309–313. doi: 10.1016/j.peptides.2012.07.014
Dlozi, P. N., Gladchuk, A., Crutchley, R. D., Keuler, N., Coetzee, R., and Dube, A. (2022). Cathelicidins and defensins antimicrobial host defense peptides in the treatment of TB and HIV: Pharmacogenomic and nanomedicine approaches towards improved therapeutic outcomes. Biomed. Pharmacother. 151:113189. doi: 10.1016/j.biopha.2022.113189
Droin, N., Jacquel, A., Hendra, J.-B., Racoeur, C., Truntzer, C., Pecqueur, D., et al. (2010). Alpha-defensins secreted by dysplastic granulocytes inhibit the differentiation of monocytes in chronic myelomonocytic leukemia. Blood 115, 78–88. doi: 10.1182/blood-2009-05-224352
Duncan, V. M. S., and O’Neil, D. A. (2013). Commercialization of antifungal peptides. Fungal Biol. Rev. 26, 156–165. doi: 10.1016/j.fbr.2012.11.001
Eckmann, L. (2004). Innate immunity and mucosal bacterial interactions in the intestine. Curr. Opin. Gastroenterol. 20, 82–88. doi: 10.1097/00001574-200403000-00006
Feng, X., Jin, S., Wang, M., Pang, Q., Liu, C., Liu, R., et al. (2020). The critical role of tryptophan in the antimicrobial activity and cell toxicity of the duck antimicrobial peptide DCATH. Front. Microbiol. 11:1146. doi: 10.3389/fmicb.2020.01146
Fernandez de Caleya, R., Gonzalez-Pascual, B., García-Olmedo, F., and Carbonero, P. (1972). Susceptibility of phytopathogenic bacteria to wheat purothionins in vitro. Appl Microbiol. 23, 998–1000. doi: 10.1128/am.23.5.998-1000.1972
Fjell, C. D., Hiss, J. A., Hancock, R. E. W., and Schneider, G. (2012). Designing antimicrobial peptides: form follows function. Nat. Rev. Drug Discov. 11, 37–51. doi: 10.1038/nrd3591
Foureau, D. M., Mielcarz, D. W., Menard, L. C., Schulthess, J., Werts, C., Vasseur, V., et al. (2010). TLR9-dependent induction of intestinal α-Defensins by Toxoplasma gondii. J. Immunol. 184, 7022–7029. doi: 10.4049/jimmunol.0901642
Funderburg, N. T., Jadlowsky, J. K., Lederman, M. M., Feng, Z., Weinberg, A., and Sieg, S. F. (2011). The toll-like receptor 1/2 agonists Pam3CSK4 and human β-defensin-3 differentially induce interleukin-10 and nuclear factor-κB signalling patterns in human monocytes: differential activation of APCs by TLR1/2 ligands. Immunology 134, 151–160. doi: 10.1111/j.1365-2567.2011.03475.x
Gilding, E. K., Jackson, M. A., Poth, A. G., Henriques, S. T., Prentis, P. J., Mahatmanto, T., et al. (2016). Gene coevolution and regulation lock cyclic plant defence peptides to their targets. New Phytol. 210, 717–730. doi: 10.1111/nph.13789
Gomes, D., Santos, R., Reis, S., Carvalho, S., Rego, P., Tavares, L., et al. (2020). Pexiganan in combination with Nisin to control Polymicrobial diabetic foot infections. Antibiotics 9:128. doi: 10.3390/antibiotics9030128
Guryanova, S. V., and Ovchinnikova, T. V. (2022). Immunomodulatory and allergenic properties of antimicrobial peptides. Int. J. Mol. Sci. 23:2499. doi: 10.3390/ijms23052499
Hancock, R. E. W., Haney, E. F., and Gill, E. E. (2016). The immunology of host defence peptides: beyond antimicrobial activity. Nat. Rev. Immunol. 16, 321–334. doi: 10.1038/nri.2016.29
Heinbockel, L., Weindl, G., Correa, W., Brandenburg, J., Reiling, N., Wiesmüller, K.-H., et al. (2021). Anti-infective and anti-inflammatory mode of action of peptide 19-2.5. Int. J. Mol. Sci. 22:1465. doi: 10.3390/ijms22031465
