- 1Foundational Sciences Directorate, Bacteriology Division, United States Army Medical Research Institute of Infectious Diseases, Frederick, MD, United States
- 2Faculty of Chemistry and Department of Microbiology and Ecosystem Science, University of Vienna, Vienna, Austria
Melioidosis is caused by Burkholderia pseudomallei, an opportunistic Gram-negative pathogen that inhabits soil and water in tropical and subtropical regions. B. pseudomallei infections often occur following contact with contaminated water or soil or by inhalation of contaminated dust and water droplets. There is limited knowledge about how B. pseudomallei is able to survive in harsh environmental conditions and compete with the microbes that inhabit these niches. Previous research demonstrated that 3-methyl-2-alkylquinolones (MAQs), and their corresponding N-oxides (MAQNOs), are produced by B. pseudomallei and provide a competitive advantage when grown in the presence of Gram-positive bacteria. In this study, 39 Gram-negative environmental bacteria in the Pseudomonadota and Bacteroidota phyla were isolated and characterized. Intriguingly, B. pseudomallei inhibited 71% of bacteria in the phylum Bacteroidota in zone of inhibition and coculture competition assays, but no Pseudomonadota isolates were similarly inhibited. Transposon mutagenesis was utilized to identify B. pseudomallei genes required for the inhibition of Sphingobacterium sp. ST4, a representative member of the Bacteroidota. Three mutations mapped to hmqA-G, the locus encoding 2-alkylquinolone derivatives, and two mutations were identified in scmR, a gene encoding a quorum-sensing controlled LysR-type transcriptional regulator. B. pseudomallei strains with deletion mutations in hmqD and scmR were unable to produce 2-alkylquinolone derivatives or inhibit Bacteroidota isolates in competition assays. RAW264.7 murine macrophage cells were infected with B. pseudomallei 1026b and 1026b ΔhmqD and there was a 94-fold reduction in the number of intracellular 1026b ΔhmqD bacteria relative to 1026b. The 50% lethal dose (LD50) of 1026b and 1026b ΔhmqD in BALB/c mice was determined to be 3 x 105 colony forming units (CFU) and > 1 x 106 CFU, respectively. Taken together, the results indicate that the products of the B. pseudomallei hmqA-G locus are important for intracellular replication in murine macrophages, virulence in a mouse model of melioidosis, and competition with bacteria that utilize naphthoquinones for aerobic respiration.
Introduction
Burkholderia pseudomallei, the etiologic agent of melioidosis, is a Gram-negative bacterium found in water and soil in tropical and subtropical environments worldwide (Inglis and Mayo, 2012; Limmathurotsakul et al., 2016; Limmathurotsakul et al., 2012; Meumann et al., 2024). Humans and animals are exposed to the pathogen via inhalation, ingestion, and/or direct contact with contaminated water or soil. Melioidosis patients often present with bacteremia and/or pneumonia, but infections can also affect multiple organs and lead to a variety of clinical manifestations. People with diabetes, liver disease, renal disease, chronic lung disease, and other immunocompromising conditions are more likely to develop melioidosis than individuals without known risk factors. The global distribution of B. pseudomallei continues to grow (Currie et al., 2023) and a recent report demonstrates that it’s now endemic in the Mississippi Gulf Coast region of the United States (Petras et al., 2023). B. pseudomallei is classified as a Tier 1 Select Agent because it is infectious by the aerosol route, it is relatively difficult to treat with antibiotics, and no approved vaccine is currently available (Meumann et al., 2024).
Members of the genus Burkholderia harbor a diverse array of biosynthetic gene clusters (BGCs) that encode natural products with potential uses in medicine and agriculture (Klaus et al., 2020a; Kunakom and Eustáquio, 2019). As many as 21 distinct BGCs have been bioinformatically identified in the genomic sequences of B. pseudomallei strains and a majority of the encoded bioactive compounds have been chemically and biologically characterized (Biggins et al., 2014; Klaus et al., 2020a; Kunakom and Eustáquio, 2019). B. pseudomallei and B. thailandensis, a closely-related nonpathogenic species, both produce a variety of 2-alkylquinolone derivatives, including 2-alkylquinolones (AQs), AQ N-oxides (AQNOs), 3-methyl-2-alkylquinolones (MAQs), and MAQ N-oxides (MAQNOs) (Diggle et al., 2006; Klaus et al., 2020a; Klaus et al., 2020b; Mou et al., 2021; Piochon et al., 2020; Szamosvári et al., 2020; Vial et al., 2008). In fact, the biosynthetic enzymes encoded by the hmqA-G operon, and the unlinked hmqL gene, produce greater than twenty 2-alkylquinolone derivatives with unsaturated or saturated alkyl groups of seven to eleven carbons at the 2-position, a proton or methyl group at the 3-position, and molecules with a N-oxide at the 1-position (Diggle et al., 2006; Okada et al., 2016; Saalim et al., 2020; Savchenko et al., 2024; Vial et al., 2008; Wu and Seyedsayamdost, 2017). The variety of chemical substitutions on the AQ core can result in important differences in biological activity, which can include antibacterial, antimalarial, antifungal, antialgal, and antioxidant properties (Dow et al., 2020; Piochon et al., 2020; Saalim et al., 2020; Szamosvári et al., 2019).
In order to survive and persist in environmental niches, bacteria must compete with complex mixtures of microorganisms for limited nutrients (Srinivasan et al., 2024). Previous studies have demonstrated that B. pseudomallei and B. thailandensis effectively compete with environmental Gram-positive bacteria in a hmqA-G-dependent manner (Klaus et al., 2020b; Mou et al., 2021). In addition, Gram-positive bacteria are more effectively inhibited by chemically synthesized AQs, AQNOs, MAQs, and MAQNOs than Gram-negative bacteria (Piochon et al., 2020; Szamosvári and Böttcher, 2017; Szamosvári et al., 2020). The diverse 2-alkylquinolone compounds produced by the Burkholderia hmq genes may act synergistically to inhibit bacterial growth by acting on different targets (Klaus et al., 2020b; Wu and Seyedsayamdost, 2017). Wu and Seyedsayamdost demonstrated that 4-hydroxy-3-methyl-2-(2-nonenyl) quinoline (HMNQ) and 2-heptyl-4(1H)-quinoline N-oxide (HQNO) inhibit pyrimidine biosynthesis by acting on a common target, but interrupt the proton motive force by acting on different targets (Wu and Seyedsayamdost, 2017). It has been proposed that MAQNOs mimic the native bacterial menaquinone (MK)/menaquinol (MKH2) redox couple that shuttle electrons in the respiratory chain (Nowicka and Kruk, 2010; Szamosvári and Böttcher, 2017). MK is the most ancient type of isoprenoid quinone and is the only quinone used by Gram-positive bacteria for aerobic respiration (Franza and Gaudu, 2022; Schoepp-Cothenet et al., 2013). By comparison, the model Gram-negative bacterium Escherichia coli produces MK, demethylmenaquinone (DMK), and ubiquinone (UQ), but only employs UQ for aerobic respiration.
The goal of this study was to isolate a diverse collection of Gram-negative environmental bacteria and evaluate their ability to compete with B. pseudomallei in zone of inhibition and coculture assays. The results demonstrated that ∼13% of the environmental isolates were unable to survive and grow as well in coculture with B. pseudomallei as they did in monoculture. Further evaluation of the molecular mechanism by which B. pseudomallei inhibited the susceptible bacteria indicated that the inhibition was dependent on the production of AQ derivatives encoded by the hmqA-G gene cluster. Transcriptional profiling also indicated that the expression of the hmqA-G operon is dependent on ScmR, a LysR-type transcriptional regulator (LTTR) that is positively regulated by quorum sensing (QS). Infection of RAW 264.7 murine macrophage cells with B. pseudomallei 1026b and 1026b ΔhmqD revealed an intracellular replication defect in the mutant. The relative virulence of these strains was also assessed in BALB/c mice and 1026b ΔhmqD was found to be less virulent than 1026b in this animal model of melioidosis. Furthermore, a previously described Burkholderia ambifaria HmqD enzyme inhibitor exhibited strong activity against the production of MAQs and MAQNOs by B. pseudomallei and may represent a novel melioidosis therapeutic countermeasure. The results show that the wide variety of AQ, AQNO, MAQ, and MAQNO molecules produced by B. pseudomallei are important for virulence in a mammalian model of infection and are critical for competition with Gram-negative bacteria that utilize naphthoquinones such as MK and DMK for aerobic respiration.
Materials and methods
Bacterial strains, plasmids, and growth conditions
The bacterial strains and plasmids used in this study are shown in Supplementary Table 1. Escherichia coli and B. pseudomallei were grown at room temperature (RT) or 37°C on Luria-Bertani (LB) agar (Lennox formulation; Sigma-Aldrich) or in LB broth. One hundred micrograms per milliliter adenine HCl and 5 μg/ml thiamine HCl were added to solid and liquid media for growth of the purM select agent exempt strain B. pseudomallei Bp82 and mutant derivatives. Broth cultures were grown in 14-ml Falcon round-bottom polypropylene tubes with snap caps (Fisher Scientific) or in 13 ml polypropylene tubes with snap caps (Sarstedt) containing 3 ml of LB and shaken at 250 rpm unless indicated otherwise. When appropriate, antibiotics were added at the following concentrations: 25 μg/ml kanamycin (Km) and streptomycin (Sm) for E. coli, and 25 μg/ml polymyxin B (Pm) and 500 to 1,000 μg/ml Km for B. pseudomallei. Growth curves for Bp82 and CM139 were conducted by growing the bacteria in LB broth for 18 h at 37°C and 180 rpm. One hundred fifty microliters of saturated culture were inoculated into 10 ml of LB medium in a sterile 50 ml polypropylene tube with a screw cap (VWR). The caps of the tubes were loosely opened by a 180-degree turn and fixed in this position with tape to ensure equal oxygen supply. The cultures were incubated at 37°C and 180 rpm and growth evaluated at 1.5, 3.0, 4.5, 6.0, 7.5, 9.0, and 24 h by measurement of the OD600 of 250 μl of bacterial culture in plastic cuvettes. The experiment was performed in biological triplicates. A 20 μg/ml stock solution of the chromogenic indicator 5-bromo-4-chloro-3-indolyl-β-D-galactopyranoside (X-Gal) was prepared in N,N-dimethylformamide, and 40 μl was spread onto the surface of plate medium for blue/white screening in E. coli TOP10 or E. cloni® 10G chemically competent cells.
Environmental bacteria were isolated from several sources in Frederick, MD, USA (latitude and longitude coordinates, 39.396509 and 277.368223, respectively) from 2017 to 2022. Bacterial river (R) isolates were obtained by spreading aliquots of Monocacy River water serially diluted in sterile phosphate-buffered saline (PBS) onto the surfaces of sheep blood agar plates, LB agar plates, LB agar plates supplemented with 60 μg/ml X-Gal, BD Difco M9 Minimal Salts (Becton, Dickinson and Company) agar plates containing 0.4% glucose, and Difco™ R2A agar (BD) plates. The plates were incubated at 37°C for 2 days, and colonies that could be easily distinguished from B. pseudomallei colonies on solid medium due to morphology, pigmentation, and/or β-galactosidase production were selected for further characterization. Bacterial colonies that could not easily be differentiated from B. pseudomallei colonies were also retained for further analysis. A similar strategy was employed for water obtained from a stream (ST) that flows directly into the Monocacy River. River sediment (RS) was obtained using sterile 50 ml conical tubes, resuspended in PBS, serially diluted in PBS, and spread onto agar plates as described above. Bacterial rhizosphere (RZ) isolates associated with the root microbiome of lawn weeds were placed into sterile 50 ml conical tubes and resuspended in PBS, vigorously vortexed, and serially diluted in PBS, and aliquots were spread onto agar plates.
Unique bacterial colonies were selected and PCR products were generated from purified genomic DNA using the universal 16S rRNA primers 533F (Weisburg et al., 1991) and 1492R (Lane, 1991). The nucleotide sequences of the partial 16S rRNA genes were used to search against the nonredundant nucleotide collection database using BLASTN (McGinnis and Madden, 2004) and the top nucleotide hits indicated that 39 distinct Gram-negative bacteria were isolated.
DNA manipulation
Restriction enzymes (Roche Molecular Biochemicals and New England BioLabs), Antarctic phosphatase (New England BioLabs), and T4 DNA ligase (Roche Molecular Biochemicals) were used according to the manufacturer’s instructions. When necessary, the End-It DNA End-Repair kit (Epicentre) was used to convert 5′ or 3′ protruding ends to blunt-ended DNA. The DNA fragments used in the cloning procedures were excised from agarose gels and purified with a PureLink Quick Gel Extraction Kit (Thermo Fisher Scientific). Bacterial genomic DNA was prepared from overnight LB broth cultures with the GenElute Bacterial Genomic DNA Kit (Sigma-Aldrich). Plasmids were purified from overnight LB broth cultures by using the Wizard Plus SV Miniprep DNA Purification System (Promega).
PCR amplifications
The PCR primers used in this study are shown in Supplementary Table 1. The PCR products were sized and isolated by using agarose gel electrophoresis, cloned using the pCR2.1-TOPO TA Cloning Kit (Life Technologies), and transformed into chemically competent TOP10 or E. cloni® 10G. The PCR amplifications were performed in a final reaction volume of 50 or 100 μl containing 1x FailSafe PCR PreMix D (Epicentre), 1.25 U FailSafe PCR Enzyme Mix (Epicentre), 1 μM PCR primers, and approximately 200 ng of genomic DNA. Genomic DNA was isolated from all environmental bacterial isolates, and their 16S rRNA genes were PCR amplified using the primers 533F and 1492R (Supplementary Table 1) and cloned into pCR2.1-TOPO. Colony PCR was utilized to screen for B. pseudomallei deletion mutants. Briefly, sucrose-resistant and Km-sensitive colonies were resuspended in 50 μl of water, and 2 μl were added to the PCR mixture rather than purified genomic DNA. PCR cycling was performed using a Mastercycler pro S (Eppendorf) and heated to 97°C for 5 min. This was followed by 30 cycles of a three-temperature cycling protocol (97°C for 30 s, 55°C for 30 s, and 72°C for 1 min) and 1 cycle at 72°C for 10 min. The scmR-5′-F/scmR-5′-R and scmR-3′-F/scmR-3′-R PCR products were joined by splicing by overlap extension (SOE) and the resulting product was incorporated into pMo130 using the In-Fusion HD EcoDry Cloning Kit (Takara Bio USA, Inc) to construct Bp82 ΔscmR (Supplementary Table 1). For PCR products larger than 1 kb, an additional 1 min per kb was added to the extension time.
DNA sequencing
DNA inserts cloned into pCR2.1-TOPO were PCR amplified with M13 forward and M13 reverse primers (Supplementary Table 1), and unincorporated deoxynucleoside triphosphates (dNTPs) and primers were removed using the DyeEx 2.0 Spin Kit (Qiagen). The PCR products were then sequenced with the M13 forward and M13 reverse primers using the ABI BigDye Terminator v3.1 Cycle Sequencing Kit (Thermo Fisher Scientific) and an Applied Biosystems SeqStudio Genetic Analyzer (Thermo Fisher Scientific) according to the manufacturer’s instructions. The nucleotide sequences were analyzed with DNASTAR Lasergene 17 software.
