- School of Grassland Science, Beijing Forestry University, Beijing, China
Hibernation, an evolved survival trait among animals, enables them to endure frigid temperatures and food scarcity during the winter months, and it is a widespread phenomenon observed in mammals. The gut microbiota, a crucial component of animal nutrition and health, exhibits particularly dynamic interactions in hibernating mammals. This manuscript comprehensively evaluates the impacts of fasting, hypothermia, and hypometabolism on the gut microbiota of hibernating mammals. It suggests that alterations in the gut microbiota may contribute significantly to the maintenance of energy metabolism and intestinal immune function during hibernation, mediated by their metabolites. By delving into these intricacies, we can gain a deeper understanding of how hibernating mammals adapt to their environments and the consequences of dietary modifications on the symbiotic relationship between the gut microbiota and the host. Additionally, this knowledge can inform our comprehension of the protective mechanisms underlying long-term fasting in non-hibernating species, including humans, providing valuable insights into nutritional strategies and health maintenance.
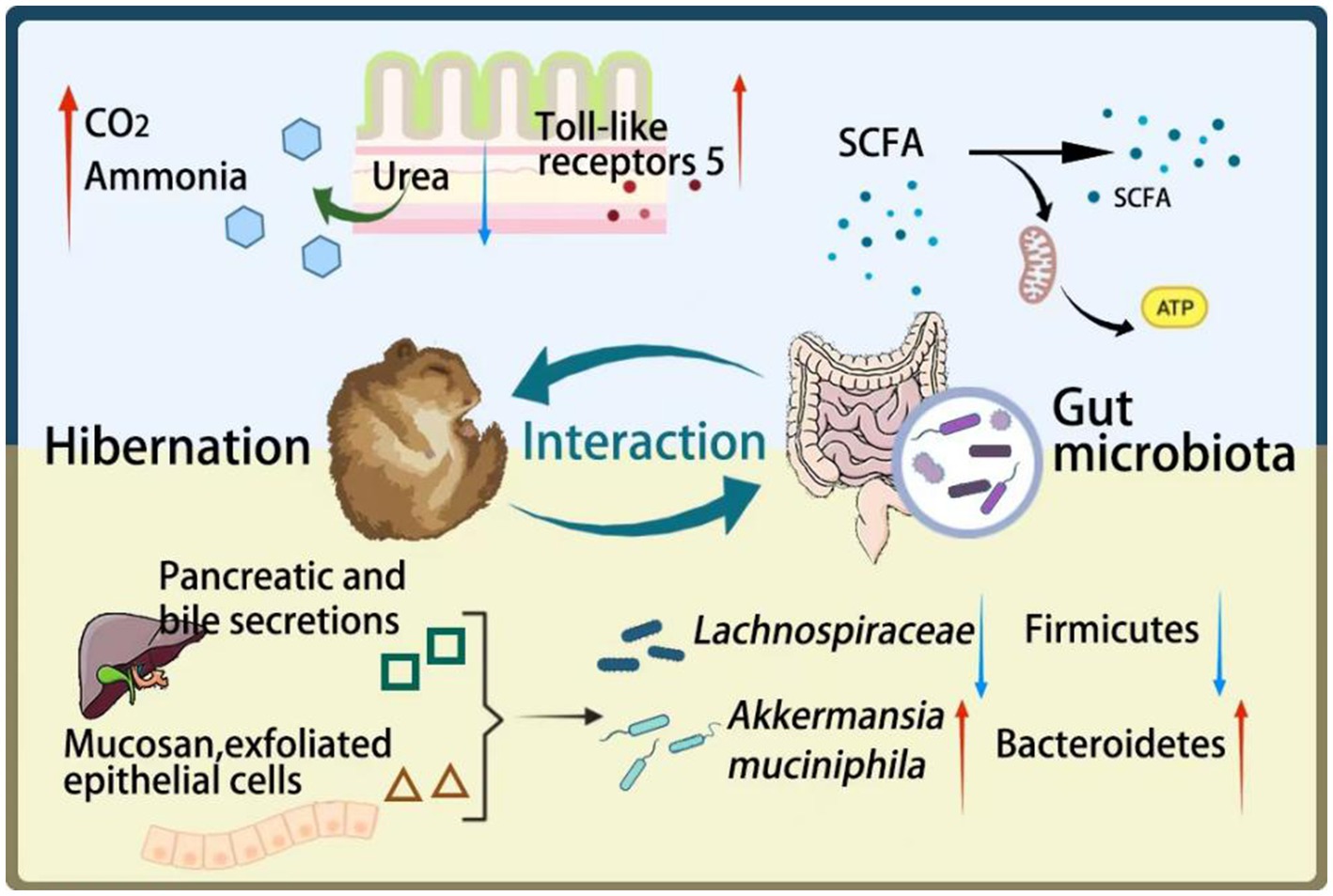
Graphical Abstract. During hibernation, there is an interaction between the gut microbiota and the host. The yellow part: Hibernation alters the composition of microbial communities. Due to fasting, bile and mucus from the epithelial cells of the digestive tract can serve as energy sources for some gut microbiota, leading to an increase in bacteria such as Akkermansia muciniphila and a decrease in bacteria such as Firmicutes. The blue part: Changes in gut microbiota may contribute to the maintenance of energy metabolism and intestinal immune function during hibernation through their metabolites. 1. The metabolic product SCFAs of gut microbiota will serve as the main energy substance. 2. The gut microbiota hydrolyzes urea in the intestine to produce ammonia, which can further synthesize amino acids and help produce new proteins for the host to utilize. 3. During hibernation, the expression of TLR5 in the intestine increases, which can minimize inflammation.
1 Introduction
Birds and mammals have evolved the capacity to maintain a consistently high body temperature, which allows them to perform essential survival activities independently of external temperature fluctuations (McNab, 2002). However, this physiological trait necessitates a substantial energy expenditure. To cope with this energetic demand, certain small mammals have adopted a unique physiological strategy called torpor. Torpor represents a temporary heterothermia, characterized by a reduction in body temperature, metabolic rate, and physiological function, occurring periodically within a day or during specific seasons as a response to ecological stressors (Lyman et al., 1982; Heldmaier et al., 2004; Dausmann et al., 2009; Geiser, 2013). This physiological adaptation is widespread among mammals and has also been observed in several bird species (Shankar et al., 2023; Heldmaier et al., 2004). Statistics indicate that 11 out of 25 mammalian orders exhibit torpor, highlighting its prevalence in vertebrate physiology (Heldmaier et al., 2004). Torpor manifests in two primary forms: daily torpor and hibernation. Daily torpor occurs without obvious seasonal patterns, typically lasting less than 24 h. During this period, the animal’s body temperature drops moderately, generally remaining above 20°C, and its metabolic rate during torpor is approximately 30% of its basal metabolic rate (BMR). In contrast, hibernation represents a prolonged torpor episode that occurs seasonally, often during winter. During hibernation, the animal’s body temperature can drop significantly, approaching ambient temperatures. For instance, the Arctic Ground Squirrel (Urocitellus parryii) has been recorded to reach a body temperature of −2.9°C during deep hibernation (Barnes, 1989). Metabolic rates during hibernation can decrease to as little as 5% of BMR, and hibernation bouts can last from several days to weeks (Heldmaier et al., 2004; Geiser, 2013; Ruf and Geiser, 2015). Notably, hibernation is not a continuous state; animals periodically awaken, dividing the hibernation period into distinct bouts (Geiser, 2013).