Hellgren, O., and Ekblom, R. (2010). Evolution of a cluster of innate immune genes (β-defensins) along the ancestral lines of chicken and zebra finch. Immunome Res. 6:3. doi: 10.1186/1745-7580-6-3
Hemshekhar, M., Choi, K.-Y. G., and Mookherjee, N. (2018). Host defense peptide LL-37-mediated chemoattractant properties, but not anti-inflammatory cytokine IL-1RA production, is selectively controlled by Cdc42 rho GTPase via G protein-coupled receptors and JNK mitogen-activated protein kinase. Front. Immunol. 9:1871. doi: 10.3389/fimmu.2018.01871
Hoover, D. M., Chertov, O., and Lubkowski, J. (2001). The structure of human β-Defensin-1. J. Biol. Chem. 276, 39021–39026. doi: 10.1074/jbc.M103830200
Hu, Y., Jo, H., DeGrado, W. F., and Wang, J. (2022). Brilacidin, a COVID-19 drug candidate, demonstrates broad-spectrum antiviral activity against human coronaviruses OC43, 229E, and NL63 through targeting both the virus and the host cell. J. Med. Virol. 94, 2188–2200. doi: 10.1002/jmv.27616
Huan, Y., Kong, Q., Mou, H., and Yi, H. (2020). Antimicrobial Peptides: Classification, Design, Application and Research Progress in Multiple Fields. Front Microbiol. 11:582779. Published 2020 Oct 16. doi: 10.3389/fmicb.2020.582779
Insuela, D. B. R., and Carvalho, V. F. (2017). Glucagon and glucagon-like peptide-1 as novel anti-inflammatory and immunomodulatory compounds. Eur. J. Pharmacol. 812, 64–72. doi: 10.1016/j.ejphar.2017.07.015
Izumiya, N., Kato, T., and Waki, M. (1981). Synthesis of biologically active cyclic peptides. Biopolymers 20, 1785–1791. doi: 10.1002/bip.1981.360200903
Jarczak, J., Kościuczuk, E. M., Lisowski, P., Strzałkowska, N., Jóźwik, A., Horbańczuk, J., et al. (2013). Defensins: natural component of human innate immunity. Hum. Immunol. 74, 1069–1079. doi: 10.1016/j.humimm.2013.05.008
Javed, A., Oedairadjsingh, T., Ludwig, I. S., Wood, T. M., Martin, N. I., Broere, F., et al. (2024). Antimicrobial and immunomodulatory activities of porcine cathelicidin Protegrin-1. Mol. Immunol. 173, 100–109. doi: 10.1016/j.molimm.2024.07.011
Jhong, J.-H., Yao, L., Pang, Y., Li, Z., Chung, C.-R., Wang, R., et al. (2022). dbAMP 2.0: updated resource for antimicrobial peptides with an enhanced scanning method for genomic and proteomic data. Nucleic Acids Res. 50, D460–D470. doi: 10.1093/nar/gkab1080
Kahlenberg, J. M., and Kaplan, M. J. (2013). Little peptide, big effects: the role of LL-37 in inflammation and autoimmune disease. J. Immunol. 191, 4895–4901. doi: 10.4049/jimmunol.1302005
Kavanagh, K., and Dowd, S. (2004). Histatins: antimicrobial peptides with therapeutic potential. J. Pharm. Pharmacol. 56, 285–289. doi: 10.1211/0022357022971
Kim, H., Jang, J. H., Kim, S. C., and Cho, J. H. (2014). De novo generation of short antimicrobial peptides with enhanced stability and cell specificity. J. Antimicrob. Chemother. 69, 121–132. doi: 10.1093/jac/dkt322
Kim, E.-K., Kim, Y.-S., Hwang, J.-W., Kang, S. H., Choi, D.-K., Lee, K.-H., et al. (2013). Purification of a novel nitric oxide inhibitory peptide derived from enzymatic hydrolysates of Mytilus coruscus. Fish Shellfish Immunol. 34, 1416–1420. doi: 10.1016/j.fsi.2013.02.023
Kim, J. H., Kim, K.-H., Kim, H. J., Lee, J., and Myung, S. C. (2015). Expression of Beta-Defensin 131 promotes an innate immune response in human prostate epithelial cells. PLoS One 10:e0144776. doi: 10.1371/journal.pone.0144776
