- Key Laboratory of Animal Disease and Human Health of Sichuan Province, College of Veterinary Medicine, Sichuan Agricultural University, Chengdu, China
Background: Heat stress significantly impacts dairy cows, primarily through oxidative stress, which undermines their health. The problem is exacerbated by the ongoing global warming trend. Lactic acid bacteria (LAB) are safe, economical, and readily accessible options for enhancing the host’s antioxidant defenses and preventing oxidative damage. They have been proven effective in alleviating heat stress-related damage, making them an excellent choice for protecting dairy cows from the adverse effects of heat stress.
Method: In this study, five strains of LAB from Holstein cow milk (Lactobacillus plantarum L5, L14, L17, L19, L20) were evaluated for their heat resistance and antioxidant capacity by evaluating the growth characteristics and tolerance of the strains under high-temperature conditions, as well as their H2O2 tolerance, free radical scavenging ability (DPPH, OH−, ABTS), reducing ability, and EPS production ability. Furthermore, we employed Caco-2 cells to assess the adhesion rate of the strain, thereby confirming its ability to successfully colonize the host’s intestinal tract and ensuring the effective execution of its probiotic functions. The strain with excellent heat resistance and antioxidant capacity was then subjected to genomic analysis to gain insight into the molecular mechanisms behind their heat resistance, antioxidant capacity, and safety.
Results: Among the two strains, Lactobacillus plantarum L19 emerges as a highly promising candidate. The strain exhibits robust growth even at high temperatures at 40°C and maintains a survival rate of 16.42% at the extreme temperature of 65°C. Furthermore, it demonstrates superior tolerance to hydrogen peroxide (27.3%), and possesses a notably higher free radical scavenging capacity with a high adhesion rate to Caco-2 cell (22.19%) compared to the other four strains tested. Genomic analysis revealed its’ genome has 17 genes related to antioxidants and three genes related to heat resistance. Importantly, L19 lacks any resistance genes, ensuring its safety as a probiotic.
Conclusion: The results imply that Lactobacillus plantarum L19 has the potential to serve as an effective food additive in mitigating damages associated with heat stress. This research offers a valuable reference for the prevention and management of heat stress in dairy cows, while also expanding the scope of applications for LAB derived from cow milk.
Introduction
Heat stress (HS) involves systemic adaptive responses to prolonged high temperatures, including non-specific defenses and specific physiological dysfunctions, occurring when thermoregulation is disrupted by extreme environmental heat (Peiris et al., 2017). Dairy cows are more prone to HS because of the high metabolic rate and small surface-to-volume ratio (Cartwright et al., 2021). Research indicates that HS not only leads to mastitis (Wang et al., 2021), liver metabolic (Fan et al., 2018), intestinal injury (Koch et al., 2019) and granulosa cell apoptosis in dairy cows (Wang et al., 2019), but also disrupts the immune system and causes reproductive disorders (Bagath et al., 2019) and decreased milk production (Bernabucci et al., 2014; Rhoads, 2023), seriously hindering the development of dairy farming industry. Due to global warming and rising temperatures, HS’s adverse effects will worsen. To the best of our knowledge, no safe and effective methods have been developed to prevent and control these effects in dairy cows. Experts suggest that without intervention, HS could directly cause losses of $40 billion by the end of this century (Thornton et al., 2022). Therefore, there is an urgent need to seek effective Intervention measures for HS damage to ensure the health of dairy cows and the prosperous development of the dairy farming industry.
Studies have shown that during HS, the energy metabolism and oxygen demand of body tissues increase, pushing mitochondrial activity beyond its thermal limit. This leads to mitochondrial damage and reactive oxygen species (ROS) release. The resulting overload of free radicals causes oxidative stress, which is responsible for tissue damage and cell apoptosis (Christen et al., 2018; Li et al., 2020; Liu et al., 2017). Therefore, boosting the antioxidant capacity in animals is a highly effective strategy for alleviating oxidative damage associated with HS (Li et al., 2024).
Probiotics are characterized as “live microorganisms that, when administered in sufficient quantities, confer health benefits to the host.” Lactic acid bacteria (LAB), a prominent group within the probiotic family, are naturally inhabitants of the gut flora. Notably, Lactobacillus species have been granted GRAS (Generally Recognized As Safe) status by the FDA (United States Food and Drug Administration) (Kelleher et al., 2017), making them a commonly used feed or food additive for both humans and animals, leveraging their advantageous effects. In recent years, a great many of research has highlighted the antioxidant potential of specific strains of LAB. These strains, along with their fermentation products, have demonstrated the ability to assist the host in resisting oxidative damage, thereby positioning them as high-quality natural antioxidants (Amaretti et al., 2013; Mishra et al., 2015). Therefore, Lactobacillus possesses the potential to prevent and control HS-related damage, a capability that has been validated by current research findings. In 2023, Cai’s research revealed that probiotics are capable of mitigating inflammation in male reproductive cells triggered by HS (Cai et al., 2023). Similarly, in 2014, Moore and colleagues explored the protective effects of Bacillus subtilis against HS-induced complications in rats, with their findings demonstrating that Bacillus subtilis can effectively alleviate the detrimental effects of HS (Moore et al., 2014). Unfortunately, there has been no report on the use of LAB to prevent and treat HS in dairy cows. Given that the beneficial properties of microorganisms are closely related to the particularity of the host, strains from the same host have stronger host adaptability and can better exert their beneficial properties (Jang et al., 2021; Zoetendal et al., 2006). In addition, high temperatures significantly affect the survival rate of probiotics, with most clinical probiotics requiring low-temperature storage to maintain their viability (Mahmoodian et al., 2024). During heat stress, the animal’s body temperature rises, potentially inactivating the probiotics and nullifying their beneficial effects. Consequently, strains that demonstrate robust tolerance to high temperatures exhibit more stable probiotic properties under heat stress, ensuring their efficacy in prevention and control strategies. Moreover, probiotics are frequently utilized as food additives, and they are often exposed to extreme temperatures. Heat-resistant strains not only withstand the production process’s high temperatures, enhancing strain utility and reducing costs but also facilitate clinical preservation, streamlining production and application (Matsushita et al., 2016). Therefore, screening cow-derived LAB with excellent heat resistance and antioxidant capacity is an promising approach method to alleviate HS oxidative damage for dairy cows.
In our previous work, we successfully isolated 26 strains of LAB from the milk of Holstein cows. Through a meticulous screening process, we identified five strains of Lactobacillus plantarum that exhibited resilience to acidic and bile conditions, possessed potent antibacterial properties and were non-hemolytic (Lactobacillus plantarum L5, L14, L17, L19, L20) (Zhang et al., 2022). The purpose of this study is to combine phenotypic and genomic analyses to select a strain with excellent heat resistance and antioxidant ability from the five LAB strains, as a candidate strain to alleviate heat stress-related damage, ensuring the health of dairy cows and the development of dairy farming.
Materials and methods
Source of LAB
The Lactobacillus plantarum strains L5, L14, L17, L19 and L20 are LAB with potential probiotic properties that were initially isolated from Holstein cow milk in our laboratory (Zhang et al., 2022).
Preparation of cell-free supernatant and bacterial suspension
The LABs were activated for three generations and cultured overnight at 37°C in MRS liquid medium, centrifuged to collected the supernatant as cell-free supernatant (CFS) of LAB (8,000×g for 10 min at 4°C). The strain pellet was resuspended in sterile PBS to adjust the concentrations for bacterial suspension (BS) (1 × 108 CFU/mL). In this study, all evaluations of LAB were performed by treating the strains according to the above method to obtain BS or CFS.
High temperature resistance of LAB
Growth capacity at 40°C
The method was determined according to Liu et al. with some modifications (Liu et al., 2020). 50 μL BS of LAB was inoculation in 50 mL MRS broth (1%), cultured at 40°C under anaerobic conditions for 48 h. Measure the absorbance of the bacterial solution every 2 h from 0 to 24 h, every 4 h from 25 to 48 h (600 nm). Sterile MRS Medium was used as control group. Finally, draw the growth curve with times as the x-axis and OD600 as the y-axis.
