- 1Laboratory of Anaerobic Microbiology, Institute of Animal Physiology and Genetics, CAS, Prague, Czechia
- 2Department of Veterinary Medicine, University of Sassari, Sassari, Italy
- 3Department of Microbiology, Nutrition and Dietetics, Faculty of Agrobiology, Food and Natural Resources, Czech University of Life Sciences, Prague, Czechia
- 4Institute for Clinical and Experimental Medicine, Diabetes Centre, Prague, Czechia
- 5Department of Internal Medicine, Second Faculty of Medicine, Charles University, Prague, Czechia
Diabetes mellitus represents a significant global health problem. The number of people suffering from this metabolic disease is constantly rising and although the incidence is heterogeneous depending on region, country, economic situation, lifestyle, diet and level of medical care, it is increasing worldwide, especially among youths and children, mainly due to lifestyle and environmental changes. The pathogenesis of the two most common subtypes of diabetes mellitus, type 1 (T1DM) and type 2 (T2DM), is substantially different, so each form is characterized by a different causation, etiology, pathophysiology, presentation, and treatment. Research in recent decades increasingly indicates the potential role of the gut microbiome in the initiation, development, and progression of this disease. Intestinal microbes and their fermentation products have an important impact on host metabolism, immune system, nutrient digestion and absorption, gut barrier integrity and protection against pathogens. This review summarizes the current evidence on the changes in gut microbial populations in both types of diabetes mellitus. Attention is focused on changes in the abundance of specific bacterial groups at different taxonomic levels in humans, and microbiome shift is also assessed in relation to geographic location, age, diet and antidiabetic drug. The causal relationship between gut bacteria and diabetes is still unclear, and future studies applying new methodological approaches to a broader range of microorganisms inhabiting the digestive tract are urgently needed. This would not only provide a better understanding of the role of the gut microbiome in this metabolic disease, but also the use of beneficial bacterial species in the form of probiotics for the treatment of diabetes.
1 Introduction
Diabetes mellitus (DM) is a metabolic disease characterized by hyperglycemia resulting from insufficient control of blood glucose levels and impaired insulin secretion (Petersmann et al., 2018). DM is classified into several categories. The main types are type 1 (T1DM) and type 2 (T2DM), and other forms include maturity-onset diabetes of the young (MODY) (Horikawa, 2018), gestational diabetes (Buchanan and Xiang, 2005), neonatal diabetes (Beltrand et al., 2020), steroid-induced diabetes or Latent Autoimmune Diabetes in Adults (LADA) (Carlsson, 2019) and genetically determined metabolic disorders connected with abnormal glucose metabolism (Vekic et al., 2022). Diabetes prevalence in Europe is very high. According to the International Diabetes Federation, 9.2% of people suffer from DM and this number is expected to increase to 13% by 2045 (International Diabetes Federation, 2021). China and India are particularly affected by this disease, experiencing a dramatic increase in T2DM prevalence despite having a relatively low rate of obesity (DeFronzo et al., 2015). The reasons for the development of diabetes can be different and depend on many aspects. Genetic predisposition, family history of diabetes, health status, ethnic background, inactive lifestyle, unhealthy eating habits, and obesity are considered the most common risk factors that can lead to diabetes (Gowd et al., 2019; Li W.-Z. et al., 2020). The rapid increase in disease incidence, which is expected to reach 700 million people worldwide by 2045 (Saeedi et al., 2019), indicates that environmental factors presumably play a key role in this phenomenon. In the last decade, the role of the gut microbiota in the development of this disease has been implicated as an important factor. Based on the knowledge that human intestinal microbiome play an essential role in health and disease, including the development of a fully functional immune system (Lynch and Pedersen, 2016; Zheng et al., 2020), the gut microbiome received considerable attention as a phenomenon involved in the development and progression of DM disease. The causal relationship between gut bacteria and diabetes is still unclear, and future studies on the pathophysiological role of the gut microbiome in DM are expected and needed. The current status of research and understanding of the relationship between gut microorganisms and diabetes is described here, with a focus on the human microbiome.
2 Pathogenesis of type 1 and type 2 DM
T1DM and T2DM are the most common subtypes. Type 1 occurs mainly in children or adolescents (Katsarou et al., 2017), while type 2 usually affects middle-aged and elderly adults who have persistent hyperglycemia mainly due to genetic variants, inappropriate lifestyle and dietary habits. The pathogenesis of these two types is meaningly different, so each type is characterized by a different etiology, pathophysiology, presentation, and treatment.
T1DM is characterized by elevated blood glucose levels (hyperglycemia) caused by deficient insulin production due to destruction of the β-cells of the pancreatic islets of Langerhans predominantly as a result of autoimmune inflammation (Katsarou et al., 2017). The classic view is that autoreactive T cells mistakenly destroy healthy β-cells, resulting in insulin deficiency that causes hyperglycemia in patients with subsequent overproduction of glucose by the liver via glycogenolysis and gluconeogenesis enhanced by glucagon and decreased ability of glucose cellular uptake and degradation by peripheral tissues, such as muscle and adipose tissue (Eiselein et al., 2004). However, recent opinions suggest the alternative view that the key contributors to disease are the β-cells themselves, which dysfunction and destruction is controlled by their own metabolic activity. In fact, β-cells are susceptible to biosynthetic stress and have limited self-defense mechanisms. When under stress, β-cells trigger an immune response that can significantly impair the production of a vital hormone (Roep et al., 2020).
While patients with T1DM are rarely obese (but the presence of obesity is not incompatible with the diagnosis), T2DM is closely associated with obesity and metabolic syndrome. Type 2 diabetes is a metabolic disease in which the driving force for insulin resistance and impaired insulin secretion lies in patient’s overweight or obesity. Obesity is characterized by chronic low-grade inflammation associated with increased secretion of pro-inflammatory cytokines from adipose tissue and infiltration of leukocytes, including macrophages, into adipose tissue. Chronic inflammation thus impairs insulin signaling in adipocytes, leading to insulin resistance and the development of metabolic disorders (Kim and Nam, 2020). Approximately 86% of T2DM patients are overweight (Thingholm et al., 2019) and obesity-induced insulin resistance is the major underlying pathophysiological factor. T2DM is more common than T1DM and accounts for more than 90% of all cases. These data indicate that this disease has become a global pandemic and the number of patients is rapidly increasing, especially in industrialized countries (Khan et al., 2020).
DM patients suffer from acute and chronic complications. Hypoglycemia and hyperglycemia are the most frequent acute symptoms and in certain cases could be life-threatening. Chronic complications are common in both T1DM and T2DM patients and are responsible for significant morbidity and mortality. These types of complications are generally divided into microvascular and macrovascular. Microvascular complications are more common and include neuropathy, diabetic kidney disease, and retinopathy. Macrovascular complications induce cardiovascular comorbidities leading to ischemic heart disease, stroke and peripheral artery disease (Lotfy et al., 2017). Diabetic foot (DF) is also a common problem associated with diabetes, which can ultimately lead to lower limb amputation (Tuttolomondo et al., 2015; Yazdanpanah et al., 2018; Pourkazemi et al., 2020 Sechovcová et al., 2023). Growing evidence also indicate that lung injury could be one of the long-term consequences of diabetes (Wang G. et al., 2021).
3 Healthy intestinal microbiome
Microorganisms in the gastrointestinal tract have received considerable attention in the last two decades, and intensive research on the gut microbiota and microbiome has revealed their irreplaceable importance for human health. Intestinal bacteria, together with archaea, fungi, and viruses, are now considered a “virtual organ” providing essential metabolic functions which cannot be performed by the mammalian host (Koboziev et al., 2014). Apart from the obvious contribution to degradation of complex carbohydrates, resulting in the production of energy and health important short-chain fatty acids (SCFA), there are many other critical functions that have been gradually discovered. Gut microbiota synthesizes essential vitamins, eliminates toxins, maintains the integrity of the intestinal epithelium, regulates development, homeostasis, and function of the innate and adaptive immune systems, regulates gut endocrine function and neurological signaling, produces neuroactive compounds, and participates in a bidirectional network of signaling pathways known as the gut-brain axis (Morowitz et al., 2011; Koboziev et al., 2014; Rutsch et al., 2020; Zheng et al., 2020; Fan and Pedersen, 2021) and gut-skin axis (De Pessemier et al., 2021; Patel et al., 2022). Recent research also shows a close correlation between the gut microbiome and respiratory disorders referred to as a gut-lung axis (Enaud et al., 2020; Tang et al., 2021).
Human gut microbiota is rich consortium of microorganisms, in which bacteria form the most numerous part, reaching a density of about 1012 cells/g of colon contents. About 500–1,000 different species are highly variable at lower taxonomic levels, but generally belong to four dominant phyla. Firmicutes and Bacteroidetes account for more than 90% of the population, while Actinobacteria and Proteobacteria are less represented (< 1–5%) (Almeida et al., 2019; Rutsch et al., 2020). Other phyla, including Verrucomicrobia, Planctomycetes, and Spirochaetes, have very low abundance but contain some important beneficial bacterial species (e.g., Akkermansia muciniphila in Verrucomicrobia) (Rinninella et al., 2019). Despite their low abundance, members of the rare biosphere can play a crucial role in the gut microbiome’s metabolic processes. They may significantly enhance microbial diversity and contribute to the stability of the human gut. Intestinal rare taxa may be involved in detoxification of ingested chemicals, digestion of unusual food components and stimulation of the human immune system, thus promoting overall gut health and resilience (American Society for Microbiology, 2011; Bhute et al., 2017a).However, no uniform optimal composition of intestinal microbiome can be determined because the gut microbiota of each individual is different, highly variable at the genus level, and characterized by a specific combination of bacterial species (Rinninella et al., 2019). Therefore, the concept of a healthy microbiome cannot be described unambiguously. On the other hand, there are some generally accepted parameters, characteristics and taxonomic traits associated with a healthy gut. Species richness, diversity, and a stable functional microbial core are indicative of a good gut condition. The richer and more diverse the microbiome, the better the intestinal community resists extrinsic threatening influences (Rinninella et al., 2019; Manor et al., 2020). This is associated with the high functional response diversity, which is defined as the extent to which species in a community that contribute to the same ecosystem function vary in their sensitivity to changes in the ecosystem (Lozupone et al., 2012). Research has shown that a richer, more diverse and balanced microbiome composition, referred to as eubiosis (Iebba et al., 2016; Bajinka et al., 2020), represents a promising therapeutic target for addressing various conditions influenced by the microbiome, including infections caused by serious pathogens (Goldberg et al., 2014; Seekatz et al., 2022). Another prerequisite for a healthy microbiome is the presence of bacterial species capable of decomposing structural polysaccharides in the diet. This fermentation results in the production of short-chain fatty acids (SCFAs), and important is also the biosynthesis of some essential amino acids and vitamins. SCFAs, mainly acetate, butyrate, and propionate, have a positive effect on intestinal health by maintaining intestinal barrier integrity, mucus production, protection against inflammation, glucose homeostasis, and immunomodulation (Silva et al., 2020; Portincasa et al., 2022). SCFAs influence the occurrence and development of various diseases, and the roles of individual volatile fatty acids vary depending on the disease (Fan and Pedersen, 2021; Zhang D. et al., 2023). Of particular importance is butyrate, which provides an energy source for colonocytes and plays a significant physiological role (Singh et al., 2023). Major butyrate producers in the human gut include strains of Faecalibacterium prausnitzii, Eubacterium rectale, Eubacterium hallii, Ruminococcus bromii, Butyricicoccus pullicaecorum, Roseburia spp. (R. faecis, R. inulinivorans, R. intestinalis, R. hominis) and Anaerostipes spp. (A. butyraticus, A. caccae, A. hadrus) (Louis et al., 2010; Plöger et al., 2012; Rivière et al., 2016) (see Table 1). King et al. (2019) recently published a list of healthy human reference microbiome and an abundance profile. This list, called GutFeelingKB, is based on the combination of the complete National Center for Biotechnology Information NCBI non-redundant nucleotide database and sequences of healthy people from the Human Microbiome Project (HMP). A comprehensive methodology was used to link bacterial species with intestinal health. Healthy participants provided fecal samples, which were sequenced and analyzed using bioinformatic pipelines. CensuScope and HIVE-hexagon were used to generate taxonomic profiles, while MaAsLin and cosine similarity coefficients linked bacterial abundance to clinical and dietary data. The resulting profile provides a summary of 157 organisms (8 phyla, 18 classes, 23 orders, 38 families, 59 genera, and 109 species) that can be used as healthy controls for studies related to dysbiosis.
Lower microbiome diversity and low abundance of SCFA-producing bacteria is a common feature of many diseases, not only chronic intestinal inflammations such as Crohn’s disease and ulcerative colitis, but also most metabolic and autoimmune diseases, including both types of diabetes mellitus.
A recent article of Wu et al. (2024) also makes a remarkable advance in the definition of a healthy microbiome. The authors constructed co-abundance networks of high-quality metagenome-assembled genomes based on 26 datasets including healthy controls, individuals under various environmental perturbations, dietary regimes and patients with 15 different diseases and discovered a core set of health-relevant microbiomes. All bacteria from the beneficial microbiome of the group of healthy subjects belonged to the phylum Firmicutes. Their genomes were rich in CAZy genes, which are crucial for the dietary fiber digestion (cellulose, arabinoxylan) and the production of butyrate, but had a lower proportion of genes for inulin utilization and propionate production. The detrimental microbiome was composed of five different phyla, including Firmicutes, Bacteroidota, Proteobacteria, Actinobacteriota, and Fusobacteriota, and their genomes were rich in genes conferring antibiotic resistance and expressing virulence factors that may activate a pathogenic interaction with the host. Surprisingly, the data indicate that a high abundance of certain species of microbes is not so important as previously thought, while stable microbial interaction is critical parameter of healthy microbiome (Wu et al., 2024).
4 Gut microbiota and T1DM
The role of the gut microbiome in T1DM activation is poorly understood, and there is still insufficient evidence to unequivocally support the idea that intestinal microorganisms activate this disease. A causal relationship between the gut microbiota and T1DM has not yet been disclosed and remains unclear. The complexity and diversity of the microbiota, as well as inter-individual variations, make it difficult to confirm cause-effect relationships. In addition, the interactions between microbiota and disease can be bidirectional, making it even more difficult to provide clear evidence. Therefore, it is not yet evident whether gut microbial changes are the cause or the effect of the disease. Understanding this relationship is further complicated by the fact that the onset and manifestation of the disease occurs in children and young adults, whose intestinal microbiota undergoes dynamic development (Knip and Siljander, 2016). However, the link between the pathogenesis of insulin dysfunction and alterations in the microbial composition of people suffering from T1DM seems obvious. Several studies have observed reduced bacterial diversity and low numbers of butyrate-producing bacteria (Brown et al., 2011; Giongo et al., 2011; Vaarala, 2012; De Goffau et al., 2013; Qi et al., 2016). Analysis at the family level indicated in T1DM subjects significantly increased Bacteroidaceae (De Goffau et al., 2013; Leiva-Gea et al., 2018), Rikenellaceae (Giongo et al., 2011; Leiva-Gea et al., 2018), Prevotellaceae (Leiva-Gea et al., 2018), Ruminococcaceae (Leiva-Gea et al., 2018), Veillonellaceae (Giongo et al., 2011; Leiva-Gea et al., 2018), Streptococcaceae (Leiva-Gea et al., 2018), and Enterobacteriaceae (Soyucen et al., 2014; Leiva-Gea et al., 2018), while Lachnospiraceae (Giongo et al., 2011; Leiva-Gea et al., 2018), Bifidobacteriaceae (Leiva-Gea et al., 2018) and Eubacteriaceae (Giongo et al., 2011) were significantly more abundant in healthy controls. At the genus level the T1DM subjects were enriched with Bacteroides (Brown et al., 2011; Giongo et al., 2011; De Goffau et al., 2013; Leiva-Gea et al., 2018), Prevotella (Leiva-Gea et al., 2018), Blautia (Qi et al., 2016; Leiva-Gea et al., 2018), Veillonella (Brown et al., 2011; Leiva-Gea et al., 2018), Streptococcus (Brown et al., 2011; De Goffau et al., 2014; Leiva-Gea et al., 2018), Clostridium (Giongo et al., 2011; De Goffau et al., 2013, 2014), Sutterella (Leiva-Gea et al., 2018), Enterobacter (Leiva-Gea et al., 2018), Alistipes (Brown et al., 2011), and Ruminococcus (Giongo et al., 2011; De Goffau et al., 2014; Leiva-Gea et al., 2018).
On the other hand, the abundance of strains of Lachnospira (Qi et al., 2016; Leiva-Gea et al., 2018), Roseburia (Brown et al., 2011; De Goffau et al., 2013; Cinek et al., 2018; Leiva-Gea et al., 2018), Anaerostipes (Brown et al., 2011; Leiva-Gea et al., 2018), Faecalibacterium (Brown et al., 2011; Giongo et al., 2011; Huang et al., 2018; Leiva-Gea et al., 2018), Eubacterium (Brown et al., 2011; Giongo et al., 2011; Cinek et al., 2018), Bifidobacterium (Soyucen et al., 2014; Leiva-Gea et al., 2018), Akkermansia (Brown et al., 2011), and Lactobacillus (De Goffau et al., 2014; Alkanani et al., 2015) was reduced in diabetics with T1DM and significantly increased in healthy controls. However, in the work of Brown et al. (2011), Prevotella was much more abundant in controls, and the lactate producers including Lactobacillus, Lactococcus, and Bifidobacterium were increased in T1DM cases, contradicting the findings of Leiva-Gea et al. (2018) and Alkanani et al. (2015). Several strains of Bifidobacteria and Lactobacilli have been nevertheless successfully tested as probiotics showing beneficial effects both in pediatric (Groele et al., 2017; Kumar et al., 2021) and adult (Ahola et al., 2017; Zhang X. et al., 2023) T1DM patients. However, the effect strongly depends on the particular strain(s) and it still remains questionable which probiotic bacteria are potentially the most useful for treatment of T1DM (Groele et al., 2021).
