- College of Animal Science and Technology, Shihezi University, Shihezi, China
We evaluated the effects of cottonseed meal protein hydrolysate (CPH) on the intestinal microbiota of yellow-feather broilers. We randomly divided 240 chicks into four groups with six replicates: basal diet with 0% (CON), 1% (LCPH), 3% (MCPH), or 5% (HCPH) CPH. The test lasted 63 days and included days 1–21, 22–42, and 43–63 phases. The ACE, Chao1, and Shannon indices in the MCPH and HCPH groups of 42-day-old broilers were higher than those in the CON group (p < 0.05), indicating that the cecum microbial diversity and richness were higher in these groups. Firmicutes and Bacteroidetes were the dominant phyla; however, the main genera varied during the different periods. The abundance of Lactobacillus in CPH treatment groups of 21-day-old broilers was high (p < 0.05); in the 42-day-old broilers, the abundances of Barnesiella, Clostridia_vadinBB60_group, and Parasutterella in the LCPH group, Desulfovibrio, Lactobacillus, Clostridia_vadinBB60_group, and Butyricicoccus in the MCPH group, and Megamonas and Streptococcus in the HCPH group increased; in the 63-day-old broilers, the abundance of Clostridia_UCG-014 and Synergistes in the LCPH and HCPH group, respectively, increased (p < 0.05), and that of Alistipes in the LCPH and MCPH groups decreased (p < 0.05). And changes in the abundance of probiotics were beneficial to improve the intestinal morphology and growth performance. In addition, the LCPH treatment increased the complexity of the microbial network, while the MCPH treatment had the same effect in 42-day-old broilers. Thus, CPH increased the relative abundance of beneficial intestinal microbiota and enhanced the richness and diversity of the bacterial microbiota in broilers aged <42 days; this effect was weakened after 42 days.
1 Introduction
Antibiotics are widely used in poultry production; however, their continued use can lead to the emergence of antibiotic-resistant pathogens, which in turn can adversely affect human and animal health (Zhang et al., 2022). Therefore, the development of substitutes for antibiotics for animal husbandry has attracted considerable attention, with protein hydrolysates (PH) mooted as possible replacements for antibiotics (Zhang et al., 2024). PH, which can be produced by enzymatic hydrolysis, contribute largely to the health benefits of food (Tang et al., 2023). Owing to its efficiency and safety, enzymatic hydrolysis is currently one of the most promising methods for preparing peptides. As shown previously, PH may contain prebiotic peptides such as tripeptides (Glu-Leu-Met) (Arihara, 2006; Brody, 2000; Safari et al., 2012). Cottonseed meal protein hydrolysate (CPH) is a product of the enzymolysis of cottonseed meal protein, which possesses antioxidant, antibacterial, angiotensin-I-converting enzyme inhibitory activities and benefificial pharmacological properties (de Oliveira Filho et al., 2021; Gao et al., 2010; Mahdavi-Yekta et al., 2023; Ozturk-Kerimoglu et al., 2023; Sharma and Thakur, 2024; Song et al., 2020; Wang et al., 2021) and exerts a positive effect on animals (Alizadeh-Ghamsari et al., 2023; Cheng et al., 2019; Gisbert et al., 2021; Gui et al., 2010; Hartnoll, 2001; Tolba et al., 2023); thus, it is expected to be one of substitutes for antibiotics.
The intestinal microbiota plays an important role in animals, and the poultry intestinal microbiota responds to environmental changes (Ma et al., 2021; Singh et al., 2022; Stanley et al., 2013, 2014). The human microbiota can respond rapidly to changes in diet, which is the most influential variable (Candela et al., 2012; Turnbaugh et al., 2009). The effect of the intestinal microbiota on weight gain and productivity has been well documented in both humans and poultry (Abudabos et al., 2017; Ridaura et al., 2013; van Kuijk et al., 2021). The cecum, an important part of the gastrointestinal tract that is the site of most fermentations, significantly affects intestinal health and animal nutrition (Zhang et al., 2022).
Although CPH has been studied in the feed of agricultural animals, to date, its effects on the intestinal microbial populations in chickens have not been evaluated. This study was designed to evaluate the effects of graded levels of CPH on the intestinal microbial populations of yellow-feather broilers and provide a theoretical reference for the development of biological protein feeds.
2 Materials and methods
2.1 Ethics statement
Ethical requirements and recommendations for animal care for the experiment were approved by the Biology Ethics Committee of Shihezi university (Xinjiang, China) (no. A2021-47).
2.2 Preparation of CPH
Cottonseed meal protein (CP; protein purity, 91.03%) was isolated from cottonseed meal and hydrolyzed using 1:1 ratio of neutral protease (CAS:9068-59-1; Beijing Solarbio Science and Technology Co. Ltd., China) and papain (CAS:9001-73-4; Beijing Biotoppted Science and Technology Co. Ltd., China) (each enzyme/substrate ratio was 6,000 IU g−1) at 49.65°C and pH 6.99 for 2.83 h. CPH was heated at 95°C for 10 min to stop the reaction and was freeze-dried.
2.3 Diets and experimental design
The experimental diets used in this study are shown in Table 1. Four experimental diets supplemented with different concentrations (0, 1, 3, and 5%) of CPH were formulated for each trial period. Low CPH (LCPH), Medium CPH (MCPH) and High CPH (HCPH) group were supplemented with 1, 3 and 5% CPH, respectively. In total of 240 1-day-old healthy male yellow-feather chickens (initial weight 40.75 ± 1.27 g) were purchased from a commercial breeding base (Shihezi, China) and randomly distributed into four groups with six replicates of 10 birds. The test lasted 63 days and included three phases: days 1–21, 22–42, and 43–63.
2.4 Gut microbiota study
2.4.1 DNA extraction and polymerase chain reaction (PCR)
Cecal samples were collected immediately after the broilers were euthanized. Total bacterial DNA was extracted from the cecal samples using the E.Z.N.A.® soil DNA kit (Omega Bio-Tek, Norcross). The quantity and quality of the extracted DNA were determined by electrophoresing on a 1.0% agarose gel and using a NanoDrop 2000 UV–visible spectrophotometer, respectively (Thermo Scientific, Wilmington, NC, United States). The V3-V4 region of the 16S rRNA was amplified using PCR with forward (ACTCCTACGGGAGGCAGCAG) and reverse primers (GGACTACHVGGGTWTCTAAT). The PCR mixture included 5× FastPfu buffer (4 μL), dNTPs (2 μL), primers (0.8 μL × 2), FastPfu polymerase (0.4 μL), bovine serum albumin (0.2 μL), DNA template (10 ng), and ddH2O (to make up to a final volume of 20 μL). The amplification conditions were as follows: initial denaturation at 95°C for 3 min; 27 cycles of denaturation at 95°C for 30 s, 55°C for 30 s, and 72°C for 45 s; followed by holding at 72°C for 10 min. The resulting PCR products were analyzed using electrophoresis on a 2% agarose gel.
2.4.2 High-throughput sequencing
The purified amplicons were pooled in equimolar amounts, and double-end sequencing was performed using the Illumina MiSeq PE300 platform (Illumina, San Diego, CA, United States) according to the standard protocols of Majorbio Bio-Pharm Technology Co., Ltd. (Shanghai, China). The sequences were submitted to NCBI under the accession number PRJNA1031419.
2.4.3 Bioinformatics analysis
Operational taxonomic units (OTUs) were obtained based on a 97% similarity cut-off using UPARSE (version 11) after mass filtering and removal of chimeric sequences. The taxonomy of each 16S rRNA gene sequence was analyzed using the Ribosomal Database Project Classifier algorithm (version 11.5) against the Silva 16S rRNA database (version 138).
2.5 Statistical analysis
Bioinformatics analysis of the gut microbiota was performed using the Majorbio Cloud platform. Based on the OTU information, rarefaction curves and alpha diversity indices were calculated using Mothur (version 1.30.2). Similarity was determined using principal coordinate analysis (PCoA) based on Bray–Curtis dissimilarity in the Vegan package of the R (V3.3.1) software. Non-metric multidimensional scaling (NMDS) analysis was conducted using the Vegan package in the R (V3.3.1) software. Partial least squares discriminant analysis (PLS-DA) was performed using the mixOmics package in the R (V3.3.1) software. The correlation network of cecal microorganisms was constructed using Networkx software (version 1.11).
3 Results and analysis
3.1 Sequencing results of the cecal microflora
In this study, 3,588,955 high-quality reads were acquired from 72 cecal chyme samples, with an average of 49,847 sequences per sample. There were 1,079,687, 1,206,267, and 1,303,001 high-quality reads with averages of 44,987, 50,261, and 54,292 sequences per sample in 21-, 42-, and 63-day-old yellow-feather broilers, respectively. As shown in Figure 1, after quality and chimera checking, 344 shared OTUs were determined (with 97% similarity) in 21-day-old broilers, and 46, 21, 31, and 171 characteristic OTUs were found for in the CON, LCPH, MCPH, and HCPH groups, respectively; 573 shared OTUs were determined (with 97% similarity) in 42-day-old broilers, and 20, 40, 33, and 35 characteristic OTUs were found for in the CON, LCPH, MCPH, and HCPH groups, respectively; and 742 shared OTUs were determined (with 97% similarity) in 63-day-old broilers, and 31, 21, 19, and 23 characteristic OTUs were found in the CON, LCPH, MCPH, and HCPH groups, respectively. The dilution curve of each sample reached a plateau stage, indicating that the sequencing depth was sufficient to reflect the vast majority of microbial diversity information in the samples.
