- 1Avian Disease Research Center, College of Veterinary Medicine, Sichuan Agricultural University, Chengdu, China
- 2Institute of Veterinary Medicine and Immunology, Sichuan Agricultural University, Chengdu, China
- 3Key Laboratory of Animal Disease and Human Health of Sichuan Province, Sichuan Agricultural University, Chengdu, China
- 4Engineering Research Center of Southwest Animal Disease Prevention and Control Technology, Ministry of Education of the P.R. China, Chengdu, China
Escherichia coli (E. coli) serves as a critical indicator microorganism for assessing the prevalence and dissemination of antibiotic resistance, notably harboring various antibiotic-resistant genes (ARGs). Among these, the emergence of the blaNDM gene represents a significant threat to public health, especially since carbapenem antibiotics are vital for treating severe infections caused by Gram-negative bacteria. This study aimed to characterize the antibiotic resistance features of blaNDM-5-positive E. coli strains isolated from waterfowl in several regions of China and elucidate the dissemination patterns of the blaNDM-5 gene. We successfully isolated 103 blaNDM-5-positive E. coli strains from 431 intestinal fecal samples obtained from waterfowl across five provincial-level units in China, with all strains exhibiting multidrug resistance (MDR). Notably, the blaNDM-5 gene was identified on plasmids, which facilitate efficient and stable horizontal gene transfer (HGT). Our adaptability assays indicated that while the blaNDM-5-positive plasmid imposed a fitness cost on the host bacteria, the NDM-5 protein was successfully induced and purified, exhibiting significant enzymatic activity. One strain, designated DY51, exhibited a minimum inhibitory concentration (MIC) for imipenem of 4 mg/L, which escalated to 512 mg/L following exposure to increasing imipenem doses. This altered strain demonstrated stable resistance to imipenem alongside improved adaptability, correlating with elevated relative expression levels of the blaNDM-5 and overexpression of efflux pumps. Collectively, this study highlights the horizontal dissemination of the blaNDM-5 plasmid among E. coli strains, confirms the associated fitness costs, and provides insights into the mechanisms underlying the stable increase in antibiotic resistance to imipenem. These findings offer a theoretical framework for understanding the dissemination dynamics of blaNDM-5 in E. coli, which is essential for developing effective strategies to combat carbapenem antibiotic resistance.
Introduction
The discovery and use of antimicrobial agents have significantly advanced human public health, animal welfare, and agriculture (Endale et al., 2023). However, the rapid growth of antimicrobial resistance (AMR) and the emergence of “superbugs” pose significant challenges to global public health (Mitra et al., 2022; Collineau et al., 2023). In intensive farming operations, antimicrobial agents are often employed to prevent and treat animal diseases, which can lead to the presence of residual antibiotics in the environment (Wang X. R. et al., 2021; Tang et al., 2022). Consequently, antibiotic-resistant bacteria frequently withstand the pressure of antimicrobial agents through their acquired ARGs, which are capable of HGT (Christaki et al., 2020). Escherichia coli is a common Gram-negative bacterium, typically found as a symbiotic inhabitant in the intestines of humans and animals, and it can be frequently detected in the environment (Arbab et al., 2022). Pathogenic strains of E. coli can cause various diseases, such as urinary tract infections, bacteremia, cystitis, and others (Croxen et al., 2013). As a result, E. coli is often used as an indicator for monitoring antibiotic resistance and is also regarded as a reservoir for ARGs, which can be horizontally transferred to other bacteria, posing a serious threat to public health and safety (Zhang et al., 2020; Zhang et al., 2021a; Li Q. et al., 2024).
Compared to other antibiotics, carbapenem antibiotics exhibit excellent antibacterial activity, a broad spectrum of coverage, and enhanced stability against β-lactamases. They are commonly used to treat severe bacterial infections and are thus considered “a class of last-resort antibiotics” (Ma et al., 2023). However, the frequent use of carbapenem antibiotics has led to an increase in infections caused by carbapenem-resistant Enterobacterales (CRE), which has become one of the three most significant challenges in the global fight against infections. Although carbapenem antibiotics are banned for use in animals in China, the incidence of CRE isolated from animal sources continues to rise (Poirel et al., 2018).
Since the discovery of the first patient carrying the blaNDM-1 gene in India in 2008 (Yong et al., 2009), 41 variants of blaNDM have been identified. Currently, the most prevalent types are blaNDM-1 and blaNDM-5; notably, blaNDM-5 is commonly found in animals in China. Various studies have detected blaNDM-5-positive E. coli in livestock, poultry, and the surrounding environment of farms in China (He et al., 2017; Wang M. G. et al., 2021; He et al., 2023). It is evident that blaNDM-5-producing CRE are widespread in China, particularly among livestock and poultry, where blaNDM-5 appears to be the predominant variant. At present, carbapenem antibiotics are approved solely for clinical use, but the presence of blaNDM has been observed in animals, the environment, and humans. The spread of blaNDM-5 is primarily facilitated by plasmids, which play a crucial role in the evolution of bacterial antibiotic resistance. Plasmids contribute to the spread of ARGs through HGT, creating conditions conducive to the emergence of “superbugs” (Lerminiaux and Cameron, 2019; Das, 2023; Pu et al., 2023).
Frequent or inappropriate use of antibiotics often prompts bacteria to develop resistance, which can increase the risk of MDR bacteria (Chen et al., 2024; Guan et al., 2024). One of the most evident changes is the alteration of the MIC value. In addition, bacteria may exhibit multidrug resistance and cross-resistance (Gu et al., 2020; Li et al., 2020; Li et al., 2022), further enhancing their survival capabilities in antibiotic-rich environments. These changes are coping strategies employed by bacteria in response to environmental pressures and are of significant importance in studying the mechanisms of bacterial resistance.
In this study, 103 MDR blaNDM-5-positive E. coli strains were isolated and identified from waterfowl source samples in select regions of China. Furthermore, one blaNDM-5-positive E. coli strain (DY51) was observed to be inducible from low-level resistance (MIC = 4 mg/L) to high-level resistance (MIC = 512 mg/L). Studying the molecular transmission characteristics of blaNDM-5 can enhance our understanding of its transmission pathways in the environment and its implications for animals and humans, laying a foundation for further research on the specific resistance mechanisms of blaNDM-5. This study not only deepens our understanding of carbapenem antibiotic resistance in blaNDM-5-positive E. coli strains derived from waterfowl but also provides new insights and methods for preventing, controlling, and treating antibiotic resistance.
Materials and methods
Study design, isolation, and identification of positive blaNDM-5 Escherichia coli
China is a major producer of waterfowl. To investigate the prevalence of blaNDM-5 carried by bacteria in waterfowl, our research team established close contact with numerous domestic farms from 2021 to 2023. This collaboration enabled us to collect a significant number of disease samples from the Sichuan, Shaanxi, Chongqing, Xinjiang, and Anhui regions, which are provincial-level administrative units in China. A total of 431 carcasses of diseased ducks were sent by farmers to the laboratory under cold conditions for testing. We obtained intestinal fecal contents by dissecting the carcasses in a sterile laboratory to ensure that the isolated bacteria accurately represent the local situation without contamination from various environmental factors. The intestinal content samples were initially inoculated in Luria-Bertani (LB) broth (HOPEBIO, Qingdao, China, #HB0128) at 37°C for enrichment culture, followed by inoculation onto MacConkey agar (HOPEBIO, Qingdao, China, #HB6238) containing 4 mg/L of imipenem (Meilun Biotechnology Co., Ltd., Dalian). Pink colonies were subjected to further identification using 16S rRNA sequencing with universal primers. The identified E. coli isolates were preserved in LB broth supplemented with 30% glycerol and stored at −80°C for subsequent analyses. Imipenem-resistant strains were selected and subjected to PCR amplification and sequencing. Universal 16S rRNA and blaNDM-5 identification primers were used to identify blaNDM-5-positive E. coli (Hornsey et al., 2011; Zhang et al., 2021b).
Carbapenemase phenotype screening
The modified Hodge test (MHT), modified carbapenem inactivation test (mCIM), and EDTA-modified carbapenem inactivation test (eCIM) for all blaNDM-5-positive E. coli isolates were performed according to the standards of the American Clinical and Laboratory Standards Institute (CLSI) (Humphries et al., 2021). Isolates were resuspended in 1.5 mL of LB and then treated with 2 mL of LB containing 5 mM EDTA (15 μL of 0.5 M). Meropenem tablets (Oxoid Ltd., London, England, # CT0774B) were incubated at 35°C for 4 h ± 15 min and then placed on MH agar (Hope Bio-Technology Co., Ltd., Qingdao, #HB6231) inoculated with carbapenem-sensitive E. coli ATCC 25922. After 16–20 h of incubation, the mCIM results were interpreted as follows: an inhibition zone of ≥19 mm = negative; 6–15 mm = positive; and 16–18 mm with a tip-sized colony = intermediate (positive). A ≥5 mm increase in the eCIM inhibition zone compared to the mCIM indicates a positive result for metallo-carbapenemase; an increase of <4 mm indicates a negative result for metallo-carbapenemase. Escherichia coli ATCC 25922 served as the control. All experiments were conducted in triplicate.
DNA extraction and detection of antibiotic sensitivity tests
DNA extraction was performed using the heating extraction method as previously described (Zhang et al., 2021b), and the extracted DNA was stored at −80°C. All isolates were assessed for antibiotic sensitivity to 11 antibiotics, following the methodology recommended by CLSI. In brief, bacterial cultures were prepared and dispensed into sterile 96-well polystyrene plates at a volume of 200 μL per well. The concentrations of antibiotics in wells 1 to 11 were 512, 256, 128, 64, 32, 16, 8, 4, 2, 1, and 0.5 mg/L, respectively. The 12th well served as a blank control containing Mueller-Hinton medium without antibiotics. The 11 antibiotics tested included imipenem (IMP, #S24020), tetracycline (TE, #S17051), norfloxacin (NFX, #S17080) (Yuanye Biotechnology Co., Ltd., Shanghai), azithromycin (AZM, #MB1024), kanamycin (KAN, #MB1130), trimethoprim (SXT, #MB1317), polymyxin B (PB, #MB1188) (Meilun Biotechnology Co., Ltd., Dalian), chloramphenicol (C, #IC0320) (Solarbio Science & Technology Co., Ltd., Beijing), aztreonam (ATM, #A801653), cefotaxime (CTX, #C804340), and ampicillin (AMP, #A830931) (Macklin Biochemical Technology Co., Ltd., Shanghai). Escherichia coli ATCC25922 was utilized as the negative control strain.
Screening for ARGs in Escherichia coli isolates
Based on the MIC results, it was necessary to detect the presence of ARGs in the isolates. PCR was used to detect ARGs associated with different classes of antibiotics, including β-lactams (blaTEM, blaSHV, blaCTX-M, blaNDM, blaKPC), quinolones (qnrA, qnrB, and qnrS), aminoglycosides [aac(6′)-Ib-cr, aadA1, and aac(3)-I], sulfonamides (sul1, sul2, and sul3), tetracyclines (tetA, tetB, tetC, and tetM), glucocorticoids (floR, cat1), macrolides (ermA, ermB), and polymyxin (mcr-1). The primers used for PCR are detailed in Supplementary Table S1. All positive PCR products were sent to Tsingke Biotechnology Co., Ltd. for DNA sequencing. The resulting sequences were analyzed using the BLAST tool available at the online gene database.1
Conjugational transfer and conjugational frequency
We used a blaNDM-5-positive E. coli strain as the donor and NaN3-resistant E. coli J53 as the recipient. The donor and recipient bacteria were cultured on LB agar plates containing 4 mg/L IMP, 150 mg/L NaN3, and 4 mg/L IMP + 150 mg/L NaN3, respectively. After incubating for 18 h in a constant temperature incubator at 37°C, we observed the results. Both the donor and recipient bacteria were combined in a new tube at a ratio of 1:4 and incubated at 37°C for 24 h, followed by a 10-fold dilution in sterile saline to achieve the appropriate concentration. A volume of 100 μL of the mixed bacterial solution was then plated on LB agar containing 4 mg/L IMP + 150 mg/L NaN3 and incubated at 37°C for 24 h. Single colonies from the 4 mg/L IMP + 150 mg/L NaN3 LB agar were selected for blaNDM-5 detection, and blaNDM-5-positive strains were identified as transconjugants.
