- 1College of Ocean Science and Engineering, Shanghai Maritime University, Shanghai, China
- 2Engineering Technology Research Center for Corrosion Control and Protection of Materials in Extreme Marine Environment, Guangzhou Maritime University, Guangzhou, China
The adhesion of microorganisms and the subsequent formation of mineralized layers in biofilms are of great significance in inhibiting the corrosion of metal materials. In this work, we found that the adhesion and subsequent mineralization of Bacillus subtilis on the surface of low-alloy steel are influenced by the molybdenum in the material. The addition of molybdenum will lead to increased adhesion of B. subtilis on the material surface, and the subsequent biomineralization ability has also been improved. Through transcriptome and physiological and biochemical tests, we found that molybdenum can affect the chemotaxis, mobility and carbonic anhydrase secretion related genes of B. subtilis, and then affect the formation and mineralization of the biofilm of B. subtilis.
1 Introduction
Bacillus subtilis, a gram-positive bacterium species of Bacillus, has a single cell size of 0.7–0.8 × 2–3 μm, no capsule, and peritrichous flagella and is motile. B. subtilis can form endogenous spores under stress and resist various adverse environments; therefore, it has a wide survival range and has been observed in soil, freshwater, and seawater (Mohsin et al., 2021). As a gram-positive bacterium, B. subtilis has the functionality of biological mineralization (Yan et al., 2021; Wightman and Fein, 2005; Mudgil et al., 2018; Lin et al., 2015; Guo et al., 2019; Chalia et al., 2017), with its abundant extracellular products providing many nucleation sites for mineralization (Yin et al., 2020; Fein et al., 2002; Keren-Paz et al., 2022). More importantly, B. subtilis can secrete carbonic anhydrase (CA) that is a ubiquitous enzyme. It can efficiently catalyze the reversible reactions (Han et al., 2019; Mukherjee and Venkata Mohan, 2021; Barabesi et al., 2007) of CO2 and H2O to generate HCO3−and H+, and plays a crucial role in the process of biomineralization. As a result, this property of B. subtilis has been widely used in the preparation of biomineralized CaCO3 crystals (Song et al., 2019; Perito et al., 2018; Marvasi et al., 2010), as adsorptive materials (Arias et al., 2018), catalytic materials, ceramic materials, protective layers for sensitive materials (such as enzymes, proteins), and drug release materials, with broad application prospects in the fields of chemical, environmental protection, biological, medical, and building material industries (Kang et al., 2021). It can also be used in engineering, such as concrete micro-crack repair (Mondal and Ghosh, 2021; Huynh et al., 2019; Feng et al., 2021; Huynh et al., 2022; Mahmood et al., 2022), building restoration and protection (Perito et al., 2014), and preparation of calcium carbonate micro- and nano-particles (Sazanova et al., 2020). Thus, its role in the protection and restoration of stone surfaces (Perito et al., 2014; Sazanova et al., 2020; Shim et al., 2011), foundation stabilization, earthquake prevention, and the capture of radionuclides and heavy metal ions (Johnson and Fein, 2019) should not be disregarded (Arias et al., 2020).
The calcium carbonate layer formed by microbial mineralization on metal surfaces can protect materials and prevent metal material corrosion. As a green, environmentally friendly, and self-healing anti-corrosion method, this approach has attracted the attention of scholars. A previous study has reported that the presence and concentration of Mo in metal materials affects the adhesion of microorganisms, thus affecting the subsequent mineralization or corrosion mediated by the microorganisms (Guo et al., 2022; Guo et al., 2019). However, although the B. subtilis bacterium has an excellent mineralization ability, the effect of metal alloy elements on the adhesion and mineralization performance of B. subtilis has not been reported. Moreover, in the CA-induced mineralization process, the CA activity will be affected by a number of factors, such as temperature, pH, and Ca2+ concentration. Also, whether metals affect the expression of CA remains unclear. Therefore, a study on the effects of elements present in metal materials on B. subtilis and its CA activity is of great significance to further clarify the mechanism of B. subtilis mineralization, providing an important scientific basis for the preparation of biomineralization coatings on metal surfaces. In this study, low-alloy steels containing Mo with different gradient concentrations were designed to study the influence of changes in Mo content on the adhesion and mineralization behavior of B. subtilis. Finally, the influence mechanism of Mo on the adhesion and mineralization behavior of B. subtilis was investigated through transcriptome analysis.
2 Materials and methods
2.1 Steel sample
The steel sample used in the test was low-alloy steel, and its composition is shown in Supplementary Table S1. The steel samples were processed into 10 × 10 mm square samples by wire cutting, and then we used 50–1,200 grit sandpaper to polish the samples in sequence. The steel samples were soaked in anhydrous ethanol and acetone, and then washed under ultrasonication for 15 min and dried with nitrogen. The samples were placed on a clean workbench for 1 h of ultraviolet sterilization before use.
2.2 Strain and culture
The B. subtilis used in this study was supplied by Marine Culture Collection of China (No. 1A14806), it could mineralize on the surfaces of metal materials, thereby inhibiting metal material corrosion. The strain was stored in a −80°C freezer. The medium was 2216E medium (Qingdao Hopebio), and 37.5 g of the medium was dissolved in deionized water to prepare the experimental medium. 2216E medium: 19.45 g/L NaCl, 5.98 g/L MgCl2, 5.0 g/L Peptone, 3.24 g/LNa2SO4, 1.8 g/L CaCl2, 1.0 g/L yeast extract powder, 0.55 g/L KCl, 0.16 g/L Na2CO3, 0.1 g/L FeC6H5O7, 0.08 g/L KBr, 0.034 g/L SrCl2, 0.022 g/L H3BO3, 0.008 g/L NaH2PO4, 0.004 g/L Na2SiO3, 0.0024 g/L NaF, 0.0016 g/L NH4NO3. After the culture medium was sterilized at 121°C for 20 min, we cultured 100 μL of the bacterial solution overnight and inoculated the culture medium (200 mL). Then, the steel sample was placed in the culture medium and cultured in a light incubator at a temperature of 30°C and a shaker speed of 120 r/min. The growth of the bacterium was monitored by plate counting, using a series of dilutions (in a total volume of 100 μL). The concentration of viable cells was calculated based on the number of colonies grown in each well on the plate. In addition, pH and dissolved oxygen levels were measured once a day in the cultures. A pH meter (Sartorius, Germany) and a dissolved oxygen meter (Inesa JPBJ-611Y, China) were used to obtain the respective measurements. Throughout the experimental period, the changes in calcium ion concentration were measured in filtered samples (0.22 μm) using an inductively coupled plasma mass spectrometer (PerkinElmer NexION 2,200, United States).