TnMod-OKm’ mutagenesis and plasmid conjugations
TnMod-OKm’ (Dennis and Zylstra, 1998) was delivered to Bp82 via conjugation with E. coli S17-1 (pTnMod-OKm’) by using a membrane filter mating technique. Briefly, S17-1 (pTnMod-OKm’) was inoculated into 3 ml of LB broth containing Km and Sm and grown at 37°C for 18 to 20 h with shaking (250 rpm). B. pseudomallei was also grown under these conditions but without antibiotic selection. One hundred microliters of each saturated culture were added to 3ml of sterile 10 mM MgSO4, mixed, and filtered through a 0.45-mm-pore-size nitrocellulose filter using a 25-mm Swinnex filter apparatus (Millipore). Filters were placed on LB plates supplemented with 10 mM MgSO4 and incubated for 8 h in a 37°C incubator. The filters were washed with 1-2 ml of sterile phosphate-buffered saline (PBS), and 100–300 μl aliquots were spread onto LB agar plates containing Km and Pm. Kmr and Pmr colonies were identified after 48 h of incubation at 37°C. TnMod-OKm’ contains a Kmr gene and a pMB1 conditional origin of replication that does not function in B. pseudomallei, allowing the rapid cloning of DNA adjacent to the transposon’s site of insertion into E. coli. The in vitro cloning of DNA flanking the TnMod-OKm’ insertion sites in B. pseudomallei CCM1, CCM2, CCM6, CCM7, and CCM9 was performed by digesting total genomic DNA with the restriction endonucleases AscI, SalI or NotI, self-ligating, and transforming into an E. coli host (Supplementary Table 1). The resulting plasmids were then sequenced with outward facing primers (TnMod-LT2 or KM-RT) that binds to the left or right end of TnMod-OKm’. The resulting sequence reactions revealed the junction of the transposon and B. pseudomallei genomic DNA. Plasmids pMo130 and pBHR2 and their derivatives were likewise conjugated to B. pseudomallei by using E. coli S17-1 as the donor strain (Supplementary Table 1).
Screening for B. pseudomallei Bp82 transposon mutants that do not produce a zone of inhibition on lawns of Sphingobacterium sp. ST4
Sphingobacterium sp. ST4 is naturally resistant to Km and this antibiotic was incorporated into the screening plates in order to ensure retention of TnMod-OKm’ in the Bp82 mutants. Individual TnMod-OKm’ mutants of Bp82 were picked from 150 x 15 mm polystyrene petri plates containing LB agar with adenine HCl (100 μg/ml), thiamine HCl (5 μg/ml), and Km (1,000 μg/ml) using sterile toothpicks. Prior to transfer, the agar medium was inoculated with Sphingobacterium sp. ST4 by submersing a sterile swab into a saturated LB broth culture and spreading it across the entire surface of the agar in back-and-forth motions. The agar plate was rotated 90° three times, and this process was repeated and the surface of the agar was allowed to dry in a class II microbiological safety cabinet prior to co-inoculation with B. pseudomallei Bp82 transposon mutants. The plates were incubated at 37°C for 1 to 2 days and screened for mutants that did not produce zones of inhibition (clearing) around the colonies. Potential Bp82 mutants were transferred to Ashdown Select Media agar plates (Ashdown, 1979) containing adenine HCl (100 μg/ml), thiamine HCl (5 μg/ml), carbenicillin (10 μg/ml) and polymyxin B (25 μg/ml) to select against Sphingobacterium sp. ST4 contamination. Approximately 6,000 transposon mutants were screened by this method.
Construction of B. pseudomallei gene replacement mutants
Gene replacement experiments with B. pseudomallei were performed using the sacB-based vector pMo130 (Supplementary Table 1), as previously described (Burtnick et al., 2011; Hamad et al., 2009; Logue et al., 2009). Recombinant derivatives of pMo130 were electroporated into E. coli S17-1 (12.25 kV/cm) and conjugated with B. pseudomallei Bp82 or 1026b for 8 h. Pm was used to counterselect E. coli S17-1. The optimal conditions for the resolution of the sacB constructs were found to be LB agar lacking NaCl and containing 15% (wt/vol) sucrose with incubation at 37°C for 2 days. B. pseudomallei deletion mutants were identified by colony PCR using the primers flanking the deleted regions of the targeted genes. The mutants containing promoterless lacZ fusions to hmqD and scmR were identified by using a gene-specific primer and lacZ-dn2 (Supplementary Table 1).
Bacterial competition assays
Thirty-nine unique environmental Gram-negative bacteria were initially assessed for their potential to form a zone of inhibition when grown as a bacterial lawn in the presence of Bp82. Briefly, each environmental bacterial isolate was grown in LB broth for 18 h, diluted 1:10 or 1:50 in PBS, and a sterile swab was used to spread the diluted culture across the entire surface of a LB agar in back-and-forth motions. The agar plate was co-inoculated with B. pseudomallei Bp82 in triplicate. The plates were incubated at 37°C for 1 to 2 days and screened for strains that produced zones of inhibition (clearing) around the Bp82 colonies and the inhibition zones were measured with a ruler. For those bacteria that formed zones of inhibition with Bp82, the same process was repeated with Bp82 ΔhmqD to identify environmental isolates that were inhibited in a 2-alkylquinolone-dependent manner. These bacteria were further assessed in a bacterial coculture competition assay.
A modified quantitative bacterial coculture competition assay (Hachani et al., 2013) was employed to assess the ability of B. pseudomallei to compete with the environmental Gram negative bacteria. Briefly, B. pseudomallei and environmental competitors were grown in LB broth at 37°C for 18 h, and three independent cultures of each strain were used for each competition assay performed. Two hundred microliters of each of the saturated cultures was pelleted by centrifugation, washed with sterile PBS, and diluted to ∼1 x 107 CFU/ml, and 10 μl aliquots of each competitor were spotted onto the surface of LB agar or LB agar containing X-Gal. One hundred microliters of B. pseudomallei and of the environmental competitor were also combined and mixed, and a 20 μl aliquot of the 1:1 mixture was spotted onto the solid medium and incubated for 48 h at room temperature (RT). The remaining competition mixture was serially diluted in PBS, and 100–μl aliquots were spread onto LB agar or LB agar containing X-Gal to determine the input concentration of B. pseudomallei and environmental species present in the mixture. Following incubation, the bacteria present in each spot were resuspended in 1 ml of PBS using sterile swabs and serially diluted in PBS, and 100 μl aliquots were spread onto LB agar or LB agar containing X-Gal and incubated for 1 to 2 days at 37°C to determine the number of CFU present. The quantity of each competitor present in the competition mixture was assessed by enumerating the number of B. pseudomallei off-white colonies compared to the number of Gram-negative pigmented or blue colonies. The fold difference between the B. pseudomallei/environmental isolate ratio when the bacteria were grown alone relative to the B. pseudomallei/environmental isolate ratio when the bacteria were grown in mixed culture was used to establish the overall competitive index. For Gram-negative environmental strains that could not easily be differentiated from B. pseudomallei Bp82 by pigmentation or β-galactosidase production, selective agar medias were incorporated. R2A agar plates were utilized to select against Bp82 and LB agar plates with adenine HCl, thiamine HCl, Km, and Pm were used to select against Gram-negative competitors. Three independent pairs of cultures were performed for each B. pseudomallei-environmental isolate competition assay, and the results were recorded as the mean ± the standard deviation.
β-galactosidase assays
B. pseudomallei strains harboring hmqD-lacZ and scmR-lacZ transcriptional fusions were inoculated into LB broth and grown overnight, diluted 1:100 in LB broth, and 1 ml aliquots were removed at 5 h and assayed for β-galactosidase activity as described previously (Miller, 1972). When indicated, synthetic N-octanoyl-L-homoserine lactone (C8-HSL; Sigma-Aldrich, St. Louis, MO), N-3-hydroxydecanoyl-L-homoserine lactone (3OHC10-HSL; Sigma-Aldrich, St. Louis, MO), and N-(3-hydroxy-octanoyl)-homoserine lactone (3OHC8-HSL; Cayman Chemical Company, Ann Arbor, MI)) were added to the culture medium of CM139 derivatives at a final concentration of 5 μM. AHLs were resuspended in acidified ethyl acetate at 10 mg/ml and stored at −20°C.
Quantification of quinolones in bacterial cultures
Overnight cultures of Bp82, Bp82 ΔhmqD, Bp82 ΔscmR, and CM139 (150 μl) were inoculated into 10 ml of LB medium in sterile 50 ml polypropylene tubes with screw caps (VWR). The caps of the tubes were loosely opened by a 180-degree turn and fixed in this position with tape to ensure equal oxygen supply. The cultures were incubated at 37°C and 180 rpm. The samples (1 ml) were collected at 1.5, 3.0, 4.5, 6.0, 7.5, 9.0, and 24 h of incubation, centrifuged at 4500 rpm for 10 min, and supernatants were sterile filtered. Three hundred microliters of culture supernatant were added to 1.5 ml glass vials (LABSOLUTE, Art. Nr. 7612960) with caps containing a PTFE membrane (LABSOLUTE, Art. Nr. 7623097) and 300 μl of ethyl acetate (EtOAc) was added and vortexed for 5 s. After separation of organic and water phases, 100 μl of the EtOAc layer were transferred via pipetting into mass spectrometry vials containing a glass insert (MACHEREY-NAGEL, Art. Nr. 702007). The EtOAc was evaporated by a gentle stream of nitrogen. For liquid chromatography with tandem mass spectrometry (LC-MS/MS) analysis, 100 μl of sample solvent (MeOH/H2O 1:1) was added into glass inserts and the residue redissolved. The experiment was performed in biological triplicates.
Bacterial survival and replication within the RAW264.7 murine macrophage-like cell line
RAW264.7 cells were infected at a MOI of 10:1 bacteria/cell. After a 2 h incubation at 37°C with 5% CO2, the cells were washed with PBS (Thermo Fisher Scientific) and fresh medium containing 1 mg/ml Km was added to kill the extracellular bacteria. The infected RAW 264.7 cells were incubated for an additional 10 h and were lysed with 0.1% SDS at designated time points and the number of intracellular bacteria were enumerated by serial dilution and plating on LB agar. The experiment was performed in biological triplicates and the results were recorded as the mean ± the standard deviation.
Bacterial virulence in BALB/c mice
Groups of 6–8 week old BALB/c mice (n = 10) were infected intraperitoneally with increasing doses of 103–106 CFU of B. pseudomallei 1026b or B. pseudomallei 1026b ΔhmqD. Bacteria were grown overnight in a 37°C shaker at 200 rpm in LB broth and the inoculum counts were verified by serial dilution and plated on LB agar. Infected animals were monitored several times a day for 28 days to evaluate for morbidity or mortality. At the completion of the experiment, spleens from three randomly selected survivors per group were homogenized, diluted in PBS, and spread onto Sheep Blood Agar to assess bacterial colonization of the spleen.
Inhibition of quinolone production in B. pseudomallei Bp82
An overnight culture (45 μl) of B. pseudomallei Bp82 was inoculated into 3 ml of LB broth in a sterile 15 ml polypropylene centrifuge tube with a screw cap (VWR). Compound 4 (3 μl) was added from a DMSO stock, to reach its final concentration of either 1 or 0.1 μM. DMSO (3 μl) was added as a control. The caps of the tubes were loosely opened by a 180-degree turn and fixed in this position with tape to ensure equal oxygen supply. The cultures were incubated at 37°C, 180 rpm, for 6.5 h and reached a 1.28 average OD600 value. After the incubation, the samples (3 ml) were centrifuged at 4500 rpm for 10 min, and supernatants were sterile filtered. Three hundred microliters of culture supernatant were added in 1.5 ml glass vials (LABSOLUTE, Art. Nr. 7612960) with caps containing a PTFE membrane (LABSOLUTE, Art. Nr. 7623097) and 300 μl of EtOAc were added and vortexed for 5 s. After separation of organic and water phases, 100 μl of the EtOAc layer were transferred via pipetting into mass spec vials containing a glass insert (MACHEREY-NAGEL, Art. Nr. 702007). The EtOAc was evaporated by a gentle stream of nitrogen. For LC-MS/MS analysis, 100 μl of sample solvent (MeOH/H2O 1:1) was added into glass inserts and the residue redissolved. The experiment was performed in biological triplicates.
LC-MS/MS analysis
LC-MS/MS analysis was performed as described by Prothiwa et al. (Prothiwa et al., 2021) and quinolone standards were synthesized as described by Szamosvari et al. (Szamosvári et al., 2020). Ultra-high performance liquid chromatography was performed on a Vanquish™ UHPLC system (Thermo Fisher Scientific) using a Nucleodur C18 Gravity-SB 100 x 2 mm, 3 μm column (Macherey-Nagel). The flow rate was 0.5 ml min–1 and the column temperature was held at 40°C. The injection volume was 5 μl. Eluent A was 0.1% formic acid in water and eluent B was 0.1% formic acid in acetonitrile. The gradient was 20–100 % B in 10 min, 100 % B for 2 min, 100–20 % B in 1 min, and 20 % B for 2 min. MS/MS analysis was performed by TSQ® Series II Quantis (Thermo Fisher Scientific) mass spectrometer. A heated electrospray ionization (HESI-II probe, Thermo Scientific) was used as an ion source. In the optimized conditions the ion spray voltage was 3500 V, vaporizer temperature 300°C, ion transfer tube temperature 380°C, sheath gas pressure 60 psi, ion sweep gas pressure 2 psi, and aux gas 10 psi. The fragmentation pattern of quinolone standards was acquired in a Product Ion Scan mode using a fixed collision energy of 30 V to fragment the corresponding precursor ion before recording the fragments in a mass range of m/z 130–350. Quinolones we quantified in Selected Reaction Monitoring scan mode. MS/MS spectra were acquired in a positive mode. The software Quan Browser Thermo Xcalibur was used for quantitative analysis.
Statistical analyses
Three independent pairs of cultures were performed for each coculture competition assay and the results were recorded as the mean ± the standard deviation. A statistical analysis of the results involved ANOVA and pairwise group comparisons. The β-galactosidase assays, the production of Δ2-MNQ, MNQ, and Δ2-MNQNO, and the RAW 264.7 intracellular assays were conducted in triplicate and were recorded as the mean ± the standard deviation. The 1026b LD50 in mice was estimated under a Probit Model with Log transformation of the dose variable. The survival rates at selected time points were compared by Fisher exact test and the times to death (TTD) were analyzed by Log-rank test for the pairwise comparison between the challenged groups. Analysis was implemented using SAS version 9.4.
Results
Isolation and characterization of Gram-negative environmental bacteria for competition studies
B. pseudomallei is a saprophytic organism that encounters microbial competitors in the soil, water, and rhizosphere in many regions around the world (Inglis and Mayo, 2012; Kaestli et al., 2012; Limmathurotsakul et al., 2012; Petras et al., 2023). In a previous study, environmental bacteria were isolated from diverse sources, including soil (S), river water (R), river sediment (RS), stream water (ST), and the rhizosphere (RZ), and Gram-positive bacteria were evaluated for their ability to compete with B. pseudomallei (Mou et al., 2021). A similar strategy was employed here to isolate additional environmental bacteria and examine the competitive interactions between Gram-negative isolates and B. pseudomallei. Such experiments cannot replicate the natural environment of the competitors but can provide information about the bacterial factors that facilitate competition under specific laboratory conditions. Bacteria that could be easily distinguished from B. pseudomallei colonies on solid medium due to colony morphology, pigmentation, and/or β-galactosidase production were isolated from all environmental sources except soil. In addition, multiple bacterial colonies that could not be easily differentiated from B. pseudomallei were also retained and further characterized. When combined with the environmental bacteria isolated previously (Mou et al., 2021), a total of 39 distinct Gram-negative bacterial species were identified. Figure 1 shows the evolutionary relationships of these bacteria and demonstrates that there were 32 isolates in the phylum Pseudomonadota and 7 isolates in the phylum Bacteroidota (Oren and Garrity, 2021).
