- 1Laboratory of Microbial Biotechnologies, Agrosciences and Environment, Labeled Research Unit-CNRST N°4, Faculty of Sciences Semlalia, Universiry Cadi Ayyad, Marrakesh, Morocco
- 2Laboratory of Innovative Materials, Energy and Sustainable Development (IMED-Lab), Cadi Ayyad University, Marrakesh, Morocco
- 3Department of Physics, Faculty of Sciences Semlalia, Cadi Ayyad University, Marrakesh, Morocco
- 4AgroBiosciences Program, College of Agriculture and Environmental Sciences, University Mohammed VI Polytechnic (UM6P), Ben Guerir, Morocco
- 5African Sustainable Agriculture Research Institute (ASARI), College of Agriculture and Environmental Sciences, University Mohammed VI Polytechnic (UM6P), Laâyoune, Morocco
Hexavalent chromium removal from the environment remains a crucial worldwide challenge. To address this issue, microbiological approaches are amongst the straightforward strategies that rely mainly on the bacteria's and fungi's survival mechanisms upon exposure to toxic metals, such as reduction, efflux system, uptake, and biosorption. In this work, scanning electron microscopy, energy-dispersive X-ray spectrophotometry, Fourier transform infrared spectroscopy, and zeta potential measurements were used to investigate the ability of chromium adsorption by Bacillus licheniformis, Bacillus megaterium, Byssochlamys sp., and Candida maltosa strains isolated from tannery wastewater. Scanning electron microscopy combined with energy dispersive X-ray spectroscopy revealed alterations in the cells treated with hexavalent chromium. When exposed to 50 mg/L Cr6+, Bacillus licheniformis and Candida maltosa cells become rough, extracellular secretions are reduced in Bacillus megaterium, and Byssochlamys sp. cells are tightly bound and exhibit the greatest Cr weight percentage. In-depth analysis of Fourier transform infrared spectra of control and Cr-treated cells unveiled Cr-microbial interactions involving proteins, lipids, amino acids, and carbohydrates. These findings were supported by zeta potential measurements highlighting significant variations in charge after treatment with Cr(VI) with an adsorption limit of 100 mg/L Cr6+ for all the strains. Byssochlamys sp. showed the best performance in Cr adsorption, making it the most promising candidate for treating Cr-laden wastewater.
1 Introduction
Chromium (Cr) and its salts are involved in plenty of industrial processes, such as the manufacturing of paints, dyes, plastics, and stainless steel, the wood treatment, and the leather tanning. Unlike its trivalent form (Cr3+), hexavalent chromium (Cr6+) poses a serious environmental problem (Fu et al., 2021; Lara et al., 2021; Wang et al., 2022; Aguilar et al., 2023; Mohanty et al., 2023) and triggers harmful effects that make its elimination essential (Wang et al., 2021; Aké et al., 2022; Alharbi et al., 2022). Moreover, Cr6+ is found to be particularly hazardous to health (Tangahu et al., 2020; Aparicio et al., 2021) and responsible for dysfunction in living organisms (Tripathi et al., 2018; Chromikova et al., 2022). Hence, Cr6+ removal has become the focus of attention in health and safety projects (Tumolo et al., 2020; Anupong et al., 2022; Ariram et al., 2022).
Based on these considerations, it is obvious that substantial efforts were deployed toward building environmentally friendly solutions for the treatment of chromium (VI). In this context, researchers developed biological methods making use plants and microorganisms to detoxify the environment (Xia et al., 2019; Yasir et al., 2021). Bacteria and fungi repeatedly demonstrated their ability to resist or remove Cr6+ present in environmental or culture media (Chang et al., 2016; Kalsoom K. et al., 2021; Aké et al., 2023). Indeed, it has been shown that anaerobically or aerobically cultivated bacteria in laboratory can eliminate the synthetic Cr6+ contained in culture media via a number of mechanisms (Lin et al., 2020; Plestenjak et al., 2022). In an earlier work, we demonstrated the aerobic reduction of Cr6+ to trivalent chromium by cultures of Bacillus licheniformis, Bacillus megaterium, Byssochlamys sp., and Candida maltosa strains isolated from tannery effluents (Aké et al., 2023). Similar reduction of Cr6+ to Cr3+ was observed for Penicillium sp. PL17, Fusarium proliferatum FBL1, Aspergillus fumigatus ML43, and Rhizopus sp. CUC23 (Bibi et al., 2018). The ability to reduce Cr6+ is one of the mechanisms of strain resistance to Cr (Aké et al., 2023) that include biosorption, bioaccumulation, biotransformation, and efflux systems (Gang et al., 2019; Xia et al., 2019; Ayele et al., 2021; Elahi et al., 2022; Plestenjak et al., 2022). Microorganisms can act as adsorbents, similar to carbon nanotubes, activated carbon, graphene oxide, polymers, clays, green slow-releasing denaturing colloidal substrates (such as gelatin, agar, and cane molasses), and their recently-used modifications (Sathvika et al., 2019). Once Cr6+ is in the vicinity of the cell, it can be attached (adsorption) to the cell's surface by specific molecules (COOH, , etc.) and subsequently reduced to Cr3+. This reduction may be spontaneous or due to a cytochrome network in the cell wall (Thatoi et al., 2014; Upadhyay et al., 2017; Li et al., 2021; Mat Arisah et al., 2021). Chromium adsorption can be checked by measuring its content after the cell washing (exposure), allowing one to quantitatively assess the concentrations of adsorbed ionic species, especially Cr6+ and Cr3+ ions. On the other hand, the researchers can identify the families of molecules involved in the adsorption of Cr6+ using Fourier transform infrared spectroscopy (FTIR) analyses (Kaduková and Virčíková, 2005; Sharma et al., 2022). Besides adsorption on the cell surface, chromium can penetrate the microbial cell through sulfate and phosphate transporters (, ), and Cr6+ can undergo enzymatic or non-enzymatic reduction (indirect reduction) inside the cell (Thatoi et al., 2014). The ability of microorganisms to adsorb Cr6+ and Cr3+ was also demonstrated using scanning electron microscopy (SEM) combined to energy dispersive X-ray spectroscopy (EDX) (Abo-Alkasem et al., 2022; Chromikova et al., 2022). At high concentrations, the authors found cellular modifications due to high Cr6+ concentrations (Abo-Alkasem et al., 2022; Chromikova et al., 2022). Therefore, the combination of FTIR and SEM-EDX analyses is a powerful tool to understand the Cr resistance mechanisms. While FTIR relies on the vibrational behavior of Cr-loaded cells to identify the chemical environments and involved functional groups, SEM and EDX provide an overview of the surface morphology and elemental contents in treated specimens. However, this approach is not sufficient since it does not provide insight into the electrical phenomena that occur between Cr molecules and ligands on the surface of microorganisms. The study of the electrical charges surrounding the cells enables a better understanding of the interactions between metals and microbial cells. To that end, zetametry is an effective tool to assess the electric charge that a particle, in suspension or in solution, acquires from the surrounding ion cloud using the potential difference between the particle-medium interfacial layer and the medium (the so-called zeta potential) (Samaké, 2008; Al-Jubory et al., 2020; Youssef et al., 2020). The combination of FTIR, SEM, EDX, and zetametry measurements has been used successfully in the study of Cd(II) biosorption by Bacillus cereus RC−1 cells, for instance (Huang et al., 2013) but not in a study of Cr6+ adsorption by bacteria and fungi.
Accordingly, the aim of the present work is to examine the potential of chromium (VI) adsorption and its biotransformation into chromium (III) using indigenous strains of Bacillus licheniformis, Bacillus megaterium, Byssochlamys sp., and Candida maltosa that were isolated from tannery effluents. SEM-EDX characterizations were carried out for the investigated biomasses to probe the modifications in morphology and chemical makeup after Cr loading. Similarly, FTIR characterizations were also performed to reveal the major vibrational alterations after treatment with chromium in order to incriminate the possible functional groups involved in the metal-microbial strain interactions. In this respect, we carried out in-depth analyses of vibrational modes in the investigated strains to provide a useful literature impetus about the infrared signature of investigated strains. Moreover, zeta potential characterizations were performed to assess the modifications in the electrical behavior of the strain environment upon Cr loading.
2 Materials and methods
2.1 Microorganism isolation
The raw tannery effluent was sampled from Bab Dbagh and the industrial district at Marrakech tannery manufacturing, which operates with chrome-tanning processes. Bacillus licheniformis, Bacillus megaterium, Byssochlamys sp., and Candida maltosa strains were isolated from tanneries effluents and identified using the previously reported molecular methods (Aké et al., 2023).
2.2 SEM-EDX analysis
Culture pellets were subjected to SEM and EDX characterizations in order to confirm Cr adsorption and to examine the impact of Cr on cell morphologies. The experimental procedure was similar to that applied by Karthik et al. (2017). After incubation for 96 h, the cells in 100-μL precultures with 0, 5, and 50 mg/L Cr6+ were harvested by centrifugation at 5000 rpm. After washing with a 0.1 mM phosphate buffer solution (pH 7) to remove the cells from the culture medium, the pellets were fixed overnight in 3% glutaraldehyde at 4 °C. Subsequent dehydration cycles using ethanol at different volume concentrations (20%, 40%, 60%, 80%, 90%, and 100%) were applied to the pellets treated with glutaraldehyde. The surface morphology and the chemical composition of dehydrated strains supported on carbon sheets were analyzed using a TESCAN VEGA 3 scanning electron microscope equipped with an energy-dispersive X-ray spectrometer. The SEM images were obtained by detecting the secondary electrons emitted by the samples when excited with a beam of 10 keV energy. The EDX spectra were recorded at a beam energy of 5 keV to detect the prominent Cr Lα radiation with an energy of about 0.57 keV. Further analyses were performed with a beam energy of 10 keV to check the detection of Cr Kα and Kβ characteristic lines located at 5.41 and 5.95 keV, respectively. Microbial cells cultured without Cr6+ were used as a control and are referred to as “native” hereafter.
2.3 FTIR analysis
In order to determine the impact of Cr6+ on the functional groups of the microbial cell surface, 100 μL of preculture (24 h) of each strain was introduced into Luria-Bertani (LB) broth containing 50 mg/L Cr6+ (30 °C, pH 7). At the same time, a control without Cr6+ was prepared for each strain. After 96 h incubation, the cells were then collected in 15 mL tubes and centrifuged at 5000 rpm (20 min, 4 °C). After that, the preparation procedure was performed according to the analytical method used by Maurya et al. (2022). The pellets were washed with a 0.1 mM phosphate buffer solution (pH 7) to remove to remove the cells from the culture medium, then freeze-dried using a Martin Christ Alpha 1-2 LO plus lyophilizer. A total 0.099 g of KBr powder was mixed with 0.001 g of each powder pellet obtained and grinded in a mortar. After mixing, the product was ground, homogenized, and then pressed into pellets using a compressor. The as-obtained pellets were introduced into a Bruker Vertex 70 FTIR spectrometer. Each IR spectrum was obtained by averaging 31 scans recorded in the 400–4000 cm−1 range with a wavenumber step of 2 cm−1.
2.4 Zeta potential analysis
In order to understand the electrostatic interactions between the microbial cell and Cr6+, the zeta potential measurements were carried out using a Malvern zetasizer ver. 7.12. To that end, a modified experiment derived from Beiranvand et al. (2022) was applied. Different concentrations of Cr6+, namely 25, 50, 100, 250, 500, and 800 mg/L, were prepared in distilled water. The inoculum of each strain was added to each Cr6+ concentration (5 mL). Positive controls, i.e., solutions without inocula, were also prepared. The zeta potential was also measured in pure distilled water.
2.5 Statistical analysis
The experiments from the study were carried out in triplicate. The data obtained were statistically analyzed and are presented with the appropriate standard deviation. ANOVA tests were carried out to better understand the effect of the microbial strain treatments. Post-hoc tests were then applied to observe the significance between groups. The least significant differences among means were evaluated at the 5% significance level. IBM SPSS Statistics 25 was used for the statistical analysis of the data.