Koeninger, L., Armbruster, N. S., Brinch, K. S., Kjaerulf, S., Andersen, B., Langnau, C., et al. (2020). Human β-Defensin 2 mediated immune modulation as treatment for experimental colitis. Front. Immunol. 11:93. doi: 10.3389/fimmu.2020.00093
Kudrimoti, M., Curtis, A., Azawi, S., Worden, F., Katz, S., Adkins, D., et al. (2016). Dusquetide: A novel innate defense regulator demonstrating a significant and consistent reduction in the duration of oral mucositis in preclinical data and a randomized, placebo-controlled phase 2a clinical study. J. Biotechnol. 239, 115–125. doi: 10.1016/j.jbiotec.2016.10.010
Kühnle, N., Dederer, V., and Lemberg, M. K. (2019). Intramembrane proteolysis at a glance: from signalling to protein degradation. J Cell Sci. 132:jcs217745. Published 2019 Aug 15. doi: 10.1242/jcs.217745
Kumar, V., Kancharla, S., Kolli, P., and Jena, M. (2021). Reverse vaccinology approach towards the in-silico multiepitope vaccine development against SARS-CoV-2. F1000Research 10:44. doi: 10.12688/f1000research.36371.1
Lammers, K. M., Khandelwal, S., Chaudhry, F., Kryszak, D., Puppa, E. L., Casolaro, V., et al. (2011). Identification of a novel immunomodulatory gliadin peptide that causes interleukin-8 release in a chemokine receptor CXCR3-dependent manner only in patients with coeliac disease: gliadin-induced IL-8 is CXCR3-dependent only in CD patients. Immunology 132, 432–440. doi: 10.1111/j.1365-2567.2010.03378.x
Lee, I. H., Cho, Y., and Lehrer, R. I. (1997). Effects of pH and salinity on the antimicrobial properties of clavanins. Infect. Immun. 65, 2898–2903. doi: 10.1128/iai.65.7.2898-2903.1997
Lehrer, R. I., and Lu, W. (2012). α-Defensins in human innate immunity: α-Defensins. Immunol. Rev. 245, 84–112. doi: 10.1111/j.1600-065X.2011.01082.x
Lesiuk, M., Paduszyńska, M., and Greber, K. E. (2022). Synthetic antimicrobial immunomodulatory peptides: ongoing studies and clinical trials. Antibiotics 11:1062. doi: 10.3390/antibiotics11081062
Lin, W.-C., Chang, H.-Y., and Chen, J.-Y. (2016). Electrotransfer of the tilapia piscidin 3 and tilapia piscidin 4 genes into skeletal muscle enhances the antibacterial and immunomodulatory functions of Oreochromis niloticus. Fish Shellfish Immunol. 50, 200–209. doi: 10.1016/j.fsi.2016.01.034
Liu, P., Liao, W., Qi, X., Yu, W., and Wu, J. (2020). Identification of immunomodulatory peptides from zein hydrolysates. Eur. Food Res. Technol. 246, 931–937. doi: 10.1007/s00217-020-03450-x
Luo, Y., and Song, Y. (2021). Mechanism of antimicrobial peptides: antimicrobial, anti-inflammatory and Antibiofilm activities. Int. J. Mol. Sci. 22:11401. doi: 10.3390/ijms222111401
Luong, H. X., Thanh, T. T., and Tran, T. H. (2020). Antimicrobial peptides - Advances in development of therapeutic applications. Life Sci. 260:118407. doi: 10.1016/j.lfs.2020.118407
Lyapina, I., Filippova, A., and Fesenko, I. (2019). The role of peptide signals hidden in the structure of functional proteins in plant immune responses. Int. J. Mol. Sci. 20:4343. doi: 10.3390/ijms20184343
Mallet, J.-F., Duarte, J., Vinderola, G., Anguenot, R., Beaulieu, M., and Matar, C. (2014). The immunopotentiating effects of shark-derived protein hydrolysate. Nutrition 30, 706–712. doi: 10.1016/j.nut.2013.10.025
Mangoni, M. L. (2006). Temporins, anti-infective peptides with expanding properties. Cell. Mol. Life Sci. 63, 1060–1069. doi: 10.1007/s00018-005-5536-y