The significance of torpor in mammalian survival is multifaceted. It conserves energy, enabling animals to cope with the challenges of low temperatures and food scarcity during winter. It also provides a means of avoiding natural predators, as hibernating mammals often seek shelter in caves or other concealed locations. Additionally, it potentially extends lifespan, as evidenced by reduced telomere shortening rates in hibernating species such as the Edible Dormouse (Glis glis) and Djungarian Hamster (Phodopus sungorus) (Turbill et al., 2013; Turbill et al., 2012). Collectively, these adaptations contribute to enhancing the survival rates of hibernating mammals and, from a broader perspective, may contribute to slowing down the extinction rate of species (Geiser and Turbill, 2009).
The intricate composition, intricate structure, and diverse functionality of gut microbiota exert profound influences on the overall health status of their hosts. These microbiota are intricately linked to numerous physiological processes within the host, including metabolism, behavioral patterns, nutritional absorption, and immunological responses (Carey and Assadi-Porter, 2017; Makki et al., 2018; Di Vincenzo et al., 2024). Furthermore, the gut microbiota dynamically adapts its composition and activity in response to the available food resources within the digestive tract and the varying nutritional states of the host, particularly during fasting or hibernation. This comprehensive review examines the specific impacts of hibernation on the gut microbiota and its associated metabolites in animals. It delves into the intricate relationship between the alterations in gut microbiota during hibernation and crucial physiological processes such as host energy metabolism, gut immune function, and nitrogen cycling. By elucidating these interconnections, this paper provides valuable insights and serves as a reference for future investigations into the gut microbiota of hibernating mammals, thus advancing our understanding of the microbiota’s role in the adaptation and survival of animals during periods of inactivity and limited food availability.
2 Effects of hibernation on gut microbiota
The combined effects of mammals and gut microbiota have created a complex relationship, which has brought benefits to both sides. The effects of fasting, hypothermia, and low metabolic rate during hibernation on gut microbiota were discussed in the following text.
2.1 Effects of fasting (nutritional restriction) on gut microbiota diversity and structure
For hibernators, eating a lot before hibernation and long-term fasting during hibernation, this natural circulating food intake provides an opportunity to check the response of the intestinal microbiome to changes in substrate availability. Hibernation typically leads to a reduction in the diversity of the gut microbiota. During hibernation, the alpha diversity were significantly decreased compared to other periods, which have been found in 13-lined ground squirrel (Ictidomys tridecemlineatus), Daurian ground squirrel (Spermophilus Dauricus), greater horseshoe bat (Rhinolophus ferrumequinum), and brown bear (Ursus arctos) (Bao et al., 2023; Yang et al., 2021; Xiao et al., 2019; Sommer et al., 2016). The competition of limited resources in the intestines of hibernators may be an important factor in the formation of microbiota. During hibernation, due to a long-term lack of food intake, only the substrate derived from the host’s intestine (including mucosaccharides, exfoliated epithelial cells, and pancreatic and bile secretions) to support the survival of the intestinal microbiota (Leser et al., 2000; Martens et al., 2008; Backhed et al., 2005). Most host-derived substrates in the mammalian gut are gel-forming mucins, complex glycoproteins produced in large quantities by intestinal goblet cells that can coat the protective mucous layer above the epithelium (McGuckin et al., 2011). In fact, only a few intestinal bacteria have the ability to completely degrade mucin (Derrien et al., 2010; Hoskins et al., 1985; Martens et al., 2008; Salyers et al., 1977), for example, Akkermansia muciniphila, which can use mucin as carbon and nitrogen source (Derrien et al., 2010; Derrien et al., 2004). Bacteria that cannot use mucin, such as Lachnospiraceae and Lactobacillus, which feed on monosaccharides, are almost non-existent during hibernation. Bacteroidetes typically utilize starch, cellulose, and pectin as substrates, but the increase in the relative abundance of Bacteroidetes during hibernation may be due to their ability to metabolize host mucosaccharides, enabling them to metabolize host polysaccharides when dietary polysaccharides are scarce (Sonnenburg et al., 2005).
The gastrointestinal microbiota of hibernating mammals exhibits a distinctive composition, characterized by notable fluctuations in the abundance of specific bacterial groups that are adapted to the physiological conditions associated with torpor. A significant body of research indicates that Bacteroides, a genus of bacteria known for their ability to break down complex polysaccharides, consistently dominates the intestinal ecosystem of various hibernating species such as the 13-lined ground squirrel (Ictidomys tridecemlineatus), Arctic ground squirrel (Spermophilus parryii), and brown bear (Ursus arctos) during their period of inactivity (Dill-Mcfarland et al., 2014; Stevenson et al., 2014; Sommer et al., 2016). However, these general trends are subject to exceptions reflective of the complex adaptive strategies employed by different microbial communities. In particular, studies have shown a reduced representation of the Prevotella within the gut microbiota of 13-lined ground squirrels and Arctic ground squirrels during hibernation, contrasted against non-hibernating periods (Dill-Mcfarland et al., 2014; Stevenson et al., 2014). This observation is attributed to the limited capacity of Prevotella spp. to degrade host-derived mucous polysaccharides, which become the predominant substrates in the absence of dietary intake (Ley, 2016). Conversely, the family Enterobacteriaceae, known for its capacity to metabolize intestinal mucopolysaccharides, has been observed to increase in relative abundance in hibernating brown bears (Ma et al., 2012). The restructuring of the gut microbiota during hibernation appears to be driven by substrate preferences of the constituent microorganisms. Those capable of utilizing host-derived substrates, such as members of the Verrucomicrobia and certain taxa within the Bacteroidetes phylum, may undergo proliferation due to their ability to modulate carbohydrate-degrading enzymes in response to variations in nutrient availability (Sonnenburg et al., 2005). Concomitantly, there is often a depletion of microbial species that rely on dietary polysaccharides, including many within the Firmicutes phylum, suggesting a selective pressure favoring those adapted to metabolic host-derived compounds over those specialized in plant polysaccharide degradation. Table 1 summarized the changes in gut microbiota during mammalian hibernation.
However, hibernation patterns also vary among different hibernators. Depending on the specific strategies they employ to acquire energy during their inactive period, hibernating mammals can be categorized into two groups: fat-storing hibernators and food-storing hibernators. Fat-storing hibernators accumulate energy-rich substances primarily in the form of body fat to sustain them through the winter, whereas food-storing hibernators stockpile a significant quantity of non-decaying seeds before entering hibernation to ensure a constant source of nourishment (Humphries et al., 2003). Remarkably, despite their different energetic approaches, the gut microbiota of both fat-storing and food-storing hibernators share notable similarities (Xiao et al., 2019). Food-storing hibernators, such as hamsters, exhibit minimal changes in their microbiota composition during hibernation, suggesting a stability that is maintained even under conditions of reduced food intake. However, when these animals are subjected to fasting conditions similar to those experienced by ground squirrels (for instance, a four-day fast), they display microbiota alterations that are comparable to those observed in the latter species (Sonoyama et al., 2009). Collectively, these studies provide compelling evidence that a core intestinal microbiota may exist during hibernation in mammals. This core microbiota appears to be resilient and capable of maintaining functional stability across diverse hibernation strategies, highlighting the adaptability and resilience of the gut microbiome in response to the physiological challenges of hibernation.