Tolerance to high temperatures
The tolerance to high temperatures of LAB was assessed according to the method described by Da and Zhao with some modifications (Da et al., 2023; Zhao et al., 2023). 50 μL BS was inoculation in 50 mL of MRS broth (1%), and the inoculated culture was subjected to different temperature conditions for 30 min at 45°C, 55°C, and 65°C, respectively. Following this, the cultures were incubated at 37°C under anaerobic conditions for a period of 8 h. Upon completion of the 8 h incubation, the optical density of the experimental solutions was measured at 600 nm. Concurrently, BS inoculation in MRS broth without prior temperature treatment as control. Calculate the survival rate of each strain at different temperatures according to the following formula: Survival rate (%) = (experimental OD600/control OD600) × 100.
Antioxidative ability
Resistance to hydrogen peroxide (H2O2)
The method of Li et al. was used with some modifications (Li et al., 2012). Prepare the BS according to the method described above. BS with a volume ratio of 2% was inoculated into MRS liquid medium supplemented with varying concentrations of H2O2: 0, 0.5, 1.0, 1.5 and 2.0 mmol/L. The inoculated cultures were then incubated at 37°C under anaerobic conditions for 8 h, then measured the OD600 of the culture. The BS inoculated into MRS liquid medium without H2O2 as the control group. Resistance rate (%) = (ODexperimental/ODcontrol) × 100.
1, 1-diphenyl-2-picrylhydrazyl (DPPH) free radical scavenging ability
According to the method reported by Wang et al., to measure the DPPH free radical scavenging ability of LAB (Wang et al., 2023). Specifically, Prepare the CFS and BS of LAB using the method described above. Then, 2 mL 0.2 mmol/L DPPH anhydrous ethanol solution was mixed with 1 mL CFS or BS. React in the dark at room temperature for 30 min, then collect the supernatant (8,000×g for 10 min at 4°C). The OD517 of the supernatant was measured as test groups’ results (ODtest). Concurrently, a blank group and a control group were established for comparative analysis. In the blank group, anhydrous ethanol was substituted for DPPH; while in the control group, sterile distilled water replaced both the BS and the CFS. The same determination methods were then replicated to acquire the test results for the blank group (ODblank) and the control group (ODcontrol), respectively. The DPPH free radical clearance rate (%) was defined as: [1 − (ODtest − ODblank)/ODcontrol] × 100.
Hydroxyl (OH−) radical scavenging ability
The OH− radical scavenging assay was referred to Alam’s report (Alam et al., 2013). Add 1 mL ortho-phenanthroline (0.1%), 1 mL PBS, 1 mL FeSO4 (2.5 mmol/L), and 1 mL H2O2 (20 mmol/L) to 0.5 mL of CFS or BS of LAB, culturing in 37°C under anaerobic conditions for 1.5 h. Then measured the OD536 of the reaction solution (ODtest). At the same time, anhydrous ethanol was used to replace the same volume of H2O2 as the blank group; distilled water was used to replace the same volume of sample solution as the control group. Repeat the above test method to obtain the results of the blank group (ODblank) and the control group (ODcontrol). The OH− scavenging activity rate (%) was expressed as: [1 − (ODtest − ODblank)/ODcontrol] × 100.
3-ethylbenzthiazoline-6-sulfonic acid (ABTS) free radical scavenging ability
The ability to scavenge ABTS free radicals was measured according to Prete et al. (Prete et al., 2020). Mix ABTS stock solution (7 mM) with 2.45 mM potassium persulfate to generate ABTS radical cation (ABTS.+), and store the mixture in the dark for 16 h at room temperature. Before use, adjust the ABTS.+ to 0.9 ± 0.0.02 at OD734 nm using PBS as the working solution. Next, add 0.25 mL BS or PBS (as control) to 1 mL working solution prepared above. Allow the mixture to react for 5 min, followed by centrifugation (4,000×g for 5 min at 4°C). Subsequently, detect the absorbance of the supernatant at OD734 nm. The ABTS free radical scavenging ability (%) was defined as the following equation: (1 − ODtest/ODcontrol) × 100.
The ability of reducing
Referring to the method of Kuda et al. (Kuda et al., 2009). Mixed 500 μL CFS or BS with 500 μL potassium ferricyanide (1%) and 500 μL PBS (pH = 6.6), reacting at 50°C water bath for 20 min. Waiting for cooling and adding 500 μL trichloride acetic acid (TAC, 10%). After centrifugation (8,000×g for 10 min at 4°C), 1 mL of supernatant was mixed with 1 mL ferric chloride (0.1%) and reacting at room temperature for 10 min, then measured OD700 of the resulting solution.
Exopolysaccharide (EPS) generation capacity
The ability to produce EPS was evaluated regarding the method of Wang et al. (Wang et al., 2023). The CFS of LAB was mixed with 800 mg/mL trichloroacetic acid (TCA) to get a final concentration of 40 mg/mL. The mixture was then incubated at 4°C overnight and centrifuged to collect the supernatant (8,000×g for 10 min at 4°C). Then, 250 μL of the supernatant was mixed with 250 μL phenol solution (6%) and 1 mL concentrated sulfuric acid on ice. Finally, OD490 of the resulting solution was measured. The standard curve was made by Glucose with different concentrations (3.125 mg/L, 6.25 mg/L, 12.5 mg/L, 25 mg/L, 50 mg/L, and 100 mg/L) to calculate the concentration of EPS.
Adhesion to Caco-2 cell line
According to the method in the report with minor modifications (Foongsawat et al., 2023). Caco-2 cells were seeded into a 12-well plate (1 × 105/well) and cultured in the DMEM medium containing 10% FBS to achieve a confluent monolayer cell. Then, washed the cells’ monolayer thrice with PBS, and 1 mL of BS with a concentration of 1 × 108 CFU/mL (C0) in DMEM medium was added to each well in three replicates. The plates were incubated at 37°C with 5% CO2 for 2 h. After incubation, aspirated the bacterial suspension and washed the cells monolayer thrice with PBS to remove non-adherent bacteria. Subsequently, the cells were digested with 100 μL trypsin (0.25%). The collected digested cell liquid yielded a suspension of colonies adherent to the cells. This suspension was then plated onto MRS solid medium for colony enumeration, allowing for the calculation of the number of colonies adhering to the cells (C1). Calculate the adhesion rate (%) according to the formula: Adhesion rate (%) = C1/C0 × 100.
Whole-genome analyses
DNA extraction, sequencing
The genomic DNA of the candidate strain was extracted using a bacterial genomic DNA extraction kit (Tiangen, Beijing, China), then the purified DNA was submitted to Nanjing Paison Gene Technology Co., Ltd. for simultaneous sequencing using the second-generation platform Illumina NovaSeq and the third-generation platform Oxford Nanopore ONT.
Using Adapter Removal software to remove adapter contamination and SOAPec software for quality correction to obtain high-quality second-generation sequencing data (Schubert et al., 2016); use HGAP and CANU software to splice third-generation offline data to obtain counting sequences. Finally, Pilon software was used to correct the data to obtain the complete genome sequence (Walker et al., 2014).
Genome annotation
Transfer RNA (tRNA) were identified using the tRNA scan-SE, ribosomal RNA (rRNA) was determined using the Barrnapand other non-coding RNAs (ncRNAs) were predicted by comparing with the Rfam database (Kalvari et al. 2018).
The clustered regularly interspaced short palindromic repeat sequences (CRISPR) finder was used to determine CRISPR in the LAB (Grissa et al., 2007). The coding genes were annotation by Clusters of Orthologous Groups (COG), Kyoto Encyclopedia of Genes and Genomes (KEGG) (Moriya et al., 2007) and Gene Ontology (GO) (Conesa and Götz, 2008). Finally, the genome sequence, prediction information, and functional annotation information were integrated into a standard GBK (Genbank) format file, and the genome circle map was drawn using CGview software (Stothard and Wishart, 2005).