2.3 Corrosion morphology characterization
After 14 days of cultivation, the steel samples were removed, washed with PBS, and soaked in 2.5% glutaraldehyde solution for 2 h. Afterward, the steel samples were dried with alcohol (stepwise in: 30, 50, 70, 80, 90, and 100% v/v, 15 min each) and then dried under pure nitrogen. The corrosion morphology of the steel samples was observed by scanning electron microscope (SEM, ZEISS Gemini 300, Germany), and X-ray diffractometer (XRD, Nalytical X’Pert PRO, Netherlands) was used to identify the corrosion product components on the sample surfaces (Cu-Ka radiation source at 40 kV and 10 mA with 2θ = 20–90°). The adhesion of the bacterial biofilm on the steel surface was investigated using acridine orange as staining agent, with the bacterial cells showing green fluorescence under an inverted fluorescence microscope (after 15 min staining, at 502 nm maximum excitation wavelength). Then, the specimens were washed successively with concentrated hydrochloric acid, saturated sodium bicarbonate, and deionized water to clean the product layer on the steel surface. After the samples were air-dried, pitting on the material surface was observed by an optical profiler (Bruker ContourGT, Germany).
2.4 Electrochemistry test
An electrochemistry test was conducted using a three-electrode system, where the sample served as the working electrode, the saturated calomel electrode was the reference electrode, the platinum electrode was the opposite electrode, and the test solution was 2216E medium. Before impedance testing was conducted, the sample was soaked for 60 min, and then the OCP test was performed. After a stable open-circuit potential was obtained, the EIS test was performed by an electrochemical workstation (Gamry Interface 100E, United States), using a test frequency range of 105–10−2 Hz, and the interference amplitude was ±10 mV. ZSimpWin analysis software was used.
2.5 RNA-seq test
A biofilm developed on the low-alloy steel surface, and biofilm samples were collected after 2 days of inoculation. After removal from the media, the coupons were quickly and gently washed in 0.85% NaCl buffer at 0°C to remove the contaminated suspended cells and ultrasonically treated in 0.85% NaCl buffer at 0°C for 2 min to collect the biofilm cells from the metal surface. The buffer containing biofilm cells was centrifuged at 10000 rpm and −2°C for 3 min, then the supernatant was discarded and the precipitated biofilm cells were resuspended in 6 mL of 0.85% NaCl buffer at 0°C, transferred to a cold bead stirrer tube, and centrifuged at 10000 rpm and room temperature for 15 s. Then, the cell particles were immediately frozen in a dry ice ethanol bath, and subsequently the samples were sent to Shanghai Majorbio Bio-pharm Technology Co., Ltd., for transcriptome testing.
3 Results
3.1 Bacterial adhesion to the surface of low-alloy steel
In order to explore the effect of Mo on the adhesion of B. subtilis on the low-alloy steel surface and the formation of microbial film, the surfaces of the low-alloy steel samples with different Mo element contents were characterized by an inverted fluorescence microscope, after 3 days of immersion (Figure 1). The number of bacteria on the low-alloy steel surface without Mo (Figure 1A) elements was significantly lower than that of low-alloy steel containing 0.4 wt% Mo elements (Figure 1B). The number of bacteria attached to the low-alloy steel containing 1.0 wt% Mo elements (Figure 1C) was decisively the highest. Therefore, it can be seen that the adhesion number of bacteria on the low-allow steel surface increases with the increase of Mo content. According to our previous study (Guo et al., 2022; Guo et al., 2019), this was possibly due to the presence of Mo elements, which affected the chemotaxis of the bacteria and thus increased their quantity on the steel surface and affected their mineralization.
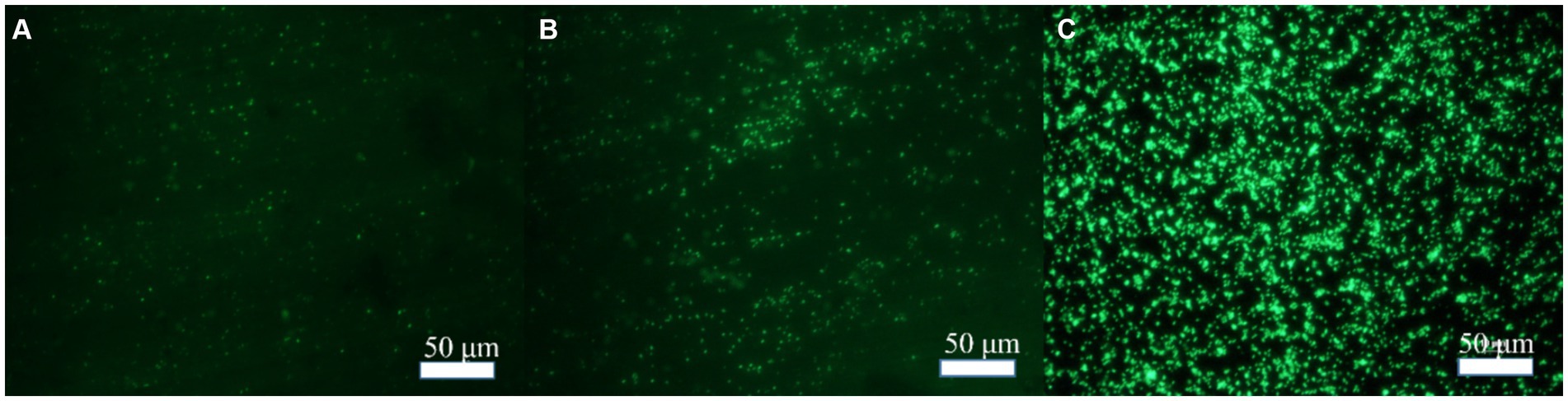
Figure 1. Bacterial adhesion on blank steel and steel samples containing Mo. (A) Blank steel, (B) 0.4 wt% Mo steel, and (C) 1.0 wt% Mo steel.
