- 1Institute for Energy and Nuclear Research, National Nuclear Energy Commission-IPEN/CNEN-SP, São Paulo, Brazil
- 2Department of Radiology, School of Medicine, University of Missouri, Columbia, MO, United States
- 3Federal University of São Paulo, Institute of Environmental, Chemical and Pharmaceutical Sciences, São Paulo, Brazil
Nanoparticles play a crucial role in the field of nanotechnology, offering different properties due to their surface area attributed to their small size. Among them, silver nanoparticles (AgNPs) have attracted significant attention due to their antimicrobial properties, with applications that date back from ancient medicinal practices to contemporary commercial products containing ions or silver nanoparticles. AgNPs possess broad-spectrum biocidal potential against bacteria, fungi, viruses, and Mycobacterium, in addition to exhibiting synergistic effects when combined with certain antibiotics. The mechanisms underlying its antimicrobial action include the generation of oxygen-reactive species, damage to DNA, rupture of bacterial cell membranes and inhibition of protein synthesis. Recent studies have highlighted the effectiveness of AgNPs against various clinically relevant bacterial strains through their potential to combat antibiotic-resistant pathogens. This review investigates the proteomic mechanisms by which AgNPs exert their antimicrobial effects, with a special focus on their activity against planktonic bacteria and in biofilms. Furthermore, it discusses the biomedical applications of AgNPs and their potential non-preparation of antibiotic formulations, also addressing the issue of resistance to antibiotics.
1 Introduction
Nanomaterials are structures with dimensions between 1 and 100 nanometers, encompassing the field of nanotechnology (Husain et al., 2023). They include nanostructures, nanocomposites, nanofibers, and nanofilms, and can be composed of polymers, biomaterials, ceramics, metals, among others. They encompass a variety of structures with different morphologies, such as rods, tubes, films, and particles. Nanoparticles, a subset of nanomaterials, are specifically characterized by their three-dimensional nanoscale size and generally have spherical or quasi-spherical shapes. Their unique properties, such as high reactivity and large surface area, make them particularly suitable for applications in drug delivery, imaging, and catalysis (Khan et al., 2019; Cheng et al., 2023).
Obtaining nanoparticles can be carried out by chemical, physical and biological methods, often involving high costs and the use of toxic substances. On the other hand, synthesis using natural substances, such as phytochemicals, has been widely discussed due to their reducing potential, biocompatibility and low environmental toxicity. Green nanotechnology, based on the principles of green chemistry, seeks economic, social, health and environmental benefits by prioritizing the reduction of the generation of hazardous chemical waste and promoting safer applications (Nasrollahzadeh et al., 2019; Brar et al., 2022).
Analytical techniques for the physicochemical characterization of nanoparticles are fundamental for the detailed investigation of their properties, including structure, crystallinity, morphology, size, and surface chemistry. UV–Visible spectroscopy is used for the primary characterization of colloidal suspension particles, confirming the formation of NPs by the intensity of the surface plasmon resonance (SPR) band. The Dynamic Light Scattering (DLS) technique allows the determination of the hydrodynamic particle size, influenced by Brownian motion as a function of the diffusion coefficient, analyzing fluctuations in the intensity of scattered light to determine particle diffusion and directly relate it to size. The relationship between the diffusion coefficient and size is variable for spherical particles, as the diffusion coefficient decreases as the hydrodynamic radius of the particles increases, as described by the Stokes-Einstein equation. Additionally, it provides the polydispersity index, indicating system homogeneity. Nanoparticle Tracking Analysis (NTA) individually tracks the movement of each particle, determining its size based on Brownian motion. The Zeta potential measures the surface charge and stability of colloidal suspensions, indicating the tendency of particles to aggregate or repel each other. Inductively Coupled Plasma Mass Spectrometry (ICP-MS) is used for multielemental investigation, quantifying silver uptake in different systems. X-ray Diffraction (XRD) provides information on the crystallinity level and molecular structure of nanoparticles. Fourier Transform Infrared Spectroscopy (FTIR) and Raman spectroscopy identify the surface chemical composition and investigate particle functionalization, vibrational, and rotational modes. X-ray Photoelectron Spectroscopy (XPS) analyzes the surface chemistry of NPs, while Energy Dispersive X-ray Spectroscopy (EDX) identifies and quantifies the elemental composition of materials. Nuclear Magnetic Resonance (NMR) spectroscopy is used to identify and quantify surface functional groups. Imaging techniques, such as Scanning Electron Microscopy (SEM) and Transmission Electron Microscopy (TEM), provide detailed images of particle morphology and size, allowing the creation of size distribution histograms through statistical analysis. Atomic Force Microscopy (AFM) allows for the analysis of aggregation state, dispersion, topography, structure, shape, and size of nanoparticles. Together, these techniques provide comprehensive data on the structural, chemical, and morphological properties of nanoparticles, enabling an in-depth investigation of their characteristics (Pryshchepa et al., 2020; Patil and Chougale, 2021; Joudeh and Linke, 2022).
Silver has a long history as an antimicrobial agent and has been used since ancient times, with documented evidence in several medicinal applications. In 1881, Credé’s method introduced the use of a solution containing 2% AgNO3 to treat neonatal conjunctivitis. In the 19th century, doctors used silver thread to suture surgical wounds, while during the First World War, silver foil was applied to soldiers’ wounds to prevent infection and aid wound healing. By the early 20th century, colloidal silver became popular in hospitals as a germicidal agent. Additionally, silver salts were used to treat various infections, such as conjunctivitis, gastroenteritis, gonorrhea and syphilis (Medici et al., 2019; Kaiser et al., 2023).
Silver nanoparticles (AgNPs) are widely described in the literature as having a broad biocidal spectrum, covering Gram-positive and Gram-negative bacteria, fungi, viruses and mycobacteria, in addition to acting synergistically with antibiotics to enhance their effectiveness (Misirli et al., 2021). These properties are based on diverse and complex mechanisms, including the generation of reactive oxygen species (ROS), DNA damage, impairment of bacterial cell membranes and inhibition of protein synthesis (Dakal et al., 2016; Salleh et al., 2020; Bruna et al., 2021).
Recent studies have highlighted the effectiveness of AgNPs against a variety of bacterial strains, including clinical isolates and standard strains (ATCCs, NCTCs, PCCs, MTCCs) of various species, such as Acinetobacter baumannii, Escherichia coli, Staphylococcus aureus, Staphylococcus epidermidis, Klebsiella pneumoniae, Pseudomonas aeruginosa, Pseudomonas fluorescens, Salmonella typhi, Enterococcus faecalis, Enterobacter spp., Bacillus subtilis, Micrococcus luteus, Proteus vulgari, among others (Shaik et al., 2018; Wang H. et al., 2021; Wang L. et al., 2021; Khane et al., 2022; Basheer et al., 2023; Kaiser et al., 2023). Furthermore, the synergy between AgNPs and antibiotics, such as penicillin G, amoxicillin, erythromycin, clindamycin, vancomycin, piperacillin, ciprofloxacin, tetracycline, levofloxacin, cefixime, cefazolin, neomycin, rifampicin, gentamicin, chloramphenicol, imipenem; has been shown to increase the effectiveness of these medications (Singh et al., 2013; Mazur et al., 2020; Aabed and Mohammed, 2021; Hassan et al., 2021; Haji et al., 2022).
This review addresses the mechanisms of action of AgNPs against planktonic and biofilm bacteria at the proteomic level, as well as their biomedical applications, aiming to enhance formulations and investigate synergism with antibiotics, highlighting advances relevant to nanomedicine and public health.
2 Biological interaction and compatibility of AgNPs
The diverse applications and the increase of AgNPs in the marketplace makes them inevitably present in the environment, as they are released not only during the use of products such as cosmetics, wound dressings, textiles, food packaging and personal care products, but also through their productive processes. This fact entails the ingestion of this NPs by humans by food or drinks that were in direct contact with these NPs or through contaminated by water containing these nanostructures, and their intake can also occur via dermal contact, inhalation or intravenous or intraperitoneal injections (Jaswal and Gupta, 2021; Zhang et al., 2022; Ren et al., 2023).
Once inside the body AgNPs are distributed through the organs and tissues, firstly accumulating in the lungs when entering via the respiratory tract. These nanoparticles can penetrate both healthy or injured skin when present in formulations for topical application such as cosmetics and wound dressings or even textiles and then enter circulatory system. Toxicity and pathological symptoms of AgNPs intake are modulated by some properties of these nanostructures, such as size, stability and aggregation condition. Zhang et al. (2022) summarized their toxic effects on different animal models, with different organs being affected.
Juling et al. (2016) administered commercial AgNPs (15–30 nm) coated with polyoxyethylene glycerol trioleate and Tween 20 (polyoxyethylene (20) sorbitan monolaurate) to rats via oral or intravenous injection and after 24 h measured their content on different tissues and feces. For the orally administered group, AgNPs accumulated in feces and colon, while intravenous administration resulted in a more distributed accumulation, being present mainly in lungs, then in feces, followed by the colon, intestine, kidney, testis and thymus, with an expected silver ions content below 15%. Juling et al. (2016) still also indicate the reduction in AgNPs size to 5 to 12 nm and the formation of silver acetate NPs of 6 nm in animals treated with silver ions (AgNO3).
Since the gastrointestinal tract is one of the main entry ways for many invaders, as pathogens and toxins, including AgNPs, Ren et al. (2023) evaluated the intestinal toxicity of these NPs in vivo. Mice were exposed daily for 4 weeks with 10 mL/kg body weight of commercial suspensions of AgNPs capped with poly(vinylpyrrolidone) of 5 mg/mL through oral gavage were compared to animals being treated with other NPs or water. The authors indicated severe damage for the animals treated with AgNPs including damage in the epithelia structure, reduction in the mucosa layer thickness and alterations in the local microbiota. Additionally, the interaction of immune cells with these NPs resulted in the generation of reactive oxygen species (ROS) that induced uncontrolled apoptosis of dendritic cells, affecting the normality of the immune microenvironment in the intestine of these animals. Among the tested NPs (Ag, TiO2, SiO2, and ZnO) silver was the most harmful.
The cytotoxicity of AgNPs is generally related to the production of large amounts of ROS, affecting cytoskeleton, DNA, DNA repair enzymes and inducing cell apoptosis. However, these effects are associated with size and the synthetic methods employed. Larger NPs present less cytotoxicity due to the reduction in surface area and, consequently, the reduction in interaction with cell membranes and organelles (Jaswal and Gupta, 2021; Zhang et al., 2022). Amr et al. (2023) biosynthesized AgNPs employing aqueous extract of a mushroom (Agaricus bisporus) and evaluated their cytotoxicity against human skin fibroblasts, obtaining cell viability of 98.2% for the diluted solutions (0.04 μg/mL) until 82.3% for 25.0 μg/mL samples. Besides the dose-dependent cytotoxicity, it is worth pointing out that the size of the NPs was 766 nm, and the use of safer aqueous extracts as reducing agents instead of commonly applied chemicals for this purpose. This approach eliminates purification steps to reduce the presence of these compounds that tend to increase cytotoxicity.
Yadav and Preet (2023) compared AgNPs prepared by chemical (sodium borohydride) and green (clove bud extract) methods of synthesis and observed a better larvicidal (Aedes aegypti) effect with lower toxicity (Daphnia magna) for the samples prepared with plant extract. Despite the recognized toxicity of AgNPs some approaches can reduce this drawback, such as the use of natural reducing and capping agents, the control of size and the use of these NPs in combination to other antimicrobial agents to attempt microbial control through different and complementary mechanisms, reducing the necessary concentration of AgNPs.
3 Applications of silver nanoparticles as antimicrobial agents
Nanomaterials are structures that exist on the nanometer scale (10-9). According to the American Society for Testing and Materials (ASTM), nanoparticles are a specific category of particles with lengths in two or more dimensions within the range of 1 to 100 nm (Loiseau et al., 2019).
The characteristics of these metallic nanoparticles depend on factors such as size, morphology, stability, surface charge, and other physical and chemical properties. These properties can be influenced through the kinetic interaction conditions between metal ions and reducing agents, by modifying synthesis methods, characteristics of the reducing agents, and the use of stabilizers. Additionally, various morphologies of AgNPs can be obtained, such as cubic shapes, spherical, nanorods, nanowires, nanobars, triangular and pyramidal, in a controlled manner by modifying thermodynamics and kinetics during synthesis, through factors such as the type of reducer (chemical or physical), use of solvent systems, variations in temperature, pH, pressure, and controlled atmosphere (Zhang et al., 2016; Burdușel et al., 2018).
The preference for biological methods in the synthesis of AgNPs has driven significant advances in the search for more ecological and efficient processes. This approach is based on the use of bacteria, plant extracts, and biomolecules as non-toxic reducing agents, offering a safe, environmentally friendly, and economically advantageous alternative for AgNPs production. Biological methods are distinguished by their safer and less harmful approach, supported by green nanotechnology stemming from ‘green chemistry’, aiming for beneficial innovations for health, the environment, and industry, ensuring efficiency and safety in meeting current needs with greater sustainability (Salleh et al., 2020; Bamal et al., 2021).
AgNPs can be used in different sectors, such as medicine, engineering, agriculture, and the environment sciences (Dawadi et al., 2021). Major applications include surface coating and medical device coating, advanced dressings, theranostics, cosmetics, textiles and electronics, sensors, and catalysts (Prabhu and Poulose, 2012). Their properties include antibacterial, antifungal, antiviral, anti-inflammatory, anti-angiogenic, and antitumor activities (Pryshchepa et al., 2020).
Bacterial resistance to antibiotics represents a global health challenge. The World Health Organization (WHO) warns that drug-resistant pathogens significantly contribute to high mortality rates (Bamal et al., 2021). Consequently, research and development of new antimicrobial agents have attracted considerable interest. Among the various known metallic nanoparticles, such as Au, Pd, Cu, and Zn, silver stands out as one of the most effective in combating pathogenic microorganisms. Studies often cite its broad-spectrum antimicrobial capacity due to its large surface area and multivalent interactions (Sánchez-López et al., 2020; Bruna et al., 2021).
Table 1 presents significant studies exploring the potential of AgNPs in various applications, such as antimicrobial, antifungal, and antiviral action. These studies address synthetic methods, morphology, size, and the different targets to which these nanoparticles are currently being applied.
The search for new antimicrobial agents has been driven by the increasing number of infections presenting bacterial resistance to conventional antibiotic treatment, a which poses significant challenge for global health. The indiscriminate use of antibiotics has led to the development of multidrug-resistant microorganisms and consequently antimicrobial resistance (MDR; Hamad et al., 2020; Salleh et al., 2020). This microbial resistance represents a serious health problem, potentially raising morbidity and mortality rates, especially in pandemic and epidemic diseases, as highlighted by the World Health Organization (WHO). The emergence of bacteria resistant to antibacterial agents underscores the need to development of more effective antimicrobial agents to overcome these resistance profiles (Das et al., 2020).