Bioinformatics analyses
To further elucidate the heat resistance and antioxidant capabilities of the strain at the molecular level, the heat resistance genes and antioxidant-related genes of the strain were mined. In addition, the antibiotic-resistance genes (AMR) were identified using ResFinder 4.0 with a 90% identity threshold and 60% minimum coverage.
Nucleotide sequence accession numbers
The genome of the candidate strain (Lactobacillus plantarum L19) was submitted and deposited in the NCBI database, accession numbers: SRR28342006.
Statistical analysis
The measurements were independently replicated three times, and the findings were shown as the mean ± standard deviation by analysis in SPSS 27.0 (SPSS Inc., Chicago, USA). Statistical analyses were conducted using GraphPad Prism version 9.5 for Windows (GraphPad Software, San Diego, CA, USA).
Results
Growth capability under typical HS temperature
Under typical HS temperature conditions (40°C), Lactobacillus plantarum (L5, L14, L17 and L19) entered the logarithmic growth phase after a lag period of 0–2 h and reached a stabilizer in about 10 h, showing excellent growth characteristics. However, Lactobacillus plantarum L20 entering the logarithmic growth phase after about 6 h and entering the stable phase after 16 h, showing relatively poor growth ability compared with the other four strains. Among the strains tested, L5 and L19 demonstrated robust proliferation capabilities, as evidenced by their OD600 values surpassing 1.0 upon reaching the plateau phase of growth. In contrast, the OD600 values for the remaining three strains did not rise above 1.0 throughout their entire growth cycles (Figure 1A).
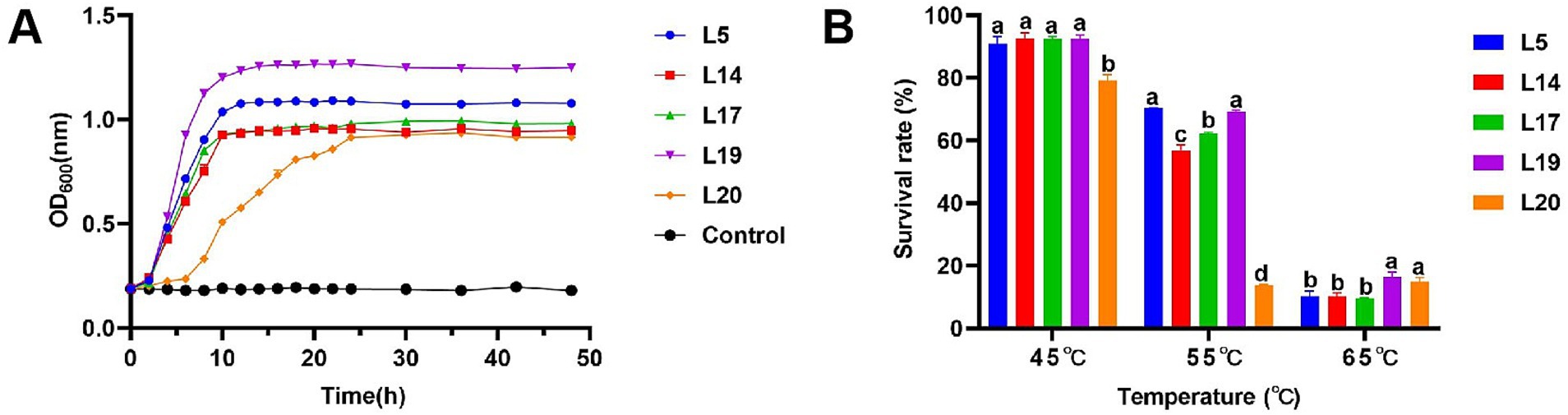
Figure 1. High temperature resistance of LAB. (A) Growth curve of LAB at 40°C; (B) Survival rate in different high temperature of LAB. The data are expressed as mean ± SD; Different letters represent significant difference (p < 0.05).
High temperature resistance of LAB
The heat resistance test revealed that as temperatures rose, the survival rates of the five bacterial strains decreased. At 45°C, their survival rates ranged from 79.25 to 92.62%. Notably, L20 (79.25%) exhibited the lowest survival rate, while L14 (92.64%) demonstrated the highest survival rate. L5 (90.91%), L17 (92.44%), L19 (92.36%), and L14 (92.64%) all exhibited commendable tolerance with no significant differences observed in their survival rates. At 55°C, survival rates dropped to between 13.88 and 70.39%. Among the five strains, L5 (70.39%) and L19 (69.22%) exhibited notably higher survival rates, which were significantly greater than the other three strains. At 65°C, the survival rates of the five strains ranged from 9.62 to 16.42%, with L19 (16.42%) showing the highest survival rate, followed by L20 (14.92%), L5 (10.26%), L14 (10.16%), L17 (9.62%). Analyzing the survival rate data across different temperatures, L5 and L19 exhibited relatively robust survival rates under various high-temperature conditions, indicating a more stable heat resistance in these strains (Figure 1B).
Antioxidative ability
Tolerance to H2O2 of LAB
The tolerance results of LAB to H2O2 are shown in Table 1. At concentrations of 0.5 mol/L H2O2, L5 (96.04%), and L19 (99.05%) exhibit strong tolerance, with their survival rates significantly surpassing those of the other three strains. When the H2O2 concentration is raised to 1 mmol/L, L19 (94.36%), L17 (92.15%), and L5 (80.89%) emerge as the most tolerant strains. At a concentration of 1.5 mmol/L, L5 (48.67%) demonstrates a particularly robust tolerance, significantly outperforming the other four strains. Finally, at an H2O2 concentration of 2 mmol/L, L19 (27.30%) showed the highest survival rate. Considering the survival rates of the strains across various concentrations, it is evident that L5, L17, and L19 exhibit relatively high tolerance to H2O2.
The scavenging ability of LAB to DPPH and OH− free radicals
The results of the DPPH and OH− free radical scavenging ability of the five strains are shown in Table 2. Among them, the CFS of L19 had the highest scavenging rate to DPPH (92.92%), followed by the CFS of L5 (90.86%) and L14 (89.71%). In addition, the CFS of L5 showed the highest ability to clear OH−, reaching 88.43%, which was significantly different from that of other strains (p < 0.05). The CFS of L19 (83.97%) and L14 (79.92%) followed in OH− scavenging capacity. In conclusion, L5, L14, and L19 strains exhibited robust abilities in scavenging both DPPH and OH− free radicals.
The ability to scavenge ABTS radicals
Figure 2 illustrates the ABTS scavenging capacity of the CFS and BS of LAB. Notably, the antioxidant capabilities of the CFS across all strains are markedly superior to those of the BS. The scavenging efficacy of the CFS across the five strains varied from 15.30 to 39.53%, while the BS demonstrated a less pronounced range, from 1.31 to 9.46%. Specifically, the CFS from strains L5 (39.04%) and L19 (39.53%) exhibited a particularly high ABTS scavenging ability, especially L19 which significantly differs from the other three strains (p < 0.05). Conversely, L20 exhibited the lowest scavenging rates in CFS (15.30%) and BS (1.31%).