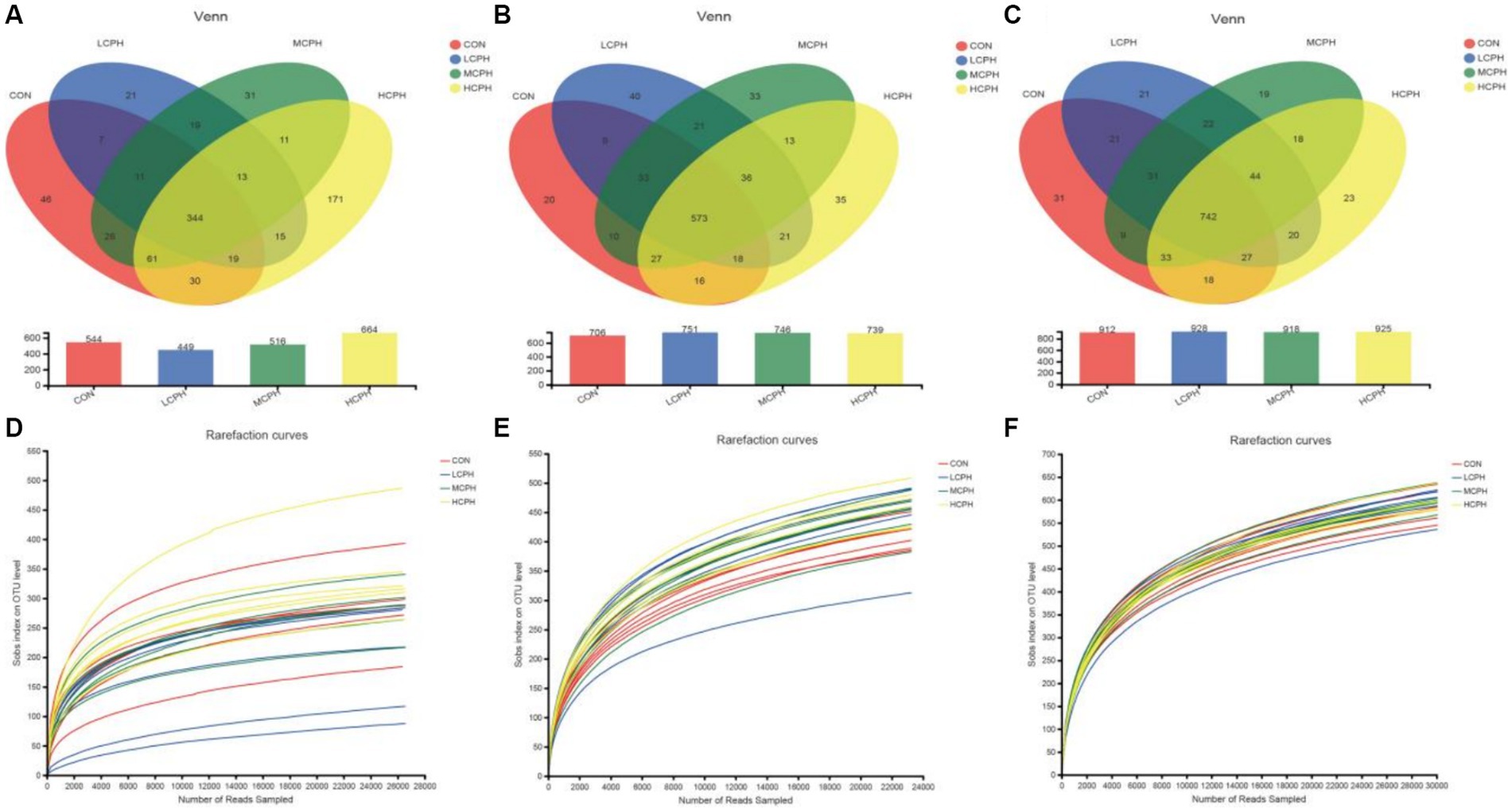
Figure 1. Venn diagrams and dilution curve of dietary treatments at the OTUs level. (A–C) Venn diagrams in 21-day-old, 42-day-old, and 63-day-old yellow-feather broilers, respectively. (D–F) Dilution curve in 21-day-old, 42-day-old, and 63-day-old yellow-feather broilers, respectively. CON, basal diet; LCPH, diet with 1% CPH; MCPH, diet with 3% CPH; HCPH, diet with 5% CPH.
3.2 Diversity analysis of cecal microbiota
3.2.1 Effect of CPH on cecal microbial alpha diversity
The effects of CPH on cecal microbial alpha diversity are shown in Figure 2. The coverage rate of each group was >99%, indicating that the probability of sequence detection in the sample was very high, whereas that of not being detected was very low. The sequencing results represented the actual conditions of the microorganisms in the sample. Alpha diversity represents the abundance and diversity of species in each sample. No significant differences were observed in the ACE, Chao1, Simpson, and Shannon indices of the LCPH, MCPH, and HCPH groups compared with that of the CON group, while the ACE and Chao1 indices of the HCPH group were significantly higher than those of the LCPH group in 21-day-old broilers. The ACE, Chao1 and Shannon indices of the MCPH and HCPH groups were significantly higher than those of the CON group, whereas the Simpson index did not differ significantly among the four treatments, indicating that cecum microbial diversity and richness were higher in the MCPH and HCPH groups of the 42-day-old broilers. Alpha diversity did not differ significantly among the four treatments in 63-day-old broilers.
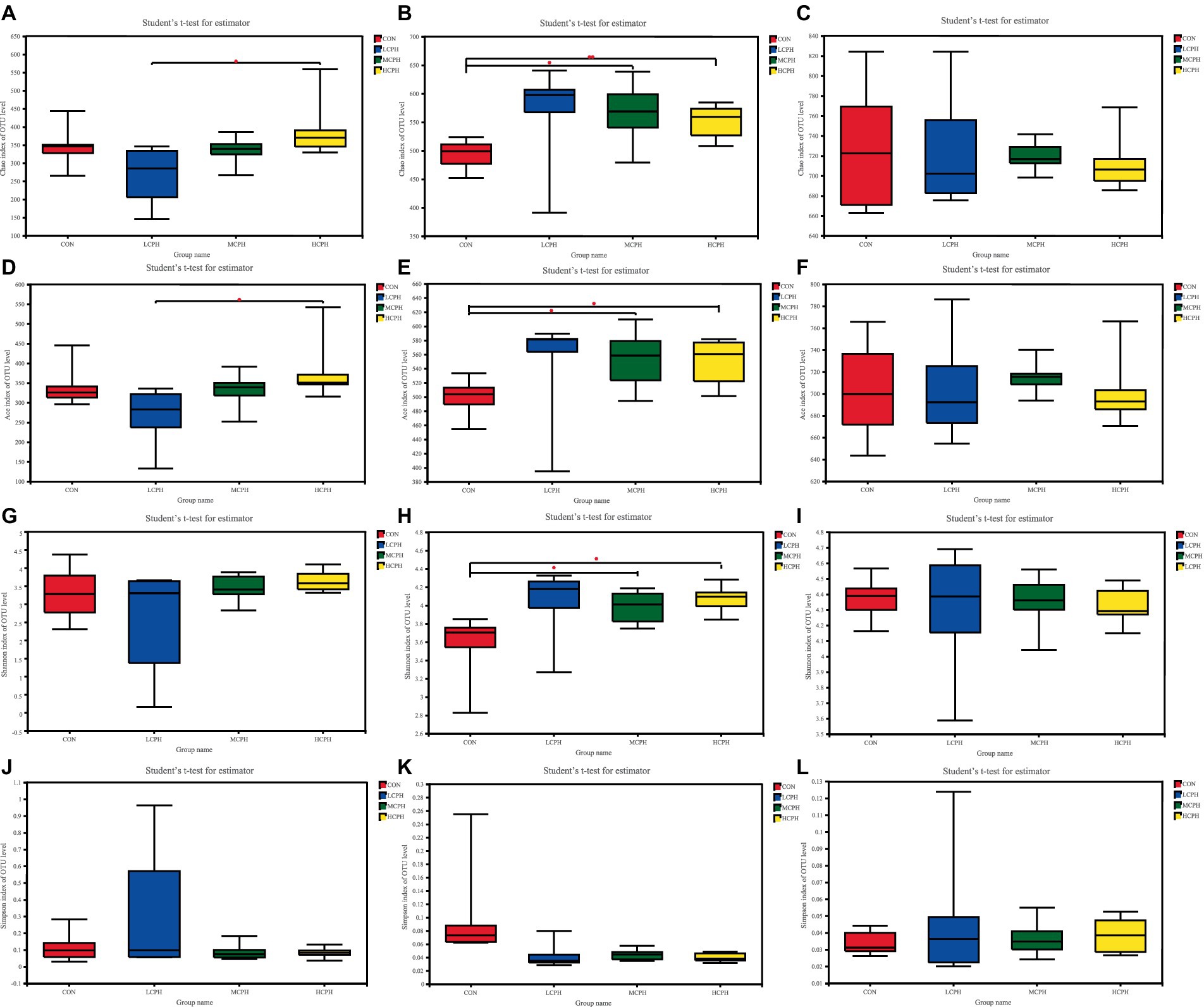
Figure 2. The bacterial α-diversity of the cecum in broilers dietary with CPH supplementation. (A–C) Chao index of cecal microbiota in 21-day-old, 42-day-old, and 63-day-old yellow-feather broilers, respectively. (D–F) Ace index of cecal microbiota in 21-day-old, 42-day-old, and 63-day-old yellow-feather broilers, respectively. (G–I) Shannon index of cecal microbiota in 21-day-old, 42-day-old, and 63-day-old yellow-feather broilers, respectively. (J–L) Simpson index of the OTUs community of cecal microbiota in 21-day-old, 42-day-old, and 63-day-old yellow-feather broilers, respectively. CON, basal diet; LCPH, diet with 1% CPH; MCPH, diet with 3% CPH; HCPH, diet with 5% CPH.
3.2.2 Effect of CPH on cecal microbial beta diversity
Beta diversity analysis revealed the natural distribution of the samples, reflecting their similarity. The effects of CPH on cecal microbial beta diversity are shown in Figure 3. PCoA with Bray–Curtis distance was used to analyze the cecal microflora structure in the treatment groups. To further analyze the overall structural changes in the gut microbiota among the different groups, an NMDS diagram based on the Bray–Curtis distance was constructed.
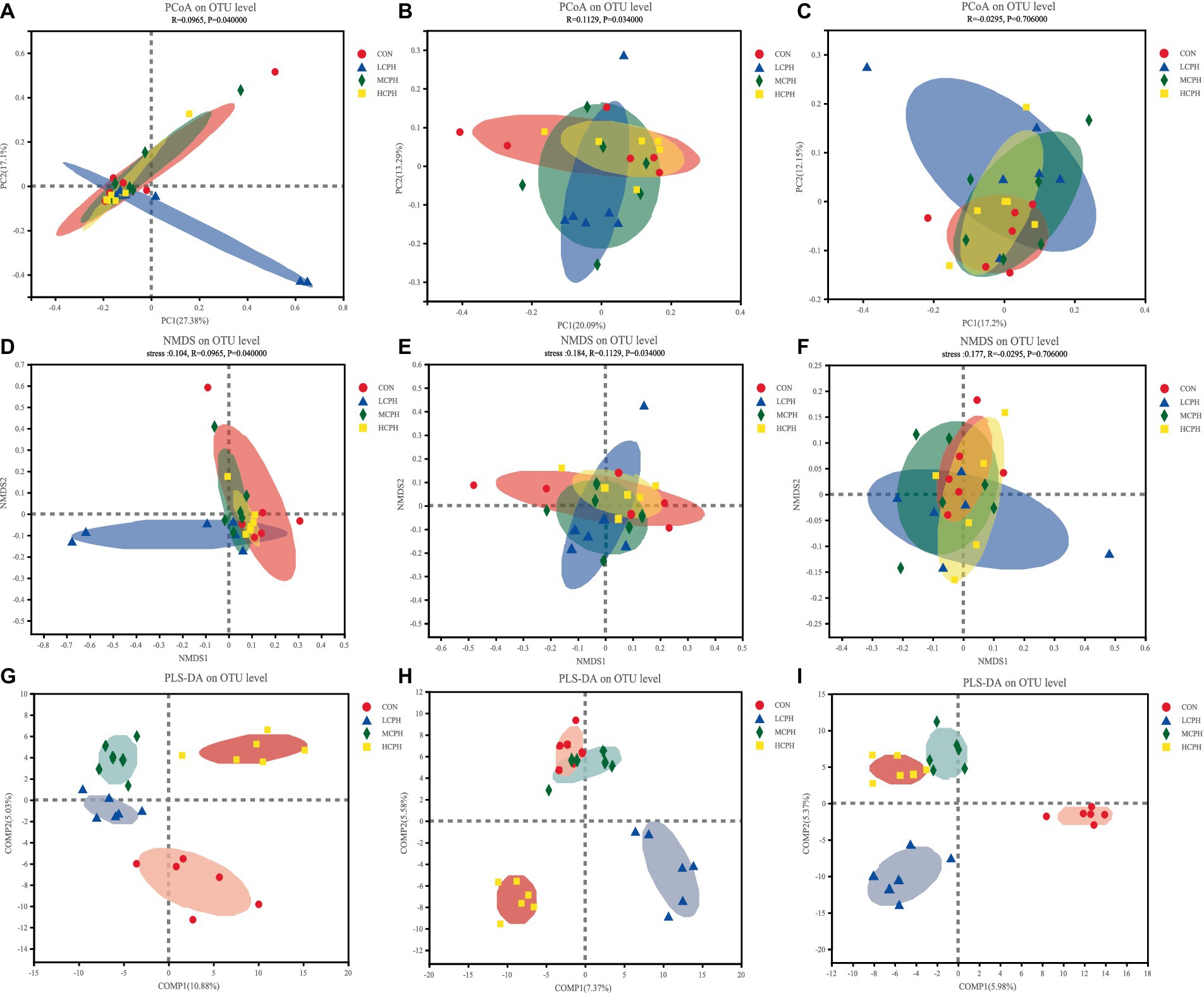
Figure 3. The bacterial β-diversity of the cecum in broilers dietary with CPH supplementation. (A–C) Principal coordinates analysis (PCoA) of cecal microbiota (based on the Bray distance) in 21-day-old, 42-day-old, and 63-day-old yellow-feather broilers, respectively. (D–F) NMDS analysis of cecal microbiota in 21-day-old, 42-day-old, and 63-day-old yellow-feather broilers, respectively. (G–I) PLS-DA analysis of cecal microbiota in 21-day-old, 42-day-old, and 63-day-old yellow-feather broilers, respectively. CON, basal diet; LCPH, diet with 1% CPH; MCPH, diet with 3% CPH; HCPH, diet with 5% CPH.