According to the WHO, pathogens identified as top-level are categorized as ESKAPE, namely: Enterococcus faecium, Staphylococcus aureus, Klebsiella pneumoniae, Acinetobacter baumannii, Pseudomonas aeruginosa, and Enterobacter species. These pathogens pose significant challenges to human health, as they are the most prevalent in infections caused by MDR. Gram-negative bacteria exhibit a more pronounced profile of microbial resistance compared to Gram-positive strains (Kaiser et al., 2023).
The use of nanoparticles represents a substantial advance in the development of new therapeutics to tackle microbial resistance. Several authors have demonstrated the ability of AgNPs to significantly reduce biofilm formation and bacterial adhesion (Agreles et al., 2022). There is also the possibility of employing NPs in synergy with traditional antibiotics. Due to the unique properties of these nanomaterials, they could potentially reduce the dose and toxicity of antibiotics optimizing treatment compared to the exclusive use of antibiotic (Lee et al., 2019; Kukushkina et al., 2021).
The advantages of using AgNPs lie in their ability to interact with different bacterial mechanisms by targeting multiple pathways, thereby potentially increasing the spectrum of antimicrobial action (Ribeiro et al., 2022). By interacting with a broad spectrum, they can interfere with metabolic pathways by inducing ROS formation, inhibiting and/or modifying enzymes and proteins, reducing cell permeability, and causing homeostatic imbalance (Lee et al., 2019; Agreles et al., 2022).
Several authors have demonstrated the importance of synergy between silver nanoparticles and antibiotics, as evidenced in Table 2. This table presents relevant information on the interaction of these NPs with different antibiotics, highlighting potential synergistic effects that can result in greater effectiveness in combating various strains of microorganisms, including resistant ones.
In summary, the diverse applications of AgNPs as antimicrobial agents encompass a wide range of microorganisms of significant interest in hospital setting, particularly for hospital-acquired infections. By exploring these applications, it is possible to advance the search for innovative solutions to combat and reduce microbial infections and MDR strains through the use of nanotechnology and the unique properties of silver. Current applications of nanomedicine demonstrate significant advancements in the field. These technologies improve existing medical products, offering substantial benefits for patients and healthcare professionals in terms of efficacy, safety and infection control.
4 Silver nanoparticles applied as antibiofilm agents
Bacteria can be found in a mobile, also called planktonic state, being free to disperse and go in search of the nutrients present in their microenvironment or attached to surfaces in a community of microorganisms, called biofilm. In biofilms, bacteria can be protected against harmful conditions, as antibiotic agents, by an extracellular polymeric matrix composed of polysaccharides, protein, lipid and extracellular DNA, produced to promote their adhesion. This makes it difficult for antimicrobial substances to penetrate through the biofilms, making these multicellular structures an additional challenge to overcome microbial contamination (Figure 1). Surgical site infections are common examples of biofilms infections occasioned by surgery procedures that can increase the recovery time, medical costs or even result in death (Bajire et al., 2023; Al-Sawarees et al., 2024).
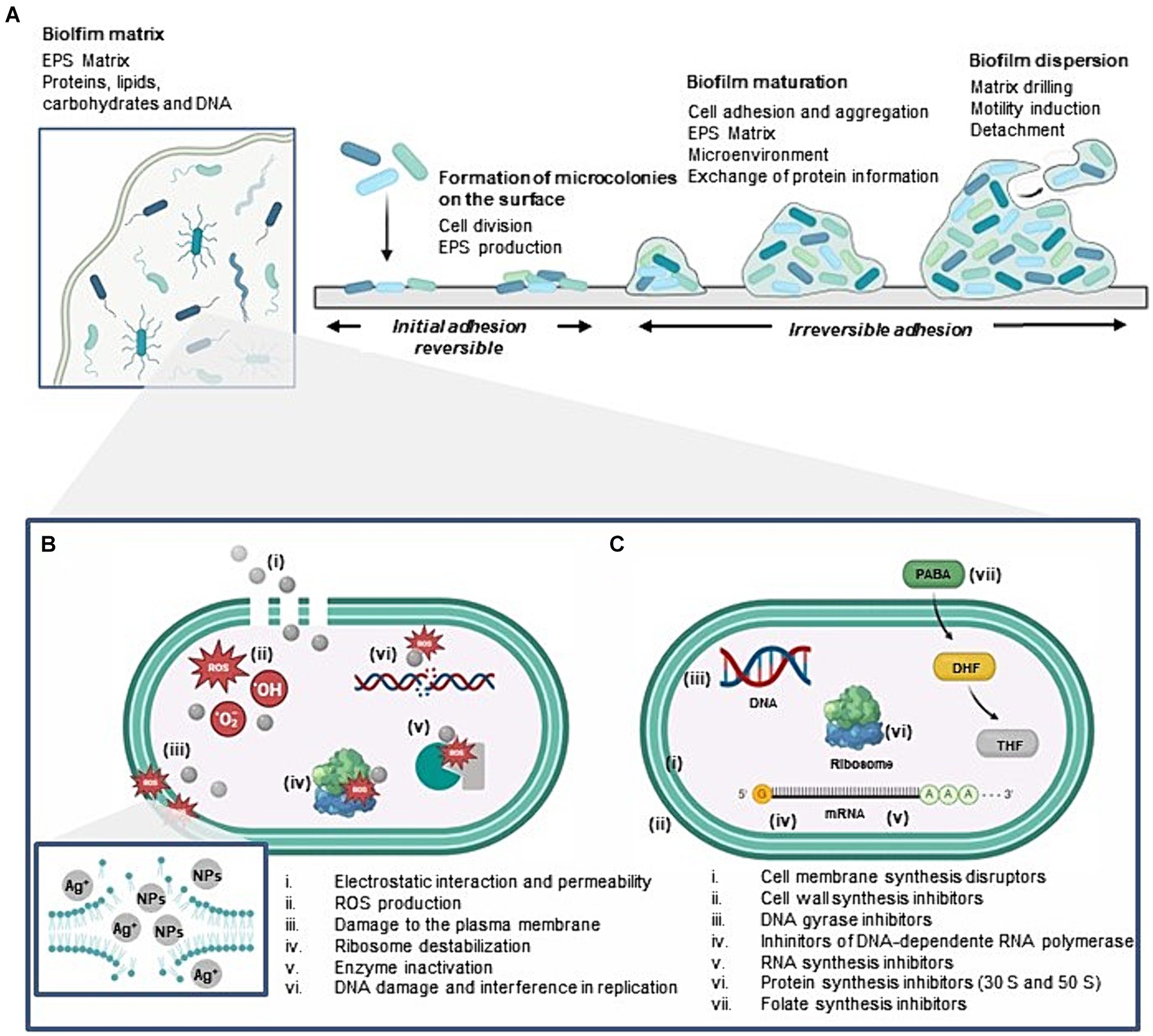
Figure 1. Graphical representation of bacterial biofilm formation (A) and comparison between the mechanism of action of AgNPs (B) and classes of antibiotics in standard use (C) in bacteria: Scattered colonies are deposited on the surface where the biofilm will begin to form. Bacteria adhere to the surface in a reversible way mediated by physicochemical interactions. Bacterial multiplication and formation of microcolonies follow as a consequence of cell division and production of extracellular polymeric substances (EPS). These substances forms an extracellular matrix that surrounds and protects the bacterial cells, providing adherence to the biofilm. As the biofilm matures, bacteria accumulate and more EPS are produced, increasing the biofilm. Eventually, parts of the biofilm may detach from the surface and disperse, carrying bacterial colonies that can colonize new surfaces and begin the biofilm formation process again.
Besides the increased defense obtained with this polymeric matrix, that can act as a semipermeable barrier, some phenotype properties of these microorganisms are affected by the formation of these structures, such as the growth rate and gene transcription, which are commonly increased, allowing the formation of biofilms within a few hours. Furthermore, the bacterial genetic diversity inside the biofilm is mediated by the communication between the cells close to the surface (in contact to the outer environment) and the inner cells through the exchange of plasmids, which strongly contributes to the development of antimicrobial resistance (Pon Janani et al., 2022; Chauhan et al., 2023).
Although biofilms are statically attached to inert or living surfaces, when there is a reduction in the nutrients supply or other changes in physical or chemical factors, the biofilms can propagate by the detachment of clumps of cells or individual organisms, in a process known as seeding dispersal, allowing the proliferation of the infection. Due to all these differences between planktonic and static multicellular structures, biofilm infections may require different agents or approaches for treatment, hence the need for the development of antibiofilm agents (Halsted et al., 2022; Pon Janani et al., 2022; Bajire et al., 2023).
Among the established options to control biofilms formation, we can include ultraviolet irradiation, chlorine and chloramine treatment, hydrogen peroxide and nitrous oxide. However, in some situations these agents cannot be used because of their toxicity, other potential damages or even due to the antibiotic resistance. The environment of biofilms presents a gradient of oxygen content, decreasing from the surface to its deep layers due to the oxygen consumption by the first bacteria, forming anaerobic niches. Additionally, differences in pH between the different regions of these structures can be noticed, resulting in a variety of cells that may differ genotypically and phenotypically. Since higher levels of conventional antimicrobials are required to combat biofilm infections, the risk of systemic toxicity also rises. Nanoscale antimicrobial agents emerge as a promising solution, aiming to enhance antibiofilm activity without inducing harmful side effects (Al-Sawarees et al., 2024.; Chauhan et al., 2023).
When applying metallic nanomaterials to control biofilms growth, three critical steps are known to govern their action; (i) the interactions with the biofilm surface, affected by NPs charge; (ii) entry in the biofilm, dependent on nanomaterials properties such as surface chemistry and charge, size and concentration, as well as to biofilm surface composition and maturity; and (iii) the ability of NPs and their ions to migrate internally to interact with all the components of the biofilm (Chauhan et al., 2023).
AgNPs have been extensively studied as antibiofilm agents, even being used commercially as a coating material to prevent the infection of surfaces of medical supplies as catheters and wound dressings (Al-Sawarees et al., 2024). Table 3 presents the results of some applications of AgNPs as antibiofilm agents.
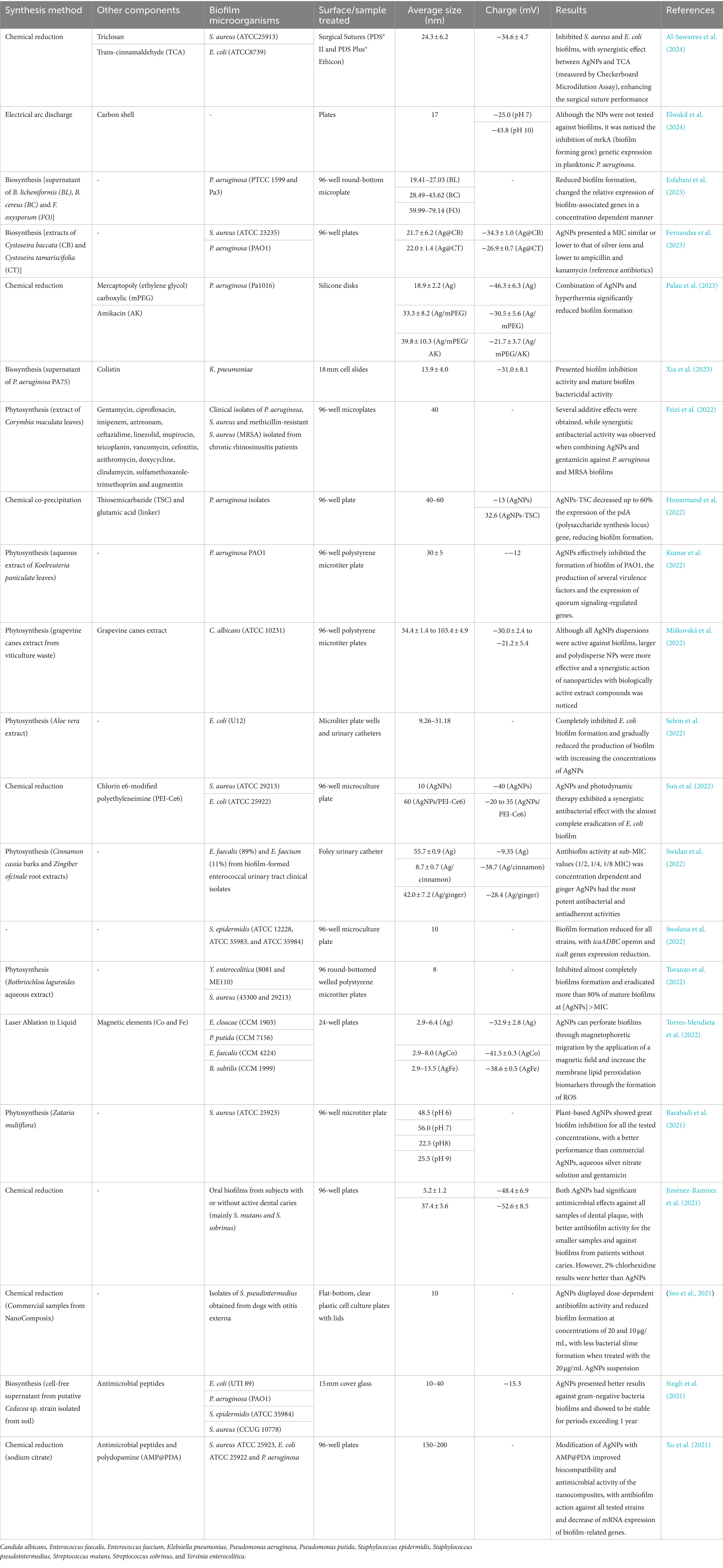
Table 3. Silver nanoparticle formulations applied in reducing and inhibiting the growth of bacterial biofilms.
Table 3 presents a variety of synthesis methods applied in the production of AgNPs with antibiofilm properties. Traditional chemical reduction procedures typically involve hazardous and sometimes expensive substances, necessitating purification steps to remove excess unreacted reagents and reduce toxicity. This increases both the time and cost of nanoparticle production. In contrast, green synthetic methods not only make the produced nanoparticles safer and more cost-effective but also environmentally friendly and conducive to scalability. These methods utilize natural compounds such as proteins, reducing carbohydrates, polyphenols, flavonoids, terpenes, and other secondary metabolites to reduce metal ions and/or act as capping agents. Two prominent classes of green synthesis for producing antibiofilm AgNPs have emerged: biosynthesis and phytosynthesis. Biosynthesis involves algae, microorganisms, or their cell-free extracts (filtrates or supernatants), while phytosynthesis utilizes plant extracts (Miškovská et al., 2022; Selem et al., 2022; Swidan et al., 2022; Toranzo et al., 2022; Esfahani et al., 2023; Fernandes et al., 2023; Xia et al., 2023).