3.2 Surface morphology of low-alloy steel
Figure 2 indicates that the occurrence of triangular calcite structures raised with increasing Mo content, based on a comparison of the surface morphology of materials containing increasing Mo (Figures 2A,E,I), with the greatest number of mineralized products being observed on the low-alloy steel surface with 1.0 wt% Mo elements (Figures 2I,J). According to the mapping diagram, with blue representing the content of Ca elements on the surface, we observed that the content of Ca elements on the low-alloy steel surface rose with increasing Mo content in the low-alloy steel, which further supported the observation of elevated mineralized products on the steel surface. Green represented the content of Fe elements on the surface, and the results indicate that Fe content decreased with increasing Mo content, showing that both the amount of corrosion products and the area of uncovered steel surface decreased, while the amounts of mineralized products gradually increased.
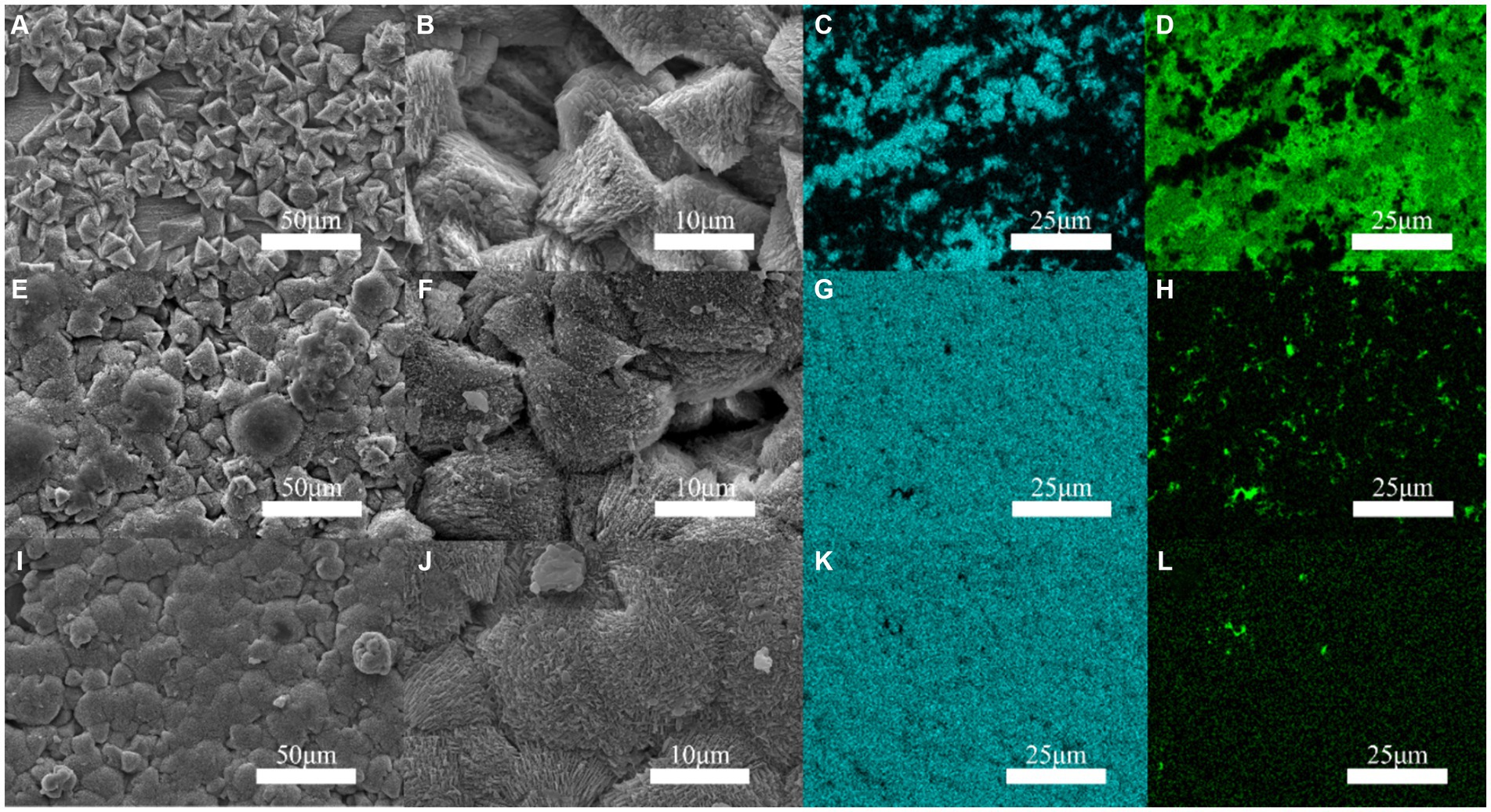
Figure 2. Surface mineralization of the blank steel and Mo-containing steel. (A–D) Blank steel, (E–H) 0.4 wt% Mo steel, (I–L) 1.0 wt% Mo steel.
It is apparent in Figure 3 that the thickness of the mineralized product layer on the low-alloy steel surface without Mo elements (Figure 3A) was thinner than that of low-alloy steel with Mo elements (Figures 3B,C), which also proved that the thickness of the mineralized product layer on the low-alloy steel surface increases with the increase of Mo element content.
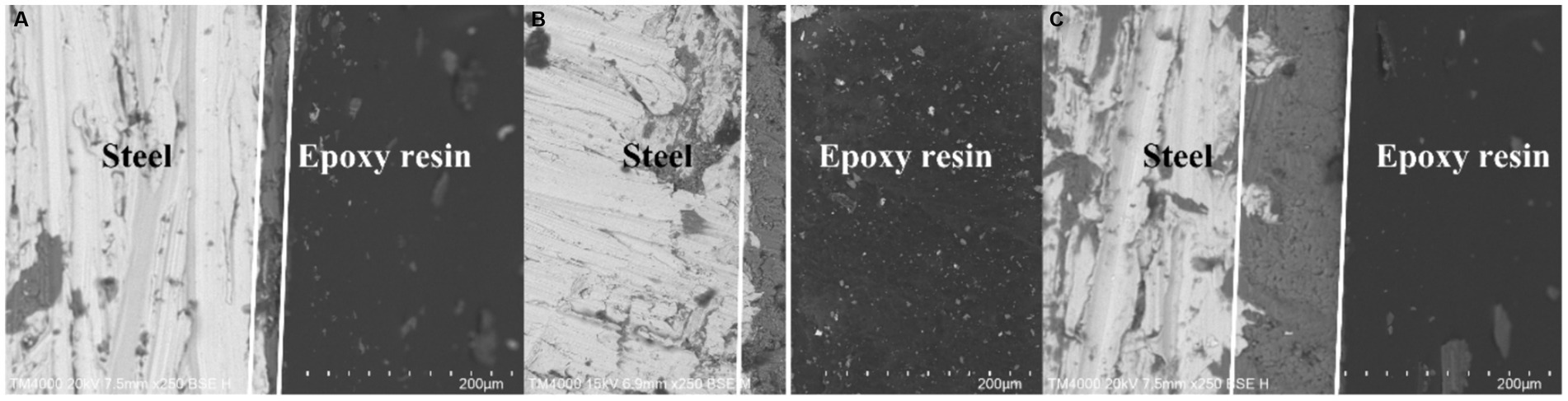
Figure 3. Surface mineralized thicknesses of the blank steel and steel containing Mo. (A) Blank steel (19 μm), (B) 0.4 wt% Mo steel (42 μm), (C) 1.0 wt% Mo steel (106 μm).
