- 1Key Laboratory for Quality Regulation of Livestock and Poultry Products of Hunan Province, College of Animal Science and Technology, Hunan Agricultural University, Changsha, China
- 2Yuelushan Laboratory, Changsha, China
Akkermansia muciniphila (A. muciniphila), an intestinal symbiont residing in the mucosal layer, shows promise as a probiotic. Our previous study found that the abundance of A. muciniphila was significantly higher in Ningxiang suckling piglets compared to other breeds, suggesting that early breast milk may play a crucial role. This study examines A. muciniphila’s ability to utilize Ningxiang pig milk oligosaccharides. We discovered that A. muciniphila can thrive on both Ningxiang pig colostrum and purified pig milk oligosaccharides. Genetic analysis has shown that A. muciniphila harbors essential glycan-degrading enzymes, enabling it to effectively break down a broad spectrum of oligosaccharides. Our findings demonstrate that A. muciniphila can degrade pig milk oligosaccharides structures such as 3′-FL, 3′-SL, LNT, and LNnT, producing short-chain fatty acids in the process. The hydrolysis of these host-derived glycan structures enhances A. muciniphila’s symbiotic interactions with other beneficial gut bacteria, contributing to a dynamic microbial ecological network. The capability of A. muciniphila to utilize pig milk oligosaccharides allows it to establish itself in the intestines of newborn piglets, effectively colonizing the mucosal layer early in life. This early colonization is key in supporting both mucosal and metabolic health, which is critical for enhancing piglet survival during lactation.
1 Introduction
Ensuring the survival and robust health of newborn piglets is crucial for the economic sustainability of pig farming (Heuß et al., 2019). Notably, approximately 15–20% of piglets succumb within the first 3 days post-birth, primarily due to inadequate immune development and environmental stressors (Bæk et al., 2019; Farmer and Edwards, 2022). Finding certain measures to address these challenges is critical for enhancing piglet survival and overall production efficiency.
Colostrum plays a vital dual role in the early life of piglets. The intake of colostrum within the first 24 h post-birth is essential, not only nourishing the piglets but also fortifying their systemic immune systems, thereby promoting their growth and increasing their resilience against infections (Ogawa et al., 2016; Kogut and Zhang, 2022). Overall, it serves as an indispensable source of nutrients and crucially shapes the neonatal gut microbiota through its rich content of milk oligosaccharides (MOs) (Zivkovic et al., 2011). MOs are composed of a linear or branched backbone containing galactose, N-acetylglucosamine, and glucose, which can be decorated with fucose or sialic acid residues (Li et al., 2022). Although these complex carbohydrates are indigestible by piglets themselves, they are vital for the proliferation of specific beneficial bacteria. This strategic nourishment results in a beneficial microbiota composition that bolsters health and disease resistance, a reflection of evolutionary adaptations aimed at optimizing both infant survival and maternal health.
The Ningxiang breed is known among China’s four famous pig breeds for its resilience and notably low rates of diarrhea in neonates (Wang et al., 2014; Ma et al., 2022). In our previous investigations, we observed that Akkermansia muciniphila (A. muciniphila) was notably more prevalent in Ningxiang suckling piglets compared to other breeds (Unpublished data). This Gram-negative anaerobe from the Verrucomicrobia phylum is renowned for its ability to degrade mucin and colonize the mucus layer of the gastrointestinal tract and convert this polymer into mostly acetate and propionate (Dao et al., 2015; Zhang et al., 2019). The presence of A. muciniphila is beneficial for host metabolism and immunity, particularly in mitigating risks associated with chronic conditions such as obesity and diabetes (Zheng et al., 2023). The early colonization by A. muciniphila, evident from as early as the first month of life, plays a significant role in the developmental stages of the gut microbiota, facilitated by its ability to utilize MOs (Collado et al., 2007; Derrien et al., 2008).
Based on the above, we hypothesize that the significant presence of A. muciniphila in Ningxiang piglets is linked to its ability to metabolize pig milk oligosaccharides (PMOs), which, in turn, would have a beneficial effect on newborn piglets. In this study, an in vitro fermentation model to explore the fermentative behavior of A. muciniphila on PMOs derived from Ningxiang colostrum, examining growth kinetics and acid production as indicators of PMOs utilization. Through genomic analysis, we have identified the key enzymes responsible for the breakdown of these oligosaccharides, further reinforcing the potential role of A. muciniphila in promoting a healthy gut microbiota from the earliest stages of life. By understanding how specific microbial strains such as A. muciniphila exploit the unique glycobiome of breast milk, we pave the way for potential interventions aimed at fostering optimal microbial colonization patterns in early life, setting the stage for a healthier adult life.
2 Materials and methods
2.1 Materials
A. muciniphila DSM 22959 was purchased from the German Collection of Microorganisms and Cell Cultures (Braunschweig, Germany). 2′-fucosyllactose [2′-FL] (purity≥95%), 3-fucosyllactose [3′-FL] (purity≥95%), 3′-siallylactose [3′-SL] (purity≥95%), 6′-sialyllactose [6′-SL] (purity≥95%), lacto-N-tetraose [LNT] (purity≥95%) and lacto-N-neotetraose [LNnT] (purity≥95%) were kindly provided by Zhuo Wang and Siming Jiao, from State Key Laboratory of Biochemical Engineering, Institute of Process Engineering, Chinese Academy of Sciences (Beijing, China). All the reagents used for this study were of analytical grade.
2.2 Bacterial growth curves
A. muciniphila DSM 22959 growth were performed with cells grown in brain heart infusion broth medium (BHI) (1 mL per replicate) broth at 37°C in anaerobic chamber (Whitley A35 anaerobic workstation). Cells were inoculated in BHI broth supplemented with Ningxiang-pig colostrum (10% v/v), Ningxiang-pig purified PMOs (1 mg/mL), neutral trioses (2′-FL and 3′-FL), tetraoses (LNT, LNnT), and acidic trioses (3′-SL, 6′-SL) (10 mM respectively). Medium without any supplementation was included as a control. Cells were incubated anaerobically at 37°C until reaching stationary phase (48 h).
2.3 PMOs extraction
The PMOs were isolated and purified as previously described, with minor modification (Barile et al., 2010). Briefly, frozen milk samples were completely thawed, and a 20 mL aliquot of each sample was mixed with an equal volume of nanopure water and centrifuged at 14,000 × g in a microfuge for 30 min at 4°C to remove lipids. The top fat layer was removed, and 4 volumes of chloroform: methanol (2:1, vol/vol) were added, vigorously mixed, and the resulting emulsion was centrifuged at 4,000 × g for 30 min at 4°C. The upper methanol layer containing PMOs was transferred to a tube, 2 volumes of cold ethanol were added, and the solution was frozen for 1 h at −30°C, followed by centrifugation for 30 min at 4,000 × g and 4°C to precipitate the denatured protein. The supernatant (PMOs-rich fraction) was collected and further purified. Oligosaccharides were purified from the mixture by GCC-SPE. Prior to use, each GCC-SPE cartridge was activated with 3 column volumes of 80% acetonitrile (ACN) and 0.1% trifluoroacetic acid (TFA, vol/vol) and equilibrated with 3 column volumes of nanopure water. The carbohydrate-rich solution was loaded onto the cartridge, and salts and mono- or disaccharides were removed by washing with 10 column volumes of nanopure water. Then, it was eluted with a solution of 40% ACN with 0.1% TFA (vol/vol) in water and the solution was frozen for 1 h at −80°C, with freeze-dried using a lyophilizer overnight to collected purified PMO.