However, these studies offer an incomplete understanding of the intricate relationship between hibernation, dietary habits, and the microbiome, considering that squirrels, for instance, maintain a minimum body temperature that approaches or slightly dips below 0°C during hibernation. Consequently, the specific factors that drive microbiota differences during hibernation, whether dietary intake, hypothermic conditions, or a combination of both, remain elusive. Until 2016, Sommer et al. made a significant discovery in brown bears, a large hibernating mammal. They observed a notable reduction in gut microbiota diversity during hibernation, accompanied by a decrease in the relative abundance of Firmicutes and an increase in Bacteroidetes (Sommer et al., 2016). Notably, brown bears do not experience a drastic drop in body temperature during hibernation, suggesting that winter fasting in these animals primarily accounts for the depletion of degradable substrates that typically support gut microbial growth. This deprivation may significantly influence the structure of the microbial community and its interactions with the host. Consistent with these findings, similar patterns have been observed in studies focusing solely on fasting. For instance, the proportion of A. maciniphila within the microbiota of fasting hamsters increased, while the proportion of Firmicutes decreased (Sonoyama et al., 2009). Similarly, mice subjected to a 24-h fasting period exhibited an increase in Bacteroidetes and a decrease in Firmicutes in their intestinal tract (Crawford et al., 2009). These results collectively indicate that fasting during hibernation is a critical factor that significantly shapes the composition and proportion of the gut microbiota.
2.2 Effects of low temperature on gut microbiota
In addition to fasting, hypothermia—characterized by a significant drop in body temperature—emerges as a pivotal factor that shapes the composition and structure of the gut microbiota. Animals, in their natural habitats, must adapt to fluctuations in food availability and temperature extremes, resulting in dynamic changes in the species and proportions of gut microorganisms (Stevenson et al., 2014). This adaptation process is particularly evident in wildlife species residing in diverse environments. For instance, the plateau pika (Ochotona curzoniae), a species that inhabits alpine regions with harsh climatic conditions, exhibits a higher diversity of gut microorganisms compared to its low-altitude counterpart, the Daurian pika (Ochotona daurica) (Li et al., 2018). This observation suggests that cold environments can significantly impact the composition of the gut microbiota. Similarly, studies on Brandt’s voles (Lasiopodomys brandtii) have revealed that exposure to low temperatures modifies the composition and proportions of gut bacteria, such as an increase in Ruminococcus and Helicobacter species. While these studies primarily focus on ambient temperature, it is noteworthy that for homeothermic animals, variations in body temperature serve as the direct influencing factors on the gut microbiota. Dill-Mcfarland et al. conducted research demonstrating that as body temperature decreases in homeothermic animals, there are consequent changes in the gut microbiota. These include a decrease in bacterial diversity, a reduction in the relative abundance of Firmicutes, and a decrease in pathogenic bacteria like Salmonella. Concurrently, there is an increase in the number of Bacteroidetes (Dill-Mcfarland et al., 2016). Therefore, during hibernation, where animals experience both limited food resources and hypothermic conditions, both factors play crucial roles in determining the composition and structure of the gut microbiota. Hibernation, thus, represents a unique physiological state that significantly alters the gut microbial ecosystem, necessitating further investigation to fully understand its complex interactions and potential consequences for animal health.
2.3 Effects of hypometabolism on gut microbiota
Hibernation, an energy-conserving survival strategy utilized by numerous mammalian species, is characterized by a significant reduction in metabolic rate (Storey and Storey, 2004). This reduction, which can plummet to merely 4.3% of the animal’s basal metabolic rate (Ruf and Geiser, 2015), is pivotal in maintaining energy homeostasis during periods of limited food availability. During hibernation, the gut microbiota of hibernating mammals assumes a crucial role in maintaining the health and function of the intestinal tract by feeding on intestinal substrates. Recent studies, such as the one conducted by Bo et al., have revealed that modifications in the gut microbiota composition of hibernating mammals, such as Brandt’s voles, are significantly associated with the host’s resting metabolic rate (RMR) after undergoing low-temperature domestication or microbial transplantation. Similarly, in laboratory mice, alterations in the gut microbiota have been intimately linked to both RMR and nonshivering thermogenesis (NST). These findings suggest that the gut microbiota plays a pivotal role in regulating metabolic processes in hibernating mammals. Among the various microbial species present in the gut, Desulfovibrio has been identified as a key player. Earley et al. (2015) demonstrated that Desulfovibrio can metabolize sulfates derived from mucin, ultimately producing hydrogen sulfide (H2S). Notably, H2S, as a potent inhibitor of cytochrome C oxidase, is implicated in metabolic suppression during hibernation (Nicholls et al., 2013; Revsbech et al., 2014). While the exact mechanisms underlying the interaction between hibernation, metabolic suppression, and gut microbiota remain incompletely understood, it is reasonable to speculate that the low metabolism observed during hibernation is among the factors that shape and influence the composition and activity of the gut microbiota.
3 Effects of hibernation on gut microbiota metabolites
The genetic diversity of gut microbiota during hibernation endows this intricate system with an array of enzymes and biochemical metabolic pathways that are absent in the host. This diversity enables the microbiota to harness energy through the fermentation and catabolism of polysaccharides (including starch, cellulose, and pectin) as well as unabsorbed oligosaccharides. The breakdown of these diverse substrates results in the production of distinct metabolites, each exerting unique physiological effects on the host. Among the metabolites generated by the gut microbiota, short-chain fatty acids (SCFAs) such as acetic acid, propionic acid, butyric acid, and valeric acid stand out as particularly significant. Once absorbed by the host, these SCFAs serve as a source of energy (McNeil, 1984; Koh et al., 2016). Specifically, butyric acid fulfills the energetic demands of intestinal epithelial cells and ensures the proper growth of intestinal mucosal cells. Meanwhile, propionic acid contribute to the host’s glucose metabolism (Venter et al., 1990). When comparing intestinal SCFAs concentrations between hibernation and summer periods (assuming similar body temperatures), a decrease of approximately 30% is observed during hibernation. SCFA levels reach their nadir during the hibernation phase but subsequently rise during arousal, potentially attributed to the stimulatory effect of elevated body temperature on bacterial enzyme activity. Additionally, hibernation induces alterations in the molar ratio of individual SCFAs (Carey et al., 2013). During hibernation, the gut microbiota undergoes spontaneous structural reorganization, modifying its composition. Under conditions of prolonged fasting, these microorganisms maintain a balanced relationship with their hosts, often characterized as mutualistic. This is evident as intestinal epithelial cells derive 60–70% of their energy requirements from SCFAs produced by microbial metabolism (Ardawi and Newsholme, 1985). Conversely, the epithelial cells provide a favorable niche for the hibernating microbiota to persist by offering components such as epithelial cells and mucin glycans for their survival (Carey and Martin, 1996). Notably, the impact of hibernation on individual SCFAs varies. Acetate maintains the highest molar ratio throughout all seasons, with its proportion further increasing during hibernation (Stevenson et al., 2014). These changes in metabolites are summarized in Table 2, highlighting the dynamic interplay between hibernation, gut microbiota, and their metabolic outputs.