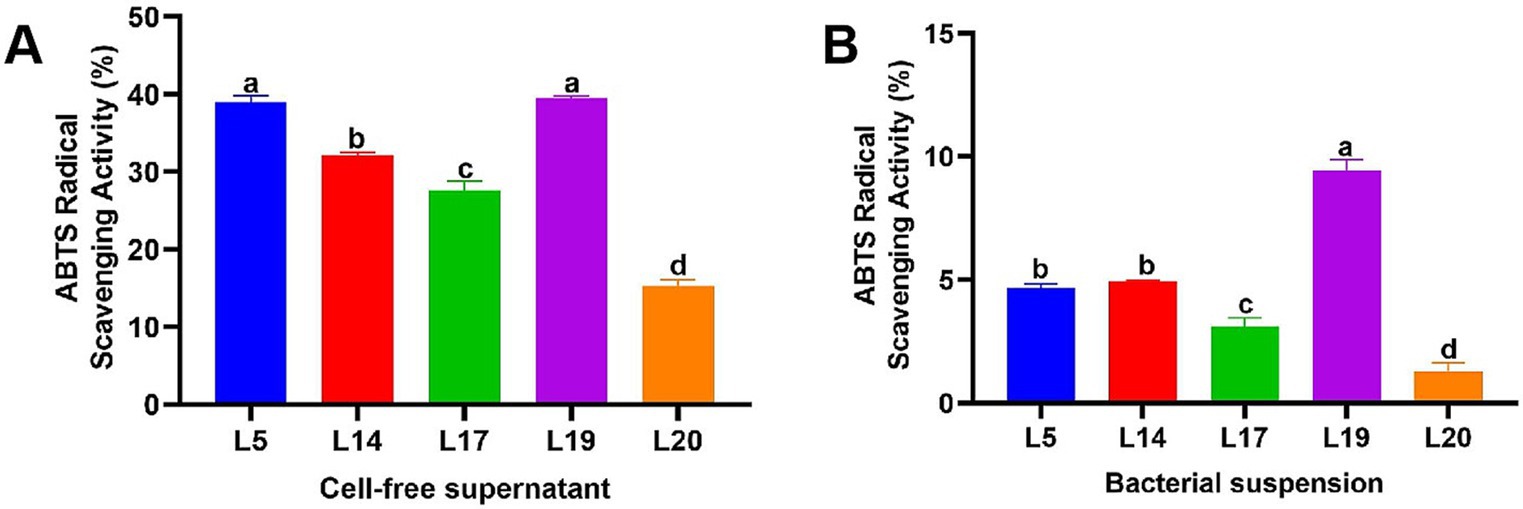
Figure 2. ABTS free radical scavenging rate of LAB. (A) ABTS free radical scavenging ability of the CFS; (B) ABTS free radical scavenging ability of the BS. The data are expressed as mean ± SD. Different letters represent significant difference (p < 0.05).
Reducing ability
Table 3 presents the results of the reducing capacity of LAB. It is evident that the reducing ability of the CFS from all five strains surpasses that of the bacterial BS. Among these, the CFS derived from L5 exhibited the most superior reducing ability, this was closely followed by the CFS of strains L19 and L14, which also exhibited considerable reducing properties. In terms of the BS, the reduction analysis revealed that L19 had the most potent reducing ability among the five strains, significantly outperforming the other four LAB strains in this regard (p < 0.05).
EPS production
Figure 3 displays the EPS production capabilities of the five strains. All five strains possess the ability to produce EPS, with strain L19 exhibiting the most robust production capacity at 581.34 mg/L, which significantly differs from the other four strains (p < 0.05). The EPS production of the other four strains were L14 (567.40 mg/L), L5 (546.5 mg/L), L20 (522.21 mg/L) and L17 (450.2 mg/L) respectively. Apart from strain L17 (450.2 mg/L), the remaining four strains all demonstrated EPS production capabilities exceeding 520 mg/L.
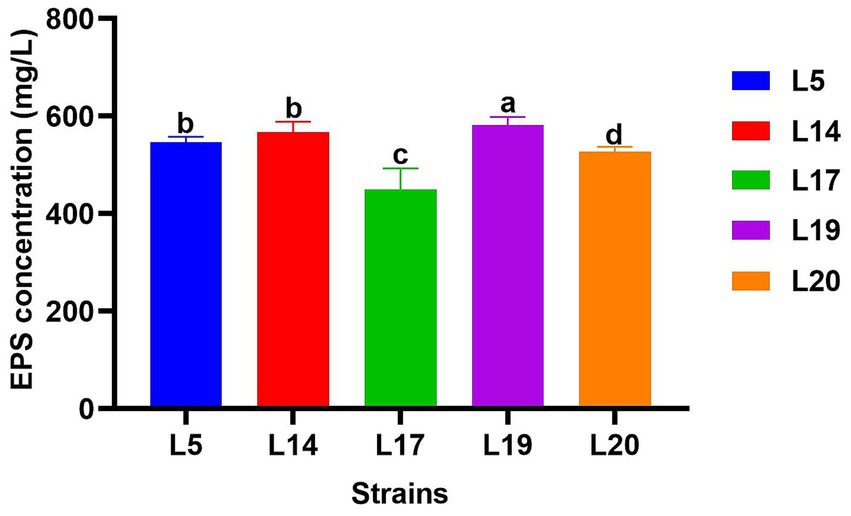
Figure 3. EPS production of LAB. The data are expressed as mean ± SD. Different letters represent significant difference (p < 0.05).
Adhesion rate to Caco-2 cell line
Figure 4 illustrates the adhesion abilities of the LAB to Caco-2 cells. All strains exhibited adhesion to these cells, with adhesion rates varying from 12.6 to 22.19%. Notably, L19 demonstrated the highest adhesion rate at 22.19%, which significantly differs from the other four strains (p < 0.05). The adhesion rates of the remaining strains ranked in descending order are L5 (16.20%), L14 (14.33%), L20 (13.01%) and L17 (12.60%).
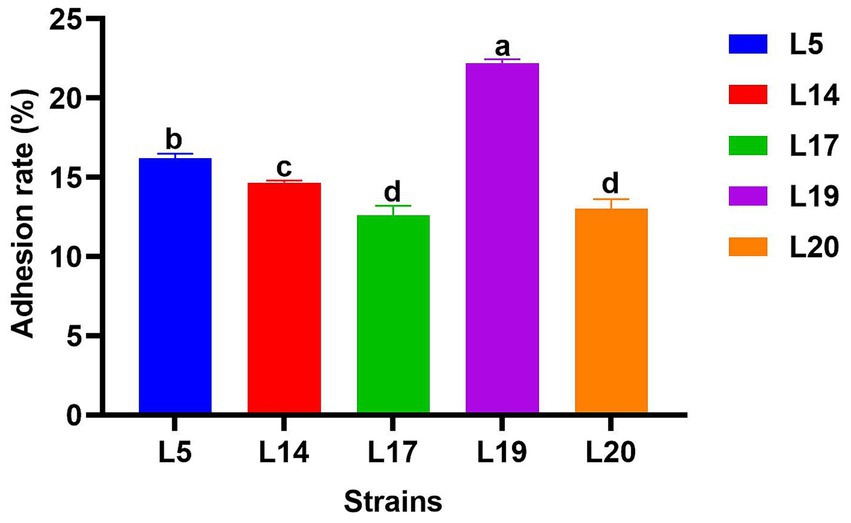
Figure 4. Adhesion rate of LAB to Caco-2 cell line. The data are expressed as mean ± SD. Different letters represent significant difference (p < 0.05).
Genomic analysis
Based on the comprehensive evaluation of heat resistance, antioxidant capacity of the five strains, L19 emerged as the most robust and stable in terms of heat resistance and antioxidant properties. Additionally, L19 possesses outstanding adhesion capabilities, holding promise for promising in vivo applications. Its exceptional characteristics position it as a promising candidate for the prevention and management of heat stress-related damage. Consequently, the genomic analysis of L19 was conducted, and the findings are presented as follows.
Genomic feature
The genome of Lactobacillus plantarum L19 comprises one chromosome and six plasmids. The total genome size is approximately 3,039,293 base pairs, with a GC content of 44.76%. It encodes a diverse array of RNAs (54 ncRNAs, 67 tRNAs and 16 rRNAs) and harbors one CRISPR sequence. The key genomic features of L19 are summarized in Supplementary Table S1, while the circular genome maps are depicted in Figure 5.
Functional annotation results
COG annotation
Figure 6A reveals that Lactobacillus plantarum L19 possesses 19 distinct types of coding genes, as cataloged in the COG database. A detailed examination of Figure 6A indicates that the majority of these genes are primarily associated with the categories of Transcription (247), Carbohydrate transport and metabolism (217), Amino acid transport and metabolism (196), and Replication, recombination, and repair (189).