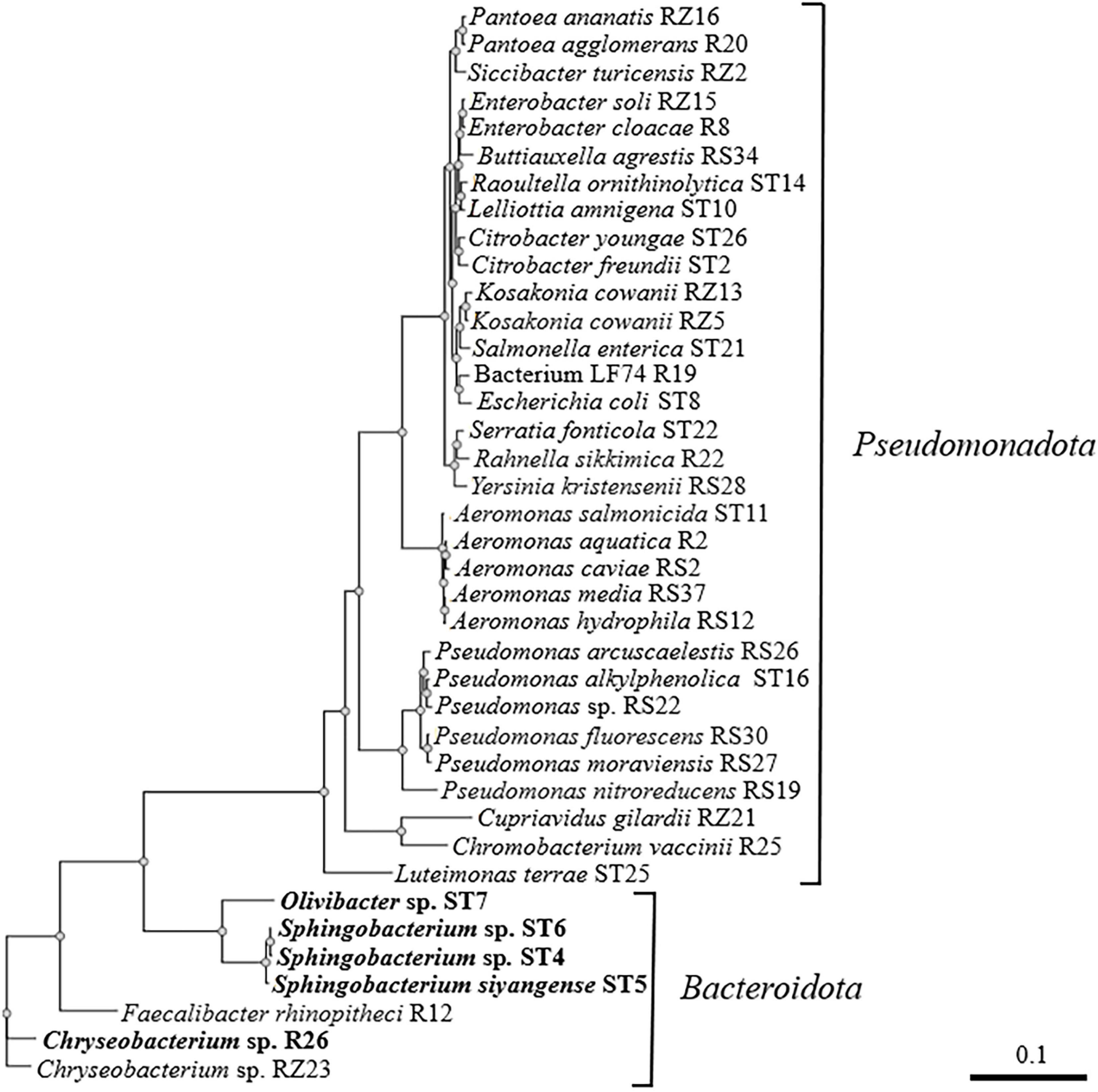
Figure 1. Phylogenetic tree based on partial 16S rRNA sequences of Gram-negative bacterial isolates from river water (R), river sediment (RS), stream water (ST), and rhizosphere (RZ) samples. NGPhylogeny.fr was used to build the tree in “FastME/OneClick” mode and it utilized MAFFT for multiple alignment, BMGE for automatic alignment curation, FastME for tree inference, and Newick Display for tree rendering (Lemoine et al., 2019). The bacterial strains that are inhibited by B. pseudomallei Bp82 in both zone of inhibition and coculture competition assays are shown in bold font. The scale bar indicates the number of substitutions per site.
An initial antimicrobial activity assay was utilized to identify environmental bacteria that produced a zone of inhibition (clearing) when grown as an agar lawn in the presence of B. pseudomallei. Strain Bp82 formed a zone of clearing on agar lawns of 10 environmental Gram-negative bacteria, including Aeromonas hydrophila RS12, Pseudomonas arcuscaelestis RS26, Pseudomonas moraviensis RS27, Faecalibacter rhinopitheci R12, Chryseobacterium sp. R26, Sphingobacterium sp. ST4, Sphingobacterium siyangense ST5, Sphingobacterium sp. ST6, Olivibacter sp. ST7, and Chryseobacterium sp. RZ23 (Table 1). By comparison, 29/39 (∼74%) of the Gram-negative bacteria did not produce a zone of inhibition when grown in the presence of Bp82 (data not shown).
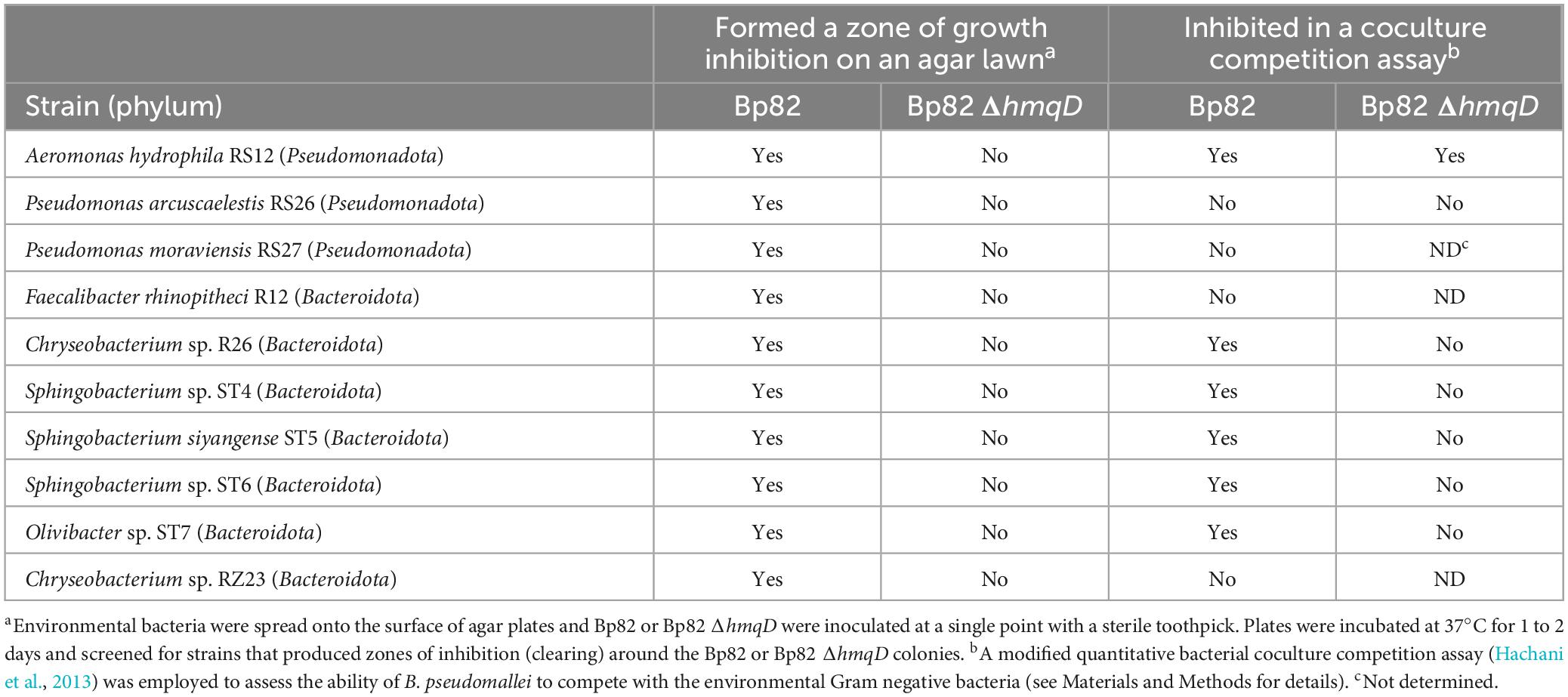
Table 1. In vitro competition assays used to identify environmental bacteria inhibited by B. pseudomallei.
TnMod-OKm’ mutagenesis of B. pseudomallei Bp82 identifies two distinct loci required for inhibiting the growth of Sphingobacterium sp. ST4
Mou et al. developed a TnMod-OKm’ screen to identify B. pseudomallei mutants that did not inhibit the growth of the Gram-positive bacterium Neobacillus bataviensis S4 (Dennis and Zylstra, 1998; Mou et al., 2021). A similar strategy was used in this study to identify Bp82 mutants that could not form a zone of clearing on the Gram-negative bacterium Sphingobacterium sp. ST4. Approximately 6,000 Bp82 transposon mutants were screened, and five, termed CCM1, CCM2, CCM6, CCM7, and CCM9, did not exhibit antimicrobial activity against ST4 (Figure 2A). CCM1 and CCM2 contained transposon insertions in scmR (Figure 2B), a LTTR that serves as a global regulator of BGCs in B. pseudomallei and B. thailandensis (Klaus et al., 2018; Mao et al., 2017). The sites of TnMod-OKm’ insertions in CCM6, CCM7, and CCM9 were mapped to the hmqA-G locus (Figure 2C), which encodes the biosynthetic enzymes involved in the production of AQs, AQNOs, MAQs and MAQNOs in B. pseudomallei, B. thailandensis, and some members of the Burkholderia cepacia complex (Diggle et al., 2006; Klaus et al., 2020a; Klaus et al., 2020b; Mou et al., 2021; Piochon et al., 2020; Szamosvári et al., 2020; Vial et al., 2008; Figure 2D). Bp82 strains with defined deletion mutations in hmqD and scmR, Bp82 ΔhmqD and Bp82 ΔscmR (Supplementary Table 1), also did not produce zones of inhibition on a ST4 lawn (Supplementary Figure 1). The full-length scmR gene was cloned into the broad-host-range vector pBHR2 and conjugated to Bp82 ΔscmR in an attempt to complement the ΔscmR mutation. The result demonstrated that Bp82 ΔscmR (pBHR2-scmR) formed a zone of clearing on a ST4 lawn, but Bp82 ΔscmR (pBHR2) did not (Supplementary Figure 2). The fact that Bp82 ΔscmR could be complemented by providing scmR in trans suggests that the ΔscmR mutation does not have a polar effect on downstream genes, including ldhA (Figure 2B). Importantly Bp82 ΔhmqD could also be complemented by providing full length hmqD on pBHR2 (Mou et al., 2021). Further experiments were conducted with the other Gram-negative bacteria that produced zones of inhibition on agar lawns with Bp82 and the results indicated that formation of inhibition zones were strictly dependent on a functioning copy of hmqD (Table 1). Taken together, the results show the hmqA-G operon and the regulatory gene scmR are involved in the ability of Bp82 to form zones of clearing on agar lawns of ten distinct environmental Gram-negative species.
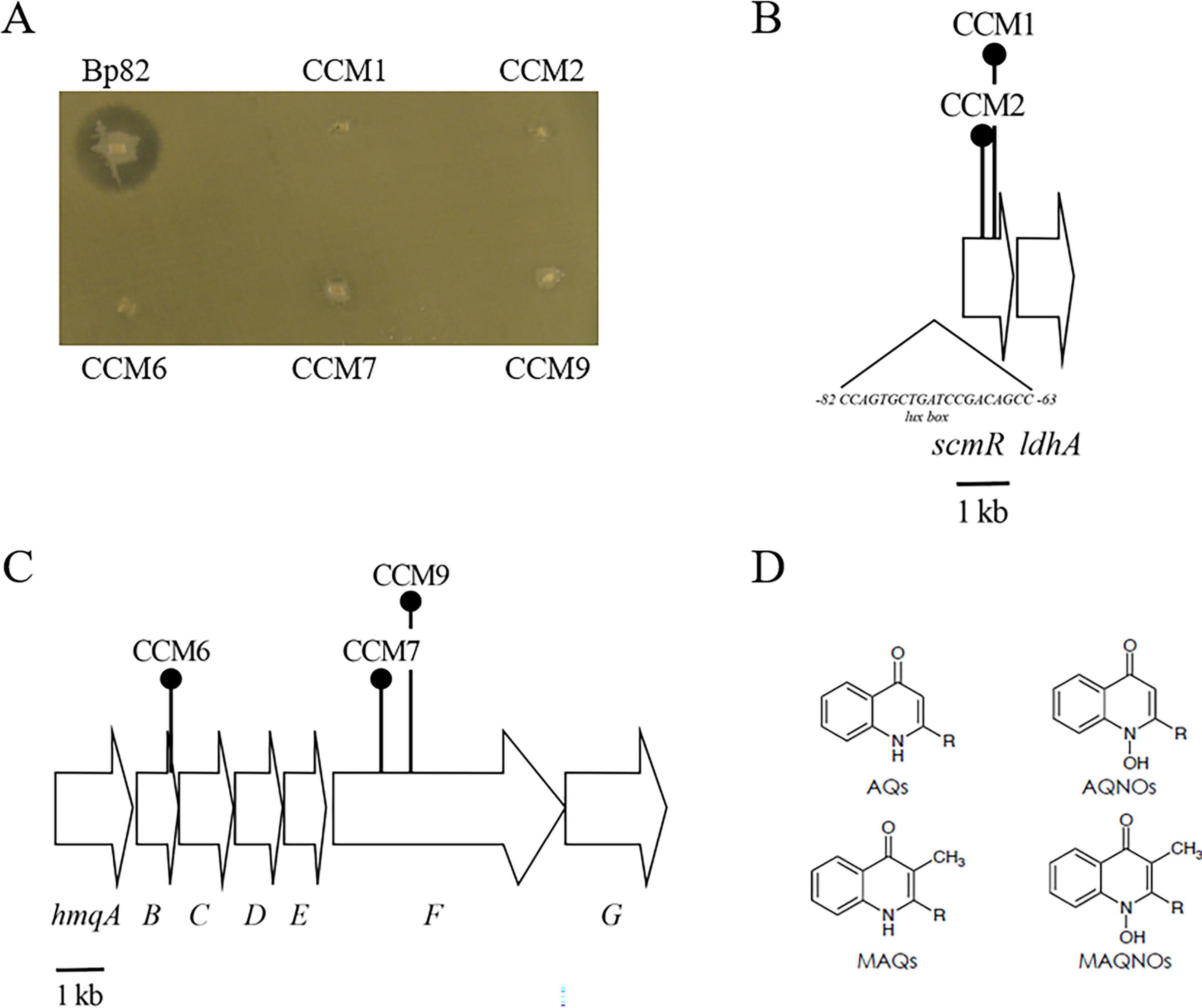
Figure 2. The B. pseudomallei hmqA-G locus and the scmR gene are required for antimicrobial activity against Sphingobacterium sp. ST4. (A) Bp82 produces a zone of inhibition on a lawn of ST4, but CCM1, CCM2, CCM6, CCM7, and CCM9 are unable to produce such zones. The inoculated LB agar plate was incubated for 24 h at 37°C. (B) Genetic map of the scmR and ldhA genes, locus tags BP1026B_I0582 - BP1026B_I0581, in B. pseudomallei 1026b. The location of a putative lux box at −82 to −63 nucleotides upstream of the scmR start codon is also shown (Le Guillouzer et al., 2020). The scmR gene encodes a LTTR and ldhA encodes a putative lactate dehydrogenase. A 1 kilobase (kb) scale is shown at the bottom. (C) Genetic map of the hmqA-G gene cluster, locus tags BP1026B_II0535 - BP1026B_II0541, in B. pseudomallei 1026b. The location and direction of transcription of genes are represented by arrows. The gene products of this locus are required for the biosynthesis of AQs and MAQs. The locations of TnMod-OKm’ insertions in CCM6, CCM7, and CCM9 are shown schematically by round-top push pins. The unlinked hmqL gene required for generating AQNOs and MAQNOs (Klaus et al., 2020b), locus tag BP1026B_II2272 in 1026b, is not shown. (D) Chemical structure of AQs, AQNOs, MAQs, and MAQNOs encoded by the B. pseudomallei hmqA-G locus. The R represents the presence of unsaturated or saturated alkyl groups of seven to eleven carbons at the 2-position.