2.4 Carbohydrate-active enzyme (CAZyme) annotation
CAZymes within A. muciniphila DSM 22959 (NCBI Reference Sequence: GCF_008000975.1) was annotated using the dbCAN2 metaserver.1 CAZymes identified by at least two out of three tools were considered for further analysis.
2.5 Quantitative real-time PCR (qPCR)
The abundance of A. muciniphila was determined by qPCR as described previously10. Cells (1 mL) were harvested at 21,000 × g for 15 min. DNA extractions were performed using the MasterPure™ Gram Positive DNA Purification Kit (Epicentre, Lucigen, United States). DNA concentrations were measured fuorometrically (Qubit dsDNA BR assay, Invitrogen) and adjusted to 1 ng/μL prior to use as the template in qPCR. Primers targeting the 16S rRNA gene of A. muciniphila (5′-CAGCACGTGAAGGTGGGGAC-3′ and 5′-CCTTGCGGTTGGCTTCAGAT-3′; 327 bp) were used for quantification. A standard curve was prepared with nine standard concentrations from 100 to 108 gene copies/μL. qPCR was performed in triplicate with iQ SYBR green supermix (Bio-Rad, United States) in a total volume of 10 μL prepared with primers at 500 nM in 384-wells plates with the wells sealed with optical sealing tape. Amplification was performed with an iCycler (Bio-Rad): one cycle of 95°C for 10 min; 40 cycles of 95°C for 15 s, 60°C for 20 s, and 72°C for 30 s each; one cycle of 95°C for 1 min; and a stepwise increase of temperature from 60 to 95°C (at 0.5°C per 5 s) to obtain melt curve data. Data were analyzed using Bio-Rad CFX Manager 3.0. The copy number was corrected for the DNA concentration and for the number of 16S rRNA genes encoded in A. muciniphila’s genome.
2.6 SCFAs analysis
The concentrations of SCFAs in A. muciniphila fermentation were analyzed using the gas chromatographic (GC) method. Briefly, 1 mL A. muciniphila fermentation was first centrifuged at 15,000 × g for 10 min at 4°C. The samples were acidified with 25% metaphosphoric acid at a ratio of 1:5 for 30 min on ice. Samples were injected into a GC 8890 series gas chromatograph (Agilent, United States) for detection.
2.7 Statistical analysis
The data were analyzed using SPSS 26.0 statistical software (ver. 26.0 for Windows, SPSS Inc., Chicago, IL, United States). All data were analyzed by Student’s t-test and expressed as means with their standard errors. And *p < 0.05, **p < 0.01, and ***p < 0.001, Colostrum group compared with the CON group. #p < 0.05, ##p < 0.01, and ###p < 0.001 compared with the same treatment at different time.
3 Results
3.1 A. muciniphila DSM 22959 growth in Ningxiang-pig colostrum