Figure 4 shows the X-ray diffraction patterns results. As shown in the figure, compared with the low-alloy steel surface without Mo, the surface of the low-alloy steel with a higher Mo content was greater (Mg.064Ca.936) (CO3), with a thicker mineralized product layer, as shown also in Figure 3.
3.3 Surface pitting
As shown in the Figure 5, more extensive pitting was observed on the low-alloy steel surface without Mo elements (Figure 5A), compared with the low-alloy steel containing Mo, and with an increase in Mo content, pitting essentially disappeared (1.0 wt% Mo). Supplementary Figure S1 shows the scanning electron microscope images and pitting corrosion after 14 days of immersion in a sterile solution. We observed that the addition of Mo had no effect on corrosion under sterile conditions. In previous work (Guo et al., 2022; Guo et al., 2019), we compared pitting, weight loss, and electrochemical data in sterile environments, the addition of molybdenum did not alter the corrosion resistance of low-alloy steel.
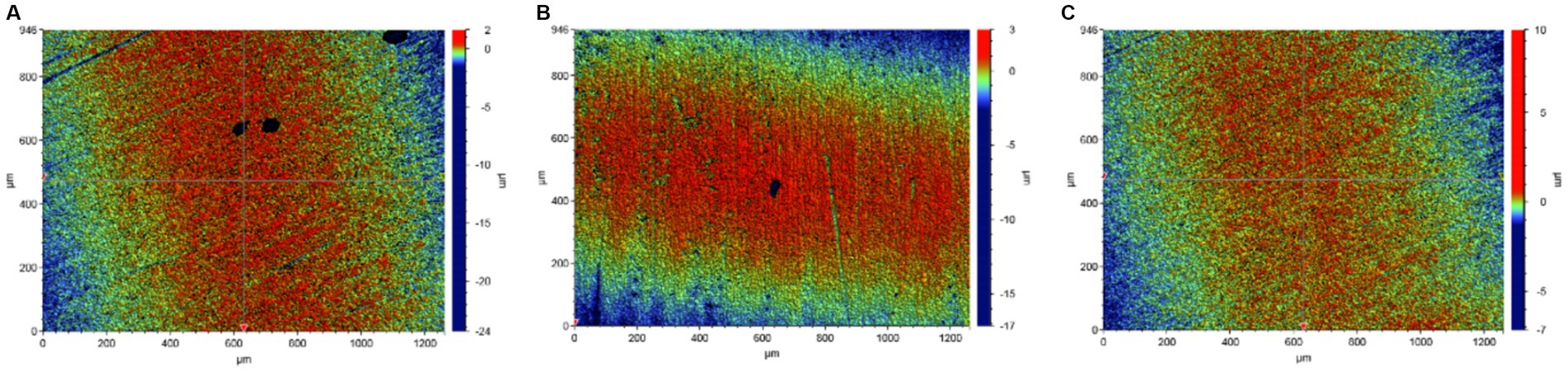
Figure 5. Corrosion of the blank steel and steel containing Mo in the presence of B. subtilis. (A) Blank steel, (B) 0.4 wt% Mo steel, (C) 1.0 wt% Mo steel.
3.4 Bacillus subtilis growth kinetics
Figure 6 shows kinetics of the bacterial growth. As indicated by the solution pH change (Figure 6A), pH value decreased with increasing Mo content in the low-alloy steel, which was ascribed to a series of biochemical reactions. The total number of B. subtilis colonies (Figure 6B) was not significantly affected by the Mo content. However, the change in Ca2+ concentration in the solution (Figure 6C) decreased with increasing Mo content, which supported the hypothesis that the increase in Mo content increased the formation of mineralized products on the sample surface, as this process consumes Ca2+ in the solution.
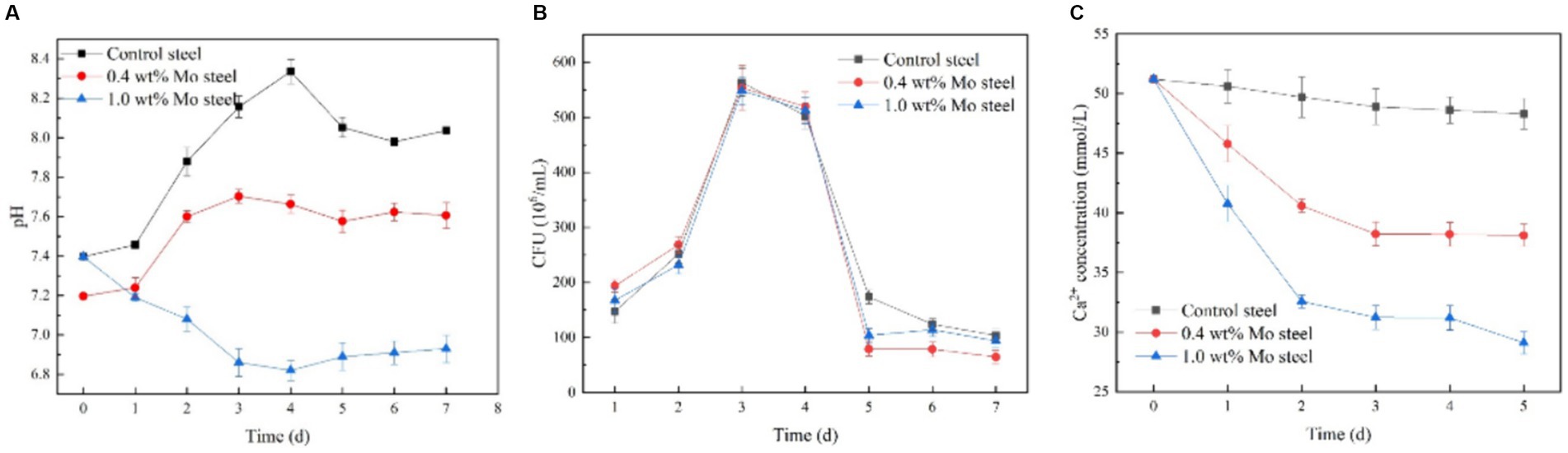
Figure 6. Growth and physiological and biochemical analysis of B. subtilis. (A) pH, (B) growth curve, (C) Ca2+ content in the solution.