B. pseudomallei outcompetes bacteria in the phylum Bacteroidota in zone of inhibition and coculture competition assays
As demonstrated above, Bp82 was able to form a zone of clearing on 100% of the bacteria in the Bacteroidota phylum and 9% of the bacteria in the Pseudomonadota phylum (Figure 1 and Table 1). To further assess the interaction of B. pseudomallei with the 10 environmental Gram-negative bacteria that were inhibited in a hmqD-dependent fashion in coculture competition assays, a quantitative bacterial competition assay was employed (Hachani et al., 2013). Briefly, B. pseudomallei and environmental competitors were grown in liquid broth and 10 μl aliquots of each were spotted onto the surface of agar media (Figure 3A). Twenty-microliter aliquots of 1:1 mixtures of B. pseudomallei and environmental competitors were also spotted onto the solid medium and incubated for 48 h at RT. The competition results were quantitated by resuspending the bacterial spots in PBS, performing serial dilutions, and spreading aliquots onto agar plates. The quantity of bacterial competitors present in the competition mixtures was assessed by enumerating the number of B. pseudomallei off-white colonies and comparing it to the number of Gram-negative pigmented or blue colonies. For those environmental bacteria with colony morphotypes that could not be readily differentiated from B. pseudomallei, selective agar media was used to quantitate the competitors (see Materials and Methods). The Bp82/environmental strain solitary growth ratio was compared to the Bp82/environmental strain mixed growth ratio and used to assess the fold difference between the two growth indices. The results of quantitative coculture competition assays between B. pseudomallei Bp82 and the environmental strains are shown in Table 1. B. pseudomallei Bp82 outcompeted Sphingobacterium sp. ST4, S. siyangense ST5, Sphingobacterium sp. ST6, Olivibacter sp. ST7, and Chryseobacterium sp. R26 and the results were dependent on an intact copy of the hmqD gene as the Bp82 ΔhmqD mutant was unable to compete with these Gram-negative species (Figures 3B–F). Interestingly, F. rhinopitheci R12, Chryseobacterium sp. RZ23, P. arcuscaelestis RS26, and P. moraviensis RS27 were inhibited by Bp82 when grown on agar lawns, but were not inhibited by Bp82 in coculture competition assays (Table 1 and Supplementary Figure 3). These species may possess factors that allow them to effectively compete with B. pseudomallei when grown in coculture. Both Bp82 and Bp82 ΔhmqD outcompeted A. hydrophila RS12, indicating that the inhibition of this bacterium requires other factors than the natural quinolones produced by the B. pseudomallei hmqA-G locus. The results demonstrate that only Gram-negative bacteria in the phylum Bacteroidota were inhibited in a hmqD-dependent fashion in both zone of inhibition and coculture competition assays (Figure 1).
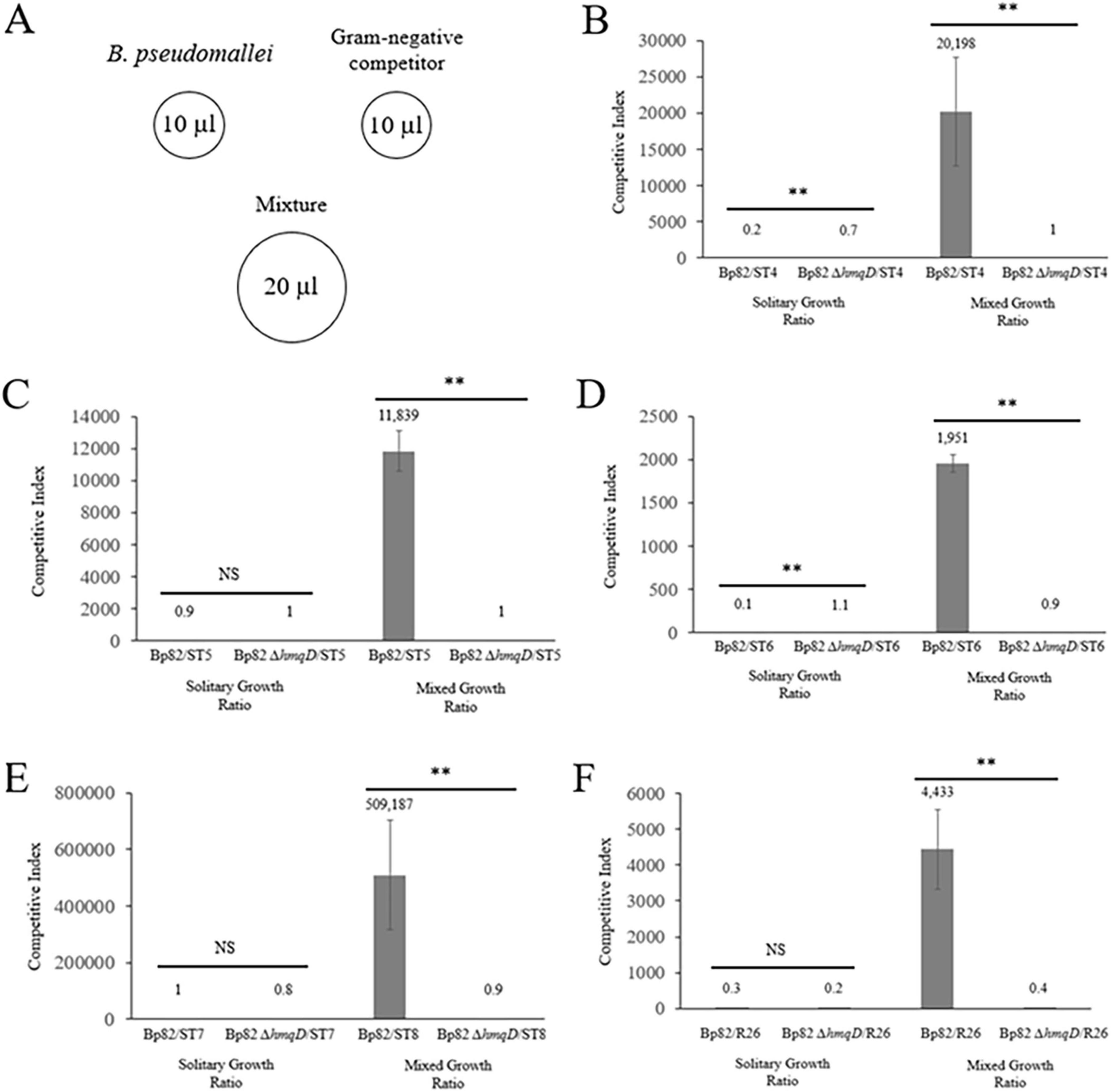
Figure 3. B. pseudomallei outcompetes environmental Gram-negative bacteria in the phylum Bacteroidota in an hmqD-dependent manner. (A) Schematic illustration of coculture competition assays performed with B. pseudomallei Bp82 and (B) Sphingobacterium sp. ST4, (C) Sphingobacterium sp. ST5, (D) Sphingobacterium sp. ST6, (E) Olivibacter sp. ST7, and (F) Chryseobacterium sp. R26. The fold difference between the B. pseudomallei/environmental isolate ratio when the bacteria were grown alone (solitary growth ratio) or in mixed culture (mixed culture ratio) was used to establish the competitive indexes. The bacteria were incubated at RT for 48 h on solid medium and the surviving competitors were quantitated. Three independent pairs of cultures were performed for each competition assay and the results were recorded as the mean ± the standard deviation. A statistical analysis of the results involved ANOVA and pairwise group comparisons. NS, not significant; **, P < 0.0001.
Expression of hmqD in B. pseudomallei is dependent on ScmR, a LTTR that is positively regulated by quorum sensing
Previous studies have examined the expression of hmqA-G and scmR in Burkholderia, with most focusing exclusively on B. thailandensis (Coulon et al., 2019; Le Guillouzer et al., 2020; Klaus et al., 2018; Majerczyk C. et al., 2014; Majerczyk C. D. et al., 2014; Mao et al., 2017; Martinez et al., 2020). Given the importance of these two loci for the competitive interaction of B. pseudomallei with environmental bacteria, the expression of these genes was further evaluated here. The promoterless lacZ gene was fused to hmqD and scmR in B. pseudomallei Bp82 and CM139, a quorum sensing (QS) deficient mutant (Supplementary Table 1). CM139 is a Bp82 derivative with deletions in all three of the AHL synthase genes (bpsI1 to –3). B. pseudomallei produces three N-acyl homoserine lactones (AHLs), C8-HSL, 3OH-C10-HSL, and 3OH-C8-HSL, that are involved in population density-dependent gene expression (Fuqua et al., 2001; Majerczyk C. D. et al., 2014). Figure 4A shows that Bp82 and CM139 only produce background levels of β-galactosidase activity, 24 and 25 Miller units, respectively, because B. pseudomallei strains do not naturally possess this enzyme. Beta-galactosidase assays were conducted with Bp82 hmqD-lacZ and CM139 hmqD-lacZ and the results showed that the transcription of hmqD was decreased by approximately one third in the absence of AHLs (Figure 4A). By comparison, the expression of hmqD in Bp82 hmqD-lacZ and CM139 hmqD-lacZ was decreased by nine- to ten-fold in the absence of scmR (Figure 4A). The results show that QS and ScmR are both important for maximal transcription of the hmqA-G operon, but demonstrate that ScmR plays a more important role in the positive regulation of this locus than QS. When Bp82 scmR-lacZ and CM139 scmR-lacZ were subjected to β-galactosidase assays, the transcription of scmR was decreased by 60% in the absence of AHLs (Figure 4B). Similarly, Klaus et al. found that scmR expression in CM139 was three-fold lower than in Bp82 (Klaus et al., 2018). Taken together, the results indicate that QS positively regulates scmR transcription and ScmR subsequently activates transcription of the hmqA-G locus (Figure 4).
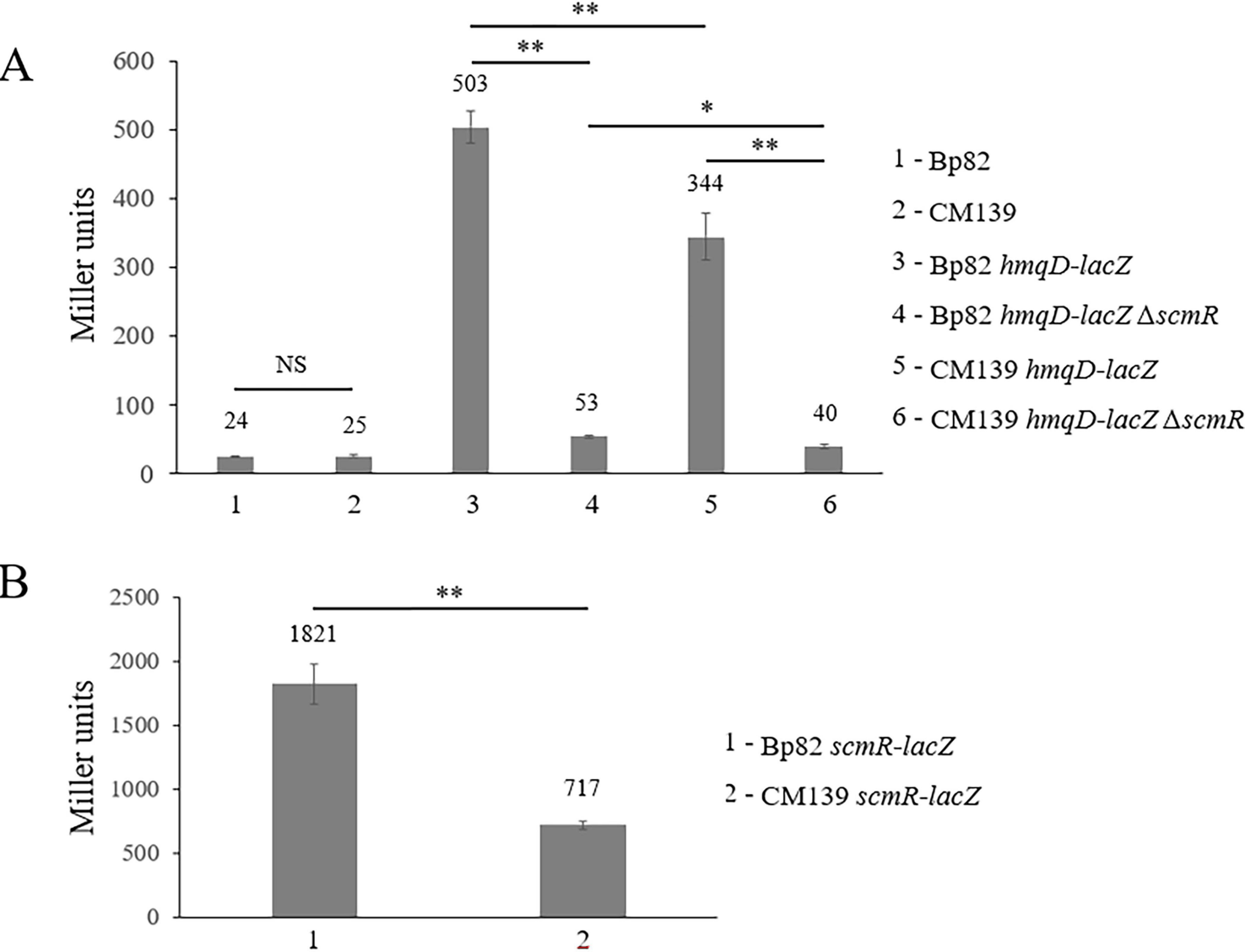
Figure 4. Expression of the B. pseudomallei hmqD gene is positively regulated by ScmR which is activated by quorum sensing. β-galactosidase production by B. pseudomallei strains containing chromosomal promoterless lacZ reporter fusions to assess hmqD (A) and scmR (B) expression during mid-logarithmic phase growth in LB broth. β-galactosidase was measured spectrophotometrically and the Miller units were assessed as described (Miller, 1972). Each numerical value for β-galactosidase production is the mean of experiments performed on at least three separate occasions ± standard deviation (bars). Pairwise treatment groups were compared by negative binomial generalized linear model and no multiplicity adjustment was applied. NS, not significant; *, P < 0.05; **, P < 0.0001.