3 Results
3.1 SEM analysis
The SEM images of Figure 1 depict the morphologies of the Bacillus licheniformis, Bacillus megaterium, Byssochlamys sp., and Candida maltosa strains without Cr treatment (upper images) and after exposure to 5 and 50 mg/L of hexavalent chromium (middle and bottom images, respectively). These SEM images are displayed with the same magnifications to show specific features in structure of each sample.
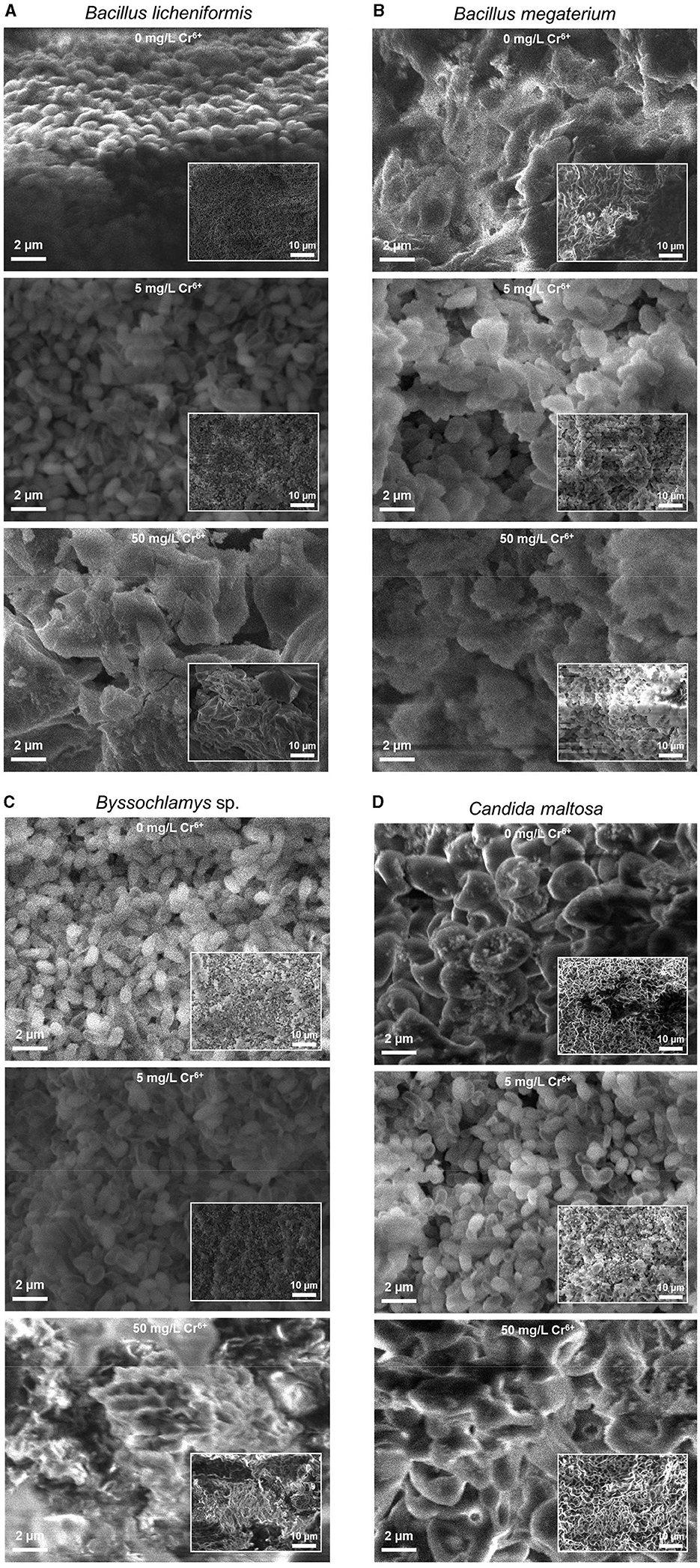
Figure 1. Secondary electron SEM images of the native (upper images) and treated strains with 5 (middle images) and 50 mg/L Cr6+ (bottom images) in LB medium for Bacillus licheniformis (A), Bacillus megaterium (B), Byssochlamys sp. (C), and Candida maltosa (D) strains isolated from tannery effluents. The SEM images and the inset low-magnification secondary electron SEM images are displayed with the same scales for all the samples.
In the case of the Cr6+-free Bacillus licheniformis strains, the cells are smooth, compact, and firmly grouped in the form of asymmetric clusters (Figure 1A). In addition, the native cells are typically regular in shape, and their assembly gives rise to a domed structure with corrugations. After contact with 5 mg/L Cr6+, the cells are still smooth and clustered, with a quite regular cell size. In other words, both of the native bacterial biomass and that exposed to 5 mg/L Cr6+ show similar cell surfaces. However, at 50 mg/L Cr6+, the appearance of the bacterial biomass is altered and shows wrinkled, rough, and irregular clusters of less distinct cells, with possible extracellular secretions.
In contrast, the Bacillus megaterium strains do not show very distinct cell shapes, either in the absence or presence of Cr6+ (Figure 1B). The cells form aggregates with apparent depressions and exhibit a very compact texture with irregular edges that appear to be bound with an extracellular matrix. The visual examination of SEM images suggests a higher content of extracellular secretions in the control than in the strains grown at 5 and 50 mg/L Cr6+.
For the Byssochlamys sp. strains (Figure 1C), the control and the strain loaded with 5 mg/L Cr6+ show the same appearance. Indeed, the cells appear smooth, less bound, and exhibit a few corrugations. In contrast, the strain cultured with 50 mg/L Cr6+ show a different topography since the cells are tightly bound and offer a compact space upon exposure to Cr6+.
Similarly, the Candida maltosa control cells and those treated with 5 mg/L Cr6+ show a smooth outline morphology with visible depression regions (Figure 1D). The SEM image of native strains shows structures of a regular size in the 1–2 μm range, which corresponds to the typical size of the Candida maltosa cells. However, at 5 mg/L Cr6+, some cells are less visible and form irregular blocks. At a concentration of 50 mg/L Cr6+, several cells had lose their initial structures and appear slightly rough and deformed.
3.2 EDX analysis
EDX analysis was performed to determine whether the microbial cell surfaces are capable of bio-adsorbing Cr. Figure 2 shows the EDX analysis of Bacillus licheniformis, Bacillus megaterium, Byssochlamys sp., and Candida maltosa strains grown without Cr treatment and after loading with 5 and 50 mg/L Cr6+. The determined weight and atomic percentages of Cr (Cr wt.% and Cr at.%, respectively) are given in Table 1.
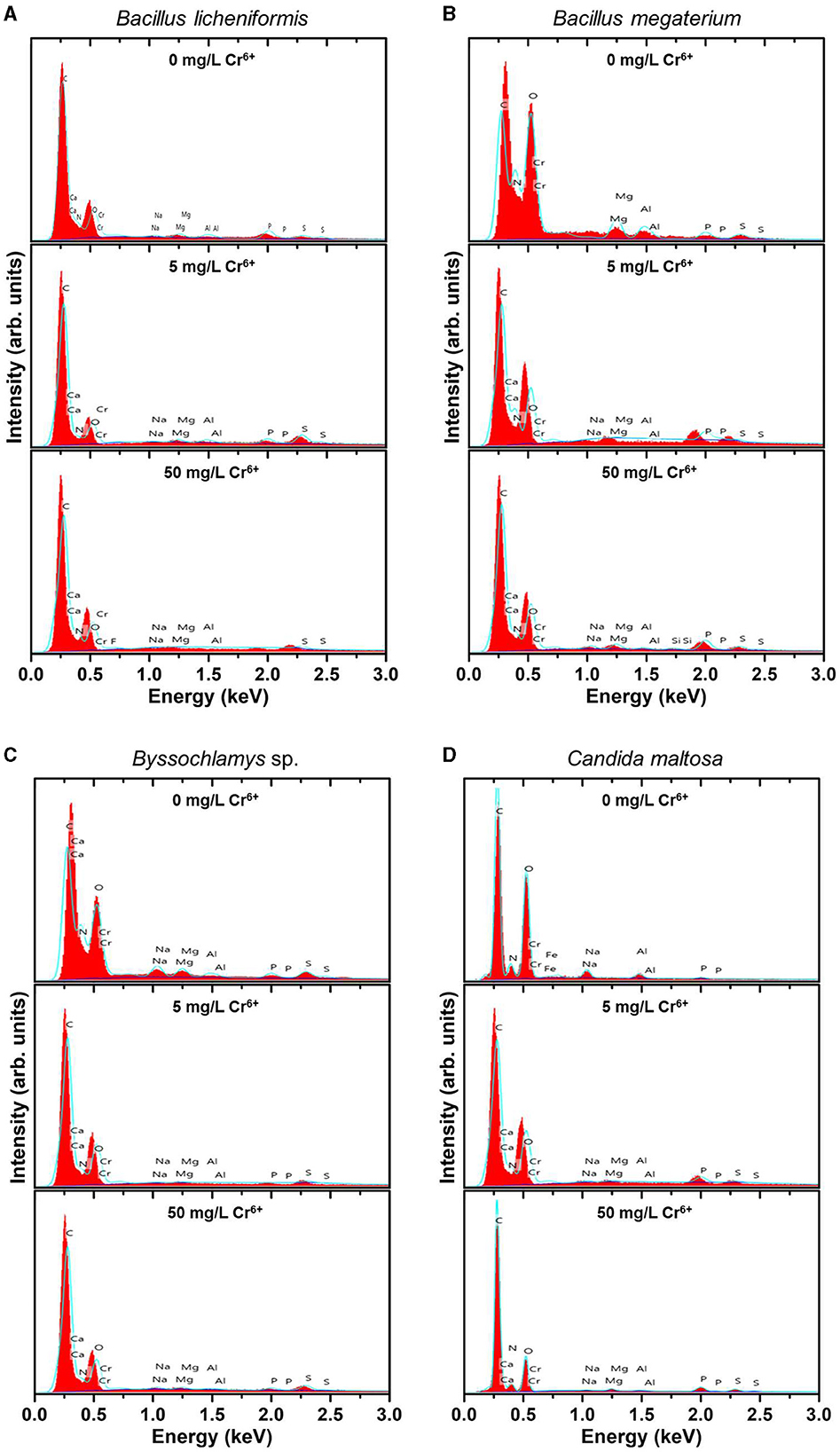
Figure 2. EDX spectra of the native (upper spectra) and treated strains with 5 mg/L Cr6+ (middle spectra) and 50 mg/L Cr6+ (bottom spectra) in LB medium for Bacillus licheniformis (A) Bacillus megaterium (B), Byssochlamys sp. (C), and Candida maltosa (D) strains isolated from tannery effluents.
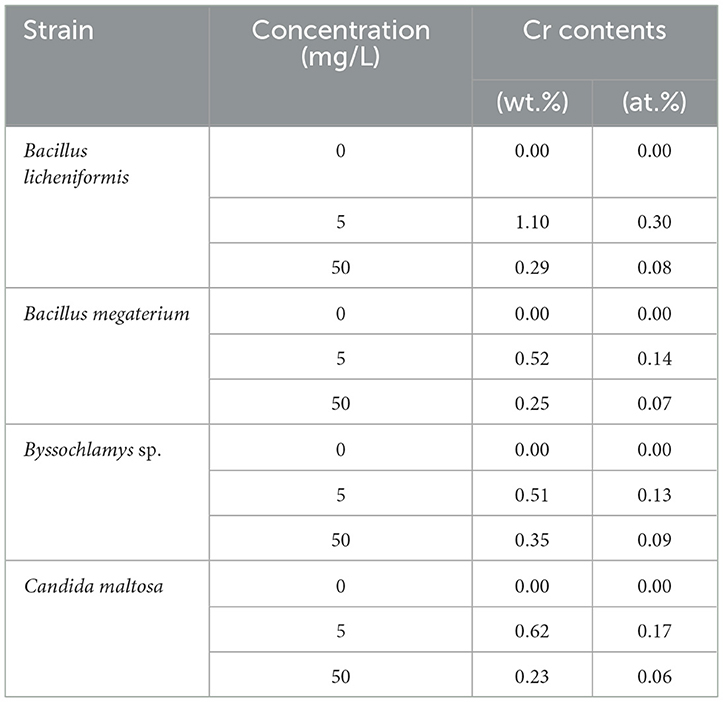
Table 1. Weight and atomic percentages of Bacillus licheniformis, Bacillus megaterium, Byssochlamys sp., and Candida maltosa microbial biomasses before (0 mg/L Cr6+) and after treatment with 5 and 50 mg/L Cr6+.
