- 1Section of Geogenetics, Globe Institute, University of Copenhagen, Øster Voldgade, Denmark
- 2Section for Hologenomics, Globe Institute, University of Copenhagen, Øster Farimagsgade, Denmark
- 3Section of Cell and Tissue Biology Cancer Research, Department of Experimental Medical Sciences, Lund University, Sölvegatan, Sweden
- 4Section of Microbiology, Department of Biology, University of Copenhagen, Universitetsparken, Denmark
Horizontal gene transfer is one of the most important drivers of bacterial evolution. Transformation by uptake of extracellular DNA is traditionally not considered to be an effective mode of gene acquisition, simply because extracellular DNA is degraded in a matter of days when it is suspended in e.g. seawater. Recently the age span of stored DNA was increased to at least 2 Ma. Here, we show that Acinetobacter baylyi can incorporate 60 bp DNA fragments adsorbed to common sedimentary minerals and that the transformation frequencies scale with mineral surface properties. Our work highlights that ancient environmental DNA can fuel the evolution of contemporary bacteria. In contrast to heritable stochastic mutations, the processes by which bacteria acquire new genomic material during times of increased stress and needs, indicate a non-random mechanism that may propel evolution in a non-stochastic manner.
1 Introduction
Horizontal gene transfer (HGT) is recognized as the key source of accelerating genome innovation (Jain et al., 2003). In HGT, extracellular or intracellular DNA from one species can be incorporated into a related or non-related species, and thereby efficiently increase genetic diversity. HGT is considered a common route to adaptation in microbes and has significantly impacted the evolution of life of both prokaryotes and eukaryotes (Ochman et al., 2000; Keeling and Palmer, 2008). HGT through conjugation and transduction is relatively well explored and conjugation is considered a key mechanism for the propagation of antibiotic resistance genes. HGT by transformation, i.e., uptake of extracellular DNA, is currently not considered an effective mode of gene acquisition because extracellular DNA degrades in a matter of days when it is suspended in seawater or a soil environment (Dejean et al., 2011; Thomsen et al., 2012a; Thomsen et al., 2012b; Sirois and Buckley, 2019). However, advances made in the field of sedimentary ancient DNA underline that adsorption of DNA to mineral surfaces significantly enhances DNA preservation (Kjær et al., 2022). The time-span of DNA preservation was just increased from 1.45 million years to at least 2 Ma years (Kjær et al., 2022). Currently, there is about 0.4 Gt of extracellular DNA associated with sediments just in the top 10 cm of the ocean floor (Dell'Anno and Danovaro, 2005) and in principle, all sedimentary minerals hold a potential to adsorb some amount of extracellular DNA.
The adsorption capacity determines how much DNA the mineral can adsorb at a given DNA concentration Minerals with an overall positive surface charge and high densities of active sites such as clay edges, carbonates and oxyhydroxides are shown to have a higher DNA adsorption capacity and are less dependent on solution composition than negatively charged minerals such as clay basal planes and silicate minerals. Active sites of minerals are a measure of the surface reactivity of the minerals and describe the capability of the minerals to participate in surface-mediated processes, binding to various ions and large molecules such as DNA. The minerals applied in this study have a large variability in parameters such as surface structures, charges, charge densities resulting in a wide range of surface reactivities and active site density between them. To retain DNA, the negatively charged minerals depend on positively charged background ions. Further, it has been shown that the higher the charge density and topographic surface features, the more the DNA is immobilized (Freeman and Harding, 2023). Besides preserving adsorbed DNA, mineral surfaces can also act as vehicles for DNA transport. When adsorbed to a mineral surface, DNA from a niche in a given environment can enter a distal ecosystem through common sedimentary pathways, and potentially offer beneficial traits. It is generally found that if an acquired gene encodes beneficial traits in a given environment, it will provide the host with a fitness advantage, resulting in stabilization of the trait(s) in the population due to natural selection (Hall et al., 2020).
The likelihood of a transformation event from mineral adsorbed DNA is enhanced by the anchoring of many bacteria to mineral surfaces and the vast amount of DNA stored in sediments. Bacteria are found to colonize any surface in most sedimentary systems (Flemming and Wuertz, 2019; Morono et al., 2020), and have ample opportunities to encounter mineral surfaces both in the water column and minerals depositing or deposited on, e.g., a sea floor. Contemporary bacteria also inhabit sedimentary deposits formed in the past and gain access to past genes. The mixing of modern microbes and ancient DNA in principle represents a pathway for gene transfer, across a scale of time and space, unrivaled by any other avenue.
Transformation of mineral-adsorbed large DNA molecules such as plasmid and whole genomic DNA was shown from clays (kaolinite and montmorillonite) and iron oxy-hydroxides (goethite; Khanna and Stotzky, 1992; Huang et al., 2021). The mechanism of integration of kilobase sized DNA molecules is complex requiring the enzyme RecA and a strong sequence homology. While DNA molecules are found to be associated with many sediments (Heuer and Smalla, 2012), it is highly unlikely that the DNA will remain intact for long timescales, owing to degradation processes such as spontaneous hydrolysis, action of nucleases and UV-mediated degradation (Hunting, 2013). Sedimentary DNA molecules are highly fragmented and degraded and are usually shorter than 100 base pairs (bp; Pääbo, 1989; Armbrecht et al., 2020).
Uptake through transformation of short DNA fragments can, in principle, occur in any bacterial species, owing to the simple and spontaneous uptake mechanism and the sheer abundance of fragmented DNA in the environment (Overballe-Petersen and Willerslev, 2014). In contrast to integration of kilobase sized DNA, during uptake of fragmented DNA, the bacteria degrade the DNA double helix and release single-stranded DNA into the cell. When the bacterial genome replicates, the released single stranded fragments can act as primers of new DNA and bind to the open chromosome near the replication fork, thereby incorporating foreign DNA in the genome of one of the daughter cells (Overballe-Petersen and Willerslev, 2014). Successful transformation of bacteria by short suspended extracellular DNA fragments has been shown for Acinetobacter baylyi (Overballe-Petersen et al., 2013). Acinetobacter baylyi is a soil bacterium that is naturally highly competent and recombinogenic with no specific sequence requirements for DNA uptake.
The involvement of a mineral surface in a transformation event brings the interfacial reactions between the molecule, surfaces and/ or bacteria in play as potential co-drivers for the uptake process. I.e. it is yet not known if a strong DNA-mineral affinity (bond strength, density of binding sites, types of bonds etc.) decrease or even prevent uptake by decreasing the availability of the DNA molecule to the bacteria. Here, we explore if mineral-adsorbed short DNA fragments are available for bacterial uptake via HGT in A. baylyi. We pre-adsorbed 60 bp DNA molecules encoding the trpE gene, to a broad range of environmentally relevant soil minerals, (iron oxides and hydroxides (hematite and goethite); clays (kaolinite and mica); carbonates and non-clay silicates (quartz)) and measured the bacterial uptake efficiency per μg of the adsorbed DNA for nutritionally deficient and rich conditions. We evaluate how mineral surface characteristics impact transformation efficiency and further set the results in sedimentologic and ecologic contexts and elaborate on how this process could influence natural selection and the evolution of life as suggested by Sand and Jelavić (2018).
2 Materials and methods
2.1 Minerals and their characterization
The Hematite (001) face used for AFM was a polished, single crystal of hematite (HEM). The face was cleaned in 1 M NaOH at 60°C for 1 h to remove fatty contamination. Subsequently, it was sonicated for 20 min, rinsed with molecular grade water and UV-ozone cleaned for 20 min. Goethite was synthesized by modifying the protocol of Martina et al., (2022). Briefly, 24 grams of Ferric (III) nitrate nonahydrate (Fe(NO3)3.9H2O; Sigma) were added to 300 mL MilliQ water while stirring vigorously using a magnetic stirrer. The solution was simultaneously titrated with 2 M NaOH until it reached a pH of 9. The solution was stirred for another 30 min to allow the reaction to reach completion. The resulting brown precipitate was allowed to age to the yellow-colored goethite by incubation at 60°C for 24 h. Goethite was then washed thoroughly with MilliQ water until no nitrate was detected in the supernatant (Nitrate detection strips, Supelco, Sigma). The purity of the synthesized goethite was confirmed using FTIR spectroscopy (Bruker Vertex 80v FTIR-ATR spectrometer; Supplementary Figure S1.1).