Aiming to develop a sustainable synthesis method for AgNPs, Miškovská et al. (2022) applied a hydroethanolic solution of grape canes, a waste product of vine growing, to sustainably produce mono or polydisperse NPs suspensions that were effective against biofilms, also revealing a synergy between the AgNPs and the plant extract.
Fernandes et al. (2023) used microalgae, brown seaweeds, to biosynthesize AgNPs that presented better action against biofilms than the reference antibiotics kanamycin and ampicillin, while Esfahani et al. (2023) and Xia et al. (2023) applied biological compounds (bacterial or fungal metabolites) from the supernatant or biomass of microorganisms as reducing, stabilizer, and dispersant agents, both obtaining AgNPs with good results in biofilm control.
Physical methods can also be applied in AgNPs production. This class of synthesis methods can produce NPs with minimal impurities and, sometimes, in a most effective way than chemical and biological methods. One physical method applied for antibiofilm AgNPs production is the laser ablation in liquid, a low-cost and eco-friendly procedure in which a metal foil (or more than one, when producing multi element metallic NPs) is immersed in a solvent, followed by ablation with a laser beam, which results in NPs free of organic contaminants or capping agents, needing only of purification steps to change the solvent, for example, from a hydroethanolic solution to water (Torres-Mendieta et al., 2022).
Electrical arc discharge (EAD) is another example of this class in which two electrodes are positioned inside an open vessel containing ultra-pure water and, with the initiation of the arc discharge the silver electrode vaporizes being then quenched by the cold solution, forming nanoparticles (Tseng et al., 2011; Elwakil et al., 2024).
Although Elwakil et al. (2024) assessed the antimicrobial activity of the AgNPs covered with carbon shells produced by EAD only against planktonic bacteria, the authors reported the inhibition of mrkA (biofilm forming gene), fimH (virulence adhesion gene) and rmpA (mucoid factor encoding gene) genetic expression in P. aeruginosa, affecting its intracellular signaling pathway. Honarmand et al. (2022), in turn, observed the importance of inhibiting the polysaccharide synthesis locus (psl) gene for P. aeruginosa biofilm formation control. The authors produced AgNPs and conjugated it with TSC, antimicrobial compound that did not present an expressive effect in gene inhibition, but enhanced the antibiofilm activity when conjugated with the NPs. Since Psl, along with Pel polysaccharide are responsible for the formation of the primary matrix of biofilm, this effect of partial inhibition can be understood.
In view to interfere in quorum sensing (QS)-regulated P. aeruginosa biofilm formation, AgNPs were phytochemically prepared using aqueous extract of K. paniculata leaves, an anti-QS substance. The NPs reduced biofilm formation of a multidrug-resistant model strain of P. aeruginosa almost completely, downregulating several QS-virulence factors, such as pyocyanin, pyochelin, alginate, protease and rhamnolipid, and QS-regulated genes (lasR, rhlR, rhlI, lasI, lasA, lasB, rhlA, and rhlB; Kumar et al., 2022).
Xu et al. (2021) produced nanocomposites of AgNPs modified with antimicrobial peptides and polydopamine that were able to improve both HEK293T cells viability and antibacterial activity against S. aureus, E. coli, and P. aeruginosa. Thickness, biomass and the semi-quantitative analysis of crystal violet staining results indicated an effective reduction of biofilms of all strains, with the reduction of the mRNA expression of biofilm-related genes las I, rh II and fim H.
The size of AgNPs significantly impacts their ability to combat biofilms. The dense extracellular matrix of biofilms acts as a barrier to NPs penetration. Moreover, smaller AgNPs possess a greater surface area, allowing for increased interaction with biofilms and potentially enhanced antibiofilm activity (Selem et al., 2022). As shown in Table 3, most antibiofilm AgNPs are under 100 nm, with many falling below 50 nm. Polydispersity also plays a role, as Miškovská et al. (2022) noted that polydisperse NPs were more effective than monodisperse suspensions of AgNPs in controlling C. albicans biofilms.
Fernandes et al. (2023) compared the effectiveness of silver nitrate and AgNPs against S. aureus and P. aeruginosa biofilms, finding similar or lower MIC values for the phytosynthesized NPs. This suggests that silver ions, released from AgNPs play a crucial role in inhibiting microbial growth within biofilms, overcoming the compact biofilm structure that impedes antibiotic penetration.
Many microorganisms developed resistant strains that are not susceptible to the classic antibiotics. In these cases, a common strategy is the combination of antimicrobial agents aiming to obtain a synergistic effect, since these components can present complementary mechanisms to inhibit microbial growth and form stable complexes via weak intermolecular interactions that prolong their release and reduce their potential local toxicity (Feizi et al., 2022; Miškovská et al., 2022; Torres-Mendieta et al., 2022; Palau et al., 2023; Xia et al., 2023; Al-Sawarees et al., 2024). This approach can include mixing AgNPs with classical antibiotics (Feizi et al., 2022; Palau et al., 2023; Xia et al., 2023), plants extract rich in bioactive molecules as antioxidants (Miškovská et al., 2022) or magnetic elements (Torres-Mendieta et al., 2022), which allows the use of magnetic fields to damage biofilms, promoting NPs penetration by highways created via mechanical disruption without additional associated risks.
Al-Sawarees et al. (2024) mixed AgNPs with Triclosan (5-chloro-2-(2,4-dichlorophenoxy) phenol), a synthetic antimicrobial compound used in a variety of consumer products, including hygiene products and to coat medical supplies as sutures (PDS Plus®, Vicryl Plus® and Monocryl 250 Plus®) or trans-cinnamaldehyde (TCA), a phytochemical extracted from cinnamon with antimicrobial activity and generally recognized as safe by U.S. Food and Drug Administration (FDA). For both combinations the AgNPs effect against S. aureus and E. coli biofilms formed in surgical sutures was improved, with additive effect when combining the NPs to Triclosan and synergistic effect when used with TCA.
Besides combining substances, an approach applied in biofilms control involves the use of hyperthermia, i.e., the use of heat as a therapeutic method to control microbial growth. Hyperthermia is specially used in cancer treatment and involves cell death by local heating (41°C to 50°C). Palau et al. (2023) observed important biofilm reduction combining AgNPs functionalized with mercaptopoly (ethylene glycol) carboxylic and acid amikacin to hyperthermia, significantly reducing biofilm formation by P. aeruginosa.
Another well-established approach for microbial control is the photodynamic therapy (PDT). In this case, a photosensitizer agent is used to produce ROS or singlet oxygen when activated by light irradiation (typically UV–vis or near infrared light) in the presence of oxygen, since these species eradicate microorganisms via oxidative damage of cell wall or membranes, cytoplasm leakage, lipid peroxidation, metabolic inhibition and also damages to mitochondria, membrane transport system or DNA (Pon Janani et al., 2022). Sun et al. (2022) coated negatively charged AgNPs (−40 mV) with positively charged polyethylenimine (PEI) and chlorin e6 (Ce6), a natural molecule approved by FDA and commonly used as a photosensitizer. Although AgNPs and PEI-Ce6, in separate, were able to reduce the microbial adhesion, when combined, this system completely inhibited the biofilm of E. coli formation and significantly reduced S. aureus biofilm formation. The exposure of biofilms of S. aureus and E. coli to AgNPs-PEI-Ce6 suspensions in combination to PDT significantly reduced the microbial survival in vitro, and in mice both PEI-Ce6 and AgNPs-PEI-Ce6 promoted a faster recovery of S. aureus wound infection, showing the benefits of combining NPs and PDT.
To mimic real-world infections more accurately, researchers (Jiménez-Ramírez et al., 2021; Seo et al., 2021; Feizi et al., 2022; Swidan et al., 2022), utilized biofilms derived from clinical isolates. These biofilms often harbor multiple bacterial strains, requiring antibiofilm agents with diverse modes of action. Similarly, to treating standard reference strains, studies have shown that AgNPs are effective against biofilms formed by clinical isolates, demonstrating similar success to those observed with standard lab strains. For instance, Jiménez-Ramírez et al. (2021) investigated the impact of caries on oral biofilms (primarily composed of S. mutans and S. sobrinus). They found that AgNPs were more effective in controlling biofilms from individuals without caries.
The scenario of AgNPs in biofilm control shows that, despite the well-known properties of these NPs, the combination with other compounds or approaches can be a good option in the development of antibiofilm agents that efficiently control the formation or eliminate these bacteria structures, even when involving resistant strains.
5 Bacterial resistance to AgNPs and Ag ions
The fight against infections associated with the previously mentioned bacterial biofilms, particularly those caused by MDR strains has driven the development of novel approaches (Joshi et al., 2020). Persistent bacteria exhibit phenotypic changes that enable them to evade antimicrobial agents, often through genetic mutations (Salas-Orozco et al., 2022). Among the various bacterial species described in the literature, Gram-negative bacteria have shown the most significant resistance to silver. This resistance mechanism is partly due to presence of lipopolysaccharides (LPS) on their cell wall, which induce electrostatic repulsion with negatively charged AgNPs (Maillard and Hartemann, 2013). Factors contributing to silver resistance in bacteria involve chemical detoxification mechanisms and active efflux systems. These systems, such as P-type ATPases and cation/proton transporters facilitate the transport of silver ions into or out of bacterial cells. Additionally, silver ions can be reduced to their inactive elemental metallic form, decreasing their antimicrobial activity (Maillard and Hartemann, 2013).
Like the mechanism of action of AgNPs as an antimicrobial agent involves a series of factors and multiple ways of interacting, studies suggest that the mechanism of bacterial resistance to silver involves a combination of interrelated systems. Among the main mechanisms of bacterial resistance to silver described in the literature, the following may occur: induction of aggregation of silver nanoparticles; reduction of Ag+ ions to Ag0; contact protection and prevention of silver entry into cells; efflux of silver nanoparticles and Ag+ ions through cellular efflux pumps; activation of defense mechanisms and repair of cellular damage; biofilm defense; antibiotic-mediated silver resistance; mutation; modulating the surface charge of cell membranes and response to stress and vitality (Li and Xu, 2024).
Exposure to sublethal concentrations of AgNPs can trigger the development of resistance phenotypes due to oxidative stress and DNA damage. These phenotypes may arise through active and passive resistance mechanisms. Active resistance mechanisms involve the direct response of the bacterium to the antimicrobial stimulus, including the use of efflux pumps and genetic mutations. Passive resistance mechanisms involve intrinsic characteristics of the bacterium, such as the thickening of the cell wall and the formation of bacterial biofilm (Salas-Orozco et al., 2022).
Factors contributing to silver resistance in bacteria involve chemical detoxification mechanisms and active efflux systems. These systems, such as P-type ATPases and cation/proton transporters, play a role in transporting silver ions into or out of bacterial cells. Additionally, it is possible to reduce the silver concentration by converting the Ag+ ion to the inactive elemental metallic form Ag0, thus not presenting antimicrobial activity and other properties associated with the silver ion (Maillard and Hartemann, 2013).
AgNPs can interact with nucleic acids, aiding in the aggregation, formation, structure, and integrity of bacterial biofilm, as well as with membrane proteins and enzymes that promote homeostasis, as well as polysaccharides and lipids. These interactions can originate hydrophobic, Van der Waals, hydrogen bonding, π–π, ionic, or electrostatic, with the latter contributing most to the biocidal activity of nanoparticles (Joshi et al., 2020).
The ability of bacterial resistance to AgNPs was evidenced through TEM and UV–visible spectroscopy of nanoparticles. In the case of the E. coli CCM 3954 strain, classified as ‘silver-resistant’, aggregation and/or precipitation of AgNPs were induced. Flagellin, a structural protein of bacterial flagella responsible for bacterial locomotion, played a role in promoting the aggregation of AgNPs, resulting in the reduction of their antibacterial effect (Panáček et al., 2018).
A fact with few studies yet relates that resistance to antibiotics in bacteria can also lead to the development of resistance to silver. A study demonstrated that both AgNPs and Ag+ can remove mature P. aeruginosa biofilms depending on the concentration of these compounds, however, AgNPs and Ag+ at the same concentrations were not able to destroy the biofilm formed by gentamicin-resistant P. aeruginosa (Mann et al., 2021). Therefore, the use of inorganic antimicrobial agents and antibiotics needs to follow more rigorous protocols, as improper use can lead to the emergence of superbugs and compromise the action of various antibiotic therapy strategies (Yonathan et al., 2022).
Researchers studied the heredity and stability of resistance to AgNPs. By removing and reintroducing AgNPs in various growth passages, the hypermotile E. coli strain K-12 MG1655 maintained resistance, indicating a hereditary, irreversible, and permanent genetic alteration due to the expression of resistance genes, even in the absence of silver. Genomic analyses identified a missense mutation (R292L) in the cusS gene, which remained constant in all lineages. This mutation, located in the active site of dimerization and the histidine phosphotransfer domain, enhances silver ion efflux, thereby conferring resistance to AgNPs (Stabryla et al., 2021).
Analyzing the impact of bacterial motility on AgNPs resistance, researchers found that non-motile E. coli strain did not exhibit significant MIC increase upon repeated AgNPs exposition. Genomic analysis did not reveal the presence of resistance genes, indicating that resistance to AgNPs may be specific to strains with bacterial motility (Stabryla et al., 2021).
To assess physiological changes triggered by AgNPs exposure, E. coli pre-treated with AgNPs showed increased MIC and MBC values (of two to eight times) when subsequently exposed to antibiotics like aminoglycosides, penicillins, and phenicols compared to the group that did not receive pre-treatment with AgNPs, indicating adaptive responses mechanism that promove antimicrobial resistance (Kaweeteerawat et al., 2017).
Most studies determining the effectiveness of AgNPs as antimicrobials are performed in single-species cultures. However, bacteria are rarely found in a single-species group in the environment. Researchers have demonstrated mechanisms that allow the sharing of resistance between bacteria that are usually found in association. For example, multiple compounds secreted by P. aeruginosa have been found to increase the tolerance of S. aureus to silver, both microorganisms are found associated in severe chronic infections leading to increased virulence and tolerance to antibiotics. The secretion of Pseudomonas quinolone signal (PQS) or compounds directly controlled by PQS, as well as the amino acids serine and threonine, generate silver tolerance in S. aureus. These compounds probably affect the physiology and metabolism of S. aureus (PQS, serine and threonine) by decreasing the availability of silver in the medium through bonds (Monych Nadia and Turner Raymond, 2020).
Understanding these complex interactions between bacteria, AgNPs and silver ions is crucial for developing effective strategies against bacterial resistance. Such insights contribute to significant advancements in nanomedicine and infection control strategies.