Incubation of A. muciniphila DSM 22959 on Ningxiang-pig colostrum resulted in growth (Figure 1A). The results showed that presence of Ningxiang-pig colostrum in the basal medium (10% v/v) resulted in a modest increase in A. muciniphila growth in vitro (Figure 1A). And basal medium supplemented with Ningxiang-pig colostrum (10% v/v) significantly increased the cell number of A. muciniphila in vitro at 48 h (Figure 1B). Next, we determined the production of SCFAs by A. muciniphila DSM 22959 during fermentation of Ningxiang-pig colostrum at 24 and 48 h. Our results showed that fermentation of Ningxiang-pig colostrum significantly increased production of acetate, propionate and total SCFAs, regardless of whether it was 24 or 48 h (Figures 1C–E). Interestingly, the ratio of acetate increase (48 h/24 h) was superior in fermented Ningxiang pig colostrum compared to the increasing trend in the CON group (Figure 1C).
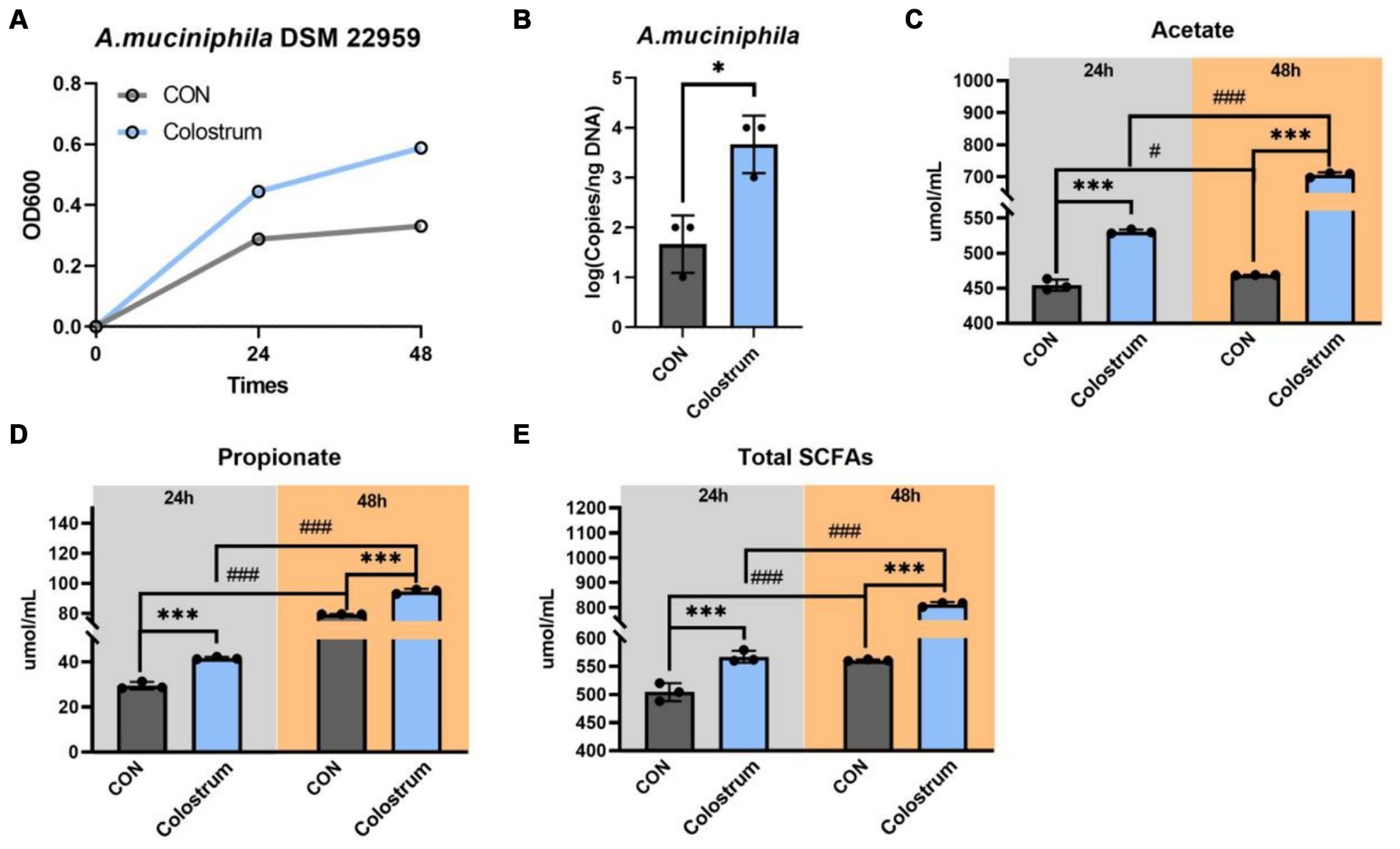
Figure 1. Akkermansia muciniphila DSM 22959 growth in Ningxiang-pig colostrum. (A) A. muciniphila DSM 22959 growth was assessed over a 48 h period by measuring optical density at a wavelength of 600 nm (OD600). Bacteria were grown in brain heart infusion broth medium (BHI), or BHI supplemented with Ningxiang-pig colostrum (10% v/v). (B) A. muciniphila DSM 22959 was grown in vitro in the absence or presence of 10% v/v Ningxiang-pig colostrum until 48 h of growth; A. muciniphila was quantified by qPCR. (C–E) Changes in SCFA levels in fermentation solutions. Data are expressed as the mean ± standard deviation (n = 3). *p < 0.05, and ***p < 0.001, Colostrum group compared with the CON group. #p < 0.05, and ###p < 0.001 compared with the same treatment at different time.
3.2 A. muciniphila DSM 22959 growth in Ningxiang-pig purified PMOs
Human milk oligosaccharides (HMOs) play an important role in the early nutrition of nursing infants and can act as substrates to support bacterial growth and thus dominate the gut early in life. In order to determine whether A. muciniphila DSM 22959 can utilize breast milk oligosaccharides to promote its own growth, we purified PMOs from the Ningxiang-pig colostrum (Figure 2A). Our results showed that supplemented with PMOs (1 mg/mL) increased the proliferation rate and significantly increased the cell number of A. muciniphila in vitro at 48 h (Figures 2B,C). Additionally, PMOs also significantly increased production of acetate, propionate and total SCFAs, regardless of whether it was 24 or 48 h (Figures 2D–F).
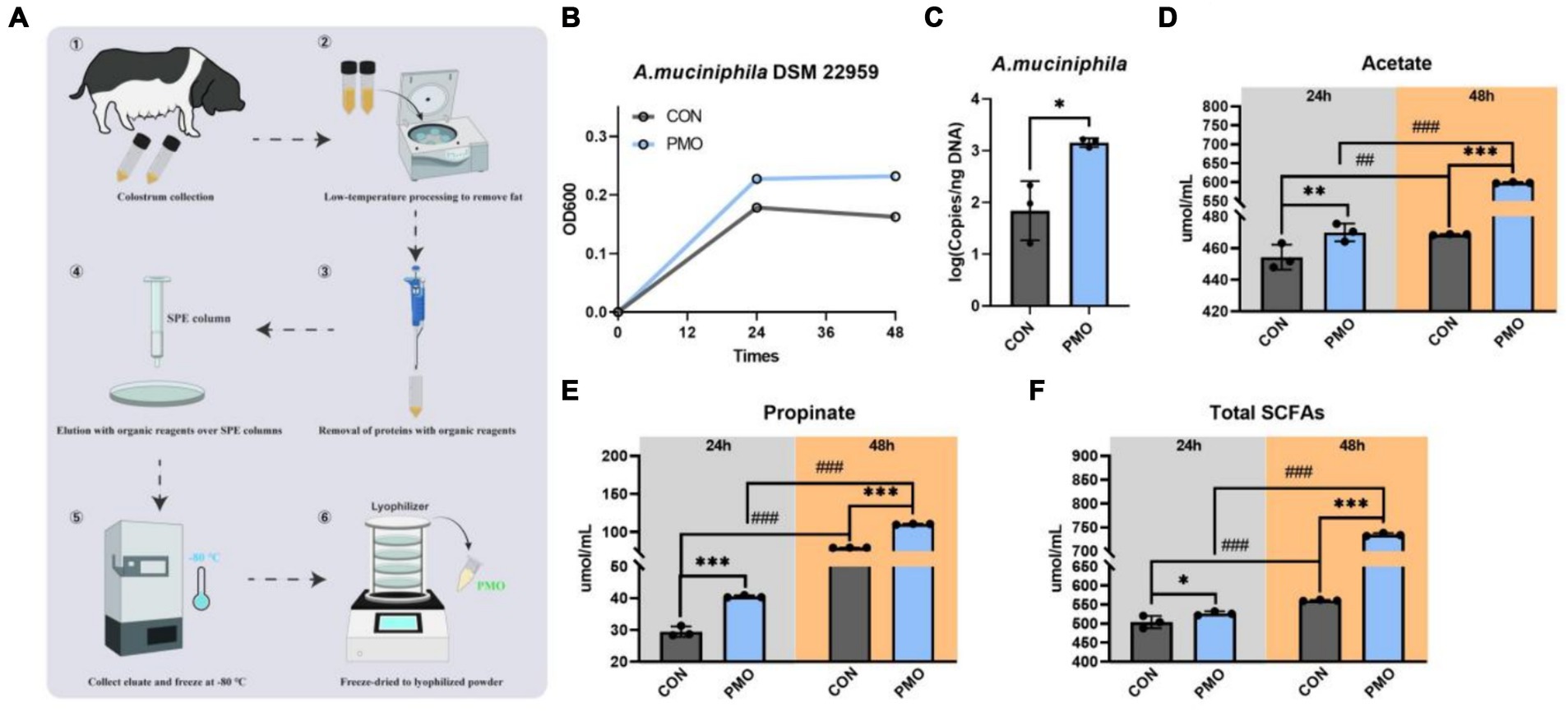
Figure 2. Akkermansia muciniphila DSM 22959 growth in Ningxiang-pig purified PMO. (A) Schematic illustration of Ningxiang-pig purified PMO preparation. (B) A. muciniphila DSM 22959 growth was assessed over a 48 h period by measuring optical density at a wavelength of 600 nm (OD600). Bacteria were grown in brain heart infusion broth medium (BHI), or BHI supplemented with Ningxiang-pig purified PMO (1 mg/mL). (C) A. muciniphila DSM 22959 was grown in vitro in the absence or presence of 1 mg/mL Ningxiang-pig purified PMO until 48 h of growth; A. muciniphila was quantified by qPCR. (D–F) Changes in SCFA levels in fermentation solutions. Data are expressed as the mean ± standard deviation (n = 3). *p < 0.05, **p < 0.01, and ***p < 0.001, Colostrum group compared with the CON group. ##p < 0.01, and ###p < 0.001 compared with the same treatment at different time.