For testing conjugational frequency, both donor and recipient bacteria were activated and diluted to an OD600 of 0.5. They were then mixed in a new tube at a ratio of 1:1 and incubated at 37°C for 24 h, followed by a 10-fold dilution with sterile saline to reach the desired concentration. Subsequently, 100 μL of the mixed bacterial solution was plated on LB agar containing 4 mg/L IMP + 150 mg/L NaN3, while 100 μL of E. coli J53 was spread on LB agar with 150 mg/L NaN3. Both were incubated at 37°C for 24 h. Single colonies on the 4 mg/L IMP +150 mg/L NaN3 agar were counted as transconjugants (designated as T), while single colonies on the 150 mg/L NaN3 agar were counted as receptors (designated as R). The conjugation frequency was calculated as T/R. All experiments were conducted in triplicate.
Plasmid replicon typing
The PCR-based replicon typing (PBRT) technique was used to detect plasmid incompatibility groups in transconjugants, as previously described with specific primers (Carattoli et al., 2005; Johnson et al., 2007).
S1-PFGE
Salmonella H9812 served as the reference strain for molecular quality standards. For the analyses, the blaNDM-5-positive strains were embedded in 1% SeaKem Gold Agarose (Lonza Group Co., Ltd.) and subjected to enzymatic digestion using S1 nuclease (Takara Biomedical Technology Co., Ltd., Beijing) at 37°C for 4 h. Electrophoresis was subsequently performed using 1% agarose gel under specific conditions: an operating voltage of 6 V/cm and a pulse time ranging from 6.76 s to 35.38 s, utilizing the CHEF DR III system for a total duration of 18 h at 14°C.
Impact of imipenem on the stability of a plasmid harboring blaNDM-5
The transconjugants were cultured in LB broth with 4 mg/L of imipenem (IMP) added continuously, with transfers to fresh medium every 12 h. Stability was measured every 24 h. Bacteria were diluted 10-fold to the appropriate concentration using sterile saline, and 100 μL of the diluted bacteria was applied to LB agar containing 4 mg/L of IMP, as well as to regular LB agar. The plates were then incubated in a constant temperature incubator at 37°C for 18 h. The number of single colonies on the LB agar with 4 mg/L of IMP indicated the number of blaNDM-5-positive strains, while the number of single colonies on regular LB agar represented the total number of strains. The genetic stability of the blaNDM-5-positive plasmid was calculated as the ratio of blaNDM-5-positive strains to the total number of strains. All studies were performed in triplicate.
Construction of the pET32a (+)-blaNDM-5 plasmid
To amplify the complete blaNDM-5 sequence, primers blaNDM-5-F (5′-CGCCATATGGCGATGGAATTGCCCAAT-3′) and blaNDM-5-R (5′-CGCGGATCCGCGTCAATGATGATGATGAGATGGCGCAGCTTGTC-3′) were employed, incorporating Nde I and BamH I restriction sites at their respective termini. The amplification reaction mixture had a total volume of 20 μL and included 10 μL of 2 × Taq PCR Green Mix, 1 μL of each primer (blaNDM-5-F and blaNDM-5-R), 2 μL of DNA, and 6 μL of ddH2O. The PCR cycling conditions were as follows: initial denaturation at 95°C for 15 s, annealing at 55°C for 15 s, and extension at 65°C for 1 min, for a total of 32 cycles. The PCR products were analyzed using 1% agarose gel electrophoresis (120 V for 20 min) and purified using a PCR purification kit (Tiangen Biotech Co., Ltd., Beijing). A digestion reaction was performed with 5 μL of the purified PCR product (blaNDM-5) and the pET32a(+) vector containing 6 × His. The ligation was allowed to proceed overnight at 4°C. After sequencing the recombinant plasmid and confirming its accuracy through BLAST alignment, the plasmid was transformed into E. coli-BL21(DE3) and E. coli DH5α.
Expression of the NDM-5 protein and activity detection
Escherichia coli-BL21(DE3)-pET32a(+)-blaNDM-5 was cultured with an optical density (OD600) of 0.6, and 0.5 M isopropyl-β-D-thiogalactoside (IPTG) (Solarbio Science & Technology Co., Ltd., Beijing) was added for induction at 16°C overnight. The bacteria were then collected and centrifuged. The resulting pellet was resuspended in 0.05 M Tris–HCl solution and disrupted using ice bath ultrasound. Subsequently, purification was performed according to the Ni-NTA Beads 6FF kit (Changzhou Smart-Lifesciences Biotechnology Co., Ltd.). The purified samples were then analyzed using sodium dodecyl sulfate-polyacrylamide gel electrophoresis (SDS-PAGE) (Li L. et al., 2024).
To evaluate the changes in MIC values of the tested strains against imipenem in the presence of NDM-5 protein, a reaction system was prepared consisting of 30 μg/mL of total protein and 11 concentrations of imipenem (512 mg/L, 256 mg/L, 128 mg/L, 64 mg/L, 32 mg/L, 16 mg/L, 8 mg/L, 4 mg/L, 2 mg/L, 1 mg/L, and 0.5 mg/L). The reaction was incubated at 37°C in triplicate alongside a negative control group that lacked protein. The changes in OD600 of the strains were measured using a microplate reader every 4 h over a total period of 24 h (at 4 h, 8 h, 12 h, 16 h, 20 h, and 24 h) to determine alterations in MIC values against imipenem.
Fitness cost of blaNDM-5-positive plasmids
To investigate the fitness associated with blaNDM-5-positive plasmids, we employed a combination of growth curve analyses, biofilm formation assessments, and in vitro competition assays. These experiments utilized the conjugants E. coli DH5α-pET32a(+)-blaNDM-5 and E. coli DH5α-pET32a(+), following previously described methods (Lu et al., 2021; Huo et al., 2024). All experiments were conducted in triplicate.
Exposure to varying doses of imipenem
A low-level imipenem-resistant strain carrying blaNDM-5 was selected and cultured to an OD600 of 1. Based on the MIC results for imipenem, a concentration of 1 × MIC was chosen for induction on the first day. The concentration of imipenem was doubled daily, following the sequence: 1 × MIC, 2 × MIC, 4 × MIC, 8 × MIC, and so on. Each day, 500 μL of the culture was transferred to 20 mL of LB broth supplemented with the corresponding concentration of imipenem, and the culture was incubated at 37°C for 24 h until no further growth was observed.
Real-time PCR (qRT-PCR)
The expression of blaNDM-5 in high-level resistant strains after induction was assessed using qRT-PCR. The fluorescent quantitative primers for blaNDM-5 were selected based on a previous study (Zhao et al., 2023). The TAKARA RNAiso Easy Kit (Takara Biomedical Technology Co., Ltd., Beijing) was used to isolate mRNA from imipenem-exposed strains, and the Hifair ® II 1st Strand cDNA Synthesis SuperMix Kit (Yeasen Biotechnology Co., Ltd., Shanghai) was used for reverse transcription to synthesize cDNA. The expression levels of blaNDM-5 were quantified using cDNA as a template, with the 16S rRNA gene serving as the internal reference. The results were expressed as the ratio of the expression levels of the blaNDM-5 target gene compared to the internal reference gene (Zhao et al., 2023).
MIC determination of antibiotic-resistant strains post-induction with the addition of efflux pump inhibitors
The MIC of imipenem was determined using the broth microdilution method, with 5 mg/L of carbonyl cyanide m-chlorophenylhydrazine (CCCP) (Solarbio Science & Technology Co., Ltd., Beijing) as an efflux pump inhibitor. This method followed the same protocol as the antibiotic sensitivity test.
Fitness cost and stability of induced strains
To study the adaptive cost and stability of the altered strains, a combination of growth curve analysis, in vitro competition tests, and stability assessments was employed. The experiment utilized strains both before and after induction, based on previous references (Lu et al., 2021; Huo et al., 2024). All experiments were conducted in triplicate.
Statistical analysis
Statistical analyses were performed using SPSS 27.0 software. Pearson’s chi-square test and Fisher’s exact test were utilized to compare the antimicrobial resistance of 103 E. coli isolates and evaluate the correlation among different ARGs, or between AMR phenotypes and their corresponding ARGs. Odds ratios (OR) and their 95% confidence intervals were calculated, with OR < 1 indicating a negative correlation and OR > 1 indicating a positive correlation. A p value of <0.05 was considered statistically significant.
Results
Isolation and identification of blaNDM-5-positive Escherichia coli from waterfowl sources
Upon transporting ducks from selected areas to the laboratory, researchers collected intestinal contents in a clean and disinfected animal facility to ensure non-repetitive samples of intestinal feces from each duck. A total of 431 intestinal fecal samples were collected from each duck. From a total of 431 intestinal feces collected from waterfowl sources in Sichuan, Anhui, Shaanxi, Xinjiang, and Chongqing, 103 strains were identified as blaNDM-5-positive E. coli, resulting in a positive rate of 30.5%. The majority of blaNDM-5-positive E. coli were isolated from Sichuan Province, specifically from areas such as Deyang, Meishan, Xinjin, Chongzhou, Jintang, Suining, Dayi, and Leshan. Overall, with the exception of Shaanxi Province, the farms from which the positive bacterial strain samples were collected are located between 20 and 300 km from our laboratory. Specific details are provided in Table 1.
Results of carbapenemase phenotype screening
All blaNDM-5-positive E. coli strains tested positive in the MHT, mCIM, and eCIM tests. The results of the MHT are presented in Supplementary Figure S1, while the mCIM and eCIM results are shown in Supplementary Table S2 and Supplementary Figure S2. Specific values can be found in the Supplementary Material.
Analysis of ARGs in blaNDM-5-positive Escherichia coli strains
The detection of ARGs in 103 blaNDM-5-positive E. coli strains is presented in Figure 1. Among these, the chloramphenicol resistance gene floR had the highest detection rate (n = 91, 88.3%), followed by the β-lactam resistance gene blaCTX-M (n = 90, 87.4%), the tetracycline resistance gene tetA (n = 87, 84.5%), the sulfonamide resistance gene sul2 (n = 84, 81.6%), the aminoglycoside resistance gene aadA1 (n = 72, 69.9%), and the sulfonamide resistance gene sul1 (n = 58, 56.3%). The detection rates of the other ARGs were less than 50%.
The analysis results indicate that there are significant correlations, primarily positive, among the ARGs carried by the 103 blaNDM-5-positive E. coli strains. As shown in Supplementary Table S3, a total of 45 pairs of ARGs exhibited significant positive correlations, while 23 pairs showed significant negative correlations. Among these, tetB has the strongest positive correlation with sul3 or ermB (OR, 101.000; 95% CI, 3.370–3027.447). In terms of negative correlations, sul2 and tetC displayed the strongest negative correlation (OR, 0.030; 95% CI, 0.006–0.156). Detailed analyses of the other correlation results are presented in Supplementary Table S3.
Antimicrobial susceptibility analysis
Except for strain DY51, all strains exhibited high-level resistance to imipenem, with MIC values ranging from 64 to ≥512 mg/L (Supplementary Table S4). The resistance rates for the remaining 10 antibiotics were as follows: AMP (n = 103, 100%), CTX (n = 102, 99.0%), and NFX (n = 100, 97.1%) showed the highest resistance rates, followed by SXT (n = 92, 89.3%), KAN (n = 92, 89.3%), and C (n = 92, 89.3%). Finally, the resistance rates for AZM (n = 28, 27.2%) and PB (n = 16, 15.5%) were relatively low.
These results indicate that all 103 blaNDM-5-positive E. coli strains are MDR, with resistance levels ranging from 3 to 8 antibiotics, primarily six antibiotics (n = 57, 55.3%). The specific antimicrobial resistance profiles are presented in Table 2.
The correlation analysis between resistance genes and AMR phenotypes revealed significant correlations between the resistance genes and AMR in the 103 blaNDM-5-positive E. coli strains from waterfowl, primarily negative correlations. As shown in Supplementary Table S5, a total of seven pairs of genes exhibited significant positive correlations, while nine pairs showed significant negative correlations. Among these, KAN and aadA1 exhibited the strongest positive correlation (OR, 13.696; 95% CI, 2.757–68.036), while NFX and emrB displayed the strongest negative correlation (OR, 0.020; 95% CI, 0.001–0.446). Other analyses are detailed in Supplementary Table S5.