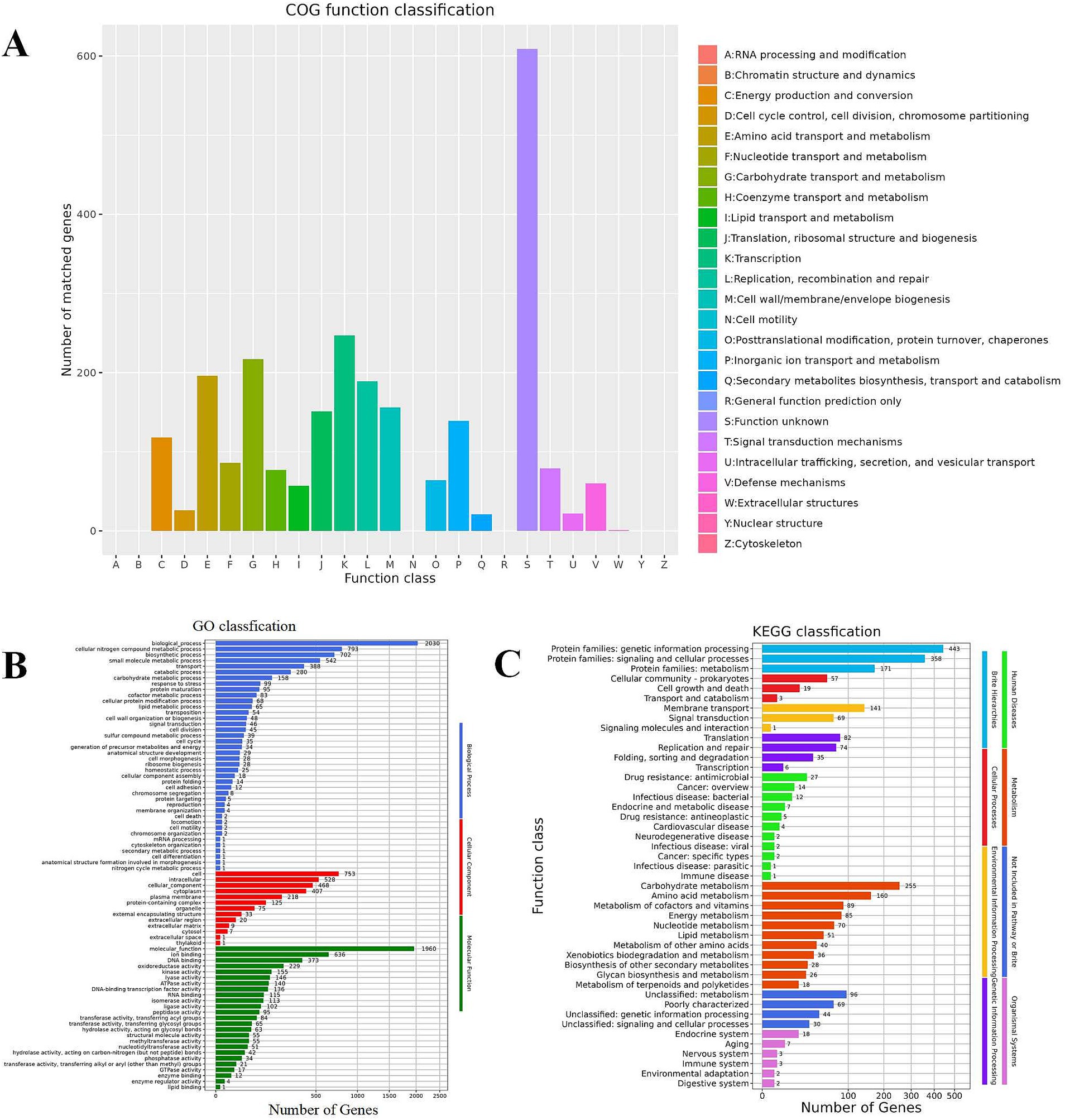
Figure 6. Function annotation results of Lactobacillus plantarum L19. (A) COG database annotation of Lactobacillus plantarum L19; (B) GO database annotation of Lactobacillus plantarum L19; (C) KEGG database annotation of Lactobacillus plantarum L19.
GO annotation
The results of the GO functional annotation for Lactobacillus plantarum L19 are depicted in Figure 6B. The GO system categorizes gene functions into three primary domains: Biological Process, Molecular Function, and Cellular Component. The analysis reveals that the majority of genes are primarily involved in cellular nitrogen compound metabolic processes, biosynthetic processes, ion binding, and small molecule metabolic processes. Notably, 229 genes are specifically associated with antioxidant reductase activity, highlighting the strain’s potential in antioxidant-related functions.
KEGG annotation
Figure 6C illustrates that Lactobacillus plantarum L19 has a total of 1,416 genes annotated in the KEGG database. These genes are categorized into 46 distinct pathways, encompassing eight major functional groups. The most prevalent pathways include Protein families involved in genetic information processing, signaling and cellular processes, and Carbohydrate metabolism. This distribution suggests that L19 possesses robust metabolic capabilities and a strong potential for proliferation. Notably, 85 genes are annotated in the energy metabolism pathway, which is positively correlated with the strain’s antioxidant capacity.
Antioxidant-related genes in the genome of Lactobacillus plantarum L19
The genome of Lactobacillus plantarum L19 was analyzed to identify genes associated with the antioxidant enzyme system, encompassing catalase, superoxide dismutase (SOD), the thioredoxin system, the NADH oxidase-NADH peroxidase system and the glutathione system. As presented in Supplementary Table S2, the L19 genome contains 17 genes related to antioxidant activity.
Heat-resistant genes in the genome of Lactobacillus plantarum L19
According to the study by Da et al. (Da et al., 2023), as shown in Supplementary Table S3, four genes are intimately associated with the heat resistance of LAB (adhE, hisE, ykuN and folB). The genome of Lactobacillus plantarum L19 harbors 3 out of the 4 heat resistance-related genes (adhE, hidE, and folB).
AMR genes in the genome of Lactobacillus plantarum L19
No AMR genes were identified in the genomes of Lactobacillus plantarum L19 using ResFinder 4.0.
Discussion
As global temperatures escalate, the dairy industry faces an increasingly dire challenge from HS, necessitating the urgent development of effective strategies to prevent and mitigate HS-related damages in dairy cows (Ranjitkar et al., 2020). Research shows that enhancing the body’s antioxidant defenses can significantly reduce damage caused by HS. LAB, known for their robust antioxidant properties, safety, and accessibility, have been demonstrated to bolster the body’s antioxidant defenses and counteract oxidative damage (Nakagawa and Miyazaki, 2017), representing a promising intervention for heat stress-related damage in dairy cows. It is widely recognized that probiotics sourced from the same host exhibit greater compatibility with the host, thereby optimizing their probiotic efficacy and enhancing the overall utility of probiotic preparations (Argyri et al., 2013). Therefore, this study aims to screen out heat-resistant and antioxidant strains from cows’ milk to provide effective means and scientific references for preventing and treating of damage caused by HS in dairy cows.
Maintaining superior growth characteristics is crucial for a strain to exert its probiotic properties effectively. Thus, the chosen strain for mitigating HS must withstand high temperatures while retaining growth viability, a factor easily overlooked in strain screening. During HS, body temperature can exceed the normal range, typically reaching 40 ± 0.5°C, which is comparable to the rectal temperature of cows during heat stress (Abeyta et al., 2023; Ebi et al., 2021; Qi et al., 2022). The intestine is the primary site where probiotics exert their beneficial effects. Consequently, in this study, 40°C was used to simulate temperature under HS to evaluate the growth capabilities of LAB under HS. The evaluation results of heat resistance and high temperature of five strains showed L5 and L19 exhibited superior heat resistance and sustained robust growth capabilities under the simulated temperature of HS, which indicates that these two strains can exert good probiotic properties under HS conditions. Furthermore, most probiotic products undergo a spray-drying process (involving high temperatures) during manufacturing, which can significantly impact the survival rate of the strains (Simpson et al., 2005). Therefore, screening strains with excellent thermal stability is also conducive to commercial production.