The B. pseudomallei scmR gene contains a lux box 63–82 bp upstream of the ATG start codon (Figure 2B) which supports the fact that this gene is regulated by QS (Klaus et al., 2018; Le Guillouzer et al., 2020). Each of the three B. pseudomallei AHLs were added to CM139 scmR-lacZ to determine if commercially acquired C8-HSL, 3OH-C10-HSL, or 3OH-C8-HSL could activate the transcription of scmR. The AHLs were individually added to CM139 scmR-lacZ cultures at a final concentration of 5 μM and β-galactosidase assay results were compared to Bp82 scmR-lacZ and CM139 scmR-lacZ cultures grown without AHLs (Figure 5). The AHL C8-HSL, produced by BpsI1 AHL synthase, completely restored scmR transcription in CM139 scmR-lacZ to the expression levels of Bp82 scmR-lacZ (Figure 5A). In fact, CM139 scmR-lacZ with added C8-HSL resulted in approximately 30% higher scmR transcription than in Bp82 scmR-lacZ. The AHL produced by BpsI2 AHL synthase, 3OH-C10-HSL, also restored scmR expression levels in CM139 scmR-lacZ to those present in Bp82 scmR-lacZ (Figure 5B). The BpsI3 AHL synthase product, 3OH-C8-HSL, also increased scmR transcription in CM139 scmR-lacZ to levels that were approximately 23% higher than in Bp82 scmR-lacZ (Figure 5C). The concentration of AHLs added to the CM139 scmR-lacZ growth medium was above physiological concentrations, but allowed for saturation of the three B. pseudomallei QS systems (Majerczyk C. D. et al., 2014). The elevated levels of C8-HSL and 3OH-C8-HSL in the culture medium may be responsible for the increased transcription of scmR in CM139 scmR-lacZ relative to Bp82 scmR-lacZ. The results show that all three AHLs stimulate scmR expression in B. pseudomallei (Figure 5) which is consistent with experiments conducted in B. thailandensis (Majerczyk C. et al., 2014).
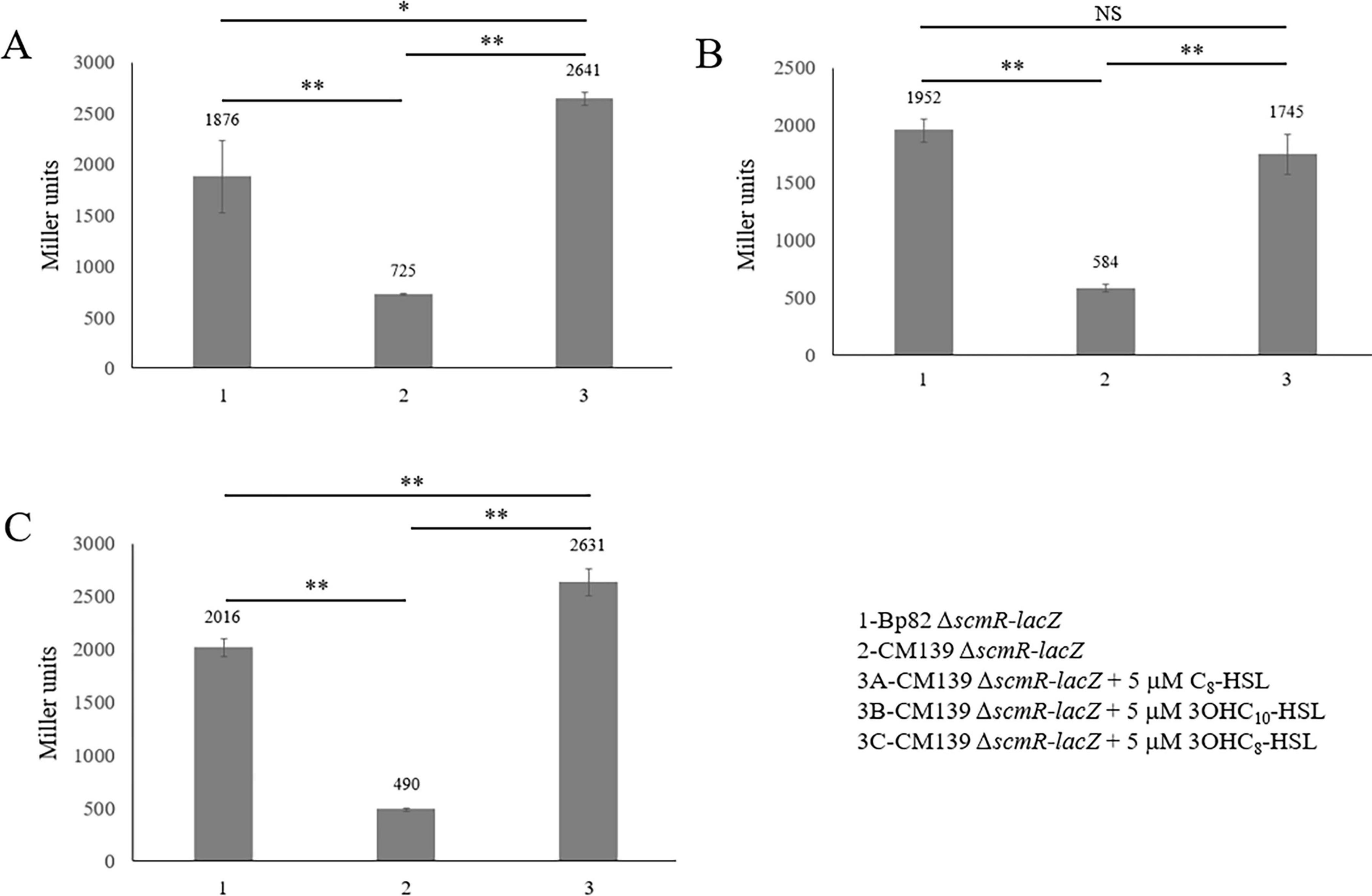
Figure 5. Addition of B. pseudomallei homoserine lactones to growth media increased the expression of scmR-lacZ in the quorum sensing-deficient strain CM139. β-galactosidase production by B. pseudomallei CM139 scmR-lacZ was assessed during mid-logarithmic phase growth in LB broth with HSLs exogenously added to a final concentration of 5 μM. C8-HSL (A), 3OHC10-HSL (B), and 3OHC8-HSL (C). Each numerical value for β-galactosidase production is the mean of experiments performed on at least three separate occasions ± standard deviation (bars). Pairwise treatment groups were compared by negative binomial generalized linear model and no multiplicity adjustment was applied. *, P < 0.005; **, P < 0.0001.
Production of 2-alkylquinolone derivatives by Bp82 is modulated by QS and is dependent on hmqA-G and scmR
LC-MS/MS analysis was performed on culture supernatants of Bp82, Bp82 ΔhmqD, Bp82 ΔscmR, and CM139 to assess the production of AQ, AQNO, MAQ, and MAQNO using the corresponding synthetic standards. Table 2 shows that Bp82 produced a variety of AQ and MAQ derivatives and the results were consistent with LC-MS/MS experiments previously described for B. pseudomallei (Diggle et al., 2006; Mou et al., 2021; Vial et al., 2008). It is important to emphasize that the 2-alkylquinolone molecules that possessed unsaturated alkyl chains contained a double bond at position 2′ rather than position 1′, as previously described (Mou et al., 2021). Bp82 ΔhmqD and Bp82 ΔscmR did not produce any AQ derivatives (Table 2) which confirms the importance of these compounds for B. pseudomallei competition with environmental Gram-negative bacterial species in the phylum Bacteroidota (Figures 2, 3, and Supplementary Figure 1). B. pseudomallei CM139 produced AQs and MAQs; however, compounds with N-oxide groups were not detected (Table 2). A further analysis demonstrated that the growth rates of Bp82 and CM139 in LB broth were nearly identical (Figure 6A) and the production levels of Δ2-MNQ, MNQ, and Δ2-MNQNO were assessed by LC-MS/MS over a 24 h period of growth (Figures 6B–D). All three metabolites were maximally produced by Bp82 during the late logarithmic to early stationary phase of growth followed by a steady decrease in production throughout the stationary phase (Figures 6B–D). The production of Δ2-MNQ and MNQ followed a similar trend in CM139 until the late logarithmic stage of growth when the production of these compounds continued to rise, rather than decrease, throughout the stationary phase (Figures 6B–C). As mentioned above, no Δ2-MNQNO was produced by CM139 during any stage of growth. The LC-MS/MS experiments support the bacterial competition and gene expression results and further demonstrate that the production of AQs, AQNOs, MAQs, and MAQNOs are dependent on QS, ScmR, and the products of the hmqA-G locus. The data also suggest that the unlinked gene hmqL, encoding a FAD-dependent monooxygenase required for the synthesis of AQNOs and MAQNOs, may also be regulated in a QS-dependent fashion (see below also).
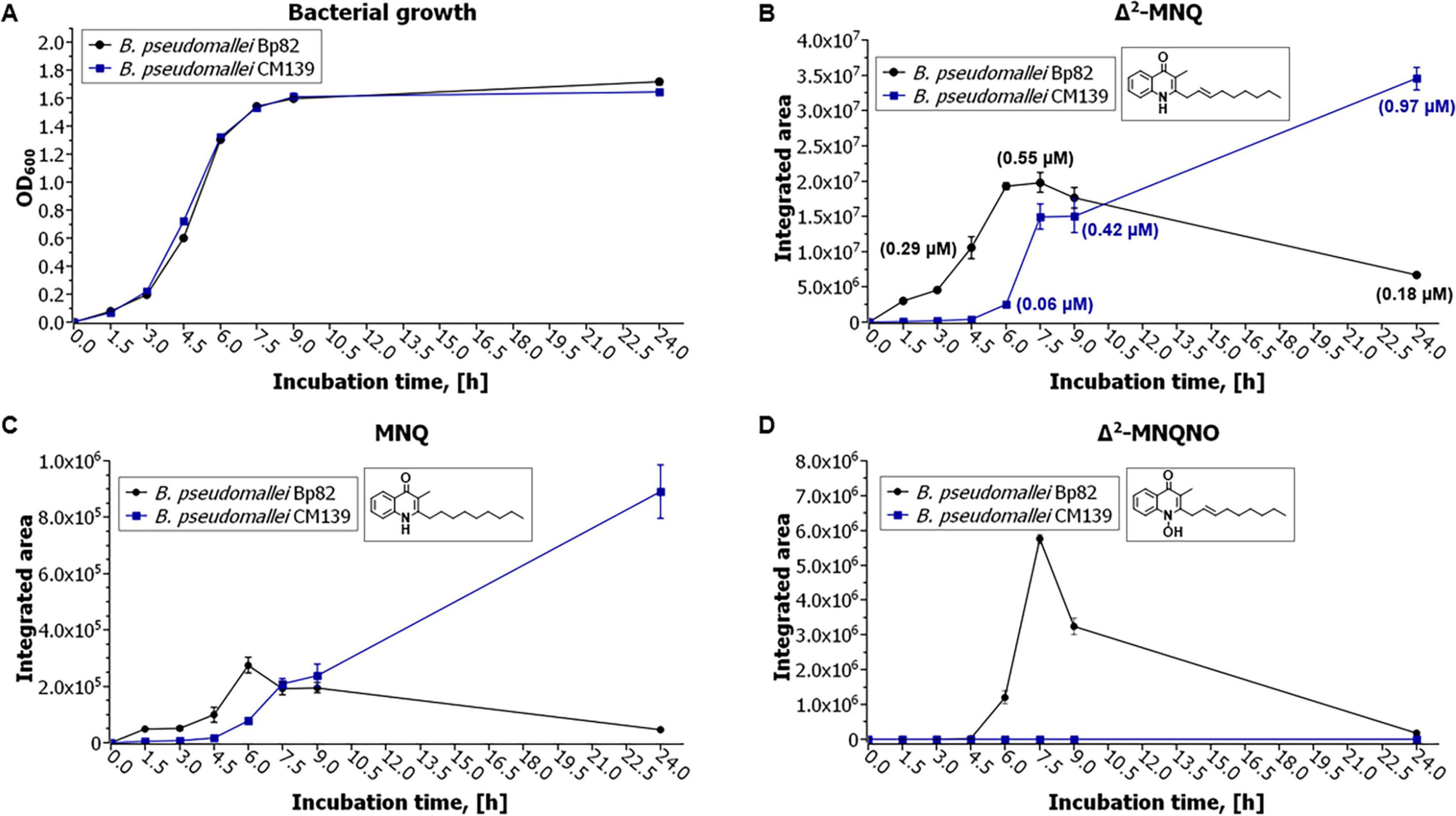
Figure 6. Growth and production of Δ2-MNQ, MNQ, and Δ2-MNQNO by B. pseudomallei Bp82 and CM139. (A) Bacterial growth curves of Bp82 (black) and CM139 (blue). The production levels of Δ2-MNQ (B), MNQ (C), and Δ2-MNQNO (D) by Bp82 (black) and CM139 (blue) during growth at 37°C for 24 h. Mean values are given for biological triplicates with the error bars representing the population standard deviation. The only time point that not statistically significant (p ≤ 0.05) for (B) was 9 h and for (C) was 7.5 h and 9 h.
B. pseudomallei 1026b ΔhmqD exhibits reduced intracellular replication in RAW264.7 cells and diminished virulence in the BALB/c animal model of melioidosis
Aiosa et al. recently demonstrated that B. thailandensis produced AQ derivatives when grown inside RAW264.7 murine macrophage cells, but the importance of these compounds for replication and/or survival in this niche was not assessed (Aiosa et al., 2022). The ΔhmqD mutation was introduced into B. pseudomallei 1026b (Supplementary Table 1), the virulent parental strain of Bp82, and used to infect RAW264.7 cells. Figure 7A shows that survival and replication of 1026b and 1026b ΔhmqD were not significantly different during the first 3 h of infection, but by 12 h post infection there was a 94-fold reduction in the number of intracellular 1026b ΔhmqD bacteria relative to 1026b. This result indicates that AQ derivatives are required for optimal replication inside RAW264.7 cells, but the mechanism by which this occurs is currently unknown.