Analysis of plasmid-mediated horizontal transfer of blaNDM-5
Fifty-three blaNDM-5-positive E. coli strains were able to transfer imipenem resistance to the recipient strain J53, with the MIC of the recipient strain increasing from 0.5 mg/L to either 4 mg/L or 512 mg/L. PCR and DNA sequencing confirmed that all 53 transconjugants were blaNDM-5-positive. By counting the number of colonies of the 53 blaNDM-5-positive transconjugants on double-resistant plates and the colonies of E. coli J53 on NaN3-resistant plates, the range of conjugative transfer frequency was calculated to be 5.22 × 10−7 to 9.48 × 10−4 (Figure 2). The plasmid profiles of randomly selected donor and recipient bacteria were analyzed using S1-PFGE (Supplementary Figure S3). The donor bacteria carried 3–4 plasmids, while the recipient bacteria carried 1 plasmid, indicating that blaNDM-5 was located on a mobile plasmid. Furthermore, replicon typing of all transconjugants was performed via PCR and sequencing; the results indicated that the 53 blaNDM-5-positive transconjugants harbored 13 different replicon types, namely, IncX1, IncX3, HI2, I1, N, FIA, FIB, Y, P, FIC, FIIS, K, and B/O. Notably, IncX1 (28.3%) was the dominant type, followed by HI2 (26.4%) and K (15.1%). The plasmid profiles of randomly selected donor and recipient bacteria were shown by S1-PFGE (Supplementary Figure S3). The donor bacteria carried 3–4 plasmids, while most recipient bacteria carried one plasmid, indicating that blaNDM-5 was located on a mobile plasmid.
The impact of imipenem on the stability of blaNDM-5-positive plasmids
To investigate whether the blaNDM-5-positive plasmids acquired by the transconjugants possess stable genetic characteristics, this study conducted a plasmid stability test on 53 strains carrying blaNDM-5-conjugative plasmids. During the experiment, the genetic stability of these plasmids was compared under conditions of both imipenem selection pressure and in its absence, as illustrated in Figure 3. In the absence of antibiotic selection pressure, the plasmid stability of the 53 transconjugants showed a slight decline with increasing passage numbers, while the plasmid stability rate remained above 75%. Under a selection pressure of 4 mg/L imipenem, the plasmid stability rate of the 53 transconjugants was even higher than that observed without antibiotics, maintaining above 92%, indicating stronger stability. This suggests that the stable presence of blaNDM-5 conjugative positive plasmids is influenced not only by the stable genetic characteristics of the gene itself but also by the presence of antibiotics in the environment. These findings imply that residual antibiotics in the environment can contribute to the stable presence of blaNDM-5-positive plasmids in resistant strains, thereby enhancing the dissemination of antibiotic resistance.
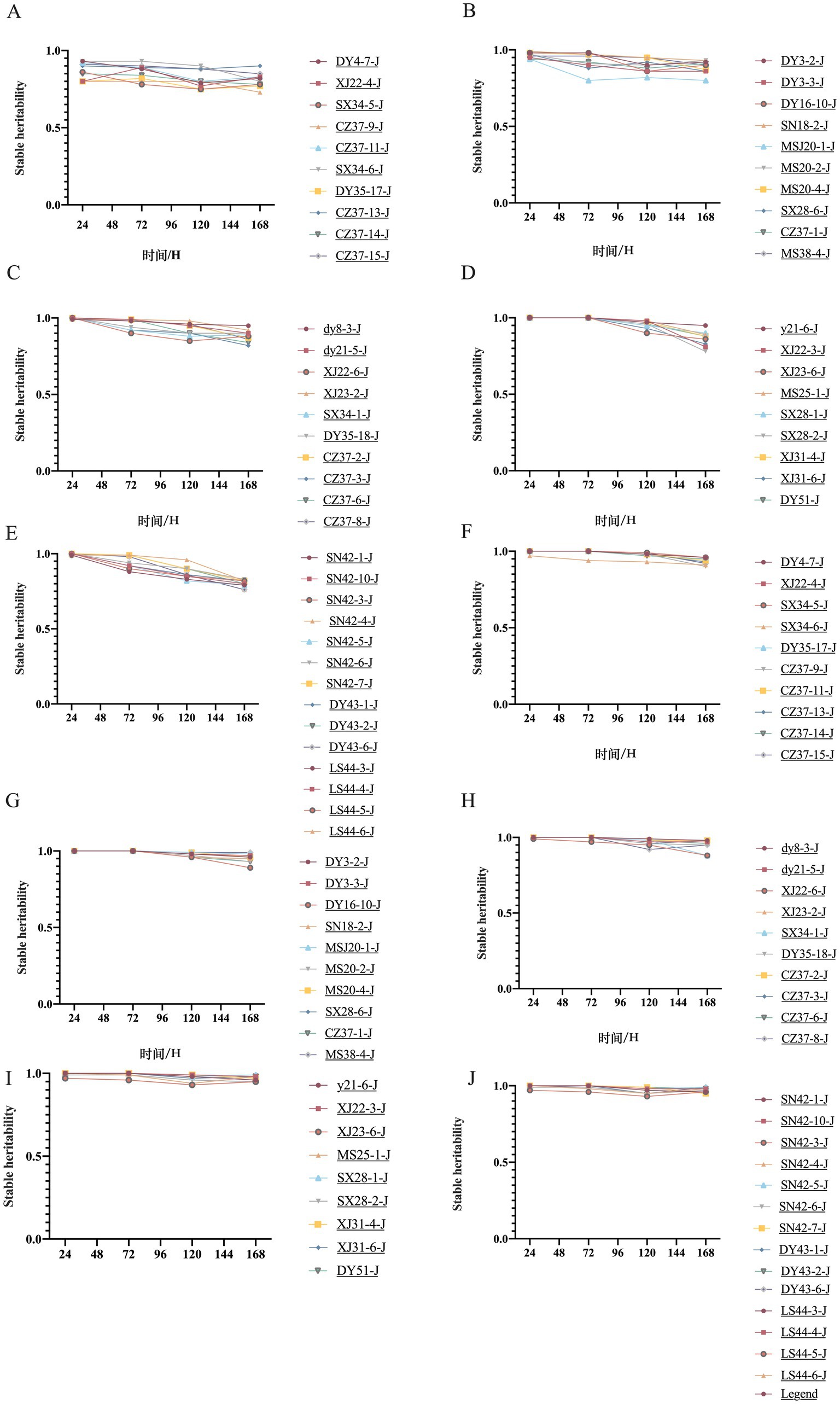
Figure 3. Plasmid genetic stability of 53 transconjugants with or without imipenem selection pressure.
Construction results of the pET32a (+)-blaNDM-5 recombinant plasmid
Full-length amplification of blaNDM-5 was performed on the transformant E. coli DH5α carrying the pET32a(+)-blaNDM-5 plasmid, and the electrophoresis gel results are presented in Supplementary Figure S4. The band size is approximately 813 bp, which is consistent with the expected size. The amplification product was sequenced, and the sequencing results were subjected to BLAST alignment, confirming the successful construction of the recombinant plasmid.
Expression and activity detection of NDM-5 protein
Total protein was extracted from the modified E. coli-BL21(DE3) strain harboring the pET32a(+)-blaNDM-5 plasmid. The blaNDM-5 protein was subsequently purified using nickel column affinity chromatography. After SDS-PAGE analysis, a clear protein band was observed at the 28.5 kDa position, as shown in Supplementary Figure S5, aligning with the expected size of the NDM-5 protein.
Initially, the concentration of the purified NDM-5 protein was determined. Based on this concentration, the activity of the purified NDM-5 protein was assayed. Table 3 and Figure 4 described the changes in the MIC values of imipenem against the low-level resistant strain DY51 in the presence of NDM-5 protein. Due to the hydrolytic activity of the NDM-5 protein on imipenem, the resistance of the low-level resistant strain DY51 and the non-blaNDM-5-carrying strain E. coli-BL21(DE3) to imipenem gradually increased, resulting in rising MIC values over time. This clearly demonstrates that the purified NDM-5 protein exhibits significant activity, capable of hydrolyzing imipenem and enabling the strains to survive in the presence of high antibiotic concentrations.
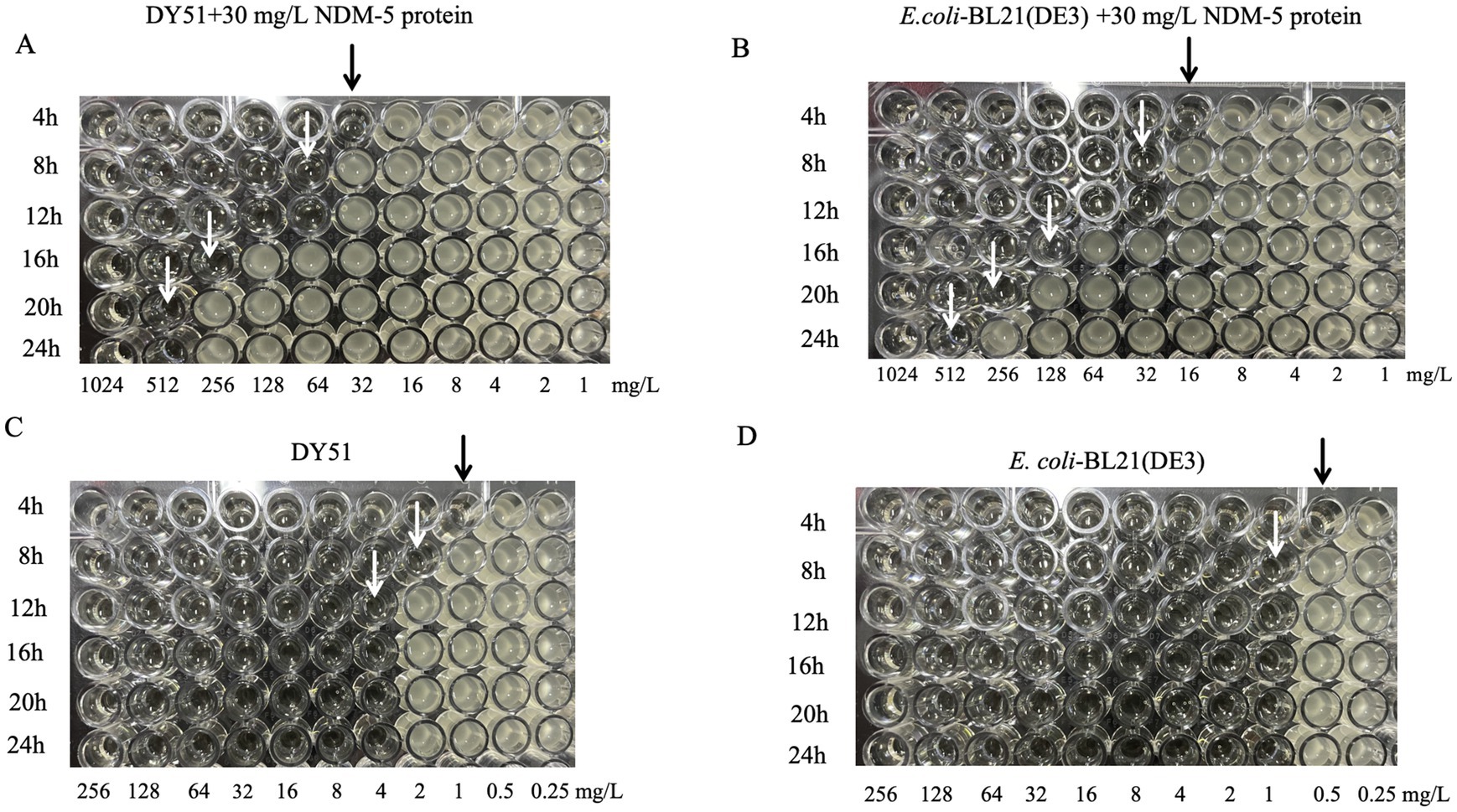
Figure 4. The change of MIC value of imipenem to DY51 and E. coli-BL21(DE3) with or without purified NDM-5 protein. Note: Each well in Figures A and B contains 30mg/L purified NDM-5 protein, while each well in Figures C and D does not contain purified NDM-5 protein.