3.3 Identification of CAZymes in A. muciniphila DSM 22959 genome
To investigate how A. muciniphila DSM 22959 can utilize complex PMO, we interrogated the available A. muciniphila DSM 22959 genome for the presence of CAZymes using the dbCAN2 metaserver pipeline for automated CAZyme annotation. This pipeline uses a combination of three different annotation tools (Diamond, HMMER and dbCAN_sub) to increase accuracy in predicting and annotating CAZymes within bacterial genomes. This analysis revealed a total of 165 putative ORFs with putative CAZy domains (Figure 3A).
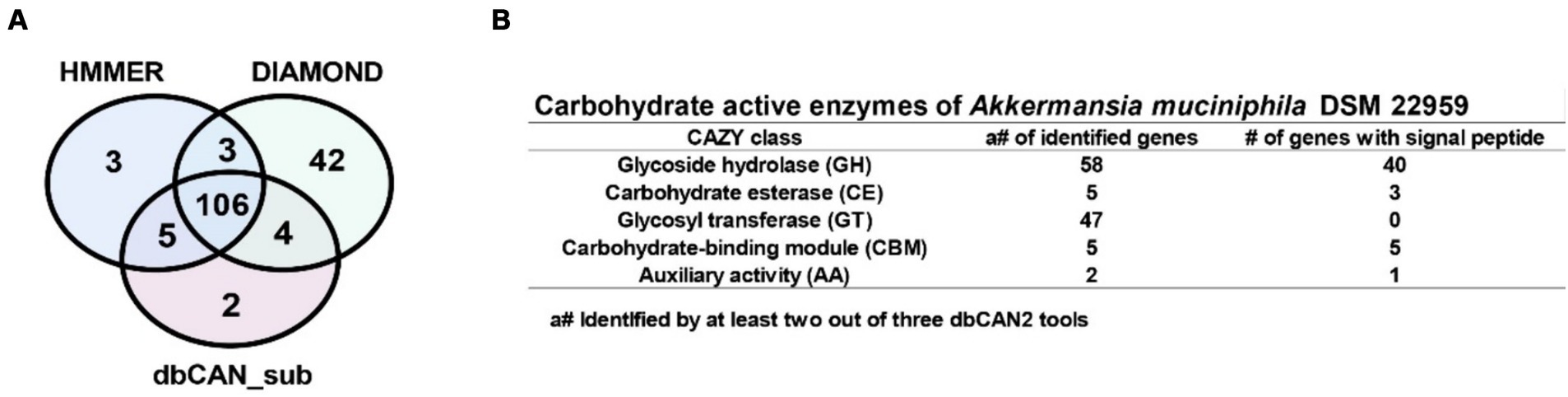
Figure 3. Identification of carbohydrate active enzymes (CAZymes) in A. muciniphila DSM 22959 genome. (A) Proportional Venn diagram showing the number of identified CAZyme encoding genes using the Diamond, HMMER and dbCAN_sub tools that are part of the dbCAN2 metaserver analysis. (B) The table shows the CAZymes and the presence of signal peptides, separated by CAZyme class, that were identified by at least two out of three dbCAN2 tools.
For increased accuracy, we discarded hits that were not identified by at least two out of three tools for further analysis. Among the remaining 118 ORFs multiple types of CAZy domains could be detected, such as glycoside hydrolase (GH), carbohydrate esterase (CE), glycosyl transferase (GT) domains, and carbohydrate-binding modules (CBM), with some genes encoding a combination of multiple domains (Figure 3B). Additionally, most of the putative ORFs belong to the GH and GT classes, and also encode signal peptides, suggests that A. muciniphila DSM 22959 was able to utilize multiple MOs.
3.4 The genome of A. muciniphila predicts the presence of a wide range of MOs targeting CAZymes
We performed further analysis on the A. muciniphila CAZy domains to identify the active enzymes that could contribute to the degradation of PMO (Figure 4A). Closer inspection of the Research Topic of CAZymes identified in the A. muciniphila DSM 22959 genome revealed at least 30 glycoside hydrolases that were predicted to utilize MOs, of which the majority contains a signal peptide (Figures 4B,C). Among the 30 glycoside hydrolases are two sialidases (GH33) and four fucosidases (GH29/95), which target the monosaccharides often found at terminal positions of MOs, and six different galactosidases (GH2) and 10 hexosaminidases (GH20/GH40), which are predicted to hydrolyze the underlying glycosidic linkages. The gene structure of the 30 putative enzymes, with the locations of the predicted glycoside hydrolase domains and additional domains clearly demonstrates the great diversity in domains, domain organization and predicted protein sizes – also between genes predicted to encode the same class of enzymes (Figure 4B). Transcription and translation of the entire repertoire of MO-targeting CAZymes would allow degradation of the wide variety of linkages commonly found within MOs. These data demonstrate that A. muciniphila DSM 22959 has the enzymatic capacity to utilize a broad range of MOs as well as their constituents.