In 21-day-old broilers, PCoA indicated that the LCPH group tended to be distinct from the CON group, although this was not the case for the other treatment groups. The greater the distance between the sample points in the NMDS diagram, the lower similarity of the samples. The bacterial members showed higher differences in the LCPH group than in the CON group. Points in the MCPH and HCPH groups were more concentrated than those in the CON group. In addition, PLS-DA indicated that the treatment groups could be clearly distinguished, and that there was no sample crossover between the groups.
In 42-day-old broilers, PCoA indicated that the LCPH and MCPH groups tended to be distinct from the CON group, and that the points in the HCPH group were more concentrated than those in the CON group, suggesting that CPH changed the intestinal flora in the characteristic direction. The sample points in the LCPH, MCPH, and HCPH groups were more concentrated than those in the CON group in the NMDS diagram, indicating that the samples in the LCPH, MCPH, and HCPH groups were highly similar. As shown in the PLS-DA plot, the LCPH and HCPH groups were clearly distinguishable from the CON group, with no sample crossover.
In 63-day-old broilers, PCoA indicated that the CPH-supplemented groups differed from the CON group. The NMDS diagram showed that the samples distribution within the LCPH, MCPH, and HCPH groups were more dispersed than that in the CON group. PLS-DA indicated that the treatment groups could be clearly distinguished and tha sample crossover between the groups was absent.
3.3 Analysis of the composition of cecal microbiota
The results of the analysis of the phylum-level composition in broiler chickens is shown in Figure 4 and Supplementary Table S1. Firmicutes, Bacteroidetes, and Actinobacteriota were the top three phyla in 21-day-old broilers, and Firmicutes and Bacteroidetes accounted for 62.46 and 35.59% of the population, respectively. In addition, based on the analysis of the microflora composition, 246 genus-level microbial taxa were obtained, including 100 genera in all samples and 20 taxa with high abundance: Lactobacillus, Coprobacter, Faecalibacterium, Bacteroides, Alistipes, Ruminococcus_torques_group, unclassified_f__Lachnospiraceae, Clostridia_UCG-014, Clostridia_vadinBB60_group, Barnesiella, Ruminococcaceae, Eisenbergiella, Erysipelatoclostridium, Subdoligranulum, Blautia, unclassified_f__Oscillospiraceae, Flavonifractor, Colidextribacter, Phascolarctobacterium, and Butyricicoccus (Supplementary Table S2).
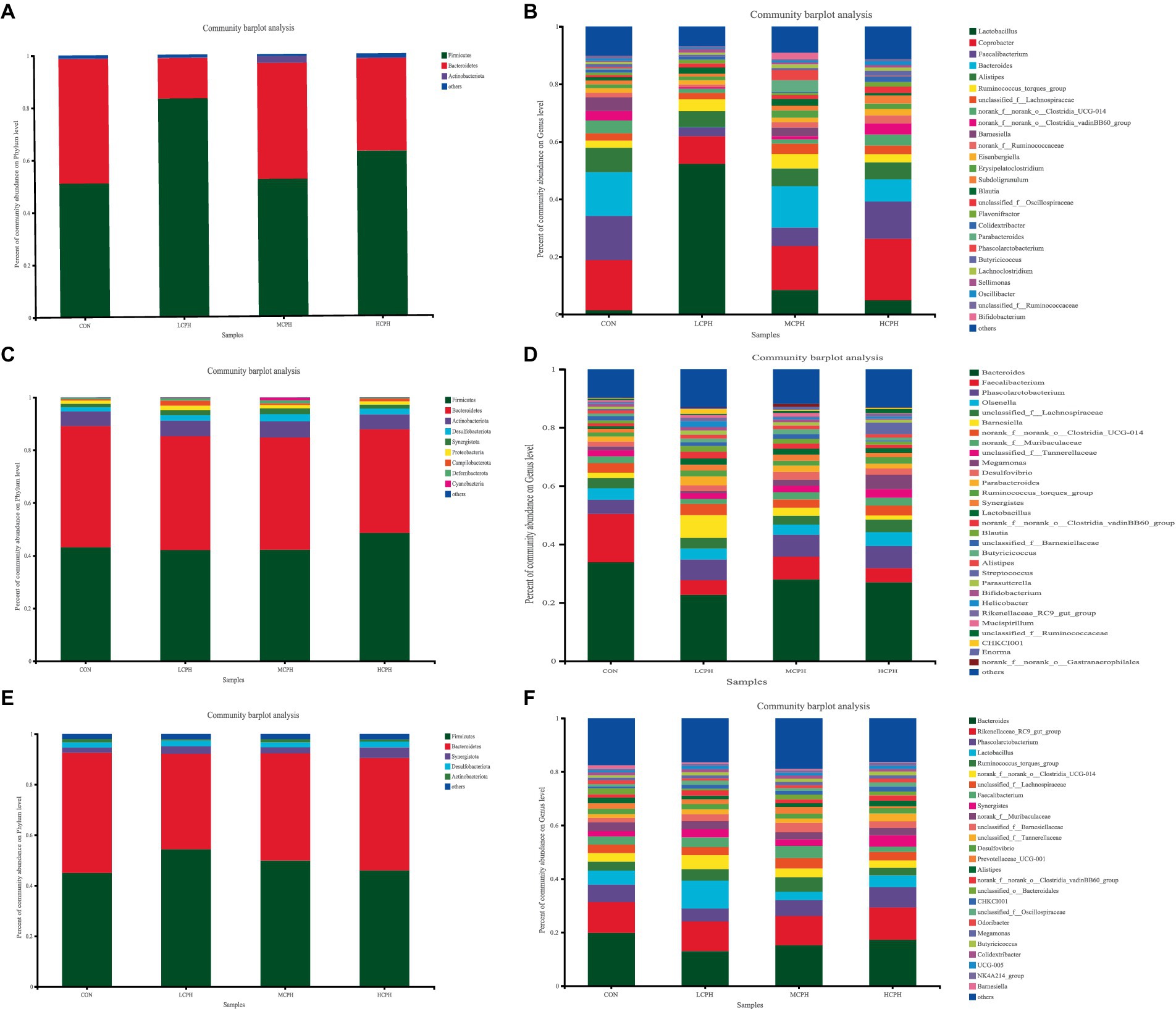
Figure 4. Relative abundance of the broilers’ caecal microbiota in level phylum and genus. (A,B) Relative abundance of the broilers’ caecal microbiota in level phylum and genus in 21-day-old yellow-feather broilers. (C,D) Relative abundance of the broilers’ caecal microbiota in level phylum and genus in 42-day-old yellow-feather broilers. (E,F) Relative abundance of the broilers’ caecal microbiota in level phylum and genus in 63-day-old yellow-feather broilers. Each mean represents six samples. CON, basal diet; LCPH, diet with 1% CPH; MCPH, diet with 3% CPH; HCPH, diet with 5% CPH.
In 42-day-old broilers, the top nine phyla identified were Firmicutes, Bacteroidetes, Actinobacteriota, Desulfobacteriota, Synergistota, Proteobacteria, Campylobacterota, Deferribacterota, and Cyanobacteria. Among these, Firmicutes, Bacteroidetes, and Actinobacteriota were the dominant phyla, accounting for 44.03, 42.78, and 5.73% of the population, respectively. In addition, based on the analysis of the microflora composition, 170 genera were identified, including 132 genera in all samples and 22 taxa with high abundance: Bacteroides, Faecalibacterium, Phascolarctobacterium, Olsenella, unclassified_f__Lachnospiraceae, Barnesiella, Clostridia_UCG-014, Muribaculaceae, unclassified_f__Tannerellaceae, Megamonas, Desulfovibrio, Parabacteroides, Ruminococcus_torques_group, Synergistes, Lactobacillus, Clostridia_vadinBB60_group, Blautia, unclassified_f__Barnesiellaceae, Butyricicoccus, Alistipes, Streptococcus, and Parasutterella (Supplementary Table S3).
In 63-day-old broilers, the top nine phyla identified were Firmicutes, Bacteroidetes, Synergistota, Desulfobacteriota, and Actinobacteria. And Firmicutes and Bacteroidetes accounted for 48.84 and 43.03% of the population, respectively. In addition, based on the analysis of the microflora composition, 204 genus-level microbial taxa were obtained, including 160 genera in all samples and 24 taxa with high abundance: Bacteroides, Rikenellaceae_RC9_gut_group, Phascolarctobacterium, Lactobacillus, Ruminococcus_torques_group, Clostridia_UCG-014, unclassified_f__Lachnospiraceae, Faecalibacterium, Synergistes, Muribaculaceae, unclassified_f__Barnesiella, unclassified_f__Tannerellaceae, Desulfovibrio, Prevotellaceae_UCG-001, Alistipes, Clostridia_vadinBB60_group, unclassified_o__Bacteroidales, CHKCI001, unclassified_f__Oscillospiraceae, Odoribacter, Megamonas, Butyricicoccus, Colidextribacter, and UCG-005 (Supplementary Table S4).