Kaolinite (Kga-1b) was purchased from the Clay Minerals Society. Calcite was purchased from Sigma and cleaned according to Belova et al. (2014). Quartz was purchased as large crystals which were ground and sieved to obtain <50 μm particles. The Mica powder was from an outcrop in the French alps and the mica substrate used for AFM is V1 grade purchased at Ted Pella Inc.
For mineral characterization, specific surface areas (SSAs) of the minerals were calculated by the Brunauer–Emmett–Teller (BET) method on a Quantachrome Autosorb 1. Approximately 0.5 g of each mineral powder was degassed in vacuum (<10–3 Torr) for 2 h at 90°C. Surface area was determined using the BET relationship and nitrogen adsorption isotherms, at liquid nitrogen temperature (77 K), in the relative pressure range, 0.1 < P/P0 < 0.3, where P and P0 are the equilibrium and saturation pressure of the adsorbate (nitrogen gas) respectively.
Using the SSA data obtained by BET studies, the total available mineral surface area in the horizontal gene transformation experiments (SAHGT) was calculated using the following equation:
Detailed calculations are shown as footnotes in Supplementary Table S1.1.
We chose to operate with SSA rather than particle size as SSA is more applied than average particle sizes when it comes to discussing adsorption capacity. Active site density calculations were obtained from literature and extrapolated to our work using the following equation:
Details are present as footnotes in Supplementary Table S1.1.
2.2 Adsorption isotherms of DNA to minerals
The minerals were weighed according to their surface area as listed in Supplementary Table S1.1. Each mineral was disaggregated using a sonicator for 30 min and autoclaved at 121°C, 15 lbs. pressure for 20 min to remove any microbial contamination and resuspended in 1 mL sterile 150 mM NaCl at pH 7. The mineral suspensions were mixed with 0–200 ng/μL salmon sperm DNA in triplicates (also at pH 7; Sigma). Salmon sperm DNA was used as a model DNA molecule for the adsorption experiments to obtain insights into the adsorption capacities for the different minerals and to provide an estimate for the DNA concentration for HGT experiments. The mineral-DNA suspensions were incubated at room temperature for 16 h under constant shaking at 70 rpm. Post incubation, the suspensions were centrifuged at 14000 rpm for 5 min and the DNA released in the supernatant was measured using absorbance at 260 nm using a UV–visible spectrophotometer (Eppendorf). The amount of mineral-adsorbed DNA was estimated using the following equation:
Adsorption isotherms were plotted as equilibrium DNA concentration on the x axis and adsorbed DNA concentration on the y axis. The data were fitted using either the Langmuir equation or the Freundlich equation. The Langmuir model for adsorption followed the equation:
Where Qe is the amount of DNA adsorbed per unit mass of mineral.
KL is the Langmuir adsorption constant.
Qmax is the maximum adsorption capacity of the mineral.
Ce is the equilibrium concentration of the DNA.
The Freundlich adsorption isotherm follows the equation:
where Qe is the amount of DNA adsorbed per unit mass of mineral.
Ce is the equilibrium solution concentration of the DNA,
Kf is the Freundlich adsorption constant, n is the empirical constant.
2.3 Atomic force microscopy
AFM imaging in both air and liquid was performed using a Cypher VRS system (Oxford Instruments). Imaging in air was performed using Tap150 tip with spring constants between 1.5 and 15 N/m and a resonant frequency of 150 kHz. For liquid imaging, AC40 tip (Olympus; Spring constant 0.1 N/m; Resonant Frequency 500 kHz). Scan angle, scan rate, and set-point were systematically varied and multiple sites on every sample were imaged.
All AFM experiments were performed using AFM grade minerals and ultrapure 60 bp DNA (Integrated DNA Technologies, Belgium). All solutions were filtered twice using a 0.2 μm filter before adding the DNA. For drying the samples, we used a gentle stream of N2 gas passed through a 5 μm filter, ensuring rapid drying.
2.3.1 Samples and relevance
AFM is limited to flat surfaces which makes minerals such as mica and calcite suitable. Considering overall surface charge, DNA is expected to display a similar adsorption behavior between mica and quartz. The basal planes of kaolinite are similar to the structure of mica and hence we chose mica to represent these minerals (quartz and kaolinite). Calcite surfaces display charge dense step edges and terraces that are overall positively charged. The iron oxides we applied (goethite and hematite) are nanoparticulate and display a range of mineral surfaces and local charge densities. It is not feasible to image the individual faces with AFM and we applied a flat 001 hematite surface to represent a general trend for DNA adsorption on iron oxides terraces. DNA adsorption to step edges on iron oxides are expected to behave similar to DNA adsorbed on calcite step edges.
2.3.2 Sample preparation, imaging and analysis
A single-phase crystal of hematite was used for AFM imaging. 60 bp ds DNA at a final concentration 0.5 ng/μL in 150 mM NaCl was added to the surface of the hematite crystal and imaged under liquid mode. A clean and uniform calcite surface was obtained by cutting the calcite crystal using a scalpel blade and immediately removing the dust using a gentle N2 stream.
Mica sheet was stuck on the AFM stub using glue and was cleaved using the tape stripping method to obtain a clean sterile surface. For hematite, a flat {001} crystal was used which was cleaned with 1 M NaOH and sterilized under UV-ozone before use. A smooth surface for calcite was obtained by cutting a calcite block with a scalpel. All surfaces were cleaned with a gentle stream of Nitrogen gas.
A stock DNA concentration of 1 ng/uL in 150 mM NaCl was used for all the AFM experiments. DNA was diluted to 0.5 ng/uL using 150 mM NaCl before being added to the mineral surfaces (hematite, mica and calcite). For liquid imaging, the mineral surface was covered with ~15–20 uL 150 mM NaCl and imaged directly under AFM. Thereafter, DNA was introduced, allowed to incubate for 2 min and imaged.
For imaging under air conditions, ~ 40 μL of 0.5 ng/μL DNA was added to the mineral surface and allowed to incubate for 2 min. Post incubation, the mineral surface was gently rinsed with 2X filtered DNAse free ultra pure water, blotted with a kimwipe and dried completely under a gentle stream of N2 gas.
For tracking the movement of DNA across hematite and calcite, images in the TIFF format were retrieved from AFM movies using software Igor Pro v 6.38 and processed using MATLAB. In MATLAB, hematite images pixel values were converted into double data type and then the negative images were obtained by subtracting the scaled pixel values to 1. After this step, the background became light gray and the particles on the surface appeared as black dots. A mask was applied to remove most of the particles (black dots) in the images. The mask assigned the average background pixel value to all the pixels with a value higher than 0.89 except in five specific locations in the images (those corresponding to the particles of interest). Calcite images were also processed in MATLAB and their pixel values were converted into double data type. In this case, instead of obtaining the negative image, the background features were highlighted by using a mask. This mask assigned a value of 0 to all the pixels that had values higher than a number in the range from 0.75 to 0.9. The selection of a value within this range depended on the overall pixel values in the image: images with lower pixel values required a lower value for the mask and images with higher pixel values required a higher value. After this, the processed images were exported in TIFF format and superimposed using the background features as guides in PowerPoint. The DNA molecules in the images (highlighted as black dots) were tracked throughout the images and their area of movement was colored green.