Acetate: During hibernation, the proportion of acetate rises, partially due to an increase in Akkermansia muciniphila. Microbially-derived acetate can affect hibernation physiology in multiple ways. Intestinal epithelial cells and peripheral gut tissues can utilize acetate as an energy source, and acetate transported to the liver can also be used for the synthesis of fatty acids and cholesterol (Macfarlane and Macfarlane, 2003). Acetate contributes to ketogenesis in the liver (Crawford et al., 2009), which can serve as an alternative fuel to glucose, providing energy to the brain and heart (Andrews et al., 2009), protecting the heart from ischemia–reperfusion injury, and facilitating the transition of animals from a hypometabolic to a hypermetabolic state upon arousal from hibernation.
Butyrate: During hibernation, butyrate levels in the host’s gut decrease to very low levels. This may be attributed to the reduction of certain types of metabolizable substrates and alterations in the composition of the microbiota. The lack of complex plant polysaccharides during hibernation diminishes the Firmicutes phylum, subsequently reducing butyrate production, as most major butyrate producers, including Roseburia, Eubacterium, and Faecaliberium, belong to the Firmicutes (Duncan et al., 2007). The lower cecal butyrate concentration in winter may contribute to changes in the gut structure and function of hibernating squirrels. As the preferred fuel source for epithelial cells, butyrate stimulates epithelial cell proliferation and recovery, and aids in maintaining the integrity of the intestinal barrier by regulating apoptosis, permeability, and mucus production (Louis and Flint, 2009; Peng et al., 2007; Brahe et al., 2013).
Propionate: In hibernating mammals, the proportion of propionate remains unchanged (12–13%) (Stevenson et al., 2014). Propionat produced by microbial metabolism, is a substrate for gluconeogenesis, another metabolic function crucial for maintaining blood glucose levels during hibernation (Galster and Morrison, 1975).
Lactic acid and succinic acid: During animal hibernation, bacteria in the gut microbiota that produce lactic acid, such as Lactobacillus and Lactococcus (Wells and Mercenier, 2008), decrease in their relative abundance, leading to a decrease in lactate levels in the intestine. L-Lactate can serve as an important source of energy and regulate lipid and carbohydrate metabolism in aerobic environments. In hibernating brown bears, the relative abundance of Enterobacteriaceae significantly increases compared to non hibernating periods. The increase in succinic acid content during hibernation is related to the relative abundance of succinic acid producing bacteria such as Enterobacteriaceae (Sommer et al., 2016). Succinic acid is an intermediate substance in the tricarboxylic acid cycle and plays an important role in ATP generation (Mills and O’Neill, 2014).
4 Effects of gut microbiota on the host during hibernation
4.1 Gut microbiota affects energy metabolism
The resident gut microbiota significantly influences the host’s nutritional uptake and energy homeostasis by augmenting the extraction of energy from ingested food and facilitating its storage within the body (Bäckhed et al., 2004; Crawford et al., 2009). This microbial community contributes substantially to the maintenance of energy metabolic phenotypes in hibernating mammals. Through fecal microbiota transplantation experiments using brown bears, it has been discovered that the microbial communities native to these animals can modulate recipient mice phenotypes, resulting in increased body weight, obesity, and insulin resistance (Sommer et al., 2016). The gut microbiota, along with its metabolites such as short-chain fatty acids (SCFAs), play an integral role in maintaining equilibrium within the host’s glucose and lipid metabolism, affecting processes like glycolysis, lipogenesis, and insulin sensitivity (Zhang et al., 2023; Wang et al., 2023; Anhê et al., 2023). Research findings from Bao et al. (2023) suggest that Himalayan marmots exhibit a dietary preference for unsaturated fatty acids (UFA) prior to hibernation, hypothesized to facilitate fat accretion in these animals. Concurrently, the class Firmicutes, specifically the cluster CAG: 110, partakes in UFA synthesis, thus aiding in supporting the host’s metabolic processes during hibernation. In a subsequent fecal transplantation experiment, there was an observed increment in the weight of the recipient mice, further corroborating the upregulating effect of Firmicutes CAG: 110 on lipid metabolism during the hibernation phase. In hibernaors that store fat, specific lipid types fulfill distinct functional roles in both regular physiology and during hibernation. Lipids such as cholesterol and fatty acids can regulate pathways associated with torpor. For instance, dietary cholesterol enrichment in chipmunks enhances hibernation parameters (Kolomiytseva, 2011), and provisioning Alpine marmots with an omega-6 fatty acid-rich diet can ameliorate survival rates during hibernation (Ruf and Arnold, 2008). Studies on bears also reveal that a specialized lipid metabolic mode is instrumental in preventing diabetes during hibernation (Tekin et al., 2023). Therefore, during the hibernation period, the gut microbiota undergo transformations due to fasting and reduced temperatures. Such changes are instrumental in sustaining the energy metabolic phenotype throughout the hibernation cycle (Figure 1).
4.2 Gut microbiota affects immunity
During hibernation, fasting, low temperature conditions, and alterations in gut microbiota converge to induce significant remodeling of the host’s intestinal immune system. A depletion of SCFAs is hypothesized to be the primary culprit behind the atrophy of the small intestinal mucosa and the subsequent elevation in intestinal barrier permeability, as described by Carey (1992). This increase in permeability poses a greater risk for bacterial translocation and the hyperactivation of the immune system. Butyrate, being the primary energy source for epithelial cells, its absence results in suppressed proliferation of these cells, decreased integrity of the intestinal barrier, and consequently, elevated intestinal permeability (Gaudier et al., 2004; Louis and Flint, 2009; Bugaut and Bentéjac, 1993). Nonetheless, the gut microbiota plays a pivotal role in maintaining immunity during hibernation. Notably, during hibernation, there is a reduction in the number of microorganisms within the intestinal lumen, coupled with an increase in those adherent to the mucosal layer. The proximity of these mucosal microorganisms to the intestinal epithelium allows for direct interactions with host cells and indirect regulation of the immune system through the diffusion of microbial-associated molecular patterns (MAMPs) or their metabolites (Van den Abbeele et al., 2011). Research on the 13-lined ground squirrel demonstrates that hibernation is associated with shortened caecal crypts and enhanced MUC2 expression during early winter and spring compared to summer. Additionally, hibernation is observed to decrease the expression of TLR4 and increase TLR5 in the caecum, suggestive of a protective response aimed at minimizing inflammation. Activation of TLR5 by its ligand, flagellin, elicits a protective anti-inflammatory response and maintains intestinal barrier function (Cullender et al., 2013). Therefore, the intricate interaction between toll-like receptor expression in epithelial cells and the mucosal microbiota appears to constitute a protective mechanism for intestinal immunity during hibernation (Carey et al., 2013). This enhancement of mucosal immunity serves to minimize inflammation triggered by microbial leakage from the intestine, thereby safeguarding intestinal health (Maynard et al., 2012).