Besides the characteristic C K line (overlapping of the Kα and Kβ peaks) located at about 0.28 keV, the EDX spectra of all the control strains show no signature of the Cr Lα feature (at 0.57 keV) but the neighboring O K feature arising from the overlapping Kα and Kβ peaks (located at ~0.52 and 0.53 keV, respectively). The nitrogen signature (expected at 0.39 and 0.4 keV) is not discernible from the prominent carbon peak. Additional small peaks at energies consistent with the Kα and Kβ lines of Na (1.04 and 1.07 keV), Mg (1.25 and 1.3 keV), Al (1.49 and 1.55 keV), P (2.01 and 2.14 keV), and S (2.31 and 2.47 keV) are also observed. The presence of these peaks probably originates from compounds present in the cell wall.
In contrast, the biomasses that has grown in the Cr6+-amended culture media show the abovementioned Cr peak (Cr Lα line at 0.57 keV). After treatment of the microorganisms with 5 mg/L Cr6+, the EDX analyses reveal Cr weight percentages of 1.1, 0.52, 0.51, and 0.62 and atomic percentages of 0.30, 0.14, 0.13, and 0.17 for Bacillus licheniformis, Bacillus megaterium, Byssochlamys sp., and Candida maltosa, respectively. Nevertheless, chromium contents decrease in each strain after exposure to 50 mg/L Cr6+; the Cr weight percentages were 0.29, 0.25, 0.35, and 0.23, and the Cr atomic percentages were 0.08, 0.07, 0.09, and 0.06 for Bacillus licheniformis, Bacillus megaterium, Byssochlamys sp., and Candida maltosa, respectively.
3.3 FTIR analysis
The FTIR spectra of the investigated strains consist of numerous overlapping bands, most of which are derived from protein, lipid, amino acid, and carbohydrate functional groups. Table 2 summarizes the prominent identified vibrations and corresponding assignments for the Bacillus licheniformis, Bacillus megaterium, Byssochlamys sp., and Candida maltosa specimens, before and after chromium loading.
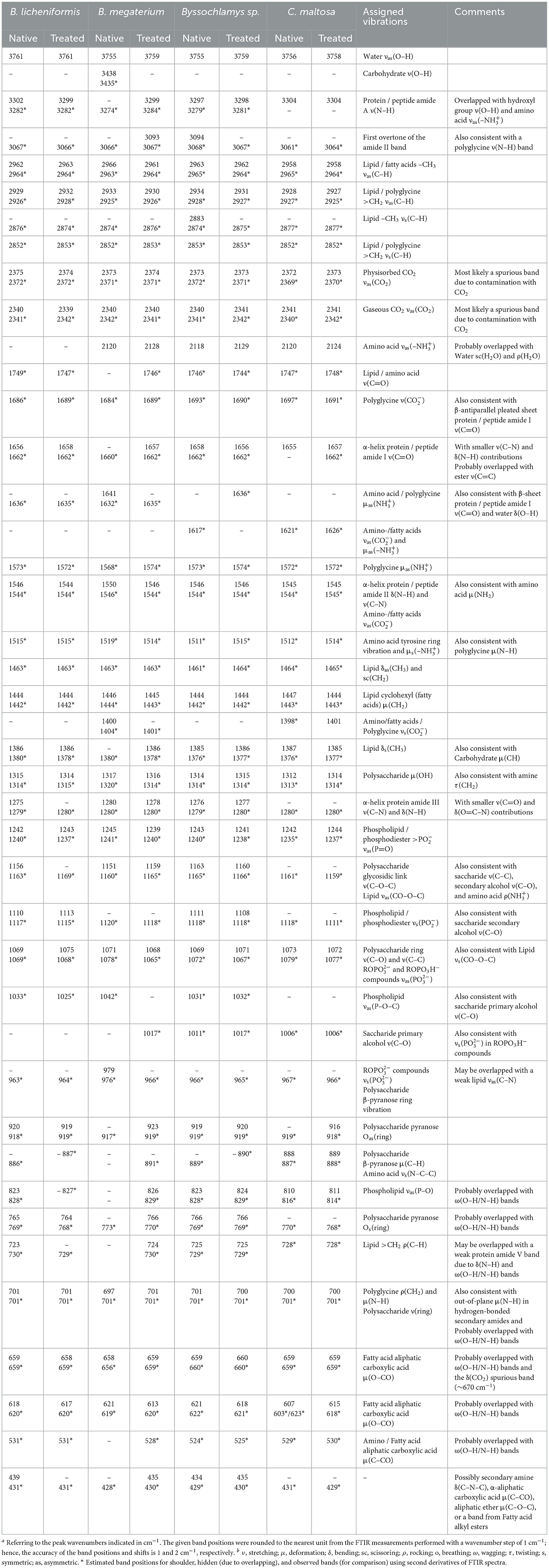
Table 2. Frequenciesa and tentative assignment of prominent vibrational modesb in native and chromium(VI)-treated Bacillus licheniformis, Bacillus megaterium, Byssochlamys sp., and Candida maltosa strains as identified from FTIR measurements.
As depicted in Figure 3, the FTIR spectrum of the native Bacillus licheniformis strains shows a prominent and asymmetric absorption band peaking at 3302 cm−1 due to protein and peptide N–H stretching (amide A band), which overlaps with a broad band related to O–H stretching from hydroxyl groups in carbohydrates (~3550–3150 cm−1), along with the asymmetric stretching band from amino acids (~3200–3000 cm−1). Two strong secondary polyamide bands can be seen at ~1656 and 1546 cm−1. The vibration at 1656 cm−1 is ascribed to the amide I band in α-helical structures (proteins and polypeptides), which is dominated by C=O stretching (Venyaminov and Kalnin, 1990b; Socrates, 2001). In this regard, it is noteworthy to mention that C=C stretching in lipid esters (expected at about 1650 cm−1), β-type secondary structures of proteins (indiscernible features), along with and vibrations in amino acids (around 1686 and 1636 cm−1), may have contributed to the above-assigned amide I band (Venyaminov and Kalnin, 1990a,b; Naumann, 2000; Socrates, 2001). The band at 1546 cm−1 corresponds to the amide II band originating from in-plane N–H bending and C–N stretching and gives rise to an overtone feature, as shown by the shoulder around 3090 cm−1 (~3067 cm−1 as determined using the second derivative).
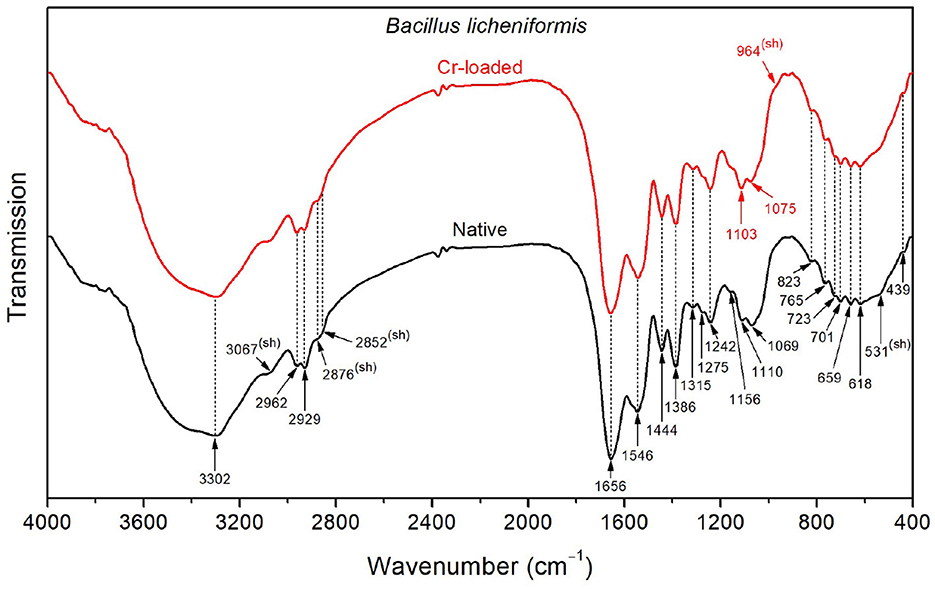
Figure 3. FTIR spectra of the Bacillus licheniformis bacterial strains before Cr loading (native) and after treatment with 50 mg/L Cr6+ (Cr-loaded).
Moreover, the CH3 and CH2 functional groups (fatty acid chains in lipids) exhibit characteristic C–H asymmetric stretching vibrations at 2962 and 2929 cm−1, respectively. The corresponding C–H asymmetric stretching vibrations were resolved into components at 2876 and 2852 cm−1, respectively, yielding a single shoulder-like feature at about 2860 cm−1. These aliphatic groups are also seen at 1444, 1386, and 723 cm−1, corresponding to CH2 deformation, CH3 symmetric bending, and CH2 rocking characteristic bands, respectively (Socrates, 2001). The FTIR spectra also display weak features corresponding to the amide III band in α-helical protein and peptide structures (caused by coupled C–N stretching and N–H bending) and the amide V band (originating from N–H bending), as shown by the shoulders at ~1275 and 726 cm−1, respectively.
Characteristic bands of phosphodiester, free phosphate, and polysaccharide functional groups are found in the 1250–900 cm−1 region. Indeed, the bands at 1242 and 1110 cm−1 are attributed to the phosphodiester stretching modes, while the band at 1069 cm−1 refers to free phosphate ionic species. However, the latter is also consistent with the stretching of C–O and C–C bonds in polysaccharide rings and C–O–C bonds in lipids (Wilson et al., 2000; Socrates, 2001). Similarly, the band at 1156 cm−1 is consistent with C–O–C stretching in lipids, C–O stretching in monosaccharide compounds (such as glucose), and C–O–C stretching of the glycosidic link in polysaccharides (Naumann, 2000; Socrates, 2001). The bands at 765 and 920 cm−1 also refer to symmetric and asymmetric breathing of the pyranose ring in polysaccharide compounds (Copíková et al., 2001; Socrates, 2001). In this regard, the vibration at 886 cm−1 is characteristic of the anomer C–H deformation in carbohydrates and provides evidence of the major β-form of the polysaccharide pyranose rings (Socrates, 2001; Hong et al., 2021).
In the wavenumber range below 900 cm−1, weak-to-medium intensity features are overlapped with a broad medium-to-strong band centered at roughly 660 cm−1. The latter is typical of hydroxyl group O–H wagging, but it can also include contributions from other hydrogen-bonded groups, such as water O–H or amide N–H wagging motions. The presence of α-amino-acids (such as glutamic acid and alanine) in the Bacillus licheniformis spore is supported by features related to O–CO (bands at 659 and 618 cm−1) and C–CO (shoulder at 531 cm−1) deformation vibrations in α-aliphatic carboxylic acids in this spectral range (Hughes, 1968). The band at 439 cm−1 cannot be restrainedly assigned by cross-referencing with other bands and is not used in the discussion hereafter.