2.4 Bacterial strains, culture conditions and competent cell preparation
Acinetobacter baylyi BD413 was kindly provided by Klaus Harm, University of Tromsø, Norway and carries the trpE27 mutation making the strain auxotrophic for tryptophan. TrpE codes for anthranilate synthase subunit I which a part of the tryptophan biosynthesis pathway. G to A transition at the 883rd position of the 1,494 bp long trpE ORF results in an amino acid change (E295K) making the gene product nonfunctional (Overballe-Petersen et al., 2013). To prepare competent cells, fresh overnight culture was diluted 1:100 in LB and incubated at 30°C under shaking at 180 rpm till an O.D of 0.3 was obtained (~4 h), corresponding to ~107 cells. The cells were centrifuged at 5000 rpm at 4°C for 10 min and resuspended in prechilled LB containing 20% glycerol, aliquoted and stored at −80°C until further use.
2.5 Horizontal gene transfer experiments in the presence of mineral-DNA complexes
The experimental strategy and protocols used for DNA adsorption and horizontal gene transfer experiments are depicted in Figure 1. For HGT experiments, the DNA used was a 60 bp double stranded DNA fragment with the sequence (3’CAATACCATCTTCCAAGCGTGACAGGATTTCGGGTGATGAGCCAACAATGTGAAAAGGCG5’.
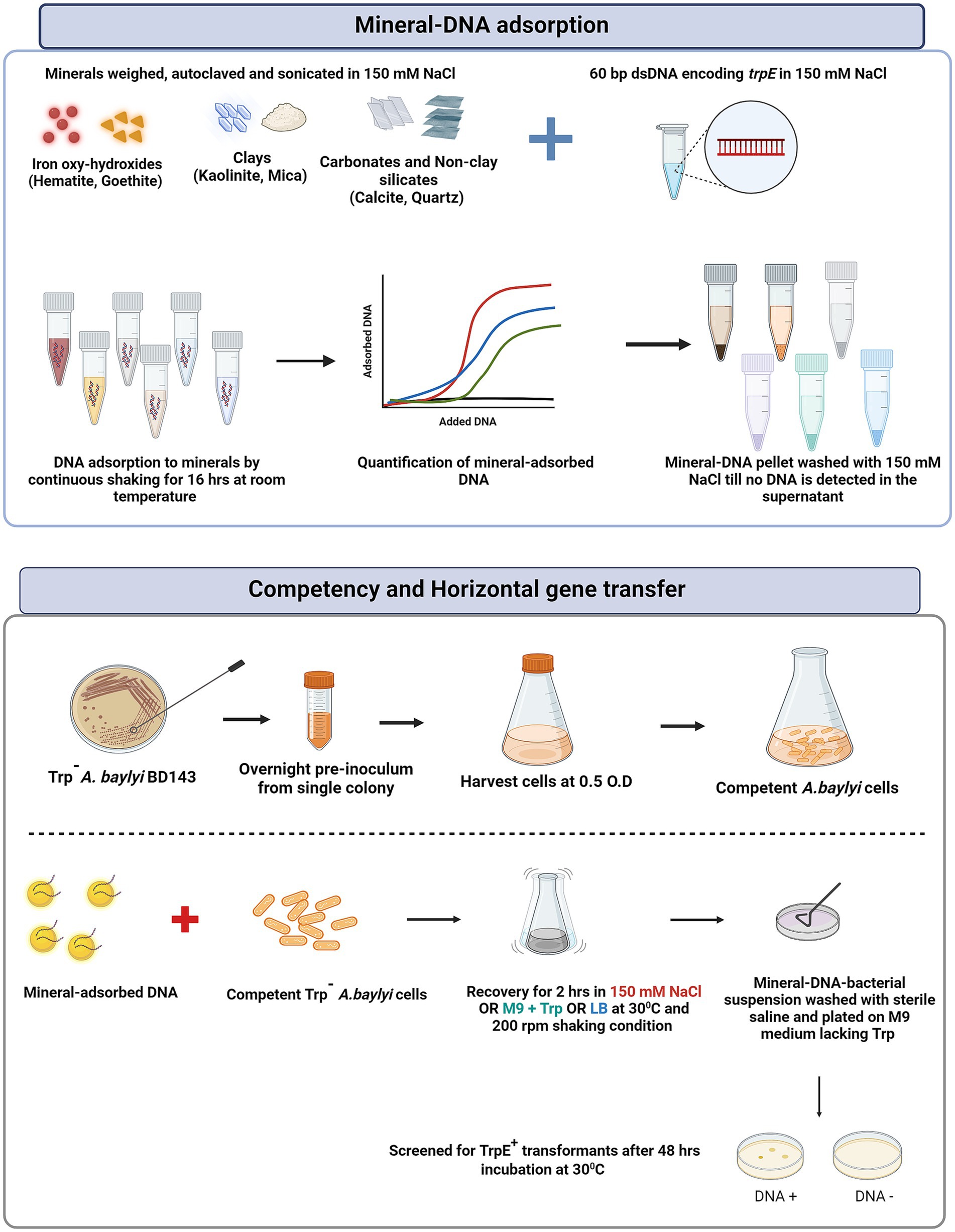
Figure 1. Schematic outlining the experimental strategy used for preparation of mineral-DNA complexes for adsorption as well as competent cell preparation and mineral-facilitated horizontal gene transfer.
5’GTTATGGTAGAAGGTTCGCACTGTCCTAAAGCCCACTACTCGGTTGTTACACTTTTCCGC-3′) procured from Integrated DNA Technologies (IDT, Belgium). Autoclaved and sonicated minerals were mixed with the appropriate concentration of DNA (as derived from the adsorption isotherm data (see Supplementary Table S1.2; Figure 2).
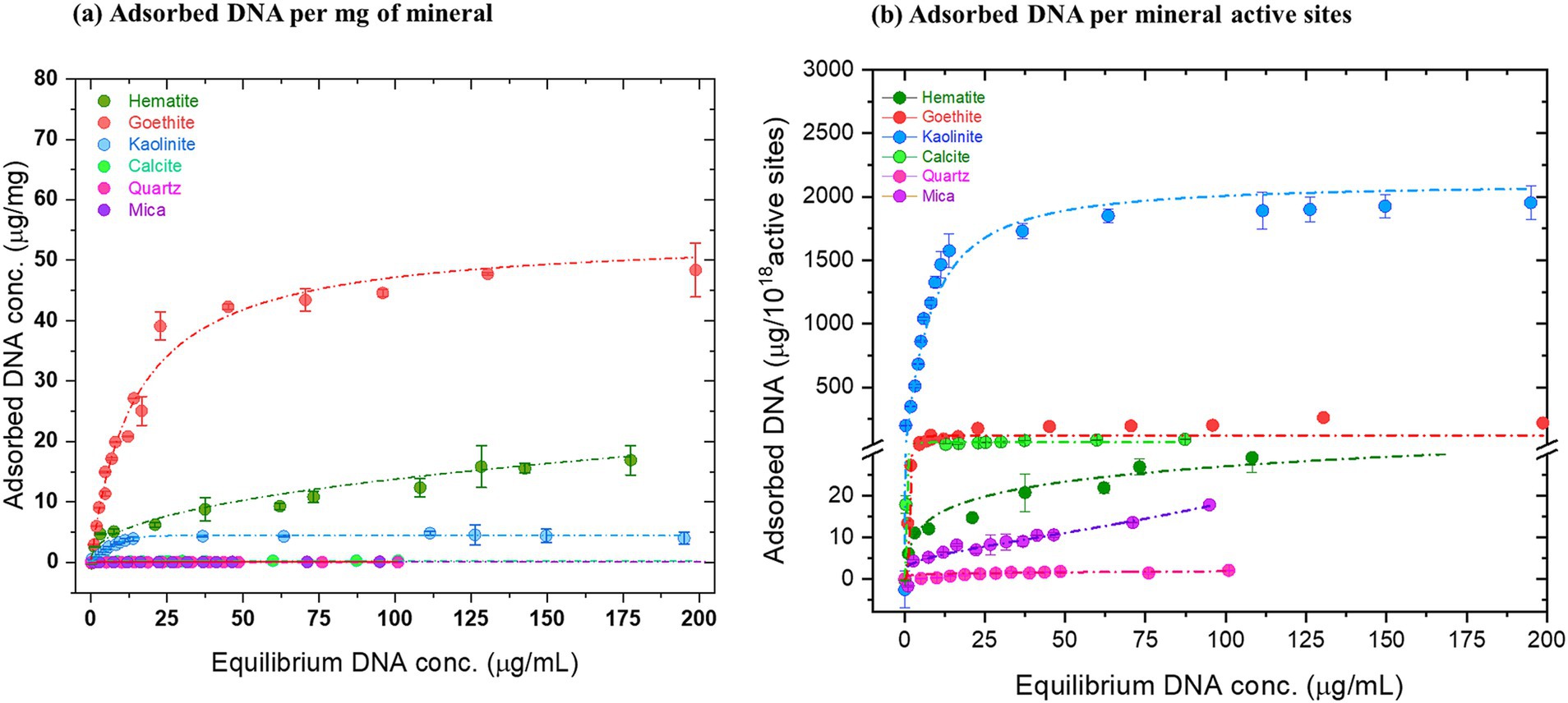
Figure 2. Equilibrium adsorption of salmon sperm DNA in saline under physiological conditions. Serially increasing concentrations of salmon sperm DNA (0–200 μg/mL) were mixed with sonicated mineral suspensions in 150 mM NaCl at pH 7. After incubation for 16 h, the amount of adsorbed DNA was estimated using absorbance at 260 nm for the indicated minerals. (a) μg of DNA adsorbed per milligram of mineral and (b) μg of DNA adsorbed per 1018 active sites present on each mineral. Langmuir or Freundlich fitting was performed to generate the adsorption isotherms for each DNA-mineral pair and are indicated by the dashed lines. (Langmuir fitting: Goethite, kaolinite, calcite, mica; Freundlich fitting: Hematite and quartz). The data are representative of three experiments and are plotted as mean ± S.D.