In 21-day-old broilers, Firmicutes were more abundant in the LCPH group (p < 0.05), whereas Bacteroidetes were more abundant in the CON group (p < 0.10) (Figure 5). At the genus level, the LCPH group showed higher relative proportion of Lactobacillus (p < 0.05) and a trend towards decreased relative abundance of Clostridia_vadinBB60_group (p < 0.10) than that in the CON group. Lactobacillus and Phascolarctobacterium were more abundant in the MCPH group than in the CON group (p < 0.05), and the abundance of Ruminococcus_torques_group tended to decrease (p < 0.10). The HCPH group showed higher relative proportions of Lactobacillus (p < 0.05) and a trend for increased abundance of Colidextribacter compared to that in CON group (p < 0.10) (Figure 6).
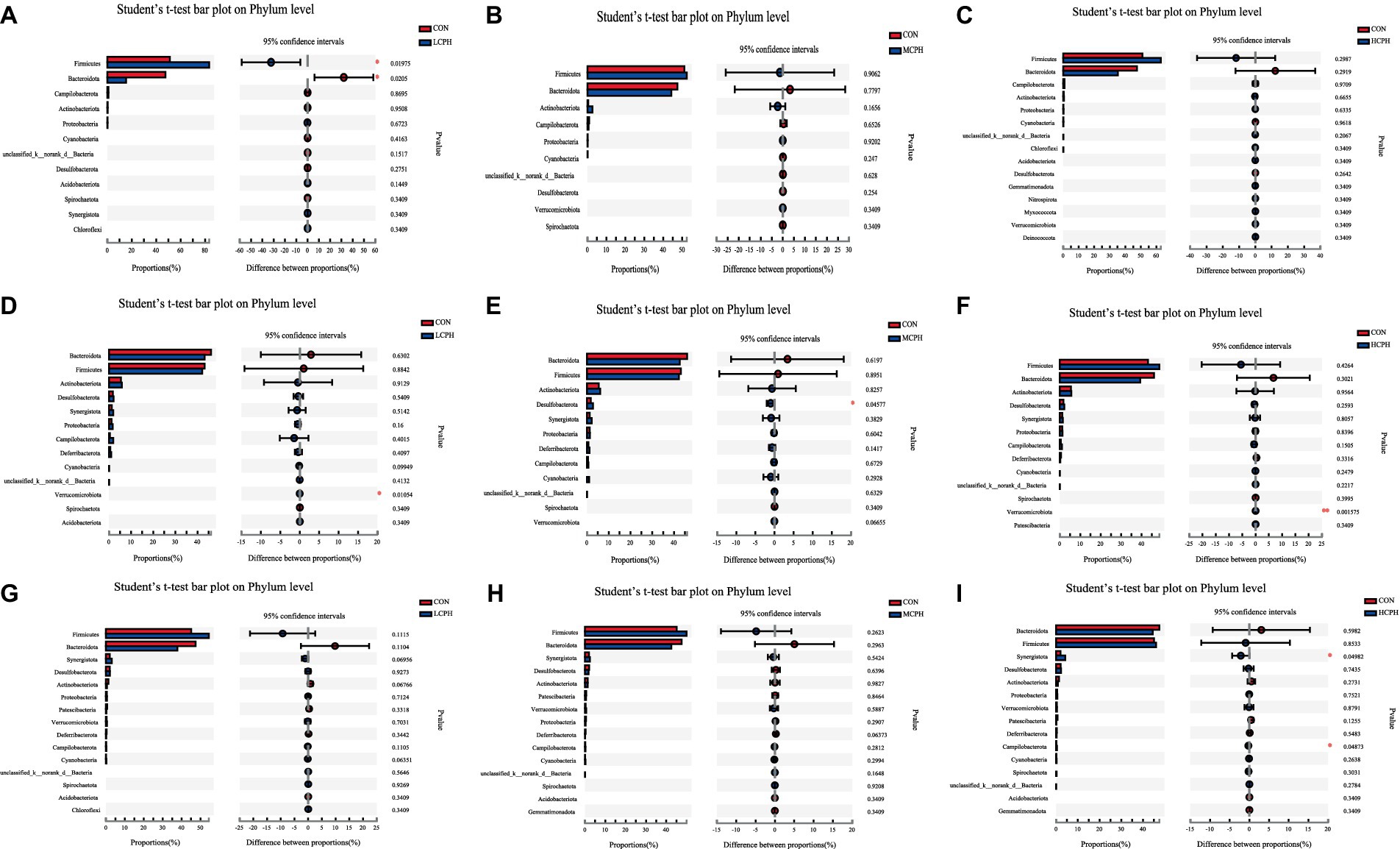
Figure 5. One-way ANOVA analysis of bacterial composition at the phylum level. (A–C) One-way ANOVA analysis of bacterial composition at the phylum level in 21-day-old yellow-feather broilers. (D–F) One-way ANOVA analysis of bacterial composition at the phylum level in 42-day-old yellow-feather broiler. (G–I) One-way ANOVA analysis of bacterial composition at the phylum level in 63-day-old yellow-feather broiler. The asterisk (*) level presented the degree of significant difference, *p < 0.05, **p < 0.01, and ***p < 0.001. Each mean represents six samples. CON, basal diet; LCPH, diet with 1% CPH; MCPH, diet with 3% CPH; HCPH, diet with 5% CPH.
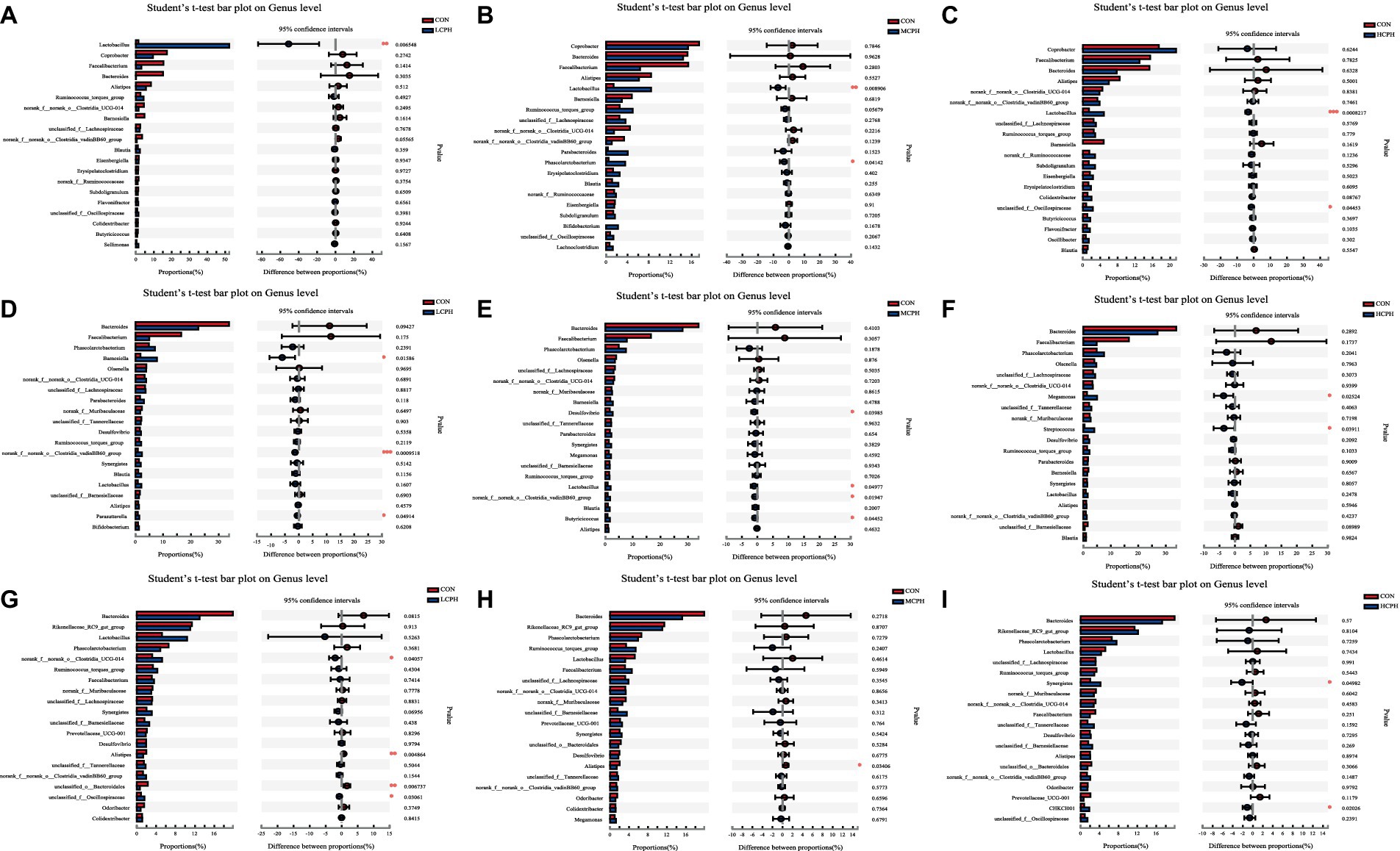
Figure 6. One-way ANOVA analysis of bacterial composition at the genus level. (A–C) One-way ANOVA analysis of bacterial composition at the genus level in 21-day-old yellow-feather broilers. (D–F) One-way ANOVA analysis of bacterial composition at the genus level in 42-day-old yellow-feather broilers. (G–I) One-way ANOVA analysis of bacterial composition at the genus levelin 63-day-old yellow-feather broilers. The asterisk (*) level presented the degree of significant difference, *p < 0.05, **p < 0.01, and ***p < 0.001. Each mean represents six samples. CON, basal diet; LCPH, diet with 1% CPH; MCPH, diet with 3% CPH; HCPH, diet with 5% CPH.
In 42-day-old broilers, the abundance of Verrucomicrobiota in the LCPH and HCPH groups was higher than that in the CON group (p < 0.05), whereas the abundance of Verrucomicrobiota in the MCPH group tended to be higher (p < 0.10). In addition, Desulfobacteriota was more abundant in the MCPH group than in the CON group (p < 0.05) (Figure 5). At the genus level, the relative abundances of Barnesiella, Clostridia_vadinBB60_group, and Parasutterella in the LCPH group; Desulfovibrio, Lactobacillus, Clostridia_vadinBB60_group, and Butyricicoccus in the MCPH group; and Megamonas and Streptococcus in the HCPH group were higher than those in the CON group (p < 0.05) (Figure 6).
In 63-day-old broilers, the abundance of Synergistota and Campylobacterota was significantly higher in the HCPH group than that in the CON group (p < 0.05) (Figure 5). At the genus level, the abundances of Clostridia_UCG-014 in the LCPH group and Synergistes in the HCPH group were higher than those in the CON group (p < 0.05). The abundance of Alistipes in the LCPH and MCPH groups was lower than that in the CON group (p < 0.05) (Figure 6).
To better understand the correlation between crucial gut microbiota alterations and the growth performance (Zhang et al., 2024) of yellow-feather broilers, the Spearman correlation analysis (Supplementary Figure S1) was performed. Spearman correlations showed a varying degrees of correlation between the number of observed species at the genus level and the growth performance. At the genus level, Lactobacillus was positively correlated with average daily gain (ADG) in 21-day-old broilers (p < 0.05). Streptococcus was negatively correlated with ADG and average daily feed intake (ADFI) in 42-day-old broilers (p < 0.05).