The impact of blaNDM-5-positive plasmids on recipient bacteria
The OD600 values of 53 transconjugants, the transformant E. coli DH5α-pET32a(+)-blaNDM-5, E. coli DH5α-pET32a(+), and the recipient bacteria E. coli J53 were measured at various time points to plot the changes in growth curves before and after the transfer of blaNDM-5-positive plasmids. The results are presented in Figure 5. Among the 53 transconjugants, 32 of them (DY3-2-J, DY4-7-J, dy8-3-J, SN18-2-J, dy21-5-J, dy21-6-J, XJ22-4-J, XJ22-6-J, XJ23-2-J, XJ23-6-J, MS25-1-J, SX28-1-J, SX28-2-J, SX28-6-J, XJ31-4-J, XJ31-6-J, SX34-1-J, CZ37-2-J, CZ37-3-J, CZ37-6-J, CZ37-8-J, CZ37-11-J, CZ37-13-J, CZ37-14-J, CZ37-15-J, SX34-6-J, MS38-4-J, SN42-1-J, SN42-7-J, DY43-2-J, LS44-4-J, and LS44-5-J) exhibited slower growth rates after acquiring the blaNDM-5-positive plasmid, while the growth rates of the remaining 21 transconjugants (DY3-3-J, MS20-1-J, MS20-2-J, MS20-4-J, XJ22-3-J, SX34-5-J, DY35-17-J, DY35-18-J, CZ37-1-J, CZ37-9-J, SN42-10-J, SN42-3-J, SN42-4-J, SN42-5-J, SN42-6-J, DY43-1-J, DY43-6-J, LS44-3-J, LS44-6-J, DY16-10-J, and DY51-J) were comparable to that of E. coli J53. In the transformant group, the growth rate of E. coli DH5α-pET32a(+)-blaNDM-5, which acquired the blaNDM-5 gene, was slower compared to E. coli DH5α-pET32a(+).
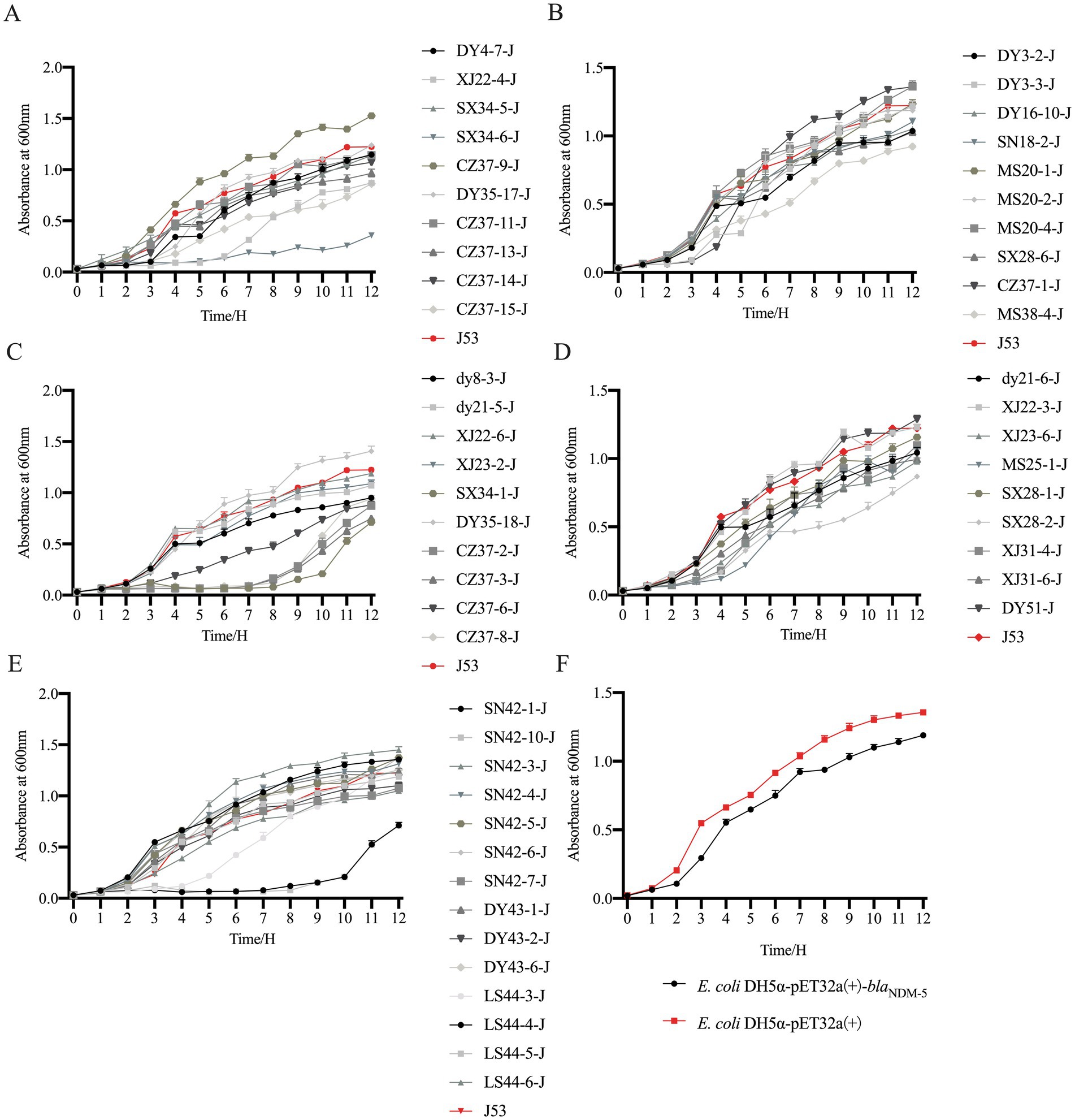
Figure 5. Growth curve of blaNDM-5 transconjugants and transformant strains. Note: Figure A, B, C, D, E are the growth curve of blaNDM-5 transconjugants, and Figure F is the growth curve of blaNDM-5 transformants.
The biofilm formation ability of the 53 blaNDM-5 transconjugants and E. coli J53 was assessed by measuring the OD value at a wavelength of 570 nm. A higher OD570 value indicates stronger biofilm formation ability. The results are displayed in Figure 6. Among the 53 blaNDM-5 transconjugants, 40 transconjugants (XJ22-4-J, SX34-5-J, DY35-17-J, CZ37-11-J, CZ37-13-J, CZ37-14-J, CZ37-15-J, DY3-2-J, DY3-3-J, SN18-2-J, MS20-2-J, MS20-4-J, SX28-6-J, MS38-4-J, dy21-5-J, XJ23-2-J, SX34-1-J, DY35-18-J, CZ37-2-J, CZ37-3-J, CZ37-6-J, dy21-6-J, XJ22-3-J, MS25-1-J, SX28-1-J, XJ31-6-J, SN42-1-J, SN42-10-J, DY51-J, SN42-3-J, SN42-4-J, SN42-5-J, SN42-6-J, SN42-7-J, DY43-1-J, DY43-2-J, DY43-6-J, LS44-3-J, LS44-5-J, and LS44-6-J) demonstrated significantly enhanced biofilm formation ability after acquiring the blaNDM-5 conjugative plasmid. In contrast, 13 transconjugants (DY4-7-J, SX34-6-J, CZ37-9-J, DY16-10-J, MS20-1-J, CZ37-1-J, DY8-3-J, XJ22-6-J, CZ37-8-J, XJ23-6-J, SX28-2-J, XJ31-4-J, and LS44-4-J) showed no significant difference in biofilm formation ability compared to the recipient bacteria E. coli J53 after acquiring the blaNDM-5 plasmid.
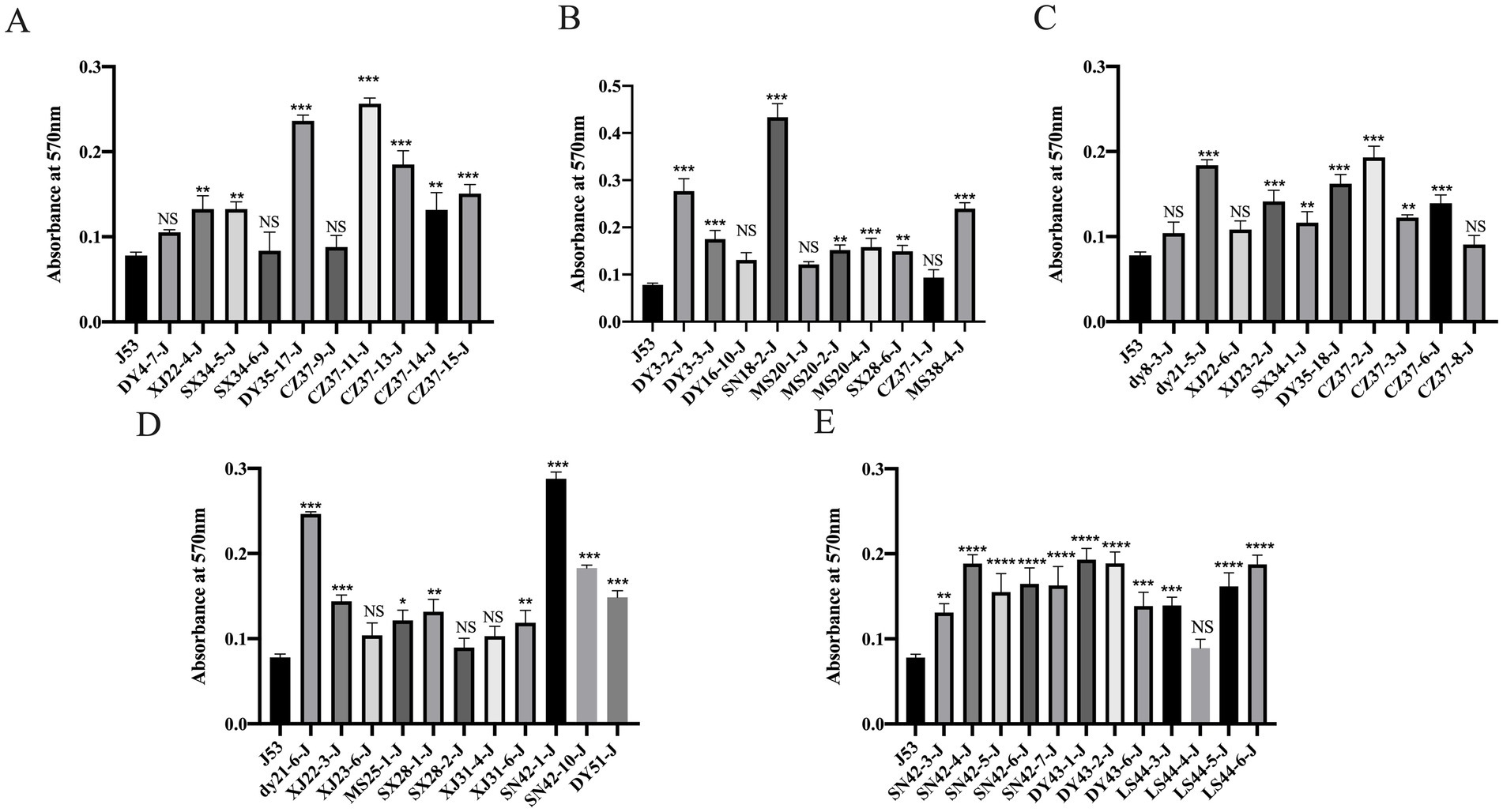
Figure 6. Results of biofilm formation ability of 53 strains of blaNDM-5 transconjugants Note: Figure A, B, C, D and E are the results of biofilm formation ability of different blaNDM-5 transconjugants.
To investigate the effect of blaNDM-5 on the fitness of transformants, an in vitro competition experiment was conducted between E. coli DH5α-pET32a(+)-blaNDM-5 and E. coli DH5α-pET32a(+). As shown in Supplementary Figure S6, the relative fitness (RF) values were less than 1 at 24, 48, and 72 h, indicating that the acquisition of blaNDM-5 imposed a certain fitness cost on the transformants, although this cost was relatively small.