Alterations in the immunological status are frequently correlated with the detection of host-derived microbial signals, which may contribute to the establishment and maintenance of a mutualistic and tolerogenic equilibrium (Maynard et al., 2012). The period of hibernation is characterized by shifts in the gut microbiota and their metabolic byproducts, which can elicit an augmentation in the population of intraepithelial and lamina proprialymphocytes, augmented expression of immunoglobulin A (IgA), and heightened concentrations of mucosal cytokines (Kurtz and Carey, 2007; Hooper et al., 2012). The role of IgA as a pivotal regulatory element in the intricate relationship between the commensal microbiota, epithelial cells, and the immune system is well established (Peterson et al., 2007; Sutherland and Fagarasan, 2012). The hibernation phase also induces an enhanced distribution of occludin within the intestinal and caecal epithelia, a tight junction protein instrumental in fortifying the intestinal barrier, particularly under conditions of inflammation (Carey et al., 2012; Turner, 2009). Concurrently, the hibernation state is associated with an upregulation of mucosal interleukin-10 (IL-10), an efficacious anti-inflammatory cytokine that fosters immunological tolerance toward the resident microbial community (Flint et al., 2012; Hooper et al., 2002). Furthermore, the intestinal epithelium’s defensive mechanisms in hibernating species are reinforced through an increased expression of proteins that counteract apoptosis (Dill-McFarland et al., 2014).
The intestinal microbiota performs a pivotal function in immunity. For instance, Bacteroides thetaiotaomicron demonstrates the ability to degrade host glycans, even in the absence of dietary polysaccharides. This bacterium not only assists the host in efficiently utilizing nutrients, such as carbohydrates, but it also enhances immune responses and contributes significantly to maintaining the delicate balance of the intestinal microecosystem (Flint et al., 2012; Koropatkin et al., 2012). During hibernation, a noteworthy shift occurs in the intestinal microbiota of brown bears, specifically with an increase in the proportion of Coprococcus species. This bacterium is capable of producing butyrate, a compound that is essential for preserving the integrity and function of the intestinal barrier. Additionally, Akkermansia muciniphila stimulates the production of mucus by the host, thereby strengthening the integrity of epithelial cells (Derrien et al., 2004; Geerlings et al., 2018). This bacterial species further enhances intestinal immune function during hibernation, ensuring that the bear maintains a robust immune defense despite its inactive state.
4.3 Gut microbiota affects urea metabolism
The urea nitrogen cycle plays a pivotal role in maintaining protein homeostasis and averting ammonia toxicity in animals during hibernation (Lee et al., 2012; Rice et al., 2020). Mammalian hibernators employ sophisticated mechanisms to minimize nitrogen losses during prolonged winter fasting, thereby preserving vital nutrients (Nelson, 1989). Within the intestine, the gut microbiota facilitates the hydrolysis of urea into carbon dioxide and ammonia. Subsequently, this ammonia can be further metabolized into amino acids, which contribute to the biosynthesis of new proteins (Carey and Assadi-Porter, 2017; Regan et al., 2022). This microbial “recycling” of urea nitrogen back to the host represents a crucial aspect of host-microbiota cometabolism, significantly contributing to the metabolic phenotype of hibernating mammals. In ground squirrel species, the downregulation of urea cycle enzymes during hibernation suggests a preferential utilization of bacterial-derived urea nitrogen for amino acid synthesis rather than urea production (Epperson et al., 2010). Consequently, during winter hibernation, both the plasma urea concentration and intestinal NH3 levels are significantly reduced in ground squirrels compared to their summer state (Regan et al., 2022). Notably, the enhanced nitrogen metabolism observed during hibernation exhibits a negative correlation with various physiological parameters such as body weight, food intake, blood glucose, and body temperature in Daurian ground squirrels. This suggests that the cecal microbiota of these animals plays a vital role in converting potential toxic substances through nitrogen metabolism, thereby providing supplemental energy to the host during hibernation. Regan et al.’s study further highlights the ability of ground squirrels to harness their gut microbiota for urea nitrogen recycling, ensuring protein balance even in the absence of dietary nitrogen sources (Regan et al., 2022). This mechanism confers two key benefits to hibernating mammals: Firstly, it enhances protein synthesis during periods of nitrogen scarcity, crucial for ground squirrels approaching the breeding season during the later stages of hibernation, potentially translating into reproductive advantages. Secondly, the urea nitrogen cycle redirects urea away from the kidneys, reducing the water requirements for urine production and thus conserving precious bodily resources for hibernating mammals.
5 Significance of hibernation microbiota research
The intricate co-evolutionary between mammals and their gut microbiota has engendered a highly complex and mutually beneficial relationship. This symbiosis is characterized by the changes in microbial structure, modulation of intestinal structure and function, stimulation of immune system development, and enhancement of dietary energy extraction. At the same time, the host mammal provides an array of nutritional and environmental niches tailored to the microbiota’s needs, while also shaping its composition and structure through genetic predisposition, immune status, intestinal milieu, and dietary patterns.
The exploration of the gut microbiota in hibernating mammals holds particular scientific significance due to several key reasons. Firstly, hibernating mammals present a unique and naturally occurring model system for elucidating the intricate relationship between gut microbiota and host co-evolution. Secondly, investigating the dynamic shifts in gut microbiota composition during hibernation cycles offers insights into the microbiota’s regenerative capabilities and its impact on hibernators’ rapid transition into reproductive readiness following arousal. Thirdly, the study of gut microbiota in hibernators and their urea nitrogen salvage mechanisms reveals a functional role for intestinal microorganisms in this adaptive physiological process, providing valuable knowledge about the microbiota’s role in metabolic regulation. Lastly, understanding the adaptive mechanisms employed by hibernators and their gut microbiota to cope with nutritional deprivation during winter fasting can inform our comprehension of potential protective mechanisms against long-term fasting in non-hibernating species, such as humans. This knowledge has the potential to inform nutritional strategies, therapeutic interventions, and even inform the design of novel microbiome-based therapeutics.
Author contributions
PG: Software, Writing – original draft. WS: Formal analysis, Writing – original draft. TB: Conceptualization, Funding acquisition, Supervision, Writing – review & editing.
Funding
The author(s) declare that financial support was received for the research, authorship, and/or publication of this article. This research was supported by the Natural Science Foundation of Beijing Municipality [5242016], the National Natural Science Foundation of China for Youth [No. 32200381], Fundamental Research Funds for the Central Universities [BLX202266], Young Elite Scientists Sponsorship Program by CAST [2021QNRC001], and Science & Technology Fundamental Resources Investigation Program [2023FY100305].
Conflict of interest
The authors declare that the research was conducted in the absence of any commercial or financial relationships that could be construed as a potential conflict of interest.
Publisher’s note
All claims expressed in this article are solely those of the authors and do not necessarily represent those of their affiliated organizations, or those of the publisher, the editors and the reviewers. Any product that may be evaluated in this article, or claim that may be made by its manufacturer, is not guaranteed or endorsed by the publisher.