6 Proteomic analysis of the antibacterial mechanism of action
Proteomics is a branch of molecular biology that studies the set of proteins expressed by an organism or cell in response to different conditions. For instance, when microorganisms are exposed to a certain antimicrobial agent such as AgNPs, proteomic analysis provides information about action and resistance mechanisms of these microorganisms to the antimicrobial agent.
Recent findings have revealed molecular processes underlying the action of AgNPs against microorganisms. Understanding these proteomic mechanisms can guide the development of new antimicrobial strategies. Here are some relevant considerations regarding the proteomics of microorganisms exposed to AgNPs which aid in understanding the results of such analyses.
6.1 Proteomic profile analysis
Proteomic analysis allows for the identification and quantification of specific proteins from a given organism or cell. Generally, each microorganism has a distinct identity (fingerprint) in its protein expression profile. When microorganisms are exposed to AgNPs, it is possible to investigate how these nanoparticles affect protein expression. Techniques such as two-dimensional gel electrophoresis (2D-PAGE) and mass spectrometry are frequently used to analyze the proteomic profile.
The study of microbial proteomics opens a universe of possibilities; different bacterial strains can exhibit different protein expression profiles even when exposed to a single formulation of silver nanoparticles. Similarly, a standard strain may display distinct proteomic profiles when exposed to different AgNPs. AgNPs are generally considered a unique type of antibacterial agent, but their physical and chemical properties determine the way they interact with bacterial cells, the mode of action, and the response of the exposed bacterial cell (Kędziora et al., 2021).
6.2 Mechanisms of action of AgNPs
AgNPs can interact with cellular proteins, leading to changes in protein expression. They can affect metabolic pathways, signaling processes, and stress responses. Proteomic analysis contributes to the identification of specific proteins involved in these mechanisms. Table 4 represents the data of proteins expressed in bacteria after treatment with AgNPs.
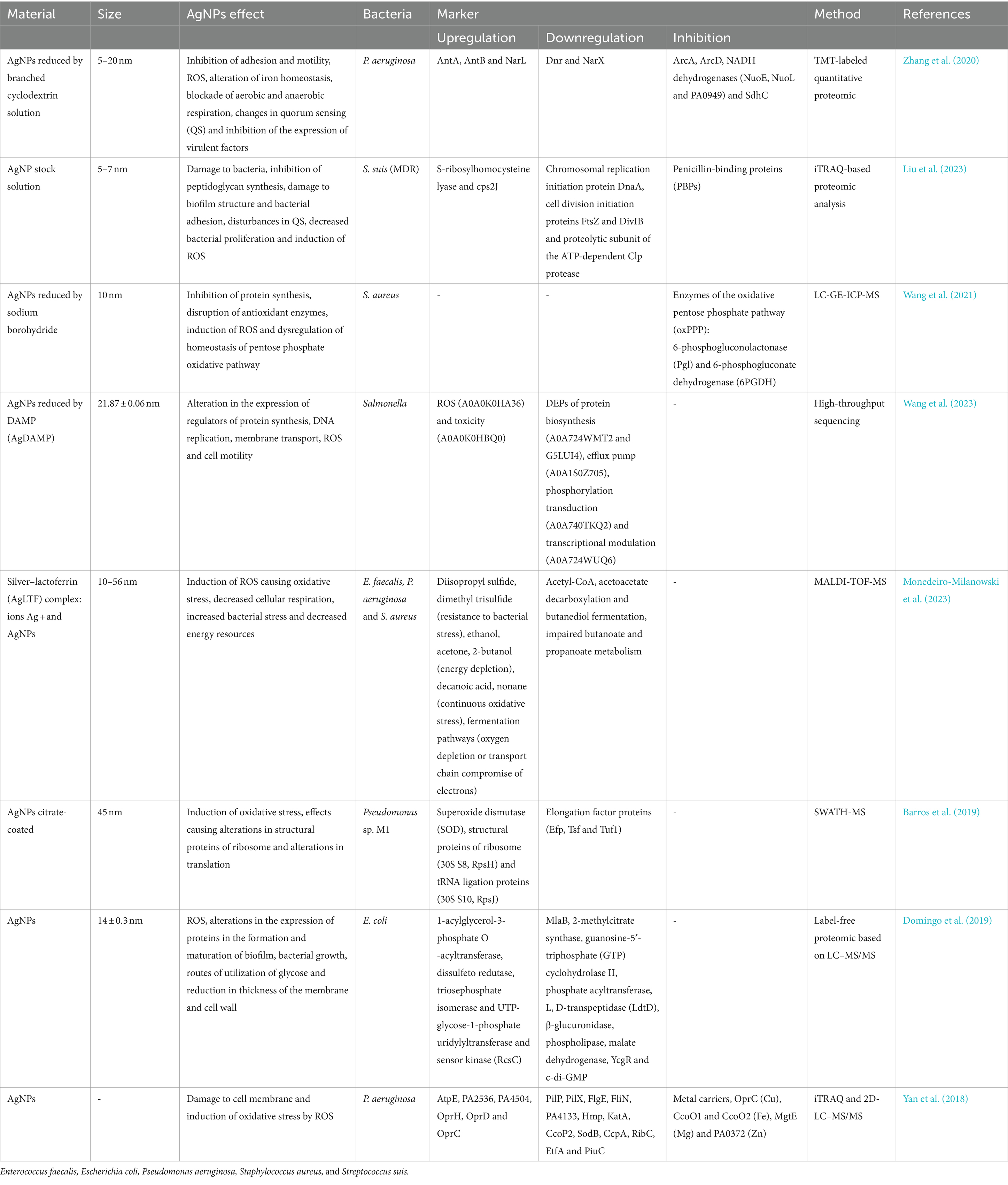
Table 4. Mechanism of action detected by proteomics: proteins expressed in bacteria after treatment with AgNPs.
In early reports of proteomic analyses, researchers identified a potential mode of action underlying the antibacterial action of AgNPs. An accumulation of protein precursors from the envelope was found in the proteomic signatures of E. coli cells treated with AgNPs, suggesting that AgNPs may interact with the bacterial membrane. Brief exposure of E. coli cells to AgNPs resulted in changes in the expression of envelope proteins (OmpA, OmpC, OmpF, OppA, MetQ) and heat shock proteins (IbpA, IbpB, and ribosomal subunit 30S S6). Additionally, AgNPs and Ag+ ions in the form of AgNO3 exhibited similar mechanisms of action targeting the membrane. AgNPs and Ag+ ions were used at nanomolar and micromolar levels, respectively, suggesting that AgNPs have significantly greater antimicrobial activity than Ag+ ions (Lok et al., 2006).
Studies conducted with P. aeruginosa (Gram-negative bacteria) treated with AgNPs yielded comprehensive proteomic responses, aiding in the elucidation of the antimicrobial mechanism. Proteomic analysis of bacteria treated with AgNPs revealed elevated levels of enzymes such as superoxide dismutase (SOD), catalase (CAT), and peroxidase (POD), as well as alkyl hydroperoxide reductase and hydroperoxide resistance proteins. There was also significant positive regulation of low-oxygen regulatory oxidases, such as subunits P2, N2, and O2 of cytochrome c oxidase type cbb3. This demonstrates that the primary mechanism of action of AgNPs involves the imbalance of oxidation and antioxidation processes and the failure to eliminate excess ROS (Liao et al., 2019). Another study identified 5 silver-binding proteins and 59 proteins regulated by silver (27 positively regulated proteins and 32 negatively regulated proteins). Proteomic profiling showed that the cell membrane was the primary target of AgNPs, leading to oxidative stress induced by ROS generation. The release of silver ions and specific particle effects synergistically contribute to the antibacterial action of AgNPs. Moreover, the same silver-binding proteins were obtained with AgNPs and silver ions, indicating that AgNPs likely affect the cell membrane and react with proteins, releasing silver ions. These results demonstrate that the antimicrobial activity of AgNPs is due to the synergistic action of dissolved silver ions release and specific particle effects (Yan et al., 2018).
In another investigation, proteomic analysis of AgNPs against multidrug-resistant P. aeruginosa analyzed antibiofilm mechanisms. Based on Tandem Mass Tag (TMT)-labeled quantitative proteomics, various antibiofilm mechanisms of AgNPs were revealed. These included inhibition of adhesion and motility, strong stimulation of oxidative stress response, disruption of iron homeostasis, blocking of energy production (both aerobic and anaerobic), and affecting quorum sensing detection. The latter is a communication mechanism used by bacteria to coordinate gene expression based on their population density. Proteomic analysis showed alterations in protein expression at different functional levels, suggesting that AgNPs may eliminate P. aeruginosa biofilms in multiple ways (Zhang et al., 2020).
Based on quantitative proteomic analysis (iTRAQ), it was discovered that AgNPs disrupt the natural morphology environment of S. suis and its biofilm. Several proteins related to peptidoglycan synthesis were negatively regulated, including penicillin-binding proteins (PBPs), glycosyltransferases, and LytR family transcriptional regulators, leading to bacterial membrane rupture. Additionally, AgNPs can prevent bacterial adhesion, interfere with QS system, and inhibit bacterial growth by targeting the cell division protein FtsZ and the chromosomal replication initiator protein DnaA. Increased oxidative stress is also a relevant factor contributing to bacterial death (Liu et al., 2023).
6.3 Applications
Conventional antibiotics have revolutionized the treatment of bacterial infections and led to the emergence of strains resistant to various antibiotics restricting antimicrobial molecules to hospital to avoid the development of superbugs that cannot be combated with the existing arsenal of antibiotics. The continuous and excessive use of such antibiotics has led to outbreaks of superbugs in hospitals and communities. In recent decades, silver has been used in medical treatments such as burns, wounds, and bacterial infections. Metallic silver, silver nitrate, and silver sulfadiazine have been used in these treatments. Nowadays, AgNPs and silver ions are used as antibacterial agents in the medical field in the form of nanoparticles and ions, with proven effectiveness against bacteria, fungi, and viruses. These nanostructures can still be modified or functionalized to be more effective than pure AgNPs. Size, shape, concentration, surface charge, and functionalization are the main properties that determine antimicrobial efficiency (Hamad et al., 2020). The broad-spectrum antimicrobial activity of nanomaterials compared to antibiotics may off a solution to combat MDR bacterial strains. For example, AgNPs act through various mechanisms, including overcoming antibiotic resistance mechanisms, damaging membranes, and preventing biofilm formation. When applied together with other antimicrobial molecules, therapy can be enhanced and the emergence of new MDR strains avoided (Alavi and Hamblin, 2023).
Spherical AgNPs showed efficacy against Porphyromonas gingivalis and S. mutans (MIC = 8 μg/mL) and significantly prevented the adhesion of S. mutans in vitro. Proteomic analysis showed that after a short period of treatment of bacteria with AgNPs, changes in the expression of various heat shock proteins and bacterial cell coating proteins were observed, suggesting that nanoparticles may penetrate the bacterial membrane leading to destruction. AgNPs can also cause a decrease in intracellular potassium, resulting in a reduction in ATP levels. Possible molecular targets of AgNPs may be thiol protein groups and the phospholipid part of the bacterial cell membrane. Therefore, AgNPs@Abelmoschus esculentus may be good candidates for oral hygiene agents to prevent periodontopathic conditions and dentures (Nie et al., 2023).
Researchers studied a strategy combining hollow mesoporous silica nano spheres (HMSN) loaded with AgNPs, vancomycin and hemin (HAVH) for the elimination of MDR bacteria in abscess therapy. Demonstrating that the multi-path antibacterial mechanisms of HAVH could reduce the risk of drug resistance development by addressing the limitation of conventional antibiotics. In addition to the strategy combining AgNPs with antibiotics (HAVH), NIR irradiation showed improved synergistic effects, suggesting the potential of combinatory strategies for treating MDR bacterial infection. Enrichment analysis of genetic ontology (GO) indicated that differentially expressed proteins were associated with biological processes, cellular components, and molecular functions, including changes in nucleotide binding sites, cytoplasm, and transcription processes (Yan et al., 2023).
6.4 Resistance and adaptation: a proteomic view/approach
Prolonged exposure to AgNPs can lead to the selection of resistant microorganisms. Proteomics can reveal how microorganisms adapt to AgNPs by modifying their protein expression. Thus, application studies should consider the possibility that AgNPs may increase antibiotic resistance by causing mutations in resistance genes (Alavi and Hamblin, 2023). A study using E. faecalis demonstrated the ability of these microorganisms to develop resistance to AgNPs. This resistance consists of the expression of multifaceted mechanisms. Proteomic analysis revealed that 1,080 proteins were unique to E. faecalis exposed to AgNPs compared to 8 identified in the control sample. Among the affected biological processes and molecular functions are oxidative stress (superoxide dismutase and peroxiredoxin AhpC); transcriptional factors (Cro, Lux, and Rex); universal stress protein; and fibronectin adhesin Pav-type (FIBR). Therefore, strategies should be explored to prevent the emergence of this type of resistance, which, when combined with co-stimulation of antibiotic resistance, could increase the emergence of bacteria and infections resistant to antimicrobial drugs. To avoid resistance generation against AgNPs, the study suggested the combined use of nanoparticles prepared with different metals to prevent the spread of resistant strains (Salas-Orozco et al., 2022).
The transfer of antibiotic resistance genes (ARGs) is considered one of the main ways of spreading resistance, which occurs through horizontal gene transfer (HGT). There are reports that AgNPs enhance the transfer of ARGs via plasmid transmission between bacterial genera. It was determined that both AgNPs and ionic silver, in environmentally relevant and sublethal concentrations, facilitate the transfer of the IncP-α RP4 plasmid produced and supplied by Escherichia coli K-12 LE392 to the recipient bacterium Pseudomonas putida KT2440. Based on proteomic analyses, genetic sequencing, the potential ecological risks of environmental levels of AgNPs and silver ions in the promotion and dissemination of ARGs by HGT were exposed. Thus, warning against the excessive use of AgNPs in personal care formulations as antimicrobial agents (Lu et al., 2020).