3.5 Electrochemical impedance
Figure 7 shows the electrochemical impedance test results. As shown in the figure, the electrochemical impedance curves formed incomplete semicircles, and the radius changed with different Mo element contents. The radius of the impedance curve of the low-alloy steel without Mo elements (Figure 7A) during the immersion process was significantly smaller than that of the low-alloy steel with 0.4 wt% Mo element (Figure 7B) and low-allow steel containing 1.0 wt% Mo element (Figure 7C). Similarly, the radius of the impedance curve rose with increasing Mo content, correlating with the electrochemical impedance value. Electrochemical impedance parameters fitted from the measured impedance plots in Figure 7 are listed in Supplementary Table S2. Thus, with rising Mo content, the impedance increases, as well as the thickness of the surface mineralized product layer, which corresponded to the results obtained from the above characteristic maps. In general, the thicker and denser mineralized layers are the more electron transfer on the material surface is hindered and conductivity lowered (Guo et al., 2019).
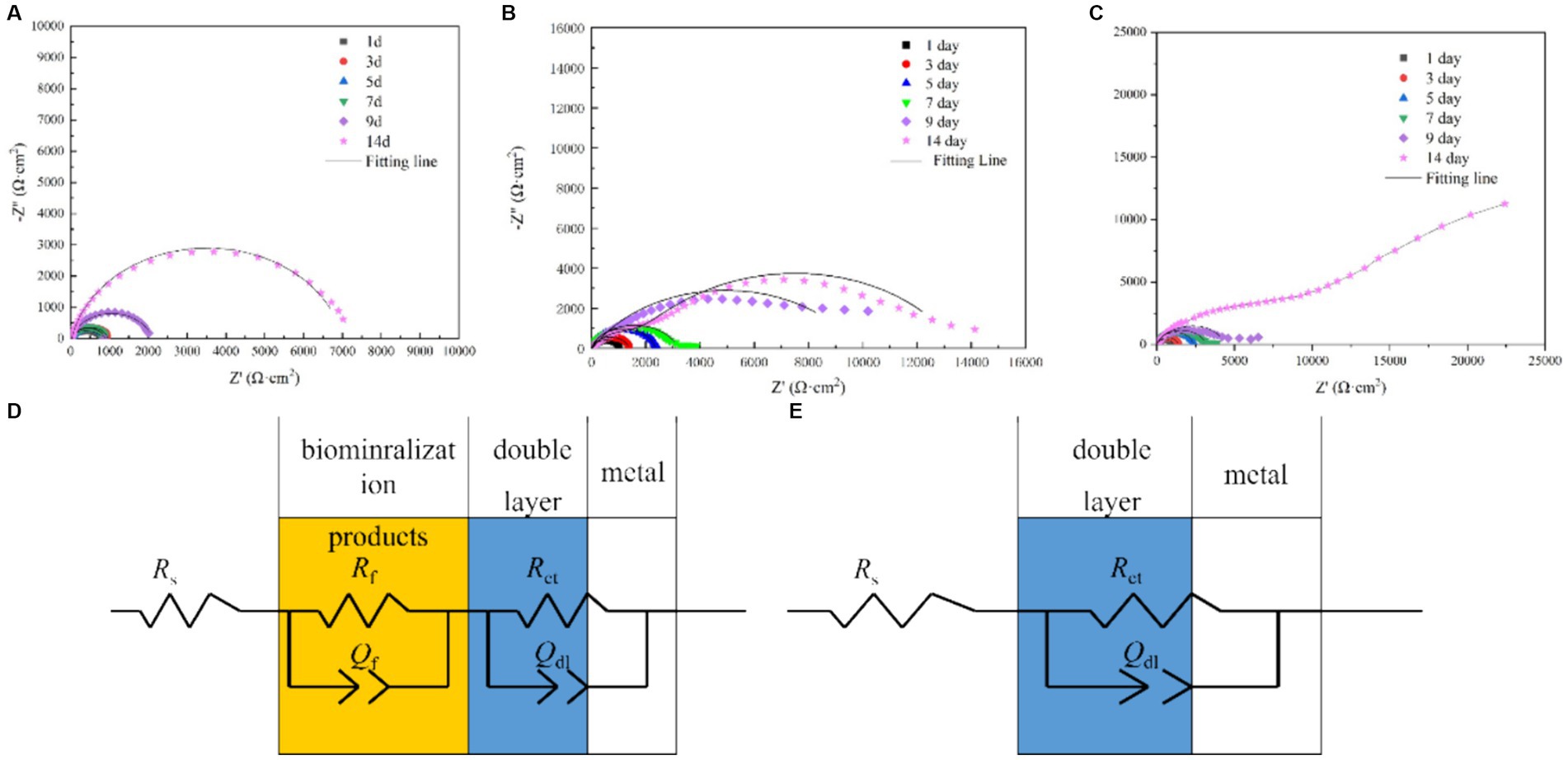
Figure 7. EIS spectra of the blank steel and steel containing Mo in the presence of B. subtilis. (A) Blank steel, (B) 0.4 wt% Mo steel, (C) 1.0 wt% Mo steel, and (D,E) corresponding fitting circuits.
3.6 Transcriptome analysis results
Figure 8 summarizes the observed differences in gene expression. Based on findings of previous studies (Guo et al., 2022), we mainly focused on bacterial chemotaxis and flagellar pathways. Figure 8A shows a Venn diagram, with nine common differential genes in the experimental group. As shown in Figure 8B, fliL, yvyG, flgK, hag, fliK, flgL, fliJ and ca genes were upregulated in cells collected from the steel surface with elevated Mo content.