References
Andrews, M. T., Russeth, K. P., Drewes, L. R., and Henry, P. G. (2009). Adaptive mechanisms regulate preferred utilization of ketones in the heart and brain of a hibernating mammal during arousal from torpor. Am. J. Phys. Regul. Integr. Comp. Phys. 296, R383–R393. doi: 10.1152/ajpregu.90795.2008
Anhê, F. F., Zlitni, S., Zhang, S. Y., Choi, B. S., Chen, C. Y., Foley, K. P., et al. (2023). Human gut microbiota after bariatric surgery alters intestinal morphology and glucose absorption in mice independently of obesity. Gut 72, 460–471. doi: 10.1136/gutjnl-2022-328185
Ardawi, M. S. M., and Newsholme, E. A. (1985). Fuel utilization in colonocytes of the rat. Biochem. J. 231, 713–719. doi: 10.1042/bj2310713
Bäckhed, F., Ding, H., Wang, T., Hooper, L. V., Koh, G. Y., Nagy, A., et al. (2004). The gut microbiota as an environmental factor that regulates fat storage. Proc. Natl. Acad. Sci. 101, 15718–15723. doi: 10.1073/pnas.0407076101
Backhed, F., Ley, R. E., Sonnenburg, J. L., Peterson, D. A., and Gordon, J. I. (2005). Host-bacterial mutualism in the human intestine. Science 307, 1915–1920. doi: 10.1126/science.1104816
Bao, Z., Guo, C., Chen, Y., Li, C., Lei, T., Zhou, S., et al. (2023). Fatty acid metabolization and insulin regulation prevent liver injury from lipid accumulation in Himalayan marmots. Cell Rep. 42:112718. doi: 10.1016/j.celrep.2023.112718
Barnes, B. M. (1989). Freeze avoidance in a mammal: body temperatures below 0 degree in an arctic hibernator. Science 244, 1593–1595. doi: 10.1126/science.2740905
Brahe, L. K., Astrup, A., and Larsen, L. H. (2013). Is butyrate the link between diet, intestinal microbiota and obesity-related metabolic diseases? Obes. Rev. 14, 950–959. doi: 10.1111/obr.12068
Bugaut, M., and Bentéjac, M. (1993). Biological effects of short-chain fatty acids in nonruminant mammals. Annu. Rev. Nutr. 13, 217–241. doi: 10.1111/obr.12068
Carey, H. V. (1992). Effects of fasting and hibernation on ion secretion in ground squirrel intestine. Am. J. Phys. Regul. Integr. Comp. Phys. 263, R1203–R 1208. doi: 10.1152/ajpregu.1992.263.6.R1203
Carey, H. V., and Assadi-Porter, F. M. (2017). The hibernator microbiome: host-bacterial interactions in an extreme nutritional Symbiosis. Annu. Rev. Nutr. 37, 477–500. doi: 10.1146/annurev-nutr-071816-064740
Carey, H. V., and Duddleston, K. N. (2014). Animal-microbial symbioses in changing environments. J. Therm. Biol. 44, 78–84. doi: 10.1016/j.jtherbio.2014.02.015
Carey, H. V., and Martin, S. L. (1996). Preservation of intestinal gene expression during hibernation. American journal of physiology-gastrointestinal and liver. Physiology 271, G805–G813. doi: 10.1152/ajpgi.1996.271.5.G805
Carey, H. V., Pike, A. C., Weber, C. R., Turner, J. L., Visser, A., Beijer-Liefers, S. C., et al. (2012). Impact of hibernation on gut microbiotaand intestinal barrier function in ground squirrels. Living Seasonal World, 281–291. doi: 10.1007/978-3-642-28678-0_25
Carey, H. V., Walters, W. A., and Knight, R. (2013). Seasonal restructuring of the ground squirrel gut microbiota over the annual hibernation cycle. Am. J. Phys. Regul. Integr. Comp. Phys. 304, 33–42. doi: 10.1152/ajpregu.00387.2012
Crawford, P. A., Crowley, J. R., Sambandam, N., Muegge, B. D., Costello, E. K., Hamady, M., et al. (2009). Regulation of myocardial ketone body metabolism by the gut microbiota during nutrient deprivation. Proc. Natl. Acad. Sci. 106, 11276–11281. doi: doi: 10.1073/pnas.0902366106
Cullender, T. C., Chassaing, B., Janzon, A., Kumar, K., Muller, C. E., Werner, J. J., et al. (2013). Innate and adaptive immunity interact to quench microbiome flagellar motility in the gut. Cell Host Microbe 14, 571–581. doi: 10.1016/j.chom.2013.10.009
Dausmann, K. H., Glos, J., and Heldmaier, G. (2009). Energetics of tropical hibernation. J Comp Physiol B. 179, 345–57. doi: 10.1007/s00360-008-0318-0
Derrien, M., van Passel, M. W., van de Bovenkamp, J. H., Schipper, R. G., de Vos, W., and Dekker, J. (2010). Mucin-bacterial interactions in the human oral cavity and digestive tract. Gut Microbes 1, 254–268. doi: 10.4161/gmic.1.4.12778
Derrien, M., Vaughan, E. E., Plugge, C. M., and de Vos, W. M. (2004). Akkermansia muciniphila gen. Nov., sp. nov., a human intestinal mucin-degrading bacterium. Int. J. Syst. Evol. Microbiol. 54, 1469–1476. doi: 10.1099/ijs.0.02873-0
Di Vincenzo, F., Del Gaudio, A., Petito, V., Lopetuso, L. R., and Scaldaferri, F. (2024). Gut microbiota, intestinal permeability, and systemic inflammation: a narrative review. Intern. Emerg. Med. 19, 275–293. doi: 10.1007/s11739-023-03374-w
Dill-Mcfarland, K. A., Neil, K. L., Zeng, A., Sprenger, R. J., Kurtz, C. C., Suen, G., et al. (2014). Hibernation alters the diversity and composition of mucosa-associated bacteria while enhancing antimicrobial defence in the gut of 13-lined ground squirrels. Mol. Ecol. 23, 4658–4669. doi: 10.1111/mec.12884
Dill-Mcfarland, K. A., Suen, G., and Carey, H. V. (2016). Bears arouse interest in Microbiota's role in health. Trends Microbiol. 24, 245–246. doi: 10.1016/j.tim.2016.01.011
Duncan, S. H., Belenguer, A., Holtrop, G., Johnstone, A. M., Flint, H. J., and Lobley, G. E. (2007). Reduced dietary intake of carbohydrates by obese subjects results in decreased concentrations of butyrate and butyrate-producing bacteria in feces. Appl. Environ. Microbiol. 73, 1073–1078. doi: 10.1128/AEM.02340-06
Earley, H., Lennon, G., Balfe, A., Kilcoyne, M., Clyne, M., Joshi, L., et al. (2015). A preliminary study examining the binding capacity of Akkermansia muciniphila and Desulfovibrio spp., to colonic mucin in health and ulcerative colitis. PLoS One 10:e0135280. doi: 10.1371/journal.pone.0135280
Epperson, L. E., Rose, J. C., Carey, H. V., and Martin, S. L. (2010). Seasonal proteomic changes reveal molecular adaptations to preserve and replenish liver proteins during ground squirrel hibernation. Am. J. Phys. Regul. Integr. Comp. Phys. 298, R329–R340. doi: 10.1152/ajpregu.00416.2009