It is evident that the aforementioned shifts in the microbiome of T1DM individuals have been observed in a number of studies and seem to be characteristic for diabetes 1 disease, but on the other hand, the results are highly dependent on geographic location, age, gender, and diet. Cinek et al. (2018), who studied children and adolescents from African and Asian countries, described a positive association of T1DM with the genus Escherichia, which was twice more abundant in patients compared to controls. Huang et al. (2018) observed increased Ruminococcaceae and Veillonellaceae in healthy subjects in a Chinese cohort, which contradicts the findings of Leiva-Gea et al. (2018) and Giongo et al. (2011). In studies with non-European populations (Mejía-León et al., 2014; Cinek et al., 2018; Huang et al., 2018), no decreased bacterial diversity was observed in T1DM patients. Alkanani et al. (2015) and De Goffau et al. (2013, 2014) indicated that changes in abundances of certain bacterial groups, particularly in the Bacteroides phylum, may be age-dependent, in facts, Bacteroidetes were found to be more prevalent in the younger diabetic children and this finding was also observed in the recent work of Mokhtari et al. (2021). In particular, the genus Bacteroides, seems to play a crucial role in the development of T1DM, probably through the production of glutamate decarboxylase, which can induce glutamic acid decarboxylase autoimmunity via molecular mimicry (De Goffau et al., 2014). Giongo et al. (2011) observed the decreased diversity with increasing age in young children with development of T1DM. Bacterial changes associated with diabetes are summarized in Supplementary Table S1 showing unambiguous findings, while Supplementary Table S2 outlines controversial results achieved by different author groups.
T1DM is a complex disease that affects a variety of biochemical and immunological processes in the body, and research brings many other important new findings. One interesting aspect is changes in the metabolomic profile, which reflect the autoimmune nature of diabetes. Lipidomic changes are characterized by higher levels of low-carbon-number saturated lipid classes, including myristic, stearic and palmitic acid (Arneth et al., 2019), and lower levels of certain sphingolipids and glycerophospholipids (Greenhill, 2018; Arneth et al., 2019). The shift in amino acids (AA) profile is often associated with an increase in circulating AA, particularly branched chain AA, during insulin deprivation in T1DM individuals (Charlton and Nair, 1998; Hebert and Nair, 2009). Research also shows that T1DM patients suffer from altered intestinal barrier function (Dedrick et al., 2020). The increased gut permeability, often referred to as leaking gut syndrome, allows antigens and pathogens to cross the intestinal barrier, which may trigger or exacerbate immune responses against pancreatic beta cells, contributing to the autoimmune attack characteristic for T1DM (Vaarala, 2018; Li and Atkinson, 2015). This phenomenon once again emphasizes the significance and importance of the intestinal microorganisms. Microbiota-host interactions significantly influence immune regulation and inflammatory responses, which are central to the autoimmune destruction of pancreatic beta cells (Majumdar et al., 2022). Dysbiosis in T1DM is associated with an increase in pro-inflammatory cytokines, such as IL-17 and IFN-γ, which can further exacerbate the autoimmune attack on pancreatic tissue (Li et al., 2015; Roep et al., 2020). Changes in microbial composition can therefore modulate immune signaling and influence the progression of T1DM.
Diet and lifestyle definitely have a significant impact on onset and incidence of T1DM, and both aspects are closely related to the composition of the gut microbiome. In childhood, breastfeeding and late inclusion of gluten, fruit, and cow’s milk in the diet may reduce the risk of T1DM (Lampousi et al., 2021). On the other hand, over the life course, increased risk is associated with inappropriate dietary habits, such as frequent consumption of foods and beverages with a high glycemic index (GI) and a diet low in fiber and rich in saturated fats (Piłaciński and Zozulińska-Ziółkiewicz, 2014).
All of these aspects and factors are reflected in the varying geographic distribution of T1DM, with lower incidence in most Asian populations (with the exception of Kuwait) and low or intermediate incidence in African populations. Incidence in South American populations varied from very low to high, and the highest incidence was found in North American and European populations. Finland, Sweden, and Norway are known to be the three countries with the highest T1DM incidences (Paun et al., 2017; Xia et al., 2019). However, the incidence of this disease is on the rise worldwide, especially among children. Regions that previously had a lower incidence tend to show a steep annual increase, whereas countries with an already established high incidence show a moderate increase or even stabilization in the incidence of T1DM (Xia et al., 2019). Geographic location is undoubtedly associated with a specific type of diet that influences the gut microbiome. Exploring this relationship certainly deserves attention and could help clarify the link between dietary habits and T1DM. A proinflammatory diet (mainly rich in high-fat dairy products and sugar-sweetened beverages and low in fiber content and vegetable) can induce alterations in the composition of the microbiota and may lead to intestinal inflammation and modification of the metabolic and immunological profile within the intestinal mucosa of patients with TD1M (Vatanen et al., 2018). Notably, the onset of T1DM is usually preceded by clinical signs of modification on mucus layer and intestinal inflammation associated with lymphocyte infiltration, increased permeability, and the presence of inflammatory cytokines within the intestinal mucosa (Secondulfo et al., 2004; Sorini et al., 2019). The functional impairment of gut barrier integrity is linked to the pathogenesis of T1DM rather than being a secondary effect of diabetes-induced metabolic changes. It can lead to the uncontrolled passage of bacterial components into the systemic circulation, directly contributing to beta cell damage, or activating beta cell autoimmunity through pancreatic lymph nodes and tissues (Sun et al., 2015). An alternative mechanism involves the activation of autoimmune T cells specifically targeting beta cell antigens. These T cells are activated by the presentation of the cognate antigens by professional antigen-presenting cells in an inflammatory context. Research emphasizes the critical role of the intestinal innate immune system in disrupting T cell tolerance to islet beta cells during episodes of gut dysbiosis. The changes in gut microbial diversity can cause a shift toward pro-inflammatory state, which compromises the integrity of the intestinal barrier. This weakened barrier allows bacterial components to penetrate the leaky gut and trigger activation of innate immune cells. The resulting inflammatory state creates favorable conditions for the activation of diabetogenic T cells, which contribute to beta cell damage, ultimately leading to the development of autoimmune type 1 diabetes (Turley et al., 2005; Majumdar et al., 2022).
5 Gut microbiota and T2DM
Type 2 diabetes mellitus (T2DM) is a complex chronic metabolic disorder, that differs in many aspects from T1DM. Dysregulation of carbohydrate, lipid, and protein metabolism, typical for T2DM, is followed by insulin resistance or impaired insulin secretion, or a combination of both (DeFronzo et al., 2015). The risk factors for T2DM appear to be clearer than for T1DM and are closely related to environmental factors such as obesity and unwholesome lifestyle characterized by an unhealthy diet and physical inactivity. Although other factors such as mental stress, infections, and genetic predisposition should also be considered, obesity appears to be the most important factor contributing to the pathophysiological disturbances responsible for impaired glucose homeostasis. Obesity, characterized by excessive fat accumulation and low grade systemic and chronic inflammation, is closely related to the composition of the intestinal microbiota, and several works have highlighted a crucial role of the gut microbiota in the development of T2DM (Lambeth et al., 2015; Tai et al., 2015; Thingholm et al., 2019; Sikalidis and Maykish, 2020). The effect of inflammation on intestinal bacteria has not been yet fully understood, but systemic inflammation negatively affects the barrier function and immune system due to the action of pro-inflammatory cytokines, and consequently affects the composition and diversity of the microbiota (Riedel et al., 2022). Impaired gut barrier function can even result in metabolic endotoxemia (Sharma and Tripathi, 2019; Mohammad and Thiemermann, 2021). This adverse state is characterized by a diet-induced increase of bacterial lipopolysaccharide (LPS) levels in plasma, which leads to low-grade inflammation contributing to the development of a metabolic disease phenotype (Cani et al., 2007). Figure 1 presents the interplay between diet, gut microbiome and gut barrier dysfunction in diabetes mellitus.
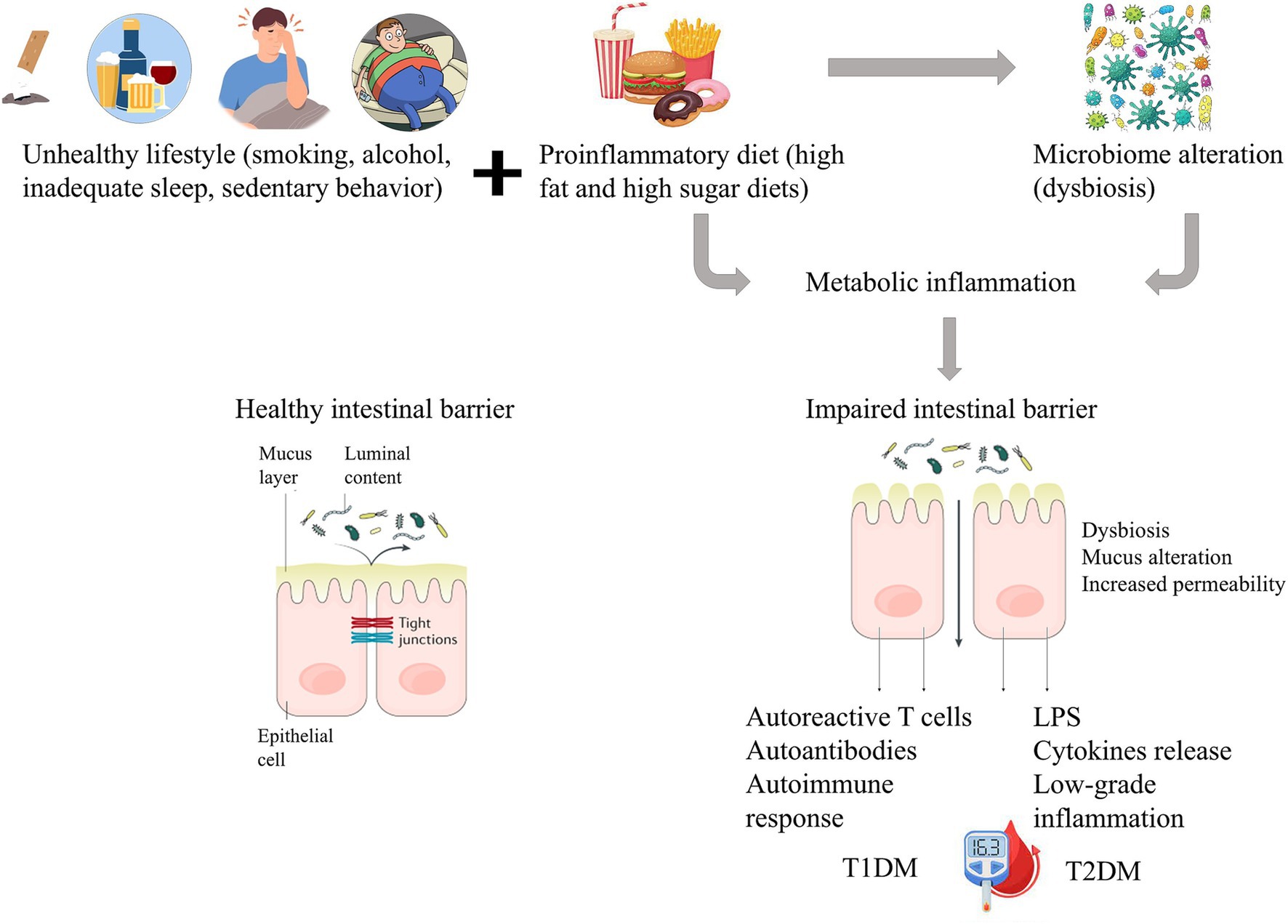
Figure 1. Schematic representation of the interplay between diet, gut microbiome, and intestinal barrier dysfunction in diabetes. In TD1M the increased gut permeability triggers an autoimmune response causing beta cell damage. In TD2M dysbiosis leads to low-grade inflammation and a reduction in insulin sensitivity.
Gut bacterial diversity and the number of butyrate-producing bacteria are significantly lower in patients with T2DM compared with healthy individuals (Qin et al., 2012; Tilg and Moschen, 2014; Candela et al., 2016; Zhao et al., 2019; Gurung et al., 2020), consistent with the same trend seen in type 1 diabetes. However, the results at the different taxonomic levels are quite contradictory, as described below.
Within Firmicutes, Clostridia was found to be dominant, however Larsen et al. (2010) found that this class was significantly lower in adult TD2 patients, whereas Zhang et al. (2013) described the opposite. The higher abundance of non-butyrate producing and opportunistic pathogenic Clostridiales was also observed by Karlsson et al. (2013) and Tilg and Moschen (2014), respectively, while according to Doumatey et al. (2020) the Clostridiaceae and Peptostreptococcaceae families (both class Clostridia) were lower in T2DM subjects. Within Proteobacteria, the class Betaproteobacteria was greatly increased in TD2 patients (Larsen et al., 2010; Zhang et al., 2013).
At the genus level, T2DM subjects were enriched with Prevotella (Zhang et al., 2013; Doumatey et al., 2020), Dorea (Zhang et al., 2013), Subdoligranulum (Qin et al., 2012; Zhang et al., 2013), Ruminococcus (Zhang et al., 2013; Candela et al., 2016), Eubacterium (Zhang et al., 2013; Zhao et al., 2019; Doumatey et al., 2020), Sporobacter (Zhang et al., 2013), Abiotrophia (Zhang et al., 2013), Peptostreptococcus (Zhang et al., 2013; Doumatey et al., 2020), Collinsella (Zhang et al., 2013; Lambeth et al., 2015; Candela et al., 2016), and Lactobacilus (Sato et al., 2014; Sedighi et al., 2017). Increased levels of Coprococcus, Blautia, the Eubacterium hallii group, and Parasutterella were described in T2DM cases by Zhao et al. (2019). However, these results are not consistently found in all published works. Opposite findings, i.e., significantly decreased abundances, were reported in T2DM patients for Subdoligranulum (Forslund et al., 2015; Zhang et al., 2019; Cunningham et al., 2021), Prevotella (Zhao et al., 2019), Eubacterium (Qin et al., 2012), Ruminococcus (Zhang et al., 2019), or Collinsella (Doumatey et al., 2020). Larsen et al. (2010) did not observe significant changes in relative abundances at the bacterial genus level.
At the species level, the increase of Bacteroides caccae, Clostridium hathewayi, Clostridium ramosum, Clostridium symbiosum, Eggerthella lenta, and Escherichia coli was described by Qin et al. (2012) in T2DM patients based on a metagenome-wide association study in Chinese cohort. Wu et al. (2020) described highly significant shifts (p < 0.001, mostly a decrease) in the abundance of 27 bacterial species in T2DM patients. Karlsson et al. (2013) described increased abundance of four Lactobacillus species and decreased abundance of five Clostridium species in the T2DM group of European women. The shifts in the microbiome in T2DM patients are presented in Supplementary Tables S1, S2. The inconsistency of the results can be caused by several parameters, in our opinion the composition of the study group is decisive, especially the age, gender, nationality, diet and lifestyle of the included individuals. However, assessing this problem is complicated as it can be influenced by a number of factors.
While the above mentioned genera and species are considered to be positively associated with T2DM, there is a group of beneficial gut bacteria that may play an important role in preventing and possibly treating the diabetes disease. Most studies have described low numbers of butyrate-producing bacteria such as Eubacterium rectale, Faecalibacterium prausnitzii, Roseburia sp. in T2DM patients, which were enriched only in healthy controls (Furet et al., 2010; Qin et al., 2012; Karlsson et al., 2013; Zhang et al., 2013, 2019; Forslund et al., 2015; Wu et al., 2020; Kwan et al., 2022). Butyrate is known to affect insulin sensitivity (Vrieze et al., 2012; Sanna et al., 2019), therefore, increased butyrate synthesis caused by an enhancement of the butyrate-producing intestinal bacteria is considered as one of the methods to prevent or treat diabetes (Zhang et al., 2021). On the other hand, butyrate can promote postprandial insulin secretion and propionate formation in the stool, which increases the risk of T2DM (Sanna et al., 2019; Zhang et al., 2021). Therefore, the role of propionate in T2DM will require more attention. In general, propionate is considered to be beneficial for health (Louis and Flint, 2017) showing positive effects on β-cell function, leading to increased insulin secretion (Pingitore et al., 2017). However, Sanna et al. (2019) described that abnormalities in propionate production or absorption are causally associated with increased T2DM risk.
Bifidobacterium is also considered a bacterium potentially protective against T2DM. This genus is consistently decreased in diabetics (Candela et al., 2016; Sedighi et al., 2017; Zhao et al., 2019; Ejtahed et al., 2020; Wu et al., 2020; He et al., 2023). It is well established that Bifidobacteria provide positive health benefits to their host through their metabolic activities by providing nutrients by degrading indigestible carbohydrates from the diet (Derrien et al., 2022), protecting against pathogens through competitive exclusion, reducing and treating gastrointestinal infections (O’Callaghan and van Sinderen, 2016), and eliciting various immune effects (e.g., decrease of pro-inflammatory cytokines such as TNF-α, IFN-γ, IL-2, IL-6, IL-17, IL-22, and IL-12; increase the number of CD4+ T cells, and IgA plasma cells) in the host (Dong et al., 2022). The effect of Bifidobacteria is intensively studied (Qian et al., 2022), but there is still a lack of knowledge about the molecular mechanisms explaining probiotic properties of Bifidobacterium strains.
Akkermansia muciniphila is thought to be negatively associated with T2DM, its decreased abundance in diabetic patients has been reported by several authors (Vaarala, 2013; Tilg and Moschen, 2014; Zhao et al., 2019; Gurung et al., 2020). A. muciniphila is an important bacterium that colonizes the human intestinal mucosa. Its metabolic activity is related to the integrity of the mucus layer, increasing mucus thickness, improving intestinal barrier function, and eliciting immune responses (Ottman et al., 2017). Studies in rodents showed a positive effect of A. muciniphila on host glucose metabolism (Everard et al., 2013; Greer et al., 2016). These findings induced the idea that A. muciniphila could be considered a promising next-generation probiotic (Ottman et al., 2017; Ropot et al., 2020). Several works however described elevated levels of this bacterium in T2DM cases (Qin et al., 2012; Zhang et al., 2013, 2021), suggesting that the results are inconclusive. Cekanaviciute et al. (2017) even described increased abundance of A. muciniphila in multiple sclerosis patients, raising the question of whether A. muciniphila is a beneficial bacterium with exclusively positive effects. Together with the above results, the possible use of A. muciniphila as a probiotic agent in diabetic patients should be supported by further thorough research.