We aimed to add a concentration of DNA to the transformation experiments where the mineral was not fully saturated with DNA and the adsorption isotherms were used as guides to select the DNA:mineral ratio applied in the transformation experiments. The initial DNA concentration was chosen such that the DNA adsorbed by the positively charged minerals (hematite, goethite, kaolinite and calcite) was ~10 ng/μL whereas for the negatively charged minerals (mica and quartz), it was ~1 ng/μL. The mineral-DNA suspensions were shaken for 16 h at room temperature at 70 rpm and centrifuged at 5000 rpm for 30 min. The residues were washed with 1 mL sterile saline until no free DNA was detected in the supernatant (estimated by measuring absorbance at 260 nm.). For all the minerals, two washings were sufficient to obtain supernatant absorbance readings of 0 ng/μL, indicating negligible unbound DNA. The mineral-DNA complexes were then used for transformation experiments. For horizontal gene transfer experiments, competent cells were thawed on ice and washed once with sterile saline. The cells were then added to the mineral-DNA complex, resuspended in 150 mM NaCl or M9 supplemented with tryptophan or LB and incubated for 2 h at 30°C under shaking at 100 rpm. Post incubation, the mineral-DNA-bacteria complexes were washed once and resuspended in 300 μL of sterile saline. The cells were plated on M9 agar containing no tryptophan and incubated at 30°C for 48 h to screen for transformants. As a control experiment, A. baylyi cells were exposed to the minerals in the absence of DNA and plated both on M9 supplemented with tryptophan as well as without tryptophan. The transformation efficiency of trpE+ DNA into the parent A. baylyi strain lacking trpE (referred to as trpE−) was estimated by screening the transformants on M9 agar plates lacking exogenous tryptophan. Cells incubated with 10 ng/μL suspended DNA served as the positive control.
The transformation efficiency per μg DNA was calculated as follows:
2.6 Statistical analysis
All experiments were performed in triplicates and are plotted as mean ± S.D. Statistical analysis was performed using two-way ANOVA using GraphPad Prism 8 where *represents p < 0.05; **p < 0.01; ***p < 0.001 and ****p < 0.0001.
3 Results and discussion
3.1 Uptake by transformation
3.1.1 DNA adsorption
The various minerals used in our study, along with the experimental strategy, are depicted in Figure 1. All minerals used in this study display a range of different crystal faces, surface chemistries, surface composition and active site densities (nm−1; Supplementary Table S1.1). All these parameters give rise to differences in surface charge, adsorption behavior and adsorption parameters where the main parameters are the density of active sites and the surface charge. Figure 2 shows the distinct adsorption capacities between the minerals for 60 bp DNA fragments encoding trpE in 150 mM NaCl solution. Plotted as μg DNA /mg mineral, goethite showed the highest maximum adsorption capacity (~45 μg/mg), followed by hematite (~10 μg/mg) and kaolinite (~5 μg/mg; Figure 2A). Calcite, mica, and quartz adsorbed the lowest amount of DNA per mg of mineral (0.1–0.2 μg/mg; Refer to supporting information for details on isotherm models).
We also plotted the adsorbed DNA as a function of number of mineral surface active sites and found that when represented as μg DNA/ 1018 active sites, kaolinite showed the highest adsorption capacity followed by goethite, calcite, hematite, mica and quartz (Figure 2B). Plotted as μg DNA/ surface area, the order is: goethite, kaolinite, calcite, hematite, mica and quartz (Supplementary Figure S1.3). The mass of mineral (mg), mineral surface area (m2), DNA concentration (μg/mL), amount of DNA adsorbed (μg/mg) and the derived adsorption parameters in the experiments are listed in Supplementary Table S1.2.
3.1.2 DNA uptake by transformation
For all the nutrient conditions, the transformation efficiency of A. baylyi ranged from 0.1 to 4 cells/μg DNA and was strongly dependent on the mineral type (Figure 3). Bacteria exposed to the negatively charged minerals, quartz, and mica, which had the lowest DNA adsorption capacity, displayed the highest DNA uptake efficiency. The positively charged hematite, which had the highest number of active sites used in the HGT experiments, displayed the lowest transformation efficiency, followed by calcite, goethite and kaolinite (positively charged edge sites and negatively charged basal planes).