6.5 Challenges in the applications of AgNPs: a proteomic view
Among the metallic nanoparticles consumed daily, AgNPs have become the most common due to their antimicrobial effect. A study concerned with the effects of sublethal concentrations of AgNPs on intestinal biofilms investigated the in vitro proteomic response of a simulated intestinal biofilm with E. coli monospecies to chronic and acute exposures of sublethal concentrations of AgNPs. Of the 1,917 proteins identified, 212 showed different levels of expression. Several pathways were altered, including biofilm formation, bacterial adhesion, response to reactive oxygen species stress, and glucose utilization (Domingo et al., 2019). These findings contribute to a better understanding of the molecular basis of the antibacterial activity of AgNPs, highlighting potential targets for the development of new antimicrobial agents or new therapies that combine classical antimicrobial agents and nanoparticles. Thereby, improving the antimicrobial arsenal against infections caused by multidrug-resistant microorganisms affecting humans and animals (Liu et al., 2023). However, alongside these advancements, it is crucial to consider the potential impact on the natural microbiota (Domingo et al., 2019), as well as the interaction of AgNPs with other cells and biological structures of organisms. Since AgNPs are known to significantly impact living cells, their widespread use raises concerns about both immediate and long-term effects following exposure. For example, a proteomic study with macrophages showed that some functions of these specialized cells, such as lipopolysaccharide-induced cytokine production and nitric oxide, did not return to baseline even 72 h after exposure, showing that some effects of AgNPs persist even after exposure has ceased. This process of recovery of homeostasis after acute exposure to AgNPs involves hundreds of proteins and an enormous energy consumption, 50% higher than normal. The persistence of changes in the proteomic profile may be related to the intracellular silver persistence during the recovery period. Although the concentration of cellular lethality is not reached, post-exposure effects can impact the health status of living organisms (Dalzon et al., 2019). Proteomic analysis has become a valuable tool for identifying new biomarkers with greater sensitivity and specificity, contributing to a better understanding of disease progression, the development of new drugs and combined therapeutic strategies, as well as the improvement of toxicological studies. The identification and quantification of proteins in cells, tissues, or organisms provide insight into the activity of new therapeutics at specialized and personalized molecular levels (Piatek et al., 2023).
7 Future perspectives and emerging trends
Silver nanoparticles (AgNPs) have emerged as a promising antimicrobial agent, exhibiting potent activity against a broad spectrum of microorganisms, facilitated by a multifaceted mechanistic approach that enables them to interact with microbial cells in multiple ways. Proteomics has played a crucial role in elucidating the mechanisms of action of AgNPs at the molecular level, revealing that they disrupt cellular processes, including protein synthesis, membrane transport, and cell signaling pathways. These insights have been pivotal in understanding the multifaceted antimicrobial mechanisms of AgNPs (More et al., 2023). The use of AgNPs as antimicrobial agents offers a promising solution to combat the growing threat of antibiotic resistance, a global health concern and their applications in medical devices, wound healing, and water treatment are being explored.
Future perspectives for AgNPs as antimicrobial agents lie in their potential to be combined with other therapeutic agents, such as antibiotics and antioxidants, to enhance their efficacy and reduce toxicity (Chakravarty et al., 2022). Emerging trends include the development of targeted AgNPs that can selectively target specific microorganisms and reduce off-target effects. Additionally, the application of AgNPs in understanding microbial proteomics and identifying novel antimicrobial targets presents a thrilling research avenue, particularly in the face of the escalating global crisis of AMR. As AMR poses a significant threat to the effectiveness of conventional antibiotics against bacterial infections, the WHO’s Global Antimicrobial Resistance and Use Surveillance System (GLASS) has reported alarmingly high resistance rates among common bacterial pathogens, underscoring the urgent need for innovative solutions. Therefore, the potential of AgNPs to revolutionize the field of antimicrobial therapy, and further research is required to fully explore their potential and address the challenges associated with their use.
Author contributions
AR: Conceptualization, Writing – original draft, Writing – review & editing. JB: Conceptualization, Writing – original draft, Writing – review & editing. MR: Writing – original draft, Writing – review & editing. VT: Writing – review & editing, Writing – original draft. LM: Supervision, Funding acquisition, Writing – review & editing. PL: Supervision, Funding acquisition, Writing – review & editing. AL: Funding acquisition, Supervision, Conceptualization, Resources, Writing – review & editing.
Funding
The author(s) declare that financial support was received for the research, authorship, and/or publication of this article. This study was financed in part by the Coordination for the Improvement of Higher Education Personnel – Brazil (CAPES) – Finance Code 001. The authors thank the National Council for Scientific and Technological Development (CNPq) process 406923/2021-3, National Institute of Science and Technology (INCT) 403193/2022-2 and process 2022/08705-2 São Paulo Research Foundation (FAPESP).
Acknowledgments
The authors thank the home institutions.
Conflict of interest
The authors declare that the research was conducted in the absence of any commercial or financial relationships that could be construed as a potential conflict of interest.
Publisher’s note
All claims expressed in this article are solely those of the authors and do not necessarily represent those of their affiliated organizations, or those of the publisher, the editors and the reviewers. Any product that may be evaluated in this article, or claim that may be made by its manufacturer, is not guaranteed or endorsed by the publisher.
References
Aabed, K., and Mohammed, A. E. (2021). Synergistic and antagonistic effects of biogenic silver nanoparticles in combination with antibiotics against some pathogenic microbes. Front. Bioeng. Biotechnol. 9:652362. doi: 10.3389/fbioe.2021.652362
Abada, E., Galal, T., and Ismail, I. (2021). Biosynthesis of silver nanoparticles by Nocardiopsis sp.-MW279108 and its antimicrobial activity. J. Basic Microbiol. 61, 993–1001. doi: 10.1002/jobm.202100248
Abdel-Rahman, L. H., Al-Farhan, B. S., El-ezz, D. A., Sayed, M., Zikry, M. M., and Abu-Dief, A. M. (2022). Green biogenic synthesis of silver nanoparticles using aqueous extract of Moringa oleifera: access to a powerful antimicrobial, anticancer, pesticide and catalytic agents. J. Inorg. Organomet. Polym. Mater. 32, 1422–1435. doi: 10.1007/s10904-021-02186-9
Agreles, M. A. A., Cavalcanti, I. D. L., and Cavalcanti, I. M. F. (2022). Synergism between metallic nanoparticles and antibiotics. Appl. Microbiol. Biotechnol. 106, 3973–3984. doi: 10.1007/s00253-022-12001-1
Akter, S., and Huq, M. A. (2020). Biologically rapid synthesis of silver nanoparticles by Sphingobium sp. MAH-11T and their antibacterial activity and mechanisms investigation against drug-resistant pathogenic microbes. Artif. Cells Nanomed. Biotechnol. 48, 672–682. doi: 10.1080/21691401.2020.1730390
Alahmad, A., Al-Zereini, W. A., Hijazin, T. J., Al-Madanat, O. Y., Alghoraibi, I., Al-Qaralleh, O., et al. (2022). Green synthesis of silver nanoparticles using Hypericum perforatum L. aqueous extract with the evaluation of its antibacterial activity against clinical and food pathogens. Pharmaceutics 14:1104. doi: 10.3390/pharmaceutics14051104
Al-asbahi, M. G. S. S., Al-Ofiry, B. A., Saad, F. A. A., Alnehia, A., and Al-Gunaid, M. Q. A. (2024). Silver nanoparticles biosynthesis using mixture of Lactobacillus sp. and Bacillus sp. growth and their antibacterial activity. Sci. Rep. 14:10224. doi: 10.1038/s41598-024-59936-1
Alavi, M., and Hamblin, M. (2023). Antibacterial silver nanoparticles: effects on bacterial nucleic acids. Cell. Mol. Biomed. Rep. 3, 35–40. doi: 10.55705/cmbr.2022.361677.1065
Al-Dbass, A. M., Daihan, S. A., Al-Nasser, A. A., Al-Suhaibani, L. S., Almusallam, J., Alnwisser, B. I., et al. (2022). Biogenic silver nanoparticles from two varieties of Agaricus bisporus and their antibacterial activity. Molecules 27:21. doi: 10.3390/molecules27217656
Alowaiesh, B. F., Alhaithloul, H. A. S., Saad, A. M., and Hassanin, A. A. (2023). Green biogenic of silver nanoparticles using polyphenolic extract of olive leaf wastes with focus on their anticancer and antimicrobial activities. Plants 12:6. doi: 10.3390/plants12061410
Al-Sawarees, D. K., Darwish, R. M., Abu-Zurayk, R., Al, M., and Al Masri, M. (2024). Assessing silver nanoparticle and antimicrobial combinations for antibacterial activity and biofilm prevention on surgical sutures. J. Appl. Microbiol. 135:4. doi: 10.1093/jambio/lxae063/7627444
Amr, M., Abu-Hussien, S. H., Ismail, R., Aboubakr, A., Wael, R., Yasser, M., et al. (2023). Utilization of biosynthesized silver nanoparticles from Agaricus bisporus extract for food safety application: synthesis, characterization, antimicrobial efficacy, and toxicological assessment. Sci. Rep. 13:15048. doi: 10.1038/s41598-023-42103-3
Anju, T. R., Parvathy, S., Valiya Veettil, M., Rosemary, J., Ansalna, T. H., Shahzabanu, M. M., et al. (2020). Green synthesis of silver nanoparticles from Aloe vera leaf extract and its antimicrobial activity. Mater. Today: Proc. 43, 3956–3960. doi: 10.1016/j.matpr.2021.02.665
Ankudze, B., and Neglo, D. (2023). Green synthesis of silver nanoparticles from peel extract of Chrysophyllum albidum fruit and their antimicrobial synergistic potentials and biofilm inhibition properties. Biometals 36, 865–876. doi: 10.1007/s10534-022-00483-5
Arshad, H., Sami, M. A., Sadaf, S., and Hassan, U. (2021). Salvadora persica mediated synthesis of silver nanoparticles and their antimicrobial efficacy. Sci. Rep. 11:5996. doi: 10.1038/s41598-021-85584-w
Asghar, M. A., Yousuf, R. I., Shoaib, M. H., Asghar, M. A., Ansar, S., Zehravi, M., et al. (2020). Synergistic nanocomposites of different antibiotics coupled with green synthesized chitosan-based silver nanoparticles: characterization, antibacterial, in vivo toxicological and biodistribution studies. Int. J. Nanomedicine 15, 7841–7859. doi: 10.2147/IJN.S274987
Attallah, N. G. M., Elekhnawy, E., Negm, W. A., Hussein, I. A., Mokhtar, F. A., and Al-Fakhrany, O. M. (2022). In vivo and in vitro antimicrobial activity of biogenic silver nanoparticles against Staphylococcus aureus clinical isolates. Pharmaceuticals 15:194. doi: 10.3390/ph15020194
Awad, M. A., Hendi, A., Ortashi, K. M., Alzahrani, B., Soliman, D., Alanazi, A., et al. (2021). Biogenic synthesis of silver nanoparticles using Trigonella foenum-graecum seed extract: characterization, photocatalytic and antibacterial activities. Sensors Actuators A Phys. 323:112670. doi: 10.1016/j.sna.2021.112670
Bajire, S. K., Sanjeeva, S. G., Johnson, R. P., and Shastry, R. P. (2023). “Chapter 4—growth of microbes and biofilm formation on various materials” in Antiviral and antimicrobial smart coatings: Fundamentals and applications. eds. A. Kumar, A. Behera, T. A. Nguyen, M. Bilal, and R. K. Gupta (Amsterdam: Elsevier), 87–111.
Balachandar, R., Navaneethan, R., Biruntha, M., Ashok Kumar, K. K., Govarthanan, M., and Karmegam, N. (2022). Antibacterial activity of silver nanoparticles phytosynthesized from Glochidion candolleanum leaves. Mater. Lett. 311:131572. doi: 10.1016/j.matlet.2021.131572
Bamal, D., Singh, A., Chaudhary, G., Kumar, M., Singh, M., Rani, N., et al. (2021). Silver nanoparticles biosynthesis, characterization, antimicrobial activities, applications, cytotoxicity and safety issues: an updated review. Nanomaterials 11:2086. doi: 10.3390/nano11082086
Barabadi, H., Mojab, F., Vahidi, H., Marashi, B., Talank, N., Hosseini, O., et al. (2021). Green synthesis, characterization, antibacterial and biofilm inhibitory activity of silver nanoparticles compared to commercial silver nanoparticles. Inorg. Chem. Commun. 129:108647. doi: 10.1016/j.inoche.2021.108647
Baran, A., Baran, M. F., Keskin, C., Kandemir, S. I., Valiyeva, M., Mehraliyeva, S., et al. (2021). Ecofriendly/rapid synthesis of silver nanoparticles using extract of waste parts of artichoke (Cynara scolymus L.) and evaluation of their cytotoxic and antibacterial activities. J. Nanomater. 2021, 1–10. doi: 10.1155/2021/2270472
Barros, D., Pradhan, A., Mendes, V. M., Manadas, B., Santos, P. M., Pascoal, C., et al. (2019). Proteomics and antioxidant enzymes reveal different mechanisms of toxicity induced by ionic and nanoparticulate silver in bacteria. Environ. Sci. Nano 6, 1207–1218. doi: 10.1039/c8en01067f
Basheer, M. A., Abutaleb, K., Abed, N. N., and Mekawey, A. A. I. (2023). Mycosynthesis of silver nanoparticles using marine fungi and their antimicrobial activity against pathogenic microorganisms. J. Genet. Eng. Biotechnol. 21:127. doi: 10.1186/s43141-023-00572-z
Behravan, M., Hossein Panahi, A., Naghizadeh, A., Ziaee, M., Mahdavi, R., and Mirzapour, A. (2019). Facile green synthesis of silver nanoparticles using Berberis vulgaris leaf and root aqueous extract and its antibacterial activity. Int. J. Biol. Macromol. 124, 148–154. doi: 10.1016/j.ijbiomac.2018.11.101
Bhuyar, P., Rahim, M. H. A., Sundararaju, S., Ramaraj, R., Maniam, G. P., and Govindan, N. (2020). Synthesis of silver nanoparticles using marine macroalgae Padina sp. and its antibacterial activity towards pathogenic bacteria. Beni Suef Univ J Basic Appl Sci 9:3:3. doi: 10.1186/s43088-019-0031-y
Bindhu, M. R., Umadevi, M., Esmail, G. A., Al-Dhabi, N. A., and Arasu, M. V. (2020). Green synthesis and characterization of silver nanoparticles from Moringa oleifera flower and assessment of antimicrobial and sensing properties. J. Photochem. Photobiol. B 205:111836. doi: 10.1016/j.jphotobiol.2020.111836
Brar, K. K., Magdouli, S., Othmani, A., Ghanei, J., Narisetty, V., Sindhu, R., et al. (2022). Green route for recycling of low-cost waste resources for the biosynthesis of nanoparticles (NPs) and nanomaterials (NMs)-A review. Environ. Res. 207:112202. doi: 10.1016/j.envres.2021.112202
Bruna, T., Maldonado-Bravo, F., Jara, P., and Caro, N. (2021). Silver nanoparticles and their antibacterial applications. Int. J. Mol. Sci. 22:7202. doi: 10.3390/ijms22137202
Burdușel, A. C., Gherasim, O., Grumezescu, A. M., Mogoantă, L., Ficai, A., and Andronescu, E. (2018). Biomedical applications of silver nanoparticles: an up-to-date overview. Nanomaterials 8:681. doi: 10.3390/nano8090681
Cao, X., Zhu, L., Bai, Y., Li, F., and Yu, X. (2021). Green one-step synthesis of silver nanoparticles and their biosafety and antibacterial properties. Green Chem Lett Rev 15, 28–34. doi: 10.1080/17518253.2021.2018506
Chakravarty, A., Ahmad, I., Singh, P., Ud Din Sheikh, M., Aalam, G., Sagadevan, S., et al. (2022). Green synthesis of silver nanoparticles using fruits extracts of Syzygium cumini and their bioactivity. Chem. Phys. Lett. 795:139493. doi: 10.1016/j.cplett.2022.139493
Chauhan, A. K., Singh, S. P., Yadav, B., Khatri, S., and Husen, A. (2023). “Chapter 6—smart nanomaterials and control of biofilms” in Advances in smart nanomaterials and their applications. eds. A. Husen and K. S. Siddiqi (Amsterdam: Elsevier), 109–125.