Faecalibacterium prausnitzii is considered a beneficial bacterium providing positive health benefits. Its reduction is associated with various inflammatory diseases and several studies have reported a decrease in F. prausnitzii abundance in individuals with T2DM (Furet et al., 2010; Qin et al., 2012; Karlsson et al., 2013; Kwan et al., 2022). This species is crucial for the maintenance of gut homeostasis by producing short-chain fatty acids (SCFAs), which support gut epithelial integrity and contribute to protection against systemic inflammatory diseases (Effendi et al., 2022). The production of butyrate by F. prausnitzii is especially important, as it has anti-inflammatory effects, improves insulin sensitivity, and maintains the integrity of the intestinal epithelium (Maioli et al., 2021). Supplementation with F. prausnitzii has been associated with an improvement in gut barrier function and a reduction in inflammation, suggesting its potential as a therapeutic probiotic strain (He et al., 2021).
The metabolomic profile in T2DM, which is primarily driven by insulin resistance, shows clear changes compared to healthy controls, especially elevated levels of branched-chain amino acids (BCAAs), aromatic amino acids, and changes in organic acids (Roberts et al., 2014). Particularly, increased valine, maltose, glutamate, urate, and decreased glucuronolactone, lysine and lactate have been reported (Zhang et al., 2014). Unlike T1DM, lipid profile changes are less explored, but include higher concentrations of low-carbon lipids, such as glycerophospholipids. Organic acids and certain purine and urea cycle metabolites, such as arginine and citrulline, are also elevated in T2DM patients (Arneth et al., 2019).
Regarding gut permeability in T2DM patients, the impaired intestinal barrier function is associated rather to metabolic factors than autoimmunity (Di Vincenzo et al., 2024). Poor glycemic control weakens barrier integrity, increases inflammatory responses, and potentially exacerbates insulin resistance (Yuan et al., 2021). T2DM patients often have elevated levels of serum lipopolysaccharides (LPS), zonulin, and intestinal fatty acid-binding protein (IFABP), indicating compromised barrier function. This impairment contributes to chronic inflammation by allowing bacterial endotoxins to enter the bloodstream, which can exacerbate insulin resistance (Yuan et al., 2021).
The prevalence of T2DM is rising worldwide, and statistical data indicate a global epidemiology with no signs of stabilization. Similar to T1DM, type 2 diabetes is more widespread in U.S.A (11.6%) and developed regions of Western Europe, particularly in the Netherlands, Switzerland, and Sweden, where it exceeds 10%. Alarming is also the number of prediabetics and undiagnosed individuals whose world number is estimated at 30 and 44%, respectively (International Diabetes Federation, 2021). There are no gender differences in T2DM prevalence; men have a slightly higher incidence than women without being statistically significant, and men tend to be diagnosed at a younger age (Kautzky-Willer et al., 2023). Age is an important factor, as T2DM occurs in adults affecting about 15% of people at the age 50–70 years, 22% of the population over 70 years, but only 4.4% of younger people (15–49 years old) (Khan et al., 2020). These factors are linked to diet and lifestyle, and all of these aspects are related to the composition of the gut microbiome. Research focused on comparing different groups, whether by age, nationality, gender, medication, or health status, could elucidate specific changes in the microbiome and help understand the role of intestinal bacteria in T2DM disease in more specific contexts, with a greater opportunity to favorably influence gut microbiome composition.
6 Antidiabetic drugs and gut microbiome
As described above, in T1DM patients the beta cells in the pancreas are destroyed by the immune system and can no longer produce insulin. In T2DM patients, insulin is still produced, but the body is unable to use it properly due to insulin resistance. These characteristics are therefore reflected in a different treatment approach. People with type 1 diabetes must be treated by insulin (through multiple daily injections or an insulin pump), whereas in type 2 diabetes, blood glucose levels are lowered, insulin sensitivity and insulin secretion is improved through oral medication, with metformin generally being the first choice. However, several other classes of peroral glucose-lowering drugs, including selective inhibitor of sodium-glucose cotransporter 2 (SGLT2i), glucagon-like peptide 1 (GLP-1) receptor agonists, inhibitors of dipeptidyl peptidase 4 (DPP-4 inhibitors or gliptins), insulin sensitizer thiazolidinediones (glitazones), and insulin secretagogue sulfonylureas, may be used (Yang T. et al., 2023).
Antidiabetic drugs are directly or indirectly influenced by the composition of the gut microbiota, which may affect the therapeutic efficacy of treatment (Crudele et al., 2023). To assess the effect of insulin on intestinal bacteria, it would be necessary to compare the same patient group before treatment and at several time points after treatment, which would be very problematic to perform since patients with Type 1 DM are treated by insulin immediately from the beginning of their disease. Therefore, it is impossible to uncover the real impact of insulin on gut microbiota. However, studies show a negative correlation between HbA1c levels and the abundance of the family Ruminococcaceae (Mrozinska et al., 2021), the genus Faecalibacterium (Huang et al., 2018) and the genus Akkermansia muciniphila (Fassatoui et al., 2019) in T1DM patients, suggesting a positive effect of butyrate-producing bacteria.
Evaluating the effect of orally administered antidiabetic drugs is more straightforward because these medicinal substances pass through the digestive tract and interact with the gut microbiota. Intestinal bacteria can be sensitive to administered drugs or can chemically modified them and thus influence their pharmacological effect. The ability of human gut bacteria to metabolize orally administered drugs was documented by Zimmermann et al. (2019), who mapped 76 intestinal bacterial species and showed their metabolizing effect on two-thirds of 271 tested drugs (Zimmermann et al., 2019). Metformin, one of the most commonly prescribed drugs for T2DM patients, is known to have a strong impact on gut bacteria, as described in several review articles (Zhang and Hu, 2020; Hu et al., 2021; Kant et al., 2022; Ezzamouri et al., 2023).
Metformin is believed to influence the microbiome in the right direction, having a beneficial effect on lowering blood glucose levels and improving intestinal epithelial permeability and gut barrier function (Wu et al., 2017). This effect could be supported by increased abundances of the beneficial genera Bifidobacterium, Faecalibacterium, Butyricicoccus, Butyrivibrio, Ruminococcus torques and Akkermansia muciniphila (Forslund et al., 2015; De La Cuesta-Zuluaga et al., 2017; Lau et al., 2021). The positive effect of butyrate-producing bacteria is known and (Wu et al., 2017) observed a negative correlation between the increase in Bifidobacterium adolescentis and diabetes control (% of HbA1c), while any significant correlation was found between % of HbA1c and the abundance of A. muciniphila. Some other metformin-induced shifts, such as increased abundance of Megasphaera (De La Cuesta-Zuluaga et al., 2017; Wu et al., 2017) and Turicibacter (Zhang et al., 2019) or decreased abundance of Intestinibacter bartlettii (Wu et al., 2017; Mueller et al., 2021), could also be considered beneficial according to current knowledge. On the other hand, some authors detected the decrease in beneficial Roseburia intestinalis, Roseburia faecis (Hiel et al., 2020; Mueller et al., 2021) and Oscillospira (De La Cuesta-Zuluaga et al., 2017) and an increase in harmful Salmonella, Klebsiella, Shigella (Karlsson et al., 2013; Wu et al., 2017), Escherichia (Karlsson et al., 2013; Forslund et al., 2015; De La Cuesta-Zuluaga et al., 2017; Wu et al., 2017; Hiel et al., 2020; Mueller et al., 2021) and Fusobacterium (Zhang et al., 2019). Assessing the impact of changes in some of the higher bacterial taxa is problematic, as individual species of families and genera can play very different roles in the gut microbiome. However, the metformin-associated microbiota seems to be more similar to healthy individuals than untreated diabetics (Crudele et al., 2023), and metformin is generally considered beneficial (Lv and Guo, 2020). Interestingly, the therapeutic effect of metformin is also influenced by the composition of the patient’s gut microbiota before starting the medication. The study of Elbere et al. (2020) showed an increased abundance of Prevotella copri at baseline in the group that did not respond to metformin treatment, while Enterococcus faecium, Lactococcus lactis, Odoribacter and Dialister were enriched in the responder group. In addition, Streptococcus parasanguinis was associated with the occurrence of severe side effects of metformin administration. Information on the state of the resident microbiota prior to treatment could therefore be useful to predict the tolerability of therapy. This is especially relevant in the context of personalized treatment, as differences in response to therapeutic interventions are often observed between individuals. The growing recognition that microbiome plays an important role in drug modification underscores the need to consider differential microbiota compositions and functions when developing treatment strategies. Future pharmaceutical developments should therefore consider how these variations may affect drug metabolism, absorption, efficacy, and toxicity. Additionally, understanding microbiota differences can help explain the different efficacies of generic drugs with similar active compounds (Zmora et al., 2016). The role of specific genera/species in the individual response to treatments should be taken into account and represents a new challenge in the study of the human microbiome.
Other oral antidiabetic agents are less researched and the results are inconsistent. Empagliflozin (SGLT2 inhibitor) significantly increased the richness and diversity of the gut microbiota, enriched the relative abundance of beneficial species of Roseburia, Eubacterium and Faecalibacterium, and decreased the harmful species of Escherichia/Shigella, Bilophila and Hungatella in the treatment-naïve T2DM patients (Deng et al., 2022). On the other hand, no changes in fecal bacteria composition were observed by van Bommel et al. (2020) when studying the effects of dapagliflozin. However, the T2DM participants in this study had been treated with metformin, which represents fundamentally different starting conditions. Liraglutide (GLP-1 agonist) significantly induced Collinsella, Akkermansia and Clostridium species and suppressed Fusobacteria (Shang et al., 2021), which is a positive result as some species of this phylum are associated with intestinal diseases such as inflammatory bowel disease (Lee et al., 2016) and colorectal cancer (Wang and Fang, 2023). In the study by Smits et al. (2021), liraglutide was administered to T2DM patients receiving stable doses of metformin and no effects on gut bacteria were observed. Similar results were obtained with sitagliptin (DPP-4 inhibitor) (Smits et al., 2021), indicating that the use of these drugs as add-on therapy to metformin is not associated with changes in the gut microbiota. This opinion is supported by the study of Wang Z. et al. (2021), who described a significant impact of both vildagliptin and saxagliptin on gut bacteria in T2DM patients not treated with metformin. After 3 months of treatment, vildagliptin decreased beneficial Turicibacter and increased Megamonas, which genus is thought to have a positive effect on human health (Yang X. et al., 2023). Saxagliptin reduced Pseudomanas and Blautia and increased beneficial Faecalibacterium and Roseburia, but also the opportunistic human pathogen Klebsiella. Acarbose (α-glucosidase inhibitor) inhibits not only human but also bacterial α-glucosidases, so its effects on the composition of the gut microbiome are to be expected. Increased abundance of Bifidobacteria, Lactobacilli (Su et al., 2015; Gu et al., 2017; Takewaki et al., 2021), Eubacterium (Takewaki et al., 2021) and Enterococcus faecalis (Su et al., 2015) and a reduction of Bacteroides (Gu et al., 2017; Takewaki et al., 2021) was associated with a decrease of inflammatory factors in plasma (Su et al., 2015). Overall, the results suggest a positive influence, however the effect of acarbose is highly dependent on diet and the original composition of the host gut microbiome (Takewaki et al., 2021). Only two clinical trials have investigated the impact of sulfonylureas on the gut microbiome in T2DM patients, but without evidence of positive effects (Gu et al., 2017; van Bommel et al., 2020). To date, no clinical study has been conducted to clarify the effect of treatment with thiazolidinediones on the human gut microbiota (Wang Z. et al., 2021). In summary, the antidiabetic drugs mainly enriched the beneficial Faecalibacterium, Roseburia, Akkermansia muciniphila, Eubacterium and Bifidobacterium, while the diminished taxa are the potentially pathogenic, such as Escherichia/Shigella, Klebsiella and Fusobacterium. However, this conclusion must be taken with caution, as studies vary in their conclusions, as noted above. Research in this area suggests that metformin and various other glucose-lowering agents, particularly SGLT2 inhibitors, DPP-4 inhibitors and α-glucosidase inhibitors, have a similar impact on the gut microbiota, primarily by promoting SCFA-producing bacteria and species with probiotic activity. Butyrate in particular is critical for insulin sensitivity and glycemic control. According to recent research by Martínez-López et al. (2024), metformin and linagliptin medications in combination with lifestyle changes also play an important role in people with prediabetes. The applied therapies improved insulin sensitivity, pancreatic β-cell function and increased the abundance of beneficial bacteria, such as Roseburia, Bifidobacterium and Eubacterium hallii, in prediabetics. Such an intervention could therefore reduce the incidence of T2DM. However, based on structural equation modeling, the authors concluded that the improvement in metabolic status in prediabetics was more related to pharmacological treatment, while bacterial changes had a minor impact. Further research is therefore needed to better understand the relationship between specific bacterial clusters and the regulation of insulin sensitivity, energy metabolism and systemic inflammation, and to uncover the complex interaction between the gut microbiota and the health status of the host.
7 Future challenges and research directions
The amount of information supporting the important role of gut microbiota in relation to diabetes is steadily increased. The mainstream of research in this area focuses on next-generation sequencing (NGS) using bacterial 16S rRNA fragments (Figure 2). This marker mostly allows identification at the genus level, while identification at the species level is somewhat limited. For unidentified microorganisms, the NGS analysis provides part of results at the bacterial family or even order level. This deficiency makes it impossible to properly evaluate the results and identify beneficial or, on the contrary, harmful bacteria. An example of this is the genus Ruminococcus, which includes beneficial species such as R. bromii and on the other hand Crohn’s disease associated R. gnavus (Henke et al., 2019). By using the full 16S rRNA gene (~1,500 bp), the weakness of the method based on amplification of part of the variable region can be overcome and this approach becomes realistic (Johnson et al., 2019; Jeong et al., 2021). A major step forward is also the PacBio third-generation long-read sequencing platform, whose widespread use is hampered by the high cost of metagenomics analysis and the need for large amounts of samples (micrograms of DNA, while NGS requires nanograms of DNA).
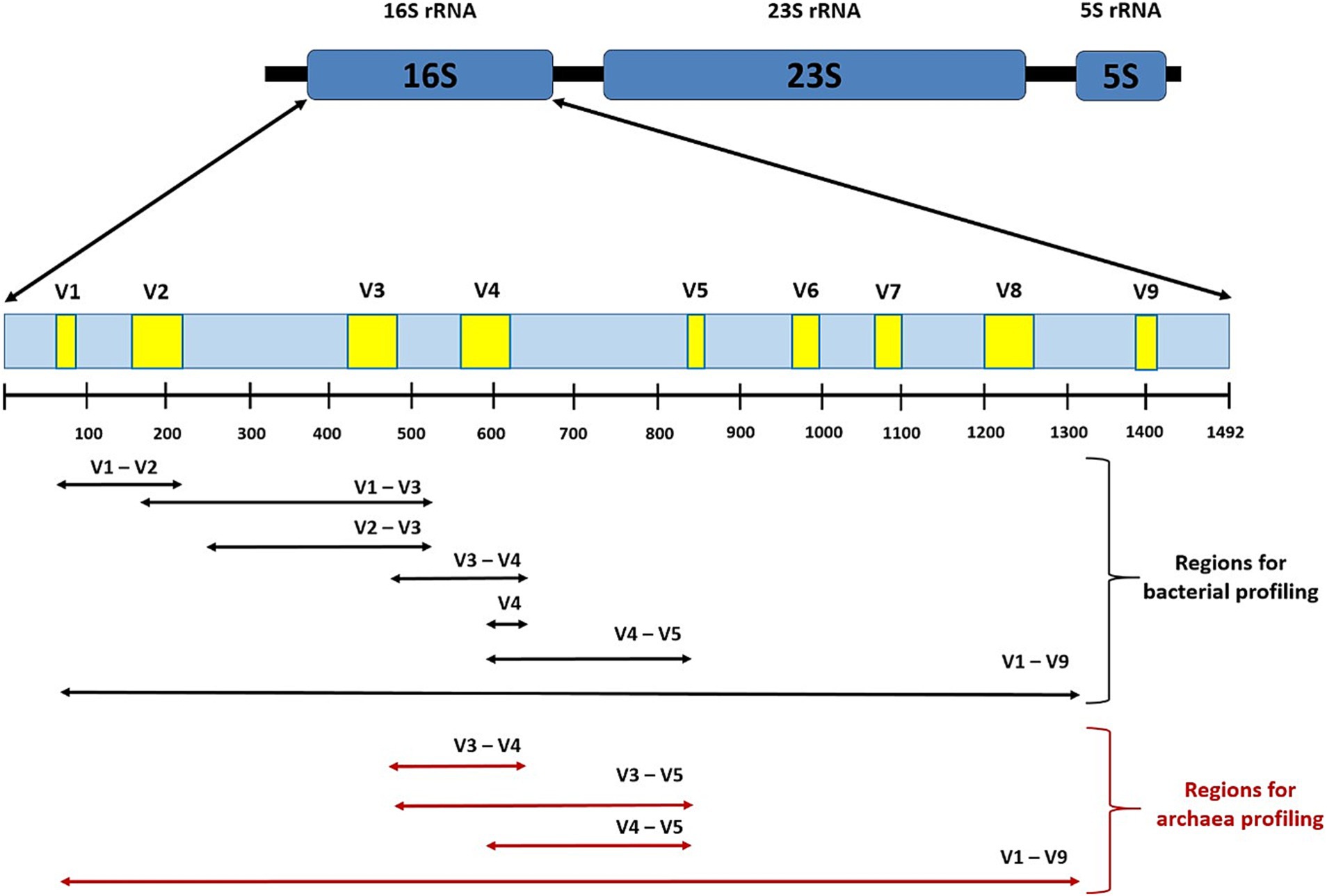
Figure 2. A schema of the prokaryotic rRNA operon with full-length 16S rRNA gene with variable regions V1-V9 showing the respective amplicons used for microbial profiling. For short-read-based NGS bacterial genotyping using Illumina or Ion Torrent device the primers targeting the following marked regions can be used: V1-V2, V1-V3 (Park et al., 2021; López-Aladid et al., 2023), V2-V3 (Bukin et al., 2019), V3-V4 (López-Aladid et al., 2023), hypervariable regions V4 alone (Bharti and Grimm, 2021), and V4-V5 (Fadeev et al., 2021). Regions V3-V4, V3-V5, and V4-V5 provide concurrent coverage of the archaeal domain (Pinto and Raskin, 2012; Takahashi et al., 2014; Fadeev et al., 2021), respectively. The V2 and V3 regions permit higher resolution at genera and species level (Bukin et al., 2019), while the V9 region provides the lowest resolution (Sirichoat et al., 2021). Long-read-based third generation sequencing targeting full V1-V9 regions using Sequel (PacBio technology) or MinION (Oxford Nanopor technology) device offers the best accuracy and discrimination between closely related species of bacteria (Matsuo et al., 2021; Park et al., 2021) and archaea (Meslier et al., 2022).