In vitro induction of resistance to imipenem in Escherichia coli
Using MIC testing, this study identified a strain DY51 with low-level resistance to imipenem (MIC = 4 mg/L). Furthermore, the recombinant strain E. coli DH5α-pET32a(+)-blaNDM-5 constructed in this study also exhibited a MIC value of 4 mg/L for imipenem. Based on these results, the low-level resistant strains DY51 and E. coli DH5α-pET32a(+)-blaNDM-5 were selected for an in vitro antibiotic induction experiment with imipenem. After exposure to varying doses of imipenem, two high-level imipenem-resistant strains, namely, DY51-I and E. coli DH5α-pET32a(+)-blaNDM-5-I, were obtained. The MIC value of strain DY51-I for imipenem increased to 512 mg/L, representing a 128-fold increase. Similarly, the MIC value of E. coli DH5α-pET32a(+)-blaNDM-5-I for imipenem also rose to 512 mg/L, also a 128-fold increase.
Results of blaNDM-5 gene expression in the exposed resistant strains
Since there is a significant relationship between the expression level of the blaNDM-5 gene and bacterial resistance, qRT-PCR was utilized to determine the expression levels of the blaNDM-5 gene in E. coli DH5α-pET32a(+)-blaNDM-5 and DY51 strains before and after induction. As illustrated in Figures 7A,B, the expression level of the blaNDM-5 gene in DY51 increased following exposure, with the expression level in the exposed resistant strain DY51-I rising approximately 8-fold compared to the pre-exposed strain, showing a significant difference (p < 0.05). Similarly, the blaNDM-5 expression level in the exposed resistant strain E. coli DH5α-pET32a(+)-blaNDM-5-I increased approximately 4-fold in comparison with the pre-changed strain, also showing a statistically significant difference (p < 0.05). This suggests that the high-level resistance to imipenem in the exposed resistant strains E. coli DH5α-pET32a(+)-blaNDM-5-I and DY51-I may be attributed to a substantial increase in the expression level of the blaNDM-5 gene.
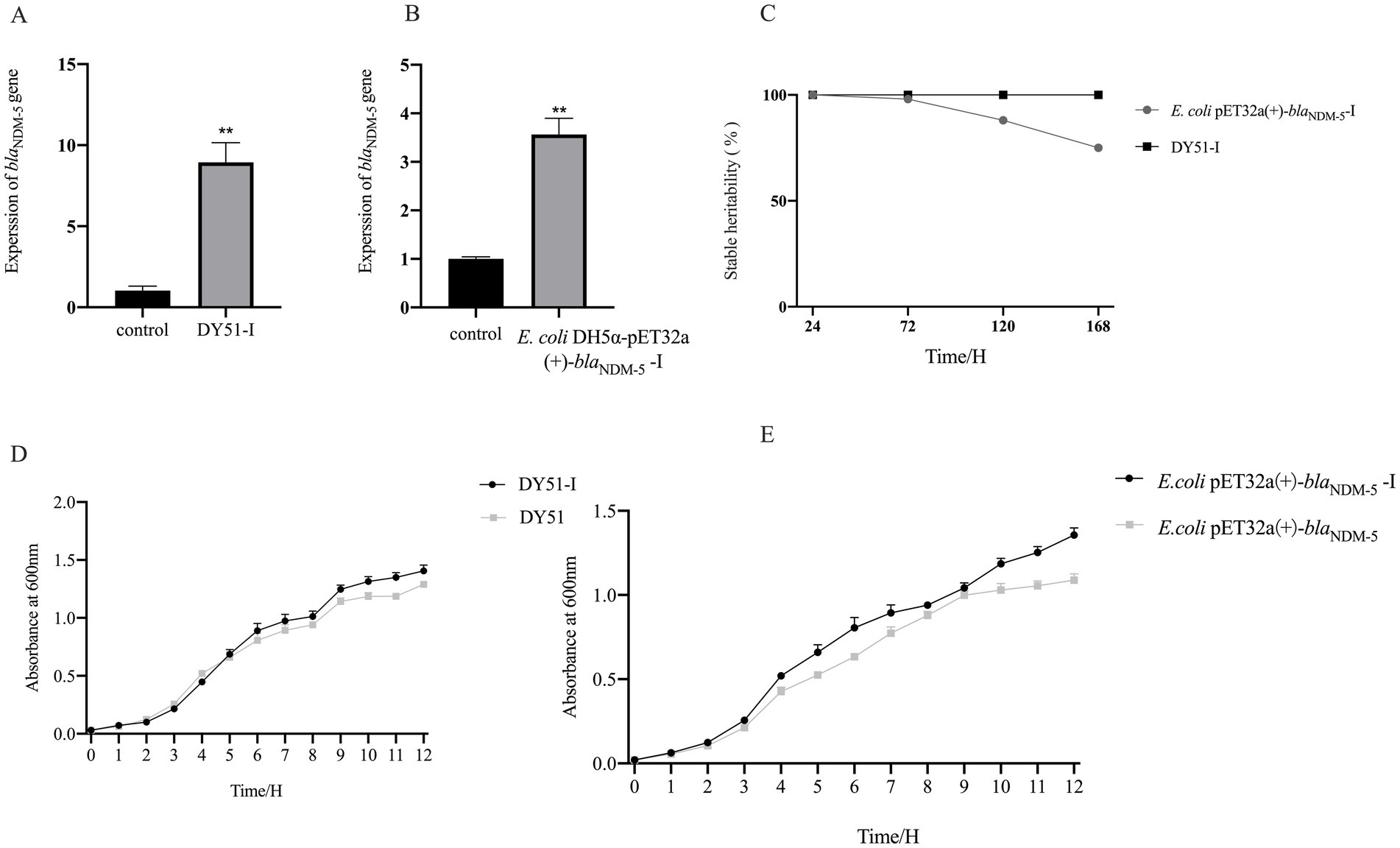
Figure 7. It shows the gene expression, genetic stability, and growth curve results of imipenem exposed strains, demonstrating their ability to adapt to antibiotic stress environments. Note: Figure A and B are the relative expression of blaNDM-5 gene, Figure C shows the genetic stability of blaNDM-5-positive plasmid in induced strains, Figure D is the growth curve comparison before and after DY51 induction, and Figure E is the growth curve comparison before and after E.coli DH5ɑ-pET32a(+)-blaNDM-5 induction.
MIC of imipenem-exposed resistant strains with efflux pump inhibitors
Overexpression of efflux pumps can decrease the sensitivity of E. coli to imipenem. Therefore, the MICs of the highly imipenem-resistant changed strains DY51-I and E. coli DH5α-pET32a(+)-blaNDM-5-I were determined following the addition of the efflux pump inhibitor CCCP. As indicated in Table 4, the MIC values of the highly imipenem-resistant changed strains decreased in the presence of CCCP, suggesting that the high resistance to imipenem in these strains is related to the overexpression of efflux pumps.
Stability and adaptability of in vitro-induced bacterial strains
To investigate the stability of high-level imipenem resistance in induced strains, resistance stability tests were conducted on the induced resistant strains E. coli DH5α-pET32a(+)-blaNDM-5-I and DY51-I. As shown in Figure 7C and Supplementary Table S6, the MIC values of the induced strains E. coli DH5α-pET32a(+)-blaNDM-5-I and DY51-I against imipenem remained at 512 mg/L. However, it is noteworthy that under conditions without antibiotic pressure, the induced strain E. coli DH5α-pET32a(+)-blaNDM-5-I gradually lost the blaNDM-5-positive plasmid. In contrast, the high resistance to imipenem in the induced strain DY51-I could be stably maintained for a considerable period without antibiotic pressure.
To investigate the growth characteristics of the induced resistant strains, growth curve experiments were performed on E. coli DH5α-pET32a(+)-blaNDM-5-I and DY51-I. The results, as shown in Figures 7D,E, indicate that both induced resistant strains exhibited faster growth rates compared to their non-changed counterparts.
Pairwise in vitro competition experiments were conducted between the high-level resistant strains E. coli DH5α-pET32a(+)-blaNDM-5-I and DY51-I and their pre-induced counterparts E. coli DH5α-pET32a(+)-blaNDM-5 and DY51. As shown in Supplementary Figure S10, after 24 h, the high-resistant strains E. coli DH5α-pET32a(+)-blaNDM-5-I and DY51-I displayed relatively strong competitive advantages over the pre-induced strains. However, at 48 h, the high-resistant strains demonstrated absolute competitive superiority.
Discussion
Although carbapenem antibiotics are approved only for human clinical treatment, resistance to them has been detected in bacteria from environmental, animal, and food sources, posing a challenge to clinical practice. The emergence and prevalence of bacteria carrying the blaNDM gene significantly increase the risk of bacterial resistance to carbapenem antibiotics while also seriously affecting the efficacy of β-lactam antibiotics. This represents a major challenge in clinical anti-infective treatment. In this study, 431 intestinal fecal samples were collected from waterfowl in Sichuan, Anhui, Shaanxi, Xinjiang, and Chongqing. The positive isolation rate of blaNDM-5-positive E. coli was found to be 30.5%. Strains carrying the blaNDM gene have been isolated from animal feces, food, and environmental sources across China, with blaNDM-5 being the predominant carrier type found in animals. BlaNDM-5-positive strains have also been isolated from the feces of pigs, chickens, seagulls, and bar-headed geese in China. Interestingly, E. coli carrying blaNDM-5 was also isolated from neonatal patients in Sichuan province (Bai et al., 2023; Wen et al., 2023; Wu et al., 2023).
Antibiotic-resistant genes have been detected in various environmental matrices, including surface water, hospital wastewater, groundwater, urban wastewater, soil, manure, and air. These matrices interact with humans and animals, accelerating the spread of ARGs. Therefore, ARGs are considered as a new type of environmental pollutant (Tan et al., 2018; Han et al., 2022). In this study, ARGs were detected in 103 blaNDM-5-positive E. coli strains, resulting in the identification of a total of 19 ARGs. Among them, floR had the highest detection rate at 88.3%, followed by blaCTX-M (87.4%) and tetA (84.5%). Compared with the results of ARG detection in E. coli isolated from waterfowl in select regions of China between 2021 and 2022 (Zhang et al., 2023), the types of ARGs detected in this study were similar to previous studies; however, the detection rate of ARGs in blaNDM-5-positive E. coli was found to be higher. The overuse or improper use of antibiotics may exacerbate the spread of ARGs and the development of bacterial resistance. Based on the ARGs with the highest detection rates, it is speculated that tetracyclines, sulfonamides, and β-lactam antibiotics have been widely used in the waterfowl breeding industry in the selected regions of this study. Notably, the simultaneous detection of mcr-1 and blaNDM in a single bacterium poses a threat to the treatment of multidrug-resistant bacteria, thus representing significant risks to the healthy development of livestock and poultry breeding. This threat has been evident since the discovery of plasmid-mediated mcr-1 in E. coli isolated from humans and pigs in China in 2015 (Liu et al., 2016), when the effectiveness of antibiotics was significantly reduced. Many studies have reported the coexistence of mcr-1 and blaNDM (Cao et al., 2021; Abe et al., 2022; Kuang et al., 2022; Wang et al., 2023). In addition, in this study, we conducted computational statistical analyses to examine correlations among ARGs as well as between AMR phenotypes and ARGs. The results revealed significant correlations among ARGs as well as between AMR phenotypes and ARGs. However, it is crucial to acknowledge that the correlation among AMR genes is somewhat compromised by the lack of whole-genome sequencing data, including precise plasmid sequence data, which should be a focus of future research.
Increasing research indicates that blaNDM-positive E. coli frequently exhibits resistance to several antibiotics. Consequently, the microbroth dilution method was employed to determine the MIC of 11 antibiotics among 103 strains. Except for DY51, all strains demonstrated high-level resistance to imipenem with MIC values ranging from 64 to ≥512 mg/L. The highest resistance rates were observed for AMP at 100%, CTX at 99.0%, and NFX at 97.1%. In contrast, the lowest resistance rates were noted for AZM at 27.2% and PB at 15.5%. Notably, despite the Chinese government’s ban on the use of chloramphenicol in all food-producing animals since 2002, the resistance rate to chloramphenicol in this study remains high, consistent with previous findings (Li et al., 2013; Mehanni et al., 2023; Qin et al., 2023). The continued use of other antibiotics within the chloramphenicol family, such as florfenicol and thiamphenicol, maybe a significant contributing factor. In addition, the prevalence of multidrug resistance among E. coli strains in this study is alarming, with all strains exhibiting multidrug resistance characterized by resistance to between three and eight different antibiotics, predominantly sextuple resistance (55.3%). Thus, continuous monitoring of bacterial resistance and stringent regulation of antibiotic usage are essential to curb the spread of antibiotic resistance and safeguard human health as well as ecological balance.