Chen, A., Hernandez-Vargas, J., Han, R., Cortazar-Martínez, O., Gonzalez, N., Patel, S., et al. (2021). Small RNAs as a new platform for tuning the biosynthesis of silver nanoparticles for enhanced material and functional properties. ACS Appl. Mater. Interfaces 13, 36769–36783. doi: 10.1021/acsami.1c07400
Cheng, X., Xie, Q., and Sun, Y. (2023). Advances in nanomaterial-based targeted drug delivery systems. Front. Bioeng. Biotechnol. 11:23. doi: 10.3389/fbioe.2023.1177151
Chuy, G. P., Muraro, P. C. L., Viana, A. R., Pavoski, G., Espinosa, D. C. R., Vizzotto, B. S., et al. (2022). Green nanoarchitectonics of silver nanoparticles for antimicrobial activity against resistant pathogens. J. Inorg. Organomet. Polym. 32, 1213–1222. doi: 10.1007/s10904-021-02162-3
Dakal, T. C., Kumar, A., Majumdar, R. S., and Yadav, V. (2016). Mechanistic basis of antimicrobial actions of silver nanoparticles. Front. Microbiol. 7:1831. doi: 10.3389/fmicb.2016.01831
Dalzon, B., Torres, A., Diemer, H., Ravanel, S., Collin-Faure, V., Pernet-Gallay, K., et al. (2019). How reversible are the effects of silver nanoparticles on macrophages? A proteomic-instructed view. Environ. Sci. Nano 6, 3133–3157. doi: 10.1039/c9en00408d
Das, C. G. A., Kumar, V. G., Dhas, T. S., Karthick, V., Govindaraju, K., Joselin, J. M., et al. (2020). Antibacterial activity of silver nanoparticles (biosynthesis): A short review on recent advances. Biocatal. Agric. Biotechnol. 27:101593. doi: 10.1016/j.bcab.2020.101593
Dawadi, S., Katuwal, S., Gupta, A., Lamichhane, U., Thapa, R., Jaisi, S., et al. (2021). Current research on silver nanoparticles: synthesis, characterization, and applications. J. Nanomater. 2021, 1–23. doi: 10.1155/2021/6687290
Devanesan, S., AlSalhi, M. S., Balaji, R. V., Ranjitsingh, A. J. A., Ahamed, A., Alfuraydi, A. A., et al. (2018). Antimicrobial and cytotoxicity effects of synthesized silver nanoparticles from Punica granatum peel extract. Nanoscale Res. Lett. 13:315. doi: 10.1186/s11671-018-2731-y
Domingo, G., Villa, F., Vannini, C., Garuglieri, E., Onelli, E., Bracale, M., et al. (2019). Label-free proteomic approach to study the non-lethal effects of silver nanoparticles on a gut bacterium. Front. Microbiol. 10:2019. doi: 10.3389/fmicb.2019.02709
Dridi, R., Essghaier, B., Hannachi, H., Khedher, G. B., Chaffei, C., and Zid, M. F. (2022). Biosynthesized silver nanoparticles using Anagallis monelli: evaluation of antioxidant activity, antibacterial and antifungal effects. J. Mol. Struct. 1251:132076. doi: 10.1016/j.molstruc.2021.132076
Dutta, T., Ghosh, N. N., Das, M., Adhikary, R., Mandal, V., and Chattopadhyay, A. P. (2020). Green synthesis of antibacterial and antifungal silver nanoparticles using Citrus limetta peel extract: experimental and theoretical studies. J. Environ. Chem. Eng. 8:104019. doi: 10.1016/j.jece.2020.104019
El-Bendary, M. A., Afifi, S. S., Moharam, M. E., Abo El-Ola, S. M., Salama, A., Omara, E. A., et al. (2020). Biosynthesis of silver nanoparticles using isolated Bacillus subtilis: characterization, antimicrobial activity, cytotoxicity, and their performance as antimicrobial agent for textile materials. Prep. Biochem. Biotechnol. 51, 54–68. doi: 10.1080/10826068.2020.1789992
Elwakil, B. H., Eldrieny, A. M., Almotairy, A. R. Z., and El-Khatib, M. (2024). Potent biological activity of newly fabricated silver nanoparticles coated by a carbon shell synthesized by electrical arc. Sci. Rep. 14:5324. doi: 10.1038/s41598-024-54648-y
Esfahani, M. B., Khodavandi, A., Alizadeh, F., and Bahador, N. (2023). Antibacterial and anti-biofilm activities of microbial synthesized silver and magnetic iron oxide nanoparticles against Pseudomonas aeruginosa. IEEE Trans. Nanobioscience 22, 956–966. doi: 10.1109/TNB.2023.3268138
Feizi, S., Cooksley, C. M., Nepal, R., Psaltis, A. J., Wormald, P. J., and Vreugde, S. (2022). Silver nanoparticles as a bioadjuvant of antibiotics against biofilm-mediated infections with methicillin-resistant Staphylococcus aureus and Pseudomonas aeruginosa in chronic rhinosinusitis patients. Pathology 54, 453–459. doi: 10.1016/j.pathol.2021.08.014
Fernandes, M., González-Ballesteros, N., da Costa, A., Machado, R., Gomes, A. C., and Rodríguez-Argüelles, M. C. (2023). Antimicrobial and anti-biofilm activity of silver nanoparticles biosynthesized with Cystoseira algae extracts. J. Biol. Inorg. Chem. 28, 439–450. doi: 10.1007/s00775-023-01999-y
Feroze, N., Arshad, B., Younas, M., Afridi, M. I., Saqib, S., and Ayaz, A. (2020). Fungal mediated synthesis of silver nanoparticles and evaluation of antibacterial activity. Microsc. Res. Tech. 83, 72–80. doi: 10.1002/jemt.23390
Figueiredo, E. P., Ribeiro, J. M., Nishio, E. K., Scandorieiro, S., Costa, A. F., Cardozo, V. F., et al. (2019). New approach for simvastatin as an antibacterial: synergistic effect with bio-synthesized silver nanoparticles against multidrug-resistant bacteria. Int. J. Nanomedicine 14, 7975–7985. doi: 10.2147/IJN.S211756
Garibo, D., Borbón-Nuñez, H. A., de León, J. N. D., García Mendoza, E., Estrada, I., Toledano-Magaña, Y., et al. (2020). Green synthesis of silver nanoparticles using Lysiloma acapulcensis exhibit high-antimicrobial activity. Sci. Rep. 10:12805. doi: 10.1038/s41598-020-69606-7
Haji, S. H., Ali, F. A., and Aka, S. T. H. (2022). Synergistic antibacterial activity of silver nanoparticles biosynthesized by carbapenem-resistant gram-negative bacilli. Sci. Rep. 12:15254. doi: 10.1038/s41598-022-19698-0
Halsted, M. C., Bible, A. N., Morrell-Falvey, J. L., and Retterer, S. T. (2022). Quantifying biofilm propagation on chemically modified surfaces. Biofilms 4:100088. doi: 10.1016/j.bioflm.2022.100088
Hamad, A., Khashan, K. S., and Hadi, A. (2020). Silver nanoparticles and silver ions as potential antibacterial agents. J. Inorg. Organomet. Polym. Mater. 30, 4811–4828. doi: 10.1007/s10904-020-01744-x
Hasanin, M., Elbahnasawy, M. A., Shehabeldine, A. M., and Hashem, A. H. (2021). Ecofriendly preparation of silver nanoparticles-based nanocomposite stabilized by polysaccharides with antibacterial, antifungal and antiviral activities. Biometals 34, 1313–1328. doi: 10.1007/s10534-021-00344-7
Hassan, K. T., Ibraheem, I. J., Hassan, O. M., Obaid, A. S., Ali, H. H., Salih, T. A., et al. (2021). Facile green synthesis of ag/AgCl nanoparticles derived from Chara algae extract and evaluating their antibacterial activity and synergistic effect with antibiotics. J. Environ. Chem. Eng. 9:105359. doi: 10.1016/j.jece.2021.105359
Hemlata, M., Meena, P. R., Singh, A. P., and Tejavath, K. K. (2020). Biosynthesis of silver nanoparticles using Cucumis prophetarum aqueous leaf extract and their antibacterial and antiproliferative activity against cancer cell lines. ACS Omega 5, 5520–5528. doi: 10.1021/acsomega.0c00155
Honarmand, T., Sharif, A. P., Salehzadeh, A., Jalali, A., and Nikokar, I. (2022). Does conjugation of silver nanoparticles with Thiosemicarbazide increase their antibacterial properties? Microb. Drug Resist. 28, 293–305. doi: 10.1089/mdr.2020.0557
Hu, L., Yang, X., Yin, J., Rong, X., Huang, X., Yu, P., et al. (2021). Combination of AgNPs and domiphen is antimicrobial against biofilms of common pathogens. Int. J. Nanomedicine 16, 7181–7194. doi: 10.2147/IJN.S334133
Huang, W., Yan, M., Duan, H., Bi, Y., Cheng, X., and Yu, H. (2020). Synergistic antifungal activity of green synthesized silver nanoparticles and epoxiconazole against Setosphaeria turcica. J. Nanomater. 2020, 1–7. doi: 10.1155/2020/9535432
Huong, T. L., and Nguyen, N. T. (2019). Green synthesis, characterization and antibacterial activity of silver nanoparticles using Sapindus mukorossi fruit pericarp extract. Mater. Today: Proc. 42, 88–93. doi: 10.1016/j.matpr.2020.10.015
Husain, S., Nandi, A., Simnani, F. Z., Saha, U., Ghosh, A., Sinha, A., et al. (2023). Emerging trends in advanced translational applications of silver nanoparticles: A progressing dawn of nanotechnology. J. Funct. Biomater. 14:47. doi: 10.3390/jfb14010047
Jalab, J., Abdelwahed, W., Kitaz, A., and Al-Kayali, R. (2021). Green synthesis of silver nanoparticles using aqueous extract of Acacia cyanophylla and its antibacterial activity. Heliyon 7:e08033. doi: 10.1016/j.heliyon.2021.e08033
Jaswal, T., and Gupta, J. (2021). A review on the toxicity of silver nanoparticles on human health. Mater. Today Proc. 81, 859–863. doi: 10.1016/j.matpr.2021.04.266
Jiménez-Ramírez, A. J., Martínez-Martínez, R. E., Ayala-Herrera, J. L., Zaragoza-Contreras, E. A., Domínguez-Pérez, R. A., Reyes-López, S. Y., et al. (2021). Antimicrobial activity of silver nanoparticles against clinical biofilms from patients with and without dental caries. J. Nanomater. 2021, 1–13. doi: 10.1155/2021/5587455
Joshi, A. S., Singh, P., and Mijakovic, I. (2020). Interactions of gold and silver nanoparticles with bacterial biofilms: molecular interactions behind inhibition and resistance. Int. J. Mol. Sci. 21:7658. doi: 10.3390/ijms21207658
Joudeh, N., and Linke, D. (2022). Nanoparticle classification, physicochemical properties, characterization, and applications: a comprehensive review for biologists. J. Nanobiotechnol 20:262. doi: 10.1186/s12951-022-01477-8
Juling, S., Bachler, G., von Götz, N., Lichtenstein, D., Böhmert, L., Niedzwiecka, A., et al. (2016). In vivo distribution of nanosilver in the rat: the role of ions and de novo-formed secondary particles. Food Chem. Toxicol. 97, 327–335. doi: 10.1016/j.fct.2016.08.016
Kaiser, K. G., Delattre, V., Frost, V. J., Buck, G. W., Phu, J. V., Fernandez, T. G., et al. (2023). Nanosilver: an old antibacterial agent with great promise in the fight against antibiotic resistance. Antibiotics 12:1264. doi: 10.3390/antibiotics12081264
Katva, S., Das, S., Moti, H. S., Jyoti, A., and Kaushik, S. (2017). Antibacterial synergy of silver nanoparticles with gentamicin and chloramphenicol against Enterococcus faecalis. Pharmacogn. Mag. 13, S828–S833. doi: 10.4103/pm.pm_120_17
Kaur, A., Preet, S., Kumar, V., Kumar, R., and Kumar, R. (2019). Synergetic effect of vancomycin loaded silver nanoparticles for enhanced antibacterial activity. Colloid. Surface B 176, 62–69. doi: 10.1016/j.colsurfb.2018.12.043
Kaweeteerawat, C., Na Ubol, P., Sangmuang, S., Aueviriyavit, S., and Maniratanachote, R. (2017). Mechanisms of antibiotic resistance in bacteria mediated by silver nanoparticles. J. Toxicol. Env. Health A Curr Issues 80, 1276–1289. doi: 10.1080/15287394.2017.1376727
Kędziora, A., Speruda, M., Wernecki, M., Dudek, B., Kapczynska, K., Krzyżewska, E., et al. (2021). How bacteria change after exposure to silver nanoformulations: analysis of the genome and outer membrane proteome. Pathogens 10:817. doi: 10.3390/pathogens10070817