Another challenge lies in the study of other components of the microbiome. The bacterial 16S rRNA marker should be complemented by other markers covering methanogens (Figure 2), fungi, protozoa (Figure 3), and viruses to provide a more representative picture of microbial diversity and structure. Methanogens are a less numerous but important component of the human gastrointestinal tract. These microorganisms are involved in the final steps of fermentation processes in the human gut due to their syntrophic interactions with bacteria. Intestinal methanogens, mostly hydrogenotrophs, use H2 (and often formate) to reduce CO2 to methane. However, their diversity is still incomplete (Borrel et al., 2020), and their clinical relevance has not yet been clearly assessed. Methanogenic archaea have been largely overlooked in human studies, but a growing body of work points to their association with various diseases, including colorectal cancer (CRC), inflammatory bowel disease (IBD), irritable bowel syndrome (IBS), diverticulosis, chronic constipation, and obesity (Djemai et al., 2022; Hoegenauer et al., 2022). Microbiome studies in diabetic patients have mostly omitted methanogens, with only the study by Bhute et al. (2017b) describing an increase of Methanobrevibacter associated with a decrease in Methanosphaera genus in T2DM patients.
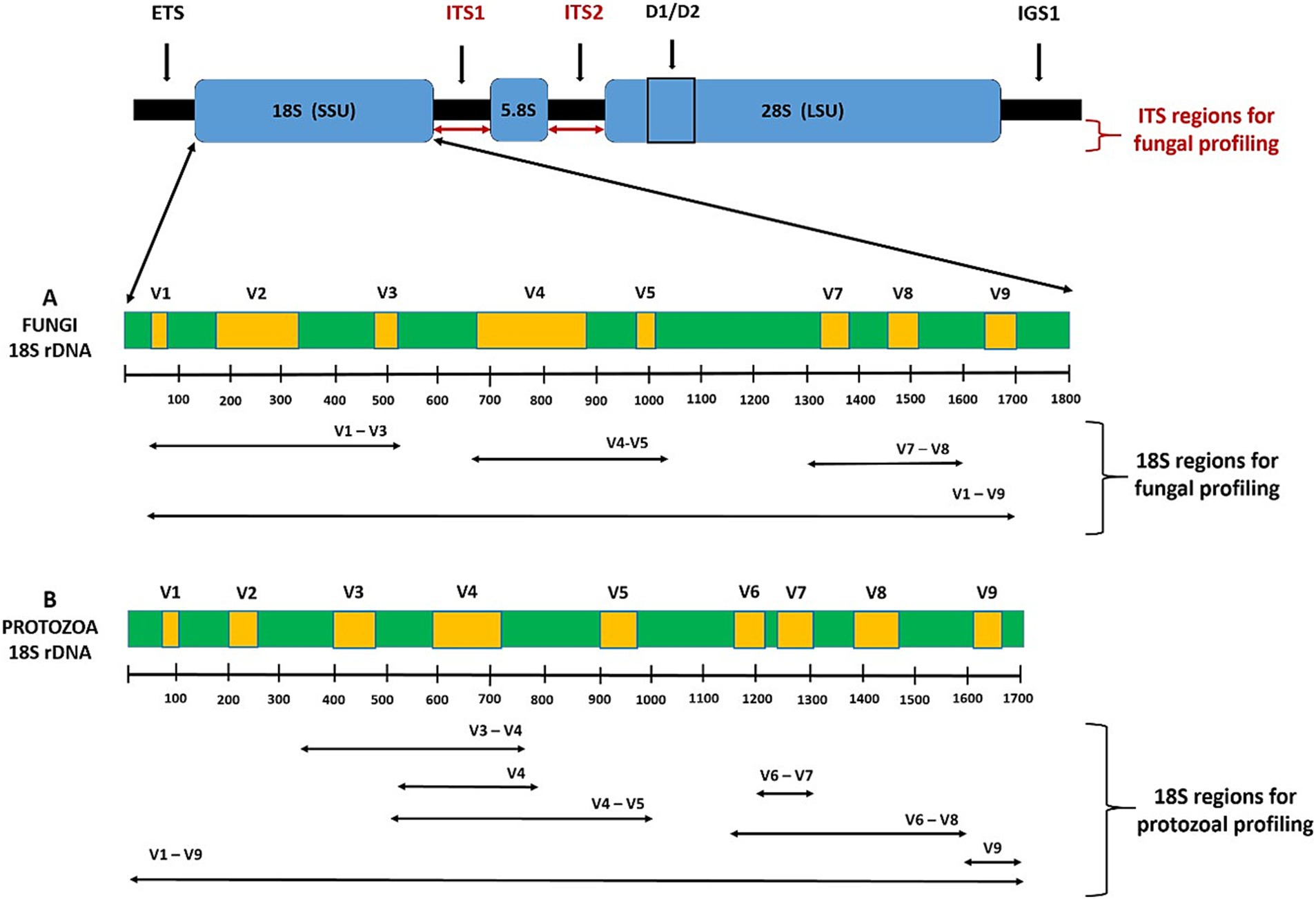
Figure 3. (A-B) A schema of the eukaryotic rDNA operon with full-length 18S rRNA gene with variable regions V1-V9 showing the respective amplicons used for microbial profiling. For NGS fungal genotyping using Illumina or Ion Torrent device the primers targeting the following marked 18S regions can be used: V1-V3 (Banos et al., 2018; Hoggard et al., 2018), V4-V5 (Wu et al., 2015; Banos et al., 2018), V7-V8 (Banos et al., 2018). Some regions are group specific, thus primers have to be selected according to fungi of interest (Banos et al., 2018). Long-read-based third generation sequencing targeting full V1-V9 regions using Sequel (PacBio technology) or MinION (Oxford Nanopor technology) device offers the best accuracy and discrimination between closely related species of fungi (D’Andreano et al., 2020). Fungal ITS1 and ITS2 (internal transcribed spacer) regions are more variable and suitable as the genetic marker to measure intraspecific genetic diversity (Schoch et al., 2012; Yang et al., 2018; Raimondi et al., 2019). 18S rRNA is mainly used for high resolution taxonomic studies of fungi, while the ITS region is mainly used for fungal diversity studies as a fungal barcode marker. The best results would be achieved with third generation sequencing of amplicons of the whole fungal ribosomal operon (i.e., ETS, 18S, ITS1, 5.8S, ITS2, 28S, and IGS), which is however expensive and demanding on bioinformatics (Wurzbacher et al., 2019). For NGS protozoal genotyping using Illumina or Ion Torrent device the primers targeting the following marked 18S regions can be used: V3-V4 (Ishaq and Wright, 2014), V4 (Maritz et al., 2019), V4-V5 (Maloney et al., 2019), V6-V7 (Bhute et al., 2017b), V6-V8 (Ishaq and Wright, 2014), and V9 (Maritz et al., 2019). Long-read-based third generation sequencing targeting the full V1-V9 protozoal regions using Sequel (PacBio technology) or MinION (Oxford Nanopor technology) device offers the best accuracy and species discrimination (Gad et al., 2023).
The role of fungi has also been neglected in diabetic patient studies, but recent research suggests a significant impact of intestinal mycobiota on host health (van Tilburg Bernardes et al., 2020). A limited body of work addressing this issue suggests higher diversity of species belonging to Candida genus in T1DM children, and interestingly, fungal species were more resistant to antifungal treatment in these patients (Kowalewska et al., 2016). The increased incidence of Candida spp. was also observed in adults with both types of diabetes (Gosiewski et al., 2014; Bhute et al., 2017b; Jayasudha et al., 2020), indicating a detrimental role of this genus in such diseases and gut bacterial microbiome assembly. According to Zhang et al. (2022), an increase in Candida spp. is consistently observed in urbanisation-associated diseases (obesity and inflammatory bowel disease), which we believe may include also diabetes.
Other gut microorganisms that have not yet been studied are protozoa. These unicellular eukaryotes were previously thought to be parasites that cause gastroenteritis problems. However, recent research indicates that Blastocystis species and Dientamoeba fragilis are more likely enteric commensals (Guzzo et al., 2022). They have been found to be the most abundant protozoa in the stool of healthy humans (de Boer et al., 2020) and have even been associated with increased bacterial diversity in the gut (Nash et al., 2017). The only mention of Blastocystis in diabetics was published by Bhute et al. (2017b), who found no differences in protozoan abundance between healthy controls and T2DM patients. However, the inclusion of eukaryotic organisms in microbial analysis would expand and improve the overview of the composition, diversity, and dysbiosis of the gut microbiome of diabetic patients.
The greatest challenge is probably the study of intestinal bacteriophages (phages), viruses that infect intestinal bacteria. Phages are simple organisms composed of nucleic acid (DNA or RNA, double- or single-stranded) packed in a protein capsid. They are parasitic by nature, requiring a bacterial host to reproduce. The phage inserts its genetic material into the bacterial cell, and this infection is followed by a lytic or lysogenic life cycle. Lytic phages use the bacterium to produce phage components, then destroy the cell and release new phage particles. Lysogenic phages incorporate their nucleic acid into the chromosome and replicate with the cell without destroying the host bacterium. In this way, phages can invade bacteria and thus transfer some additional genetic traits, such as antibiotic resistance genes. Thus, both types of cycles can affect the populations of intestinal bacteria and change their composition. In addition, phages interact with the host immune system, affecting the homeostasis of the GIT (Zuppi et al., 2022). The gut virome is an emerging topic in the study of the human microbiome, and several studies have indicated a link between the gut virome and various diseases (Bai et al., 2022), including T1DM (Zhao et al., 2017; Kim et al., 2019; Tetz et al., 2019; Vehik et al., 2019; Cinek et al., 2021) and T2DM (Ma et al., 2018; Chen et al., 2021). Although viruses are highly abundant in the gut and have significant impacts on microbial ecosystems, they are still the least studied members of the gut microbiome, and the genomic diversity of gut phages is still largely unknown (Zuppi et al., 2022).
All of these microorganisms can be identified simultaneously through shotgun sequencing. This approach, also called whole genome sequencing (WGS), enables the sequencing of the entire genome, i.e., not only rRNA, but identifies all genes (metagenome) and thus can provide additional information about the functional potential of the microbiome (Ranjan et al., 2016; Durazzi et al., 2021). WGS method is however more expensive and requires sequencing with high coverage and analysis with more extensive data.
Culture-independent research has brought great progress in understanding the composition and diversity of the gut microbiome in the context of disease. However, these methods have also revealed a large number of unknown, uncultured bacteria whose role in the digestive tract remains unknown. Therefore, great efforts should be directed toward the cultivation of intestinal microorganisms to determine the biochemical and metabolic properties of such bacterial species. Isolation of individual species is complicated by the difficulty of operating under strictly anaerobic conditions and the lack of knowledge about proper growth requirements and suitable substrates. A considerable amount of bacterial species thus still lack a cultured representative (Hitch et al., 2021). Several large projects in recent years focused on the cultivation of microbes from the human gut have contributed significantly to increasing the number of new bacterial strains available in public collections of microorganisms (Browne et al., 2016; Lagier et al., 2018; Forster et al., 2019; Zou et al., 2019). However, Clavel et al. (2022) pointed out that isolating and studying individual microorganisms is not sufficient because the functions of a strain depend heavily on its interaction with other members of the microbial community and its interaction with the host. One approach to study host–microbe interactions is based on defined synthetic bacterial communities representing the human gut microbiota that can be applied to gnotobiotic animals or to sophisticated in vitro cell models (Elzinga et al., 2019). Another approach is the so-called culturomics (Lagier et al., 2018). This is a high-throughput culture method that allows the identification of unknown bacteria inhabiting the human gut. The basis of the method lies in the combination of in vitro cultivation of stool samples with matrix assisted laser desorption ionization-time of flight mass spectrometry (MALDI-TOF MS) identification. This setup can use different selective culture conditions, detects only viable bacteria, and thus solves the problem of ‘non-cultivable’ or ‘non-cultured’ bacteria (Greub, 2012; Lagier et al., 2018).
The expansion of microbial markers and the application of culture methods in the study of the gut microbiome of diabetic patients will provide new insights and help clarify the extent to which the microbiota contributes to disease initiation and progression, and allow better application of therapeutic interventions such as probiotic administration, dietary fiber intervention, or fecal microbiota transplantation (FMT). FMT appears to be a promising approach to reconstruct the gut microbiota in both T1DM (De Groot et al., 2021; Xie et al., 2022) and T2DM patients (Aron-Wisnewsky et al., 2019; Wang et al., 2020; Su et al., 2022) resulting in improved insulin sensitivity, weight loss, reductions in fasting blood glucose and glycated hemoglobin, increased abundance of Bifidobacterium and reduction of pro-infammatory sulfate-reducing bacteria (Bilophila, Desulfovibrio). Detailed analysis of the intestinal microbiome is therefore urgently needed to avoid possible transmission of unwanted or even harmful microorganisms. Research in this field is very important because a reliable definition of the “healthy microbiome” is still lacking (Li D. et al., 2020; Najmanová et al., 2022).
Further challenges lie in gaining a deeper insight into endocrinology, immunology and metabolomics as well as in a more complex combination of these areas with the investigation of the microbiome of diabetics. The SCFAs produced by gut bacteria are involved in the indirect regulation of neuroactive compounds and hormones, and the gut microbiota is able to produce various substances of a hormonal nature. Investigating the novel microbial immunomodulatory metabolites and understanding their effect on host immunity and pancreatic functions thus represents a complicated study goal, but one that could yield significant progress. A better understanding of the interactions between drugs and the gut microbiome and exploring the effects of different therapeutic combinations on the intestinal bacteria is also a challenging topic. Continued research is needed to gain a more comprehensive understanding of the interplay between the gut ecosystem and diabetes and to provide a substantial basis for the prediction and treatment of diabetes in the future.
8 Conclusion
The gut microbiota of patients suffering from both types of diabetes mellitus is characterized by reduced bacterial diversity, a lower number of butyrate-producing bacteria, especially Roseburia and Faecalibacterium, and a low abundance of bacteria with probiotic activity, namely Bifidobacterium and Akkermansia. Most diabetes publications agree on an increased incidence of Bacteroidetes and a decrease in Firmicutes, but there is no consensus on specific or typical biomarkers. Studies should therefore be more personalized and a multidisciplinary and well-coordinated research approach would be useful to elucidate the functional and metabolic contribution of individual microbes and provide evidence-based data when considering the gut microorganisms as a putative target for the prevention of metabolic disorders. A growing body of literature confirms a link between the structural composition of the intestinal microbiota and diabetes. Despite intensive studies, it is still unclear whether the changes in the microbiome are causal or as a consequence of the disease process, and bacterial heterogeneity does not allow simple interpretations of the results. It is still questionable whether gut bacteria could play a direct role in the prevention and treatment of disease. However, scientists are striving to use the gut microorganisms not only as a biomarker for diabetes but also as a promising target for therapeutic interventions.
The significant increase in the number of diabetes cases is alarming and, in our opinion, the situation is so serious that this problem should be seen as a social problem and not just a health problem. A lifestyle characterized by stress, fast food and lack of exercise is a global problem and should not only be perceived as a health issue by the state administration. More effort should be put into prevention and education, proper dietary habits, incorporating adequate physical activity into everyday life and boosting immunity. Attention should be focused on new treatment options for diabetes, but also on non-pharmacological strategies, such as the use of gut biotics like prebiotics, probiotics and synbiotics. All these factors can modulate the gut microbiome, prevent gut dysbiosis and play a role in the treatment of diabetes.
Author contributions
KF: Writing – original draft. TM: Writing – review & editing. HS: Writing – review & editing. CM: Writing – review & editing. JM: Writing – review & editing. RJ: Writing – review & editing. MD: Writing – review & editing. VF: Writing – review & editing.
Funding
The author(s) declare that financial support was received for the research, authorship, and/or publication of this article. This study was supported by the Ministry of Health of the Czech Republic (NU20-01-00078) and National Institute for Research of Metabolic and Cardiovascular Diseases (Programme EXCELES, Project No. LX22NPO5104) funded by the European Union Next Generation EU. TM acknowledged support from the project FDS2223MONIELLO-CUP J83C22000160007 funded by Fondazione di Sardegna, Italy.
Conflict of interest
The authors declare that the research was conducted in the absence of any commercial or financial relationships that could be construed as a potential conflict of interest.
Publisher’s note
All claims expressed in this article are solely those of the authors and do not necessarily represent those of their affiliated organizations, or those of the publisher, the editors and the reviewers. Any product that may be evaluated in this article, or claim that may be made by its manufacturer, is not guaranteed or endorsed by the publisher.