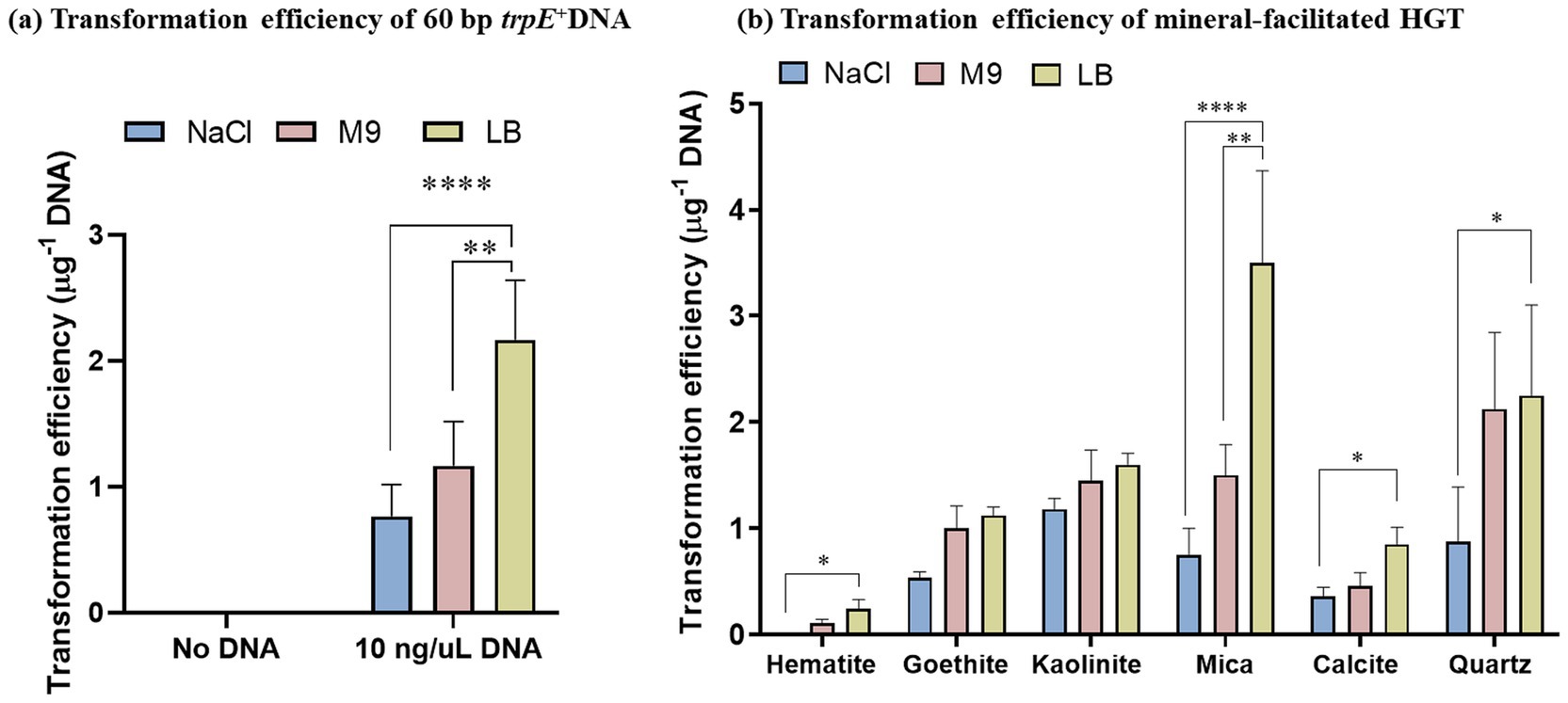
Figure 3. Acinetobacter baylyi is transformed by mineral-adsorbed DNA under nutritionally poor and rich conditions. Competent trpE− A. baylyi cells were exposed to 60 bp trpE+ DNA fragments adsorbed to different minerals as indicated. Recovery of the cells was performed in either saline (150 mM NaCl), M9 supplemented with tryptophan or LB medium for 2 h. The transformants were screened using M9 lacking tryptophan and reported as transformation efficiency per μg DNA (number of transformants recovered/μg DNA) obtained using (a) Free DNA and (b) Mineral-adsorbed DNA. The data are representative of three biological replicates and are plotted as mean ± S.D. Statistical analysis was performed using two-way ANOVA.
The transformation efficiencies were generally lowest when cells were grown in saline and highest for M9 and LB media, demonstrating that nutritionally rich conditions enhance DNA uptake owing to more metabolic activity of the cells and more energy allocated for DNA uptake and cell divisions. Moreover, rapidly growing cells have chromosomes with multiple replication forks, offering a high probability and faster uptake frequency of integration of DNA fragments in the bacterial genome (Jaramillo-Riveri et al., 2022).
To determine if there were any deleterious effects of the minerals which could influence the transformation efficiency, we assessed the cell viability of trpE− cells exposed to the minerals. With the exception of goethite and hematite, no significant reduction in the cell viability was observed for the minerals (Supplementary Figure S2.1). The growth pattern was consistent when the cells were cultured in either saline (150 mM NaCl), M9 supplemented with tryptophan or LB. Growth was observed only when trpE−A.baylyi was plated on M9 agar supplemented with tryptophan, owing to its absolute requirement for tryptophan.
We compared the transformation efficiency per μg of adsorbed DNA with the reported active site density of each mineral (Supplementary Table S1.1). Mica and quartz, both negatively charged, exhibited the highest transformation efficiencies, with values of 3.5 and 2.25, respectively. Kaolinite followed with an efficiency of 1.6, while goethite and calcite showed efficiencies of 1.1 and 0.8, respectively. Hematite displayed the lowest transformation efficiency, at 0.2. (Supplementary Figure S2.2).
It is well documented that transformation frequency is also dependent on the length of the DNA fragment and reduces as the length of the DNA shortens. Using free DNA, it was shown by Overballe-Petersen et al. (2013), that the shortest DNA fragment capable of transforming bacteria is 20 bp and that the frequency would increase with higher fragment lengths from a length of 500 bp. The transformation frequency was shown to be similar for free DNA fragments ranging from 20 bp to 120 bp (Overballe-Petersen et al., 2013). Previous reports also demonstrate that transformation frequencies of Bacillus subtilis, Pseudomonas stutzeri and Acinetobacter calcoaceticus declined with decreasing molecular size of DNA (Morrison and Guild, 1973; Carlson et al., 1983). The transformation efficiency of B. subtilis by sand-adsorbed chromosomal DNA was reported to be 50 times higher when compared to free DNA (Lorenz et al., 1988). Other soil bacteria such as P. stutzeri and A. calcoaceticus showed similar transformation frequencies irrespective of whether the chromosomal DNA was adsorbed to sand or whether it was freely suspended (Lorenz and Wackernagel, 1990; Chamier et al., 1993). In line with the results of Overballe-Petersen et al. (2013), our measured uptake efficiency of the 60 bp fragments (0.1–4 cells/μg DNA) is low relative to reported rates of mineral adsorbed plasmids and whole genomes.
3.2 Importance of interfacial geochemistry for uptake frequency
In general, a direct interaction between a positively charged mineral surface site and a negative phosphate group on the DNA backbone would facilitate a stronger bond than an association to a negatively charged surface site—mediated by the Na+ ion as in our case. However, DNA is a large molecule and it has more than one binding site to the mineral surface. The active sites on a mineral surface provide a measure for the density of binding sites and a high site density could lead to a high number of bonds to a DNA molecule. Combined, a high site density, favorable electrostatic interactions and a high surface area would lead to a relatively high adsorption capacity and a DNA molecule immobilized and strongly bonded to the surface through numerous bonds. The active sites present on the total surface area (0.025–0.63*1018 sites per 0.06–0.1 m2) used for HGT experiments are listed in Supplementary Table S1.1. Hematite has the highest number of total active sites, followed by quartz. Goethite and calcite have the same number of active sites followed by mica. Kaolinite has the least number of active sites per nm2 of the mineral. While both hematite (0.64 E18) and quartz (0.5 E18) show a similar number of active sites, in the chemical conditions used in our study, the surface charge for hematite is positive and negative for quartz which will influence the binding strength and adsorption capacity (Carlson and Kawatra, 2013; Kosmulski, 2020). Mineral surface charge is important for adsorption dynamics and capacity, but because of the heterogeneity of mineral surface topographic features and site heterogeneity, bulk measures, such as point of zero charge, do not provide the necessary detail to describe how adsorption affinities between mineral and molecules relate to uptake efficiency. Atomic force microscopy provides information on surface features to which DNA associates and fast scanning in liquid gives insight into the mobility of the adsorbed DNA. The mobility is a qualitative measure of the strength of biomolecule bonding to a surface and the availability of adsorption sites.
3.3 Mineral surface characteristics as drivers for uptake efficiency
We imaged a (001) mica, (001) hematite and (104) calcite, in 150 mM NaCl solution. Mica is atomically flat and has a permanent negative charge. The hematite (001) is the most stable hematite face in nature and is used here to represent a general trend for DNA adsorption on iron oxides terraces. The calcite surfaces display charge-dense step edges and terraces that are overall positively charged.