Kemala, P., Idroes, R., Khairan, K., Ramli, M., Jalil, Z., Idroes, G. M., et al. (2022). Green synthesis and antimicrobial activities of silver nanoparticles using Calotropis gigantea from Ie Seu-um geothermal area, Aceh Province, Indonesia. Molecules 27:5310. doi: 10.3390/molecules27165310
Keshari, A. K., Srivastava, R., Singh, P., Yadav, V. B., and Nath, G. (2020). Antioxidant and antibacterial activity of silver nanoparticles synthesized by Cestrum nocturnum. J. Ayurveda Integr. Med. 11, 37–44. doi: 10.1016/j.jaim.2017.11.003
Khalil, M. A., El-Shanshoury, A. E.-R. R., Alghamdi, M. A., Alsalmi, F. A., Mohamed, S. F., Sun, J., et al. (2022). Biosynthesis of silver nanoparticles by marine actinobacterium Nocardiopsis dassonvillei and exploring their therapeutic potentials. Front. Microbiol. 12:12. doi: 10.3389/fmicb.2021.705673
Khan, I., Saeed, K., and Khan, I. (2019). Nanoparticles: properties, applications and toxicities. Arab. J. Chem. 12, 908–931. doi: 10.1016/j.arabjc.2017.05.011
Khanal, L. N., Sharma, K. R., Paudyal, H., Parajuli, K., Dahal, B., Ganga, G. C., et al. (2022). Green synthesis of silver nanoparticles from root extracts of Rubus ellipticus Sm. and comparison of antioxidant and antibacterial activity. J. Nanomater. 2022, 1–11. doi: 10.1155/2022/1832587
Khane, Y., Benouis, K., Albukhaty, S., Sulaiman, G. M., Abomughaid, M. M., Al Ali, A., et al. (2022). Green synthesis of silver nanoparticles using aqueous Citrus limon zest extract: characterization and evaluation of their antioxidant and antimicrobial properties. Nanomaterials 12:2013. doi: 10.3390/nano12122013
Kukushkina, E. A., Hossain, S. I., Sportelli, M. C., Ditaranto, N., Picca, R. A., and Cioffi, N. (2021). Ag-based synergistic antimicrobial composites. A critical review. Nanomaterials 11:1687. doi: 10.3390/nano11071687
Kumar, S., Paliya, B. S., and Singh, B. N. (2022). Superior inhibition of virulence and biofilm formation of Pseudomonas aeruginosa PAO1 by phyto-synthesized silver nanoparticles through anti-quorum sensing activity. Microb. Pathog. 170:105678. doi: 10.1016/j.micpath.2022.105678
Labulo, A. H., David, O. A., and Terna, A. D. (2022). Green synthesis and characterization of silver nanoparticles using Morinda lucida leaf extract and evaluation of its antioxidant and antimicrobial activity. Chem. Pap. 76, 7313–7325. doi: 10.1007/s11696-022-02392-w
Lee, N. Y., Ko, W. C., and Hsueh, P. R. (2019). Nanoparticles in the treatment of infections caused by multidrug-resistant organisms. Front. Pharmacol. 10:1153. doi: 10.3389/fphar.2019.01153
Li, H., and Xu, H. (2024). Mechanisms of bacterial resistance to environmental silver and antimicrobial strategies for silver: A review. Environ. Res. 248:118313. doi: 10.1016/j.envres.2024.118313
Liao, S., Zhang, Y., Pan, X., Zhu, F., Jiang, C., Liu, Q., et al. (2019). Antibacterial activity and mechanism of silver nanoparticles against multidrug-resistant Pseudomonas aeruginosa. Int. J. Nanomedicine 14, 1469–1487. doi: 10.2147/IJN.S191340
Liaqat, N., Jahan, N., Khalil-ur-Rahman, A., and Qureshi, H. (2022). Green synthesized silver nanoparticles: optimization, characterization, antimicrobial activity, and cytotoxicity study by hemolysis assay. Front. Chem. 10:2006. doi: 10.3389/fchem.2022.952006
Lin, P., Wang, F. Q., Li, C. T., and Yan, Z. F. (2020). An enhancement of antibacterial activity and synergistic effect of biosynthesized silver nanoparticles by Eurotium cristatum with various antibiotics. Biotechnol. Bioproc. Eng. 25, 450–458. doi: 10.1007/s12257-019-0506-7
Liu, B., Liu, D., Chen, T., Wang, X., Xiang, H., Wang, G., et al. (2023). iTRAQ-based quantitative proteomic analysis of the antibacterial mechanism of silver nanoparticles against multidrug-resistant Streptococcus suis. Front. Microbiol. 14:1293363. doi: 10.3389/fmicb.2023.1293363
Loiseau, A., Asila, V., Boitel-Aullen, G., Lam, M., Salmain, M., and Boujday, S. (2019). Silver-based plasmonic nanoparticles for and their use in biosensing. Biosensors 9:78. doi: 10.3390/bios9020078
Lok, C. N., Ho, C. M., Chen, R., He, Q. Y., Yu, W. Y., Sun, H., et al. (2006). Proteomic analysis of the mode of antibacterial action of silver nanoparticles. J. Proteome Res. 5, 916–924. doi: 10.1021/pr0504079
Lu, J., Wang, Y., Jin, M., Yuan, Z., Bond, P., and Guo, J. (2020). Both silver ions and silver nanoparticles facilitate the horizontal transfer of plasmid-mediated antibiotic resistance genes. Water Res. 169:115229. doi: 10.1016/j.watres.2019.115229
Mahfouz, A. Y., Daigham, G. E., Radwan, A. M., and Mohamed, A. A. (2020). Eco-friendly and superficial approach for synthesis of silver nanoparticles using aqueous extract of Nigella sativa and Piper nigrum L. seeds for evaluation of their antibacterial, antiviral, and anticancer activities a focus study on its impact on seed germination and seedling growth of Vicia faba and Zea mays. Majallah al-ṣaydalīyah al-Miṣrīyah 19:401. doi: 10.4103/epj.epj_48_20
Mahmud, K. M., Hossain, M. M., Polash, S. A., Takikawa, M., Shakil, M. S., Uddin, M. F., et al. (2022). Investigation of antimicrobial activity and biocompatibility of biogenic silver nanoparticles synthesized using Syzigyum cymosum extract. ACS Omega 7, 27216–27229. doi: 10.1021/acsomega.2c01922
Maillard, J. Y., and Hartemann, P. (2013). Silver as an antimicrobial: facts and gaps in knowledge. Crit. Rev. Microbiol. 39, 373–383. doi: 10.3109/1040841X.2012.713323
Mann, R., Holmes, A., McNeilly, O., Cavaliere, R., Sotiriou, G. A., Rice, S. A., et al. (2021). Evolution of biofilm-forming pathogenic bacteria in the presence of nanoparticles and antibiotic: adaptation phenomena and cross-resistance. J. Nanobiotechnol. 19:291. doi: 10.1186/s12951-021-01027-8
Mazur, P., Skiba-Kurek, I., Mrowiec, P., Karczewska, E., and Drożdż, R. (2020). Synergistic ROS-associated antimicrobial activity of silver nanoparticles and gentamicin against Staphylococcus epidermidis. Int. J. Nanomedicine 15, 3551–3562. doi: 10.2147/IJN.S246484
Medici, S., Peana, M., Nurchi, V. M., and Zoroddu, M. A. (2019). Medical uses of silver: history, myths, and scientific evidence. J. Med. Chem. 62, 5923–5943. doi: 10.1021/acs.jmedchem.8b01439
Misirli, G. M., Sridharan, K., and Abrantes, S. M. P. (2021). A review on nanostructured silver as a basic ingredient in medicine: physicochemical parameters and characterization. Beilstein J. Nanotech. 12, 440–461. doi: 10.3762/BJNANO.12.36
Miškovská, A., Rabochová, M., Michailidu, J., Masák, J., Čejková, A., Lorinčík, J., et al. (2022). Antibiofilm activity of silver nanoparticles biosynthesized using viticultural waste. PLoS One 17:e0272844. doi: 10.1371/journal.pone.0272844
Mohanta, Y. K., Biswas, K., Jena, S. K., Hashem, A., Abd_Allah, E. F., and Mohanta, T. K. (2020). Anti-biofilm and antibacterial activities of silver nanoparticles synthesized by the reducing activity of phytoconstituents present in the indian medicinal plants. Front. Microbiol. 11:1143. doi: 10.3389/fmicb.2020.01143
Mondal, A. H., Yadav, D., Mitra, S., and Mukhopadhyay, K. (2020). Biosynthesis of silver nanoparticles using culture supernatant of Shewanella sp. ARY1 and their antibacterial activity. Int. J. Nanomedicine 15, 8295–8310. doi: 10.2147/IJN.S274535
Monedeiro-Milanowski, M., Monedeiro, F., and Pomastowski, P. (2023). Silver lactoferrin as antimicrobials: mechanisms of action and resistance assessed by bacterial molecular profiles. ACS Omega 8, 46236–46251. doi: 10.1021/acsomega.3c07562
Monych Nadia, K., and Turner Raymond, J. (2020). Multiple compounds secreted by Pseudomonas aeruginosa increase the tolerance of Staphylococcus aureus to the antimicrobial metals copper and silver. mSystems 5:20. doi: 10.1128/mSystems.00746-20
Morales-Lozoya, V., Espinoza-Gómez, H., Flores-López, L., Sotelo-Barrera, E. L., Núñez-Rivera, A., Cadena-Nava, R. D., et al. (2021). Study of the effect of the different parts of Morinda citrifolia L. (noni) on the green synthesis of silver nanoparticles and their antibacterial activity. Appl. Surf. Sci. 537:147855. doi: 10.1016/j.apsusc.2020.147855
More, P. R., Pandit, S., Filippis, A. D., Franci, G., Mijakovic, I., and Galdiero, M. (2023). Silver nanoparticles: bactericidal and mechanistic approach against drug resistant pathogens. Microorganisms. 11:369. doi: 10.3390/microorganisms11020369
Nasrollahzadeh, M., Sajjadi, M., Sajadi, S. M., and Issaabadi, Z. (2019). “Chapter 5—green nanotechnology” in An introduction to green nanotechnology. eds. M. Nasrollahzadeh, S. M. Sajadi, M. Sajjadi, Z. Issaabadi, and M. Atarod (London: Elsevier), 145–198.
Nie, T., Liu, G., Xiao, Y., Yari, H., and Goorani, S. (2023). Introducing a novel and natural antibiotic for the treatment of oral pathogens: Abelmoschus esculentus green-formulated silver nanoparticles. Open Chem. 21:20220349. doi: 10.1515/chem-2022-0349
Nikparast, Y., and Saliani, M. (2018). Synergistic effect between phyto-syntesized silver nanoparticles and ciprofloxacin antibiotic on some pathogenic bacterial strains. J. Med. Bacteriol. 7:36.
Niloy, M. S., Hossain, M. M., Takikawa, M., Shakil, M. S., Polash, S. A., Mahmud, K. M., et al. (2020). Synthesis of biogenic silver nanoparticles using Caesalpinia digyna and investigation of their antimicrobial activity and in vivo biocompatibility. ACS Appl Bio Mater 3, 7722–7733. doi: 10.1021/acsabm.0c00926
Palau, M., Muñoz, E., Gusta, M. F., Larrosa, N., Gomis, X., Gilabert, J., et al. (2023). In vitro antibacterial activity of silver nanoparticles conjugated with amikacin and combined with hyperthermia against drug-resistant and biofilm-producing strains. Microbiol. Spectr. 11, 1–16. doi: 10.1128/spectrum.00280-23
Panáček, A., Kvítek, L., Smékalová, M., Večeřová, R., Kolář, M., Röderová, M., et al. (2018). Bacterial resistance to silver nanoparticles and how to overcome it. Nat. Nanotechnol. 13, 65–71. doi: 10.1038/s41565-017-0013-y
Parvathalu, K., Chinmayee, S., Preethi, B., Swetha, A., Maruthi, G., Pritam, M., et al. (2023). Green synthesis of silver nanoparticles using Argyreia nervosa leaf extract and their antimicrobial activity. Plasmonics 18, 1075–1081. doi: 10.1007/s11468-023-01835-8
Patil, R. B., and Chougale, A. D. (2021). Analytical methods for the identification and characterization of silver nanoparticles: A brief review. Today Proc. 47, 5520–5532. doi: 10.1016/j.matpr.2021.03.384
Piatek, M., O’Beirne, C., Beato, Z., Tacke, M., and Kavanagh, K. (2023). Pseudomonas aeruginosa and Staphylococcus aureus display differential proteomic responses to the silver(i) compound, sbc3. Antibiotics 12:348. doi: 10.3390/antibiotics12020348
Pon Janani, S., Thillai Arasu, P., Muzaddadi, I. U., Murugan, A., Ravikumar, C. R., Yadav, N. N., et al. (2022). “Chapter 14—photodynamic therapy with nanomaterials to combat microbial infections” in Emerging nanomaterials and Nano-based drug delivery approaches to combat antimicrobial resistance. eds. M. Saravanan, H. Barabadi, E. Mostafavi, and T. Webster (Amsterdam: Elsevier), 531–576.