Mansour, S. C., de la Fuente-Núñez, C., and Hancock, R. E. W. (2015). Peptide IDR-1018: modulating the immune system and targeting bacterial biofilms to treat antibiotic-resistant bacterial infections: immunomodulatory and anti-biofilm peptide. J. Pept. Sci. 21, 323–329. doi: 10.1002/psc.2708
Matsumoto, T., Shishido, A., Morita, H., Itokawa, H., and Takeya, K. (2001). Cyclolinopeptides F–I, cyclic peptides from linseed. Phytochemistry 57, 251–260. doi: 10.1016/S0031-9422(00)00442-8
McLean, D. T. F., McCrudden, M. T. C., Linden, G. J., Irwin, C. R., Conlon, J. M., and Lundy, F. T. (2014). Antimicrobial and immunomodulatory properties of PGLa-AM1, CPF-AM1, and magainin-AM1: potent activity against oral pathogens. Regul. Pept. 194–195, 63–68. doi: 10.1016/j.regpep.2014.11.002
Mechkarska, M., Attoub, S., Sulaiman, S., Pantic, J., Lukic, M. L., and Michael Conlon, J. (2014). Anti-cancer, immunoregulatory, and antimicrobial activities of the frog skin host-defense peptides pseudhymenochirin-1Pb and pseudhymenochirin-2Pa. Regul. Pept. 194–195, 69–76. doi: 10.1016/j.regpep.2014.11.001
Miles, K., Clarke, D. J., Lu, W., Sibinska, Z., Beaumont, P. E., Davidson, D. J., et al. (2009). Dying and necrotic neutrophils are anti-inflammatory secondary to the release of α-Defensins. J. Immunol. 183, 2122–2132. doi: 10.4049/jimmunol.0804187
Min, C., Ohta, K., Kajiya, M., Zhu, T., Sharma, K., Shin, J., et al. (2012). The antimicrobial activity of the appetite peptide hormone ghrelin. Peptides 36, 151–156. doi: 10.1016/j.peptides.2012.05.006
Mookherjee, N., Anderson, M. A., Haagsman, H. P., and Davidson, D. J. (2020). Antimicrobial host defence peptides: functions and clinical potential. Nat. Rev. Drug Discov. 19, 311–332. doi: 10.1038/s41573-019-0058-8
Mookherjee, N., Chow, L. N. Y., and Hancock, R. E. W. (2012). “Immunomodulatory cationic peptide therapeutics: A new paradigm in infection and immunity” in ACS symposium series. eds. K. Rajasekaran, J. W. Cary, J. M. Jaynes, and E. Montesinos, vol. 1095 (Washington, DC: American Chemical Society), 1–19.
Morita, H., Shishido, A., Matsumoto, T., Itokawa, H., and Takeya, K. (1999). Cyclolinopeptides B - E, new cyclic peptides from Linum usitatissimum. Tetrahedron 55, 967–976. doi: 10.1016/S0040-4020(98)01086-2
Nell, M. J., Tjabringa, G. S., Wafelman, A. R., Verrijk, R., Hiemstra, P. S., Drijfhout, J. W., et al. (2006). Development of novel LL-37 derived antimicrobial peptides with LPS and LTA neutralizing and antimicrobial activities for therapeutic application. Peptides 27, 649–660. doi: 10.1016/j.peptides.2005.09.016
Nguyen, G. K. T., Zhang, S., Nguyen, N. T. K., Nguyen, P. Q. T., Chiu, M. S., Hardjojo, A., et al. (2011). Discovery and characterization of novel Cyclotides originated from chimeric precursors consisting of Albumin-1 chain A and Cyclotide domains in the Fabaceae Family. J. Biol. Chem. 286, 24275–24287. doi: 10.1074/jbc.M111.229922
Nijnik, A., Madera, L., Ma, S., Waldbrook, M., Elliott, M. R., Easton, D. M., et al. (2010). Synthetic cationic peptide IDR-1002 provides protection against bacterial infections through chemokine induction and enhanced leukocyte recruitment. J. Immunol. 184, 2539–2550. doi: 10.4049/jimmunol.0901813
Ojo, O. O., Conlon, J. M., Flatt, P. R., and Abdel-Wahab, Y. H. A. (2013). Frog skin peptides (tigerinin-1R, magainin-AM1, −AM2, CPF-AM1, and PGla-AM1) stimulate secretion of glucagon-like peptide 1 (GLP-1) by GLUTag cells. Biochem. Biophys. Res. Commun. 431, 14–18. doi: 10.1016/j.bbrc.2012.12.116