The FTIR spectrum of native Bacillus licheniformis also includes hidden vibrations, such as the band at 1749 cm−1 originating from C=O stretching of carboxylic groups (esters and fatty acids), the band at 1515 cm−1 related to C–C stretching in the tyrosine aromatic ring (side-chain vibration in amino acids), or the band at 1463 cm−1 due to methyl and methylene vibrations (Schmitt and Flemming, 1998; Socrates, 2001; Tremmel et al., 2005; El-Naggar et al., 2020).
The broad weak band at around 2120 cm−1, which overlaps with the water scissoring and rocking vibrations, is related to amino acid symmetric stretching (Socrates, 2001; Lasagabaster et al., 2006). Close to this band, the absorption features at 2375 and 2340 cm−1 correspond to asymmetric stretching of adsorbed and gaseous CO2, respectively (Busca and Lorenzelli, 1982; Seiferth et al., 1999) and are most likely brought on by residual contamination.
The Cr(VI) treatment brings about the rise of the shoulder band (~964 cm−1) and the shift (from 1069 to 1075 cm−1) of the symmetric and asymmetric stretching bands, respectively. Furthermore, the symmetric stretching band exhibits an increase in intensity along with a slight shift (from 1110 to 1113 cm−1). These changes suggest that the phosphate-containing compounds are involved in the interaction with metal ions. This interaction yields the emergence of negative phosphoryl groups as a result of a deprotonation process. This is further supported by the partial vanishing and the shift (from 1033 to 1025 cm−1) of the P–O–C stretching shoulder band. In addition, the increase of the band (~1110 cm−1) is related to the observed weakening of the CH3 stretching, as shown by the decrease in intensity of the band at 2929 cm−1 (with respect to the CH2 band). This is also substantiated by the relative decrease of the CH3 bending band (1386 cm−1) with respect to the CH3 deformation band (~1444 cm−1) (Barkleit et al., 2011).
In contrast to Bacillus licheniformis, the high-frequency region in the native Bacillus megaterium spectrum (Figure 4) is dominated by the OH stretching band (3438 cm−1) superimposed with the NH stretching band (shoulder at 3274 cm−1). This is also visible at low frequencies, where the broad OH wagging (400–900 cm−1) fully dominates the cluster of characteristic polysaccharide and amino acid absorption bands in this range. These intense OH vibrations probably stem from the monosaccharide content (mainly composed of D-glucose, D-xylose, D-galactose, and L-arabinose) in the Bacillus megaterium capsule (Cassity and Kolodziej, 1984). Additionally, the amide I and II bands appear at shifted positions (1641 and 1550 cm−1, respectively) as a result of strong stretching of and COO− in amino acids (Venyaminov and Kalnin, 1990a). This is in agreement with the medium and broad band observed at 2120 cm−1 originating from asymmetric stretching and the COO− band at 1400 cm−1. Therefore, a broad medium band arising from symmetric stretching in the 2760–2530 cm−1 region could explain the weak intensity of CH2 and CH3 bands and shoulders observed at 2966, 2933, 2874, and 2852 cm−1.
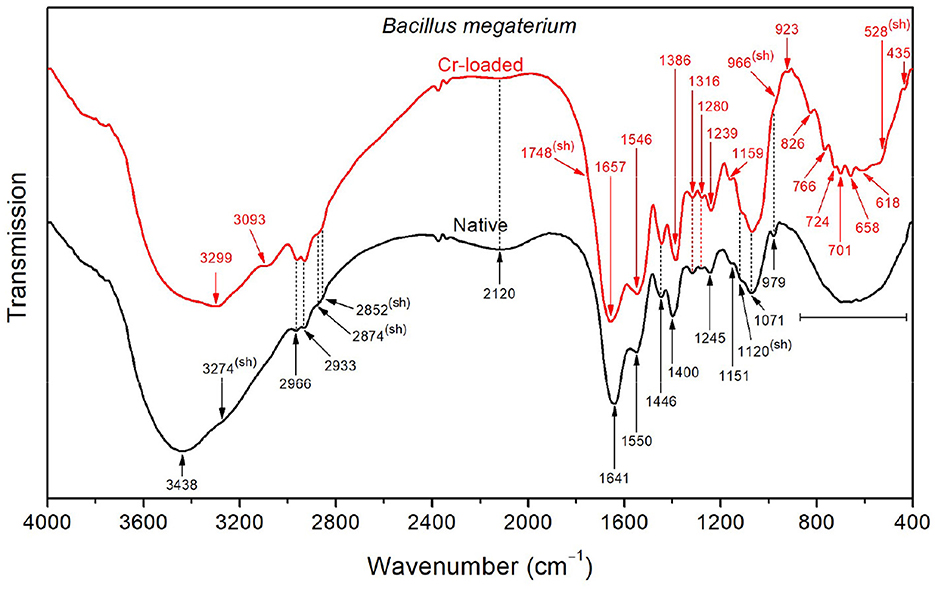
Figure 4. FTIR spectra of the native and Cr-treated (with 50 mg/L Cr6+) Bacillus megaterium bacterial strains.
Upon exposure of the Bacillus megaterium strains to Cr(VI), the intense OH stretching band undergoes a significant decrease in intensity, revealing the underlying NH stretching band at 3299 cm−1, which indicates that conformational changes occurred. Furthermore, the amide I and II bands appear at 1657 and 1546 cm−1, indicating the decrease of the amino acid and bands, as mentioned above. Accordingly, the CH3 bending band appears at 1387 cm−1 as a result of the weakened symmetric stretching band. The emergence of a noticeable shoulder at 1748 cm−1 relative to C=O stretching in COOH functional groups also witnesses that the amino acids are involved in the interaction with chromium. The bands in the region below 900 cm−1 appear after chromium treatment as a result of the weakening of presumed OH wagging. However, the overall absorption in the 1800–900 cm−1 region increases after treatment with chromium, which could be tentatively assigned to the emergence of overtone features related to NH2 wagging in the 900–400 cm−1 region.
As shown in Figure 5, the FTIR spectrum of the native Byssochlamys sp. strains exhibits typical vibrational bands as seen previously, including the overlapped OH and NH stretching bands peaking at 3297 cm−1, CH2 and CH3 asymmetric stretching bands at 2963 and 2934 cm−1, and the characteristic vibrations of carbohydrates and lipids in the 900–400 cm−1 spectral region (Table 2). The main specificities lie in the broadened amide bands at 1658 and 1546 cm−1, most likely due to motions of the carboxylate and ammonium functional groups, as previously explained.
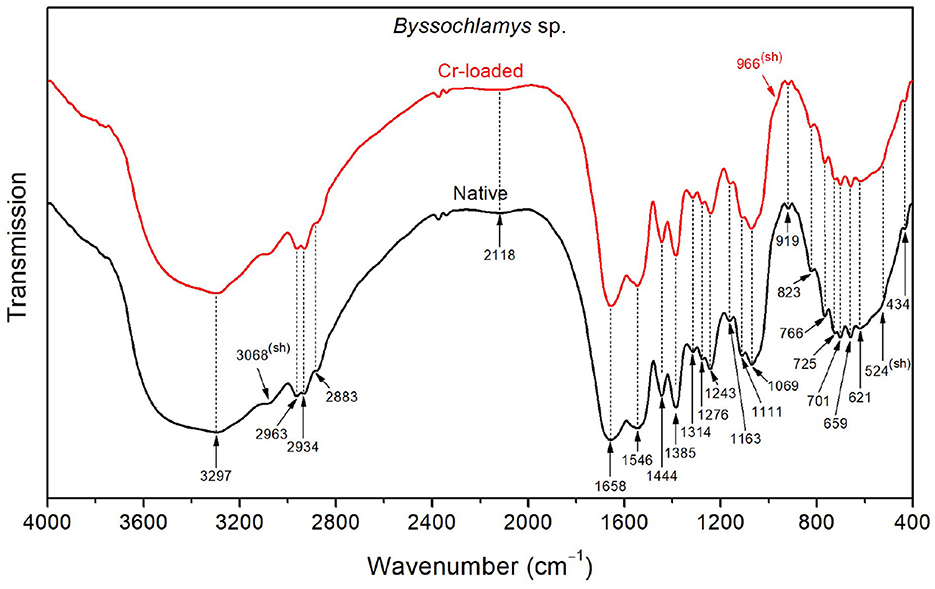
Figure 5. FTIR spectra of the Byssochlamys sp. strains before Cr loading (native) and after treatment with 50 mg/L Cr6+ (Cr-loaded).
After treatment with chromium, the high-frequency OH stretching band (~3400 cm−1) and the low-frequency OH wagging band (900–400 cm−1) slightly decreased in intensity. Additionally, the side of the amide I band toward low frequencies (i.e., lower than 1658 cm−1) and that of the amide II band toward high frequencies (i.e., <1546 cm−1) become narrower, which indicates reduced contributions from the asymmetric deformation (found at 1626 and 1572 cm−1 for the treated strains).
With respect to Bacillus licheniformis, the spectrum of the native Candida maltosa strains (Figure 6) exhibits an intense broad O–H stretching band in the region 3650–3550 cm−1, along with a stronger O–H wagging in the region 900–400 cm−1. This is likely to arise from the hydroxyl-rich glycosylphosphatidylinositol in the outer layer (mannose-containing compounds) and the polysaccharide-rich inner layer (β-glucan and chitin) in the cell wall of Candida strains (Chaffin et al., 1998; Kapteyn et al., 2000; Gow et al., 2011; Zvonarev et al., 2021). Accordingly, the methylene groups (abundant in the phospholipid tail of glycosylphosphatidylinositol) show a stronger absorption with respect to the methyl groups, as shown by the asymmetric stretching bands (2928 and 2958 cm−1, respectively) and the sharpened shoulder band at 2852 cm−1 (i.e., at the position of symmetric stretching of CH2). This is also consistent with the strong absorption in the spectral ranges 1200–950 cm−1 due to the C–O stretching in polysaccharide C–OH and C–O–C branches (mannans) and 1400–1300 cm−1 due to carbohydrate OH and CH deformation vibrations (see Table 2). Besides, the amide I band (~1655 cm−1) is relatively broad, probably due to overlapping with the stretching features (~1697 and 1621 cm−1).
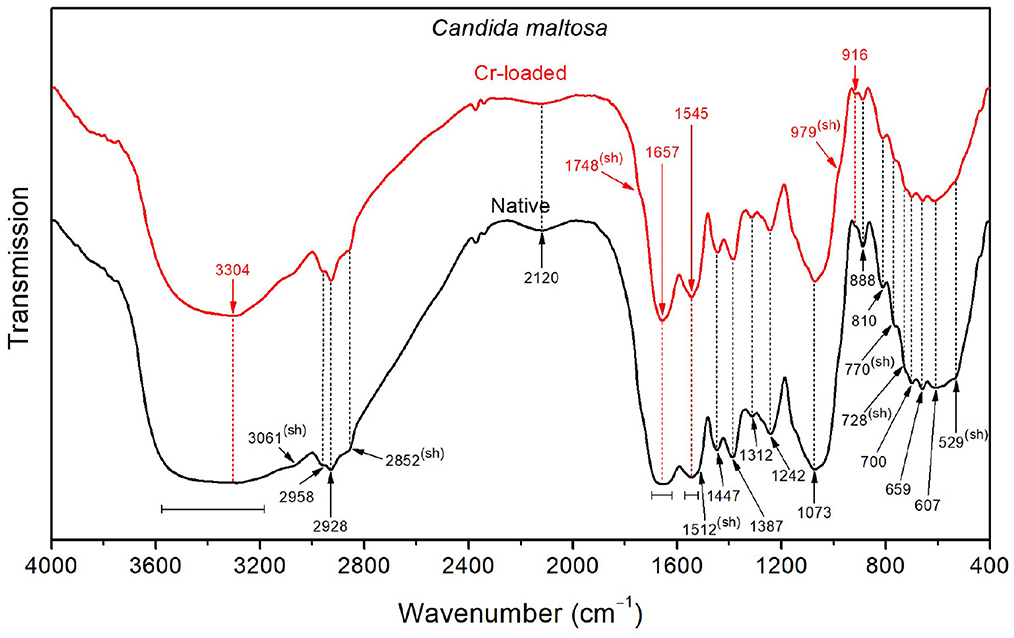
Figure 6. FTIR spectra of the Candida maltosa strains before Cr loading (native) and after treatment with 50 mg/L Cr6+ (Cr-loaded).