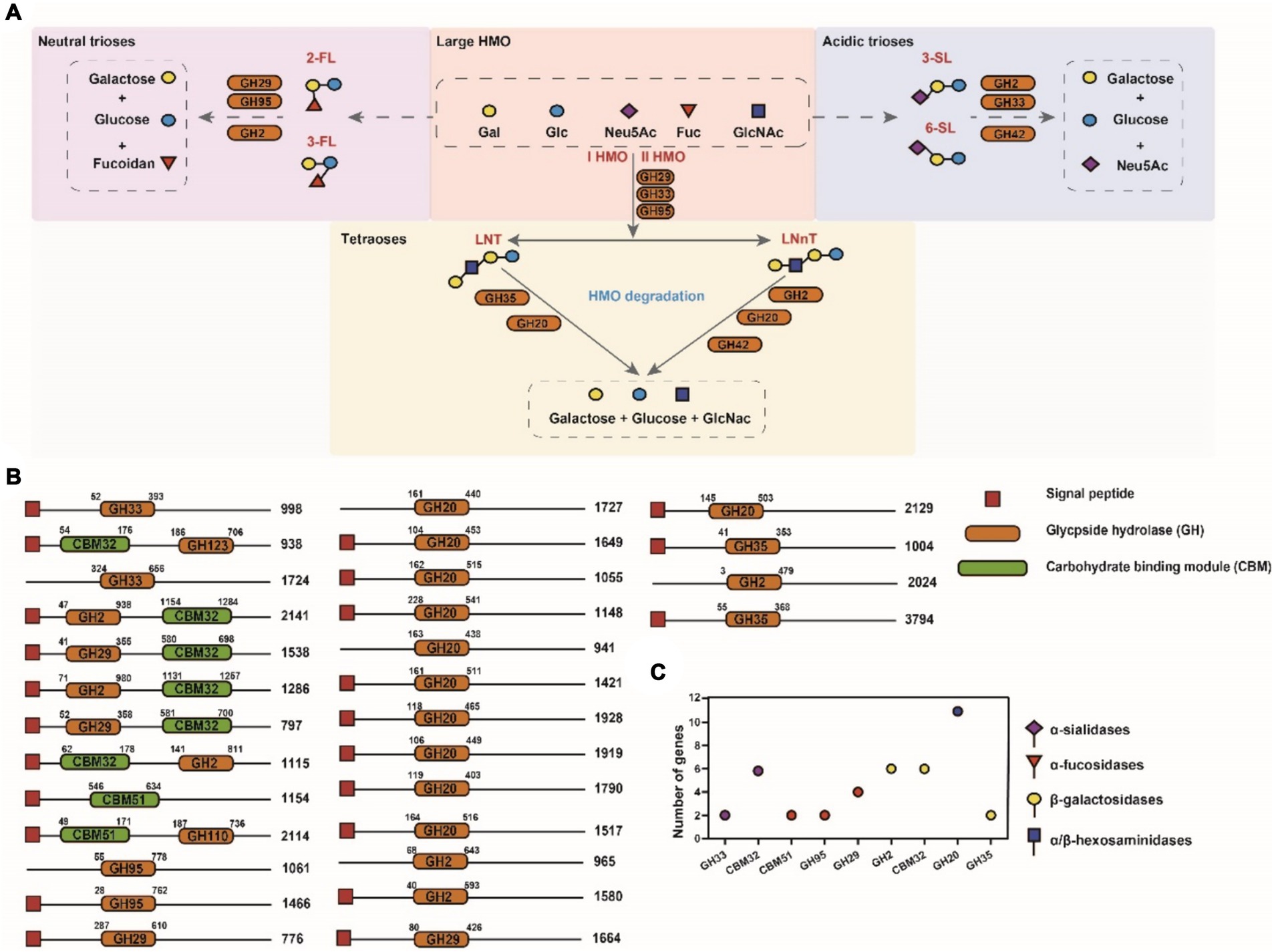
Figure 4. Domain architecture of putative MO targeting CAZymes in genome of A. muciniphila DSM 22959. (A) Simplified schematics of pathways involved in main HMO degradation (acidic trioses, neutral trioses and tetraoses). (B) CAZymes are divided in groups based upon their predicted enzyme activity: from top to bottom, α-sialidases, α-fucosidases, β-galactosidases and α/β-hexosaminidases. The displayed domains are those identified by HMMER, in number of amino acids, indicated at the right side of each protein. (C) The number of putative MO targeting CAZymes of A. muciniphila DSM 22959.
3.5 A. muciniphila DSM 22959 growth in acidic trioses, neutral trioses and tetraoses
We next sought to investigate which PMOs structures were utilized by A. muciniphila during the growth on colostrum. The results showed that A. muciniphila DSM 22959 was able to utilize acidic trioses, neutral trioses and tetraoses to grow, especially 3′-FL (Figure 5A). Interestingly, the two type of tetraoses (LNT, LNnT) can all support the growth of A. muciniphila DSM 22959, whereas only one type of acidic trioses (3′-FL) and neutral trioses (3′-SL) showed better utilization. Additionally, supplementation with 3′-FL significantly increased production of acetate, propionate and total SCFAs, regardless of whether it was 24 or 48 h (Figures 5B–D).
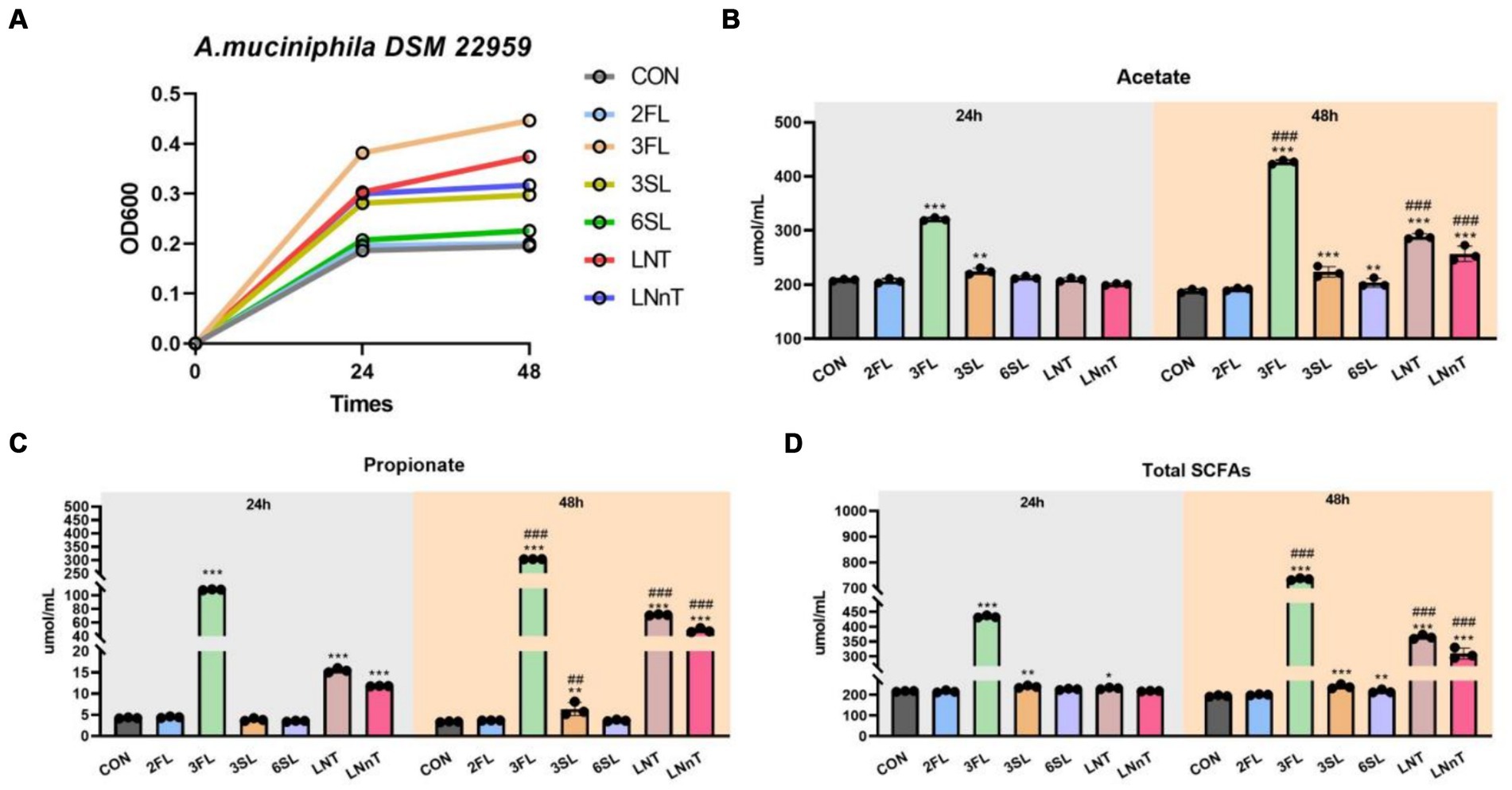
Figure 5. A. muciniphila DSM 22959 growth in acidic trioses, neutral trioses and tetraoses. (A) A. muciniphila DSM 22959 growth was assessed over a 48 h period by measuring optical density at a wavelength of 600 nm (OD600). Bacteria were grown in brain heart infusion broth medium (BHI), or BHI supplemented with neutral trioses (2-fucosyllactose [2-FL] and 3-fucosyllactose [3-FL]), tetraoses (lacto-N-tetraose [LNT], lacto-N-neotetraose [LNnT]), and acidic trioses (3-siallylactose [3-SL], 6-sialyllactose [6-SL]) (10 mM respectively). (B–D) Changes in SCFA levels in fermentation solutions. Data are expressed as the mean ± standard deviation (n = 3). *p < 0.05, **p < 0.01, and ***p < 0.001, Colostrum group compared with the CON group. ##p < 0.01, and ###p < 0.001 compared with the same treatment at different time.