Supplementary material
The Supplementary material for this article can be found online at: https://www.frontiersin.org/articles/10.3389/fmicb.2024.1451054/full#supplementary-material
References
Ahola, A. J., Harjutsalo, V., Forsblom, C., Freese, R., Mäkimattila, S., and Groop, P.-H. (2017). The self-reported use of probiotics is associated with better Glycaemic control and lower odds of metabolic syndrome and its components in type 1 diabetes. J. Probiotics Health 5:4. doi: 10.4172/2329-8901.1000188
Alkanani, A. K., Hara, N., Gottlieb, P. A., Ir, D., Robertson, C. E., Wagner, B. D., et al. (2015). Alterations in intestinal microbiota correlate with susceptibility to type 1 diabetes. Diabetes 64, 3510–3520. doi: 10.2337/DB14-1847
Allen-Vercoe, E., Daigneault, M., White, A., Panaccione, R., Duncan, S. H., Flint, H. J., et al. (2012). Anaerostipes hadrus comb. nov., a dominant species within the human colonic microbiota; reclassification of Eubacterium hadrum Moore et al. 1976. Anaerobe 18, 523–529. doi: 10.1016/J.ANAEROBE.2012.09.002
Almeida, A., Mitchell, A. L., Boland, M., Forster, S. C., Gloor, G. B., Tarkowska, A., et al. (2019). A new genomic blueprint of the human gut microbiota. Nature 568, 499–504. doi: 10.1038/s41586-019-0965-1
American Society for Microbiology (2011). The rare biosphere. The rare biosphere: This report is based on a colloquium convened by the American Academy of microbiology on April 27–29, 2009 in San Francisco, CA. doi: 10.1128/AAMCOL.27APR.2009
Arboleya, S., Watkins, C., Stanton, C., and Ross, R. P. (2016). Gut bifidobacteria populations in human health and aging. Front. Microbiol. 7:212275. doi: 10.3389/FMICB.2016.01204/BIBTEX
Arneth, B., Arneth, R., and Shams, M. (2019). Metabolomics of type 1 and type 2 diabetes. Int. J. Mol. Sci. 20:2467. doi: 10.3390/IJMS20102467
Aron-Wisnewsky, J., Clement, K., and Nieuwdorp, M. (2019). Fecal microbiota transplantation: a future therapeutic option for obesity / diabetes? Curr. Diab. Rep. 19, 1–9. doi: 10.1007/s11892-019-1180-z
Bai, J., Wan, Z., Zhang, Y., Wang, T., Xue, Y., and Peng, Q. (2022). Composition and diversity of gut microbiota in diabetic retinopathy. Front. Microbiol. 13:3267. doi: 10.3389/FMICB.2022.926926
Bajinka, O., Tan, Y., Abdelhalim, K. A., Özdemir, G., and Qiu, X. (2020). Extrinsic factors influencing gut microbes, the immediate consequences and restoring eubiosis. AMB Express 10, 1–11. doi: 10.1186/S13568-020-01066-8/TABLES/1
Banos, S., Lentendu, G., Kopf, A., Wubet, T., Glöckner, F. O., and Reich, M. (2018). A comprehensive fungi-specific 18S rRNA gene sequence primer toolkit suited for diverse research issues and sequencing platforms. BMC Microbiol. 18, 1–16. doi: 10.1186/s12866-018-1331-4
Beltrand, J., Busiah, K., Vaivre-Douret, L., Fauret, A. L., Berdugo, M., Cavé, H., et al. (2020). Neonatal Diabetes Mellitus. Front. Pediatr. 8:540718. doi: 10.3389/FPED.2020.540718
Bharti, R., and Grimm, D. G. (2021). Current challenges and best-practice protocols for microbiome analysis. Brief. Bioinform. 22, 178–193. doi: 10.1093/bib/bbz155
Bhute, S. S., Ghaskadbi, S. S., and Shouche, Y. S. (2017a). “Rare biosphere in human gut: a less explored component of human gut microbiota and its association with human health” in Mining of microbial wealth and metagenomics. Eds. Kalia, V., Shouche, Y., Purohit, H., Rahi, P. (Singapore: Springer), 133–142.
Bhute, S. S., Suryavanshi, M. V., Joshi, S. M., Yajnik, C. S., Shouche, Y. S., and Ghaskadbi, S. S. (2017b). Gut microbial diversity assessment of Indian type-2-diabetics reveals alterations in eubacteria, archaea, and eukaryotes. Front. Microbiol. 8, 1–15. doi: 10.3389/fmicb.2017.00214
Borrel, G., Brugère, J. F., Gribaldo, S., Schmitz, R. A., and Moissl-Eichinger, C. (2020). The host-associated archaeome. Nat. Rev. Microbiol. 18, 622–636. doi: 10.1038/s41579-020-0407-y
Brown, C. T., Davis-Richardson, A. G., Giongo, A., Gano, K. A., Crabb, D. B., Mukherjee, N., et al. (2011). Gut microbiome metagenomics analysis suggests a functional model for the development of autoimmunity for type 1 diabetes. PLoS One 6, 1–9. doi: 10.1371/journal.pone.0025792
Browne, H. P., Forster, S. C., Anonye, B. O., Kumar, N., Neville, B. A., Stares, M. D., et al. (2016). Culturing of “unculturable” human microbiota reveals novel taxa and extensive sporulation. Nature 533, 543–546. doi: 10.1038/nature17645
Buchanan, T. A., and Xiang, A. H. (2005). Gestational diabetes mellitus. J. Clin. Invest. 115, 485–491. doi: 10.1172/JCI24531
Bukin, Y. S., Galachyants, Y. P., Morozov, I. V., Bukin, S. V., Zakharenko, A. S., and Zemskaya, T. I. (2019). The effect of 16s rRNA region choice on bacterial community metabarcoding results. Sci. Data 6, 1–14. doi: 10.1038/sdata.2019.7
Candela, M., Biagi, E., Soverini, M., Consolandi, C., Quercia, S., Severgnini, M., et al. (2016). Modulation of gut microbiota dysbioses in type 2 diabetic patients by macrobiotic Ma-pi 2 diet. Br. J. Nutr. 116, 80–93. doi: 10.1017/S0007114516001045
Cani, P. D., Neyrinck, A. M., Fava, F., Knauf, C., Burcelin, R. G., Tuohy, K. M., et al. (2007). Selective increases of bifidobacteria in gut microflora improve high-fat-diet-induced diabetes in mice through a mechanism associated with endotoxaemia. Diabetologia 50, 2374–2383. doi: 10.1007/S00125-007-0791-0/FIGURES/7
Carlsson, S. (2019). Etiology and pathogenesis of latent autoimmune diabetes in adults (LADA) compared to type 2 diabetes. Front. Physiol. 10:320. doi: 10.3389/FPHYS.2019.00320
Cekanaviciute, E., Yoo, B. B., Runia, T. F., Debelius, J. W., Singh, S., Nelson, C. A., et al. (2017). Gut bacteria from multiple sclerosis patients modulate human T cells and exacerbate symptoms in mouse models. Proc. Natl. Acad. Sci. USA 114, 10713–10718. doi: 10.1073/PNAS.1711235114
Chang, S. C., Shen, M. H., Liu, C. Y., Pu, C. M., Hu, J. M., and Huang, C. J. (2020). A gut butyrate-producing bacterium Butyricicoccus pullicaecorum regulates short-chain fatty acid transporter and receptor to reduce the progression of 1,2-dimethylhydrazine-associated colorectal cancer. Oncol. Lett. 20:327. doi: 10.3892/OL.2020.12190
Charlton, M., and Nair, K. S. (1998). Protein metabolism in insulin-dependent diabetes mellitus. J. Nutr. 128, 323S–327S. doi: 10.1093/JN/128.2.323S
Chen, Q., Ma, X., Li, C., Shen, Y., Zhu, W., Zhang, Y., et al. (2021). Enteric Phageome alterations in patients with type 2 diabetes. Front. Cell. Infect. Microbiol. 10, 1–15. doi: 10.3389/fcimb.2020.575084
Chia, L. W., Mank, M., Blijenberg, B., Aalvink, S., Bongers, R. S., Stahl, B., et al. (2020). Bacteroides thetaiotaomicron fosters the growth of butyrate-producing Anaerostipes caccae in the presence of lactose and Total human Milk carbohydrates. Microorganisms 8, 1–13. doi: 10.3390/MICROORGANISMS8101513
Choi, S. I., Kim, N., Nam, R. H., Jang, J. Y., Kim, E. H., Ha, S., et al. (2023). The protective effect of Roseburia faecis against repeated water avoidance stress-induced irritable bowel syndrome in a Wister rat model. J. Cancer Prev. 28:93. doi: 10.15430/JCP.2023.28.3.93
Cinek, O., Kramna, L., Mazankova, K., Odeh, R., Alassaf, A., Ibekwe, M. A. U., et al. (2018). The bacteriome at the onset of type 1 diabetes: a study from four geographically distant African and Asian countries. Diabetes Res. Clin. Pract. 144, 51–62. doi: 10.1016/J.DIABRES.2018.08.010
Cinek, O., Kramna, L., Odeh, R., Alassaf, A., Ibekwe, M. A. U., Ahmadov, G., et al. (2021). Eukaryotic viruses in the fecal virome at the onset of type 1 diabetes: a study from four geographically distant African and Asian countries. Pediatr. Diabetes 22, 558–566. doi: 10.1111/pedi.13207
Clavel, T., Horz, H. P., Segata, N., and Vehreschild, M. (2022). Next steps after 15 stimulating years of human gut microbiome research. Microb. Biotechnol. 15, 164–175. doi: 10.1111/1751-7915.13970
Cockburn, D. W., Orlovsky, N. I., Foley, M. H., Kwiatkowski, K. J., Bahr, C. M., Maynard, M., et al. (2015). Molecular details of a starch utilization pathway in the human gut symbiont Eubacterium rectale. Mol. Microbiol. 95:209. doi: 10.1111/MMI.12859
Crudele, L., Gadaleta, R. M., Cariello, M., and Moschetta, A. (2023). Gut microbiota in the pathogenesis and therapeutic approaches of diabetes. EBioMedicine 97:104821. doi: 10.1016/j.ebiom.2023.104821
Cunningham, A. L., Stephens, J. W., and Harris, D. A. (2021). Gut microbiota influence in type 2 diabetes mellitus (T2DM). Gut Pathog. 13, 1–13. doi: 10.1186/s13099-021-00446-0
D’Andreano, S., Cuscó, A., and Francino, O. (2020). Rapid and real-time identification of fungi up to species level with long amplicon nanopore sequencing from clinical samples. Biol. Methods Protoc. 6, 1–6. doi: 10.1093/biomethods/bpaa026
de Boer, M. D., Schuurs, T. A., Vermeer, M., Ruijs, G. J. H. M., van der Zanden, A. G. M., Weel, J. F., et al. (2020). Distribution and relevance of Dientamoeba fragilis and Blastocystis species in gastroenteritis: results from a case-control study. Eur. J. Clin. Microbiol. Infect. Dis. 39, 197–203. doi: 10.1007/s10096-019-03710-z
De Goffau, M. C., Fuentes, S., Van Den Bogert, B., Honkanen, H., De Vos, W. M., Welling, G. W., et al. (2014). Aberrant gut microbiota composition at the onset of type 1 diabetes in young children. Diabetologia 57, 1569–1577. doi: 10.1007/s00125-014-3274-0
De Goffau, M. C., Luopajärvi, K., Knip, M., Ilonen, J., Ruohtula, T., Härkönen, T., et al. (2013). Fecal microbiota composition differs between children with β-cell autoimmunity and those without. Diabetes 62, 1238–1244. doi: 10.2337/db12-0526
De Groot, P., Nikolic, T., Pellegrini, S., Sordi, V., Imangaliyev, S., Rampanelli, E., et al. (2021). Faecal microbiota transplantation halts progression of human new-onset type 1 diabetes in a randomised controlled trial. Gut 70, 92–105. doi: 10.1136/gutjnl-2020-322630
De La Cuesta-Zuluaga, J., Mueller, N. T., Corrales-Agudelo, V., Velásquez-Mejía, E. P., Carmona, J. A., Abad, J. M., et al. (2017). Metformin is associated with higher relative abundance of mucin-degrading Akkermansia muciniphila and several short-chain fatty acid–producing microbiota in the gut. Diabetes Care 40, 54–62. doi: 10.2337/DC16-1324
De Pessemier, B., Grine, L., Debaere, M., Maes, A., Paetzold, B., and Callewaert, C. (2021). Gut–skin axis: current knowledge of the interrelationship between microbial dysbiosis and skin conditions. Microorganisms 9, 1–33. doi: 10.3390/microorganisms9020353
Dedrick, S., Sundaresh, B., Huang, Q., Brady, C., Yoo, T., Cronin, C., et al. (2020). The role of gut microbiota and environmental factors in type 1 diabetes pathogenesis. Front. Endocrinol. (Lausanne) 11:78. doi: 10.3389/FENDO.2020.00078/FULL
DeFronzo, R. A., Ferrannini, E., Groop, L., Henry, R. R., Herman, W. H., Holst, J. J., et al. (2015). Type 2 diabetes mellitus. Nat. Rev. Dis. Primers 1:15019. doi: 10.1038/NRDP.2015.19
Dempsey, E., and Corr, S. C. (2022). Lactobacillus spp. for gastrointestinal health: current and future perspectives. Front. Immunol. 13:840245. doi: 10.3389/FIMMU.2022.840245
Deng, X., Zhang, C., Wang, P., Wei, W., Shi, X., Wang, P., et al. (2022). Cardiovascular benefits of Empagliflozin are associated with gut microbiota and plasma metabolites in type 2 diabetes. J. Clin. Endocrinol. Metab. 107, 1888–1896. doi: 10.1210/clinem/dgac210
Derrien, M., Turroni, F., Ventura, M., and van Sinderen, D. (2022). Insights into endogenous Bifidobacterium species in the human gut microbiota during adulthood. Trends Microbiol. 30, 940–947. doi: 10.1016/J.TIM.2022.04.004
Di Vincenzo, F., Del Gaudio, A., Petito, V., Lopetuso, L. R., and Scaldaferri, F. (2024). Gut microbiota, intestinal permeability, and systemic inflammation: a narrative review. Intern. Emerg. Med. 19, 275–293. doi: 10.1007/S11739-023-03374-W/FIGURES/2
Djemai, K., Drancourt, M., and Tidjani Alou, M. (2022). Bacteria and methanogens in the human microbiome: a review of syntrophic interactions. Microb. Ecol. 83, 536–554. doi: 10.1007/s00248-021-01796-7
Dong, J., Ping, L., Cao, T., Sun, L., Liu, D., Wang, S., et al. (2022). Immunomodulatory effects of the Bifidobacterium longum BL-10 on lipopolysaccharide-induced intestinal mucosal immune injury. Front. Immunol. 13, 1–14. doi: 10.3389/fimmu.2022.947755
Doumatey, A. P., Adeyemo, A., Zhou, J., Lei, L., Adebamowo, S. N., Adebamowo, C., et al. (2020). Gut microbiome profiles are associated with type 2 diabetes in urban Africans. Front. Cell. Infect. Microbiol. 10, 1–13. doi: 10.3389/fcimb.2020.00063
Duncan, S. H., Louis, P., and Flint, H. J. (2004). Lactate-utilizing Bacteria, isolated from human feces, that produce butyrate as a major fermentation product. Appl. Environ. Microbiol. 70:5810. doi: 10.1128/AEM.70.10.5810-5817.2004
Durazzi, F., Sala, C., Castellani, G., Manfreda, G., Remondini, D., De Cesare, A., et al. (2021). Comparison between 16S rRNA and shotgun sequencing data for the taxonomic characterization of the gut microbiota. Sci. Rep. 11, 1–10. doi: 10.1038/s41598-021-82726-y
Eeckhaut, V., Machiels, K., Perrier, C., Romero, C., Maes, S., Flahou, B., et al. (2013). Butyricicoccus pullicaecorum in inflammatory bowel disease. Gut 62, 1745–1752. doi: 10.1136/gutjnl-2012-303611
Eeckhaut, V., Van Immerseel, F., Pasmans, F., De Brandt, E., Haesebrouck, F., Ducatelle, R., et al. (2010). Anaerostipes butyraticus sp. nov., an anaerobic, butyrate-producing bacterium from Clostridium cluster XIVa isolated from broiler chicken caecal content, and emended description of the genus Anaerostipes. Int. J. Syst. Evol. Microbiol. 60, 1108–1112. doi: 10.1099/IJS.0.015289-0
Effendi, R. M. R. A., Anshory, M., Kalim, H., Dwiyana, R. F., Suwarsa, O., Pardo, L. M., et al. (2022). Akkermansia muciniphila and Faecalibacterium prausnitzii in immune-related diseases. Microorganisms 10:2382. doi: 10.3390/MICROORGANISMS10122382
Eiselein, L., Schwartz, H. J., and Rutledge, J. C. (2004). The challenge of type 1 diabetes mellitus. ILAR J. 45, 231–236. doi: 10.1093/ILAR.45.3.231
Ejtahed, H. S., Hoseini-Tavassol, Z., Khatami, S., Zangeneh, M., Behrouzi, A., Ahmadi Badi, S., et al. (2020). Main gut bacterial composition differs between patients with type 1 and type 2 diabetes and non-diabetic adults. J. Diabetes Metab. Disord. 19, 265–271. doi: 10.1007/s40200-020-00502-7
Elbere, I., Silamikelis, I., Dindune, I. I., Kalnina, I., Ustinova, M., Zaharenko, L., et al. (2020). Baseline gut microbiome composition predicts metformin therapy short-term efficacy in newly diagnosed type 2 diabetes patients. PLoS One 15, 1–19. doi: 10.1371/journal.pone.0241338
Elzinga, J., van der Oost, J., de Vos, W. M., and Smidt, H. (2019). The use of defined microbial communities to model host-microbe interactions in the human gut. Microbiol. Mol. Biol. Rev. 83, 1–40. doi: 10.1128/mmbr.00054-18
Enaud, R., Prevel, R., Ciarlo, E., Beaufils, F., Wieërs, G., Guery, B., et al. (2020). The gut-lung Axis in health and respiratory diseases: a place for inter-organ and inter-kingdom Crosstalks. Front. Cell. Infect. Microbiol. 10:9. doi: 10.3389/FCIMB.2020.00009
Everard, A., Belzer, C., Geurts, L., Ouwerkerk, J. P., Druart, C., Bindels, L. B., et al. (2013). Cross-talk between Akkermansia muciniphila and intestinal epithelium controls diet-induced obesity. Proc. Natl. Acad. Sci. USA 110, 9066–9071. doi: 10.1073/pnas.1219451110
Ezzamouri, B., Rosario, D., Bidkhori, G., Lee, S., Uhlen, M., and Shoaie, S. (2023). Metabolic modelling of the human gut microbiome in type 2 diabetes patients in response to metformin treatment. NPJ Syst. Biol. Appl. 9, 1–8. doi: 10.1038/s41540-022-00261-6
Fadeev, E., Cardozo-Mino, M. G., Rapp, J. Z., Bienhold, C., Salter, I., Salman-Carvalho, V., et al. (2021). Comparison of two 16S rRNA primers (V3–V4 and V4–V5) for studies of Arctic microbial communities. Front. Microbiol. 12:637526. doi: 10.3389/FMICB.2021.637526/BIBTEX
Fan, Y., and Pedersen, O. (2021). Gut microbiota in human metabolic health and disease. Nat. Rev. Microbiol. 19, 55–71. doi: 10.1038/s41579-020-0433-9
Fassatoui, M., Lopez-Siles, M., Díaz-Rizzolo, D. A., Jmel, H., Naouali, C., Abdessalem, G., et al. (2019). Gut microbiota imbalances in Tunisian participants with type 1 and type 2 diabetes mellitus. Biosci. Rep. 39, 1–7. doi: 10.1042/BSR20182348
Feehley, T., Plunkett, C. H., Bao, R., Choi Hong, S. M., Culleen, E., Belda-Ferre, P., et al. (2019). Healthy infants harbor intestinal bacteria that protect against food allergy. Nat. Med. 25, 448–453. doi: 10.1038/s41591-018-0324-z
Ferreira-Halder, C. V., Faria, A. V. D. S., and Andrade, S. S. (2017). Action and function of Faecalibacterium prausnitzii in health and disease. Best Pract. Res. Clin. Gastroenterol. 31, 643–648. doi: 10.1016/J.BPG.2017.09.011
Forslund, K., Hildebrand, F., Nielsen, T., Falony, G., Le Chatelier, E., Sunagawa, S., et al. (2015). Disentangling type 2 diabetes and metformin treatment signatures in the human gut microbiota. Nature 528, 262–266. doi: 10.1038/NATURE15766
Forster, S. C., Kumar, N., Anonye, B. O., Almeida, A., Viciani, E., Stares, M. D., et al. (2019). A human gut bacterial genome and culture collection for improved metagenomic analyses. Nat. Biotechnol. 37, 186–192. doi: 10.1038/s41587-018-0009-7
Furet, J. P., Kong, L. C., Tap, J., Poitou, C., Basdevant, A., Bouillot, J. L., et al. (2010). Differential adaptation of human gut microbiota to bariatric surgery-induced weight loss: links with metabolic and low-grade inflammation markers. Diabetes 59, 3049–3057. doi: 10.2337/db10-0253
Gad, M., Fawzy, M. E., Al-Herrawy, A. Z., Abdo, S. M., Nabet, N., and Hu, A. (2023). PacBio next-generation sequencing uncovers Apicomplexa diversity in different habitats. Sci. Rep. 13, 1–10. doi: 10.1038/s41598-023-40895-y