HS in cows induces oxidative stress through the production of free radicals and peroxides, which is a major cause of oxidative damage caused by HS (Gupta et al., 2023). To combat this, strains with notable antioxidant capabilities are essential. There are various methods to assess probiotics’ in vitro antioxidant capacity, including H2O2 tolerance, reducing and free radical scavenging (DPPH, OH−, ABTS) (Zhou et al., 2022). In this study, the antioxidant capacity of five strains was evaluated using the above methods. The results showed that the five strains had different degrees of antioxidant capacity, among which L5 and L19 showed stronger antioxidant capacity, which are exceeded current reports (Li et al., 2012; Liu et al., 2020). Notably, the supernatant’s antioxidant capacity exceeded that of the bacterial suspension, aligning with Wang et al.’s findings (Wang et al., 2023). This indicates that the antioxidant capacity of the supernatant in vitro is stronger than that of the bacterial suspension, but the difference in the effects in vivo still needs further study. Extracellular polysaccharides (EPS) are natural carbohydrate polymers secreted by LAB during growth. Studies show EPS have antioxidant properties (Wang et al., 2015). This study assessed EPS production in five LAB strains, all capable of producing EPS. The results is in contrast to Espeche et al. (Espeche et al., 2012), in their study none of the LAB isolated from milk produced EPS, possibly due to environmental or strain differences.
For LAB to function as probiotics in the host, their ability to adhere effectively to the intestinal lining is essential (Collado et al., 2006). This study utilized Caco-2 cells to assess the adhesion capabilities of LAB strains. The adhesion rates of the five LAB strains ranged from 12.6 to 22.19%, significantly higher than the 0% reported for Lactobacillus plantarum isolated from food by Pinto et al. (Pinto et al., 2020), and the 3.62% for Lactobacillus plantarum G83 isolated from panda feces by Wang et al. (Wang et al., 2023). These findings indicate that the LAB strains in this study possess a strong ability to adhere and colonize, which can guarantee its effective probiotic effect in the host body.
Based on a comprehensive evaluation of various attributes, L19 showed excellent heat resistance and antioxidant capabilities, and exhibited superior adhesive capacity and utilization rate, making it a promising candidate for managing the damages of heat stress in cows. To understand its biological role and molecular mechanisms, L19’s genome was sequenced and annotated using GO, KEGG, and COG databases, revealing that L19 possesses a diverse array of antioxidation-related enzymes and energy metabolism pathways, aligning with previous findings (Aziz et al., 2023). Antioxidant enzymes such as superoxide dismutase (SOD) and catalase, the thioredoxin system, the NADH oxidase-NADH peroxidase system, and the glutathione system are collectively recognized as the primary enzymatic defense mechanisms employed by LAB to counteract oxidative stress (Zhou et al., 2022). The genome of L19 was found to harbor 17 genes associated with antioxidant functions, shedding light on its molecular basis for exceptional antioxidant properties. The study of Da et al. on Lactobacillus plantarum CGMCC8198 identified adhE-like, hisE, ykuN, and folB genes as vital for heat resistance, with hisE being significant. These genes serve as potential markers for heat-tolerant LAB selection (Da et al., 2023). Genomic analysis of L19 confirmed the presence of adhE-like, hisE, and folB genes, aligning with its demonstrated heat tolerance, further emphasizing its potential for withstanding high temperatures. This research represents the first comprehensive analysis of heat-resistant and antioxidant genes in LAB from cow’s milk.
Considering the potential for microbiomes to acquire and transfer antibiotic resistance genes to pathogenic bacteria, posing significant risks (Pinto et al., 2020), the resfinder 4.0 tool was used to analyze the genome of L19 for drug resistance genes (Bortolaia et al., 2020). Fortunately, no drug resistance genes were detected, mitigating concerns about gene transmission contributing to antibiotic resistance. Additionally, the L19 genome sequence was found to contain a CRISPR array, characterized by short, repetitive sequences that are homologous to prophages or plasmids. This feature not only limits the spread of antimicrobial resistance genes but also provides a defense mechanism against incoming extrachromosomal DNA molecules (Marraffini et al., 2006). Overall, these findings confirm the L19 is a safe and reliable strain of LAB.
Conclusion
In this investigation, a LAB strain (Lactobacillus plantarum L19) was screened from the milk of Holstein cows, which exhibited impressive heat resistance and antioxidant capabilities, holding promise for the prevention of heat stress-related damage in dairy cows. The heat resistance and antioxidant capacity of Lactobacillus plantarum L19 were verified from the molecular level by genomic analysis, and it did not carry drug resistance genes, ensuring its safety. This study offers a valuable reference and a solid scientific foundation for the application of LAB in preventing and controlling the oxidative damage of HS in dairy cows.
Data availability statement
The data presented in this study can be found in the NCBI database, accession numbers: SRR28342006.
Ethics statement
Ethical approval was not required for the studies on humans in accordance with the local legislation and institutional requirements because only commercially available established cell lines were used. Ethical approval was not required for the studies on animals in accordance with the local legislation and institutional requirements because only commercially available established cell lines were used.
Author contributions
JW: Writing – original draft, Writing – review & editing. YL: Writing – original draft, Writing – review & editing. HZ: Writing – original draft, Writing – review & editing. JX: Supervision, Validation, Writing – review & editing. ZhZ: Data curation, Supervision, Validation, Writing – review & editing. HL: Supervision, Validation, Visualization, Writing – review & editing. YH: Supervision, Validation, Writing – review & editing. HF: Supervision, Validation, Writing – review & editing. ZiZ: Conceptualization, Project administration, Writing – review & editing. GP: Conceptualization, Project administration, Writing – review & editing.
Funding
The author(s) declare that financial support was received for the research, authorship, and/or publication of this article. This study was supported by the Science and Technology Support Project of Science and Technology Department of Sichuan Province “Integrated Research and Demonstration of Key Technologies of Gastrointestinal Flora Disturbance and Its Regulation in Perinatal Dairy Cows” (2011NZ0060).
Acknowledgments
We extend our gratitude to Nanxing Li for his invaluable support in conducting this study and contributing to the improvement of this manuscript.
Conflict of interest
The authors declare that the research was conducted in the absence of any commercial or financial relationships that could be construed as a potential conflict of interest.
Publisher’s note
All claims expressed in this article are solely those of the authors and do not necessarily represent those of their affiliated organizations, or those of the publisher, the editors and the reviewers. Any product that may be evaluated in this article, or claim that may be made by its manufacturer, is not guaranteed or endorsed by the publisher.