4 Discussion
The survival rate of newborn piglets significantly impacts the economic viability of the pig industry, with a major focus in animal science being how to enhance piglet vigor through nutrition (Ji et al., 2019; Xu et al., 2019). Based on previous research findings, A. muciniphila was able to act as an early characterizing gut microbiota in Ningxiang piglets. A. muciniphila consumes mucin and exhibits beneficial effects on the host. Considering the structural similarities between MOs and intestinal mucus, it is plausible that the presence of PMOs in breast milk significantly contributes to the early colonization of A. muciniphila in piglets. Studies have identified negative associations between A. muciniphila and various diseases such as inflammatory bowel disease, diabetes, and hypertension, and its role in modulating responses to PD-1 blockers in cancer therapy (Derosa et al., 2022). Innovations in bacterial preparation, such as the use of cryopasteurized A. muciniphila, have shown metabolic improvements in mice, attributed to the activity of Amuc-1100 protein. This protein interacts with Toll-like receptor 2 to enhance intestinal barrier function and exerts probiotic benefits (Depommier et al., 2019). Furthermore, oral administration of A. muciniphila has been demonstrated to boost intestinal stem cell proliferation and promote the differentiation of Paneth and goblet cells, facilitating the regeneration of intestinal epithelia (Kim et al., 2021). Meanwhile, it has been showed that A. muciniphila can protect the intestinal health of weaned piglets from damage caused by ETEC infection (Lan et al., 2024).
Colostrum is indispensable for piglet development, serving not only as a vital nutrient source but also significantly shaping the neonatal gut microbiota through its rich content of MOs. Although piglets cannot digest these complex carbohydrates, they are crucial for the proliferation of beneficial bacteria. MOs, primarily undergraded by gastric acid and digestive enzymes, reach the hindgut to undergo microbial fermentation. As a principal carbohydrate source selectively utilized by the gut microbiota, MOs profoundly influence the microbial composition of the host’s gut microbiota and are deemed crucial regulators in the development of the neonatal gut microbiota. Extensive studies have shown that MOs provide substrates for the gut microbiota, thereby facilitating the colonization and survival of specific gut microbiota. The complete degradation of MOs, featuring diverse molecular structures, necessitates a variety of glycoside hydrolases or membrane transporters present in the neonatal gut microbiota, essential for the uptake, metabolism, and utilization of MOs by gut microbiota. Recent research has highlighted that certain Bifidobacteria spp. can utilize specific MOs in breast milk for their colonization and growth, thus benefiting the host’s immune development, particularly in early life (Lawson et al., 2020; Heiss et al., 2021; Henrick et al., 2021). Furthermore, the mechanisms of MOs degradation and utilization by Bifidobacteria spp. are categorized into strategies dependent on bacterial intracellular membrane translocation and those reliant on extracellular glycosidases (Katayama, 2016). Other bacteria like Bacteroides fragilis and Bacteroides vulgatus also exhibit high MOs metabolizing abilities (Yu et al., 2013), with Bacteroides fragilis possessing genes crucial for the metabolism of mucus-sugar conjugates (Marcobal et al., 2011). Our study’s detailed genomic analysis has identified specialized CAZymes in A. muciniphila, which are specifically adapted for breaking down PMOs from Ningxiang colostrum. These enzymes not only facilitate the ability of gut microbiota to establish and maintain a healthy microbial balance within the neonatal piglet gut but also enable the effective fermentation of a broad range of PMOs. This process highlights A. muciniphila’s critical role in shaping the gut microbiota of neonatal piglets, underscoring its evolutionary adaptability and ecological significance, similar to its beneficial functions in human infants.
The MOs have an enormous molecular weight that cannot be directly utilized by cell, but can be catabolized by gut microbiota, then producing SCFAs to respond to various life activities, such as immune regulation and glucose homeostasis (Zhu et al., 2020; Zhang et al., 2021; Chen et al., 2023). SCFAs are the main products of prebiotic fermentation, and the production of these metabolites depends on both dietary fiber types and gut microbiota composition (Bridgman et al., 2017). There is increasing evidence that gut microbial metabolites have wide systemic effects on host through acting as signaling molecules (Gasaly et al., 2021). SCFAs have been implicated in immune system development by modulating the production of immune mediators and regulating the activation and differentiation of immune cells (Yao et al., 2022; Liu et al., 2023). To investigate the metabolic performance of colostrum, purified PMOs and specific MOs fermentation by A. muciniphila dominant inocula, the yield of SCFAs were quantified. Our results showed that fermentation of Ningxiang-pig colostrum and purified PMOs significantly increased production of acetate, propionate and total SCFAs, regardless of whether it was 24 or 48 h. The production of SCFAs by A. muciniphila during the fermentation of pig colostrum oligosaccharides not only supports its own growth but also mediates significant health benefits for the host. In piglets, these SCFAs can enhance gut integrity, reduce pathogen colonization, and improve nutrient absorption—factors that are crucial for early development and long-term health (Li et al., 2021; Qin et al., 2023). By extending these findings, we can hypothesize that strategic dietary interventions in livestock could replicate these benefits, potentially transforming approaches to neonatal care in pig farming.