Horizontal gene transfer is a key pathway for bacteria to acquire exogenous ARGs and propagate these genes genetically, with plasmids playing a crucial role in facilitating this process (Carattoli, 2013; Hasegawa et al., 2018; Bethke et al., 2020). In this study, the transferability of blaNDM-5-positive E. coli was assessed through conjugation transfer experiments involving 103 blaNDM-5-positive strains. A total of 53 blaNDM-5-positive strains successfully underwent conjugation, resulting in a success rate of 51.5%. Compared to the recipient strain E. coli J53, the MIC values of the conjugants toward imipenem increased by 8–1,024 times, and the blaNDM-5 gene was detected in all 53 conjugants. The results from S1-PFGE indicated that donor strains harbored 3–4 plasmids, while most conjugants carried just one plasmid, strongly suggesting that the blaNDM-5 gene was transferred horizontally via plasmids. For donor strains that failed to successfully conjugate, it is speculated that the blaNDM-5 gene may be located on non-conjugative plasmids or integrated within the chromosome, leading to ineffective transfer. The conjugation frequency of plasmids reflects their ability to undergo horizontal transfer. In this study, the conjugation frequency of the 53 transconjugants ranged from 5.22 × 10−7 to 9.48 × 10−4, comparable to, or even slightly higher than, previously reported conjugation frequencies of plasmids carrying blaNDM-5 (Potron et al., 2011; Huang et al., 2015; Dong et al., 2022; Lv et al., 2022). Therefore, blaNDM-5-positive plasmids can efficiently spread in waterfowl-derived E. coli, potentially contributing further to the prevalence of blaNDM-5.
Plasmids typically confer potential benefits to bacteria, but they also impose certain burdens on the host, which can manifest as reduced growth rates and decreased competitiveness in strains carrying plasmids (Carattoli, 2013; San Millan et al., 2016). The adaptive costs associated with plasmids and the potential loss of plasmids during host cell division can indeed limit the widespread dissemination of plasmids within bacterial communities. However, it is perplexing that, regardless of whether the adaptive costs of plasmids to the host bacteria are high or low, they can persist in host bacteria for extended periods (Wein et al., 2019; Weiss et al., 2023). In this study, some blaNDM-5-positive plasmids were gradually lost during continuous subculturing without antibiotic pressure, while all strains carrying blaNDM-5-positive plasmids remained stable in the population under antibiotic-selective pressure. Plasmids can stably exist in bacterial populations due to intrinsic factors such as high-copy plasmids, medium and low-copy plasmids, and plasmid addiction systems (Harrison and Brockhurst, 2012; San Millan et al., 2016; Tsang, 2017). In addition, changes to plasmid-encoded traits, such as the regulation of plasmid replication and copy number, as well as the acquisition of beneficial genes, may play a role (San Millan et al., 2014; Stalder et al., 2017). Compensatory mutations and compensatory evolution can also help mitigate the adaptive costs imposed by plasmids (Harrison and Brockhurst, 2012; MacLean and San Millan, 2015; Porse et al., 2016). These factors help explain the stable existence of strains carrying blaNDM-5-positive plasmids under antibiotic pressure.
Although plasmids can provide potential benefits to host bacteria under certain conditions, such as exogenous DNA, they can also influence the regulation of bacterial energy networks or exhibit cytotoxic effects. These processes often impose adaptive costs on host bacteria, leading to decreased competitiveness when competing with other strains (San Millan and MacLean, 2017; Prensky et al., 2021). In this study, to assess the fitness cost associated with plasmids carrying blaNDM-5, the blaNDM-5 gene was cloned into the plasmid pET32a(+), resulting in the construction of the transformant E. coli-DH5α-pET32a(+)-blaNDM-5. Subsequently, this transformant was subjected to fitness cost evaluation alongside 53 conjugants and E. coli J53. In the growth curve experiment, the transformant E. coli-DH5α-pET32a(+)-blaNDM-5 and over half of the conjugants exhibited a reduction in growth rate after acquiring the blaNDM-5-positive plasmid. This may be related to the varying adaptability of different strains to the blaNDM-5-positive plasmid. In the in vitro competition experiment, the competitiveness of the transformant E. coli-DH5α-pET32a(+)-blaNDM-5 was slightly reduced, indicating that while the blaNDM-5-positive plasmid can weaken the competitiveness of the host bacteria, the fitness cost is relatively small. In the biofilm formation experiment, all conjugants exhibited an increase or no significant change in their biofilm formation ability after acquiring the blaNDM-5-positive plasmid. The formation of biofilms is influenced by different bacterial species and various external environmental factors. Biofilms can enhance bacterial resistance to harsh environments and increase their tolerance to antibiotics (Ramamurthy et al., 2022; Michaelis and Grohmann, 2023). The phenomenon of enhanced biofilm formation associated with the p3R-IncX3 plasmid carrying blaNDM-5 has been documented, indicating that it can boost the biofilm formation ability of certain host bacteria (Ma et al., 2020). Similarly, the IncHI2 plasmid carrying oqxAB has been reported to enhance biofilm formation in host bacteria (Shi et al., 2018). Although the co-integrating plasmid pSL131-IncA/C-IncX3 carrying blaNDM-1 reduces the growth rate of the host bacteria, it also increases their biofilm formation ability (Liu et al., 2021). Therefore, the enhanced biofilm formation ability in host bacteria due to blaNDM-5-positive plasmids may not only facilitate the dissemination of the blaNDM-5 gene but also complicate clinical treatment efforts. Based on this analysis, it appears that blaNDM-5-positive plasmids impose a certain fitness cost on the host bacteria; however, this cost is relatively minor. Consequently, in-depth research into the molecular transmission characteristics of blaNDM-5 and the effects of blaNDM-5-positive plasmids on host bacteria is crucial for monitoring the dissemination and prevalence of blaNDM-5. Such research will aid in developing effective prevention and control strategies to combat the spread of antibiotic resistance.
The frequent use of antibiotics plays a pivotal role in the development of bacterial resistance. Long-term reliance on antibiotics exerts continuous selective pressure on bacteria, prompting them to adapt and develop resistance through a series of complex mechanisms (Jernberg et al., 2010). In this study, in vitro antibiotic induction experiments revealed that DY51 could transition from low-level resistance (MIC = 4 mg/L) to high-level resistance (MIC = 512 mg/L). This shift in resistance is associated with increased expression of blaNDM-5. Increased MIC attributed to elevated expression and copy number of resistance genes is commonly reported in Gram-negative bacteria. For example, the overexpression of blaNDM-1 in Klebsiella pneumoniae leads to heightened resistance to carbapenem antibiotics (Ding et al., 2024). In addition, exposure to varying concentrations of ciprofloxacin results in increased expression of qnrB1, mediating resistance to fluoroquinolones (Gulyás et al., 2019).
The overexpression of efflux pumps is one of the factors contributing to resistance against carbapenem antibiotics in bacteria such as E. coli, Salmonella, Pseudomonas aeruginosa, and Klebsiella pneumoniae (Li et al., 2015; Lucaßen et al., 2021; Nishino et al., 2021; Zwama and Nishino, 2021). In E. coli, the RND-type efflux pump AcrAB-TolC system is closely associated with antibiotic resistance, mediating resistance to β-lactams, tetracyclines, macrolides, chloramphenicol, and quinolone antibiotics (Tam et al., 2021). Pump inhibition experiments have shown that efflux pumps contribute to changes in antibiotic resistance in various strains, with the AcrAB-TolC system being the main type implicated. Supporting these findings, Chetri et al. observed an increase in the expression of the blaNDM-1, acrA, and acrB genes in E. coli under the pressure of carbapenem (Chetri et al., 2019). Furthermore, research has indicated that the upregulated expression of efflux pump-related genes detected through transcriptome sequencing may result from interactions between the NDM-5 protein and efflux pump-related proteins (Li L. et al., 2024).
Conclusion
In this study, we examined 103 blaNDM-5-positive, multiantibiotic-resistant E. coli strains isolated from 431 intestinal fecal samples of waterfowl across Sichuan, Anhui, Shaanxi, Xinjiang and Chongqing. Our experiments confirmed that the blaNDM-5 gene can be horizontally transferred via highly mobile plasmids, which, although exerting a fitness cost on host bacteria, have a minimal impact overall. One strain, DY51, exhibited a significant shift from low-level (MIC = 4 mg/L) resistance to high-level resistance (MIC = 512 mg/L) following in vitro induction, which was linked to increased blaNDM-5 expression and efflux pump activity.
This study raises significant public health concerns regarding AMR, particularly the presence of blaNDM-5-positive E. coli in waterfowl, which suggests that waterfowl may serve as reservoirs for AMR pathogens. Enhanced agricultural surveillance is essential. Future research should focus on environmental practices that contribute to the spread of AMR genes among waterfowl and the stability of resistance. Targeted interventions and monitoring for AMR in waterfowl are crucial for protecting human health and informing public health policies.
Data availability statement
The original contributions presented in the study are publicly available. This data can be found here: NCBI accession numbers: PQ621134–PQ621236.
Ethics statement
The study was approved by the Committee of Experiment Operational Guidelines and Animal Welfare (License: SYXK 2019-187) of Sichuan Agricultural University. The studies were conducted in accordance with the local legislation and institutional requirements. Written informed consent was obtained from the owners for the participation of their animals in this study.
Author contributions
SZ: Data curation, Writing – original draft, Writing – review & editing. YS: Data curation, Writing – original draft, Writing – review & editing. ZY: Investigation, Writing – review & editing. ZZ: Project administration, Writing – review & editing. MW: Conceptualization, Writing – review & editing. RJ: Conceptualization, Writing – review & editing. SC: Conceptualization, Writing – review & editing. ML: Conceptualization, Writing – review & editing. DZ: Resources, Writing – review & editing. XZ: Resources, Writing – review & editing. YW: Resources, Writing – review & editing. QY: Resources, Writing – review & editing. JH: Resources, Writing – review & editing. XO: Resources, Writing – review & editing. DS: Resources, Writing – review & editing. BT: Resources, Writing – review & editing. ZW: Conceptualization, Writing – review & editing. YH: Conceptualization, Writing – original draft, Writing – review & editing. AC: Conceptualization, Funding acquisition, Writing – review & editing.
Funding
The author(s) declare that financial support was received for the research, authorship, and/or publication of this article. This study was supported by the National Key Research and Development Program of China (2023YFD1800200), the Earmarked Fund for China Agriculture Research System (CARS-42-17), and the Sichuan Veterinary Medicine and Drug Innovation Group of China Agricultural Research System (SCCXTD-2024-18).
Conflict of interest
The authors declare that the research was conducted in the absence of any commercial or financial relationships that could be construed as a potential conflict of interest.
Generative AI statement
The authors declare that no Generative AI was used in the creation of this manuscript.
Publisher’s note
All claims expressed in this article are solely those of the authors and do not necessarily represent those of their affiliated organizations, or those of the publisher, the editors and the reviewers. Any product that may be evaluated in this article, or claim that may be made by its manufacturer, is not guaranteed or endorsed by the publisher.