Overhage, J., Campisano, A., Bains, M., Torfs, E. C. W., Rehm, B. H. A., and Hancock, R. E. W. (2008). Human host defense peptide LL-37 prevents bacterial biofilm formation. Infect. Immun. 76, 4176–4182. doi: 10.1128/IAI.00318-08
Pachón-Ibáñez, M. E., Smani, Y., Pachón, J., and Sánchez-Céspedes, J. (2017). Perspectives for clinical use of engineered human host defense antimicrobial peptides. FEMS Microbiol. Rev. 41, 323–342. doi: 10.1093/femsre/fux012
Pantic, J. M., Jovanovic, I. P., Radosavljevic, G. D., Arsenijevic, N. N., Conlon, J. M., and Lukic, M. L. (2017). The potential of frog skin-derived peptides for development into therapeutically-valuable immunomodulatory agents. Molecules 22:2071. doi: 10.3390/molecules22122071
Pantic, J. M., Mechkarska, M., Lukic, M. L., and Conlon, J. M. (2014). Effects of tigerinin peptides on cytokine production by mouse peritoneal macrophages and spleen cells and by human peripheral blood mononuclear cells. Biochimie 101, 83–92. doi: 10.1016/j.biochi.2013.12.022
Pasupuleti, M., Schmidtchen, A., and Malmsten, M. (2012). Antimicrobial peptides: key components of the innate immune system. Crit Rev Biotechnol. 32, 143–171. doi: 10.3109/07388551.2011.594423
Patocka, J., Nepovimova, E., Klimova, B., Wu, Q., and Kuca, K. (2019). Antimicrobial peptides: amphibian host defense peptides. Curr. Med. Chem. 26, 5924–5946. doi: 10.2174/0929867325666180713125314
Pavlicevic, M., Marmiroli, N., and Maestri, E. (2022). Immunomodulatory peptides—A promising source for novel functional food production and drug discovery. Peptides 148:170696. doi: 10.1016/j.peptides.2021.170696
Pearce, G., Munske, G., Yamaguchi, Y., and Ryan, C. A. (2010). Structure–activity studies of GmSubPep, a soybean peptide defense signal derived from an extracellular protease. Peptides 31, 2159–2164. doi: 10.1016/j.peptides.2010.09.004
Petkovic, M., Mouritzen, M. V., Mojsoska, B., and Jenssen, H. (2021). Immunomodulatory properties of host Defence peptides in skin wound healing. Biomol. Ther. 11:952. doi: 10.3390/biom11070952
Popovic, S., Urbán, E., Lukic, M., and Conlon, J. M. (2012). Peptides with antimicrobial and anti-inflammatory activities that have therapeutic potential for treatment of acne vulgaris. Peptides 34, 275–282. doi: 10.1016/j.peptides.2012.02.010
Price, R. L., Bugeon, L., Mostowy, S., Makendi, C., Wren, B. W., Williams, H. D., et al. (2019). In vitro and in vivo properties of the bovine antimicrobial peptide, Bactenecin 5. PLoS One 14:e0210508. doi: 10.1371/journal.pone.0210508
Qiao, X., Yang, H., Gao, J., Zhang, F., Chu, P., Yang, Y., et al. (2019). Diversity, immunoregulatory action and structure-activity relationship of green sea turtle cathelicidins. Dev. Comparat. Immunol. 98, 189–204. doi: 10.1016/j.dci.2019.05.005
Rahmani, A., Baee, M., Saleki, K., Moradi, S., and Nouri, H. R. (2022). Applying high throughput and comprehensive immunoinformatics approaches to design a trivalent subunit vaccine for induction of immune response against emerging human coronaviruses SARS-CoV, MERS-CoV and SARS-CoV-2. J. Biomol. Struct. Dyn. 40, 6097–6113. doi: 10.1080/07391102.2021.1876774
Rajasekaran, G., Dinesh Kumar, S., Nam, J., Jeon, D., Kim, Y., Lee, C. W., et al. (2019). Antimicrobial and anti-inflammatory activities of chemokine CXCL14-derived antimicrobial peptide and its analogs. Biomembranes 1861, 256–267. doi: 10.1016/j.bbamem.2018.06.016