Flint, H. J., Scott, K. P., Duncan, S. H., Louis, P., and Forano, E. (2012). Microbial degradation of complex carbohydrates in the gut. Gut Microbes 3, 289–306. doi: 10.4161/gmic.19897
Galster, W., and Morrison, P. R. (1975). Gluconeogenesis in arctic ground squirrels between periods of hibernation. Amer J Physiol Legacy Content 228, 325–330. doi: 10.1152/ajplegacy.1975.228.1.325
Gaudier, E., Jarry, A., Blottiere, H. M., de Coppet, P., Buisine, M. P., Auber, T. J. P., et al. (2004). Butyrate specififically modulates MUC gene expression in intestinal epithelial goblet cells deprived of glucose. American journal of physiology-gastrointestinal and liver. Physiology 287:G1168-G 1174. doi: 10.1152/ajpgi.00219.2004
Geerlings, S. Y., Kostopoulos, I., De Vos, W. M., and Belzer, C. (2018). Akkermansia muciniphila in the human gastrointestinal tract: when, where, and how? Microorganisms 6:75. doi: 10.3390/microorganisms6030075
Geiser, F., and Turbill, C. (2009). Hibernation and daily torpor minimize mammalian extinctions. Naturwissenschaften 96, 1235–1240. doi: 10.1007/s00114-009-0583-0
Heldmaier, G., Ortmann, S., and Elvert, R. (2004). Natural hypometabolism during hibernation and daily torpor in mammals. Respir. Physiol. Neurobiol. 141, 317–329. doi: 10.1016/j.resp.2004.03.014
Hooper, L. V., Littman, D. R., and Macpherson, A. J. (2012). Interactions between the microbiota and the immune system. Science 336, 1268–1273. doi: 10.1126/science.1223490
Hooper, L. V., Midtvedt, T., and Gordon, J. I. (2002). How host-microbial interactions shape the nutrient environment of the mammalian intestine. Annu. Rev. Nutr. 22, 283–307. doi: 10.1146/annurev.nutr.22.011602.092259
Hoskins, L. C., Agustines, M., McKee, W. B., Boulding, E. T., Kriaris, M., and Niedermeyer, G. (1985). Mucin degradation in human colon ecosystems. Isolation and properties of fecal strains that degrade ABH blood group antigens and oligosaccharides from mucin glycoproteins. J. Clin. Invest. 75, 944–953. doi: 10.1172/JCI111795
Humphries, M. M., Thomas, D. W., and Kramer, D. L. (2003). The role of energy availability in mammalian hibernation: a cost-benefit approach. Physiol. Biochem. Zool. 76, 165–179. doi: 10.1086/367950
Koh, A., De Vadder, F., Kovatcheva-Datchary, P., and Bäckhed, F. (2016). From dietary Fiber to host physiology: short-chain fatty acids as key bacterial metabolites. Cell 165, 1332–1345. doi: 10.1016/j.cell.2016.05.041
Kolomiytseva, I. K. (2011). Lipids in mammalian hibernation and artificial hypobiosis. Biochemistry (Mosc) 76, 1291–1299. doi: 10.1134/S0006297911120029
Koropatkin, N. M., Cameron, E. A., and Martens, E. C. (2012). How glycan metabolism shapes the human gut microbiota. Nat. Rev. Microbiol. 10, 323–335. doi: 10.1038/nrmicro2746
Kurtz, C. C., and Carey, H. V. (2007). Seasonal changes in the intestinal immune system of hibernating ground squirrels. Develop. Comp. Immunol. 31, 415–428. doi: doi: 10.1016/j.dci.2006.07.003
Lee, T. N., Buck, C. L., Barnes, B. M., and O’Brien, D. M. (2012). A test of alternative models for increased tissue nitrogen isotope ratios during fasting in hibernating arctic ground squirrels. J Exp Biol. 1, 3354–61. doi: 10.1242/jeb.068528
Leser, T. D., Lindecrona, R. H., Jensen, T. K., Jensen, B. B., and Moller, K. (2000). Changes in bacterial community structure in the colon of pigs fed different experimental diets and after infection with Brachyspira hyodysenteriae. Appl. Environ. Microbiol. 66, 3290–3296. doi: 10.1128/AEM.66.8.3290-3296.2000
Ley, R. E. (2016). Dausmann Nat. Rev. Gastroenterol. Hepatol. 13, 69–70. doi: 10.1038/nrgastro.2016.4
Li, H., Qu, J., Li, T., Wirth, S., Zhang, Y., Zhao, X., et al. (2018). Diet simplification selects for high gut microbial diversity and strong fermenting ability in high-altitude pikas. Appl. Microbiol. Biotechnol. 102, 6739–6751. doi: 10.1007/s00253-018-9097-z
Louis, P., and Flint, H. J. (2009). Diversity, metabolism and microbial ecology of butyrate-producing bacteria from the human large intestine. FEMS Microbiol. Lett. 294, 1–8. doi: 10.1111/j.1574-6968.2009.01514.x
Lyman, C. P., Willis, J. S., Malan, A., and Wang, L. C. (1982). Hibernation and torpor in mammals and birds. New York: Academic Press.
Ma, Q., Qu, Y. Y., Tang, H. Z., Yu, H., Ma, F., Shi, S. G., et al. (2012). Genome sequence of a novel indigo-producing strain, Pseudomonas monteilii QM. J. Bacteriol. 194, 4459–4460. doi: 10.1128/JB.00867-12
Macfarlane, S., and Macfarlane, G. T. (2003). Regulation of short-chain fatty acid production. Proc. Nutr. Soc. 62, 67–72. doi: 10.1079/PNS2002207
Makki, K., Deehan, E. C., Walter, J., and Bäckhed, F. (2018). The impact of dietary Fiber on gut microbiota in host health and disease. Cell Host Microbe 23, 705–715. doi: 10.1016/j.chom.2018.05.012
Martens, E. C., Chiang, H. C., and Gordon, J. I. (2008). Mucosal glycan foraging enhances fifitness and transmission of a saccharolytic human gut bacterial symbiont. Cell Host Microbe 4, 447–457. doi: 10.1016/j.chom.2008.09.007
Maynard, C. L., Elson, C. O., Hatton, R. D., and Weaver, C. T. (2012). Reciprocal interactions of the intestinal microbiota and immune system. Nature 489, 231–241. doi: 10.1038/nature11551
McGuckin, M. A., Lindén, S. K., Sutton, P., and Florin, T. H. (2011). Mucin dynamics and enteric pathogens. Nat. Rev. Microbiol. 9, 265–278. doi: 10.1038/nrmicro2538
McNab, K. B. (2002). Short-term energy conservation in endotherms in relation to body mass, habits, and environment. J. Therm. Biol. 27, 459–466. doi: 10.1016/S0306-4565(02)00016-5
McNeil, N. I. (1984). The contribution of the large intestine to energy supplies in man. Am. J. Clin. Nutr. 39, 338–342. doi: 10.1093/ajcn/39.2.338
Mills, E., and O’Neill, L. A. J. (2014). Succinate: a metabolic signal in inflammation. Trends Cell Biol. 24, 313–320. doi: 10.1016/j.tcb.2013.11.008
Nelson, R. A. (1989). “Nitrogen conservation and its turnover in hibernation” in Living Cold. eds. A. Malan and B. Canguilhem (London: Libbey Eurotext), 299–307.