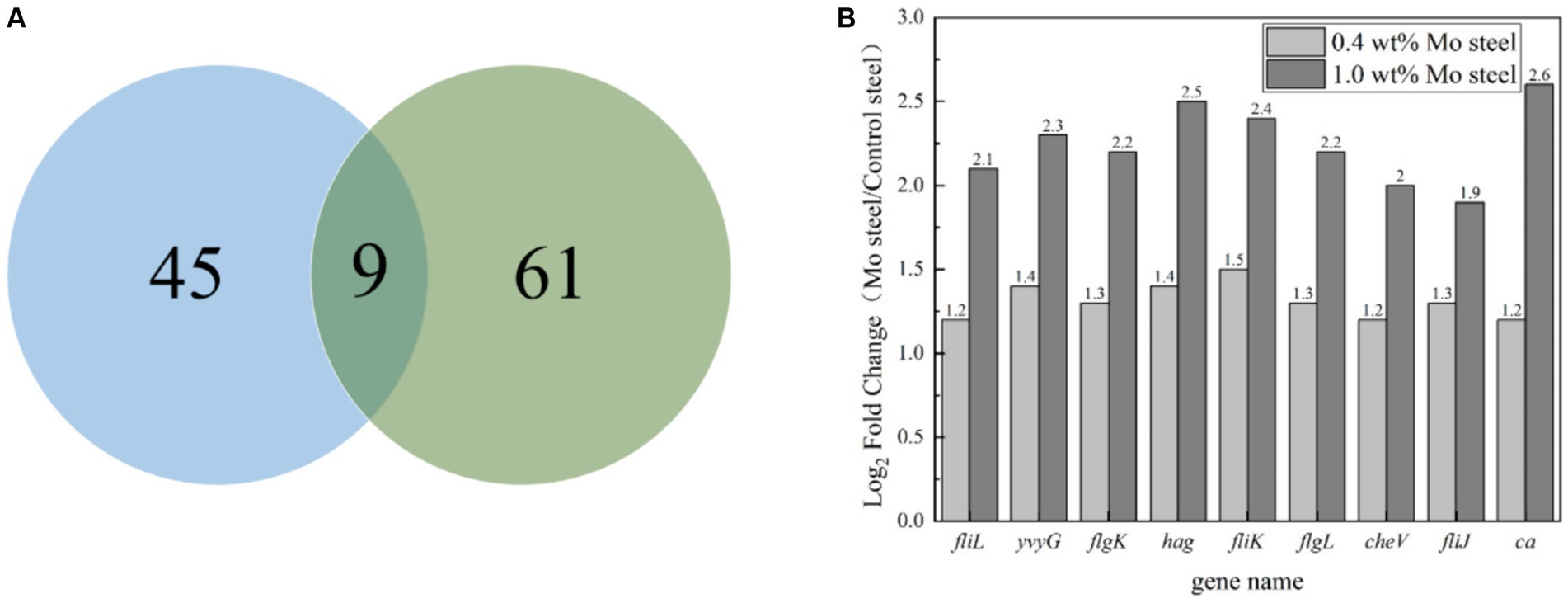
Figure 8. Gene regulation analysis of blank steel and steel containing Mo on B. subtilis. (A) Venn diagram, (B) differential gene analysis diagram.
4 Discussion
The presented study investigated regulatory mechanisms and factors influencing biomineralization on metal material surfaces mediated by B. subtilis, showing an important direction for research supporting future applications of biomineralization in corrosion prevention. In our previous study, 1.0 wt% Mo content regulated the attachment of Pseudoalteromonas lipolytica (Guo et al., 2019). In this work, fliL, yvyG, flgK, hag, fliK, flgL, fliJ and ca genes were upregulated by Mo in low-alloy steel, with fliL, yvyG, flgK, hag, fliK, flgL, and fliJ being genes involved in the assembly of bacterial flagella, and cheV affecting the chemotaxis of bacteria. In general, adhesion of bacteria is greatly affected by the assembly of flagella and chemotaxis. Therefore, this study indicated that Mo affected flagella assembly and bacterial chemotaxis. Chemotaxis refers to the process of cells responding to a chemical gradient by the movement towards a more favorable environment (Chen et al., 2024), with methyl-accepting chemotaxis protein (Mcp) being one of key signalling molecules (Song et al., 2024). Mcps interact with other chemotactic molecules, which leads to the enhancement of phosphorylation of CheV and the charge of the Mot protein subunit, thus controlling the direction of the movement by increasing the rotation rate of the flagella. In this study, we reported that bacteria adhered more to the steel surface containing Mo, compared to Mo-free steel, by the process of flagella-assisted chemotaxis, which is consistent with our previous reports (Guo et al., 2022; Guo et al., 2019). It is noteworthy that in the transcriptome test performed in this study, the ca gene was also upregulated in the presence of Mo. Carbonic anhydrase is a common enzyme in bacteria, which can catalyze a series of physiological and biochemical reactions (Baidya et al., 2024). Its role involves combining carbon dioxide and water to form bicarbonate and hydrogen ions (Zheng et al., 2023):
which will reduce the pH value of the solution, which is consistent with our pH test results. At the same time, bicarbonate can form carbonate, and then combine with the Ca ions in the solution (Zheng et al., 2023):
which will form a denser mineralized layer. This was evidenced by the continuous decreases in pH and Ca content in solution. The main conclusion based on the transcriptomic analysis performed is that both chemotaxis and CA strengthened the mineralization ability of B. subtilis on the surface of steel with Mo content.
5 Conclusion
In this study, we report that Mo in low-alloy steel had a regulatory effect on the gram-positive bacterium B. subtilis. The expression of the chemotactic gene and ca gene in B. subtilis was enhanced in the presence of Mo, which improved the adhesion ability of the bacterium and enhanced the mineralization of calcium carbonate. Furthermore, mineralized products have shown to be stable and environmentally friendly; thus, this process controlled by ca gene has become one of the most promising technologies for CO2 capture. Future research should focus on the diversity of biomineralization patterns of different microbial species under specific environmental conditions, and investigate how these patterns are influenced by gene regulation and external environment.
Data availability statement
The datasets presented in this study can be found in online repositories. The names of the repository/repositories and accession number(s) can be found in the article/Supplementary material.
Author contributions
ZG: Formal analysis, Funding acquisition, Writing – review & editing. QF: Formal analysis, Funding acquisition, Writing – original draft. NG: Writing – review & editing, Formal analysis, Funding acquisition. YY: Writing – review & editing, Formal analysis, Funding acquisition. TL: Writing – review & editing, Formal analysis, Funding acquisition.