The FTIR spectrum shows a decrease in the intensity of the OH stretching band after loading with Cr(VI), suggesting that hydroxyl groups may be involved in the interaction with metallic ions. In addition, the shoulder relative to C=O stretching of –COOH functional groups (~1748 cm−1) becomes more noticeable after chromium treatment. The narrower amide I band (~1657 cm−1) for the treated strain probably originates from the partial vanishing of the stretching modes, which were also shifted (from 1697 to 1691 cm−1 and from 1621 to 1626 cm−1). The same effect can be seen for the amide II band (~1545 cm−1), probably due to the decrease of deformation vibrations, as shown by the softened shoulder band at 1512 cm−1. This is further supported by the decrease in intensity of the stretching band at 2120 cm−1 upon chromium treatment.
3.4 Zeta potential analysis
The zeta potential was measured for the four microbial strains in the absence and presence of different Cr6+ concentrations ranging from 25 to 800 mg/L (Table 3). Before contact with Cr6+, the microbial strains show different zeta potentials of about −10.63 ± 0.38, −15.9 ± 1.39, −8.98 ± 0.40, and −10.15 ± 2.68 mV for Bacillus licheniformis, Bacillus megaterium, Byssochlamys sp., and Candida maltosa, respectively. These negative potential differences with respect to the medium are related to the amounts of negative charges present at the vicinity of the microorganisms before being brought into contact with Cr6+.
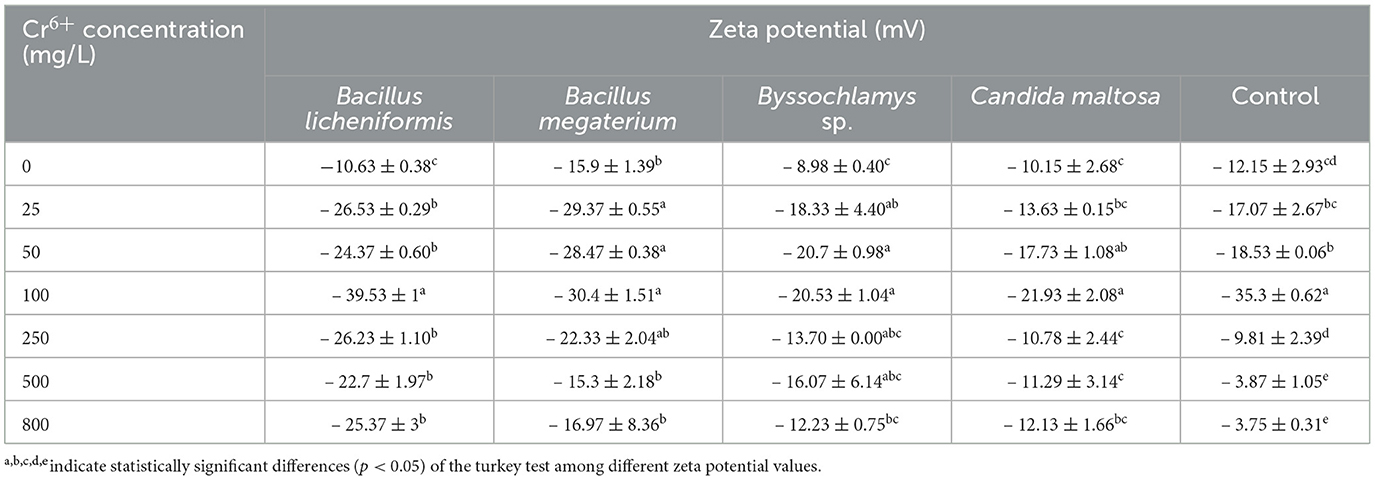
Table 3. Zeta potential of Bacillus licheniformis, Bacillus megaterium, Byssochlamys sp., and Candida maltosa strains in the absence and presence of different concentrations of Cr6+.
After exposure to chromium, the zeta potential of Bacillus licheniformis strains undergoes a significant change from −10.63 ± 0.38 (0 mg/L Cr6+) to −39.53 ± 1 mV (100 mg/L Cr6+), indicating that the cells in contact with Cr6+ have experienced an increase in negative charge. As the Cr6+ concentration increases in Bacillus licheniformis, the zeta potentials are statistically identical up to 100 mg/L. The zeta potential reaches the highest value (−39.53 ± 1 mV) at 100 mg/L before decreasing beyond that.
For Bacillus megaterium, the zeta potential range from −15.3 ± 2.18 to −30.4 ± 1.51 mV for the investigated Cr6+ concentrations. Significant differences are also observed between the control and the strains treated with different concentrations of Cr6+ (from 25 to 250 mg/L).
The Cr6+-treated Byssochlamys sp. show zeta potentials that are statistically different from those of the control. The zeta potentials are between −8.98 ± 0.40 and −20.7 ± 0.98 mV and correspond to the values for the Cr6+ concentrations of 0 and 50 mg/L. Statistically, the highest zeta potential values are −20.7 ± 0.98 and −20.53 ± 1.04 mV (50 and 100 mg/L of Cr6+). In contrast, the lowest value is recorded for the control (−8.98 ± 0.40 mV).
Finally, zeta potentials that are statistically different from the control were recorded for the Cr6+-treated Candida maltosa strains. This concerns the zeta potentials of treatments with 25, 50, 100, and 800 mg/L Cr6+. The zeta potential values range from −10.15 ± 2.68 to −21.93 ± 2.08 mV (control and 100 mg/L Cr6+).
4 Discussion
Biosorption (or bioadsorption) is a passive, rapid, and reversible physico-chemical phenomenon between a metal and a biological material (biosorbent) (Fernandez et al., 2018). It independent from cell activity carried out by active or inactive microorganisms (Fernandez et al., 2018). This process was assessed using SEM-EDX, FTIR analysis, and zeta potential measurements. Adsorption relies on hexavalent (or trivalent chromium produced by hexavalent chromium reduction) binding through a number of molecules. Biosorption is also the passive immobilization of metals by biomass. Sorption mechanisms at the cell surface take place independently of the cell's metabolism. These mechanisms are based on physico-chemical interactions between the metal and functional groups in the cell wall (Kaduková and Virčíková, 2005).
4.1 SEM analysis
The morphology of the microbial strains analyzed by SEM showed different characteristics. The Bacillus licheniformis strains have a smooth surface in the control and after treatment with 5 mg/L Cr6+. Similar results obtained by Hossan et al. (2020) showed that Klebsiella sp. have a smooth surface in the control cells. This smooth structure in untreated cells has also been observed in previous studies (Karthik et al., 2017; Bharagava and Mishra, 2018; Zhu et al., 2019; Kalola and Desai, 2020; Tan et al., 2020; Abo-Alkasem et al., 2022). Jobby et al. (2019), Selvakumar et al. (2021), and Su et al. (2021) demonstrated that the cells of Sinorhizobium sp. SAR1, Bacillus vietnamensis, Bacillus lentus, Alcaligenes faecalis, Staphylococcus cohnii, Staphylococcus saprophyticus, and Rhodobacter sphaeroides SC01 also have smooth surfaces in the absence of Cr6+. Additionally, intact and regular cells of Bacillus licheniformis were observed in the absence of metal. This case is similar to that found by Sevak et al. (2023) in Acinetobacter sp. biomass. Hossan et al. (2020) observed depression in Klebsiella sp. treated with 100 mg/L Cr6+. After exposure to 5 mg/L Cr6+, the biomass of Bacillus licheniformis retains its smooth structure and the metal does not damage the cells, likely indicating the effective resistance of the cell at this concentration level. The wrinkles, rough clusters, irregular shapes, and possible production of extracellular substances in the biomass treated at higher concentrations (50 mg/L Cr6+) revealed that the cells develops a strategy to circumvent the toxic effect of Cr6+. Elahi et al. (2022) described wrinkles in Bacillus cereus strain b-525k after contact with 2 mM Cr6+. Similarly, Hossan et al. (2020) reported ruptured surfaces in the biomass of Klebsiella sp. after treatment with Cr6+.
The Bacillus megaterium cells were not individually distinguishable with and without Cr6+ in the medium. Hossan et al. (2020) noted a similar case in Klebsiella sp. control. In addition, the irregular appearance observed in Bacillus megaterium in media with or without Cr6+ is a common phenomenon in microorganisms. This could be due to the secretion of extracellular substances around the cells. These extracellular substances are probably reduced in cells grown at 50 mg/L Cr6+ because of the toxic effect of the metal and could be exopolysaccharides. These research findings are in accordance with earlier studies (Ozturk et al., 2009; Jobby et al., 2019).
The Byssochlamys sp. control biomass and that treated with 5 mg/L Cr6+ present identical aspects. The concentration of 5 mg/L Cr6+ shows no impact on the cells, which remain smooth and less bound. However, at 50 mg/L Cr6+, the cells group together to provide a better Cr6+ adsorption surface. In this regard, Majumder et al. (2017) found ruptured surfaces with Cr6+ saturation on the biomass of Arthrinium malaysianum.
The Candida maltosa control cells and those treated with 5 mg/L Cr6+ appear in clusters with smooth outlines. However, at 5 mg/L Cr6+, some cells are less visible and form irregular blocks. This phenomenon is thought to be due to the production of extracellular substances to resist the toxic effect of Cr6+. At 50 mg/L Cr6+, several cells are unable to retain their initial structures probably due to damaged cellular constituents of strains, as a result of the toxic effect of Cr6+ (Mat Arisah et al., 2021).
In the literature, some authors mentioned that wrinkles appeared on the biomass of Aspergillus terricola after treatment with Cr6+ (Mohamed et al., 2021). In the absence of Cr6+ in the medium, Dwivedi (2023) found that the hyphae of Talaromyces pinophillus are thin, rough, and loose. However, with Cr6+ stress, the hyphae are aggregated and dense. Similarly, Saranya et al. (2020) observed the same rough structures on Trichoderma asperellum after contact with Cr6+ and indicated the adsorption of Cr6+ and Cr3+.
Majumder et al. (2017) showed that the cell surfaces are saturated by the toxic agent, and Mat Arisah et al. (2021) explain that the rough surfaces induced by Cr in the treatments are due to the adsorption of Cr6+.
4.2 EDX analysis
EDX analysis showed the presence of peaks in all the biomasses stressed with Cr6+. However, the biomasses not treated with Cr showed no chromium peaks. The appearance of Cr peaks in the biomasses treated with 5 and 50 mg/L Cr6+ indicates the presence of Cr bound to the cell surfaces of the strains (Karthik et al., 2017). The drop in the weight percentage of Cr is thought to be due to Cr toxicity, which damages parts of the cell surfaces (Su et al., 2021). Indeed, Cr is a metal capable of causing cell lysis and therefore bacteria and fungi could biosorb it. In this respect, Majumder et al. (2017) found that chromium could bind to the dried biomass of the fungus Arthrinium malaysianum (Cr peak detection). Similarly, Karthik et al. (2017) studied the ability of Cellulosimicrobium funkei strain AR6 to adsorb Cr at concentrations ranging from 100 to 250 μg/mL. They attributed the presence of Cr peaks to Cr3+ (reduction of Cr6+) bound to cell surfaces. Kalola and Desai (2020) pointed out that Cr adsorption also occurs in Halomonas sp. DK4. Additionally, Cr-specific peaks were detected in Bacillus vietnamensis, Bacillus lentus, Alcaligenes faecalis, Staphylococcus cohnii, and Staphylococcus saprophyticus treated with 3400 mg/L Cr6+ (Selvakumar et al., 2021). Su et al. (2021) and Kalsoom A. et al. (2021) indicated that the presence of Cr peaks could be due to the complexation of Cr3+ ions with molecules located on the cell surface or the presence of reduced Cr3+ species precipitated on the outer surface of the strains. The peak observed by the scientists (Kalsoom A. et al., 2021) was in the biomass of Staphylococcus simulans treated with 1500 μg/mL Cr6+ (0.19 wt.% Cr).