Rivas-Santiago, B., Castañeda-Delgado, J. E., Rivas Santiago, C. E., Waldbrook, M., González-Curiel, I., León-Contreras, J. C., et al. (2013). Ability of innate Defence regulator peptides IDR-1002, IDR-HH2 and IDR-1018 to protect against Mycobacterium tuberculosis infections in animal models. PLoS One 8:e59119. doi: 10.1371/journal.pone.0059119
Sandra Tjabringa, G., Rabe, K. F., and Hiemstra, P. S. (2005). The human cathelicidin LL-37: A multifunctional peptide involved in infection and inflammation in the lung. Pulm. Pharmacol. Ther. 18, 321–327. doi: 10.1016/j.pupt.2005.01.001
Schmidt, J. J., Weinstein, S. A., and Smith, L. A. (1992). Molecular properties and structure-function relationships of lethal peptides from venom of Wagler’s pit viper, Trimeresurus wagleri. Toxicon 30, 1027–1036. doi: 10.1016/0041-0101(92)90047-9
Schröder, J.-M., and Harder, J. (1999). Human beta-defensin-2. Int. J. Biochem. Cell Biol. 31, 645–651. doi: 10.1016/S1357-2725(99)00013-8
Schutte, B. C., Mitros, J. P., Bartlett, J. A., Walters, J. D., Jia, H. P., Welsh, M. J., et al. (2002). Discovery of five conserved β-defensin gene clusters using a computational search strategy. Proc. Natl. Acad. Sci. 99, 2129–2133. doi: 10.1073/pnas.042692699
Scorciapino, M. A., Manzo, G., Rinaldi, A. C., Sanna, R., Casu, M., Pantic, J. M., et al. (2013). Conformational analysis of the frog skin peptide, Plasticin-L1, and its effects on production of Proinflammatory cytokines by macrophages. Biochemistry 52, 7231–7241. doi: 10.1021/bi4008287
Semple, F., and Dorin, J. R. (2012). β-Defensins: multifunctional modulators of infection, inflammation and more? J. Innate Immun. 4, 337–348. doi: 10.1159/000336619
Semple, F., Webb, S., Li, H.-N., Patel, H. B., Perretti, M., Jackson, I. J., et al. (2010). Human β-defensin 3 has immunosuppressive activity in vitro and in vivo: immunomodulation. Eur. J. Immunol. 40, 1073–1078. doi: 10.1002/eji.200940041
Serra, A., Hemu, X., Nguyen, G. K. T., Nguyen, N. T. K., Sze, S. K., and Tam, J. P. (2016). A high-throughput peptidomic strategy to decipher the molecular diversity of cyclic cysteine-rich peptides. Sci. Rep. 6:23005. doi: 10.1038/srep23005
Shapira, E., Brodsky, B., Proscura, E., Nyska, A., Erlanger-Rosengarten, A., and Wormser, U. (2010). Amelioration of experimental autoimmune encephalitis by novel peptides: involvement of T regulatory cells. J. Autoimmun. 35, 98–106. doi: 10.1016/j.jaut.2010.03.004
Sharma, M., Sharma, S., Prasad, R., Rajwanshi, A., Sethi, S., Samanta, P., et al. (2011). Characterization of low molecular weight antimicrobial peptide from human female reproductive tract. Indian J. Med. Res. 134:679. doi: 10.4103/0971-5916.90996
Silva, O. N., de la Fuente-Núñez, C., Haney, E. F., Fensterseifer, I. C. M., Ribeiro, S. M., Porto, W. F., et al. (2016). An anti-infective synthetic peptide with dual antimicrobial and immunomodulatory activities. Sci. Rep. 6:35465. doi: 10.1038/srep35465
Soehnlein, O., Kai-Larsen, Y., Frithiof, R., Sorensen, O. E., Kenne, E., Scharffetter-Kochanek, K., et al. (2008). Neutrophil primary granule proteins HBP and HNP1–3 boost bacterial phagocytosis by human and murine macrophages. J. Clin. Invest. 118, 3491–3502. doi: 10.1172/JCI35740