The negatively charged mica surface did not provide a charge landscape in the 150 mM NaCl solution to facilitate a bond strong enough to retain the negatively charged DNA backbone while scanning in liquid. Drying the sample and scanning in ambient air did reveal adsorbed molecules, highlighting that the DNA and the surface had formed a weak association. At the hematite surface, the DNA was readily and stably attached during liquid scanning (Figure 4; Supplementary Figures S3.1, S3.2), likely owing to its positive surface charge and high density of active sites. A stack of sequential frames shows no-to-minor movement of adsorbed strands (Supplementary Figure S3.1a). At the calcite surface most of the DNA was associated with the step edge whereas a few DNA molecules were observed on the negatively charged calcite terraces. The DNA adsorbed to the step edges did not move during imaging in liquid conditions, whereas some of the DNA adsorbed to the terraces moved during imaging. A stack of sequential frames shows minor movement of a few selected adsorbed strands (Supplementary Figure S3.1b). with the variation of defect types on a calcite terrace. In light of how the DNA-mineral association influences mobility of the adsorbed DNA, we find the amount of surface binding sites and the bond strength could explain the observed differences in the uptake efficiencies between positively and negatively charged surfaces (more details in the supporting information).
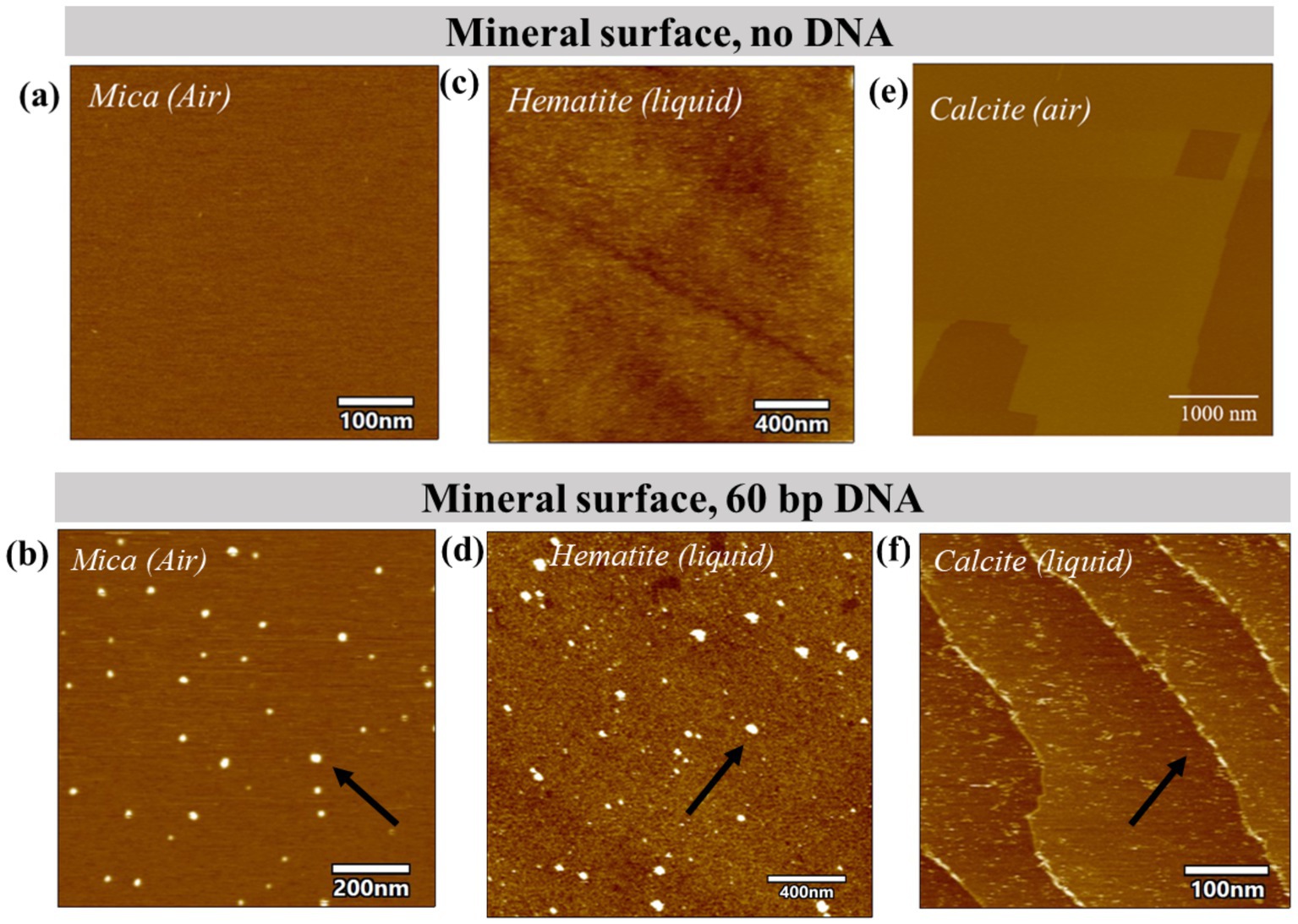
Figure 4. Atomic Force imaging in liquid shows DNA conformation on the mineral surfaces with varying charges. 60 bp DNA fragments were allowed to bind to mica (a,b); hematite crystal (c,d) and calcite crystal (e,f) in the presence of 150 mM NaCl at pH 7 and imaged in liquid or air conditions. (Air: Mica; Liquid: Hematite, calcite). Black arrows indicate DNA.
A previous study deriving adhesion forces between a phosphate group and a calcite, and a mica surface does show that calcite bonds to the phosphate group with significantly higher forces than to mica (15 pN vs. 1 pN in 100 mM NaCl; Freeman et al., 2023). In that study, the binding force represents an average across terraces and edge sites. Further, Gibbs free energy of binding between phosphate and hematite and mica also confirm that phosphate bonds stronger to Hematite than to mica (5.6 +/− 0.9 kt vs. 4.0 +/− 0.4 kT; Jelavić et al., 2017).
For studies of plasmid and chromosomal uptake from minerals into B.subtilis, differences in uptake efficiencies between minerals have also been reported. Competence in B. subtilis rely on signaling molecules and it was suggested that mineral adsorption of signaling molecules could decrease competency and hence transformation efficiency (Huang et al., 2021; Huang et al., 2023). Another potential mechanism explaining the altered transformation rates between minerals is the microbial association with the mineral surface. Strong microbe-mineral interactions have been shown to cause membrane damage (Otinov et al., 2020; Huang et al., 2021), leading to both altered expression of competency genes, and / or reduction of motility (Huang et al., 2023). We did observe a slight reduction in viability when A. baylyi were exposed to goethite and hematite, which may also contribute to the low cell numbers here compared to the other minerals (Supplementary Figure S2.1). Overall, the transformation frequencies are in line with interfacial geochemical principles and the limiting factors affecting bacterial DNA uptake from minerals is the DNA—mineral, bacteria-mineral or molecule-mineral associations.
3.4 Significance and scenarios of implications for evolution of bacterial life
For long-term evolutionary outcomes we do find it reasonable to assume that each time a cell encounters short and degraded DNA there is a probability that the cell is transformed. First, there is a large amount of fragmented DNA present in the environment (Dell’Anno and Danovaro, 2005; Overballe-Petersen and Willerslev, 2014), second, the incorporation mechanism in the bacterial genome is simple and third, the universality of the mechanism behind the uptake of short and fragmented DNA means DNA integration can, in essence, occur in any cell.
The broad range of settings in which mineral-facilitated HGT can occur may connect organisms through time and space in ways that remain unexplored. Bacteria that colonize deposits with historic DNA-mineral complexes can acquire old traits. Also, DNA molecules propagated to distant environments on mineral surfaces may be acquired by organisms far from the DNA-mineral complex where the transported DNA serves as a distal source for new innovations. The remaining question is how microbial evolution is affected by this vast extension of the known temporal and spatial boundaries within which horizontal gene transfer can contribute and how these processes may work and affect natural selection.