The weight percentage of chromium detected in Salipaludibacillus agaradhaerens strain NRC-R stressed with 4 mM Cr6+ was 0.16 % (Abo-Alkasem et al., 2022). Moreover, Elahi et al. (2022) showed that adsorption is one of the detoxification mechanisms of Bacillus cereus b-525k.
Microcharacterization of Cellulosimicrobium sp. (SCRB10), Bacillus sp. CRB-B1, and Klebsiella sp. treated with 100, 150, and 100 mg/L Cr6+, respectively, revealed Cr adsorption with weight percentages equivalent to 0.71, 3.54, and 6.02, respectively (Bharagava and Mishra, 2018; Hossan et al., 2020; Tan et al., 2020). These findings are in contrast with the results of some scientists showing that some strains do not adsorb Cr. For instance, Jobby et al. (2019) and Sevak et al. (2023) demonstrated that Sinorhizobium sp. SAR1 and Acinetobacter junii strain b2w do not adsorb Cr after contact with metal.
4.3 FTIR analysis
FTIR can be used to detect the functional groups that allow the adsorption of Cr. The chromium adsorption mechanism was carried out in a culture medium enriched with Cr6+ in order to identify the functional groups that appear only in the presence of Cr6+. This gave us a clear understanding of Cr6+ bioremediation. The presence of other metals would have obscured this understanding.
Filamentous fungi are capable of adsorbing Cr6+ and this ability has been demonstrated by several researchers (Shroff and Vaidya, 2012; Majumder et al., 2017). The absorption peaks show molecules, such as NH, CH3, CH2, C=O, C=N, OH, PO2−, P–O, and O–CO in Bacillus licheniformis control and exposed to Cr6+. This suggests that these molecules are involved in the binding of Cr6+ or Cr3+ (Ayele et al., 2021; Su et al., 2021; Elahi et al., 2022). The peaks that remain at 3067, 1275, 1156, 1110, 1069, and 531 cm−1 and that corresponding to amide II, amide III, C–O–C, PO2−, C–O, C–C, , C–CO, or those that appear at 1075 cm−1 (C–O and C–C) and 964 cm−1 () highlight the strain resistance to metal (Su et al., 2021).
Functional groups, such as CH3, CH2, , hydroxide (OH), amide III, PO2−, C–O, C–C, and found on the cell surfaces of Bacillus megaterium biomasses grown in the absence and presence of Cr6+ are involved in the binding of Cr6+ and Cr3+ ions (Ayele et al., 2021; Su et al., 2021; Elahi et al., 2022). O–H (3438 cm−1), amide A (3274 cm−1), (1641 cm−1), N–H and C–N (1550 cm−1), (1400 cm−1), PO2− (1245 cm−1), and C–O–C (1151 cm−1) were mainly identified on the control cells. These molecules play an important role in Cr resistance. In cells in contact with Cr6+, amide A (3299 cm−1), C=O (1748 cm−1; 1657 cm−1), (1546 cm−1), CH3 (1386 cm−1), PO2− (1239 cm−1), C–O–C (1159 cm−1), polysaccharide β-pyranose ring vibration (966 cm−1), asymmetric breathing of polysaccharide pyranose (ring) (923 cm−1), P–O (826 cm−1), symmetric breathing of polysaccharide pyranose (766 cm−1), C–H (724 cm−1), CH2 and N–H (701 cm−1), O–CO (658 and 618 cm−1), and C–CO (528 cm−1) were found. This indicates that the strain increase its resistance to chromium by producing other molecules to adsorb metal ions.
Byssochlamys sp. cells bind Cr6+ and Cr3+ with amide A, CH3, CH2, OH, amide III, PO2−, C–O–C, C–O, C–C, polysaccharide, P–O, N–H, N–H2, O–CO, and C–CO (Ayele et al., 2021; Su et al., 2021; Elahi et al., 2022). It was observed that a peak at 3068 cm−1 indicates the presence of the amide II. In addition, polysaccharide β-pyranose ring vibration (966 cm−1) detected in Cr6+-treated cells suggests that the polysaccharide produced adsorbs Cr in its various forms (Karthik et al., 2017; Ayele et al., 2021; Su et al., 2021; Elahi et al., 2022).
On the other hand, CH3, CH2, , amide I, amide II, OH, PO2−, C–O, C–C, polysaccharide, C–H, P–O, O–CO, and C–CO recorded on the Candida maltosa cell surface reveal their involvement in metal biding (Ayele et al., 2021; Su et al., 2021; Elahi et al., 2022). The Candida maltosa biomass control cells have cell surfaces composed of amide II (3061 cm−1) and (1512 cm−1). In contrast, there was C=O (1748 cm−1) and (979 cm−1) in the biomass treated with Cr6+. The molecules produced during treatment are part of the strategy of resistance to the toxic metal.
The disappearance of certain functional groups observed at different frequencies is thought to be due to the toxic effect of Cr6+ (Karthik et al., 2017). The appearance of new functional groups in chromium-treated cells mean that the strain is setting up a resistance mechanism (Karthik et al., 2017; Princy et al., 2020). These molecules participate in the adsorption of Cr6+ and Cr3+ (Bharagava and Mishra, 2018). According to the results obtained, some functional groups remain after chromium treatment. This phenomenon is in line with certain studies carried out by scientists. Bharagava and Mishra (2018) noted that before and after treatment, there is always an asymmetric stretching of proteins, polysaccharides, lipids, and nucleic acids. Fungal cell surfaces interact with Cr and functional groups on these surfaces include carboxyl (COOH), phosphate (), amine (–NH2), thiol (–SH), and hydroxyl (–OH) groups (García-Hernández et al., 2017). The distinctive peaks observed on the cell surfaces of cells exposed to Cr are common in many scientific papers. This reflects the ability of microbial strains to sequester chromium on their cell surfaces (Jobby et al., 2019; Kalsoom A. et al., 2021; Mat Arisah et al., 2021; Sharma et al., 2022).
Karthik et al. (2017) indicated that several functional groups such as, O–H, C–H, and C–O, are involved in metal-microbial interaction. Bharagava and Mishra (2018) pointed out that Cellulosimicrobium sp. cells sequester Cr on their surface using molecules with specific groups, such as alkene (C=C), carbonyl (C=O), nitro (–NO2), carboxyl (–COOH), amines (–NH2), and sulphonic (–SO3).
It was revealed that amine (N–H), carboxyl (C=O), ether (C–O), alkyl halides (C–Cl, C–Br, and C–I), nitrile (C=N), and alkane (C–H) contribute to the retention of Cr on the cell surface of Morganella morganii (1Ab1) (Princy et al., 2020). Moreover, functional groups, such as O–H, N–H, C–HO, and C–O, were revealed on the Exiguobacterium mexicanum cell wall (Das et al., 2021).
Maurya et al. (2022) found –OH, –CH2, –COOH, C=O, –CH3, –COO−, –SO3H, S=O, C–OH, and C–O groups in B. vallismortis, B. haynesii, and A. aquatilis exposed to Cr6+. In addition, Acinetobacter junii strain b2w was shown to make use of –OH, C–H, C=O, C–N, C–O, and C–N functional groups to bond Cr (Sevak et al., 2023).
Once Cr6+ is bound to cell surfaces, it is reduced to Cr3+, which is less harmful. The microbial biomass can be recovered after centrifugation. The pellet was further washed with 1 mL of 10 mM EDTA solution for desorption of Cr from the cell surfaces. This method of decontamination is environmentally friendly. In addition, the biomass recovered can be used for another experiment in wastewater treatment.
4.4 Zeta potential analysis
Zeta potential analysis shows the different trends of charge present in tested microorganisms in the absence and presence of different hexavalent chromium concentrations. The presence of negative charges on cell surfaces is thought to be due to functional groups and that biosorption of Cr6+ modifies the zeta potential profiles. The attachment of chromium anion to cell surfaces leads to an accumulation of negative charges (Larson et al., 2023). The negative values of the zeta potentials confirm the accumulation of negative charges on the cell surfaces (Al-Jubory et al., 2020; El Malti et al., 2021). This observation suggests that electrostatic interactions play a role in metal adsorption. Modifications to the zeta potentials were observed by Huang et al. (2013), who indicated that the adsorption of cadmium (Cd) is due to electrostatic interactions. The authors found that living and dead Bacillus cereus RC−1 cells exhibit different zeta potentials in the presence of Cd(II). They noted that the negative charges on the surface of the dead cells are higher than those on the living cells. In this study, the negative charges varied from one strain to another. In Bacillus licheniformis, the highest negative charge of about −39.53 ± 1 mV was observed at 100 mg/L Cr6+. In Bacillus megaterium and Candida maltosa, the highest negative charges recorded at 100 mg/L Cr6+ were −30.4 ± 1.51 and −21.93 ± 2.08 mV, respectively. In Byssochlamys sp., the highest negative charges were found at 50 and 100 mg/L Cr6+ of about −20.7 ± 0.98 and −20.53 ± 1.04 mV, respectively. The difference in absolute load values between the control and the Cr6+ concentrations (25 to 800 mg/L) were 15.90, 13.74, 28.90, 15.60, 12.07, and 14.74 for Bacillus licheniformis. The difference in absolute load values between the control and the 25 to 800 mg/L Cr6+ concentrations were 13.47, 12.57, 14.50, 6.43, −0.60, and 1.07 in Bacillus megaterium. Charge differences of 9.35, 11.72, 11.55, 4.72, 7.09, and 3.25 were found at concentration values ranging from 25 to 800 mg/L Cr6+ in Byssochlamys sp. The differences in absolute load values for Cr6+ concentrations ranging from 25 to 800 mg/L were 3.48, 7.58, 11.78, 0.63, 1.14, and 1.98 for Candida maltosa. These results show that the Cr adsorption limit for all strains is 100 mg/L.
In a previous study, Srinath et al. (2002) investigated the ability of Bacillus megaterium to adsorb Cr. They demonstrated that living and dead cells of this strain adsorb 15.7 and 30.7 mg Cr/g dry weight, respectively. In contrast, 23.8 and 39.9 mg Cr/g dry weight were observed as Cr adsorption values in living and dead Bacillus coagulans cells. Other studies have shown that bacteria, yeasts, and filamentous fungi are capable of adsorbing Cr (Quintelas et al., 2008; Ahluwalia, 2014; Dadrasnia et al., 2015; Iram et al., 2015; Chang et al., 2016; Vendruscolo et al., 2017). In addition, Kaduková and Virčíková (2005) showed that biosorption is possible with both living and dead microorganisms with, however, a promoted activity for dead cells. Pseudomonas strains are known to adsorb Cr6+. It has been shown that P. aeruginosa 99, P. stutzeri T3, and P. aeruginosa 78 adsorb 22%, 14%, and 12% of 10 mg/L Cr onto the cell surfaces, respectively (Ozturk et al., 2012). The ability of a fungal consortium composed of Cladosporeum perangustum and Penicillium commune (isolated from tannery wastewater-contaminated soil), Paecilomyces lilacinus, and Fusarium equiseti (isolated from tannery sludge) to detoxify Cr6+-laden wastewater was revealed by Sharma and Malaviya (2016). The fungi were able to remove 100% of the Cr6+, with adsorption as the main mechanism. Singh et al. (2016) proved that Aspergillus flavus adsorb a maximum Cr6+ concentration equivalent to 16.1 mg/g. Bibi et al. (2018) mentioned that endophytic fungi could adsorb Cr6+ and act as fertilizers.