Giongo, A., Gano, K. A., Crabb, D. B., Mukherjee, N., Novelo, L. L., Casella, G., et al. (2011). Toward defining the autoimmune microbiome for type 1 diabetes. ISME J. 5, 82–91. doi: 10.1038/ismej.2010.92
Goldberg, E., Amir, I., Zafran, M., Gophna, U., Samra, Z., Pitlik, S., et al. (2014). The correlation between Clostridium-difficile infection and human gut concentrations of Bacteroidetes phylum and clostridial species. Eur. J. Clin. Microbiol. Infect. Dis. 33, 377–383. doi: 10.1007/S10096-013-1966-X/METRICS
Gosiewski, T., Salamon, D., Szopa, M., Sroka, A., Malecki, M. T., and Bulanda, M. (2014). Quantitative evaluation of fungi of the genus Candida in the feces of adult patients with type 1 and 2 diabetes – a pilot study. Gut Pathog. 6, 1–5. doi: 10.1186/s13099-014-0043-z
Gowd, V., Xie, L., Zheng, X., and Chen, W. (2019). Dietary fibers as emerging nutritional factors against diabetes: focus on the involvement of gut microbiota. Crit. Rev. Biotechnol. 39, 524–540. doi: 10.1080/07388551.2019.1576025
Greenhill, C. (2018). Sphingolipids involved in T1DM. Nat. Rev. Endocrinol. 14, –381. doi: 10.1038/s41574-018-0027-z
Greer, R. L., Dong, X., Moraes, A. C. F., Zielke, R. A., Fernandes, G. R., Peremyslova, E., et al. (2016). Akkermansia muciniphila mediates negative effects of IFNγ on glucose metabolism. Nat. Commun. 7, 1–13. doi: 10.1038/ncomms13329
Greub, G. (2012). Culturomics: a new approach to study the human microbiome. Clin. Microbiol. Infect. 18, 1157–1159. doi: 10.1111/1469-0691.12032
Groele, L., Szajewska, H., Szalecki, M., Świderska, J., Wysocka-Mincewicz, M., Ochocińska, A., et al. (2021). Lack of effect of Lactobacillus rhamnosus GG and Bifidobacterium lactis Bb12 on beta-cell function in children with newly diagnosed type 1 diabetes: a randomised controlled trial. BMJ Open Diabetes Res. Care 9, 1–10. doi: 10.1136/bmjdrc-2020-001523
Groele, L., Szajewska, H., and Szypowska, A. (2017). Effects of Lactobacillus rhamnosus GG and Bifidobacterium lactis Bb12 on beta-cell function in children with newly diagnosed type 1 diabetes: protocol of a randomised controlled trial. BMJ Open 7:e017178. doi: 10.1136/BMJOPEN-2017-017178
Gu, Y., Wang, X., Li, J., Zhang, Y., Zhong, H., Liu, R., et al. (2017). Analyses of gut microbiota and plasma bile acids enable stratification of patients for antidiabetic treatment. Nat. Commun. 8:1785. doi: 10.1038/s41467-017-01682-2
Gurung, M., Li, Z., You, H., Rodrigues, R., Jump, D. B., Morgun, A., et al. (2020). Role of gut microbiota in type 2 diabetes pathophysiology. EBioMedicine 51:102590. doi: 10.1016/j.ebiom.2019.11.051
Guzzo, G. L., Andrews, J. M., and Weyrich, L. S. (2022). The neglected gut microbiome: Fungi, Protozoa, and bacteriophages in inflammatory bowel disease. Inflamm. Bowel Dis. 28, 1112–1122. doi: 10.1093/ibd/izab343
He, Q. L., Wang, H. C., Ma, Y. K., Yang, R. L., Dai, Z. F., Yang, J. N., et al. (2023). Changes in the microbiota and their roles in patients with type 2 diabetes mellitus. Curr. Microbiol. 80, 1–12. doi: 10.1007/s00284-023-03219-x
He, X., Zhao, S., and Li, Y. (2021). Faecalibacterium prausnitzii: a next-generation probiotic in gut disease improvement. Can. J. Infect. Dis. Med. Microbiol. 2021:6666114. doi: 10.1155/2021/6666114
Hebert, S. L., and Nair, K. S. (2009). Protein and energy metabolism in type 1 diabetes. Clin. Nutr. 29:13. doi: 10.1016/J.CLNU.2009.09.001
Henke, M. T., Kenny, D. J., Cassilly, C. D., Vlamakis, H., Xavier, R. J., and Clardy, J. (2019). Ruminococcus gnavus, a member of the human gut microbiome associated with Crohn’s disease, produces an inflammatory polysaccharide. Proc. Natl. Acad. Sci. USA 116, 12672–12677. doi: 10.1073/pnas.1904099116
Hiel, S., Gianfrancesco, M. A., Rodriguez, J., Portheault, D., Leyrolle, Q., Bindels, L. B., et al. (2020). Link between gut microbiota and health outcomes in inulin -treated obese patients: lessons from the Food4Gut multicenter randomized placebo-controlled trial. Clin. Nutr. 39, 3618–3628. doi: 10.1016/j.clnu.2020.04.005
Hitch, T. C. A., Afrizal, A., Riedel, T., Kioukis, A., Haller, D., Lagkouvardos, I., et al. (2021). Recent advances in culture-based gut microbiome research. Int. J. Med. Microbiol. 311:151485. doi: 10.1016/j.ijmm.2021.151485
Hoegenauer, C., Hammer, H. F., Mahnert, A., and Moissl-Eichinger, C. (2022). Methanogenic archaea in the human gastrointestinal tract. Nat. Rev. Gastroenterol. Hepatol. 19, 805–813. doi: 10.1038/S41575-022-00673-Z
Hoggard, M., Vesty, A., Wong, G., Montgomery, J. M., Fourie, C., Douglas, R. G., et al. (2018). Characterizing the human mycobiota: a comparison of small subunit rRNA, ITS1, ITS2, and large subunit rRNA genomic targets. Front. Microbiol. 9, 1–14. doi: 10.3389/fmicb.2018.02208
Horikawa, Y. (2018). Maturity-onset diabetes of the young as a model for elucidating the multifactorial origin of type 2 diabetes mellitus. J. Diabetes Investig. 9, 704–712. doi: 10.1111/JDI.12812
Hu, R., Yuan, Y., Liu, C., Zhou, J., Ji, L., and Jiang, G. (2021). New insights into the links between anti-diabetes drugs and gut microbiota. Endocr. Connect. 10, R36–R42. doi: 10.1530/EC-20-0431
Huang, Y., Li, S. C., Hu, J., Ruan, H. B., Guo, H. M., Zhang, H. H., et al. (2018). Gut microbiota profiling in Han Chinese with type 1 diabetes. Diabetes Res. Clin. Pract. 141, 256–263. doi: 10.1016/j.diabres.2018.04.032
Huang, R., Wu, F., Zhou, Q., Wei, W., Yue, J., Xiao, B., et al. (2022). Lactobacillus and intestinal diseases: mechanisms of action and clinical applications. Microbiol. Res. 260:127019. doi: 10.1016/J.MICRES.2022.127019
Iebba, V., Totino, V., Gagliardi, A., Santangelo, F., Cacciotti, F., Trancassini, M., et al. (2016). Eubiosis and dysbiosis: the two sides of the microbiota SuMMAry.
International Diabetes Federation (2021). IDF Diabetes Atlas 2021. International Diabetes Federation, 30–39. Available at: http://www.idf.org/about-diabetes/facts-figures (accessed July 27, 2023).
Ishaq, S. L., and Wright, A. D. G. (2014). Design and validation of four new primers for next-generation sequencing to target the 18S rRNA genes of gastrointestinal ciliate protozoa. Appl. Environ. Microbiol. 80, 5515–5521. doi: 10.1128/AEM.01644-14
Jayasudha, R., Das, T., Chakravarthy, S. K., Prashanthi, G. S., Bhargava, A., Tyagi, M., et al. (2020). Gut mycobiomes are altered in people with type 2 diabetes mellitus and diabetic retinopathy. PLoS One 15, 1–23. doi: 10.1371/journal.pone.0243077
Jeong, J., Yun, K., Mun, S., Chung, W. H., Choi, S. Y., Nam, Y., et al. (2021). The effect of taxonomic classification by full-length 16S rRNA sequencing with a synthetic long-read technology. Sci. Rep. 11, 1–12. doi: 10.1038/s41598-020-80826-9
Johnson, J. S., Spakowicz, D. J., Hong, B. Y., Petersen, L. M., Demkowicz, P., Chen, L., et al. (2019). Evaluation of 16S rRNA gene sequencing for species and strain-level microbiome analysis. Nat. Commun. 10, 1–11. doi: 10.1038/s41467-019-13036-1
Kant, R., Chandra, L., Verma, V., Nain, P., Bello, D., Patel, S., et al. (2022). Gut microbiota interactions with anti-diabetic medications and pathogenesis of type 2 diabetes mellitus. World J. Methodol. 12, 246–257. doi: 10.5662/wjm.v12.i4.246
Kant, R., Rasinkangas, P., Satokari, R., Pietilä, T. E., and Palva, A. (2015). Genome sequence of the butyrate-producing anaerobic bacterium Anaerostipes hadrus PEL 85. Genome Announc. 3, e00224–15. doi: 10.1128/GENOMEA.00224-15
Karlsson, F. H., Tremaroli, V., Nookaew, I., Bergström, G., Behre, C. J., Fagerberg, B., et al. (2013). Gut metagenome in European women with normal, impaired and diabetic glucose control. Nature 498, 99–103. doi: 10.1038/nature12198
Katsarou, A., Gudbjörnsdottir, S., Rawshani, A., Dabelea, D., Bonifacio, E., Anderson, B. J., et al. (2017). Type 1 diabetes mellitus. Nat. Rev. Dis. Prim. 3, 1–17. doi: 10.1038/nrdp.2017.16
Kautzky-Willer, A., Leutner, M., and Harreiter, J. (2023). Sex differences in type 2 diabetes. Diabetologia 986–1002. doi: 10.1007/s00125-023-05891-x
Khan, M. A. B., Hashim, M. J., King, J. K., Govender, R. D., Mustafa, H., and Al Kaabi, J. (2020). Epidemiology of type 2 diabetes – global burden of disease and forecasted trends. J. Epidemiol. Glob. Health 10, 107–111. doi: 10.2991/JEGH.K.191028.001
Kim, K. W., Allen, D. W., Briese, T., Couper, J. J., Barry, S. C., Colman, P. G., et al. (2019). Distinct gut Virome profile of pregnant women with type 1 diabetes in the ENDIA study. Open Forum Infect. Dis. 6:ofz025. doi: 10.1093/OFID/OFZ025
Kim, J., and Nam, J. H. (2020). Insight into the relationship between obesity-induced low-level chronic inflammation and COVID-19 infection. Int. J. Obes. 44, 1541–1542. doi: 10.1038/s41366-020-0602-y
King, C. H., Desai, H., Sylvetsky, A. C., LoTempio, J., Ayanyan, S., Carrie, J., et al. (2019). Baseline human gut microbiota profile in healthy people and standard reporting template. PLoS One 14, 1–25. doi: 10.1371/journal.pone.0206484
Knip, M., and Siljander, H. (2016). The role of the intestinal microbiota in type 1 diabetes mellitus. Nat. Rev. Endocrinol. 12, 154–167. doi: 10.1038/nrendo.2015.218
Koboziev, I., Reinoso Webb, C., Furr, K. L., and Grisham, M. B. (2014). Role of the enteric microbiota in intestinal homeostasis and inflammation. Free Radic. Biol. Med. 68, 122–133. doi: 10.1016/J.FREERADBIOMED.2013.11.008
Kowalewska, B., Zorena, K., Szmigiero-Kawko, M., Wąż, P., and Myśliwiec, M. (2016). Higher diversity in fungal species discriminates children with type 1 diabetes mellitus from healthy control. Patient Prefer. Adherence 10, 591–599. doi: 10.2147/PPA.S97852
Kumar, S., Kumar, R., Rohilla, L., Jacob, N., Yadav, J., and Sachdeva, N. (2021). A high potency multi-strain probiotic improves glycemic control in children with new-onset type 1 diabetes mellitus: a randomized, double-blind, and placebo-controlled pilot study. Pediatr. Diabetes 22, 1014–1022. doi: 10.1111/pedi.13244
Kwan, S.-Y., Sabotta, C. M., Joon, A., Wei, P., Petty, L. E., Below, J. E., et al. (2022). Gut microbiome alterations associated with diabetes in Mexican Americans in South Texas. mSystems 7:e0003322. doi: 10.1128/msystems.00033-22
Lagier, J. C., Dubourg, G., Million, M., Cadoret, F., Bilen, M., Fenollar, F., et al. (2018). Culturing the human microbiota and culturomics. Nat. Rev. Microbiol. 16, 540–550. doi: 10.1038/s41579-018-0041-0
Lal, S., Kandiyal, B., Ahuja, V., Takeda, K., and Das, B. (2022). Gut microbiome dysbiosis in inflammatory bowel disease. Prog. Mol. Biol. Transl. Sci. 192, 179–204. doi: 10.1016/BS.PMBTS.2022.09.003
Lambeth, S. M., Carson, T., Lowe, J., Ramaraj, T., Leff, J. W., Luo, L., et al. (2015). Composition, diversity and abundance of gut microbiome in prediabetes and type 2 diabetes. J. Diabetes Obes. 2, 108–114. doi: 10.15436/2376-0949.15.031
Lampousi, A. M., Carlsson, S., and Löfvenborg, J. E. (2021). Dietary factors and risk of islet autoimmunity and type 1 diabetes: a systematic review and meta-analysis. EBioMedicine 72, 1–9. doi: 10.1016/j.ebiom.2021.103633
Larsen, N., Vogensen, F. K., Van Den Berg, F. W. J., Nielsen, D. S., Andreasen, A. S., Pedersen, B. K., et al. (2010). Gut microbiota in human adults with type 2 diabetes differs from non-diabetic adults. PLoS One 5:e9085. doi: 10.1371/journal.pone.0009085
Lau, W. L., Tran, T., Rhee, C. M., Kalantar-Zadeh, K., and Vaziri, N. D. (2021). Diabetes and the gut microbiome. Semin. Nephrol. 41, 104–113. doi: 10.1016/j.semnephrol.2021.03.005
Lee, Y., Eun, C. S., Lee, A. R., Park, C. H., and Han, D. S. (2016). Fusobacterium isolates recovered from colonic biopsies of inflammatory bowel disease patients in Korea. Ann. Lab. Med. 36, 387–389. doi: 10.3343/alm.2016.36.4.387
Leiva-Gea, I., Sánchez-Alcoholado, L., Martín-Tejedor, B., Castellano-Castillo, D., Moreno-Indias, I., Urda-Cardona, A., et al. (2018). Gut microbiota differs in composition and functionality between children with type 1 diabetes and MODY2 and healthy control subjects: a case-control study. Diabetes Care 41, 2385–2395. doi: 10.2337/dc18-0253
Li, X., and Atkinson, M. A. (2015). The role for gut permeability in the pathogenesis of type 1 diabetes – a solid or leaky concept? Pediatr. Diabetes 16:485. doi: 10.1111/PEDI.12305
Li, D., Gao, C., Zhang, F., Yang, R., Lan, C., Ma, Y., et al. (2020). Seven facts and five initiatives for gut microbiome research. Protein Cell 11, 391–400. doi: 10.1007/s13238-020-00697-8
Li, Z., Hu, G., Zhu, L., Sun, Z., Jiang, Y., Gao, M., et al. (2021). Study of growth, metabolism, and morphology of Akkermansia muciniphila with an in vitro advanced bionic intestinal reactor. BMC Microbiol. 21:61. doi: 10.1186/s12866-021-02111-7
Li, Z., Ke, H., Wang, Y., Chen, S., Liu, X., Lin, Q., et al. (2022). Global trends in Akkermansia muciniphila research: a bibliometric visualization. Front. Microbiol. 13:1037708. doi: 10.3389/FMICB.2022.1037708/BIBTEX
Li, Y., Liu, Y., and Chu, C. Q. (2015). Th17 cells in type 1 diabetes: role in the pathogenesis and regulation by gut microbiome. Mediat. Inflamm. 2015:638470. doi: 10.1155/2015/638470
Li, W.-Z., Stirling, K., Yang, J.-J., Zhang, L., Yang, J.-J., Stirling, K., et al. (2020). Gut microbiota and diabetes: from correlation to causality and mechanism. World J. Diabetes 11, 293–308. doi: 10.4239/WJD.V11.I7.293
López-Aladid, R., Fernández-Barat, L., Alcaraz-Serrano, V., Bueno-Freire, L., Vázquez, N., Pastor-Ibáñez, R., et al. (2023). Determining the most accurate 16S rRNA hypervariable region for taxonomic identification from respiratory samples. Sci. Rep. 13, 1–10. doi: 10.1038/s41598-023-30764-z
Lotfy, M., Adeghate, J., Kalasz, H., Singh, J., and Adeghate, E. (2017). Chronic complications of diabetes mellitus: a Mini review. Curr. Diabetes Rev. 13, 3–10. doi: 10.2174/1573399812666151016101622
Louis, P., and Flint, H. J. (2017). Formation of propionate and butyrate by the human colonic microbiota. Environ. Microbiol. 19, 29–41. doi: 10.1111/1462-2920.13589
Louis, P., Young, P., Holtrop, G., and Flint, H. J. (2010). Diversity of human colonic butyrate-producing bacteria revealed by analysis of the butyryl-CoA:acetate CoA-transferase gene. Environ. Microbiol. 12, 304–314. doi: 10.1111/j.1462-2920.2009.02066.x
Lozupone, C. A., Stombaugh, J. I., Gordon, J. I., Jansson, J. K., and Knight, R. (2012). Diversity, stability and resilience of the human gut microbiota. Nature 489, 220–230. doi: 10.1038/nature11550
Lu, H., Xu, X., Fu, D., Zou, D., Han, L., and Zhao, W. (2022). Butyrate-producing Eubacterium rectale suppresses lymphomagenesis by alleviating the TNF-induced TLR4/MyD88/NF-κB axis. Cell Host Microbe 30, 1139–1150.e7. doi: 10.1016/j.chom.2022.07.003
Lv, Z., and Guo, Y. (2020). Metformin and its benefits for various diseases. Front. Endocrinol. (Lausanne) 11, 1–10. doi: 10.3389/fendo.2020.00191
Lynch, S. V., and Pedersen, O. (2016). The human intestinal microbiome in health and disease. N. Engl. J. Med. 375, 2369–2379. doi: 10.1056/NEJMRA1600266
Ma, Y., You, X., Mai, G., Tokuyasu, T., and Liu, C. (2018). A human gut phage catalog correlates the gut phageome with type 2 diabetes. Microbiome 6, 1–12. doi: 10.1186/S40168-018-0410-Y/FIGURES/6
Maioli, T. U., Borras-Nogues, E., Torres, L., Barbosa, S. C., Martins, V. D., Langella, P., et al. (2021). Possible benefits of Faecalibacterium prausnitzii for obesity-associated gut disorders. Front. Pharmacol. 12:740636. doi: 10.3389/FPHAR.2021.740636
Majumdar, S., Lin, Y., and Bettini, M. L. (2022). Host-microbiota interactions shaping T-cell response and tolerance in type 1 diabetes. Front. Immunol. 13:974178. doi: 10.3389/FIMMU.2022.974178
Maloney, J. G., Molokin, A., and Santin, M. (2019). Next generation amplicon sequencing improves detection of Blastocystis mixed subtype infections. Infect. Genet. Evol. 73, 119–125. doi: 10.1016/j.meegid.2019.04.013
Manor, O., Dai, C. L., Kornilov, S. A., Smith, B., Price, N. D., Lovejoy, J. C., et al. (2020). Health and disease markers correlate with gut microbiome composition across thousands of people. Nat. Commun. 11, 1–12. doi: 10.1038/s41467-020-18871-1
Maritz, J. M., Ten Eyck, T. A., Elizabeth Alter, S., and Carlton, J. M. (2019). Patterns of protist diversity associated with raw sewage in New York City. ISME J. 13, 2750–2763. doi: 10.1038/s41396-019-0467-z
Martínez-López, Y. E. N. R., Esquivel-Hernandez, D. A., Cristian, P. M., Aarón, V. J., Paul, S. C. J., David, G. V., et al. (2024). Effect of metformin and metformin/linagliptin on gut microbiota in patients with prediabetes. Sci. Rep. 14, 1–16. doi: 10.1038/s41598-024-60081-y
Matsuo, Y., Komiya, S., Yasumizu, Y., Yasuoka, Y., Mizushima, K., Takagi, T., et al. (2021). Full-length 16S rRNA gene amplicon analysis of human gut microbiota using MinION™ nanopore sequencing confers species-level resolution. BMC Microbiol. 21, 1–13. doi: 10.1186/s12866-021-02094-5
Mejía-León, M. E., Petrosino, J. F., Ajami, N. J., Domínguez-Bello, M. G., and De La Barca, A. M. C. (2014). Fecal microbiota imbalance in Mexican children with type 1 diabetes. Sci. Rep. 4, 1–5. doi: 10.1038/srep03814
Meslier, V., Quinquis, B., Da Silva, K., Plaza Oñate, F., Pons, N., Roume, H., et al. (2022). Benchmarking second and third-generation sequencing platforms for microbial metagenomics. Sci Data 9, 1–9. doi: 10.1038/s41597-022-01762-z
Mohammad, S., and Thiemermann, C. (2021). Role of metabolic Endotoxemia in systemic inflammation and potential interventions. Front. Immunol. 11:594150. doi: 10.3389/FIMMU.2020.594150/BIBTEX
Mojgani, N., and Dadar, M. (Eds.) (2021). Probiotic Bacteria and Postbiotic metabolites: Role in animal and human health. Singapore: Springer Singapore.