Nicholls, P., Marshall, D. C., Cooper, C. E., and Wilson, M. T. (2013). Sulfide inhibition of and metabolism by cytochrome c oxidase. Biochem. Soc. Trans. 41, 1312–1316. doi: 10.1042/BST20130070
Peng, L., He, Z., Chen, W., Holzman, I. R., and Lin, J. (2007). Effects of butyrate on intestinal barrier function in a Caco-2 cell monolayer model of intestinal barrier. Pediatr. Res. 61, 37–41. doi: 10.1203/01.pdr.0000250014.92242.f3
Peterson, D. A., McNulty, N. P., Guruge, J. L., and Gordon, J. I. (2007). IgA response to symbiotic bacteria as a mediator of gut homeostasis. Cell Host Microbe. 2, 328–339. doi: 10.1016/j.chom.2007.09.013
Regan, M. D., Chiang, E., Liu, Y., Tonelli, M., Verdoorn, K. M., Gugel, S. R., et al. (2022). Nitrogen recycling via gut symbionts increases in ground squirrels over the hibernation season. Science 375, 460–463. doi: 10.1126/science.abh2950
Revsbech, I. G., Shen, X., Chakravarti, R., Jensen, F. B., Thiel, B., Evans, A. L., et al. (2014). Hydrogen sulfide and nitric oxide metabolites in the blood of free-ranging brown bears and their potential roles in hibernation. Free Radic. Biol. Med. 73, 349–357. doi: 10.1016/j.freeradbiomed.2014.05.025
Rice, S. A., Ten Have, G.A.M., Reisz, J. A., Gehrke, S., Stefanoni, D., Frare, C., et al. (2020). Nitrogen recycling buffers against ammonia toxicity from skeletal muscle breakdown in hibernating arctic ground squirrels. Nat Metab. 2, 1459–1471. doi: 10.1038/s42255-020-00312-4
Ruf, T., and Arnold, W. (2008). Effects of polyunsaturated fatty acids on hibernation and torpor: a review and hypothesis. Am. J. Phys. Regul. Integr. Comp. Phys. 294, R1044–R1052. doi: 10.1152/ajpregu.00688.2007
Ruf, T., and Geiser, F. (2015). Daily torpor and hibernation in birds and mammals. Biol. Rev. 90, 891–926. doi: 10.1111/brv.12137
Salyers, A. A., Vercellotti, J. R., West, S. E., and Wilkins, T. D. (1977). Fermentation of mucin and plant polysaccharides by strains of Bacteroides from the human colon. Appl. Environ. Microbiol. 33, 319–322. doi: 10.1128/AEM.33.2.319-322.1977
Shankar, A., Welch, K. C., Eberts, E. R., Geiser, F., Halter, S., Keicher, L., et al. (2023). Daily torpor in birds and mammals: past, present, and future of the field. Integr. Comp. Biol. 63, 1017–1027. doi: 10.1093/icb/icad095
Sommer, F., Ståhlman, M., Ilkayeva, O., Arnemo, J. M., Kindberg, J., Josefsson, J., et al. (2016). The gut microbiota modulates energy metabolism in the hibernating brown bear Ursus arctos. Cell Rep. 14, 1655–1661. doi: 10.1016/j.celrep.2016.01.026
Sonnenburg, J. L., Jian, X., Leip, D. D., Chen, C., Westover, B. P., Weatherford, J., et al. (2005). Glycan foraging in vivo by an intestine-adapted bacterial symbiont. Science 307, 1955–1959. doi: 10.1126/science.1109051
Sonoyama, K., Fujiwara, R., Takemura, N., Ogasawara, T., Watanabe, J., Ito, H., et al. (2009). Response of gut microbiota to fasting and hibernation in Syrian hamsters. Appl. Environ. Microbiol. 75, 6451–6456. doi: 10.1128/AEM.00692-09
Stevenson, T., Duddleston, K., and Buck, C. (2014). Effects of season and host physiological state on the diversity, density, and activity of the arctic ground squirrel cecal microbiota. Appl. Environ. Microbiol. 80, 5611–5622. doi: 10.1128/AEM.01537-14
Storey, K. B., and Storey, J. M. (2004). Metabolic rate depression in animals: transcriptional and translational controls. Biol. Rev. 79, 207–233. doi: 10.1017/s1464793103006195
Sutherland, D. B., and Fagarasan, S. (2012). Iga synthesis: a form of functional immune adaptation extending beyond gut. Curr. Opin. Immunol. 24, 261–268. doi: 10.1016/j.coi.2012.03.005
Tekin, H., Frøbert, O., Græsli, A. R., Kindberg, J., Bilgin, M., and Buschard, K. (2023). Hibernation and plasma lipids in free-ranging brown bears-implications for diabetes. PLoS One 18:e0291063. doi: 10.1371/journal.pone.0291063
Turbill, C., Ruf, T., Smithand, S., and Bieber, C. (2013). Seasonal variation in telomere length of a hibernating rodent. Biol. Lett. 9:20121095. doi: 10.1098/rsbl.2012.1095
Turbill, C., Smith, S., Deimel, C., and Ruf, T. (2012). Daily torpor is associated with telomere length change over winter in Djungarian hamsters. Biol. Lett. 8, 304–307. doi: 10.1098/rsbl.2011.0758
Turner, J. R. (2009). Intestinal mucosal barrier function in health and disease. Nat. Rev. Immunol. 9, 799–809. doi: 10.1038/nri2653
Van den Abbeele, P., Van de Wiele, T., Verstraete, W., and Possemiers, S. (2011). The host selects mucosal and luminal associations of coevolved gut microorganisms: a novel concept. FEMS Microbiol. Rev. 35, 681–704. doi: 10.1111/j.1574-6976.2011.00270.x
Venter, C. S., Vorster, H. H., and Cummings, J. H. (1990). Effects of dietary propionate on carbohydrate and lipid metabolism in healthy volunteers. Amer. J. Gastroenterol. 85, 549–53.
Wang, Y., Wang, M., Chen, J., Li, Y., Kuang, Z., Dende, C., et al. (2023). The gut microbiota reprograms intestinal lipid metabolism through long noncoding RNA Snhg9. Science 381, 851–857. doi: 10.1126/science.ade0522
Wells, J. M., and Mercenier, A. (2008). Mucosal delivery of therapeutic and prophylactic molecules using lactic acid bacteria. Nat. Rev. Microbiol. 6, 349–362. doi: 10.1038/nrmicro1840
Xiao, G. H., Liu, S., Xiao, Y. H., Zhu, Y., Zhao, H. B., Li, A. Q., et al. (2019). Seasonal changes in gut microbiota diversity and composition in the greater horseshoe bat. Front. Microbiol. 10:2247. doi: 10.3389/fmicb.2019.02247
Yang, X., Yao, Y., Zhang, X., Zhong, J., Gao, F., Zhang, H., et al. (2021). Seasonal changes in the distinct taxonomy and function of the gut microbiota in the wild ground squirrel (Spermophilus dauricus). Animals 11:2685. doi: 10.3390/ani11092685
Zhang, B., Fan, X., Du, H., Zhao, M., Zhang, Z., Zhu, R., et al. (2023). Foodborne carbon dot exposure induces insulin resistance through gut microbiota Dysbiosis and damaged intestinal mucus layer. ACS Nano 17, 6081–6094. doi: 10.1021/acsnano.3c01005
Keywords: gut microbiota, hibernation, metabolite, immune, SCFAs
Citation: Gao P, Shen W and Bo T (2024) The interaction between gut microbiota and hibernation in mammals. Front. Microbiol. 15:1433675. doi: 10.3389/fmicb.2024.1433675
Edited by:
Benoit St-Pierre, South Dakota State University, United StatesReviewed by:
Xiaolong Hu, Jiangxi Agricultural University, ChinaXiaowei Song, Chengdu University of Information Technology, China
Copyright © 2024 Gao, Shen and Bo. This is an open-access article distributed under the terms of the Creative Commons Attribution License (CC BY). The use, distribution or reproduction in other forums is permitted, provided the original author(s) and the copyright owner(s) are credited and that the original publication in this journal is cited, in accordance with accepted academic practice. No use, distribution or reproduction is permitted which does not comply with these terms.
*Correspondence: Tingbei Bo, Ym90aW5nYmVpQGJqZnUuZWR1LmNu