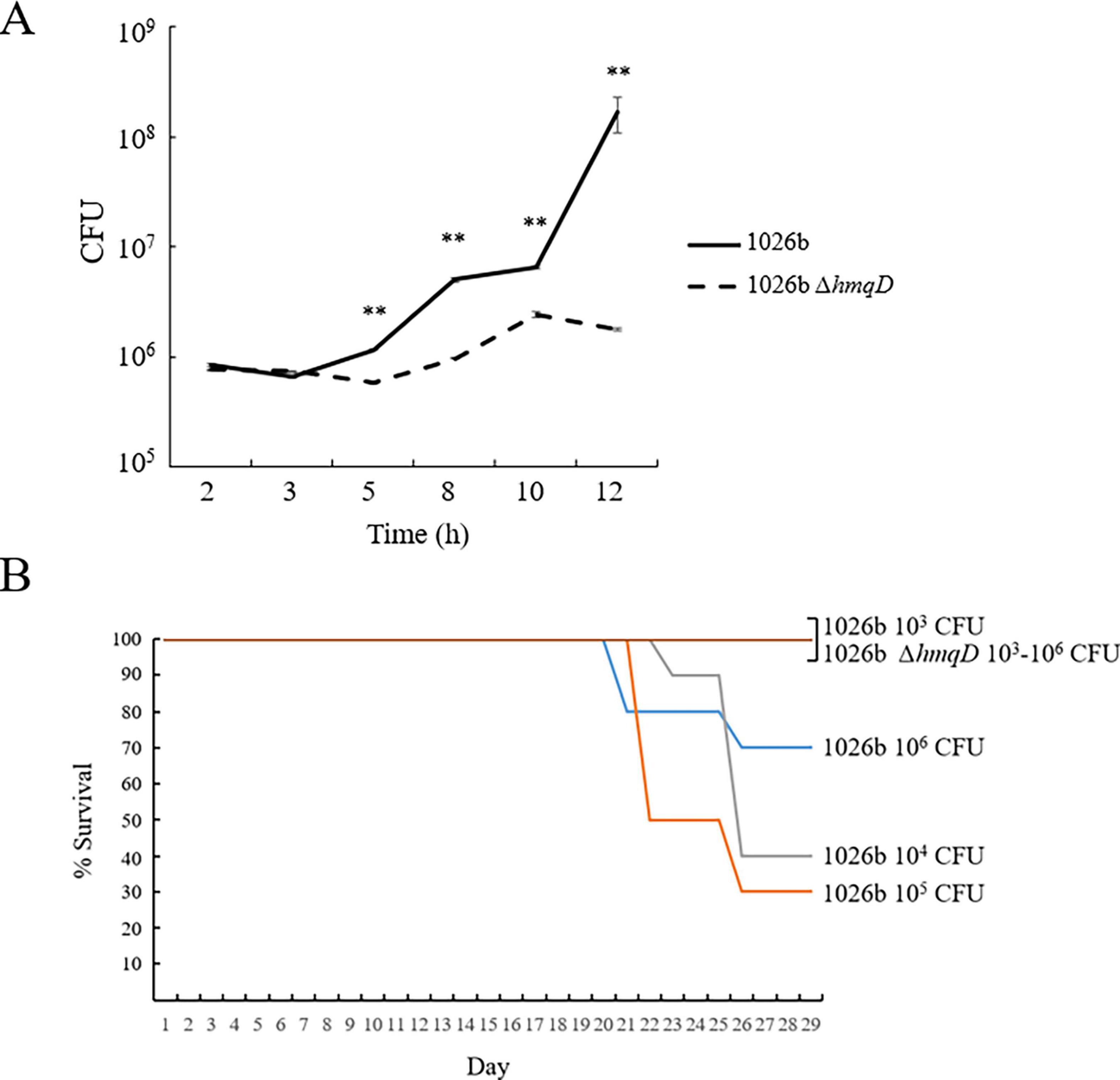
Figure 7. B. pseudomallei 1026b ΔhmqD exhibits reduced intracellular replication in RAW264.7 cells and reduced virulence in the BALB/c animal model of melioidosis. (A) RAW264.7 cells were infected with 1026b and 1026b ΔhmqD at a MOI of 10 and intracellular bacteria were enumerated for 12 h post infection. Each numerical value for CFU is the mean of experiments performed on at least three separate occasions ± standard deviation (bars). Pairwise treatment groups were compared by negative binomial generalized linear model. No multiplicity adjustment was applied. **, P < 0.0001. (B) Ten BALB/b mice were infected intraperitoneally with 103–106 CFU of 1026b and 1026b ΔhmqD and evaluated for morbidity and mortality for 28 days. The survival rates of mice that received 1026b ΔhmqD were greater than those that received 1026b, with p = 0.0108 at 104 CFU and p = 0.0031 at 105 CFU, respectively. The log-rank test p-value of Time to Death (TTD) for 1026b versus 1026b ΔhmqD was significant at 104 CFU (p = 0.0046) and 105 CFU (p = 0.0012), but not significant at 103 CFU and 106 CFU (p = 0.0669).
B. pseudomallei 1026b and 1026b ΔhmqD were also examined for their relative virulence in the BALB/c mouse model of infection (Figure 7B). Groups of 10 mice were infected via the intraperitoneal route with 103, 104, 105, and 106 CFU of 1026b and 1026b ΔhmqD and monitored for 28 days. All mice that received 1026b ΔhmqD survived during the period of observation, but morbidity and mortality was pronounced in animals that received 104, 105, and 106 CFU of 1026b (Figure 7B). These mice exhibited noticeable reduced grooming and rough fir at two to three weeks postinfection, but all animals infected with 1026b ΔhmqD exhibited a normal appearance. The spleens from animals surviving challenge with 1026b contained > 105 CFU/g at the end of the experiment. By comparison, the spleens from mice challenged with 1026b ΔhmqD harbored no detectable CFU, indicating complete immunological clearance of the mutant strain by day 28. The LD50 of 1026b and 1026b ΔhmqD was determined to be 3 x 105 CFU and > 1 x 106 CFU, respectively. This represents the first time that a mammalian model of infection has been utilized to demonstrate that the hmqA-G locus is important for B. pseudomallei virulence. Further studies will be required to reveal if there is a direct link between the 1026b ΔhmqD macrophage replication defect and the decreased virulence in vivo or if there is no connection between these biological phenomena.
A synthetic chemical inhibitor of the central quinolone biosynthesis enzyme HmqD blocks MAQ and MAQNO production in B. pseudomallei Bp82
Prothiwa et al. previously developed chemical inhibitors of the B. ambifaria HmqD enzyme binding covalent into the active site that were able to prevent quinolone production in this species at micromolar concentrations (Prothiwa et al., 2021). One of these synthetic inhibitors, 1-(2-amino-5-iodophenyl)-2-chloroethan-1-one (compound 4), was further examined here to see if it could also prevent quinolone production in B. pseudomallei Bp82. Bacterial broth cultures were grown aerobically with compound 4 at final concentrations of 1 and 0.1 μM and the filtered supernatants were assessed for quinolone production via LC-MS/MS analysis (Figure 8). The fold change of quinolone production was calculated with respect to a DMSO control. Compound 4 significantly inhibited the production of Δ2-MNQ (Figure 8A), Δ2-MNQNO (Figure 8B), MNQ (Figure 8C), and MNQNO (Figure 8D) in B. pseudomallei at both concentrations; however, all quinolones were inhibited more efficiently by the higher concentration except MNQ. Given the fact that the hmqA-G locus is important for virulence in the host (Figure 7B), the inhibition of quinolone production by chemicals like compound 4 could represent a novel therapeutic strategy against B. pseudomallei. While a preliminary study with compound 4 indicated that it is cytotoxic for HepG2 cells (data not shown), the future development of nontoxic HmqD inhibitors should allow their evaluation as anti-infectives in the murine model of melioidosis.
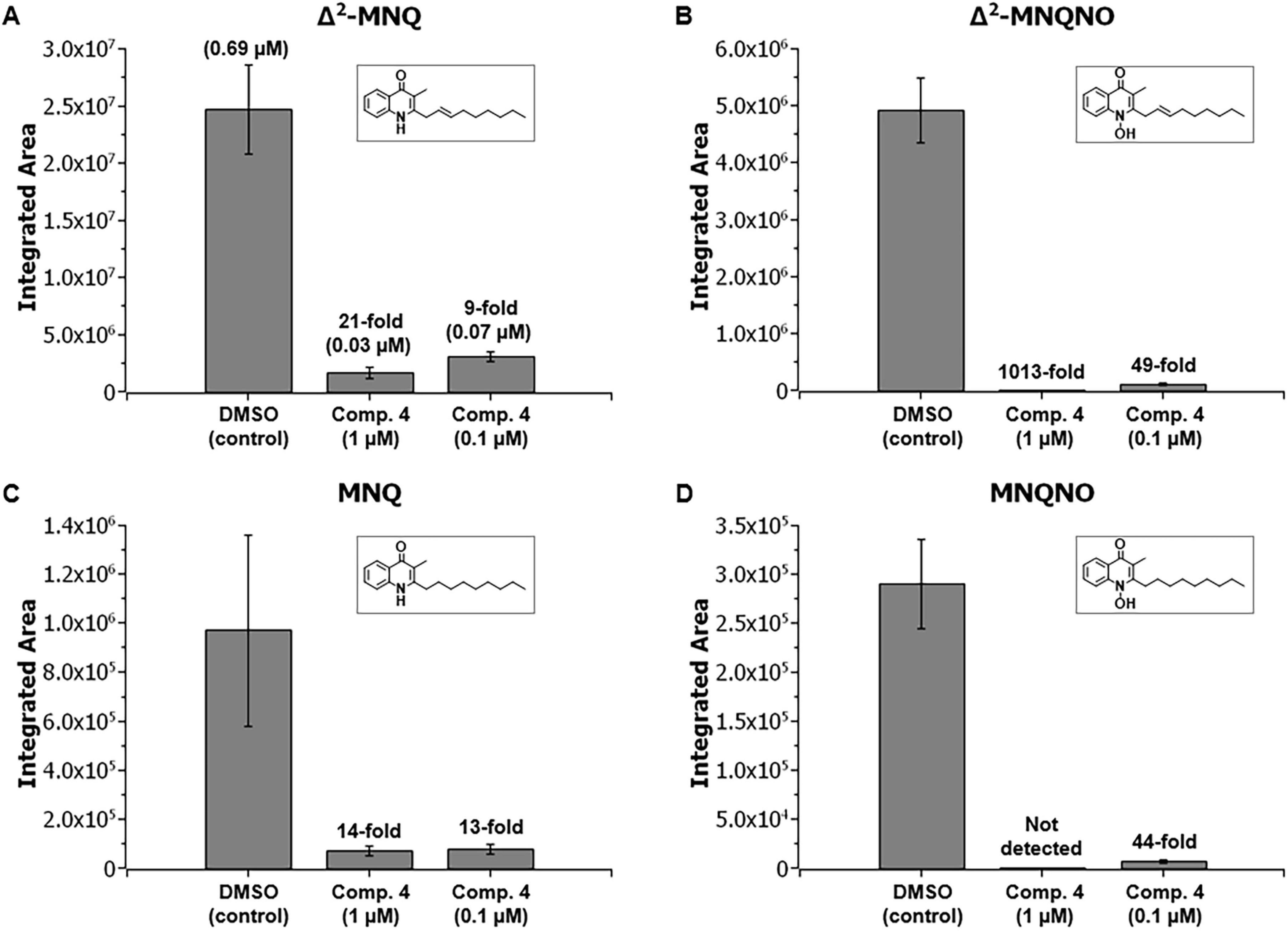
Figure 8. B. pseudomallei Bp82 production of MAQs and MAQNOs was inhibited by a compound that specifically targets the quinolone biosynthesis enzyme HmqD. Δ2-MNQ (A), Δ2-MNQNO (B), MNQ (C), and MNQNO (D) production inhibition in Bp82 after treatment with 1-(2-amino-5-iodophenyl)-2-chloroethan-1-one, compound 4 (Prothiwa et al., 2021) at 1 and 0.1 μM final concentration. Fold change is calculated with respect to DMSO control. Mean values are given for biological triplicates with the error bars representing the population standard deviation.
Discussion
B. pseudomallei is an opportunistic pathogen that resides in soil and water in tropical and subtropical regions and humans are infected by inhalation or contact with contaminated environmental sources (Inglis and Mayo, 2012; Limmathurotsakul et al., 2016; Limmathurotsakul et al., 2012; Meumann et al., 2024). The inherent ability of B. pseudomallei to persist in the environment is a key reason why melioidosis continues to be an important infectious disease around the world. Mou et al. demonstrated that B. pseudomallei AQ derivatives are required for optimal competitive fitness with environmental Gram-positive bacteria and the results presented here show that these metabolites are also important for competition with Gram-negative bacteria in the Bacteroidota phylum (Mou et al., 2021). Bacteria in the Pseudomonadota phylum employ UQ for aerobic respiration, but Gram-positive bacteria and members of the Bacteroidota phylum only utilize DMK and/or MK for aerobic respiration (Marreiros et al., 2016; Schoepp-Cothenet et al., 2013; Zhi et al., 2014). Isoprenoid quinones such as UQ, DMK, and MK are lipid-soluble electron carriers in the cytoplasmic membrane and function as mobile redox carriers in the respiratory chain. The two major types of isoprenoid quinones are benzoquinones (UQ, plastoquinone, and rhodoquinone) and naphthoquinones (MK and DMK) (Abby et al., 2020; Nowicka and Kruk, 2010). They are composed of a hydrophilic head group and an apolar isoprenoid side chain that can vary in length and saturation. Naphthoquinones are the oldest quinones that first appeared when the earth contained a more reducing atmosphere. Benzoquinones, on the other hand, arose after the appearance of photosynthetic organisms and the corresponding increase in oxygen levels. They have a higher midpoint redox potential than naphthoquinones and are ideal for aerobic metabolism. Many members of the Pseudomonadota phylum, like E. coli, contain UQ, DMK, and MK, but use UQ for aerobic respiration and DMK and MK for anaerobic respiration. Gram-positive bacteria and Gram-negative bacteria in the Bacteroidota phylum do not synthesize UQ and must use DMK and MK for aerobic respiration. It has been proposed that Burkholderia MAQNOs structurally resemble the MK/MKH2 redox couple that shuttles electrons in the respiratory chain and they impair ATP synthesis and growth by competitive inhibition of key respiratory enzymes (Nowicka and Kruk, 2010; Szamosvári et al., 2020). The results reported here support that hypothesis and suggest that environmental bacteria that only produce naphthoquinones for aerobic metabolism are more likely to be inhibited by B. pseudomallei in zone of inhibition and coculture competition assays than bacteria that produce benzoquinones or benzoquinones and naphthoquinones (Figure 3 and Table 1). The predominant respiratory quinones produced by the environmental Bacteroidota isolates in this study are MK-6 (R12, R26, and RZ23) and MK-7 (ST4, ST5, ST6, and ST7) (Collins and Jones, 1981; Ntougias et al., 2007; Wang et al., 2021). All of these bacteria were inhibited by the products of the B. pseudomallei hmqA-G locus in zone of inhibition assays (Table 1). R12 and RZ23, on the other hand, were the only Bacteroidota isolates not inhibited in coculture competition assays (Supplementary Figure 3). It is possible that these bacteria inhibit the production or export of AQs by B. pseudomallei. While no environmental Pseudomonadota isolates were identified that were inhibited by the B. pseudomallei hmqA-G locus in both zone of inhibition and coculture competition assays (Table 1), only 32 strains were evaluated and it is possible that such isolates could be identified in a more exhaustive environmental survey.
Transposon mutagenesis was used to identify two distinct loci in Bp82 that were responsible for competitive fitness in coculture with environmental Gram-negative bacteria in the Bacteroidota phylum. Three mutations were identified in the hmqA-G locus and two mapped to the scmR regulatory gene (Figure 2). LC-MS/MS experiments confirmed that both loci are absolutely essential for the production of AQs, AQNOs, MAQs, and MAQNOs (Figure 2D and Table 2). The secondary metabolite regulator, ScmR, is a LTTR that was originally identified in B. thailandensis (Mao et al., 2017). The hmqA-G gene cluster is positively regulated by ScmR in B. thailandensis (Mao et al., 2017), but this study is the first to evaluate the importance of ScmR for hmqA-G regulation in B. pseudomallei. β-galactosidase assays were utilized to evaluate hmqD-lacZ and scmR-lacZ transcriptional fusions in Bp82 and the results confirmed that ScmR is a positive regulator of the hmqA-G locus in B. pseudomallei (Figure 4A). In addition, the transcription of scmR and hmqA-G are both activated by QS in B. thailandensis and B. pseudomallei (Figure 4B; Klaus et al., 2018; Le Guillouzer et al., 2020; Majerczyk C. et al., 2014; Majerczyk C. D. et al., 2014; Martinez et al., 2020). All three of B. pseudomallei’s AHLs, C8-HSL, 3OH-C10-HSL, or 3OH-C8-HSL, activated the transcription of scmR (Figure 5). Taken together, the results suggest that the activation of scmR expression by QS is followed by the activation of hmqA-G transcription by ScmR. An in-depth analysis of the production of Δ2-MNQ, MNQ, and Δ2-MNQNO during the growth of Bp82 and CM139 revealed some interesting differences (Figure 6). The production of all three molecules peaked at the late exponential to early stationary phase of growth in Bp82 and then steadily declined throughout the stationary phase (Figures 6B–D). The production of MAQs by B. ambifaria followed a similar trend when grown aerobically (Prothiwa et al., 2021). By comparison, the production of Δ2-MNQ and MNQ by CM139 steadily increased during the stationary phase of growth (Figures 6B, C). One possible explanation for the production differences of these compounds is that there may be a QS-activated MAQ and MAQNO degradation mechanism occurring in Bp82 that is not upregulated in CM139 during the stationary phase of growth. Alternatively, the Δ2-MNQ and MNQ production differences could be due to the QS-mediated levels of ScmR. The scmR gene in B. thailandensis is negatively autoregulated and it is possible that this is also true in B. pseudomallei (Le Guillouzer et al., 2020). If this is the case, the expression of scmR in Bp82 likely hits a threshold level in early stationary phase where it automatically shuts down and leads to a decrease in hmqA-G transcription and a decrease in Δ2-MNQ and MNQ production. CM139 may never reach the threshold level of scmR expression during the stationary phase of growth and negative autoregulation may not occur. As a result, ScmR may remain at sufficient levels to continually activate the transcription of hmqA-G and the production of Δ2-MNQ and MNQ. Finally, CM139 did not produce AQNOs or MAQNOs, including Δ2-MNQNO, during any stage of growth (Figure 8D and Table 2). The hmqL gene encodes a N-hydroxylating flavoprotein monooxygenase that converts AQs and MAQs into N-oxides (Ernst et al., 2022; Klaus et al., 2020b; Savchenko et al., 2024). This gene is not linked to the hmqA-G gene cluster and it may be differentially regulated by QS and ScmR. The LC-MS/MS results suggest that this gene may not be transcribed or the encoded enzyme may not be stable in the QS-deficient strain CM139. Further studies will be required to understand why this strain does not produce AQNOs or MAQNOs.