Natural selection is a process of adaptation. Adaptation to a novel environment requires heritable variation for selection to act on. Competition and the struggle for existence between individuals will entail that the fittest individuals win the struggle for existence as proposed by Darwin. Since Darwin’s proposal, stochastic mutations that are heritable to offspring are clearly one demonstrated source for variation of the genome. Additionally, horizontal gene transfer is recognized as an important mechanism for the acquisition of beneficial complex traits, and when DNA molecules from one prokaryotic species are taken up by another prokaryote and then inherited by daughter cells, this represents heritable variation. If the genetic material confers a trait and increases the fitness for the offspring, this trait could be selected for. The potential importance of HGT through DNA-mineral complexes lies not only in the movement of traits through time and space. A geological or physical disturbance that creates a novel environment could simultaneously introduce DNA of organisms adapted to those conditions. This proposed mechanism to provide genetic variation differs from where stochastic mutations are invoked. First, the case of mineral-facilitated HGT could influence variation in a non-random fashion since the DNA molecules are specific to the environment. When there is a geological or physical disturbance such as a landslide, a flooding event, or even a new meander on a river, there would be an increased interface between previously established populations and DNA-mineral complexes. Second, the variation and the requirement for adaptation can be linked, in that the process that provides changing conditions that lead to organismal stress and new needs also provides the external DNA molecules. The new needs may arise from the shift in, for example population dynamics (e.g., altered patterns of prey, niches, or friends) or environmental chemistry (e.g., altered localization from oxygen, sulfide, and light). These needs lead to a struggle for existence and, if coupled to new genetic material (via DNA-mineral complexes) conferring a trait that is selected for (increased fitness for the offspring), propelling evolution by natural selection.
The way this proposed mechanism couples stress with increased opportunity is similar to how mutagenesis in cells (prokaryotic, eukaryotic, and cancer cells) can be upregulated in a non-random way by stress (McKenzie et al., 2000). For example, stressors such as starvation or drugs induce a DNA-break repair mechanism that is mutagenic. This stress-induced mutagenesis may increase heritable variation and therefore aid genomic solutions (traits) that increase fitness for the offspring of, e.g., E. coli. In these cases, the mechanism propels evolution in response to new needs via the acquisition of novel and heritable variation; similar to that of mineral-facilitated HGT. Although the coupling of a source for heritable variation (the DNA-mineral complex) and struggle for existence (e.g., as derived by a geological disturbance) as an evolutionary driver is yet less understood as opposed to stochastic mutations, that of mineral-facilitated HGT is not the first suggestion of such mechanism. Consequently, the geological setting can induce and remodel the struggle for existence in a way that by-passes the step of random mutations for increasing fitness. In this scenario, a geologically provided sedimentary and environmental setting for preserving DNA would enhance the adaptive potential of organisms that utilize this route for increased variation.
4 Conclusion
We demonstrate that mineral-adsorbed 60 bp trpE+ DNA can transform an auxotrophic A. baylyi strain with an absolute requirement for exogenous tryptophan into a prototrophic strain that can synthesize tryptophan endogenously. We find that the mineral surface properties have an influence on the uptake frequency such that minerals with a high number of active sites and favorable electrostatics for adsorption show a lower rate of transfer. Considering the ease of incorporation into a genome and the large amounts of DNA associated with sediments, we find that the mixing of modern microbes and ancient DNA in principle represents a pathway for gene transfer, across a scale of time and space, unrivaled by any other avenues. We argue that mineral-facilitated HGT provides a mechanism for rapid adaptations, and we argue that it would provide an efficient path to fitness when there is an abrupt change in the environmental conditions or other evolutionary drivers associated with a shift in the provenance of incoming sediments. Essentially, our results introduce interfacial geochemical and sedimentologic processes as facilitators of evolutionary innovations. Mineral-facilitated HGT could also lead to evolutionary implications that are not yet considered. If the mechanism offers new genomic material at times with increased needs or struggle for existence, it can represent a way to transiently propel evolution in a non-stochastic manner.
Data availability statement
The raw data supporting the conclusions of this article will be made available by the authors, without undue reservation.
Author contributions
TV: Conceptualization, Data curation, Formal analysis, Investigation, Methodology, Validation, Visualization, Writing – original draft, Writing – review & editing. SH: Data curation, Methodology, Writing – review & editing. CC: Software, Visualization, Writing – review & editing. SA: Writing – review & editing, Writing – original draft. EH: Writing – review & editing, Writing – original draft. MB: Conceptualization, Supervision, Writing – review & editing, Writing – original draft. KS: Conceptualization, Data curation, Formal analysis, Funding acquisition, Investigation, Methodology, Project administration, Resources, Software, Supervision, Validation, Visualization, Writing – original draft, Writing – review & editing.
Funding
The author(s) declare that financial support was received for the research, authorship, and/or publication of this article. This work was supported by a research grant from VILLUM FONDEN grant 00025352.
Acknowledgments
We would like to acknowledge Søren Overballe-Petersen for discussions on A. baylyi transformation experiments. We would also like to thank Pablo Arellano Caicedo for the goethite FTIR spectrum. We would like to sincerely thank all past and current members of the Molecular Geobiology group for directly or indirectly helping in paving the way for this work.
Conflict of interest
The authors declare that the research was conducted in the absence of any commercial or financial relationships that could be construed as a potential conflict of interest.
The author(s) declared that they were an editorial board member of Frontiers, at the time of submission. This had no impact on the peer review process and the final decision.
Publisher’s note
All claims expressed in this article are solely those of the authors and do not necessarily represent those of their affiliated organizations, or those of the publisher, the editors and the reviewers. Any product that may be evaluated in this article, or claim that may be made by its manufacturer, is not guaranteed or endorsed by the publisher.
Supplementary material
The Supplementary material for this article can be found online at: https://www.frontiersin.org/articles/10.3389/fmicb.2024.1449094/full#supplementary-material
References
Armbrecht, L., Herrando-Pérez, S., Eisenhofer, R., Hallegraeff, G. M., Bolch, C. J. S., and Cooper, A. (2020). An optimized method for the extraction of ancient eukaryote DNA from marine sediments. Mol. Ecol. Resour. 20, 906–919. doi: 10.1111/1755-0998.13162
Belova, D. A., Lakshtanov, L. Z., Carneiro, J. F., and Stipp, S. L. S. (2014). Nickel adsorption on chalk and calcite. J. Contam. Hydrol. 170, 1–9. doi: 10.1016/j.jconhyd.2014.09.007