In conclusion, this study demonstrated the ability of A. muciniphila to grow on Ningxiang PMOs thanks to its expression of a set of PMOs-degrading enzymes using an in vitro fermentation model. The fermentation of Ningxiang pig colostrum by A. muciniphila degraded acidic trioses, neutral trioses and tetraoses (the mainly PMOs). Our study offers the possibility of controlling colostrum composition or adding prebiotics to piglet diets to promote beneficial bacteria, such as A. muciniphila. Such strategies could lead to enhanced survival rates, improved immune functions, and greater resistance to gastrointestinal diseases in neonatal livestock, underscoring the potential of targeted microbial interventions in agricultural settings.
Data availability statement
The original contributions presented in the study are included in the article/supplementary material, further inquiries can be directed to the corresponding authors.
Author contributions
LLZ: Formal analysis, Visualization, Writing – original draft, Writing – review & editing. ZCW: Methodology, Software, Writing – review & editing. MK: Supervision, Validation, Writing – review & editing. JW: Conceptualization, Funding acquisition, Writing – review & editing. BET: Conceptualization, Funding acquisition, Writing – review & editing.
Funding
The author(s) declare that financial support was received for the research, authorship, and/or publication of this article. This study was funded by the National Key R&D Program (2022YFD1300403), Excellent Youth Foundation of Hunan Province (2022JJ20027), Science and Technology Major Project of Yunnan Province (202202AE090032), and Earmarked Fund for China Agriculture Research System (CARS-35).
Acknowledgments
The authors would like to thank HealthSyn Biotechnology (Zhuhai Hengqin) Co., Ltd. for helping our experiments.
Conflict of interest
The authors declare that the research was conducted in the absence of any commercial or financial relationships that could be construed as a potential conflict of interest.
Publisher’s note
All claims expressed in this article are solely those of the authors and do not necessarily represent those of their affiliated organizations, or those of the publisher, the editors and the reviewers. Any product that may be evaluated in this article, or claim that may be made by its manufacturer, is not guaranteed or endorsed by the publisher.
Footnotes
References
Bæk, O., Sangild, P. T., Thymann, T., and Nguyen, D. N. (2019). Growth restriction and systemic immune development in preterm piglets. Front. Immunol. 10, 1–13. doi: 10.3389/fimmu.2019.02402
Barile, D., Marotta, M., Chu, C., Mehra, R., Grimm, R., Lebrilla, C. B., et al. (2010). Neutral and acidic oligosaccharides in Holstein-Friesian colostrum during the first 3 days of lactation measured by high performance liquid chromatography on a microfluidic chip and time-of-flight mass spectrometry. J. Dairy Sci. 93, 3940–3949. doi: 10.3168/jds.2010-3156
Bridgman, S. L., Azad, M. B., Field, C. J., Haqq, A. M., Becker, A. B., Mandhane, P. J., et al. (2017). Fecal short-chain fatty acid variations by breastfeeding status in infants at 4 months: differences in relative versus absolute concentrations. Front. Nutr. 4:11. doi: 10.3389/fnut.2017.00011
Chen, Y., Wen, Y., Zhu, Y., Chen, Z., Mu, W., and Zhao, C. (2023). Synthesis of bioactive oligosaccharides and their potential health benefits. Crit. Rev. Food Sci. Nutr., 21, 1–13. doi: 10.1080/10408398.2023.2222805
Collado, M. C., Derrien, M., Isolauri, E., de Vos, W. M., and Salminen, S. (2007). Intestinal integrity and Akkermansia muciniphila, a mucin-degrading member of the intestinal microbiota present in infants, adults, and the elderly. Appl. Environ. Microbiol. 73, 7767–7770. doi: 10.1128/AEM.01477-07
Dao, M. C., Everard, A., Aron-Wisnewsky, J., Sokolovska, N., Prifti, E., Verger, E., et al. (2015). Akkermansia muciniphila and improved metabolic health during a dietary intervention in obesity: rela.Pdf. Gut 65, 426–436. doi: 10.1136/gutjnl-2014-308778
Depommier, C., Everard, A., Druart, C., Plovier, H., Van Hul, M., Vieira-Silva, S., et al. (2019). Supplementation with Akkermansia muciniphila in overweight and obese human volunteers: a proof-of-concept exploratory study. Nat. Med. 25, 1096–1103. doi: 10.1038/s41591-019-0495-2
Derosa, L., Routy, B., Thomas, A. M., Iebba, V., Zalcman, G., Friard, S., et al. (2022). Intestinal Akkermansia muciniphila predicts clinical response to PD-1 blockade in patients with advanced non-small-cell lung cancer. Nat. Med. 28, 315–324. doi: 10.1038/s41591-021-01655-5
Derrien, M., Collado, M. C., Ben-Amor, K., Salminen, S., and De Vos, W. M. (2008). The mucin degrader Akkermansia muciniphila is an abundant resident of the human intestinal tract. Appl. Environ. Microbiol. 74, 1646–1648. doi: 10.1128/AEM.01226-07
Farmer, C., and Edwards, S. A. (2022). Review: improving the performance of neonatal piglets. Animal 16:100350. doi: 10.1016/j.animal.2021.100350
Gasaly, N., de Vos, P., and Hermoso, M. A. (2021). Impact of bacterial metabolites on gut barrier function and host immunity: a focus on bacterial metabolism and its relevance for intestinal inflammation. Front. Immunol. 12, 1–16. doi: 10.3389/fimmu.2021.658354
Heiss, B. E., Ehrlich, A. M., Maldonado-Gomez, M. X., Taft, D. H., Larke, J. A., Goodson, M. L., et al. (2021). Bifidobacterium catabolism of human milk oligosaccharides overrides endogenous competitive exclusion driving colonization and protection. Gut Microbes 13:1986666. doi: 10.1080/19490976.2021.1986666
Henrick, B. M., Rodriguez, L., Lakshmikanth, T., Pou, C., Henckel, E., Arzoomand, A., et al. (2021). Bifidobacteria-mediated immune system imprinting early in life. Cell 184, 3884–3898.e11. doi: 10.1016/j.cell.2021.05.030
Heuß, E. M., Pröll-Cornelissen, M. J., Neuhoff, C., Tholen, E., and Groβe-Brinkhaus, C. (2019). Invited review: piglet survival: benefits of the immunocompetence. Animal 13, 2114–2124. doi: 10.1017/S1751731119000430
Ji, F. J., Wang, L. X., Yang, H. S., Hu, A., and Yin, Y. L. (2019). Review: the roles and functions of glutamine on intestinal health and performance of weaning pigs. Animal 13, 2727–2735. doi: 10.1017/S1751731119001800
Katayama, T. (2016). Host-derived glycans serve as selected nutrients for the gut microbe: human milk oligosaccharides and bifidobacteria. Biosci. Biotechnol. Biochem. 80, 621–632. doi: 10.1080/09168451.2015.1132153
Kim, S., Shin, Y. C., Kim, T. Y., Kim, Y., Lee, Y. S., Lee, S. H., et al. (2021). Mucin degrader Akkermansia muciniphila accelerates intestinal stem cell-mediated epithelial development. Gut Microbes 13, 1–20. doi: 10.1080/19490976.2021.1892441
Kogut, M. H., and Zhang, G. (2022). Gut microbiota, immunity, and health in production animals. Springer.