Supplementary material
The Supplementary material for this article can be found online at: https://www.frontiersin.org/articles/10.3389/fmicb.2024.1455849/full#supplementary-material
References
Abeyta, M. A., Al-Qaisi, M., Horst, E. A., Mayorga, E. J., Rodriguez-Jimenez, S., Goetz, B. M., et al. (2023). Effects of dietary antioxidant supplementation on metabolism and inflammatory biomarkers in heat-stressed dairy cows. J. Dairy Sci. 106, 1441–1452. doi: 10.3168/jds.2022-22338
Alam, M. N., Bristi, N. J., and Rafiquzzaman, M. (2013). Review on in vivo and in vitro methods evaluation of antioxidant activity. Saudi Pharm. J. 21, 143–152. doi: 10.1016/j.jsps.2012.05.002
Amaretti, A., Di Nunzio, M., Pompei, A., Raimondi, S., Rossi, M., and Bordoni, A. (2013). Antioxidant properties of potentially probiotic bacteria: in vitro and in vivo activities. Appl. Microbiol. Biotechnol. 97, 809–817. doi: 10.1007/s00253-012-4241-7
Argyri, A. A., Zoumpopoulou, G., Karatzas, K. G., Tsakalidou, E., Nychas, G. E., Panagou, E. Z., et al. (2013). Selection of potential probiotic lactic acid bacteria from fermented olives by in vitro tests. Food Microbiol. 33, 282–291. doi: 10.1016/j.fm.2012.10.005
Aziz, T., Naveed, M., Jabeen, K., Shabbir, M. A., Sarwar, A., Zhennai, Y., et al. (2023). Integrated genome based evaluation of safety and probiotic characteristics of lactiplantibacillus plantarum yw11 isolated from Tibetan kefir. Front. Microbiol. 14:1157615. doi: 10.3389/fmicb.2023.1157615
Bagath, M., Krishnan, G., Devaraj, C., Rashamol, V. P., Pragna, P., Lees, A. M., et al. (2019). The impact of heat stress on the immune system in dairy cattle: a review. Res. Vet. Sci. 126, 94–102. doi: 10.1016/j.rvsc.2019.08.011
Bernabucci, U., Biffani, S., Buggiotti, L., Vitali, A., Lacetera, N., and Nardone, A. (2014). The effects of heat stress in Italian Holstein dairy cattle. J. Dairy Sci. 97, 471–486. doi: 10.3168/jds.2013-6611
Bortolaia, V., Kaas, R. S., Ruppe, E., Roberts, M. C., Schwarz, S., Cattoir, V., et al. (2020). Resfinder 4.0 for predictions of phenotypes from genotypes. J. Antimicrob. Chemother. 75, 3491–3500. doi: 10.1093/jac/dkaa345
Cai, H., Qin, D., Liu, Y., Guo, X., Liu, Y., Ma, B., et al. (2023). Remodeling of gut microbiota by probiotics alleviated heat stroke-induced necroptosis in male germ cells. Mol. Nutr. Food Res. 67:e2300291. doi: 10.1002/mnfr.202300291
Cartwright, S. L., McKechnie, M., Schmied, J., Livernois, A. M., and Mallard, B. A. (2021). Effect of in-vitro heat stress challenge on the function of blood mononuclear cells from dairy cattle ranked as high, average and low immune responders. BMC Vet. Res. 17, 233–211. doi: 10.1186/s12917-021-02940-8
Christen, F., Desrosiers, V., Dupont-Cyr, B. A., Vandenberg, G. W., le François, N. R., Tardif, J., et al. (2018). Thermal tolerance and thermal sensitivity of heart mitochondria: mitochondrial integrity and ros production. Free Radic. Biol. Med. 116, 11–18. doi: 10.1016/j.freeradbiomed.2017.12.037
Collado, M. C., Jalonen, L., Meriluoto, J., and Salminen, S. (2006). Protection mechanism of probiotic combination against human pathogens: in vitro adhesion to human intestinal mucus. Asia Pac. J. Clin. Nutr. 15, 570–575
Conesa, A., and Götz, S. (2008, 2008). Blast2go: a comprehensive suite for functional analysis in plant genomics. Int. J. Plant Genomics 2008:619832. doi: 10.1155/2008/619832
da, J. Y., Xi, M., Li, H., Liu, M., Zhou, C., Li, Z., et al. (2023). Transcriptome analysis and functional gene identification reveals potential mechanisms of heat stress response of lactiplantibacillus plantarum cgmcc8198. Food Biosci. 51:102202:102202. doi: 10.1016/j.fbio.2022.102202
Ebi, K. L., Capon, A., Berry, P., Broderick, C., de Dear, R., Havenith, G., et al. (2021). Hot weather and heat extremes: health risks. Lancet 398, 698–708. doi: 10.1016/S0140-6736(21)01208-3
Espeche, M. C., Pellegrino, M., Frola, I., Larriestra, A., Bogni, C., and Nader-Macías, M. F. (2012). Lactic acid bacteria from raw milk as potentially beneficial strains to prevent bovine mastitis. Anaerobe 18, 103–109. doi: 10.1016/j.anaerobe.2012.01.002
Fan, C., Su, D., Tian, H., Li, X., Li, Y., Ran, L., et al. (2018). Liver metabolic perturbations of heat-stressed lactating dairy cows. Asian Australas. J. Anim. Sci. 31, 1244–1251. doi: 10.5713/ajas.17.0576
Foongsawat, N., Sunthornthummas, S., Nantavisai, K., Surachat, K., Rangsiruji, A., Sarawaneeyaruk, S., et al. (2023). Isolation, characterization, and comparative genomics of the novel potential probiotics from canine feces. Food Sci. Anim. Resour. 43, 685–702. doi: 10.5851/kosfa.2023.e28
Grissa, I., Vergnaud, G., and Pourcel, C. (2007). Crisprfinder: a web tool to identify clustered regularly interspaced short palindromic repeats. Nucleic Acids Res. 35, W52–W57. doi: 10.1093/nar/gkm360
Gupta, S., Sharma, A., Joy, A., Dunshea, F. R., and Chauhan, S. S. (2023). The impact of heat stress on immune status of dairy cattle and strategies to ameliorate the negative effects. Animals 13:107. doi: 10.3390/ani13010107
Jang, H., Son, S., Kim, J., Jung, M. Y., Choi, Y., Kim, D., et al. (2021). Characterization and functional test of canine probiotics. Front. Microbiol. 12:625562. doi: 10.3389/fmicb.2021.625562
Kalvari, I., Argasinska, J., Quinones-Olvera, N., Nawrocki, E. P., Rivas, E., Eddy, S. R., et al. (2018). Rfam 13.0: shifting to a genome-centric resource for non-coding rna families. Nucleic Acids Res. 46, D335–D342. doi: 10.1093/nar/gkx1038
Kelleher, P., Bottacini, F., Mahony, J., Kilcawley, K. N., and van Sinderen, D. (2017). Comparative and functional genomics of the lactococcus lactis taxon; insights into evolution and niche adaptation. BMC Genomics 18, 1–20. doi: 10.1186/s12864-017-3650-5
Koch, F., Thom, U., Albrecht, E., Weikard, R., Nolte, W., Kuhla, B., et al. (2019). Heat stress directly impairs gut integrity and recruits distinct immune cell populations into the bovine intestine. Proc. Natl. Acad. Sci. 116, 10333–10338. doi: 10.1073/pnas.1820130116
Kuda, T., and Ikemori, T. (2009). Minerals, polysaccharides and antioxidant properties of aqueous solutions obtained from macroalgal beach-casts in the noto peninsula, Ishikawa, Japan. Food Chem. 112, 575–581. doi: 10.1016/j.foodchem.2008.06.008
Li, Y., Pan, M., Meng, S., Xu, W., Wang, S., Dou, M., et al. (2024). The effects of zinc oxide nanoparticles on antioxidation, inflammation, tight junction integrity, and apoptosis in heat-stressed bovine intestinal epithelial cells in vitro. Biol. Trace Elem. Res. 202, 2042–2051. doi: 10.1007/s12011-023-03826-6
Li, L., Tan, H., Zou, Z., Gong, J., Zhou, J., Peng, N., et al. (2020). Preventing necroptosis by scavenging ros production alleviates heat stress-induced intestinal injury. Int. J. Hyperth. 37, 517–530. doi: 10.1080/02656736.2020.1763483
Li, S., Zhao, Y., Zhang, L., Zhang, X., Huang, L., Li, D., et al. (2012). Antioxidant activity of lactobacillus plantarum strains isolated from traditional Chinese fermented foods. Food Chem. 135, 1914–1919. doi: 10.1016/j.foodchem.2012.06.048
Liu, W., Chen, M., Duo, L., Wang, J., Guo, S., Sun, H., et al. (2020). Characterization of potentially probiotic lactic acid bacteria and bifidobacteria isolated from human colostrum. J. Dairy Sci. 103, 4013–4025. doi: 10.3168/jds.2019-17602