To better understand the correlation between crucial gut microbiota alterations and serum biochemical indices (Zhang et al., 2024) of yellow-feather broilers, the Spearman correlation analysis (Supplementary Figure S2) was performed. Spearman correlations showed a varying degrees of correlation between the number of observed species at the genus level and serum biochemical indices. At the genus level, Lactobacillus was positively correlated with albumin (ALB) and phosphorus (P) and negatively correlated with triglycerides (TG) and total cholesterol (T-CHO) in 21-day-old broilers (p < 0.05). Megamonas was positively correlated with P and Butyricicoccus was positively correlated with ALB and P; Lactobacillus and Butyricicoccus were negatively correlated with TG; Clostridia_vadinBB60_group was negatively correlated with blood urea nitrogen (BUN); Parasutterella was negatively correlated with BUN and TG in 42-day-old broilers (p < 0.05).
To better understand the correlation between crucial gut microbiota alterations and intestinal morphology (Zhang et al., 2024) of yellow-feather broilers, the Spearman correlation analysis (Supplementary Figures S3–S5) was performed. Spearman correlations showed a varying degrees of correlation between the number of observed species at the genus level and intestinal morphology. Lactobacillus was positively correlated with the V/C ratio and negatively correlated with the crypt depth; Ruminococcus_torques_group was negatively correlated with the crypt depth of duodenum in 21-day-old broilers (p < 0.05). Lactobacillus was positively correlated with the villus height of duodenum in 42-day-old broilers (p < 0.05). Phascolarctobacterium was positively correlated with the villus height of jejunum in 21-day-old broilers (p < 0.05). Megamonas was positively correlated with the villus height of jejunum in 42-day-old broilers (p < 0.05). Megamonas was positively correlated with the V/C ratio and negatively correlated with the crypt depth; Barnesiella and Clostridia_vadinBB60_group were negatively correlated with the V/C ratio of ileum in 42-day-old broilers (p < 0.05).
3.4 Cecal microbial network analysis
Network analysis, an effective method for assessing large and complex data, is based on the analysis of species abundance information between different samples; it can reveal the coexisting relationships and interactions between species and highlight the similarities and differences between samples. Therefore, we conducted a network analysis of microorganisms to further study the effects of CPH on cecal microorganisms in yellow-feather broilers. Figures 7–9 and Supplementary Table S5 show the microbial networks in the CON, LCPH, MCPH, and HCPH groups during the three experimental periods.
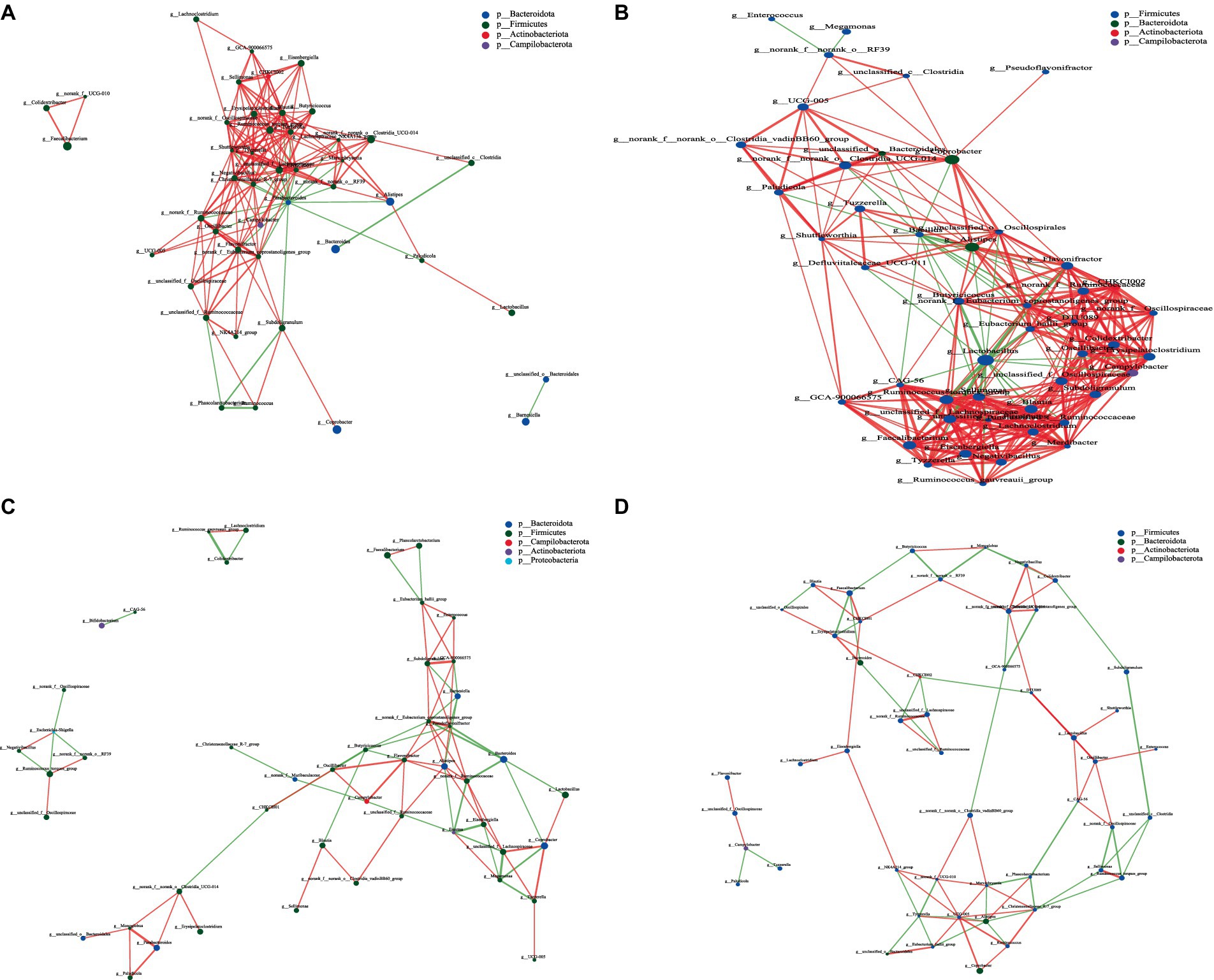
Figure 7. Interaction network diagram of cecal microbiota in 21-day-old yellow-feather broilers. (A) The network diagram of the interaction between the cecal microbiota in the CON group. (B) The network diagram of the interaction between the cecal microbiota in the LCPH group. (C) The network diagram of the interaction between the cecal microbiota in the MCPH group. (D) The network diagram of the interaction between the cecal microbiota in the HCPH group.
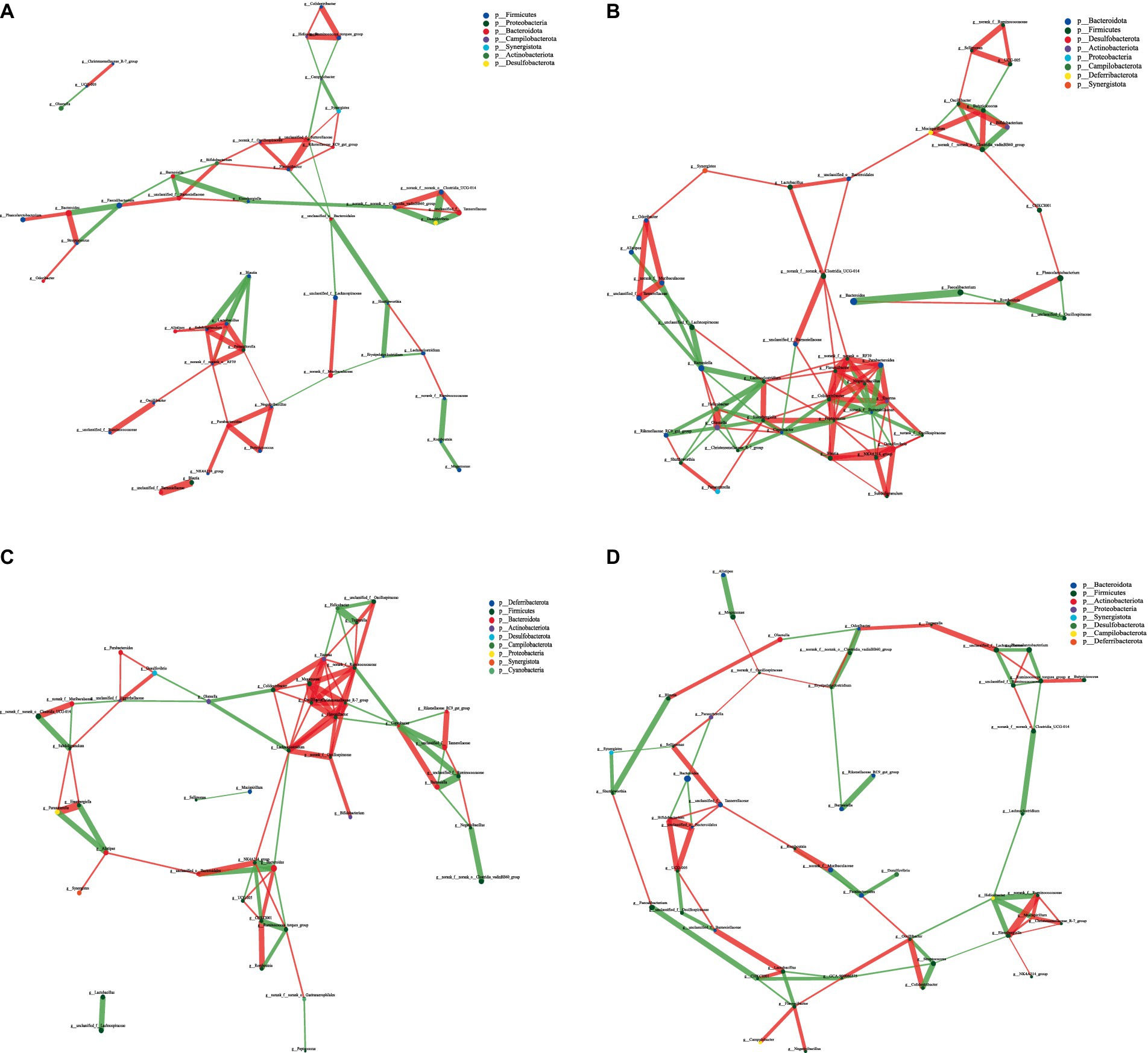
Figure 8. Interaction network diagram of cecal microbiota in 42-day-old yellow-feather broilers. (A) The network diagram of the interaction between the cecal microbiota in the CON group. (B) The network diagram of the interaction between the cecal microbiota in the LCPH group. (C) The network diagram of the interaction between the cecal microbiota in the MCPH group. (D) The network diagram of the interaction between the cecal microbiota in the HCPH group.