The importance of AQ derivatives for B. pseudomallei survival and replication within host cells was assessed in this study using 1026b and 1026b ΔhmqD. The results showed that while 1026b ΔhmqD persisted intracellularly during the 12 h experiment, it did not replicate as well as 1026b inside the murine macrophage cell line RAW264.7 (Figure 7A). Thus, the intracellular production of AQs, AQNOs, MAQs, and MAQNOs by B. pseudomallei provided an unexpected replication benefit within this niche. B. thailandensis was recently shown to produce a variety of secondary metabolites, including AQs, MAQs, MAQNOs, when grown inside RAW264.7 cells (Aiosa et al., 2022). It is tempting to speculate that these molecules also promote the replication of this facultative intracellular bacterium, but additional studies are required to address this possibility. Eukaryotic targets of the AQ derivatives include NADH:ubiquinone oxidoreductase (complex I) and ubiquinol:cytochrome c oxidoreductase (complex III) of the mitochondrial respiratory chain (Reil et al., 1997) and the pyrimidine biosynthesis enzyme dihydroorotate dehydrogenase (DHODH) (Garrett and Whalen, 2023). These enzymatic targets all interact with UQ and the AQs, AQNOs, MAQs, and MAQNOs compete with UQ binding to disrupt electron transport and pyrimidine biosynthesis (Horwitz et al., 2022; Wu and Seyedsayamdost, 2017). Fungi are also inhibited by these Burkholderia secondary metabolites and it is likely that the same enzymes are targeted (Kilani-Feki et al., 2012; Moon et al., 1996; Wang et al., 2020). We hypothesize that AQ derivatives are exported inside the RAW264.7 cell cytosol by intracellular B. pseudomallei where they competitively inhibit these enzymes and provide a replication advantage. Further studies will be required to test this hypothesis.
B. pseudomallei 1026b ΔhmqD was notably less virulent than 1026b in the murine model of melioidosis indicating that AQs, AQNOs, MAQs, and MAQNOs are important B. pseudomallei virulence factors (Figure 7B). This is the first time that the B. pseudomallei hmqA-G locus has been shown to be important for mammalian pathogenesis. Previous studies have also suggested that the products of the hmqA-G locus might be involved in virulence. When B. pseudomallei JW270 and JW270 ΔhmqD were assessed in the Madagascar hissing cockroach model of infection, the LD50 of JW270 ΔhmqD was found to be > 103 times higher than JW270 (Chua et al., 2022) suggesting that Burkholderia quinolones are also important for virulence in different host species. In addition, Price et al. found that the B. pseudomallei hmqA-G genes were upregulated in chronically adapted cystic fibrosis (CF) patient isolates following growth in artificial CF sputum medium (Price et al., 2018). The reason for the decreased virulence of B. pseudomallei 1026b ΔhmqD may be due to the intracellular replication defect in macrophages mentioned above or it could be due to another pathogenesis-associated deficiency. The first five genes of the B. pseudomallei hmqA-G locus encode proteins that are homologous to the products of the Pseudomonas aeruginosa pqsA-E locus (Diggle et al., 2006; Vial et al., 2008). This gene cluster in P. aeruginosa encodes for greater than 50 distinct 2-alkyl-4-quinolones (AHQs), including 2-heptyl-3-hydroxy-4-quinolone (PQS) and 2-heptyl-4-quinolone (HHQ) (Déziel et al., 2004; Diggle et al., 2003). PQS, also known as Pseudomonas Quinolone Signal, is important for QS-mediated expression of virulence factors and a variety of QS-independent activities (Lin et al., 2018). Importantly, homologs of B. pseudomallei hmqE and hmqG are not present in P. aeruginosa and the AHQ molecules produced by this organism do not contain a methyl group at position 3 or an alkyl group with unsaturation at position 2’ (Agarwal et al., 2012; Savchenko et al., 2024; Vial et al., 2008). This could be important as different chemical substitutions on the quinolone core scaffold can result in substantial differences in biological activity (Saalim et al., 2020). Unlike the P. aeruginosa pqsA-E gene cluster, there is limited data on the role of the B. pseudomallei hmqA-G locus in QS. Diggle et al. demonstrated that a B. pseudomallei hmqA mutant exhibited a change in colony morphology and elevated elastase production and both phenotypes were attributed to altered AQ signaling (Diggle et al., 2006). However, B. pseudomallei Bp82 ΔhmqD did not exhibit an altered colony phenotype or elevated elastase production relative to Bp82 in this study, but it did not produce a zone of clearing on 3% skim milk agar plates (data not shown). Multiple B. pseudomallei peptidases and proteases are exported by the type II secretion system and are responsible for the zone of clearing on skim milk agar plates (Burtnick et al., 2014). The reason for the defect in peptidase and protease export by Bp82 ΔhmqD is unknown, but it could be due to altered AQ signaling. Genome-wide transcriptional profiling studies need to be conducted to better understand the potential role of B. pseudomallei AQs in QS-mediated gene expression before any conclusions can be made about the involvement of these molecules in cell density-mediated phenotypes. While the exact molecular mechanism of their role in virulence is currently unknown, the results presented here clearly demonstrate that the AQ, AQNO, MAQ, and MAQNO molecules produced by B. pseudomallei are critical for competitive fitness against environmental Gram-negative bacteria in the phylum Bacteroidota and for pathogenesis in the murine model of melioidosis. In addition, inhibition of B. pseudomallei AQ production by HmqD inhibitors like compound 4 (Figure 8) could represent a novel therapeutic countermeasure for melioidosis patients in the future.
Data availability statement
The GenBank accession numbers for the Gram-negative 16S rRNA sequences described in this study are PP920381-PP920419.
Ethics statement
The animal study was approved by the Opinions, interpretations, conclusions, and recommendations are those of the authors and are not necessarily endorsed by the U.S. Army or the Department of Defense Health Agency. Research was conducted in compliance with the Animal Welfare Act and other federal statutes and regulations relating to animals and experiments involving animals and adheres to principles stated in the Guide for the Care and Use of Laboratory Animals, National Research Council, 2011. The facility where this research was conducted is fully accredited by the Association for Assessment and Accreditation of Laboratory Animal Care International. The study was conducted in accordance with the local legislation and institutional requirements.
Author contributions
SM: Investigation, Methodology, Writing – review and editing. VS: Investigation, Methodology, Writing – review and editing. VF: Investigation, Methodology, Writing – review and editing. TB: Formal analysis, Supervision, Writing – review and editing, DD: Conceptualization, Investigation, Formal analysis, Funding acquisition, Supervision, Data curation, Writing – original draft, Writing – review and editing.
Funding
The author(s) declare financial support was received for the research, authorship, and/or publication of the article. This work was funded by the U.S. Defense Threat Reduction Agency, project JSTO-CBD CB10207 (USAMRIID). TB acknowledges funding from the Austrian Science Fund (FWF) [doi.org/10.55776/COE7].
Acknowledgments
We thank Matt Meinig for evaluating the cytotoxicity of compound 4 in HepG2 cells and Ju Qiu for statistical support.
Conflict of interest
The authors declare that the research was conducted in the absence of any commercial or financial relationships that could be construed as a potential conflict of interest.
Publisher’s note
All claims expressed in this article are solely those of the authors and do not necessarily represent those of their affiliated organizations, or those of the publisher, the editors and the reviewers. Any product that may be evaluated in this article, or claim that may be made by its manufacturer, is not guaranteed or endorsed by the publisher.
Author disclaimer
The opinions, interpretations, conclusions, and recommendations presented are those of the authors and are not necessarily endorsed by the U.S. Army or the Defense Health Agency.
Research was conducted under an Institutional Animal Care and Use Committee (IACUC) approved protocol in compliance with the Animal Welfare Act, PHS Policy, and other Federal statutes and regulations relating to animals and experiments involving animals. The facility where this research was conducted is accredited by the Association for Assessment and Accreditation of Laboratory Animal Care International and adheres to principles stated in the Guide for the Care and Use of Laboratory Animals, National Research Council, 2011.
3-Methyl-2-alkylquinolones (MAQs) have been also termed HMAQs referring to their tautomeric 4-hydroxy-3-methyl-2-alkyl-4(1H)-quinolone form. To be consistent with the nomenclature of 2-alkyl-4(1H)-quinolones (AQs), we chose the abbreviation MAQs and MAQNOs for the corresponding N-oxides.
Supplementary material
The Supplementary Material for this article can be found online at: https://www.frontiersin.org/articles/10.3389/fmicb.2024.1474033/full#supplementary-material
References
Abby, S. S., Kazemzadeh, K., Vragniau, C., Pelosi, L., and Pierrel, F. (2020). Advances in bacterial pathways for the biosynthesis of ubiquinone. Biochim. Biophys. Acta Bioenerg. 1861:148259. doi: 10.1016/j.bbabio.2020.148259
Agarwal, A., Kahyaoglu, C., and Hansen, D. B. (2012). Characterization of HmqF, a protein involved in the biosynthesis of unsaturated quinolones produced by Burkholderia thailandensis. Biochemistry 51, 1648–1657. doi: 10.1021/bi201625w
Aiosa, N., Sinha, A., Jaiyesimi, O. A., da Silva, R. R., Branda, S. S., and Garg, N. (2022). Metabolomics analysis of bacterial pathogen Burkholderia thailandensis and mammalian host cells in co-culture. ACS Infect. Dis. 8, 1646–1662. doi: 10.1021/acsinfecdis.2c00233
Ashdown, L. R. (1979). An improved screening technique for isolation of Pseudomonas pseudomallei from clinical specimens. Pathology 11, 293–297. doi: 10.3109/00313027909061954
Biggins, J. B., Kang, H. S., Ternei, M. A., DeShazer, D., and Brady, S. F. (2014). The chemical arsenal of Burkholderia pseudomallei is essential for pathogenicity. J. Am. Chem. Soc. 136, 9484–9490. doi: 10.1021/ja504617n
Burtnick, M. N., Brett, P. J., and DeShazer, D. (2014). Proteomic analysis of the Burkholderia pseudomallei type II secretome reveals hydrolytic enzymes, novel proteins, and the deubiquitinase TssM. Infect. Immun. 82, 3214–3226. doi: 10.1128/IAI.01739-14
Burtnick, M. N., Brett, P. J., Harding, S. V., Ngugi, S. A., Ribot, W. J., Chantratita, N., et al. (2011). The cluster 1 type VI secretion system is a major virulence determinant in Burkholderia pseudomallei. Infect. Immun. 79, 1512–1525. doi: 10.1128/IAI.01218-10
Chua, J., Nguyenkhoa, E., Mou, S., Tobery, S. A., Friedlander, A. M., and DeShazer, D. (2022). Burkholderia pseudomallei JW270 is lethal in the Madagascar hissing cockroach infection model and can be utilized at biosafety level 2 to identify putative virulence factors. Infect. Immun. 90:e0015922. doi: 10.1128/iai.00159-22
Collins, M. D., and Jones, D. (1981). Distribution of isoprenoid quinone structural types in bacteria and their taxonomic implication. Microbiol. Rev. 45, 316–354. doi: 10.1128/mr.45.2.316-354.1981
Coulon, P. M. L., Groleau, M. C., and Déziel, E. (2019). Potential of the Burkholderia cepacia complex to produce 4-hydroxy-3-methyl-2-alkyquinolines. Front. Cell. Infect. Microbiol. 9:33–33. doi: 10.3389/fcimb.2019.00033
Currie, B. J., Meumann, E. M., and Kaestli, M. (2023). The expanding global Footprint of Burkholderia pseudomallei and melioidosis. Am. J. Trop. Med. Hyg. 108, 1081–1083. doi: 10.4269/ajtmh.23-0223
Dennis, J. J., and Zylstra, G. J. (1998). Plasposons: Modular self-cloning minitransposon derivatives for rapid genetic analysis of Gram-negative bacterial genomes. Appl. Environ. Microbiol. 64, 2710–2715. doi: 10.1128/AEM.64.7.2710-2715.1998
Déziel, E., Lépine, F., Milot, S., He, J., Mindrinos, M. N., Tompkins, R. G., et al. (2004). Analysis of Pseudomonas aeruginosa 4-hydroxy-2-alkylquinolines (HAQs) reveals a role for 4-hydroxy-2-heptylquinoline in cell-to-cell communication. Proc. Natl. Acad. Sci. U.S.A. 101, 1339–1344. doi: 10.1073/pnas.0307694100
Diggle, S. P., Lumjiaktase, P., Dipilato, F., Winzer, K., Kunakorn, M., Barrett, D. A., et al. (2006). Functional genetic analysis reveals a 2-Alkyl-4-quinolone signaling system in the human pathogen Burkholderia pseudomallei and related bacteria. Chem. Biol. 13, 701–710. doi: 10.1016/j.chembiol.2006.05.006
Diggle, S. P., Winzer, K., Chhabra, S. R., Worrall, K. E., Cámara, M., and Williams, P. (2003). The Pseudomonas aeruginosa quinolone signal molecule overcomes the cell density-dependency of the quorum sensing hierarchy, regulates rhl-dependent genes at the onset of stationary phase and can be produced in the absence of LasR. Mol. Microbiol. 50, 29–43. doi: 10.1046/j.1365-2958.2003.03672.x
Dow, L., Stock, F., Peltekis, A., Szamosvári, D., Prothiwa, M., Lapointe, A., et al. (2020). The Multifaceted Inhibitory Effects of an Alkylquinolone on the Diatom Phaeodactylum tricornutum. ChemBioChem 21, 1206–1216. doi: 10.1002/cbic.201900612
Ernst, S., Mährlein, A., Ritzmann, N. H., Drees, S. L., and Fetzner, S. (2022). A comparative study of N-hydroxylating flavoprotein monooxygenases reveals differences in kinetics and cofactor binding. FEBS J. 289, 5637–5655. doi: 10.1111/febs.16444
Franza, T., and Gaudu, P. (2022). Quinones: More than electron shuttles. Res. Microbiol. 173:103953. doi: 10.1016/j.resmic.2022.103953
Fuqua, C., Parsek, M. R., and Greenberg, E. P. (2001). Regulation of gene expression by cell-to-cell communication: Acyl-homoserine lactone quorum sensing. Annu. Rev. Genet. 35, 439–468. doi: 10.1146/annurev.genet.35.102401.090913
Garrett, O., and Whalen, K. E. (2023). A bacterial quorum sensing signal is a potent inhibitor of de novo pyrimidine biosynthesis in the globally abundant Emiliania huxleyi. Front. Microbiol. 14:1266972. doi: 10.3389/fmicb.2023.1266972
Hachani, A., Lossi, N. S., and Filloux, A. (2013). A visual assay to monitor T6SS-mediated bacterial competition. J. Vis. Exp. 73:e50103. doi: 10.3791/50103-v
Hamad, M. A., Zajdowicz, S. L., Holmes, R. K., and Voskuil, M. I. (2009). An allelic exchange system for compliant genetic manipulation of the select agents Burkholderia pseudomallei and Burkholderia mallei. Gene 430, 123–131. doi: 10.1016/j.gene.2008.10.011
Horwitz, S. M., Blue, T. C., Ambarian, J. A., Hoshino, S., Seyedsayamdost, M. R., and Davis, K. M. (2022). Structural insights into inhibition of the drug target dihydroorotate dehydrogenase by bacterial hydroxyalkylquinolines. RSC Chem. Biol. 3, 420–425. doi: 10.1039/D1CB00255D
Inglis, T. J. J., and Mayo, M. J. (2012). “Burkholderia pseudomallei in water,” in Melioidosis - A century of observation and research, ed. N. Ketheesan (Amsterdam: Elsevier B.V.), 358–364.