Funding
The author(s) declare that financial support was received for the research, authorship, and/or publication of this article. This work was financially supported by the National Natural Science Foundation of China (Nos. 51901127, 41976039, 42006039), Shanghai Engineering Technology Research Centre of Deep Offshore Material (19DZ2253100), the Shanghai Natural Science Fund (No. 19ZR1422100).
Conflict of interest
The authors declare that the research was conducted in the absence of any commercial or financial relationships that could be construed as a potential conflict of interest.
Publisher’s note
All claims expressed in this article are solely those of the authors and do not necessarily represent those of their affiliated organizations, or those of the publisher, the editors and the reviewers. Any product that may be evaluated in this article, or claim that may be made by its manufacturer, is not guaranteed or endorsed by the publisher.
Supplementary material
The Supplementary material for this article can be found online at: https://www.frontiersin.org/articles/10.3389/fmicb.2024.1428286/full#supplementary-material
References
Arias, D., Rivas, M., Guiñez, R., and Cisternas, L. A. (2018). Modeling the calcium and magnesium removal from seawater by immobilized biomass of ureolytic bacteria Bacillus subtilis through response surface methodology and artificial neural networks, desalination. Water Treat 118, 294–303. doi: 10.5004/dwt.2018.22665
Arias, D., Villca, G., Pánico, A., Cisternas, L. A., Jeldres, R. I., González-Benito, G., et al. (2020). Partial desalination of seawater for mining processes through a fluidized bed bioreactor filled with immobilized cells of Bacillus subtilis LN8B. Desalination 482:114388. doi: 10.1016/j.desal.2020.114388
Baidya, P., Zhang, M., Xiao, Y., Zhang, H., Yu, L., and Li, W. (2024). Genetically engineered whole-cell biocatalyst for efficient CO2 capture by cell surface display of carbonic anhydrase from Bacillus cereus GLRT202 on Escherichia coli. Biochem. Eng. J. 211:109446. doi: 10.1016/j.bej.2024.109446
Barabesi, C., Galizzi, A., Mastromei, G., Rossi, M., Tamburini, E., and Perito, B. (2007). Bacillus subtilis gene cluster involved in calcium carbonate biomineralization. J. Bacteriol. 189, 228–235. doi: 10.1128/JB.01450-06
Chalia, S., Baskar, S., Minakshi, P., Baskar, R., and Ranjan, K. (2017). Biomineralization abilities of Cupriavidus strain and Bacillus subtilis strains in vitro isolated from speleothems, Rani cave, Chhattisgarh, India. Geomicrobiol J. 34, 737–752. doi: 10.1080/01490451.2016.1257663
Chen, M., Trotter, V. V., Walian, P. J., Chen, Y., Lopez, R., Lui, L. M., et al. (2024). Molecular mechanisms and environmental adaptations of flagellar loss and biofilm growth of Rhodanobacter under environmental stress. ISME J. 93:e151. doi: 10.1093/ismejo/wrae151
Fein, J.B., Scott, S., and Rivera, N., The effect of Fe on Si adsorption by Bacillus subtilis cell walls: Insights into non-metabolic bacterial precipitation of silicate minerals, (2002), Available at: www.elsevier.comrlocaterchemgeo, 182, 265–273
Feng, J., Chen, B., Sun, W., and Wang, Y. (2021). Microbial induced calcium carbonate precipitation study using Bacillus subtilis with application to self-healing concrete preparation and characterization. Constr. Build. Mater. 280:122460. doi: 10.1016/j.conbuildmat.2021.122460
Guo, Z., Chai, Z., Liu, T., Gao, S., Hui, X., Zhang, C., et al. (2022). Pseudomonas aeruginosa-accelerated corrosion of Mo-bearing low-alloy steel through molybdenum-mediating chemotaxis and motility. Bioelectrochemistry 144:108047. doi: 10.1016/j.bioelechem.2021.108047
Guo, Z., Pan, S., Liu, T., Zhao, Q., Wang, Y., Guo, N., et al. (2019). Bacillus subtilis inhibits Vibrio natriegens-induced corrosion via biomineralization in seawater. Front. Microbiol. 10:e1111. doi: 10.3389/fmicb.2019.01111
Guo, Z., Wang, W., Guo, N., Zeng, Z., Liu, T., and Wang, X. (2019). Molybdenum-mediated chemotaxis of Pseudoalteromonas lipolytica enhances biofilm-induced mineralization on low alloy steel surface. Corros. Sci. 159:108123. doi: 10.1016/j.corsci.2019.108123
Han, Z., Wang, J., Zhao, H., Tucker, M. E., Zhao, Y., Wu, G., et al. (2019). Mechanism of biomineralization induced by Bacillus subtilis J2 and characteristics of the biominerals. Fortschr. Mineral. 9:40218. doi: 10.3390/min9040218
Huynh, N. N. T., Imamoto, K. I., and Kiyohara, C. (2019). A study on biomineralization using Bacillus subtilis natto for repeatability of self-healing concrete and strength improvement. J. Adv. Concr. Technol. 17, 700–714. doi: 10.3151/jact.17.700
Huynh, N. N. T., Imamoto, K. I., and Kiyohara, C. (2022). Biomineralization analysis and hydration acceleration effect in self-healing concrete using Bacillus subtilis natto. J. Adv. Concr. Technol. 20, 609–623. doi: 10.3151/jact.20.609
Johnson, C. R., and Fein, J. B. (2019). A mechanistic study of au(III) removal from solution by Bacillus subtilis. Geomicrobiol J. 36, 506–514. doi: 10.1080/01490451.2019.1573279
Kang, S. Y., Pokhrel, A., Bratsch, S., Benson, J. J., Seo, S. O., Quin, M. B., et al. (2021). Engineering Bacillus subtilis for the formation of a durable living biocomposite material. Nat. Commun. 12:7133. doi: 10.1038/s41467-021-27467-2
Keren-Paz, A., Maan, H., Karunker, I., Olender, T., Kapishnikov, S., Dersch, S., et al. (2022). The roles of intracellular and extracellular calcium in Bacillus subtilis biofilms. IScience 25:104308. doi: 10.1016/j.isci.2022.104308