On the other hand, Pseudomonas aeruginosa RW9 was found to bind 0.46 mg/L Cr6+ to the cell surface (Mat Arisah et al., 2021). Other research works corroborate the results obtained in this study, i.e., the potential of fungi and bacteria in sequestering Cr on cell surfaces. Other microorganisms have been demonstrated to serve as effective agents in eliminating toxic metals. Additionally, Anupong et al. (2022) highlighted the ability of Aspergillus flavus DDN to eliminate the Cr contained in water contaminated by mine tailings. This ability was evidenced by a drop in Cr concentration from 3.1 ± 0.35 to 2.3 ± 0.74 mg/L mainly due to adsorption. Extracellular secretions are set up by the cell to prevent Cr from entering the cytoplasm. Samuel et al. (2021) indicated that biosorption depends on temperature, pH, biomass concentration, contact duration, and initial metal concentration. Majumder et al. (2017) showed that Cr6+ biosorption is endothermic and entropy-driven.
5 Conclusion
Adsorption of Cr by Bacillus licheniformis, Bacillus megaterium, Byssochlamys sp., and Candida maltosa strains isolated from tannery effluents was investigated in this study. Scanning electron microscopy combined with energy-dispersive X-ray spectroscopy revealed Cr characteristic peaks in cells treated with Cr6+. At 50 mg/L Cr6+, Bacillus licheniformis and Candida maltosa cells are rough. Extracellular secretions are reduced in Bacillus megaterium, and Byssochlamys sp. cells are tightly bound. Adsorption of Cr by all strains was better at 5 mg/L than at 50 mg/L. At 50 mg/L Cr6+ in LB medium, Cr wt.% of Bacillus licheniformis, Bacillus megaterium, Byssochlamys sp., and Candida maltosa are 0.29, 0.25, 0.35, and 0.23, respectively. FTIR analysis revealed metal-microbial interactions. It was found that phosphoryl terminals contribute to the Cr adsorption mechanism of Bacillus licheniformis while the amino acid and functional groups are involved in interaction of Bacillus megaterium strain with metal. Similarly, amino acid infrared footprint show moderate changes in intensity and frequency, suggesting a possible contribution to the interaction between Byssochlamys sp. strains and metallic ions. The zeta potential analysis showed a variation in charges ranging from −10.63 ± 0.38 to −39.53 ± 1, −15.3 ± 2.18 to −30.4 ± 1.51, −8.98 ± 0.40 to −20.7 ± 0.98, and −10.15 ± 2.68 to −21.93 ± 2.08 for Bacillus licheniformis, Bacillus megaterium, Byssochlamys sp., and Candida maltosa. In addition, the zeta potential results indicated a Cr6+ adsorption limit of 100 mg/L for all strains. Byssochlamys sp. strain adsorb more Cr6+ at a concentration of 50 mg/L (0.35 wt.% Cr). It could therefore be used for the Cr bioremediation of tannery effluents. It is clear that in large-scale experiments, the adsorbed chromium can be recovered after washing the microbial cells, and the microbial biomass can act as a natural adsorbent. This green technology is highly advantageous.
Data availability statement
The datasets presented in this study can be found in online repositories. The names of the repository/repositories and accession number(s) can be found in the article/supplementary material.
Author contributions
AA: Data curation, Methodology, Writing—original draft, Writing—review & editing, Formal analysis. NR: Software, Formal analysis, Writing—original draft. MJ: Formal analysis, Writing—original draft. MH: Supervision, Investigation, Conceptualization, Writing—original draft, Writing—review & editing. YO: Methodology, Investigation, Formal analysis, Writing—original draft. LE: Supervision, Conceptualization, Methodology, Writing—original draft, Writing—review & editing.
Funding
The author(s) declare that no financial support was received for the research, authorship, and/or publication of this article.
Acknowledgments
We acknowledge the Centre d'Analyse et de Caractérisation (CAC) at the Cadi Ayyad Faculty in Marrakech for carrying out the SEM-EDX, FTIR, and zeta potential analyses. The authors would like to thank OCP Africa for its financial support through the project “Rhizobium” funded to Mohammed VI Polytechnic (U6MP).
Conflict of interest
The authors declare that the research was conducted in the absence of any commercial or financial relationships that could be construed as a potential conflict of interest.
Publisher's note
All claims expressed in this article are solely those of the authors and do not necessarily represent those of their affiliated organizations, or those of the publisher, the editors and the reviewers. Any product that may be evaluated in this article, or claim that may be made by its manufacturer, is not guaranteed or endorsed by the publisher.
References
Abo-Alkasem, M. I., Maany, D. A., El-Abd, M. A., and Ibrahim, A. S. S. (2022). Bioreduction of hexavalent chromium by a novel haloalkaliphilic Salipaludibacillus agaradhaerens strain NRC-R isolated from hypersaline soda lakes. 3 Biotech 12:17. doi: 10.1007/s13205-021-03082-2
Aguilar, M. V. M., Sasso, V. M., and Tabaldi, L. A. (2023). Handroanthus heptaphyllus as bioindicator of chromium-contaminated environments. South Afr. J. Bot. 155, 35–44. doi: 10.1016/j.sajb.2023.01.049
Ahluwalia (2014). Microbial removal of hexavalent chromium and scale up potential. Int. J. Curr. Microbiol. App. Sci. 3, 383–398. Available online at: http://www.ijcmas.com
Aké, A. H. J., Hafidi, M., Ouhdouch, Y., and El Fels, L. (2022). Physicochemical characterization and pollutant charge of industrial effluents from tanneries under semi-arid climate. Desalin Water Treat 260, 148–160. doi: 10.5004/dwt.2022.28431
Aké, A. H. J., Hafidi, M., Ouhdouch, Y., Jemo, M., Aziz, S., El Fels, L., et al. (2023). Microorganisms from tannery wastewater: Isolation and screening for potential chromium removal. Environ. Technol. Innov. 31:103167. doi: 10.1016/j.eti.2023.103167
Alharbi, T., Al-Kahtany, K., Nour, H. E., Giacobbe, S., and El-Sorogy, A. S. (2022). Contamination and health risk assessment of arsenic and chromium in coastal sediments of Al-Khobar area, Arabian Gulf, Saudi Arabia. Mar. Pollut. Bull 185:114255. doi: 10.1016/j.marpolbul.2022.114255
Al-Jubory, F. K., Mujtaba, I. M., and Abbas, A. S. (2020). Preparation and characterization of biodegradable crosslinked starch ester as adsorbent. Paper presented at the 2ND IConMEAS 2019:170. doi: 10.1063/5.0000170
Anupong, W., Jutamas, K., On-Uma, R., Alshiekheid, M., Sabour, A., Krishnan, R., et al. (2022). Bioremediation competence of Aspergillus flavus DDN on pond water contaminated by mining activities. Chemosphere 304:135250. doi: 10.1016/j.chemosphere.2022.135250
Aparicio, J. D., Lacalle, R. G., Artetxe, U., Urionabarrenetxea, E., Becerril, J. M., Polti, M. A., et al. (2021). Successful remediation of soils with mixed contamination of chromium and lindane: Integration of biological and physico-chemical strategies. Environ. Res. 194:110666. doi: 10.1016/j.envres.2020.110666
Ariram, N., Pradeep, S., Sundaramoorthy, S., and Madhan, B. (2022). Single pot low float chromium tanning: cleaner pathway approach to environment friendly leather manufacturing. Proc. Safety Environ. Prot. 167, 434–442. doi: 10.1016/j.psep.2022.09.024
Ayele, A., Godeto, Y. G., and Zhang, Y. (2021). Bioremediation of chromium by microorganisms and its mechanisms related to functional groups. J. Chem. 2021, 1–21. doi: 10.1155/2021/7694157
Barkleit, A., Foerstendorf, H., Li, B., Rossberg, A., Moll, H., Bernhard, G., et al. (2011). Coordination of uranium(VI) with functional groups of bacterial lipopolysaccharide studied by EXAFS and FT-IR spectroscopy. The Royal Soc. Chem. 40, 9868–9876. doi: 10.1039/c1dt10546a
Beiranvand, M., Farhadi, S., and Mohammadi-Gholami, A. (2022). Adsorptive removal of tetracycline and ciprofloxacin drugs from water by using a magnetic rod-like hydroxyapatite and MIL-101(Fe) metal-organic framework nanocomposite. RSC Adv. 12, 34438–34453. doi: 10.1039/D2RA06213E
Bharagava, R. N., and Mishra, S. (2018). Hexavalent chromium reduction potential of Cellulosimicrobium sp. isolated from common effluent treatment plant of tannery industries. Ecotoxicol. Environ. Saf. 147, 102–109. doi: 10.1016/j.ecoenv.2017.08.040
Bibi, S., Hussain, A., Hamayun, M., Rahman, H., Iqbal, A., Shah, M., et al. (2018). Bioremediation of hexavalent chromium by endophytic fungi; safe and improved production of Lactuca sativa L. Chemosphere 211, 653–663. doi: 10.1016/j.chemosphere.2018.07.197
Busca, G., and Lorenzelli, V. (1982). Infrared spectroscopic identification of species arising from reactive adsorption of carbon oxides on metal oxide surfaces. Mat. Chem. 7, 89–126. doi: 10.1016/0390-6035(82)90059-1
Cassity, T. R., and Kolodziej, B. J. (1984). Isolation, partial characterization and utilization of a polysaccharide from Bacillus megaterium ATCC 19213. J. Gen. Microbiol. 130, 535–539. doi: 10.1099/00221287-130-3-535
Chaffin, W. L., López-Ribot, J. L., Casanova, M., Gozalbo, D., and Martínez, J. P. (1998). Cell wall and secreted proteins of Candida albicans: identification, function, and expression. Microbiol. Mol. Biol. Rev. 62, 130–180. doi: 10.1128/MMBR.62.1.130-180.1998
Chang, F., Tian, C., Liu, S., and Ni, J. (2016). Discrepant hexavalent chromium tolerance and detoxification by two strains of Trichoderma asperellum with high homology. Chem. Eng. J. 298, 75–81. doi: 10.1016/j.cej.2016.04.023
Chromikova, Z., Chovanova, R. K., Tamindzija, D., Bartova, B., Radnovic, D., Bernier-Latmani, R., et al. (2022). Implantation of Bacillus pseudomycoides chromate transporter increases chromate tolerance in Bacillus subtilis. Front. Microbiol. 13:842623. doi: 10.3389/fmicb.2022.842623
Copíková, J., Cerná, M., Novotná, M., Kaasová, J., and Synytsya, A. (2001). Application of FT-IR spectroscopy in detection of food hydrocolloids in confectionery jellies and food supplements. Czech J. Food Sci. 19, 51–56. doi: 10.17221/6575-CJFS
Dadrasnia, A., Chuan Wei, K. S., Shahsavari, N., Azirun, M. S., and Ismail, S. (2015). Biosorption potential of Bacillus salmalaya strain 139SI for removal of Cr(VI) from aqueous solution. Int. J. Environ. Res. Public Health 12, 15321–15338. doi: 10.3390/ijerph121214985