Mokhtari, P., Metos, J., and Anandh Babu, P. V. (2021). Impact of type 1 diabetes on the composition and functional potential of gut microbiome in children and adolescents: possible mechanisms, current knowledge, and challenges. Gut Microbes 13, 1–18. doi: 10.1080/19490976.2021.1926841
Morowitz, M. J., Carlisle, E. M., and Alverdy, J. C. (2011). Contributions of intestinal bacteria to nutrition and metabolism in the critically ill. Surg. Clin. North Am. 91, 771–785. doi: 10.1016/J.SUC.2011.05.001
Mrozinska, S., Kapusta, P., Gosiewski, T., Sroka-Oleksiak, A., Ludwig-Słomczyńska, A. H., Matejko, B., et al. (2021). The gut microbiota profile according to glycemic control in type 1 diabetes patients treated with personal insulin pumps. Microorganisms 9, 1–13. doi: 10.3390/microorganisms9010155
Mueller, N. T., Differding, M. K., Zhang, M., Maruthur, N. M., Juraschek, S. P., Miller, E. R., et al. (2021). Metformin affects gut microbiome composition and function and circulating short-chain fatty acids: a randomized trial. Diabetes Care 44, 1462–1471. doi: 10.2337/dc20-2257
Najmanová, L., Vídeňská, P., and Cahová, M. (2022). Healthy microbiome – a mere idea or a sound concept? Physiol. Res. 71, 719–738. doi: 10.33549/physiolres.934967
Nash, A. K., Auchtung, T. A., Wong, M. C., Smith, D. P., Gesell, J. R., Ross, M. C., et al. (2017). The gut mycobiome of the human microbiome project healthy cohort. Microbiome 5:153. doi: 10.1186/s40168-017-0373-4
Nie, K., Ma, K., Luo, W., Shen, Z., Yang, Z., Xiao, M., et al. (2021). Roseburia intestinalis: a beneficial gut organism from the discoveries in genus and species. Front. Cell. Infect. Microbiol. 11:757718. doi: 10.3389/FCIMB.2021.757718
O’Callaghan, A., and van Sinderen, D. (2016). Bifidobacteria and their role as members of the human gut microbiota. Front. Microb. 7:925. doi: 10.3389/FMICB.2016.00925
Ottman, N., Geerlings, S. Y., Aalvink, S., de Vos, W. M., and Belzer, C. (2017). Action and function of Akkermansia muciniphila in microbiome ecology, health and disease. Best Pract. Res. Clin. Gastroenterol. 31, 637–642. doi: 10.1016/j.bpg.2017.10.001
Park, C., Kim, S. B., Choi, S. H., and Kim, S. (2021). Comparison of 16S rRNA gene based microbial profiling using five next-generation sequencers and various primers. Front. Microbiol. 12, 1–15. doi: 10.3389/fmicb.2021.715500
Parsaei, M., Sarafraz, N., Moaddab, S. Y., and Ebrahimzadeh Leylabadlo, H. (2021). The importance of Faecalibacterium prausnitzii in human health and diseases. New Microbes New Infect 43:100928. doi: 10.1016/J.NMNI.2021.100928
Patel, B. K., Patel, K. H., Huang, R. Y., Lee, C. N., and Moochhala, S. M. (2022). The gut-skin microbiota Axis and its role in diabetic wound healing—a review based on current literature. Int. J. Mol. Sci. 23:2375. doi: 10.3390/ijms23042375
Paun, A., Yau, C., and Danska, J. S. (2017). The influence of the microbiome on type 1 diabetes. J. Immunol. 198, 590–595. doi: 10.4049/jimmunol.1601519
Petersmann, A., Nauck, M., Müller-Wieland, D., Kerner, W., Müller, U. A., Landgraf, R., et al. (2018). Definition, classification and diagnostics of diabetes mellitus. J. Lab. Med. 42, 73–79. doi: 10.1515/labmed-2018-0016
Peterson, C. T., Perez Santiago, J., Iablokov, S. N., Chopra, D., Rodionov, D. A., and Peterson, S. N. (2022). Short-chain fatty acids modulate healthy gut microbiota composition and functional potential. Curr. Microbiol. 79, 1–13. doi: 10.1007/S00284-022-02825-5/FIGURES/5
Piłaciński, S., and Zozulińska-Ziółkiewicz, D. A. (2014). Influence of lifestyle on the course of type 1 diabetes mellitus. Arch. Med. Sci. 10, 124–134. doi: 10.5114/aoms.2014.40739
Pingitore, A., Chambers, E. S., Hill, T., Maldonado, I. R., Liu, B., Bewick, G., et al. (2017). The diet-derived short chain fatty acid propionate improves beta-cell function in humans and stimulates insulin secretion from human islets in vitro. Diabetes Obes. Metab. 19, 257–265. doi: 10.1111/DOM.12811
Pinto, A. J., and Raskin, L. (2012). PCR biases distort bacterial and archaeal community structure in pyrosequencing datasets. PLoS One 7:e43093. doi: 10.1371/journal.pone.0043093
Plöger, S., Stumpff, F., Penner, G. B., Schulzke, J. D., Gäbel, G., Martens, H., et al. (2012). Microbial butyrate and its role for barrier function in the gastrointestinal tract. Ann. N. Y. Acad. Sci. 1258, 52–59. doi: 10.1111/j.1749-6632.2012.06553.x
Portincasa, P., Bonfrate, L., Vacca, M., De Angelis, M., Farella, I., Lanza, E., et al. (2022). Gut microbiota and short chain fatty acids: implications in glucose homeostasis. Int. J. Mol. Sci. 23:1105. doi: 10.3390/IJMS23031105
Pourkazemi, A., Ghanbari, A., Khojamli, M., Balo, H., Hemmati, H., Jafaryparvar, Z., et al. (2020). Diabetic foot care: knowledge and practice. BMC Endocr. Disord. 20:40. doi: 10.1186/S12902-020-0512-Y
Qi, C. J., Zhang, Q., Yu, M., Xu, J. P., Zheng, J., Wang, T., et al. (2016). Imbalance of fecal microbiota at newly diagnosed type 1 diabetes in Chinese children. Chin. Med. J. 129, 1298–1304. doi: 10.4103/0366-6999.182841
Qian, X., Si, Q., Lin, G., Zhu, M., Lu, J., Zhang, H., et al. (2022). Bifidobacterium adolescentis is effective in relieving type 2 diabetes and may be related to its dominant Core genome and gut microbiota modulation capacity. Nutrients 14:2479. doi: 10.3390/nu14122479
Qin, J., Li, Y., Cai, Z., Li, S., Zhu, J., Zhang, F., et al. (2012). A metagenome-wide association study of gut microbiota in type 2 diabetes. Nature 490, 55–60. doi: 10.1038/nature11450
Raimondi, S., Amaretti, A., Gozzoli, C., Simone, M., Righini, L., Candeliere, F., et al. (2019). Longitudinal survey of fungi in the human gut: ITS profiling, phenotyping, and colonization. Front. Microbiol. 10, 1–12. doi: 10.3389/fmicb.2019.01575
Ranjan, R., Rani, A., Metwally, A., McGee, H. S., and Perkins, D. L. (2016). Analysis of the microbiome: advantages of whole genome shotgun versus 16S amplicon sequencing. Biochem. Biophys. Res. Commun. 469, 967–977. doi: 10.1016/j.bbrc.2015.12.083
Riedel, S., Pheiffer, C., Johnson, R., Louw, J., and Muller, C. J. F. (2022). Intestinal barrier function and immune homeostasis are missing links in obesity and type 2 diabetes development. Front. Endocrinol. (Lausanne) 12:833544. doi: 10.3389/FENDO.2021.833544/BIBTEX
Rinninella, E., Raoul, P., Cintoni, M., Franceschi, F., Miggiano, G. A. D., Gasbarrini, A., et al. (2019). What is the healthy gut microbiota composition? A changing ecosystem across age, environment, diet, and diseases. Microorganisms 7:14. doi: 10.3390/microorganisms7010014
Rivière, A., Selak, M., Lantin, D., Leroy, F., and De Vuyst, L. (2016). Bifidobacteria and butyrate-producing colon bacteria: importance and strategies for their stimulation in the human gut. Front. Microbiol. 7:979. doi: 10.3389/fmicb.2016.00979
Roberts, L. D., Koulman, A., and Griffin, J. L. (2014). Towards metabolic biomarkers of insulin resistance and type 2 diabetes: Progress from the metabolome. Lancet Diabetes Endocrinol. 2, 65–75. doi: 10.1016/S2213-8587(13)70143-8
Rodrigues, V. F., Elias-Oliveira, J., Pereira, Í. S., Pereira, J. A., Barbosa, S. C., Machado, M. S. G., et al. (2022). Akkermansia muciniphila and gut immune system: a good friendship that attenuates inflammatory bowel disease, obesity, and diabetes. Front. Immunol. 13:934695. doi: 10.3389/FIMMU.2022.934695
Roep, B. O., Thomaidou, S., van Tienhoven, R., and Zaldumbide, A. (2020). Type 1 diabetes mellitus as a disease of the β-cell (do not blame the immune system?). Nat. Rev. Endocrinol. 17, 150–161. doi: 10.1038/s41574-020-00443-4
Ropot, A. V., Karamzin, A. M., and Sergeyev, O. V. (2020). Cultivation of the next-generation probiotic Akkermansia muciniphila, methods of its safe delivery to the intestine, and factors contributing to its growth in vivo. Curr. Microbiol. 77, 1363–1372. doi: 10.1007/s00284-020-01992-7
Rutsch, A., Kantsjö, J. B., and Ronchi, F. (2020). The gut-brain Axis: how microbiota and host Inflammasome influence brain physiology and pathology. Front. Immunol. 11, 1–24. doi: 10.3389/fimmu.2020.604179
Saeedi, P., Petersohn, I., Salpea, P., Malanda, B., Karuranga, S., Unwin, N., et al. (2019). Global and regional diabetes prevalence estimates for 2019 and projections for 2030 and 2045: results from the international diabetes federation diabetes atlas, 9th edition. Diabetes Res. Clin. Pract. 157:107843. doi: 10.1016/J.DIABRES.2019.107843
Sanna, S., van Zuydam, N. R., Mahajan, A., Kurilshikov, A., Vich Vila, A., Võsa, U., et al. (2019). Causal relationships among the gut microbiome, short-chain fatty acids and metabolic diseases. Nat. Genet. 51, 600–605. doi: 10.1038/s41588-019-0350-x
Sato, J., Kanazawa, A., Ikeda, F., Yoshihara, T., Goto, H., Abe, H., et al. (2014). Gut dysbiosis and detection of “live gut bacteria” in blood of Japanese patients with type 2 diabetes. Diabetes Care 37, 2343–2350. doi: 10.2337/dc13-2817
Schoch, C. L., Seifert, K. A., Huhndorf, S., Robert, V., Spouge, J. L., Levesque, C. A., et al. (2012). Nuclear ribosomal internal transcribed spacer (ITS) region as a universal DNA barcode marker for Fungi. Proc. Natl. Acad. Sci. USA 109, 6241–6246. doi: 10.1073/pnas.1117018109
Scott, K. P., Martin, J. C., Campbell, G., Mayer, C. D., and Flint, H. J. (2006). Whole-genome transcription profiling reveals genes up-regulated by growth on fucose in the human gut bacterium “Roseburia inulinivorans. J. Bacteriol. 188, 4340–4349. doi: 10.1128/JB.00137-06
Sechovcová, H., Mahayri, T. M., Mrázek, J., Jarošíková, R., Husáková, J., Wosková, V., et al. (2023). Gut microbiota in relationship to diabetes mellitus and its late complications with a focus on diabetic foot syndrome: a review. Folia Microbiol. (Praha). 69, 259–282. doi: 10.1007/s12223-023-01119-y
Secondulfo, M., Iafusco, D., Carratù, R., deMagistris, L., Sapone, A., Generoso, M., et al. (2004). Ultrastructural mucosal alterations and increased intestinal permeability in non-celiac, type I diabetic patients. Dig. Liver Dis. 36, 35–45. doi: 10.1016/J.DLD.2003.09.016
Sedighi, M., Razavi, S., Navab-Moghadam, F., Khamseh, M. E., Alaei-Shahmiri, F., Mehrtash, A., et al. (2017). Comparison of gut microbiota in adult patients with type 2 diabetes and healthy individuals. Microb. Pathog. 111, 362–369. doi: 10.1016/j.micpath.2017.08.038
Seekatz, A. M., Safdar, N., and Khanna, S. (2022). The role of the gut microbiome in colonization resistance and recurrent Clostridioides difficile infection. Ther. Adv. Gastroenterol. 15:17562848221134396. doi: 10.1177/17562848221134396
Shang, J., Liu, F., Zhang, B., Dong, K., Lu, M., Jiang, R., et al. (2021). Liraglutide-induced structural modulation of the gut microbiota in patients with type 2 diabetes mellitus. PeerJ 9, 1–19. doi: 10.7717/peerj.11128
Sharma, S., and Tripathi, P. (2019). Gut microbiome and type 2 diabetes: where we are and where to go? J. Nutr. Biochem. 63, 101–108. doi: 10.1016/J.JNUTBIO.2018.10.003
Sikalidis, A. K., and Maykish, A. (2020). The gut microbiome and type 2 diabetes mellitus: discussing a complex relationship. Biomedicines 8:8. doi: 10.3390/BIOMEDICINES8010008
Silva, Y. P., Bernardi, A., and Frozza, R. L. (2020). The role of short-chain fatty acids from gut microbiota in gut-brain communication. Front. Endocrinol. (Lausanne) 11, 1–14. doi: 10.3389/fendo.2020.00025
Singh, V., Lee, G. D., Son, H. W., Koh, H., Kim, E. S., Unno, T., et al. (2023). Butyrate producers, “the sentinel of gut”: their intestinal significance with and beyond butyrate, and prospective use as microbial therapeutics. Front. Microbiol. 13:1103836. doi: 10.3389/FMICB.2022.1103836
Sirichoat, A., Sankuntaw, N., Engchanil, C., Buppasiri, P., Faksri, K., Namwat, W., et al. (2021). Comparison of different hypervariable regions of 16S rRNA for taxonomic profiling of vaginal microbiota using next-generation sequencing. Arch. Microbiol. 203, 1159–1166. doi: 10.1007/s00203-020-02114-4
Smits, M. M., Fluitman, K. S., Herrema, H., Davids, M., Kramer, M. H. H., Groen, A. K., et al. (2021). Liraglutide and sitagliptin have no effect on intestinal microbiota composition: a 12-week randomized placebo-controlled trial in adults with type 2 diabetes. Diabetes Metab. 47:101223. doi: 10.1016/j.diabet.2021.101223
Sorini, C., Cosorich, I., Conte, M. L., De Giorgi, L., Facciotti, F., Lucianò, R., et al. (2019). Loss of gut barrier integrity triggers activation of islet-reactive T cells and autoimmune diabetes. Proc. Natl. Acad. Sci. USA 116, 15140–15149. doi: 10.1073/PNAS.1814558116
Soyucen, E., Gulcan, A., Aktuglu-Zeybek, A. C., Onal, H., Kiykim, E., and Aydin, A. (2014). Differences in the gut microbiota of healthy children and those with type 1 diabetes. Pediatr. Int. 56, 336–343. doi: 10.1111/ped.12243
Su, L., Hong, Z., Zhou, T., Jian, Y., Xu, M., Zhang, X., et al. (2022). Health improvements of type 2 diabetic patients through diet and diet plus fecal microbiota transplantation. Sci. Rep. 12, 1–12. doi: 10.1038/s41598-022-05127-9
Su, B., Liu, H., Li, J., Sunli, Y., Liu, B., Liu, D., et al. (2015). Acarbose treatment affects the serum levels of inflammatory cytokines and the gut content of bifidobacteria in Chinese patients with type 2 diabetes mellitus. J. Diabetes 7, 729–739. doi: 10.1111/1753-0407.12232