Lin, W., Huang, Z., Li, X., Liu, M., and Cheng, Y. (2015). Bio-remediation of acephate–Pb(II) compound contaminants by Bacillus subtilis FZUL-33. J. Environ. Sci. 45, 94–99. doi: 10.1016/j.jes.2015.12.010
Mahmood, F., Rehman, S. K., Jameel, M., Riaz, N., Javed, M. F., Salmi, A., et al. (2022). Self-healing bio-concrete using Bacillus subtilis encapsulated in Iron oxide nanoparticles. Materials 15:7731. doi: 10.3390/ma15217731
Marvasi, M., Visscher, P. T., Perito, B., Mastromei, G., and Casillas-Martínez, L. (2010). Physiological requirements for carbonate precipitation during biofilm development of Bacillus subtilis etfA mutant. FEMS Microbiol. Ecol. 71, 341–350. doi: 10.1111/j.1574-6941.2009.00805.x
Mohsin, M. Z., Omer, R., Huang, J., Mohsin, A., Guo, M., Qian, J., et al. (2021). Advances in engineered Bacillus subtilis biofilms and spores, and their applications in bioremediation, biocatalysis, and biomaterials. Synth. Syst. Biotechnol. 6, 180–191. doi: 10.1016/j.synbio.2021.07.002
Mondal, S., and Ghosh, A. (2021). Spore-forming Bacillus subtilis Vis-à-Vis non-spore-forming Deinococcus radiodurans, a novel bacterium for self-healing of concrete structures: a comparative study. Constr. Build. Mater. 266:121122. doi: 10.1016/j.conbuildmat.2020.121122
Mudgil, D., Baskar, S., Baskar, R., Paul, D., and Shouche, Y. S. (2018). Biomineralization potential of Bacillus subtilis, Rummeliibacillus Stabekisii and Staphylococcus Epidermidis strains in vitro isolated from speleothems, Khasi Hill caves, Meghalaya, India. Geomicrobiol J. 35, 675–694. doi: 10.1080/01490451.2018.1450461
Mukherjee, T., and Venkata Mohan, S. (2021). Metabolic flux of Bacillus subtilis under poised potential in electrofermentation system: gene expression vs product formation. Bioresour. Technol. 342:125854. doi: 10.1016/j.biortech.2021.125854
Perito, B., Casillas, L., and Marvasi, M. (2018). Factors affecting formation of large calcite crystals (≥1 mm) in Bacillus subtilis 168 biofilm. Geomicrobiol J. 35, 385–391. doi: 10.1080/01490451.2017.1377788
Perito, B., Marvasi, M., Barabesi, C., Mastromei, G., Bracci, S., Vendrell, M., et al. (2014). A Bacillus subtilis cell fraction (BCF) inducing calcium carbonate precipitation: biotechnological perspectives for monumental stone reinforcement. J. Cult. Herit. 15, 345–351. doi: 10.1016/j.culher.2013.10.001
Sazanova, K. V., Frank-Kamenetskaya, O. V., Vlasov, D. Y., Zelenskaya, M. S., Vlasov, A. D., Rusakov, A. V., et al. (2020). Carbonate and oxalate crystallization by interaction of calcite marble with Bacillus subtilis and Bacillus subtilis–aspergillus Niger association. Crystals (Basel) 10, 1–16. doi: 10.3390/cryst10090756
Shim, H. W., Jin, Y. H., Seo, S. D., Lee, S. H., and Kim, D. W. (2011). Highly reversible lithium storage in Bacillus subtilis-directed porous Co3O4 nanostructures. ACS Nano 5, 443–449. doi: 10.1021/nn1021605
Song, J., Han, B., Song, H., Yang, J., Zhang, L., Ning, P., et al. (2019). Nonreductive biomineralization of uranium by Bacillus subtilis ATCC–6633 under aerobic conditions. J. Environ. Radioact. 208–209:106027. doi: 10.1016/j.jenvrad.2019.106027
Song, Q., Li, X., Hou, N., Pei, C., and Li, D. (2024). Chemotaxis-mediated degradation of PAHs and heterocyclic PAHs under low-temperature stress by Pseudomonas fluorescens S01: insights into the mechanisms of biodegradation and cold adaptation. J. Hazard. Mater. 469:133905. doi: 10.1016/j.jhazmat.2024.133905
Wightman, P. G., and Fein, J. B. (2005). Iron adsorption by Bacillus subtilis bacterial cell walls. Chem. Geol. 216, 177–189. doi: 10.1016/j.chemgeo.2004.11.008
Yan, H., Owusu, D. C., Han, Z., Zhao, H., Ji, B., Zhao, Y., et al. (2021). Extracellular, surface, and intracellular biomineralization of Bacillus subtilis Daniel-1 Bacteria. Geomicrobiol J. 38, 698–708. doi: 10.1080/01490451.2021.1937406
Yin, X., Weitzel, F., Griesshaber, E., Fernández-Díaz, L., Jimenez-Lopez, C., Ziegler, A., et al. (2020). Bacterial EPS in agarose hydrogels directs mineral Organization in Calcite Precipitates: species-specific biosignatures of Bacillus subtilis, Mycobacterium phley, Mycobacterium smagmatis, and Pseudomonas putida EPS. Cryst. Growth Des. 20, 4402–4417. doi: 10.1021/acs.cgd.0c00231
Keywords: bioprecipitation, molybdenum, corrosion, molecular mechanisms, RNAseq
Citation: Guo Z, Feng Q, Guo N, Yin Y and Liu T (2024) Positive effects of molybdenum on the biomineralization process on the surface of low-alloy steel catalyzed by Bacillus subtilis. Front. Microbiol. 15:1428286. doi: 10.3389/fmicb.2024.1428286
Edited by:
Eva Pakostova, Laurentian University, CanadaReviewed by:
Ruiyong Zhang, Chinese Academy of Sciences (CAS), ChinaDuorui Zhang, Northwest Normal University, China
Copyright © 2024 Guo, Feng, Guo, Yin and Liu. This is an open-access article distributed under the terms of the Creative Commons Attribution License (CC BY). The use, distribution or reproduction in other forums is permitted, provided the original author(s) and the copyright owner(s) are credited and that the original publication in this journal is cited, in accordance with accepted academic practice. No use, distribution or reproduction is permitted which does not comply with these terms.
*Correspondence: Zhangwei Guo, endndW9Ac2htdHUuZWR1LmNu; Tao Liu, bGl1dGFvQHNobXR1LmVkdS5jbg==