Carlson, J. J., and Kawatra, S. K. (2013). Factors affecting zeta potential of Iron oxides. Miner. Process. Extr. Metall. Rev. 34, 269–303. doi: 10.1080/08827508.2011.604697
Carlson, C. A., Pierson, L. S., Rosen, J. J., and Ingraham, J. L. (1983). Pseudomonas stutzeri and related species undergo natural transformation. J. Bacteriol. 153, 93–99. doi: 10.1128/jb.153.1.93-99.1983
Chamier, B., Lorenz, M. G., and Wackernagel, W. (1993). Natural transformation of Acinetobacter calcoaceticus by plasmid DNA adsorbed on Sand and groundwater aquifer material. Appl. Environ. Microbiol. 59, 1662–1667. doi: 10.1128/aem.59.5.1662-1667.1993
Dejean, T., Valentini, A., Duparc, A., Pellier-Cuit, S., Pompanon, F., Taberlet, P., et al. (2011). Persistence of environmental DNA in freshwater ecosystems. PLoS One 6:e23398. doi: 10.1371/journal.pone.0023398
Dell'Anno, A., and Danovaro, R. (2005). Extracellular DNA plays a key role in deep-sea ecosystem functioning. Science 309:2179. doi: 10.1126/science.1117475
Flemming, H.-C., and Wuertz, S. (2019). Bacteria and archaea on earth and their abundance in biofilms. Nat. Rev. Microbiol. 17, 247–260. doi: 10.1038/s41579-019-0158-9
Freeman, C. L., Dieudonné, L., Agbaje, O. B. A., Zure, M., Sanz, J. Q., Collins, M., et al. (2023). Survival of environmental DNA in sediments: Mineralogic control on DNA taphonomy. Environmental DNA 5:1691–1705. doi: 10.1002/edn3.482
Freeman, C. L., and Harding, J. H. (2023). The transformation of amorphous calcium carbonate to calcite and classical nucleation theory. J. Cryst. Growth 603:126978. doi: 10.1016/j.jcrysgro.2022.126978
Hall, R. J., Whelan, F. J., McInerney, J. O., Ou, Y., and Domingo-Sananes, M. R. (2020). Horizontal gene transfer as a source of conflict and cooperation in prokaryotes. Front. Microbiol. 11:1569. doi: 10.3389/fmicb.2020.01569
Heuer, H., and Smalla, K. (2012). Plasmids foster diversification and adaptation of bacterial populations in soil. FEMS Microbiol. Rev. 36, 1083–1104. doi: 10.1111/j.1574-6976.2012.00337.x
Huang, Q., Chen, J., Zhu, J., Hao, X., Dao, G., Chen, W., et al. (2021). Divergent bacterial transformation exerted by soil minerals. Sci. Total Environ. 784:147173. doi: 10.1016/j.scitotenv.2021.147173
Huang, Q., Zhu, J., Qu, C., Wang, Y., Hao, X., Chen, W., et al. (2023). Dichotomous role of humic substances in modulating transformation of antibiotic resistance genes in mineral systems. Environ. Sci. Technol. 57, 790–800. doi: 10.1021/acs.est.2c06410
Hunting, E. R. (2013). UV radiation and organic matter composition shape bacterial functional diversity in sediments. Front. Microbiol. 4:317. doi: 10.3389/fmicb.2013.00317
Jain, R., Rivera, M. C., Moore, J. E., and Lake, J. A. (2003). Horizontal gene transfer accelerates genome innovation and evolution. Mol. Biol. Evol. 20, 1598–1602. doi: 10.1093/molbev/msg154
Jaramillo-Riveri, S., Broughton, J., McVey, A., Pilizota, T., Scott, M., and El Karoui, M. (2022). Growth-dependent heterogeneity in the DNA damage response in Escherichia coli. Mol. Syst. Biol. 18:e10441. doi: 10.15252/msb.202110441
Jelavić, S., Tobler, D. J., Hassenkam, T., De Yoreo, J. J., Stipp, S. L. S., and Sand, K. K. (2017). Prebiotic RNA polymerisation: energetics of nucleotide adsorption and polymerisation on clay mineral surfaces. Chem. Commun. 53, 12700–12703. doi: 10.1039/C7CC04276K
Keeling, P. J., and Palmer, J. D. (2008). Horizontal gene transfer in eukaryotic evolution. Nat. Rev. Genet. 9, 605–618. doi: 10.1038/nrg2386
Khanna, M., and Stotzky, G. (1992). Transformation of Bacillus subtilis by DNA bound on montmorillonite and effect of DNase on the transforming ability of bound DNA. Appl. Environ. Microbiol. 58, 1930–1939. doi: 10.1128/aem.58.6.1930-1939.1992
Kjær, K. H., Winther Pedersen, M., De Sanctis, B., De Cahsan, B., Korneliussen, T. S., Michelsen, C. S., et al. (2022). A 2-million-year-old ecosystem in Greenland uncovered by environmental DNA. Nature 612, 283–291. doi: 10.1038/s41586-022-05453-y
Kosmulski, M. (2020). Surface charging and points of zero charge. Boca Raton: CRC Press, Taylor and Francis Group.
Lorenz, M. G., Aardema, B. W., and Wackernagel, W. (1988). Highly efficient genetic transformation of Bacillus subtilis attached to sand grains. J. Gen. Microbiol. 134, 107–112. doi: 10.1099/00221287-134-1-107
Lorenz, M. G., and Wackernagel, W. (1990). Natural genetic transformation of Pseudomonas stutzeri by sand-adsorbed DNA. Arch. Microbiol. 154, 380–385. doi: 10.1007/bf00276535
Martina, M. R., Zoli, L., and Sani, E. (2022). Synthesis and characterization of goethite (α-FeOOH) magnetic nanofluids. Int. J. Thermofluids 15:100169. doi: 10.1016/j.ijft.2022.100169
McKenzie, G. J., Harris, R. S., Lee, P. L., and Rosenberg, S. M. (2000). The SOS response regulates adaptive mutation. Proc. Natl. Acad. Sci. 97, 6646–6651. doi: 10.1073/pnas.120161797
Morono, Y., Ito, M., Hoshino, T., Terada, T., Hori, T., Ikehara, M., et al. (2020). Aerobic microbial life persists in oxic marine sediment as old as 101.5 million years. Nat. Commun. 11:3626. doi: 10.1038/s41467-020-17330-1
Morrison, D. A., and Guild, W. R. (1973). Breakage prior to entry of donor DNA in pneumococcus transformation. Biochim. Biophys. Acta 299, 545–556. doi: 10.1016/0005-2787(73)90226-8
Ochman, H., Lawrence, J. G., and Groisman, E. A. (2000). Lateral gene transfer and the nature of bacterial innovation. Nature 405, 299–304. doi: 10.1038/35012500
Otinov, G. D., Lokteva, A. V., Petrova, A. D., Zinchenko, I. V., Isaeva, M. V., Kovtunov, E. A., et al. (2020). Positive and negative effects of metal oxide nanoparticles on antibiotic resistance genes transfer. Antibiotics (Basel) 9:742. doi: 10.3390/antibiotics9110742
Overballe-Petersen, S., Harms, K., Orlando, L. A., Mayar, J. V., Rasmussen, S., Dahl, T. W., et al. (2013). Bacterial natural transformation by highly fragmented and damaged DNA. Proc. Natl. Acad. Sci. USA 110, 19860–19865. doi: 10.1073/pnas.1315278110
Overballe-Petersen, S., and Willerslev, E. (2014). Horizontal transfer of short and degraded DNA has evolutionary implications for microbes and eukaryotic sexual reproduction. BioEssays 36, 1005–1010. doi: 10.1002/bies.201400035
Pääbo, S. (1989). Ancient DNA: extraction, characterization, molecular cloning, and enzymatic amplification. Proc. Natl. Acad. Sci. USA 86, 1939–1943. doi: 10.1073/pnas.86.6.1939
Sand, K. K., and Jelavić, S. (2018). Mineral facilitated horizontal gene transfer: a new principle for evolution of life? Front. Microbiol. 9:2217. doi: 10.3389/fmicb.2018.02217
Sirois, S. H., and Buckley, D. H. (2019). Factors governing extracellular DNA degradation dynamics in soil. Environ. Microbiol. Rep. 11, 173–184. doi: 10.1111/1758-2229.12725
Thomsen, P. F., Kielgast, J., Iversen, L. L., Møller, P. R., Rasmussen, M., and Willerslev, E. (2012a). Detection of a diverse marine fish fauna using environmental DNA from seawater samples. PLoS One 7:e41732. doi: 10.1371/journal.pone.0041732
Keywords: horizontal gene transfer, bacteria, extracellular DNA, minerals, evolution
Citation: Verma T, Hendiani S, Carbajo C, Andersen SB, Hammarlund EU, Burmølle M and Sand KK (2024) Recurrence and propagation of past functions through mineral facilitated horizontal gene transfer. Front. Microbiol. 15:1449094. doi: 10.3389/fmicb.2024.1449094
Edited by:
Stephen Allen Morse, IHRC, Inc., United StatesReviewed by:
Yunxue Guo, Chinese Academy of Sciences (CAS), ChinaYuki Morono, Japan Agency for Marine-Earth Science and Technology (JAMSTEC), Japan
Copyright © 2024 Verma, Hendiani, Carbajo, Andersen, Hammarlund, Burmølle and Sand. This is an open-access article distributed under the terms of the Creative Commons Attribution License (CC BY). The use, distribution or reproduction in other forums is permitted, provided the original author(s) and the copyright owner(s) are credited and that the original publication in this journal is cited, in accordance with accepted academic practice. No use, distribution or reproduction is permitted which does not comply with these terms.
*Correspondence: Karina K. Sand, a2tzQHN1bmQua3UuZGs=