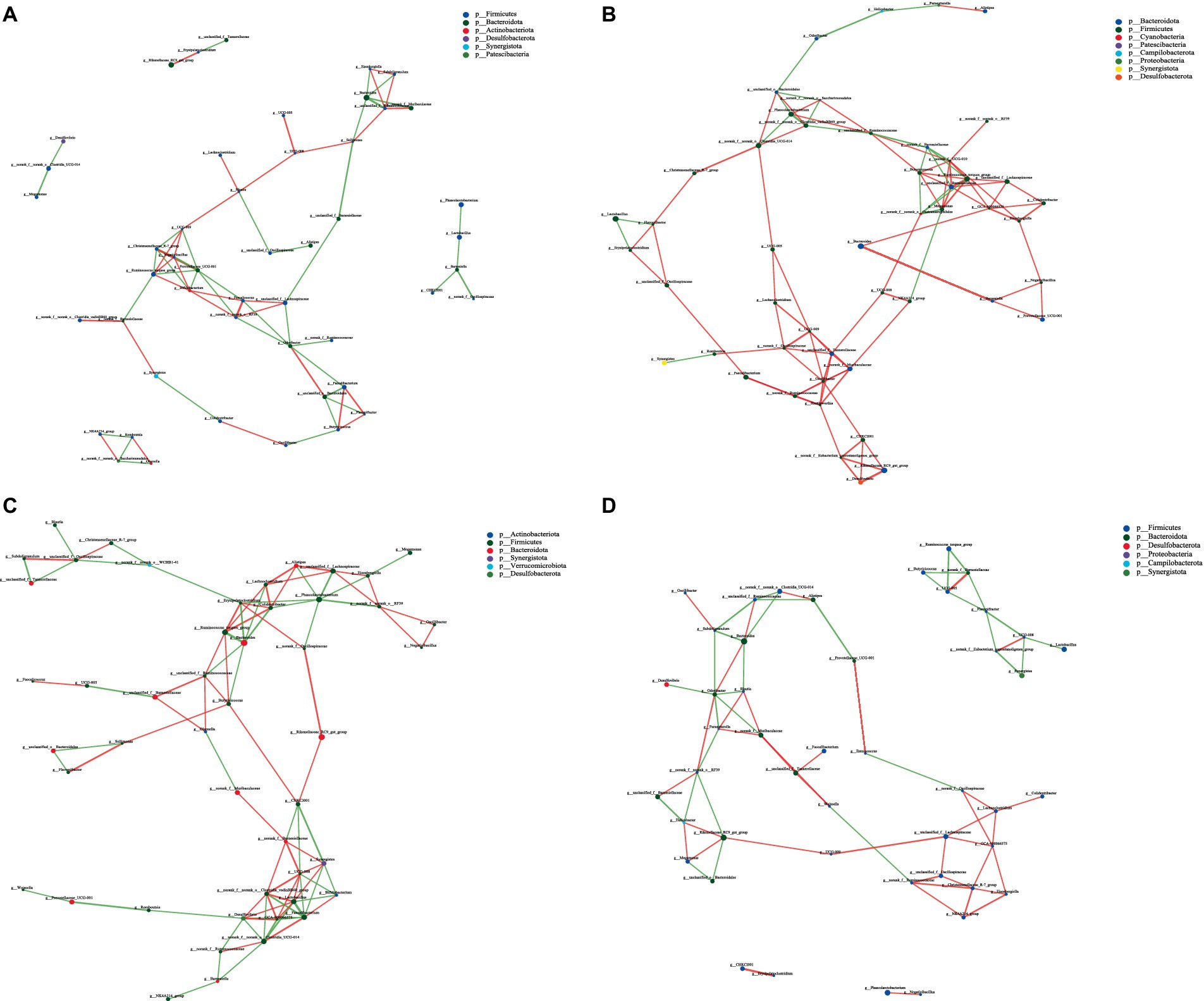
Figure 9. Interaction network diagram of cecal microbiota in 63-day-old yellow-feather broilers. (A) The network diagram of the interaction between the cecal microbiota in the CON group. (B) The network diagram of the interaction between the cecal microbiota in the LCPH group. (C) The network diagram of the interaction between the cecal microbiota in the MCPH group. (D) The network diagram of the interaction between the cecal microbiota in the HCPH group.
Among the 21-day-old broilers, the LCPH group had higher proportion of edge_Num and average node connectivity than the CON, MCPH, and HCPH groups. Our results showed that the network complexity in the LCPH group was higher than that in the CON group. In the CON group, the bacterial genera with high number of connected nodes included Parabacteroides (degree = 19) and Tuzzerella, and Blautia, and Ruminococcus_torques_group (degree =18). However, the more abundant bacterial genera, such as Lactobacillus (degree = 1) and Coprobacter (degree = 1), did not exhibit high connectivity. In addition, 165 positive and 24 negative correlations were observed. In the LCPH group, the bacterial genera with the highest number of connected nodes included norank_f__Eubacterium_coprostanoligenes_group, Lactobacillus, Lachnoclostridium, unclassified_f__Ruminococcaceae, Blautia, Subdoligranulum, unclassified_f__Oscillospiraceae (degree = 21), DTU089, Sellimonas, unclassified_f__Lachnospiraceae, and Ruminococcus_torques_group (degree = 20). Compared to that in the CON group, the node connectivity of Lactobacillus (degree = 21) improved. After CPH treatment, 291 positive and 40 negative correlations were observed.
In 42-day-old broilers, the LCPH and MCPH groups had higher proportions of edge_Num and higher average node connectivity than the CON group. In the CON group, the bacterial genera with the highest number of connected nodes included norank_f__norank_o__RF39, unclassified_f__Sutterellaceae (degree = 6), Parasutterella, Lactobacillus, Subdoligranulum, and Flavonifractor (degree = 5). In addition, 31 positive and 29 negative correlations were observed. In the LCPH group, the bacterial genera with the highest number of connected nodes are Peptococcus (degree = 12), Colidextribacter (degree = 11), Enorma, norank_f__Barnesiellaceae, Eisenbergiella (degree = 10), norank_f__norank_o__RF39, and Flavonifractor (degree = 9). In addition, 77 positive and 43 negative correlations were observed. In the MCPH group, the bacterial genera with the highest number of connected nodes included Eisenbergiella (degree = 6), Flavonifractor, GCA-900066575, Ruminococcus_torques_group, Helicobacter, and Oscillibacter (degree = 5). Following the CPH treatment, 37 positive and 37 negative correlations were observed.
In 63-day-old broilers, the LCPH and MCPH groups had higher proportions of edge_Num and higher average node connectivity than CON group. In the CON group, the bacterial genera with high number of connected nodes are Prevotellaceae_UCG-001 (degree = 7), Ruminococcus_torques_group, Bifidobacterium, and Odoribacter (degree = 6). Further, 31 positive and 40 negative correlations were observed. In the LCPH group, the bacterial genera with high number of connected nodes are norank_f__UCG-010, unclassified_f__Barnesiellaceae, Ruminococcus_torques_group (degree = 8), Butyricicoccus, Oscillibacter (degree = 7), GCA-900066575, Megamonas, unclassified_f__Ruminococcaceae, norank_f__Muribaculaceae, unclassified_f__Tannerellaceae, UCG-009, and Shuttleworthia (degree = 6). After CPH treatment, 77 positive and 22 negative correlations were observed. In the MCPH group, the bacterial genera with the highest number of connected nodes included UCG-008, Faecalibacterium, Lactobacillus (degree = 8), Phascolarctobacterium, Desulfovibrio, norank_f__norank_o__Clostridia_UCG-014, and norank_f__norank_o__Clostridia_vadinBB60_group (degree = 7). Moreover, 48 positive and 47 negative correlations were observed.
4 Discussion
The highly variable microbiota in poultry gut readily responds to many environmental changes and plays an important role in maintaining normal physiology and promoting general and immune health, which is indicative of the state of the bacteria (Ma et al., 2021; Singh et al., 2022; Stanley et al., 2013, 2014). CPH contains considerable amounts of soluble proteins and small peptides. Our results revealed that the gut microbial structure of the cecum of broilers responded differently to different CPH supplementation levels. Microbiome composition may be influenced by age and gender (Lindenberg et al., 2019). The CPH diet improved the composition of the cecal microflora and increased the microbial diversity of broilers during a specific period; however, the effect of CPH on the cecal microflora gradually decreased with increase in the growth period. Generally, the diversity and richness of the gut microbes vary with the state of the organism; increased diversity contributes to gut microbiome balance and enhances resistance to foreign pathogens. The diversity of microorganisms can be reflected by alpha diversity indices. Higher community richness was reflected by larger Chao1 or ACE values, whereas higher community diversity was indicated by larger Shannon or smaller Simpson values (Xie et al., 2020).
The results of this study showed that CPH significantly affected cecal microbial diversity in yellow-feather broilers; however, this effect was observed only in the early and middle stages of the trial (21-and 42-day-old broilers), indicating that CPH influenced microbial diversity and richness in the early growth stage of the broilers.
Species abundance may be the driving factor for these variations, as the Shannon diversity index remained unaffected; in PCoA with Bray–Curtis distance, the LCPH group of the 21-day-old broilers was distinct from the CON group. However, this was not the case for all the trial stages. The results of the PLS-DA and NMDS analyses further supported that CPH alters the intestinal microbiota of broilers. An apparent clustering of the microbiota was observed in the CPH group during the middle stage.
In our analysis, Firmicutes and Bacteroidetes were the most abundant phyla in all groups and experimental stages, as previously reported (Oakley et al., 2014; Pandit et al., 2018; Zhang et al., 2022). We generally believe that the Firmicutes contains more probiotics, with Lactobacillus being one of them. In addition, Firmicutes abundance has been suggested to improve intestinal conditions, particularly when the population of Proteobacteria is low (Abudabos et al., 2017; Shirey et al., 2015). The increase and decrease in the relative abundances of Firmicutes and Bacteroidetes, respectively, may indicate better production performance, which was also verified by our experimental results in the early stages. The abundance of Verrucomicrobia is beneficial for inducing regulatory immunity and improving glucose homeostasis; therefore, it is closely related to improvements in metabolism (Cox and Blaser, 2013; Guo et al., 2018; Lindenberg et al., 2019). The increased abundance of Verrucomicrobia in 42-day-old broilers improved the composition of cecal microorganisms. No changes were observed at the phylum level in 63-day-old broilers, except for Synergistota and Campylobacterota. Thus, the effect of CPH on the microphylum levels in broilers was observed mainly during the early and middle stages of the experiment, and some phyla, such as Firmicutes and Verrucomicrobia, favored broiler metabolism and growth. This also indicates that CPH may alter the metabolism of broilers by altering the intestinal flora, thus changing and improving the growth of broilers.
Lactobacillus, an important probiotic that functions to renovate the intestinal immune barrier (Zhang et al., 2022; Zhen et al., 2021), is well known in gastrointestinal health and fermentation communities, and is specifically associated with feed conversion. In addition, it has pathogenic and anti-inflammatory properties due to its ability to produce lactic acid or short-chain fatty acids (SCFAs) (Diepers et al., 2017; Zhao et al., 2019). Our results indicated that CPH exerted a positive effect on Lactobacillus in 21-day-old yellow-feather broilers. The increased abundance in Lactobacillus was beneficial for improving serum biochemical indices, which was beneficial for protein and fat metabolism in broilers. During the test period, ADG and F/G correlated positively and negatively with Lactobacillus, respectively. Our results showed that changes in the abundance of Lactobacillus improved the cecal microbial composition, and was beneficial for intestinal morphology and growth performance (Zhang et al., 2024) of yellow-feather broilers.