Soman, S. S., Nair, S., Issac, A., Arathy, D. S., Niyas, K. P., Anoop, M., et al. (2009). Immunomodulation by duck defensin, Apl_AvBD2: in vitro dendritic cell immunoreceptor (DCIR) mRNA suppression, and B- and T-lymphocyte chemotaxis. Mol. Immunol. 46, 3070–3075. doi: 10.1016/j.molimm.2009.06.003
Stegemann, C., Kolobov, A., Leonova, Y. F., Knappe, D., Shamova, O., Ovchinnikova, T. V., et al. (2009). Isolation, purification and de novo sequencing of TBD-1, the first beta-defensin from leukocytes of reptiles. Proteomics 9, 1364–1373. doi: 10.1002/pmic.200800569
Sun, J., Furio, L., Mecheri, R., van der Does, A. M., Lundeberg, E., Saveanu, L., et al. (2015). Pancreatic β-cells limit autoimmune diabetes via an immunoregulatory antimicrobial peptide expressed under the influence of the gut microbiota. Immunity 43, 304–317. doi: 10.1016/j.immuni.2015.07.013
Travis, S., Yap, L. M., Hawkey, C., Warren, B., Lazarov, M., Fong, T., et al. (2005). Rdp58 is a novel and potentially effective Oral therapy for ulcerative colitis. Inflamm. Bowel Dis. 11, 713–719. doi: 10.1097/01.MIB.0000172807.26748.16
Valore, E. V., Park, C. H., Quayle, A. J., Wiles, K. R., McCray, P. B., and Ganz, T. (1998). Human beta-defensin-1: an antimicrobial peptide of urogenital tissues. J. Clin. Invest. 101, 1633–1642. doi: 10.1172/JCI1861
van der Does, A. M., Hiemstra, P. S., and Mookherjee, N. (2019). “Antimicrobial host Defence peptides: immunomodulatory functions and translational prospects” in Antimicrobial Peptides. ed. K. Matsuzaki, vol. 1117 (Singapore: Springer Singapore), 149–171.
van Groenendael, R., Beunders, R., Hofland, J., Morshuis, W. J., Kox, M., van Eijk, L. T., et al. (2019). The safety, tolerability, and effects on the systemic inflammatory response and renal function of the human chorionic gonadotropin hormone-derivative EA-230 following on-pump cardiac surgery (the EASI study): protocol for a randomized, double-blind, placebo-controlled phase 2 study. JMIR Res. Protocols 8:e11441. doi: 10.2196/11441
van Hoek, M. (2014). Antimicrobial Peptides in Reptiles. Pharmaceuticals 7, 723–753. doi: 10.3390/ph7060723
von Köckritz-Blickwede, M., Goldmann, O., Thulin, P., Heinemann, K., Norrby-Teglund, A., Rohde, M., et al. (2008). Phagocytosis-independent antimicrobial activity of mast cells by means of extracellular trap formation. Blood 111, 3070–3080. doi: 10.1182/blood-2007-07-104018
Waghu, F. H., Barai, R. S., Gurung, P., and Idicula-Thomas, S. (2016). CAMP R3: A database on sequences, structures and signatures of antimicrobial peptides. Nucleic Acids Res. 44, D1094–D1097. doi: 10.1093/nar/gkv1051
Wang, G. (2014). Human antimicrobial peptides and proteins. Pharmaceuticals 7, 545–594. doi: 10.3390/ph7050545
Wang, F., Qiao, L., Lv, X., Trivett, A., Yang, R., Oppenheim, J. J., et al. (2016). Alarmin human α defensin HNP1 activates plasmacytoid dendritic cells by triggering NF-κB and IRF1 signaling pathways. Cytokine 83, 53–60. doi: 10.1016/j.cyto.2016.03.015
Wei, L., Gao, J., Zhang, S., Wu, S., Xie, Z., Ling, G., et al. (2015). Identification and characterization of the first cathelicidin from sea snakes with potent antimicrobial and anti-inflammatory activity and special mechanism. J. Biol. Chem. 290, 16633–16652. doi: 10.1074/jbc.M115.642645
Wu, Z., Hoover, D. M., Yang, D., Boulègue, C., Santamaria, F., Oppenheim, J. J., et al. (2003). Engineering disulfide bridges to dissect antimicrobial and chemotactic activities of human β-defensin 3. Proc. Natl. Acad. Sci. 100, 8880–8885. doi: 10.1073/pnas.1533186100