Lan, C., Li, H., Shen, Y., Liu, Y., Wu, A., He, J., et al. (2024). Next-generation probiotic candidates targeting intestinal health in weaned piglets: both live and heat-killed Akkermansia muciniphila prevent pathological changes induced by enterotoxigenic Escherichia coli in the gut. Anim. Nutr 17, 110–122. doi: 10.1016/j.aninu.2024.01.007
Lawson, M. A. E., O’Neill, I. J., Kujawska, M., Gowrinadh Javvadi, S., Wijeyesekera, A., Flegg, Z., et al. (2020). Breast milk-derived human milk oligosaccharides promote Bifidobacterium interactions within a single ecosystem. ISME J. 14, 635–648. doi: 10.1038/s41396-019-0553-2
Li, H., Lane, J. A., Chen, J., Lu, Z., Wang, H., Dhital, S., et al. (2022). In vitro fermentation of human milk oligosaccharides by individual Bifidobacterium longum-dominant infant fecal inocula. Carbohydr. Polym. 287:119322. doi: 10.1016/j.carbpol.2022.119322
Li, Y., Liu, Y., Wu, J., Chen, Q., Zhou, Q., Wu, F., et al. (2021). Comparative effects of enzymatic soybean, fish meal and milk powder in diets on growth performance, immunological parameters, SCFAs production and gut microbiome of weaned piglets. J. Anim. Sci. Biotechnol. 12, 106–111. doi: 10.1186/s40104-021-00625-8
Liu, X. F., Shao, J. H., Liao, Y. T., Wang, L. N., Jia, Y., Dong, P. J., et al. (2023). Regulation of short-chain fatty acids in the immune system. Front. Immunol. 14, 1–14. doi: 10.3389/fimmu.2023.1186892
Ma, N., Sun, Y., Chen, J., Qi, Z., Liu, C., and Ma, X. (2022). Micro-coevolution of genetics rather than diet with Enterotype in pigs. Front. Nutr. 9, 1–11. doi: 10.3389/fnut.2022.846974
Marcobal, A., Barboza, M., Sonnenburg, E. D., Pudlo, N., Martens, E. C., Desai, P., et al. (2011). Bacteroides in the infant gut consume milk oligosaccharides via mucus-utilization pathways. Cell Host Microbe 10, 507–514. doi: 10.1016/j.chom.2011.10.007
Ogawa, S., Tsukahara, T., Imaoka, T., Nakanishi, N., Ushida, K., and Inoue, R. (2016). The effect of colostrum ingestion during the first 24 hours of life on early postnatal development of piglet immune systems. Anim. Sci. J. 87, 1511–1515. doi: 10.1111/asj.12573
Qin, W., Yu, Z., Li, Z., Liu, H., Li, W., Zhao, J., et al. (2023). Dietary Berberine and Ellagic acid supplementation improve growth performance and intestinal damage by regulating the structural function of gut microbiota and SCFAs in weaned piglets. Microorganisms 11, 1–19. doi: 10.3390/microorganisms11051254
Wang, L.-n., Chen, X.-l., Li, X.-G., Shu, G., Yan, H.-c., and Wang, X.-q. (2014). Evaluation of adrenocorticotropin regulated glucocorticoid synthesis pathway in adrenal of different breeds of pigs. Livest. Sci. 169, 185–191. doi: 10.1016/j.livsci.2014.08.007
Xu, K., Bai, M., Bin, P., Duan, Y., Wu, X., Liu, H., et al. (2019). Negative effects on newborn piglets caused by excess dietary tryptophan in the morning in sows. J. Sci. Food Agric. 99, 3005–3016. doi: 10.1002/jsfa.9514
Yao, Y., Cai, X., Fei, W., Ye, Y., Zhao, M., and Zheng, C. (2022). The role of short-chain fatty acids in immunity, inflammation and metabolism. Crit. Rev. Food Sci. Nutr. 62, 1–12. doi: 10.1080/10408398.2020.1854675
Yu, Z. T., Chen, C., and Newburg, D. S. (2013). Utilization of major fucosylated and sialylated human milk oligosaccharides by isolated human gut microbes. Glycobiology 23, 1281–1292. doi: 10.1093/glycob/cwt065
Zhang, T., Li, Q., Cheng, L., Buch, H., and Zhang, F. (2019). Akkermansia muciniphila is a promising probiotic. Microb. Biotechnol. 12, 1109–1125. doi: 10.1111/1751-7915.13410
Zhang, Y., Xie, Q., You, L., Cheung, P. C. K., and Zhao, Z. (2021). Behavior of non-digestible polysaccharides in gastrointestinal tract: a mechanistic review of its anti-obesity effect 2, 59–72. doi: 10.2991/efood.k.210310.001
Zheng, M., Han, R., Yuan, Y., Xing, Y., Zhang, W., Sun, Z., et al. (2023). The role of Akkermansia muciniphila in inflammatory bowel disease: current knowledge and perspectives. Front. Immunol. 13, 1–19. doi: 10.3389/fimmu.2022.1089600
Zhu, W., Zhou, S., Liu, J., McLean, R. J. C., and Chu, W. (2020). Prebiotic, immuno-stimulating and gut microbiota-modulating effects of Lycium barbarum polysaccharide. Biomed. Pharmacother. 121:109591. doi: 10.1016/j.biopha.2019.109591
Keywords: Akkermansia muciniphila , Ningxiang pig, milk oligosaccharides, piglet survival, short-chain fatty acids
Citation: Zhang L, Wu Z, Kang M, Wang J and Tan B (2024) Utilization of Ningxiang pig milk oligosaccharides by Akkermansia muciniphila in vitro fermentation: enhancing neonatal piglet survival. Front. Microbiol. 15:1430276. doi: 10.3389/fmicb.2024.1430276
Edited by:
Kang Xu, Chinese Academy of Sciences (CAS), ChinaReviewed by:
Yu Bai, Tianjin University of Science and Technology, ChinaHui Han, Chinese Academy of Sciences (CAS), China
Shuting Cao, Guangdong Academy of Agricultural Sciences (GDAAS), China
Copyright © 2024 Zhang, Wu, Kang, Wang and Tan. This is an open-access article distributed under the terms of the Creative Commons Attribution License (CC BY). The use, distribution or reproduction in other forums is permitted, provided the original author(s) and the copyright owner(s) are credited and that the original publication in this journal is cited, in accordance with accepted academic practice. No use, distribution or reproduction is permitted which does not comply with these terms.
*Correspondence: Bie Tan, YmlldGFuQGh1bmF1LmVkdS5jbg==; Jing Wang, amluZ3dhbmcwMjNAaHVuYXUuZWR1LmNu