Supplementary material
The Supplementary material for this article can be found online at: https://www.frontiersin.org/articles/10.3389/fmicb.2024.1501594/full#supplementary-material
Footnotes
References
Abe, R., Akeda, Y., Sakamoto, N., Takeuchi, D., Sugawara, Y., Yamamoto, N., et al. (2022). A nationwide plasmidome surveillance in Thailand reveals a limited variety of New Delhi Metallo-β-lactamase-producing carbapenem-resistant Enterobacteriaceae clones and spreading plasmids. J. Clin. Microbiol. 60:e0108022. doi: 10.1128/jcm.01080-22
Arbab, S., Ullah, H., Wang, W., and Zhang, J. (2022). Antimicrobial drug resistance against Escherichia coli and its harmful effect on animal health. Vet. Med. Sci. 8, 1780–1786. doi: 10.1002/vms3.825
Bai, S., Fang, L., Xiao, H., Zhang, Y., Guo, W., Zhang, J., et al. (2023). Genomics analysis of KPC-2 and NDM-5-producing Enterobacteriaceae in migratory birds from Qinghai Lake, China. Appl. Microbiol. Biotechnol. 107, 7531–7542. doi: 10.1007/s00253-023-12746-3
Bethke, J. H., Davidovich, A., Cheng, L., Lopatkin, A. J., Song, W., Thaden, J. T., et al. (2020). Environmental and genetic determinants of plasmid mobility in pathogenic Escherichia coli. Science. Advances 6:eaax3173. doi: 10.1126/sciadv.aax3173
Cao, X., Zhong, Q., Guo, Y., Hang, Y., Chen, Y., Fang, X., et al. (2021). Emergence of the coexistence of mcr-1, Bla NDM-5, and blaCTX-M-55 in Klebsiella pneumoniae ST485 clinical isolates in China. Infect. Drug Resist. 14, 3449–3458. doi: 10.2147/IDR.S311808
Carattoli, A. (2013). Plasmids and the spread of resistance. Int. J. Med. Microbiol. 303, 298–304. doi: 10.1016/j.ijmm.2013.02.001
Carattoli, A., Bertini, A., Villa, L., Falbo, V., Hopkins, K. L., and Threlfall, E. J. (2005). Identification of plasmids by PCR-based replicon typing. J. Microbiol. Methods 63, 219–228. doi: 10.1016/j.mimet.2005.03.018
Chen, K.-D., Chen, W., Zhang, Q., and Li, Q. (2024). The impact of antibiotic induction on virulence and antibiotic resistance in Klebsiella pneumoniae: a comparative study of CSKP and CRKP strains. Front. Microbiol. 15:1498779. doi: 10.3389/fmicb.2024.1498779
Chetri, S., Bhowmik, D., Dhar, D., Chakravarty, A., and Bhattacharjee, A. (2019). Effect of concentration gradient carbapenem exposure on expression of blaNDM-1 and acrA in carbapenem resistant Escherichia coli. Infect. Genet. Evol. 73, 332–336. doi: 10.1016/j.meegid.2019.05.024
Christaki, E., Marcou, M., and Tofarides, A. (2020). Antimicrobial resistance in bacteria: mechanisms, evolution, and persistence. J. Mol. Evol. 88, 26–40. doi: 10.1007/s00239-019-09914-3
Collineau, L., Bourély, C., Rousset, L., Berger-Carbonne, A., Ploy, M.-C., Pulcini, C., et al. (2023). Towards one health surveillance of antibiotic resistance: characterisation and mapping of existing programmes in humans, animals, food and the environment in France, 2021. Euro Surveill. 28. doi: 10.2807/1560-7917.ES.2023.28.22.2200804
Croxen, M. A., Law, R. J., Scholz, R., Keeney, K. M., Wlodarska, M., and Finlay, B. B. (2013). Recent advances in understanding enteric pathogenic Escherichia coli. Clin. Microbiol. Rev. 26, 822–880. doi: 10.1128/CMR.00022-13
Das, S. (2023). The crisis of carbapenemase-mediated carbapenem resistance across the human-animal-environmental interface in India. Infect. Dis. Now 53:104628. doi: 10.1016/j.idnow.2022.09.023
Ding, L., Yang, Z., and Sun, B. (2024). Understanding blaNDM-1 gene regulation in CRKP infections: toward novel antimicrobial strategies for hospital-acquired pneumonia. Mol. Med. 30:29. doi: 10.1186/s10020-024-00794-y
Dong, H., Li, Y., Cheng, J., Xia, Z., Liu, W., Yan, T., et al. (2022). Genomic epidemiology insights on NDM-producing pathogens revealed the pivotal role of plasmids on blaNDM transmission. Microbiol. Spectr. 10:e0215621. doi: 10.1128/spectrum.02156-21
Endale, H., Mathewos, M., and Abdeta, D. (2023). Potential causes of spread of antimicrobial resistance and preventive measures in one health perspective-a review. Infect. Drug Resist. 16, 7515–7545. doi: 10.2147/IDR.S428837
Gu, Y., Wang, S., Huang, L., Sa, W., Li, J., Huang, J., et al. (2020). Development of resistance in Escherichia coli ATCC25922 under exposure of sub-inhibitory concentration of olaquindox. Antibiotics 9. doi: 10.3390/antibiotics9110791
Guan, Y., Wang, Z., Shang, Z., Zou, H., Zhao, L., Hou, X., et al. (2024). Steady existence of Escherichia coli co-resistant to carbapenem and colistin in an animal breeding area even after the colistin forbidden. J. Environ. Manag. 371:123084. doi: 10.1016/j.jenvman.2024.123084
Gulyás, D., Kocsis, B., and Szabó, D. (2019). Plasmid copy number and qnr gene expression in selection of fluoroquinolone-resistant Escherichia coli. Acta Microbiol. Immunol. Hung. 66, 169–178. doi: 10.1556/030.65.2018.049
Han, B., Ma, L., Yu, Q., Yang, J., Su, W., Hilal, M. G., et al. (2022). The source, fate and prospect of antibiotic resistance genes in soil: A review. Front. Microbiol. 13:976657. doi: 10.3389/fmicb.2022.976657
Harrison, E., and Brockhurst, M. A. (2012). Plasmid-mediated horizontal gene transfer is a coevolutionary process. Trends Microbiol. 20, 262–267. doi: 10.1016/j.tim.2012.04.003
Hasegawa, H., Suzuki, E., and Maeda, S. (2018). Horizontal plasmid transfer by transformation in Escherichia coli: environmental factors and possible mechanisms. Front. Microbiol. 9:2365. doi: 10.3389/fmicb.2018.02365
He, W., Gao, M., Lv, L., Wang, J., Cai, Z., Bai, Y., et al. (2023). Persistence and molecular epidemiology of blaNDM-positive gram-negative bacteria in three broiler farms: A longitudinal study (2015-2021). J. Hazard. Mater. 446:130725. doi: 10.1016/j.jhazmat.2023.130725
He, T., Wei, R., Zhang, L., Sun, L., Pang, M., Wang, R., et al. (2017). Characterization of NDM-5-positive extensively resistant Escherichia coli isolates from dairy cows. Vet. Microbiol. 207, 153–158. doi: 10.1016/j.vetmic.2017.06.010
Hornsey, M., Phee, L., and Wareham, D. W. (2011). A novel variant, NDM-5, of the New Delhi metallo-β-lactamase in a multidrug-resistant Escherichia coli ST648 isolate recovered from a patient in the United Kingdom. Antimicrob. Agents Chemother. 55, 5952–5954. doi: 10.1128/AAC.05108-11
Huang, T.-W., Lauderdale, T.-L., Liao, T.-L., Hsu, M.-C., Chang, F.-Y., Chang, S.-C., et al. (2015). Effective transfer of a 47 kb NDM-1-positive plasmid among Acinetobacter species. J. Antimicrob. Chemother. 70, 2734–2738. doi: 10.1093/jac/dkv191
Humphries, R., Bobenchik, A. M., Hindler, J. A., and Schuetz, A. N. (2021). Overview of changes to the clinical and laboratory standards institute performance standards for antimicrobial susceptibility testing, M100, 31st edition. J. Clin. Microbiol. 59:e0021321. doi: 10.1128/JCM.00213-21
Huo, B., Wei, D., Huang, Q., Huang, S., Fan, L., Li, P., et al. (2024). Acquisition of a stable and transferable plasmid coharbouring hypervirulence and MDR genes with low fitness cost: accelerating the dissemination of ST11-KL64 CR-HvKP. J. Glob. Antimicrob. Resist. 36, 350–357. doi: 10.1016/j.jgar.2024.01.010
Jernberg, C., Löfmark, S., Edlund, C., and Jansson, J. K. (2010). Long-term impacts of antibiotic exposure on the human intestinal microbiota. Microbiology 156, 3216–3223. doi: 10.1099/mic.0.040618-0
Johnson, T. J., Wannemuehler, Y. M., Johnson, S. J., Logue, C. M., White, D. G., Doetkott, C., et al. (2007). Plasmid replicon typing of commensal and pathogenic Escherichia coli isolates. Appl. Environ. Microbiol. 73, 1976–1983. doi: 10.1128/AEM.02171-06
Kuang, X., Yang, R., Ye, X., Sun, J., Liao, X., Liu, Y., et al. (2022). NDM-5-producing Escherichia coli co-harboring mcr-1 gene in companion animals in China. Animals 12. doi: 10.3390/ani12101310
Lerminiaux, N. A., and Cameron, A. D. S. (2019). Horizontal transfer of antibiotic resistance genes in clinical environments. Can. J. Microbiol. 65, 34–44. doi: 10.1139/cjm-2018-0275
Li, L., Gao, Y., Wang, L., Lu, F., Ji, Q., Zhang, Y., et al. (2024). The effects of NDM-5 on Escherichia coli and the screening of interacting proteins. Front. Microbiol. 15:1328572. doi: 10.3389/fmicb.2024.1328572
Li, X.-Z., Plésiat, P., and Nikaido, H. (2015). The challenge of efflux-mediated antibiotic resistance in gram-negative bacteria. Clin. Microbiol. Rev. 28, 337–418. doi: 10.1128/CMR.00117-14
Li, J., Shao, B., Shen, J., Wang, S., and Wu, Y. (2013). Occurrence of chloramphenicol-resistance genes as environmental pollutants from swine feedlots. Environ. Sci. Technol. 47, 2892–2897. doi: 10.1021/es304616c
Li, P., Wei, Y., Li, G., Cheng, H., Xu, Z., Yu, Z., et al. (2020). Comparison of antimicrobial efficacy of eravacycline and tigecycline against clinical isolates of Streptococcus agalactiae in China: in vitro activity, heteroresistance, and cross-resistance. Microb. Pathog. 149:104502. doi: 10.1016/j.micpath.2020.104502
Li, Q., Yang, J., Wang, M., Jia, R., Chen, S., Liu, M., et al. (2024). Global distribution and genomic characteristics analysis of avian-derived mcr-1-positive Escherichia coli. Ecotoxicol. Environ. Saf. 285:117109. doi: 10.1016/j.ecoenv.2024.117109
Li, X., Zhang, J., Yang, C., Li, J., Wang, J., Huang, W., et al. (2022). Increased expression and amplification of blaKPC-2 contributes to resistance to ceftazidime/avibactam in a sequence type 11 Carbapenem-resistant Klebsiella pneumoniae strain. Microbiol. Spectr. 10:e0095522. doi: 10.1128/spectrum.00955-22
Liu, Z., Wang, Z., Lu, X., Peng, K., Chen, S., He, S., et al. (2021). Structural diversity, fitness cost, and stability of a blaNDM-1-bearing cointegrate plasmid in Klebsiella pneumoniae and Escherichia coli. Microorganisms 9. doi: 10.3390/microorganisms9122435
Liu, Y.-Y., Wang, Y., Walsh, T. R., Yi, L.-X., Zhang, R., Spencer, J., et al. (2016). Emergence of plasmid-mediated colistin resistance mechanism MCR-1 in animals and human beings in China: a microbiological and molecular biological study. Lancet Infect. Dis. 16, 161–168. doi: 10.1016/S1473-3099(15)00424-7
Lu, X., Xiao, X., Liu, Y., Li, R., and Wang, Z. (2021). Emerging opportunity and Destiny of mcr-1- and tet(X4)-Coharboring plasmids in Escherichia coli. Microbiol. Spectr. 9:e0152021. doi: 10.1128/Spectrum.01520-21
Lucaßen, K., Müller, C., Wille, J., Xanthopoulou, K., Hackel, M., Seifert, H., et al. (2021). Prevalence of RND efflux pump regulator variants associated with tigecycline resistance in carbapenem-resistant Acinetobacter baumannii from a worldwide survey. J. Antimicrob. Chemother. 76, 1724–1730. doi: 10.1093/jac/dkab079