Xiao, Y., Cai, Y., Bommineni, Y. R., Fernando, S. C., Prakash, O., Gilliland, S. E., et al. (2006a). Identification and functional characterization of three chicken Cathelicidins with potent antimicrobial activity. J. Biol. Chem. 281, 2858–2867. doi: 10.1074/jbc.M507180200
Xiao, Y., Dai, H., Bommineni, Y. R., Soulages, J. L., Gong, Y.-X., Prakash, O., et al. (2006b). Structure-activity relationships of fowlicidin-1, a cathelicidin antimicrobial peptide in chicken. FEBS J. 273, 2581–2593. doi: 10.1111/j.1742-4658.2006.05261.x
Xu, D., and Lu, W. (2020). Defensins: A double-edged sword in host immunity. Front. Immunol. 11:764. doi: 10.3389/fimmu.2020.00764
Xuan, J., Feng, W., Wang, J., Wang, R., Zhang, B., Bo, L., et al. (2023). Antimicrobial peptides for combating drug-resistant bacterial infections. Drug Resist. Updat. 68:100954. doi: 10.1016/j.drup.2023.100954
Yang, D., Chertov, O., Bykovskaia, S. N., Chen, Q., Buffo, M. J., Shogan, J., et al. (1999). β-Defensins: linking innate and adaptive immunity through dendritic and T cell CCR6. Science 286, 525–528. doi: 10.1126/science.286.5439.525
Yang, Y., Jiang, Y., Yin, Q., Liang, H., and She, R. (2010). Chicken intestine defensins activated murine peripheral blood mononuclear cells through the TLR4-NF-κB pathway. Vet. Immunol. Immunopathol. 133, 59–65. doi: 10.1016/j.vetimm.2009.07.008
Yu, J., Mookherjee, N., Wee, K., Bowdish, D. M. E., Pistolic, J., Li, Y., et al. (2007). Host defense peptide LL-37, in synergy with inflammatory mediator IL-1β, augments immune responses by multiple pathways. J. Immunol. 179, 7684–7691. doi: 10.4049/jimmunol.179.11.7684
Zasloff, M. (1987). Magainins, a class of antimicrobial peptides from Xenopus skin: isolation, characterization of two active forms, and partial cDNA sequence of a precursor. Proc. Natl. Acad. Sci. 84, 5449–5453. doi: 10.1073/pnas.84.15.5449
Zhao, H., Gan, T.-X., Liu, X.-D., Jin, Y., Lee, W.-H., Shen, J.-H., et al. (2008). Identification and characterization of novel reptile cathelicidins from elapid snakes. Peptides 29, 1685–1691. doi: 10.1016/j.peptides.2008.06.008
Keywords: host defense peptides, eukaryotes, HDPs, anti-inflammatory activity, immune response, MDR
Citation: Chatterjee D and Sivashanmugam K (2024) Immunomodulatory peptides: new therapeutic horizons for emerging and re-emerging infectious diseases. Front. Microbiol. 15:1505571. doi: 10.3389/fmicb.2024.1505571
Edited by:
Amanda Claire Brown, Tarleton State University, United StatesReviewed by:
Vivian Angelica Salazar Montoya, Catalan Institute of Nanoscience and Nanotechnology (CIN2), SpainSujeet Kumar, The Ohio State University, United States
Copyright © 2024 Chatterjee and Sivashanmugam. This is an open-access article distributed under the terms of the Creative Commons Attribution License (CC BY). The use, distribution or reproduction in other forums is permitted, provided the original author(s) and the copyright owner(s) are credited and that the original publication in this journal is cited, in accordance with accepted academic practice. No use, distribution or reproduction is permitted which does not comply with these terms.
*Correspondence: Karthikeyan Sivashanmugam, c2l2YS5rYXJ0aGlrZXlhbkB2aXQuYWMuaW4=
†ORCID: Karthikeyan Sivashanmugam, https://orcid.org/0000-0002-9692-8633