Prabhu, S., and Poulose, E. K. (2012). Silver nanoparticles: mechanism of antimicrobial. Int. Nano Lett. 2:32. doi: 10.1186/2228-5326-2-32
Pryshchepa, O., Pomastowski, P., and Buszewski, B. (2020). Silver nanoparticles: synthesis, investigation techniques, and properties. Adv. Colloid Interface Sci. 284:102246. doi: 10.1016/j.cis.2020.102246
Pungle, R., Nile, S. H., and Kharat, A. S. (2021). Green synthesis and characterization of Solanum xanthocarpum capped silver nanoparticles and its antimicrobial effect on multidrug-resistant bacterial (MDR) isolates. Chem. Biol. Drug Des. 101, 469–478. doi: 10.1111/cbdd.13945
Rakib-Uz-Zaman, S. M., Hoque Apu, E., Muntasir, M. N., Mowna, S. A., Khanom, M. G., Jahan, S. S., et al. (2022). Biosynthesis of silver nanoparticles from Cymbopogon citratus leaf extract and evaluation of their antimicrobial properties. Challenges 13:18. doi: 10.3390/challe13010018
Ramzan, M., Karobari, M. I., Heboyan, A., Mohamed, R. N., Mustafa, M., Basheer, S. N., et al. (2022). Synthesis of silver nanoparticles from extracts of wild ginger (Zingiber zerumbet) with antibacterial activity against selective multidrug resistant oral bacteria. Molecules 27:2007. doi: 10.3390/molecules27062007
Ren, Q., Ma, J., Li, X., Meng, Q., Wu, S., Xie, Y., et al. (2023). Intestinal toxicity of metal nanoparticles: silver nanoparticles disorder the intestinal immune microenvironment. ACS Appl. Mater. Interfaces 15, 27774–27788. doi: 10.1021/acsami.3c05692
Renganathan, S., Subramaniyan, S., Karunanithi, N., Vasanthakumar, P., Kutzner, A., Kim, P. S., et al. (2021). Antibacterial, antifungal, and antioxidant activities of silver nanoparticles biosynthesized from Bauhinia tomentosa Linn. Antioxidants 10:1959. doi: 10.3390/antiox10121959
Renuka, R., Devi, K. R., Sivakami, M., Thilagavathi, T., Uthrakumar, R., and Kaviyarasu, K. (2020). Biosynthesis of silver nanoparticles using Phyllanthus emblica fruit extract for antimicrobial application. Biocatal. Agric. Biotechnol. 24:101567. doi: 10.1016/j.bcab.2020.101567
Ribeiro, A. I., Dias, A. M., and Zille, A. (2022). Synergistic effects between metal nanoparticles and commercial antimicrobial agents: A review. ACS Appl. Nano Mater. 5, 3030–3064. doi: 10.1021/acsanm.1c03891
Rodríguez-Félix, F., López-Cota, A. G., Moreno-Vásquez, M. J., Graciano-Verdugo, A. Z., Quintero-Reyes, I. E., Del-Toro-Sánchez, C. L., et al. (2021). Sustainable-green synthesis of silver nanoparticles using safflower (Carthamus tinctorius L.) waste extract and its antibacterial activity. Heliyon 7:e06923. doi: 10.1016/j.heliyon.2021.e06923
Salas-Orozco, M. F., Niño-Martínez, N., Martínez-Castañón, G. A., Méndez, F. T., Morán, G. M. M., Bendaña-Piñeiro, A. E., et al. (2022). Proteomic analysis of an Enterococcus faecalis mutant generated against the exposure to silver nanoparticles. J. Appl. Microbiol. 132, 244–255. doi: 10.1111/jam.15182
Salleh, A., Naomi, R., Utami, N. D., Mohammad, A. W., Mahmoudi, E., Mustafa, N., et al. (2020). The potential of silver nanoparticles for antiviral and antibacterial applications: A mechanism of action. Nanomaterials 10:1566. doi: 10.3390/nano10081566
Sánchez-López, E., Gomes, D., Esteruelas, G., Bonilla, L., Lopez-Machado, A. L., Galindo, R., et al. (2020). Metal-based nanoparticles as antimicrobial agents: an overview. Nanomaterials 10:292. doi: 10.3390/nano10020292
Sarwer, Q., Amjad, M. S., Mehmood, A., Binish, Z., Mustafa, G., Farooq, A., et al. (2022). Green synthesis and characterization of silver nanoparticles using Myrsine africana leaf extract for their antibacterial, antioxidant and phytotoxic activities. Molecules 27:2186. doi: 10.3390/molecules27217612
Selem, E., Mekky, A. F., Hassanein, W. A., Reda, F. M., and Selim, Y. A. (2022). Antibacterial and antibiofilm effects of silver nanoparticles against the uropathogen Escherichia coli U12. Saudi J. Biol. Sci. 29:103457. doi: 10.1016/j.sjbs.2022.103457
Sellami, H., Khan, S. A., Ahmad, I., Alarfaj, A. A., Hirad, A. H., and Al-Sabri, A. E. (2021). Green synthesis of silver nanoparticles using Olea europaea leaf extract for their enhanced antibacterial, antioxidant, cytotoxic and biocompatibility applications. Int. J. Mol. Sci. 22:12562. doi: 10.3390/ijms222212562
Senthilkumar, P., Rashmitha, S., Veera, P., Vijay Ignatious, C., SaiPriya, C., and Samrot, A. V. (2018). Antibacterial activity of neem extract and its green synthesized silver nanoparticles against Pseudomonas aeruginosa. J. Pure Appl. Microbiol. 12, 969–974. doi: 10.22207/JPaM.12.2.60
Seo, M., Oh, T., and Bae, S. (2021). Antibiofilm activity of silver nanoparticles against biofilm forming Staphylococcus pseudintermedius isolated from dogs with otitis externa. Vet. Med. Sci. 7, 1551–1557. doi: 10.1002/vms3.554
Shaik, M. R., Khan, M., Kuniyil, M., Al-Warthan, A., Alkhathlan, H. Z., Siddiqui, M. R. H., et al. (2018). Plant-extract-assisted green synthesis of silver nanoparticles using Origanum vulgare L. extract and their microbicidal activities. Sustainability 10:913. doi: 10.3390/su10040913
Sharma, A., Sagar, A., Rana, J., and Rani, R. (2022). Green synthesis of silver nanoparticles and its antibacterial activity using fungus Talaromyces purpureogenus isolated from Taxus baccata Linn. Micro Nano Syst Lett 10:2. doi: 10.1186/s40486-022-00144-9
Shehabeldine, A. M., Salem, S. S., Ali, O. M., Abd-Elsalam, K. A., Elkady, F. M., and Hashem, A. H. (2022). Multifunctional silver nanoparticles based on chitosan: antibacterial, antibiofilm, antifungal, antioxidant, and wound-healing activities. J. Fungi 8:612. doi: 10.3390/jof8060612
Shumi, G., Demissie, T. B., Eswaramoorthy, R., Bogale, R. F., Kenasa, G., and Desalegn, T. (2023). Biosynthesis of silver nanoparticles functionalized with histidine and phenylalanine amino acids for potential antioxidant and antibacterial activities. ACS Omega 8, 24371–24386. doi: 10.1021/acsomega.3c01910
Singh, R., Hano, C., Nath, G., and Sharma, B. (2021). Green biosynthesis of silver nanoparticles using leaf extract of Carissa carandas L. and their antioxidant and antimicrobial activity against human pathogenic bacteria. Biomol. Ther. 11:299. doi: 10.3390/biom11020299
Singh, R., Wagh, P., Wadhwani, S., Gaidhani, S., Kumbhar, A., Bellare, J., et al. (2013). Synthesis, optimization, and characterization of silver nanoparticles from Acinetobacter calcoaceticus and their enhanced antibacterial activity when combined with antibiotics. Int. J. Nanomedicine 8, 4277–4290. doi: 10.2147/IJN.S48913
Ssekatawa, K., Byarugaba, D. K., Kato, C. D., Wampande, E. M., Ejobi, F., Nakavuma, J. L., et al. (2021). Green strategy–based synthesis of silver nanoparticles for antibacterial applications. Front. Nanotec. 3:697303. doi: 10.3389/fnano.2021.697303
Stabryla, L. M., Johnston, K. A., Diemler, N. A., Cooper, V. S., Millstone, J. E., Haig, S. J., et al. (2021). Role of bacterial motility in differential resistance mechanisms of silver nanoparticles and silver ions. Nat. Nanotechnol. 16, 996–1003. doi: 10.1038/s41565-021-00929-w
Sun, P., Ye, L., Tan, X., Peng, J., Zhao, L., and Zhou, Y. (2022). Silver nanoparticle-assisted photodynamic therapy for biofilm eradication. ACS Appl. Nano Mater. 5, 8251–8259. doi: 10.1021/acsanm.2c01327
Swidan, N. S., Hashem, Y. A., Elkhatib, W. F., and Yassien, M. A. (2022). Antibiofilm activity of green synthesized silver nanoparticles against biofilm associated enterococcal urinary pathogens. Sci. Rep. 12:3869. doi: 10.1038/s41598-022-07831-y
Swolana, D., Kępa, M., Kruszniewska-Rajs, C., and Wojtyczka, R. D. (2022). Antibiofilm effect of silver nanoparticles in changing the biofilm-related gene expression of Staphylococcus epidermidis. Int. J. Mol. Sci. 23:257. doi: 10.3390/ijms23169257
Toranzo, A., Bustos, P. S., Ortega, M. G., Páez, P. L., and Lucero-Estrada, C. (2022). Biologically synthesized silver nanoparticles, mediated by Bothriochloa laguroides, inhibit biofilm formation and eradicate mature biofilm of Yersinia enterocolitica and Staphylococcus aureus. J. Appl. Microbiol. 132, 209–220. doi: 10.1111/jam.15195
Torres-Mendieta, R., Nguyen, N. H. A., Guadagnini, A., Semerad, J., Łukowiec, D., Parma, P., et al. (2022). Growth suppression of bacteria by biofilm deterioration using silver nanoparticles with magnetic doping. Nanoscale 14, 18143–18156. doi: 10.1039/d2nr03902h
Tseng, K. H., Chen, Y. C., and Shyue, J. J. (2011). Continuous synthesis of colloidal silver nanoparticles by electrochemical discharge in aqueous solutions. J. Nanopart. Res. 13, 1865–1872. doi: 10.1007/s11051-010-9937-y
Ulagesan, S., Nam, T.-J., and Choi, Y.-H. (2021). Biogenic preparation and characterization of Pyropia yezoensis silver nanoparticles (P.Y AgNPs) and their antibacterial activity against Pseudomonas aeruginosa. Bioprocess Biosyst. Eng. 44, 443–452. doi: 10.1007/s00449-020-02454-x
Urnukhsaikhan, E., Bold, B. E., Gunbileg, A., Sukhbaatar, N., and Mishig-Ochir, T. (2021). Antibacterial activity and characteristics of silver nanoparticles biosynthesized from Carduus crispus. Sci. Rep. 11:21047. doi: 10.1038/s41598-021-00520-2
Veeraraghavan, V. P., Periadurai, N. D., Karunakaran, T., Hussain, S., Surapaneni, K. M., and Jiao, X. (2021). Green synthesis of silver nanoparticles from aqueous extract of Scutellaria barbata and coating on the cotton fabric for antimicrobial applications and wound healing activity in fibroblast cells (L929). Saudi J. Biol. Sci. 28, 3633–3640. doi: 10.1016/j.sjbs.2021.05.007
Wang, L., Liu, L., and Zhou, X. (2023). Novel mercaptide-coupled silver nanoparticles AgDAMP against Salmonella through membrane disruption, biofilm obstruction, and protein expression alteration. Food Bioproc. Tech. 2023, 1–22. doi: 10.1007/s11947-023-03256-1
Wang, L., Periyasami, G., Aldalbahi, A., and Fogliano, V. (2021). The antimicrobial activity of silver nanoparticles biocomposite films depends on the silver ions release behaviour. Food Chem. 359:129859. doi: 10.1016/j.foodchem.2021.129859
Wang, H., Wang, M., Xu, X., Gao, P., Xu, Z., Zhang, Q., et al. (2021). Multi-target mode of action of silver against Staphylococcus aureus endows it with capability to combat antibiotic resistance. Nat. Commun. 12:3331. doi: 10.1038/s41467-021-23659-y
Wasilewska, A., Klekotka, U., Zambrzycka, M., Zambrowski, G., Święcicka, I., and Kalska-Szostko, B. (2022). Physico-chemical properties and antimicrobial activity of silver nanoparticles fabricated by green synthesis. Food Chem. 400:133960. doi: 10.1016/j.foodchem.2022.133960
Widatalla, H. A., Yassin, L. F., Alrasheid, A. A., Rahman Ahmed, S. A., Widdatallah, M. O., Eltilib, S. H., et al. (2022). Green synthesis of silver nanoparticles using green tea leaf extract, characterization and evaluation of antimicrobial activity. Nanoscale Adv. 4, 911–915. doi: 10.1039/d1na00509j
Xia, F., Tao, X., Wang, H., Shui, J., Min, C., Xia, Y., et al. (2023). Biosynthesis of silver nanoparticles using the biofilm supernatant of Pseudomonas aeruginosa PA75 and evaluation of their antibacterial, antibiofilm, and antitumor activities. Int. J. Nanomedicine 18, 2485–2502. doi: 10.2147/IJN.S410314
Xu, J., Li, Y., Wang, H., Zhu, M., Feng, W., and Liang, G. (2021). Enhanced antibacterial and anti-biofilm activities of antimicrobial peptides modified silver nanoparticles. Int. J. Nanomedicine 16, 4831–4846. doi: 10.2147/IJN.S315839
Yadav, R., and Preet, S. (2023). Comparative assessment of green and chemically synthesized glutathione capped silver nanoparticles for antioxidant, mosquito larvicidal and eco-toxicological activities. Sci. Rep. 13:8152. doi: 10.1038/s41598-023-35249-7
Yan, X., He, B., Liu, L., Qu, G., Shi, J., Hu, L., et al. (2018). Antibacterial mechanism of silver nanoparticles in Pseudomonas aeruginosa: proteomics approach. Metallomics 10, 557–564. doi: 10.1039/c7mt00328e
Yan, Y., Liu, Y., Li, J., Li, Y., Wu, H., Li, H., et al. (2023). A molecular switch-integrated nanoplatform enables photo-unlocked antibacterial drug delivery for synergistic abscess therapy. Adv. Healthc. Mater. 12:e2301157. doi: 10.1002/adhm.202301157
Yassin, M. T., Mostafa, A. A. F., Al-Askar, A. A., and Al-Otibi, F. O. (2022). Synergistic antibacterial activity of green synthesized silver nanomaterials with colistin antibiotic against multidrug-resistant bacterial pathogens. Crystals 12:1057. doi: 10.3390/cryst12081057
Yılmaz Öztürk, B., Yenice Gürsu, B., and Dağ, İ. (2020). Antibiofilm and antimicrobial activities of green synthesized silver nanoparticles using marine red algae Gelidium corneum. Process Biochem. 89, 208–219. doi: 10.1016/j.procbio.2019.10.027
Yonathan, K., Mann, R., Mahbub, K. R., and Gunawan, C. (2022). The impact of silver nanoparticles on microbial communities and antibiotic resistance determinants in the environment. Environ. Pollut. 293:118506. doi: 10.1016/j.envpol.2021.118506
Yu, X., Li, J., Mu, D., Zhang, H., Liu, Q., and Chen, G. (2021). Green synthesis and characterizations of silver nanoparticles with enhanced antibacterial properties by secondary metabolites of Bacillus subtilis (SDUM301120). Green Chem Lett Rev 14, 190–203. doi: 10.1080/17518253.2021.1894244
Zhang, X. F., Liu, Z. G., Shen, W., and Gurunathan, S. (2016). Silver nanoparticles: synthesis, characterization, properties, applications, and therapeutic approaches. Int. J. Mol. Sci. 17:1534. doi: 10.3390/ijms17091534
Zhang, Y., Pan, X., Liao, S., Jiang, C., Wang, L., Tang, Y., et al. (2020). Quantitative proteomics reveals the mechanism of silver nanoparticles against multidrug-resistant Pseudomonas aeruginosa biofilms. J. Proteome Res. 19, 3109–3122. doi: 10.1021/acs.jproteome.0c00114
Keywords: nanomaterials, silver nanoparticles, antimicrobial, antibiofilm, antimicrobial resistance, mechanism of action, proteomic analysis, protein expression
Citation: Rodrigues AS, Batista JGS, Rodrigues MÁV, Thipe VC, Minarini LAR, Lopes PS and Lugão AB (2024) Advances in silver nanoparticles: a comprehensive review on their potential as antimicrobial agents and their mechanisms of action elucidated by proteomics. Front. Microbiol. 15:1440065. doi: 10.3389/fmicb.2024.1440065
Edited by:
Miklos Fuzi, Independent Researcher, Seattle, WA, United StatesReviewed by:
Runhua Han, University of Manitoba, CanadaDibyajit Lahiri, University of Engineering and Management, India
Copyright © 2024 Rodrigues, Batista, Rodrigues, Thipe, Minarini, Lopes and Lugão. This is an open-access article distributed under the terms of the Creative Commons Attribution License (CC BY). The use, distribution or reproduction in other forums is permitted, provided the original author(s) and the copyright owner(s) are credited and that the original publication in this journal is cited, in accordance with accepted academic practice. No use, distribution or reproduction is permitted which does not comply with these terms.
*Correspondence: Adriana S. Rodrigues, YXNvdXphckB1c3AuYnI=