Phascolarctobacterium produces SCFAs, including acetate and propionate, and colonizes and exerts beneficial effects on the human gastrointestinal tract (Chen et al., 2021). Clostridia_vadinBB60_group may be helpful in reducing the abundance of maleficent bacteria. The increased abundance of Clostridia_vadinBB60_group exerts a positive impact on Treg cell counts in mice (Atarashi et al., 2013). Barnesiella spp. possesses beneficial antimicrobial properties. SCFA levels may be related to Barnesiella, which can produce butyrate and isobutyrate from glucose and positively affect SCFA generation (Gu et al., 2019). Kim et al. (2017) found that the addition of Clostridia to the intestinal flora of mouse infants was sufficient to limit colonization by the pathogenic Enterobacteriaceae. Parasutterella has been linked to multiple health outcomes and is considered a core members of the intestinal flora in humans and mice (Ju et al., 2019). We found that an increase in the abundance of Clostridia_vadinBB60_group and Parasutterella favored a decrease in BUN in serum. Desulfovibrio is a group of sulfate-reducing anaerobic bacteria with more than 30 species and is ubiquitous in nature and animal gastrointestinal tracts (Goldstein et al., 2003). Butyricicoccus is beneficial for digestion and nutrient absorption and helps improve metabolic and immune activities (Yang et al., 2021). Our results indicated that the increase of Butyricicoccus was conducive to enhancing protein metabolism and P absorption, and may improved fat metabolism of yellow-feather broilers. Megamonas, which belongs to the phylum Firmicutes, is a unique bacterium present in the cecum microbiota of male chickens; the Na/melibiose symporter and α-galactosidase expressed by Megamonas are required for breaking down the α-1,6 linkage between galactose and glucose in melibiose (Cui et al., 2021; Polansky et al., 2016). The cecal microbiota of male chickens was more dependent on glycan metabolism, as indicated by the high abundance of Megamonas; thus, the change in microflora may change the metabolism of broilers, which then affects their growth and development. Our results indicated that increased abundance of Megamonas was beneficial for improving intestinal morphology. Therefore, in the early and middle stages of the experiment, an increase in these probiotics was beneficial for improving the cecal microflora structure of yellow-feather broilers, which was conducive for their growth and development. Few non-probiotic changes were also observed. For example, Streptococcus comprises ecologically diverse species and includes some pathogenic bacteria (Lee and Andam, 2022), and an increase in its abundance may be detrimental to the organism.
Therefore, CPH increased the relative abundance of some probiotics and affected the cecal microbial diversity and community composition of yellow-feather broilers, which was related to the growth period; this effect gradually decreased with the extension of the growth period. And changes in the abundance of probiotics were beneficial to improve the intestinal morphology and growth performance of yellow-feather broilers. In addition, the CPH supplementation level was an important factor affecting microorganisms abundance; the effects of varying supplementation levels in different periods were not consistent.
Dietary composition may induce different coexisting network topologies in the intestinal microbiota. We found that the effects of CPH on network complexity varied with age. The complexity of the coexistence network varied in the CPH group, which may have been due to changes in the absorption and utilization of nutrients caused by CPH in the intestine, which then changed the interactions between cecal microorganisms. However, the change in network complexity with increasing CPH levels did not follow any trend; for example, on day 21, only the network complexity of the LCPH group was higher than that of the CON group, while that of the MCPH and HCPH groups decreased. However, after day 42, the network complexity of the LCPH and MCPH groups also increased, thus altering the cecal microbial network complexity in response to different levels of CPH, which may also be related to the growth period of broilers. Lactobacillus and Blautia are beneficial for animal health, and a more complexed correlation with other genera is favorable in broilers. Increased network complexity is helpful in resisting pathogen invasion and external influences (Li et al., 2022; Mendes et al., 2018). Enhancement of the cecal microbial network complexity benefits the health and growth of broilers; the increased network complexity of probiotics may have enhanced this effect.
5 Conclusion
This study aimed to determine the effects of CPH on the intestinal microbiota of yellow-feather broilers. We concluded that CPH affects the cecal microbial composition of yellow-feather broilers, increasing the relative abundance of beneficial gut microbiota and enhancing the richness and diversity of the bacterial microbiota in chickens <42 days of age, and changes in the abundance of probiotics were beneficial to improve the intestinal morphology and growth performance. The effect of CPH on intestinal microbiota weakened after 42 days. The LCPH treatment increased the complexity of the cecal microbial network, while the MCPH treatment had the same effect after 42 days, which may have increased resistance to pathogens. The results of this study may act as a reference for the application of CPH to animals.
Data availability statement
The datasets presented in this study can be found in online repositories. The names of the repository/repositories and accession number(s) can be found in the article/Supplementary material.
Ethics statement
Ethical requirements and recmmendations for animal care for the experiment were approved by the Biology Ethics Committee of Shihezi university (Xinjiang, China) (no. A2021-47). The study was conducted in accordance with the local legislation and institutional requirements.
Author contributions
XZ: Conceptualization, Data curation, Formal analysis, Methodology, Writing – original draft. HW: Data curation, Methodology, Writing – original draft. YN: Data curation, Methodology, Writing – original draft. CC: Funding acquisition, Project administration, Writing – review & editing. WZ: Conceptualization, Funding acquisition, Project administration, Writing – review & editing.
Funding
The author(s) declare that financial support was received for the research, authorship, and/or publication of this article. This work was supported by the National Natural Science Foundation of China (32060770), Shihezi University Youth Innovation Top Talent Project (KX01500301), and the International Science and Technology Cooperation Program of the Xinjiang Production and Construction Corps (2017BC002).
Conflict of interest
The authors declare that the research was conducted in the absence of any commercial or financial relationships that could be construed as a potential conflict of interest.
Publisher’s note
All claims expressed in this article are solely those of the authors and do not necessarily represent those of their affiliated organizations, or those of the publisher, the editors and the reviewers. Any product that may be evaluated in this article, or claim that may be made by its manufacturer, is not guaranteed or endorsed by the publisher.
Supplementary material
The Supplementary material for this article can be found online at: https://www.frontiersin.org/articles/10.3389/fmicb.2024.1434252/full#supplementary-material
References
Abudabos, A. M., Al-Atiyat, R. M., Albatshan, H. A., Aljassim, R., Aljumaah, M. R., Alkhulaifi, M. M., et al. (2017). Effects of concentration of corn distillers dried grains with solubles and enzyme supplementation on cecal microbiota and performance in broiler chickens. Appl. Microbiol. Biotechnol. 101, 7017–7026. doi: 10.1007/s00253-017-8448-5
Alizadeh-Ghamsari, A. H., Shaviklo, A. R., and Hosseini, S. A. (2023). Effects of a new generation of fish protein hydrolysate on performance, intestinal microbiology, and immunity of broiler chickens. J. Anim. Sci. Tech. 65, 804–817. doi: 10.5187/jast.2022.e99
Arihara, K. (2006). Strategies for designing novel functional meat products. Meat Sci. 74, 219–229. doi: 10.1016/j.meatsci.2006.04.028
Atarashi, K., Tanoue, T., Oshima, K., Suda, W., Nagano, Y., Nishikawa, H., et al. (2013). Treg induction by a rationally selected mixture of Clostridia strains from the human microbiota. Nature 500, 232–236. doi: 10.1038/nature12331
Brody, E. P. (2000). Biological activities of bovine glycomacropeptide. Br. J. Nutr. 84, 39–46. doi: 10.1017/S0007114500002233
Candela, M., Biagi, E., Maccaferri, S., Turroni, S., and Brigidi, P. (2012). Intestinal microbiota is a plastic factor responding to environmental changes. Trends Microbiol. 20, 385–391. doi: 10.1016/j.tim.2012.05.003
Chen, J., Wang, W., Guo, Z., Huang, S., Lei, H., Zang, P., et al. (2021). Associations between gut microbiota and thyroidal function status in Chinese patients with graves’ disease. J. Endocrinol. Investig. 44, 1913–1926. doi: 10.1007/s40618-021-01507-6
Cheng, H., Liu, M., Yuan, X., Zhang, D., Liu, W., and Jiang, G. (2019). Cottonseed meal protein hydrolysate stimulates feed intake and appetite in Chinese mitten crab, Eriocheir sinensis. Aquac. Nutr. 25, 983–994. doi: 10.1111/anu.12916
Cox, L. M., and Blaser, M. J. (2013). Pathways in microbe-induced obesity. Cell Metab. 17, 883–894. doi: 10.1016/j.cmet.2013.05.004
Cui, L., Zhang, X., Cheng, R., Ansariab, A. R., Elokil, A. A., Hu, Y., et al. (2021). Sex differences in growth performance are related to cecal microbiota in chicken. Microb. Pathog. 150:104710. doi: 10.1016/j.micpath.2020.104710
de Oliveira Filho, J. G., Rodrigues, J. M., Valadares, A. C. F., de Almeida, A. B., Valencia-Mejia, E., Fernandes, K. F., et al. (2021). Bioactive properties of protein hydrolysate of cottonseed byproduct: antioxidant, antimicrobial, and angiotensin-converting enzyme (ACE) inhibitory activities. Waste Biomass Valor 12, 1395–1404. doi: 10.1007/s12649-020-01066-6
Diepers, A. C., Krömker, V., Zinke, C., Wente, N., Pan, L., Paulsen, K., et al. (2017). In vitro ability of lactic acid bacteria to inhibit mastitis-causing pathogens. Sustain. Chem. Pharm. 5, 84–92. doi: 10.1016/j.scp.2016.06.002