Kaestli, M., Schmid, M., Mayo, M., Rothballer, M., Harrington, G., Richardson, L., et al. (2012). Out of the ground: Aerial and exotic habitats of the melioidosis bacterium Burkholderia pseudomallei in grasses in Australia. Environ. Microbiol. 14, 2058–2070. doi: 10.1111/j.1462-2920.2011.02671.x
Kilani-Feki, O., Zouari, I., Culioli, G., Ortalo-Magné, A., Zouari, N., Blache, Y., et al. (2012). Correlation between synthesis variation of 2-alkylquinolones and the antifungal activity of a Burkholderia cepacia strain collection. World J. Microbiol. Biotechnol. 28, 275–281. doi: 10.1007/s11274-011-0817-0
Klaus, J. R., Coulon, P. M. L., Koirala, P., Seyedsayamdost, M. R., Déziel, E., and Chandler, J. R. (2020a). Secondary metabolites from the Burkholderia pseudomallei complex: Structure, ecology, and evolution. J. Ind. Microbiol. Biotechnol. 47, 877–887. doi: 10.1007/s10295-020-02317-0
Klaus, J. R., Majerczyk, C., Moon, S., Eppler, N. A., Smith, S., Tuma, E., et al. (2020b). Burkholderia thailandensis methylated hydroxyalkylquinolines: Biosynthesis and antimicrobial activity in cocultures. Appl. Environ. Microbiol. 86:e001452–20. doi: 10.1128/AEM.01452-20
Klaus, J. R., Deay, J., Neuenswander, B., Hursh, W., Gao, Z., Bouddhara, T., et al. (2018). Malleilactone is a Burkholderia pseudomallei virulence factor regulated by antibiotics and quorum sensing. J. Bacteriol. 200:e00008–e18. doi: 10.1128/JB.00008-18
Kunakom, S., and Eustáquio, A. S. (2019). Burkholderia as a source of natural products. J. Nat. Prod. 82, 2018–2037. doi: 10.1021/acs.jnatprod.8b01068
Lane, D. J. (1991). “16S/23S rRNA sequencing,” in Nucleic acid techniques in bacterial systematics, eds E. Stackebrandt and M. Goodfellow (New York, NY: John Wiley & Sons), 115–175.
Le Guillouzer, S., Groleau, M. C., Mauffrey, F., and Déziel, E. (2020). ScmR, a global regulator of gene expression, quorum sensing, pH homeostasis, and virulence in Burkholderia thailandensis. J. Bacteriol. 202:e00776–19. doi: 10.1128/JB.00776-19
Lemoine, F., Correia, D., Lefort, V., Doppelt-Azeroual, O., Mareuil, F., Cohen-Boulakia, S., et al. (2019). NGPhylogeny.fr: New generation phylogenetic services for non-specialists. Nucleic Acids Res. 47, W260–W265. doi: 10.1093/nar/gkz303
Limmathurotsakul, D., Golding, N., Dance, D. A. B., Messina, J. P., Pigott, D. M., Moyes, C. L., et al. (2016). Predicted global distribution of Burkholderia pseudomallei and burden of melioidosis. Nat. Microbiol. 1:15008. doi: 10.1038/nmicrobiol.2015.8
Limmathurotsakul, D., Wuthiekanun, V., Tuanyok, A., and Peacock, S. J. (2012). “Presence and sampling of Burkholderia pseudomallei in soil,” in Melioidosis - A century of observation and research, ed. N. Ketheesan (Amsterdam: Elsevier B.V.), 349–357.
Lin, J., Cheng, J., Wang, Y., and Shen, X. (2018). The Pseudomonas quinolone signal (PQS): Not just for quorum sensing anymore. Front. Cell. Infect. Microbiol. 8:230. doi: 10.3389/fcimb.2018.00230
Logue, C.-A., Peak, I. R., and Beacham, I. R. (2009). Facile construction of unmarked deletion mutants in Burkholderia pseudomallei using sacB counter-selection in sucrose-resistant and sucrose-sensitive isolates. J. Microbiol. Methods 76, 320–323. doi: 10.1016/j.mimet.2008.12.007
Majerczyk, C., Brittnacher, M., Jacobs, M., Armour, C. D., Radey, M., Schneider, E., et al. (2014). Global analysis of the Burkholderia thailandensis quorum sensing-controlled regulon. J. Bacteriol. 196, 1412–1424. doi: 10.1128/JB.01405-13
Majerczyk, C. D., Brittnacher, M. J., Jacobs, M. A., Armour, C. D., Radey, M. C., Bunt, R., et al. (2014). Cross-species comparison of the Burkholderia pseudomallei, Burkholderia thailandensis, and Burkholderia mallei quorum-sensing regulons. J. Bacteriol. 196, 3862–3871. doi: 10.1128/JB.01974-14
Mao, D., Bushin, L. B., Moon, K., Wu, Y., and Seyedsayamdost, M. R. (2017). Discovery of scmR as a global regulator of secondary metabolism and virulence in Burkholderia thailandensis E264. Proc. Natl. Acad. Sci. U.S.A. 114:E2920. doi: 10.1073/pnas.1619529114
Marreiros, B. C., Calisto, F., Castro, P. J., Duarte, A. M., Sena, F. V., Silva, A. F., et al. (2016). Exploring membrane respiratory chains. Biochim. Biophys. Acta 1857, 1039–1067. doi: 10.1016/j.bbabio.2016.03.028
Martinez, S., Humery, A., Groleau, M. C., and Déziel, E. (2020). Quorum sensing controls both rhamnolipid and polyhydroxyalkanoate production in Burkholderia thailandensis through ScmR regulation. Front. Bioeng. Biotechnol. 8:1033. doi: 10.3389/fbioe.2020.01033
McGinnis, S., and Madden, T. L. (2004). BLAST: At the core of a powerful and diverse set of sequence analysis tools. Nucleic Acids Res. 32, W20–W25. doi: 10.1093/nar/gkh435
Meumann, E. M., Limmathurotsakul, D., Dunachie, S. J., Wiersinga, W. J., and Currie, B. J. (2024). Burkholderia pseudomallei and melioidosis. Nat. Rev. Microbiol. 22, 155–169. doi: 10.1038/s41579-023-00972-5
Miller, J. H. (1972). Experiments in molecular genetics. Cold Spring Harbor, NY: Cold Spring Harbor Laboratory.
Moon, S.-S., Kang, P. M., Park, K. S., and Kim, C. H. (1996). Plant growth promoting and fungicidal 4-quinolinones from Pseudomonas cepacia. Phytochemistry 42, 365–368. doi: 10.1016/0031-9422(95)00897-7
Mou, S., Jenkins, C. C., Okaro, U., Dhummakupt, E. S., Mach, P. M., and DeShazer, D. (2021). The Burkholderia pseudomallei hmqA-G locus mediates competitive fitness against environmental gram-positive bacteria. Microbiol. Spectr. 9:e0010221. doi: 10.1128/spectrum.00102-21
Nowicka, B., and Kruk, J. (2010). Occurrence, biosynthesis and function of isoprenoid quinones. Biochim. Biophys. Acta. 1797, 1587–1605. doi: 10.1016/j.bbabio.2010.06.007
Ntougias, S., Fasseas, C., and Zervakis, G. I. (2007). Olivibacter sitiensis gen. nov., sp. nov., isolated from alkaline olive-oil mill wastes in the region of Sitia, Crete. Int. J. Syst. Evol. Microbiol. 57, 398–404. doi: 10.1099/ijs.0.64561-0
Okada, B. K., Wu, Y., Mao, D., Bushin, L. B., and Seyedsayamdost, M. R. (2016). Mapping the trimethoprim-Induced secondary metabolome of Burkholderia thailandensis. ACS Chem. Biol. 11, 2124–2130. doi: 10.1021/acschembio.6b00447
Oren, A., and Garrity, G. M. (2021). Valid publication of the names of forty-two phyla of prokaryotes. Int. J. Syst. Evol. Microbiol. 71:10. doi: 10.1099/ijsem.0.005056
Petras, J. K., Elrod, M. G., Ty, M. C., Dawson, P., O’Laughlin, K., Gee, J. E., et al. (2023). Locally acquired melioidosis linked to environment - Mississippi, 2020-2023. N. Engl. J. Med. 389, 2355–2362. doi: 10.1056/NEJMoa2306448
Piochon, M., Coulon, P. M. L., Caulet, A., Groleau, M. C., Déziel, E., and Gauthier, C. (2020). Synthesis and antimicrobial activity of Burkholderia-related 4-hydroxy-3-methyl-2-alkenylquinolines (HMAQs) and their N-oxide counterparts. J. Nat. Prod. 83, 2145–2154. doi: 10.1021/acs.jnatprod.0c00171
Price, E. P., Viberg, L. T., Kidd, T. J., Bell, S. C., Currie, B. J., and Sarovich, D. S. (2018). Transcriptomic analysis of longitudinal Burkholderia pseudomallei infecting the cystic fibrosis lung. Microb. Genom. 4:e000194. doi: 10.1099/mgen.0.000194
Prothiwa, M., Filz, V., Oehler, S., and Böttcher, T. (2021). Inhibiting quinolone biosynthesis of Burkholderia. Chem. Sci. 12, 6908–6912. doi: 10.1039/D0SC06167K
Reil, E., Höfle, G., Draber, W., and Oettmeier, W. (1997). Quinolones and their N-oxides as inhibitors of mitochondrial complexes I and III. Biochim. Biophys. Acta 1318, 291–298. doi: 10.1016/S0005-2728(96)00150-8
Saalim, M., Villegas-Moreno, J., and Clark, B. R. (2020). Bacterial alkyl-4-quinolones: Discovery, structural diversity and biological properties. Molecules 25:5689. doi: 10.3390/molecules25235689
Savchenko, V., Jaegers, M., Rasche, R., Herrmann, E., König, S., Kümmel, D., et al. (2024). Unraveling key steps in the biosynthesis of antimicrobial methylated unsaturated 2-alkyl-4-quinolones of Burkholderia thailandensis. Cell Rep. Phys. Sci. 5:102100. doi: 10.1016/j.xcrp.2024.102100
Schoepp-Cothenet, B., van Lis, R., Atteia, A., Baymann, F., Capowiez, L., Ducluzeau, A.-L., et al. (2013). On the universal core of bioenergetics. Biochim. Biophys. Acta 1827, 79–93. doi: 10.1016/j.bbabio.2012.09.005
Srinivasan, S., Jnana, A., and Murali, T. S. (2024). Modeling microbial community networks: Methods and tools for studying microbial interactions. Microb. Ecol. 87:56. doi: 10.1007/s00248-024-02370-7
Szamosvári, D., and Böttcher, T. (2017). An unsaturated quinolone N-oxide of pseudomonas aeruginosa modulates growth and virulence of Staphylococcus aureus. Angew. Chem. Int. Ed. Engl. 56, 7271–7275. doi: 10.1002/anie.201702944
Szamosvári, D., Prothiwa, M., Dieterich, C. L., and Böttcher, T. (2020). Profiling structural diversity and activity of 2-alkyl-4(1H)-quinolone N-oxides of Pseudomonas and Burkholderia. Chem. Commun. 56, 6328–6331. doi: 10.1039/D0CC02498H
Szamosvári, D., Sylvester, K., Schmid, P., Lu, K. Y., Derbyshire, E. R., and Böttcher, T. (2019). Close the ring to break the cycle: Tandem quinolone-alkyne-cyclisation gives access to tricyclic pyrrolo[1,2-a]quinolin-5-ones with potent anti-protozoal activity. Chem. Commun. 55, 7009–7012. doi: 10.1039/C9CC01689A
Vial, L., Lépine, F., Milot, S., Groleau, M. C., Dekimpe, V., Woods, D. E., et al. (2008). Burkholderia pseudomallei, B. thailandensis, and B. ambifaria produce 4-hydroxy-2-alkylquinoline analogues with a methyl group at the 3 position that is required for quorum-sensing regulation. J. Bacteriol. 190, 5339–5352. doi: 10.1128/JB.00400-08
Wang, Q., Han, X. L., Huang, Z. P., Huang, S., An, D. F., Shen, J. Q., et al. (2021). Faecalibacter rhinopitheci sp. nov., a bacterium isolated from the faeces of Rhinopithecus bieti. Int. J. Syst. Evol. Microbiol. 71:4932. doi: 10.1099/ijsem.0.004932
Wang, Y., Hoffmann, J. P., Chou, C. W., Höner, Zu Bentrup, K., Fuselier, J. A., et al. (2020). Burkholderia thailandensis outer membrane vesicles exert antimicrobial activity against drug-resistant and competitor microbial species. J. Microbiol. 58, 550–562. doi: 10.1007/s12275-020-0028-1
Weisburg, W. G., Barns, S. M., Pelletier, D. A., and Lane, D. J. (1991). 16S ribosomal DNA amplification for phylogenetic study. J. Bacteriol. 173, 697–703. doi: 10.1128/jb.173.2.697-703.1991
Wu, Y., and Seyedsayamdost, M. R. (2017). Synergy and target promiscuity drive structural divergence in bacterial alkylquinolone biosynthesis. Cell Chem. Biol. 24:1437–1444.e1433. doi: 10.1016/j.chembiol.2017.08.024
Keywords: pathogenesis, select agent, natural products, biosynthetic gene cluster, isoprenoid quinone
Citation: Mou S, Savchenko V, Filz V, Böttcher T and DeShazer D (2024) Burkholderia pseudomallei produces 2-alkylquinolone derivatives important for host virulence and competition with bacteria that employ naphthoquinones for aerobic respiration. Front. Microbiol. 15:1474033. doi: 10.3389/fmicb.2024.1474033
Received: 31 July 2024; Accepted: 30 September 2024;
Published: 14 October 2024.
Edited by:
Axel Cloeckaert, Institut National de Recherche pour L’agriculture, L’alimentation et L’environnement (INRAE), FranceReviewed by:
Apichai Tuanyok, University of Florida, United StatesItziar Chapartegui-González, Karolinska Institutet (KI), Sweden
Erin C. Garcia, University of Kentucky, United States
Narisara Chantratita, Mahidol University, Thailand
Copyright © 2024 Mou, Savchenko, Filz, Böttcher and DeShazer. This is an open-access article distributed under the terms of the Creative Commons Attribution License (CC BY). The use, distribution or reproduction in other forums is permitted, provided the original author(s) and the copyright owner(s) are credited and that the original publication in this journal is cited, in accordance with accepted academic practice. No use, distribution or reproduction is permitted which does not comply with these terms.
*Correspondence: David DeShazer, ZGF2aWQuZGVzaGF6ZXIuY2l2QGhlYWx0aC5taWw=