Liu, N., Miao, S., and Qin, L. (2020). Screening and application of lactic acid bacteria and yeasts with l-lactic acid-producing and antioxidant capacity in traditional fermented rice acid. Food Sci. Nutr. 8, 6095–6111. doi: 10.1002/fsn3.1900
Liu, Y., Wang, Z., Xie, W., Gu, Z., Xu, Q., and Su, L. (2017). Oxidative stress regulates mitogen-activated protein kinases and c-jun activation involved in heat stress and lipopolysaccharide-induced intestinal epithelial cell apoptosis. Mol. Med. Rep. 16, 2579–2587. doi: 10.3892/mmr.2017.6859
Mahmoodian, S., Fatemi, S. S., Shamsara, M., Chaharmahali, M., Meimandipour, A., and Maniee, S. A. (2024). Impact of protectants and the method of preservation on the stability of potentially probiotic bacteria. Cryobiology 116:104912. doi: 10.1016/j.cryobiol.2024.104912
Marraffini, L. A., DeDent, A. C., and Schneewind, O. (2006). Sortases and the art of anchoring proteins to the envelopes of gram-positive bacteria. Microbiol. Mol. Biol. Rev. 70, 192–221. doi: 10.1128/MMBR.70.1.192-221.2006
Matsushita, K., Azuma, Y., Kosaka, T., Yakushi, T., Hoshida, H., Akada, R., et al. (2016). Genomic analyses of thermotolerant microorganisms used for high-temperature fermentations. Biosci. Biotechnol. Biochem. 80, 655–668. doi: 10.1080/09168451.2015.1104235
Mishra, V., Shah, C., Mokashe, N., Chavan, R., Yadav, H., and Prajapati, J. (2015). Probiotics as potential antioxidants: a systematic review. J. Agric. Food Chem. 63, 3615–3626. doi: 10.1021/jf506326t
Moore, T., Globa, L., Pustovyy, O., Vodyanoy, V., and Sorokulova, I. (2014). Oral administration of bacillus subtilis strain bsb3 can prevent heat stress-related adverse effects in rats. J. Appl. Microbiol. 117, 1463–1471. doi: 10.1111/jam.12606
Moriya, Y., Itoh, M., Okuda, S., Yoshizawa, A. C., and Kanehisa, M. (2007). Kaas: an automatic genome annotation and pathway reconstruction server. Nucleic Acids Res. 35, W182–W185. doi: 10.1093/nar/gkm321
Nakagawa, H., and Miyazaki, T. (2017). Beneficial effects of antioxidative lactic acid bacteria. Aims Microbiol. 3, 1–7. doi: 10.3934/microbiol.2017.1.1
Peiris, A. N., Jaroudi, S., and Noor, R. (2017). Heat stroke. JAMA 318:2503. doi: 10.1001/jama.2017.18780
Pinto, A., Barbosa, J., Albano, H., Isidro, J., and Teixeira, P. (2020). Screening of bacteriocinogenic lactic acid bacteria and their characterization as potential probiotics. Microorganisms 8:393. doi: 10.3390/microorganisms8030393
Prete, R., Garcia-Gonzalez, N., Di Mattia, C. D., Corsetti, A., and Battista, N. (2020). Food-borne lactiplantibacillus plantarum protect normal intestinal cells against inflammation by modulating reactive oxygen species and il-23/il-17 axis. Sci. Rep. 10:16340. doi: 10.1038/s41598-020-73201-1
Qi, Y., Zhang, Y., Zhang, J., Wang, J., and Li, Q. (2022). The alteration of n6-methyladenosine (m6a) modification at the transcriptome-wide level in response of heat stress in bovine mammary epithelial cells. BMC Genomics 23:829. doi: 10.1186/s12864-022-09067-6
Ranjitkar, S., Bu, D., van Wijk, M., Ma, Y., Ma, L., Zhao, L., et al. (2020). Will heat stress take its toll on milk production in China? Clim. Chang. 161, 637–652. doi: 10.1007/s10584-020-02688-4
Rhoads, M. L. (2023). Review: reproductive consequences of whole-body adaptations of dairy cattle to heat stress. Animal 17:100847. doi: 10.1016/j.animal.2023.100847
Schubert, M., Lindgreen, S., and Orlando, L. (2016). Adapterremoval v2: rapid adapter trimming, identification, and read merging. BMC. Res. Notes 9, 1–7. doi: 10.1186/s13104-016-1900-2
Simpson, P. J., Stanton, C., Fitzgerald, G. F., and Ross, R. P. (2005). Intrinsic tolerance of bifidobacterium species to heat and oxygen and survival following spray drying and storage. J. Appl. Microbiol. 99, 493–501. doi: 10.1111/j.1365-2672.2005.02648.x
Stothard, P., and Wishart, D. S. (2005). Circular genome visualization and exploration using cgview. Bioinformatics 21, 537–539. doi: 10.1093/bioinformatics/bti054
Thornton, P., Nelson, G., Mayberry, D., and Herrero, M. (2022). Impacts of heat stress on global cattle production during the 21st century: a modelling study. Lancet Planetary Health 6, e192–e201. doi: 10.1016/S2542-5196(22)00002-X
Walker, B. J., Abeel, T., Shea, T., Priest, M., Abouelliel, A., Sakthikumar, S., et al. (2014). Pilon: an integrated tool for comprehensive microbial variant detection and genome assembly improvement. PLoS One 9:e112963. doi: 10.1371/journal.pone.0112963
Wang, J., Pu, Y., Zeng, Y., Chen, Y., Zhao, W., Niu, L., et al. (2023). Multi-functional potential of five lactic acid bacteria strains derived from giant panda (ailuropoda melanoleuca). Probiotics Antimicrob. Proteins 15, 668–681. doi: 10.1007/s12602-021-09881-6
Wang, H., Xing, G., Qian, Y., Sun, X., Zhong, J., and Chen, K. (2021). Dihydromyricetin attenuates heat stress-induced apoptosis in dairy cow mammary epithelial cells through suppressing mitochondrial dysfunction. Ecotoxicol. Environ. Saf. 214:112078. doi: 10.1016/j.ecoenv.2021.112078
Wang, Y., Yang, C., Elsheikh, N. A. H., Li, C., Yang, F., Wang, G., et al. (2019). Ho-1 reduces heat stress-induced apoptosis in bovine granulosa cells by suppressing oxidative stress. Aging (Albany NY) 11, 5535–5547. doi: 10.18632/aging.102136
Wang, J., Zhao, X., Yang, Y., Zhao, A., and Yang, Z. (2015). Characterization and bioactivities of an exopolysaccharide produced by lactobacillus plantarum yw32. Int. J. Biol. Macromol. 74, 119–126. doi: 10.1016/j.ijbiomac.2014.12.006
Zhang, W., Lai, S., Zhou, Z., Yang, J., Liu, H., Zhong, Z., et al. (2022). Screening and evaluation of lactic acid bacteria with probiotic potential from local Holstein raw milk. Front. Microbiol. 13:918774. doi: 10.3389/fmicb.2022.918774
Zhao, M., Liu, K., Zhang, Y., Li, Y., Zhou, N., and Li, G. (2023). Probiotic characteristics and whole-genome sequence analysis of pediococcus acidilactici isolated from the feces of adult beagles. Front. Microbiol. 14:1179953. doi: 10.3389/fmicb.2023.1179953
Zhou, Y., Gong, W., Xu, C., Zhu, Z., Peng, Y., and Xie, C. (2022). Probiotic assessment and antioxidant characterization of lactobacillus plantarum gxl94 isolated from fermented chili. Front. Microbiol. 13:997940. doi: 10.3389/fmicb.2022.997940
Keywords: lactic acid bacteria, Lactobacillus plantarum, heat resistance, antioxidant capacity, heat stress, dairy cows
Citation: Wang J, Liu Y, Zheng H, Xin J, Zhong Z, Liu H, Huang Y, Fu H, Zhou Z and Peng G (2024) Screening and genome analysis of heat-resistant and antioxidant lactic acid bacteria from Holstein cow milk. Front. Microbiol. 15:1455849. doi: 10.3389/fmicb.2024.1455849
Edited by:
Astghik Zaveni Pepoyan, Armenian National Agrarian University, ArmeniaReviewed by:
Xiaolu Liu, University of Science and Technology Beijing, ChinaNing Ma, Hebei Agricultural University, China
Copyright © 2024 Wang, Liu, Zheng, Xin, Zhong, Liu, Huang, Fu, Zhou and Peng. This is an open-access article distributed under the terms of the Creative Commons Attribution License (CC BY). The use, distribution or reproduction in other forums is permitted, provided the original author(s) and the copyright owner(s) are credited and that the original publication in this journal is cited, in accordance with accepted academic practice. No use, distribution or reproduction is permitted which does not comply with these terms.
*Correspondence: Guangneng Peng, cGduLnNpY2F1QDE2My5jb20=; Ziyao Zhou, ODM5NTQ5MDNAcXEuY29t
†These authors have contributed equally to this work