Lv, L.-C., Lu, Y.-Y., Gao, X., He, W.-Y., Gao, M.-Y., Mo, K.-B., et al. (2022). Characterization of NDM-5-producing Enterobacteriaceae isolates from retail grass carp (Ctenopharyngodon idella) and evidence of blaNDM-5-bearing IncHI2 plasmid transfer between ducks and fish. Zool. Res. 43, 255–264. doi: 10.24272/j.issn.2095-8137.2021.426
Ma, T., Fu, J., Xie, N., Ma, S., Lei, L., Zhai, W., et al. (2020). Fitness cost of blaNDM-5-carrying p3R-IncX3 plasmids in wild-type NDM-free Enterobacteriaceae. Microorganisms 8. doi: 10.3390/microorganisms8030377
Ma, J., Song, X., Li, M., Yu, Z., Cheng, W., Yu, Z., et al. (2023). Global spread of carbapenem-resistant Enterobacteriaceae: epidemiological features, resistance mechanisms, detection and therapy. Microbiol. Res. 266:127249. doi: 10.1016/j.micres.2022.127249
Maclean, R. C., and San Millan, A. (2015). Microbial evolution: towards resolving the plasmid paradox. Curr. Biol. 25, R764–R767. doi: 10.1016/j.cub.2015.07.006
Mehanni, M. M., Gadow, S. I., Alshammari, F. A., Modafer, Y., Ghanem, K. Z., El-Tahtawi, N. F., et al. (2023). Antibiotic-resistant bacteria in hospital wastewater treatment plant effluent and the possible consequences of its reuse in agricultural irrigation. Front. Microbiol. 14:1141383. doi: 10.3389/fmicb.2023.1141383
Michaelis, C., and Grohmann, E. (2023). Horizontal gene transfer of antibiotic resistance genes in biofilms. Antibiotics 12. doi: 10.3390/antibiotics12020328
Mitra, S., Sultana, S. A., Prova, S. R., Uddin, T. M., Islam, F., Das, R., et al. (2022). Investigating forthcoming strategies to tackle deadly superbugs: current status and future vision. Expert Rev. Anti-Infect. Ther. 20, 1309–1332. doi: 10.1080/14787210.2022.2122442
Nishino, K., Yamasaki, S., Nakashima, R., Zwama, M., and Hayashi-Nishino, M. (2021). Function and inhibitory mechanisms of multidrug efflux pumps. Front. Microbiol. 12:737288. doi: 10.3389/fmicb.2021.737288
Poirel, L., Madec, J.-Y., Lupo, A., Schink, A.-K., Kieffer, N., Nordmann, P., et al. (2018). Antimicrobial resistance in Escherichia coli. Microbiol. Spectr. 6. doi: 10.1128/microbiolspec.ARBA-0026-2017
Porse, A., Schønning, K., Munck, C., and Sommer, M. O. A. (2016). Survival and evolution of a large multidrug resistance plasmid in new clinical bacterial hosts. Mol. Biol. Evol. 33, 2860–2873. doi: 10.1093/molbev/msw163
Potron, A., Poirel, L., and Nordmann, P. (2011). Plasmid-mediated transfer of the blaNDM-1 gene in gram-negative rods. FEMS Microbiol. Lett. 324, 111–116. doi: 10.1111/j.1574-6968.2011.02392.x
Prensky, H., Gomez-Simmonds, A., Uhlemann, A.-C., and Lopatkin, A. J. (2021). Conjugation dynamics depend on both the plasmid acquisition cost and the fitness cost. Mol. Syst. Biol. 17:e9913. doi: 10.15252/msb.20209913
Pu, D., Zhao, J., Chang, K., Zhuo, X., and Cao, B. (2023). "superbugs" with hypervirulence and carbapenem resistance in Klebsiella pneumoniae: the rise of such emerging nosocomial pathogens in China. Sci. Bull. 68, 2658–2670. doi: 10.1016/j.scib.2023.09.040
Qin, Y., Ren, X., Ju, H., Zhang, Y., Liu, J., Zhang, J., et al. (2023). Occurrence and distribution of antibiotics in a tropical mariculture area of Hainan, China: implications for risk assessment and management. Toxics 11. doi: 10.3390/toxics11050421
Ramamurthy, T., Ghosh, A., Chowdhury, G., Mukhopadhyay, A. K., Dutta, S., and Miyoshi, S.-I. (2022). Deciphering the genetic network and programmed regulation of antimicrobial resistance in bacterial pathogens. Front. Cell. Infect. Microbiol. 12:952491. doi: 10.3389/fcimb.2022.952491
San Millan, A., Escudero, J. A., Gifford, D. R., Mazel, D., and Maclean, R. C. (2016). Multicopy plasmids potentiate the evolution of antibiotic resistance in bacteria. Nat. Ecol. Evol. 1:10. doi: 10.1038/s41559-016-0010
San Millan, A., and Maclean, R. C. (2017). Fitness costs of plasmids: a limit to plasmid transmission. Microbiol. Spectr. 5. doi: 10.1128/microbiolspec.MTBP-0016-2017
San Millan, A., Peña-Miller, R., Toll-Riera, M., Halbert, Z. V., Mclean, A. R., Cooper, B. S., et al. (2014). Positive selection and compensatory adaptation interact to stabilize non-transmissible plasmids. Nat. Commun. 5:5208. doi: 10.1038/ncomms6208
Seghal Kiran, G., Anto Thomas, T., Selvin, J., Sabarathnam, B., and Lipton, A. P. (2010). Optimization and characterization of a new lipopeptide biosurfactant produced by marine Brevibacterium aureum MSA13 in solid state culture. Bioresour. Technol. 101, 2389–2396. doi: 10.1016/j.biortech.2009.11.023
Shi, H., Zhou, X., Zou, W., Wang, Y., Lei, C., Xiang, R., et al. (2018). Co-occurrence of biofilm formation and quinolone resistance in Salmonella enterica serotype typhimurium carrying an IncHI2-type oqxAB-positive plasmid. Microb. Pathog. 123, 68–73. doi: 10.1016/j.micpath.2018.06.006
Stalder, T., Rogers, L. M., Renfrow, C., Yano, H., Smith, Z., and Top, E. M. (2017). Emerging patterns of plasmid-host coevolution that stabilize antibiotic resistance. Sci. Rep. 7:4853. doi: 10.1038/s41598-017-04662-0
Tam, H.-K., Foong, W. E., Oswald, C., Herrmann, A., Zeng, H., and Pos, K. M. (2021). Allosteric drug transport mechanism of multidrug transporter AcrB. Nat. Commun. 12:3889. doi: 10.1038/s41467-021-24151-3
Tan, L., Li, L., Ashbolt, N., Wang, X., Cui, Y., Zhu, X., et al. (2018). Arctic antibiotic resistance gene contamination, a result of anthropogenic activities and natural origin. Sci. Total Environ. 621, 1176–1184. doi: 10.1016/j.scitotenv.2017.10.110
Tang, B., Elbediwi, M., Nambiar, R. B., Yang, H., Lin, J., and Yue, M. (2022). Genomic characterization of antimicrobial-resistant Salmonella enterica in duck, chicken, and pig farms and retail markets in eastern China. Microbiol. Spectr. 10:e0125722. doi: 10.1128/spectrum.01257-22
Tsang, J. (2017). Bacterial plasmid addiction systems and their implications for antibiotic drug development. Postdoc J. 5, 3–9. doi: 10.14304/SURYA.JPR.V5N5.2
Wang, X. R., Lian, X.-L., Su, T.-T., Long, T.-F., Li, M.-Y., Feng, X.-Y., et al. (2021). Duck wastes as a potential reservoir of novel antibiotic resistance genes. Sci. Total Environ. 771:144828. doi: 10.1016/j.scitotenv.2020.144828
Wang, M. G., Zhang, R.-M., Wang, L.-L., Sun, R.-Y., Bai, S.-C., Han, L., et al. (2021). Molecular epidemiology of carbapenemase-producing Escherichia coli from duck farms in south-east coastal China. J. Antimicrob. Chemother. 76, 322–329. doi: 10.1093/jac/dkaa433
Wang, D., Zou, H., Zhao, L., Li, Q., Meng, M., Li, X., et al. (2023). High prevalence of Escherichia coli co-harboring conjugative plasmids with colistin- and carbapenem resistance genes in a wastewater treatment plant in China. Int. J. Hyg. Environ. Health 250:114159. doi: 10.1016/j.ijheh.2023.114159
Wein, T., Hülter, N. F., Mizrahi, I., and Dagan, T. (2019). Emergence of plasmid stability under non-selective conditions maintains antibiotic resistance. Nat. Commun. 10:2595. doi: 10.1038/s41467-019-10600-7
Weiss, A., Wang, T., and You, L. (2023). Promotion of plasmid maintenance by heterogeneous partitioning of microbial communities. Cell Syst. 14, 895–905.e5. doi: 10.1016/j.cels.2023.09.002
Wen, R., Wei, H., Zhang, T., Ma, P., Wang, Q., Li, C., et al. (2023). Epidemiological characterisation of blaNDM-positive Enterobacterales from food-producing animal farms in Southwest China. Microorganisms 11. doi: 10.3390/microorganisms11092304
Wu, W., Jiang, Y., Zhou, W., and Kuang, L. (2023). Genomic characteristics of carbapenem-resistant Klebsiella pneumoniae isolated from neonatal patients in Southwest China during 2017-2021. Infect. Drug Resist. 16, 6725–6733. doi: 10.2147/IDR.S426565
Yong, D., Toleman, M. A., Giske, C. G., Cho, H. S., Sundman, K., Lee, K., et al. (2009). Characterization of a new metallo-beta-lactamase gene, bla(NDM-1), and a novel erythromycin esterase gene carried on a unique genetic structure in Klebsiella pneumoniae sequence type 14 from India. Antimicrob. Agents Chemother. 53, 5046–5054. doi: 10.1128/AAC.00774-09
Zhang, S., Abbas, M., Rehman, M. U., Huang, Y., Zhou, R., Gong, S., et al. (2020). Dissemination of antibiotic resistance genes (ARGs) via integrons in Escherichia coli: A risk to human health. Environ. Pollut. 266:115260. doi: 10.1016/j.envpol.2020.115260
Zhang, S., Abbas, M., Rehman, M. U., Wang, M., Jia, R., Chen, S., et al. (2021a). Updates on the global dissemination of colistin-resistant Escherichia coli: an emerging threat to public health. Sci. Total Environ. 799:149280. doi: 10.1016/j.scitotenv.2021.149280
Zhang, S., Chen, S., Abbas, M., Wang, M., Jia, R., Chen, S., et al. (2021b). High incidence of multi-drug resistance and heterogeneity of mobile genetic elements in Escherichia coli isolates from diseased ducks in Sichuan province of China. Ecotoxicol. Environ. Saf. 222:112475. doi: 10.1016/j.ecoenv.2021.112475
Zhang, S., Shu, Y., Wang, Y., Zhong, Z., Wang, M., Jia, R., et al. (2023). High rate of multidrug resistance and integrons in Escherichia coli isolates from diseased ducks in select regions of China. Poult. Sci. 102:102956. doi: 10.1016/j.psj.2023.102956
Zhao, Q., Sha, L., Wu, Z., Meng, L., Yang, F., Wu, L., et al. (2023). Evolution of carbapenem resistance in klebsiella pneumoniae and Escherichia coli carrying blaNDM-1 gene: imipenem exposure results in sustained resistance memory of strains in vitro. Ann. Clin. Microbiol. Antimicrob. 22:46. doi: 10.1186/s12941-023-00598-8
Keywords: blaNDM-5 , Escherichia coli , antibiotic resistance, horizontal gene transfer, plasmid
Citation: Zhang S, Shu Y, Yang Z, Zhong Z, Wang M, Jia R, Chen S, Liu M, Zhu D, Zhao X, Wu Y, Yang Q, Huang J, Ou X, Sun D, Tian B, Wu Z, He Y and Cheng A (2024) Decoding the enigma: unveiling the transmission characteristics of waterfowl-associated blaNDM-5-positive Escherichia coli in select regions of China. Front. Microbiol. 15:1501594. doi: 10.3389/fmicb.2024.1501594
Edited by:
Miklos Fuzi, Independent Researcher, Seattle, WA, United StatesReviewed by:
Costas C. Papagiannitsis, University of Thessaly, GreeceDongdong Yin, Anhui Academy of Agricultural Sciences (CAAS), China
Copyright © 2024 Zhang, Shu, Yang, Zhong, Wang, Jia, Chen, Liu, Zhu, Zhao, Wu, Yang, Huang, Ou, Sun, Tian, Wu, He and Cheng. This is an open-access article distributed under the terms of the Creative Commons Attribution License (CC BY). The use, distribution or reproduction in other forums is permitted, provided the original author(s) and the copyright owner(s) are credited and that the original publication in this journal is cited, in accordance with accepted academic practice. No use, distribution or reproduction is permitted which does not comply with these terms.
*Correspondence: Anchun Cheng, Y2hlbmdhbmNodW5AdmlwLjE2My5jb20=
†These authors have contributed equally to this work and share first authorship