Gao, D., Cao, Y., and Li, H. (2010). Antioxidant activity of peptide fractions derived from cottonseed protein hydrolysate. J. Sci. Food Agric. 90, 1855–1860. doi: 10.1002/jsfa.4024
Gisbert, E., Ibarz, A., Firmino, J. P., Fernández-Alacid, L., Salomón, R., Vallejos-Vidal, E., et al. (2021). Porcine protein hydrolysates (PEPTEIVA®) promote growth and enhance systemic immunity in gilthead sea bream (Sparus aurata). Animals 11:2122. doi: 10.3390/ani11072122
Goldstein, E. J., Citron, D. M., Peraino, V. A., and Cross, S. A. (2003). Desulfovibrio desulfuricans bacteremia and review of human Desulfovibrio infections. J. Clin. Microbiol. 41, 2752–2754. doi: 10.1128/JCM.41.6.2752-2754.2003
Gu, J., Thomas-Ahner, J. M., Riedl, K. M., Bailey, M. T., Vodovotz, Y., Schwartz, S. J., et al. (2019). Dietary black raspberries impact the colonic microbiome and phytochemical metabolites in mice. Mol. Nutr. Food Res. 63:e1800636. doi: 10.1002/mnfr.201800636
Gui, D., Liu, W., Shao, X., and Xu, W. (2010). Effects of different dietary levels of cottonseed meal protein hydrolysate on growth, digestibility, body composition and serum biochemical indices in crucian carp (Carassius auratus gibelio). Anim. Feed Sci. Technol. 156, 112–120. doi: 10.1016/j.anifeedsci.2010.01.012
Guo, J., Han, X., Zhan, J., You, Y., and Huang, W. (2018). Vanillin alleviates high fat diet-induced obesity and improves the gut microbiota composition. Front. Microbiol. 9:2733. doi: 10.3389/fmicb.2018.02733
Hartnoll, R. G. (2001). Growth in crustacea – twenty years on. Hydrobiologia 449, 111–122. doi: 10.1023/A:1017597104367
Ju, T., Kong, J. Y., Stothard, P., and Willing, B. P. (2019). Defining the role of Parasutterella, a previously uncharacterized member of the core gut microbiota. ISME J. 13, 1520–1534. doi: 10.1038/s41396-019-0364-5
Kim, Y. G., Sakamoto, K., Seo, S. U., Pickard, J. M., Gillilland, M. G., Pudlo, N. A., et al. (2017). Neonatal acquisition of Clostridia species protects against colonization by bacterial pathogens. Science 356, 315–319. doi: 10.1126/science.aag2029
Lee, I. P. A., and Andam, C. P. (2022). Frequencies and characteristics of genome-wide recombination in Streptococcus agalactiae, Streptococcus pyogenes, and Streptococcus suis. Sci. Rep. 12:1515. doi: 10.1038/s41598-022-04995-5
Li, Y., Li, X., Wu, Y., and Zhang, W. (2022). Effects of fecal microbiota transplantation from yaks on weaning diarrhea, fecal microbiota composition, microbial network structure and functional pathways in Chinese Holstein calves. Front. Microbiol. 13:898505. doi: 10.3389/fmicb.2022.898505
Lindenberg, F., Krych, L., Fielden, J., Kot, W., Frøkiær, H., van Galen, G., et al. (2019). Expression of immune regulatory genes correlate with the abundance of specific Clostridiales and Verrucomicrobia species in the equine ileum and cecum. Sci. Rep. 9:12674. doi: 10.1038/s41598-019-49081-5
Ma, J. Y., Mahfuz, S., Wang, J., and Piao, X. S. (2021). Effect of dietary supplementation with mixed organic acids on immune function, antioxidative characteristics, digestive enzymes activity, and intestinal health in broiler chickens. Front. Nutr. 673316:8. doi: 10.3389/fnut.2021.673316
Mahdavi-Yekta, M., Reihani, S. F. S., and Mohammadi, M. (2023). Antimicrobial activity of quinoa protein hydrolysate against Streptococcus pyogenes and Escherichia coli. J. Food Quality 2023, 1–7. doi: 10.1155/2023/9993241
Mendes, L. W., Mendes, R., Raaijmakers, J. M., and Tsai, S. M. (2018). Breeding for soil-borne pathogen resistance impacts active rhizosphere microbiome of common bean. ISME J. 12, 3038–3042. doi: 10.1038/s41396-018-0234-6
Oakley, B. B., Lillehoj, H. S., Kogut, M. H., Kim, W. K., Maurer, J. J., Pedroso, A., et al. (2014). The chicken gastrointestinal microbiome. FEMS Microbiol. Lett. 360, 100–112. doi: 10.1111/1574-6968.12608
Ozturk-Kerimoglu, B., Heres, A., Mora, L., and Toldrá, F. (2023). Antioxidant peptides generated from chicken feet protein hydrolysates. J. Sci. Food Agr. 103, 7207–7217. doi: 10.1002/jsfa.12802
Pandit, R. J., Hinsu, A. T., Patel, N. V., Koringa, P. G., Jakhesara, S. J., Thakkar, J. R., et al. (2018). Microbial diversity and community composition of caecal microbiota in commercial and indigenous Indian chickens determined using 16S rDNA amplicon sequencing. Microbiome 6:115. doi: 10.1186/s40168-018-0501-9
Polansky, O., Sekelova, Z., Faldynova, M., Sebkova, A., Sisak, F., and Rychlik, I. R. (2016). Important metabolic pathways and biological processes expressed by chicken cecal microbiota. Appl. Environ. Microbiol. 82, 1569–1576. doi: 10.1128/AEM.03473-15
Ridaura, V. K., Faith, J. J., Rey, F. E., Cheng, J., Duncan, A. E., Kau, A. L., et al. (2013). Gut microbiota from twins discordant for obesity modulate metabolism in mice. Science 341:1241214. doi: 10.1126/science.1241214
Safari, R., Motamedzadegan, A., Ovissipour, M., Regenstein, J. M., Gildberg, A., and Rasco, B. (2012). Use of hydrolysates from yellowfin tuna (Thunnus albacares) heads as a complex nitrogen source for lactic acid bacteria. Food Bioprocess Technol. 5, 73–79. doi: 10.1007/s11947-009-0225-8
Sharma, V., and Thakur, M. (2024). Plant protein hydrolysates as healthier and sustainable nutraceutical. World Sustain. Ser. 1813, 329–341. doi: 10.1007/978-3-031-46046-3_16
Shirey, T. B., Dykes, J. K., Lúquez, C., Maslanka, S. E., and Raphael, B. H. (2015). Characterizing the fecal microbiota of infants with botulism. Microbiome 3:54. doi: 10.1186/s40168-015-0119-0
Singh, A. K., Tiwari, U. P., Mishra, B., and Jha, R. (2022). Effects of in ovo delivered xylo-and mannan-oligosaccharides on growth performance, intestinal immunity, cecal short-chain fatty acids, and cecal microbiota of broilers. J. Anim. Sci. Biotechnol. 13, 1–16. doi: 10.1186/s40104-021-00666-z
Song, W., Kong, X., Hua, Y., Chen, Y., Zhang, C., and Chen, Y. (2020). Identification of antibacterial peptides generated from enzymatic hydrolysis of cottonseed proteins. LWT 125:109199. doi: 10.1016/j.lwt.2020.109199
Stanley, D., Geier, M. S., Hughes, R. J., Denman, S. E., and Moore, R. J. (2013). Highly variable microbiota development in the chicken gastrointestinal tract. PLoS One 8:e84290. doi: 10.1371/journal.pone.0084290
Stanley, D., Hughes, R. J., and Moore, R. J. (2014). Microbiota of the chicken gastrointestinal tract: influence on health, productivity and disease. Appl. Microbiol. Biotechnol. 98, 4301–4310. doi: 10.1007/s00253-014-5646-2
Tang, T., Wu, N., Tang, S., Xiao, N., Jiang, Y., Tu, Y., et al. (2023). Industrial application of protein hydrolysates in food. J. Agric. Food Chem. 71, 1788–1801. doi: 10.1021/acs.jafc.2c06957
Tolba, S. A., Amer, S. A., Gouda, A., Osman, A., Sherief, W. R. I. A., Ahmed, A. I., et al. (2023). Potential use of cowpea protein hydrolysate as a dietary supplement in broiler chickens: effects on growth, intestinal morphology, muscle lipid profile, and immune status. Ital. J. Anim. Sci. 22, 1204–1218. doi: 10.1080/1828051X.2023.2274508
Turnbaugh, P. J., Ridaura, V. K., Faith, J. J., Rey, F. E., Knight, R., and Gordon, J. I. (2009). The effect of diet on the human gut microbiome: a metagenomic analysis in humanized gnotobiotic mice. Sci. Transl. Med. 1:6ra14. doi: 10.1126/scitranslmed.3000322
van Kuijk, S. J. A., Han, Y., Garcia-Ruiz, A. I., and Rodiles, A. (2021). Hydroxychloride trace minerals have a positive effect on growth performance, carcass quality and impact ileal and cecal microbiota in broiler chickens. J. Anim. Sci. Biotechnol. 12:38. doi: 10.1186/s40104-021-00553-7
Wang, L., Ma, M., Yu, Z., and Du, S. K. (2021). Preparation and identification of antioxidant peptides from cottonseed proteins. Food Chem. 352:129399. doi: 10.1016/j.foodchem.2021.129399
Xie, Z., Zhao, Q., Wang, H., Wen, L., Li, W., Zhang, X., et al. (2020). Effects of antibacterial peptide combinations on growth performance, intestinal health, and immune function of broiler chickens. Poult. Sci. 99, 6481–6492. doi: 10.1016/j.psj.2020.08.068
Yang, J., Wang, J., Huang, K., Liu, Q., Guofang Liu, X. X., Zhang, H., et al. (2021). Selenium-enriched Bacillus subtilis yb-114246 improved growth and immunity of broiler chickens through modified ileal bacterial composition. Sci. Rep. 11:21690. doi: 10.1038/s41598-021-00699-4
Zhang, L., Ben Said, L., Hervé, N., Zirah, S., Diarra, M. S., and Fliss, I. (2022). Effects of drinking water supplementation with Lactobacillus reuteri, and a mixture of reuterin and microcin J25 on the growth performance, caecal microbiota and selected metabolites of broiler chickens. J. Anim. Sci. Biotechnol. 13, 1–13. doi: 10.1186/s40104-022-00683-6
Zhang, X., Nan, S., Zhang, L., Chen, C., Zhang, W., and Nie, C. (2024). Cottonseed meal protein hydrolysate influences growth performance, carcass characteristics, serum biochemical indices, and intestinal morphology in yellow-feather broilers. J. Anim. Physiol. An. 2, 1–13. doi: 10.1111/jpn.13995
Zhao, Y., Hong, K., Zhao, J., Zhang, H., Zhai, Q., and Chen, W. (2019). Lactobacillus fermentum and its potential immunomodulatory properties. J. Funct. Foods 56, 21–32. doi: 10.1016/j.jff.2019.02.044
Keywords: cottonseed meal protein hydrolysate, yellow-feather broilers, intestinal microbiota, microbial diversity and richness, microbial network
Citation: Zhang X, Wang H, Niu Y, Chen C and Zhang W (2024) Effects of cottonseed meal protein hydrolysate on intestinal microbiota of yellow-feather broilers. Front. Microbiol. 15:1434252. doi: 10.3389/fmicb.2024.1434252
Edited by:
Md. Abul Kalam Azad, Chinese Academy of Sciences (CAS), ChinaReviewed by:
Eric Auclair, Phileo Lesaffre Animal Care, FranceTarique Hussain, Nuclear Institute for Agriculture and Biology, Pakistan
Copyright © 2024 Zhang, Wang, Niu, Chen and Zhang. This is an open-access article distributed under the terms of the Creative Commons Attribution License (CC BY). The use, distribution or reproduction in other forums is permitted, provided the original author(s) and the copyright owner(s) are credited and that the original publication in this journal is cited, in accordance with accepted academic practice. No use, distribution or reproduction is permitted which does not comply with these terms.
*Correspondence: Cheng Chen, ZW56ZTEwNUAxNjMuY29t; Wenju Zhang, emhhbmd3ajEwMjJAc2luYS5jb20=
†ORCID: Hailiang Wang, orcid.org/0009-0005-9311-8212
Cheng Chen, orcid.org/0000-0002-0242-9332