Sun, J., Furio, L., Mecheri, R., van der Does, A. M., Lundeberg, E., Saveanu, L., et al. (2015). Pancreatic β-cells limit autoimmune diabetes via an Immunoregulatory antimicrobial peptide expressed under the influence of the gut microbiota. Immunity 43, 304–317. doi: 10.1016/J.IMMUNI.2015.07.013
Tai, N., Wong, F. S., and Wen, L. (2015). The role of gut microbiota in the development of type 1, type 2 diabetes mellitus and obesity. Rev. Endocr. Metab. Disord. 16, 55–65. doi: 10.1007/S11154-015-9309-0
Takahashi, S., Tomita, J., Nishioka, K., Hisada, T., and Nishijima, M. (2014). Development of a prokaryotic universal primer for simultaneous analysis of Bacteria and Archaea using next-generation sequencing. PLoS One 9:e105592. doi: 10.1371/journal.pone.0105592
Takewaki, F., Nakajima, H., Takewaki, D., Hashimoto, Y., Majima, S., Okada, H., et al. (2021). Habitual dietary intake affects the altered pattern of gut microbiome by acarbose in patients with type 2 diabetes. Nutrients 13, 1–14. doi: 10.3390/nu13062107
Tamanai-Shacoori, Z., Smida, I., Bousarghin, L., Loreal, O., Meuric, V., Fong, S. B., et al. (2017). Roseburia spp.: a marker of health? Future Microbiol. 12, 157–170. doi: 10.2217/FMB-2016-0130
Tang, J., Xu, L., Zeng, Y., and Gong, F. (2021). Effect of gut microbiota on LPS-induced acute lung injury by regulating the TLR4/NF-kB signaling pathway. Int. Immunopharmacol. 91:107272. doi: 10.1016/J.INTIMP.2020.107272
Tetz, G., Brown, S. M., Hao, Y., and Tetz, V. (2019). Type 1 diabetes: an association between autoimmunity, the dynamics of gut Amyloid-producing E. Coli and their phages. Sci. Rep. 9:9685. doi: 10.1038/s41598-019-46087-x
Thingholm, L. B., Rühlemann, M. C., Koch, M., Fuqua, B., Laucke, G., Boehm, R., et al. (2019). Obese individuals with and without type 2 diabetes show different gut microbial functional capacity and composition. Cell Host Microbe 26, 252–264.e10. doi: 10.1016/j.chom.2019.07.004
Tilg, H., and Moschen, A. R. (2014). Microbiota and diabetes: an evolving relationship. Gut 63, 1513–1521. doi: 10.1136/GUTJNL-2014-306928
Turley, S. J., Lee, J. W., Dutton-Swain, N., Mathis, D., and Benoist, C. (2005). Endocrine self and gut non-self intersect in the pancreatic lymph nodes. Proc. Natl. Acad. Sci. USA 102, 17729–17733. doi: 10.1073/PNAS.0509006102
Tuttolomondo, A., Maida, C., and Pinto, A. (2015). Diabetic foot syndrome as a possible cardiovascular marker in diabetic patients. J. Diabetes Res. 2015:268390. doi: 10.1155/2015/268390
Usta-Gorgun, B., and Yilmaz-Ersan, L. (2020). Short-chain fatty acids production by Bifidobacterium species in the presence of salep. Electron. J. Biotechnol. 47, 29–35. doi: 10.1016/J.EJBT.2020.06.004
Vaarala, O. (2012). Gut microbiota and type 1 diabetes. Rev. Diabet. Stud. 9, 251–259. doi: 10.1900/RDS.2012.9.251
Vaarala, O. (2013). Human intestinal microbiota and type 1 diabetes. Curr. Diab. Rep. 13, 601–607. doi: 10.1007/s11892-013-0409-5
Vaarala, O. (2018). Leaking gut in type 1 diabetes. Curr. Opin. Gastroenterol. 6, 701–6. doi: 10.1097/MOG.0b013e32830e6d98
van Bommel, E. J. M., Herrema, H., Davids, M., Kramer, M. H. H., Nieuwdorp, M., and van Raalte, D. H. (2020). Effects of 12-week treatment with dapagliflozin and gliclazide on faecal microbiome: results of a double-blind randomized trial in patients with type 2 diabetes. Diabetes Metab. 46, 164–168. doi: 10.1016/j.diabet.2019.11.005
van Tilburg Bernardes, E., Pettersen, V. K., Gutierrez, M. W., Laforest-Lapointe, I., Jendzjowsky, N. G., Cavin, J. B., et al. (2020). Intestinal fungi are causally implicated in microbiome assembly and immune development in mice. Nat. Commun. 11, 1–16. doi: 10.1038/s41467-020-16431-1
Vatanen, T., Franzosa, E. A., Schwager, R., Tripathi, S., Arthur, T. D., Vehik, K., et al. (2018). The human gut microbiome in early-onset type 1 diabetes from the TEDDY study. Nature 562, 589–594. doi: 10.1038/s41586-018-0620-2
Vehik, K., Lynch, K. F., Wong, M. C., Tian, X., Ross, M. C., Gibbs, R. A., et al. (2019). Prospective virome analyses in young children at increased genetic risk for type 1 diabetes. Nat. Med. 25, 1865–1872. doi: 10.1038/s41591-019-0667-0
Vekic, J., Silva-Nunes, J., and Rizzo, M. (2022). Glucose metabolism disorders: challenges and opportunities for diagnosis and treatment. Meta 12, 10–13. doi: 10.3390/metabo12080712
Vrieze, A., Van Nood, E., Holleman, F., Salojärvi, J., Kootte, R. S., Bartelsman, J. F. W. M., et al. (2012). Transfer of intestinal microbiota from lean donors increases insulin sensitivity in individuals with metabolic syndrome. Gastroenterology 143, 913–916.e7. doi: 10.1053/J.GASTRO.2012.06.031
Wang, N., and Fang, J. Y. (2023). Fusobacterium nucleatum, a key pathogenic factor and microbial biomarker for colorectal cancer. Trends Microbiol. 31, 159–172. doi: 10.1016/j.tim.2022.08.010
Wang, G., Hu, Y. X., He, M. Y., Xie, Y. H., Su, W., Long, D., et al. (2021). Gut-lung Dysbiosis accompanied by diabetes mellitus leads to pulmonary fibrotic change through the NF-κB signaling pathway. Am. J. Pathol. 191, 838–856. doi: 10.1016/J.AJPATH.2021.02.019
Wang, H., Lu, Y., Yan, Y., Tian, S., Zheng, D., Leng, D., et al. (2020). Promising treatment for type 2 diabetes: fecal microbiota transplantation reverses insulin resistance and impaired islets. Front. Cell. Infect. Microbiol. 9, 1–10. doi: 10.3389/fcimb.2019.00455
Wang, Z., Wang, J., Hu, J., Chen, Y., Dong, B., and Wang, Y. (2021). A comparative study of acarbose, vildagliptin and saxagliptin intended for better efficacy and safety on type 2 diabetes mellitus treatment. Life Sci. 274:119069. doi: 10.1016/j.lfs.2021.119069
Wu, H., Esteve, E., Tremaroli, V., Khan, M. T., Caesar, R., Mannerås-Holm, L., et al. (2017). Metformin alters the gut microbiome of individuals with treatment-naive type 2 diabetes, contributing to the therapeutic effects of the drug. Nat. Med. 23, 850–858. doi: 10.1038/nm.4345
Wu, H., Tremaroli, V., Schmidt, C., Lundqvist, A., Olsson, L. M., Krämer, M., et al. (2020). The gut microbiota in prediabetes and diabetes: a population-based cross-sectional study. Cell Metab. 32, 379–390.e3. doi: 10.1016/j.cmet.2020.06.011
Wu, S., Xiong, J., and Yu, Y. Y. (2015). Taxonomic resolutions based on 18S rRNA genes: a case study of subclass Copepoda. PLoS One 10, 1–19. doi: 10.1371/journal.pone.0131498
Wu, G., Xu, T., Zhao, N., Lam, Y. Y., Ding, X., Wei, D., et al. (2024). A core microbiome signature as an indicator of health. Cell 187, 6550–6565.e11. doi: 10.1016/J.CELL.2024.09.019
Wurzbacher, C., Larsson, E., Bengtsson-Palme, J., Van den Wyngaert, S., Svantesson, S., Kristiansson, E., et al. (2019). Introducing ribosomal tandem repeat barcoding for fungi. Mol. Ecol. Resour. 19, 118–127. doi: 10.1111/1755-0998.12944
Xia, Y., Xie, Z., Huang, G., and Zhou, Z. (2019). Incidence and trend of type 1 diabetes and the underlying environmental determinants. Diabetes Metab. Res. Rev. 35:e3075. doi: 10.1002/dmrr.3075
Xie, Y. C., Jing, X. B., Chen, X., Chen, L. Z., Zhang, S. H., and Cai, X. B. (2022). Fecal microbiota transplantation treatment for type 1 diabetes mellitus with malnutrition: a case report. Ther. Adv. Chronic Dis. 13:20406223221117449. doi: 10.1177/20406223221117449
Yang, R. H., Su, J. H., Shang, J. J., Wu, Y. Y., Li, Y., Bao, D. P., et al. (2018). Evaluation of the ribosomal DNA internal transcribed spacer (ITS), specifically ITS1 and ITS2, for the analysis of fungal diversity by deep sequencing. PLoS One 13, 1–17. doi: 10.1371/journal.pone.0206428
Yang, T., Wang, H., Li, C., and Duan, H. (2023). Mechanisms of drugs in the treatment of type 2 diabetes mellitus. Chin. Med. J. 136, 394–396. doi: 10.1097/CM9.0000000000002356
Yang, X., Zhang, M., Liu, Y., Wei, F., Li, X., Feng, Y., et al. (2023). Inulin-enriched Megamonas funiformis ameliorates metabolic dysfunction-associated fatty liver disease by producing propionic acid. NPJ Biofilms Microbiomes 9, 1–16. doi: 10.1038/s41522-023-00451-y
Yazdanpanah, L., Shahbazian, H., Nazari, I., Arti, H. R., Ahmadi, F., Mohammadianinejad, S. E., et al. (2018). Incidence and risk factors of diabetic foot ulcer: a population-based diabetic foot cohort (ADFC study)-two-year follow-up study. Int. J. Endocrinol. 2018:7631659. doi: 10.1155/2018/7631659
Yuan, J. H., Xie, Q. S., Chen, G. C., Huang, C. L., Yu, T., Chen, Q. K., et al. (2021). Impaired intestinal barrier function in type 2 diabetic patients measured by serum LPS, Zonulin, and IFABP. J. Diabetes Complicat. 35:107766. doi: 10.1016/J.JDIACOMP.2020.107766
Ze, X., David, Y. B., Laverde-Gomez, J. A., Dassa, B., Sheridan, P. O., Duncan, S. H., et al. (2015). Unique organization of extracellular amylases into amylosomes in the resistant starch-utilizing human colonic firmicutes bacterium ruminococcus bromii. MBio 6:e01058-15. doi: 10.1128/MBIO.01058-15
Zhang, F., Aschenbrenner, D., Yoo, J. Y., and Zuo, T. (2022). The gut mycobiome in health, disease, and clinical applications in association with the gut bacterial microbiome assembly. Lancet Microbe 3, e969–e983. doi: 10.1016/S2666-5247(22)00203-8
Zhang, L., Chu, J., Hao, W., Zhang, J., Li, H., Yang, C., et al. (2021). Gut microbiota and type 2 diabetes mellitus: association, mechanism, and translational applications. Mediat. Inflamm. 2021:5110276. doi: 10.1155/2021/5110276
Zhang, Q., and Hu, N. (2020). Effects of metformin on the gut microbiota in obesity and type 2 diabetes mellitus. Diabetes Metab. Syndr. Obes. 13, 5003–5014. doi: 10.2147/DMSO.S286430
Zhang, D., Jian, Y. P., Zhang, Y. N., Li, Y., Gu, L. T., Sun, H. H., et al. (2023). Short-chain fatty acids in diseases. Cell Commun. Signal 21:212. doi: 10.1186/S12964-023-01219-9
Zhang, A. H., Qiu, S., Xu, H. Y., Sun, H., and Wang, X. J. (2014). Metabolomics in diabetes. Clin. Chim. Acta 429, 106–110. doi: 10.1016/J.CCA.2013.11.037
Zhang, X., Shen, D., Fang, Z., Jie, Z., Qiu, X., Zhang, C., et al. (2013). Human gut microbiota changes reveal the progression of glucose intolerance. PLoS One 8:e71108. doi: 10.1371/journal.pone.0071108
Zhang, F., Wang, M., Yang, J., Xu, Q., Liang, C., Chen, B., et al. (2019). Response of gut microbiota in type 2 diabetes to hypoglycemic agents. Endocrine 66, 485–493. doi: 10.1007/S12020-019-02041-5
Zhang, X., Zhang, Y., Luo, L., Le, Y., Li, Y., Yuan, F., et al. (2023). The beneficial effects of a multispecies probiotic supplement on Glycaemic control and metabolic profile in adults with type 1 diabetes: a randomised, double-blinded, placebo-controlled pilot-study. Diabetes Metab. Syndr. Obes. 16, 829–840. doi: 10.2147/DMSO.S400119
Zhao, L., Lou, H., Peng, Y., Chen, S., Zhang, Y., and Li, X. (2019). Comprehensive relationships between gut microbiome and faecal metabolome in individuals with type 2 diabetes and its complications. Endocrine 66, 526–537. doi: 10.1007/s12020-019-02103-8
Zhao, G., Vatanen, T., Droit, L., Park, A., Kostic, A. D., Poon, T. W., et al. (2017). Intestinal virome changes precede autoimmunity in type I diabetes-susceptible children. Proc. Natl. Acad. Sci. USA 114, E6166–E6175. doi: 10.1073/PNAS.1706359114
Zheng, D., Liwinski, T., and Elinav, E. (2020). Interaction between microbiota and immunity in health and disease. Cell Res. 30, 492–506. doi: 10.1038/s41422-020-0332-7
Zimmermann, M., Zimmermann-Kogadeeva, M., Wegmann, R., and Goodman, A. L. (2019). Mapping human microbiome drug metabolism by gut bacteria and their genes. Nature 570, 462–467. doi: 10.1038/s41586-019-1291-3
Zmora, N., Zeevi, D., Korem, T., Segal, E., and Elinav, E. (2016). Taking it personally: personalized utilization of the human microbiome in health and disease. Cell Host Microbe 19, 12–20. doi: 10.1016/J.CHOM.2015.12.016
Zou, Y., Xue, W., Luo, G., Deng, Z., Qin, P., Guo, R., et al. (2019). 1,520 reference genomes from cultivated human gut Bacteria enable functional microbiome analyses. Nat. Biotechnol. 37, 179–185. doi: 10.1038/s41587-018-0008-8
Keywords: gut microbiota, diabetes mellitus, T1DM, T2DM, antidiabetic drugs
Citation: Fliegerová KO, Mahayri TM, Sechovcová H, Mekadim C, Mrázek J, Jarošíková R, Dubský M and Fejfarová V (2025) Diabetes and gut microbiome. Front. Microbiol. 15:1451054. doi: 10.3389/fmicb.2024.1451054
Edited by:
Yangyang Chai, Northeast Forestry University, ChinaReviewed by:
Zhangran Chen, Xiamen University, ChinaDiego Armando Esquivel Hernandez, Metropolitan Autonomous University, Mexico
Copyright © 2025 Fliegerová, Mahayri, Sechovcová, Mekadim, Mrázek, Jarošíková, Dubský and Fejfarová. This is an open-access article distributed under the terms of the Creative Commons Attribution License (CC BY). The use, distribution or reproduction in other forums is permitted, provided the original author(s) and the copyright owner(s) are credited and that the original publication in this journal is cited, in accordance with accepted academic practice. No use, distribution or reproduction is permitted which does not comply with these terms.
*Correspondence: Tiziana Maria Mahayri, bWFoYXlyaUBpYXBnLmNhcy5jeg==