Das, S., Chandra Behera, B., Mohapatra, R. K., Pradhan, B., Sudarshan, M., Chakraborty, A., et al. (2021). Reduction of hexavalent chromium by Exiguobacterium mexicanum isolated from chromite mines soil. Chemosphere 282:131135. doi: 10.1016/j.chemosphere.2021.131135
Dwivedi, S. K. (2023). Hexavalent chromium (Cr+6) detoxification potential and stress response of fungus Talaromyces pinophillus isolated from tannery wastewater. Environ. Adv. 13:100417. doi: 10.1016/j.envadv.2023.100417
El Malti, W., Hamieh, M., Noaman, A., Nasser El-Dine, R., Hijazi, A., Al-Khatib, W., et al. (2021). Polyurethane loaded with vegetable activated carbon for heavy metals removal from water. J. Ecol. Eng. 22, 99–110. doi: 10.12911/22998993/141362
Elahi, A., Rehman, A., Zajif Hussain, S., Zulfiqar, S., and Shakoori, A. R. (2022). Isolation and characterization of a highly effective bacterium Bacillus cereus b-525k for hexavalent chromium detoxification. Saudi J. Biol. Sci. 29, 2878–2885. doi: 10.1016/j.sjbs.2022.01.027
El-Naggar, N. E., El-Khateeb, A. Y., Ghoniem, A. A., El-Hersh, M. S., and Saber, W. I. A. (2020). Innovative low-cost biosorption process of Cr(6+) by Pseudomonas alcaliphila NEWG-2. Sci. Rep. 10:14043. doi: 10.1038/s41598-020-70473-5
Fernandez, P. M., Vinarta, S. C., Bernal, A. R., Cruz, E. L., and Figueroa, L. I. C. (2018). Bioremediation strategies for chromium removal: current research, scale-up approach and future perspectives. Chemosphere 208, 139–148. doi: 10.1016/j.chemosphere.2018.05.166
Fu, L., Feng, A., Xiao, J., Wu, Q., Ye, Q., Peng, S., et al. (2021). Remediation of soil contaminated with high levels of hexavalent chromium by combined chemical-microbial reduction and stabilization. J. Hazard Mater 403:123847. doi: 10.1016/j.jhazmat.2020.123847
Gang, H., Xiao, C., Xiao, Y., Yan, W., Bai, R., Ding, R., et al. (2019). Proteomic analysis of the reduction and resistance mechanisms of Shewanella oneidensis MR-1 under long-term hexavalent chromium stress. Environ. Int. 127, 94–102. doi: 10.1016/j.envint.2019.03.016
García-Hernández, M. A., Villarreal-Chiu, J. F., and Garza-González, M. T. (2017). Metallophilic fungi research: an alternative for its use in the bioremediation of hexavalent chromium. Int. J. Environ. Sci. Technol. 14, 2023–2038. doi: 10.1007/s13762-017-1348-5
Gow, N. A., van de Veerdonk, F. L., Brown, A. J., and Netea, M. G. (2011). Candida albicans morphogenesis and host defence: discriminating invasion from colonization. Nat. Rev. Microbiol. 10, 112–122. doi: 10.1038/nrmicro2711
Hong, T., Yin, J. Y., Nie, S. P., and Xie, M. Y. (2021). Applications of infrared spectroscopy in polysaccharide structural analysis: progress, challenge and perspective. Food Chem. X 12:100168. doi: 10.1016/j.fochx.2021.100168
Hossan, S., Hossain, S., Islam, M. R., Kabir, M. H., Ali, S., Islam, M. S., et al. (2020). Bioremediation of hexavalent chromium by chromium resistant bacteria reduces phytotoxicity. Int. J. Environ. Res. Public Health 17:6013. doi: 10.3390/ijerph17176013
Huang, F., Dang, Z., Guo, C. L., Lu, G. N., Gu, R. R., Liu, H. J., et al. (2013). Biosorption of Cd(II) by live and dead cells of Bacillus cereus RC-1 isolated from cadmium-contaminated soil. Colloids Surf B Biointerfaces 107, 11–18. doi: 10.1016/j.colsurfb.2013.01.062
Hughes, R. C. (1968). The cell wall of Bacillus licheniformis N.C.T.C. 6346. Composition of the mucopeptide component. Biochem J. 106, 41–48. doi: 10.1042/bj1060041
Iram, S., Shabbir, R., Zafar, H., and Javaid, M. (2015). Biosorption and bioaccumulation of copper and lead by heavy metal-resistant fungal isolates. Arab. J. Sci. Eng. 40, 1867–1873. doi: 10.1007/s13369-015-1702-1
Jobby, R., Jha, P., Gupta, A., Gupte, A., and Desai, N. (2019). Biotransformation of chromium by root nodule bacteria Sinorhizobium sp. SAR1. PLoS ONE 14:e0219387. doi: 10.1371/journal.pone.0219387
Kaduková, J., and Virčíková, E. (2005). Comparison of differences between copper bioaccumulation and biosorption. Environ. Int. 31, 227–232. doi: 10.1016/j.envint.2004.09.020
Kalola, V., and Desai, C. (2020). Biosorption of Cr(VI) by Halomonas sp. DK4, a halotolerant bacterium isolated from chrome electroplating sludge. Environ. Sci. Pollut. Res. Int. 27, 27330–27344. doi: 10.1007/s11356-019-05942-0
Kalsoom, A., Batool, R., and Jamil, N. (2021). Highly Cr(vi)-tolerant Staphylococcus simulans assisting chromate evacuation from tannery effluent. Green Proc. Synth. 10, 295–308. doi: 10.1515/gps-2021-0027
Kalsoom, K., Batool, A., Din, G., Din, S. U., Jamil, J., Hasan, F., et al. (2021). Isolation and screening of chromium resistant bacteria from industrial waste for bioremediation purposes. Braz. J. Biol. 83:e242536. doi: 10.1590/1519-6984.242536
Kapteyn, J. C., Hoyer, L. L., Hecht, J. E., Müller, W. H., Andel, A., Verkleij, A. J., et al. (2000). The cell wall architecture of Candida albicans wild-type cells and cell wall-defective mutants. Mol. Microbiol. 35, 601–611. doi: 10.1046/j.1365-2958.2000.01729.x
Karthik, C., Ramkumar, V. S., Pugazhendhi, A., Gopalakrishnan, K., and Arulselvi, P. I. (2017). Biosorption and biotransformation of Cr(VI) by novel Cellulosimicrobium funkei strain AR6. J Taiwan Inst. Chem. Eng. 70, 282–290. doi: 10.1016/j.jtice.2016.11.006
Lara, P., Vega-Alvarado, L., Sahonero-Canavesi, D. X., Koenen, M., Villanueva, L., Riveros-Mckay, F., et al. (2021). Transcriptome analysis reveals Cr(VI) adaptation mechanisms in klebsiella sp. strain AqSCr. Front. Microbiol. 12:656589. doi: 10.3389/fmicb.2021.656589
Larson, S. L., Ballard, J. H., Runge, K. A., Zhang, H., Breland, B. R., Nick, Z. H., et al. (2023). Effects of aluminum ion on particle sizes and surface charges of exopolysaccharides from Rhizobium tropici and pH effects. Rhizosphere 26:100713. doi: 10.1016/j.rhisph.2023.100713
Lasagabaster, A., Abad, M. J., Barral, L., and Ares, A. (2006). FTIR study on the nature of water sorbed in polypropylene (PP)/ethylene alcohol vinyl (EVOH) films. Eur. Polymer J. 42, 3121–3132. doi: 10.1016/j.eurpolymj.2006.03.029
Li, J., Tang, C., Zhang, M., Fan, C., Guo, D., An, Q., et al. (2021). Exploring the Cr(VI) removal mechanism of Sporosarcina saromensis M52 from a genomic perspective. Ecotoxicol. Environ. Saf. 225:112767. doi: 10.1016/j.ecoenv.2021.112767
Lin, W. H., Chen, S. C., Chien, C. C., Tsang, D. C. W., Lo, K. H., Kao, C. M., et al. (2020). Application of enhanced bioreduction for hexavalent chromium-polluted groundwater cleanup: Microcosm and microbial diversity studies. Environ. Res. 184:109296. doi: 10.1016/j.envres.2020.109296
Majumder, R., Sheikh, L., Naskar, A., Vineeta, M. M., and Tripathy, S. (2017). Depletion of Cr(VI) from aqueous solution by heat dried biomass of a newly isolated fungus Arthrinium malaysianum: a mechanistic approach. Sci. Rep. 7:11254. doi: 10.1038/s41598-017-10160-0
Mat Arisah, F., Amir, A. F., Ramli, N., Ariffin, H., Maeda, T., Hassan, M. A., et al. (2021). Bacterial resistance against heavy metals in Pseudomonas aeruginosa RW9 involving hexavalent chromium removal. Sustainability 13:9797. doi: 10.3390/su13179797
Maurya, A., Kumar, P. S., and Raj, A. (2022). Characterization of biofilm formation and reduction of hexavalent chromium by bacteria isolated from tannery sludge. Chemosphere 286:131795. doi: 10.1016/j.chemosphere.2021.131795
Mohamed, L. A., Aniagor, C. O., and Hashem, A. (2021). Isotherms and kinetic modelling of mycoremediation of hexavalent chromium contaminated wastewater. Cleaner Eng. Technol. 4:100192. doi: 10.1016/j.clet.2021.100192
Mohanty, S., Benya, A., Hota, S., Kumar, M. S., and Singh, S. (2023). Eco-toxicity of hexavalent chromium and its adverse impact on environment and human health in Sukinda Valley of India: a review on pollution and prevention strategies. Environ. Chem. Ecotoxicol. 5, 46–54. doi: 10.1016/j.enceco.2023.01.002
Naumann, D. (2000). Infrared spectroscopy in microbiology. Encycloped. Analyt. Chem. 102:131. doi: 10.1002/9780470027318.a0117
Ozturk, S., Aslim, B., and Suludere, Z. (2009). Evaluation of chromium(VI) removal behaviour by two isolates of Synechocystis sp. in terms of exopolysaccharide (EPS) production and monomer composition. Bioresour. Technol. 100, 5588–5593. doi: 10.1016/j.biortech.2009.06.001
Ozturk, S., Kaya, T., Aslim, B., and Tan, S. (2012). Removal and reduction of chromium by Pseudomonas spp. and their correlation to rhamnolipid production. J. Hazard Mater 232, 64–69. doi: 10.1016/j.jhazmat.2012.06.038
Plestenjak, E., Kraigher, B., Leskovec, S., Mandic Mulec, I., Markovic, S., Scancar, J., et al. (2022). Reduction of hexavalent chromium using bacterial isolates and a microbial community enriched from tannery effluent. Sci. Rep. 12:20197. doi: 10.1038/s41598-022-24797-z
Princy, S., Sathish, S. S., Cibichakravarthy, B., and Prabagaran, S. R. (2020). Hexavalent chromium reduction by Morganella morganii (1Ab1) isolated from tannery effluent contaminated sites of Tamil Nadu, India. Biocat. Agric. Biotechnol. 23:101469. doi: 10.1016/j.bcab.2019.101469
Quintelas, C., Fernandes, B., Castro, J., Figueiredo, H., and Tavares, T. (2008). Biosorption of Cr(VI) by a Bacillus coagulans biofilm supported on granular activated carbon (GAC). Chem. Eng. J. 136, 195–203. doi: 10.1016/j.cej.2007.03.082
Samaké, D. (2008). Traitement des eaux usées de tanneries à l'aide de matériaux à base d'argile. Grenoble: Université Joseph-Fourier - Grenoble I,
Samuel, M. S., Selvarajan, E., Chidambaram, R., Patel, H., and Brindhadevi, K. (2021). Clean approach for chromium removal in aqueous environments and role of nanomaterials in bioremediation: Present research and future perspective. Chemosphere 284:131368. doi: 10.1016/j.chemosphere.2021.131368
Saranya, N., Suganya, E., Narayanasamy, S., Sivaprakasam, S., Pandian, V. S. S., and Selvaraj, R. (2020). 3-level Box–Behnkenoptimization of hexavalent chromium reduction by chromate resistant Trichoderma asperellum cells from simulated and industrial effluent. Environ. Technol. Innov. 19:101024. doi: 10.1016/j.eti.2020.101024
Sathvika, T., Balaji, S., Chandra, M., Soni, A., Rajesh, V., Rajesh, N., et al. (2019). A co-operative endeavor by nitrifying bacteria Nitrosomonas and Zirconium based metal organic framework to remove hexavalent chromium. Chem. Eng. J. 360, 879–889. doi: 10.1016/j.cej.2018.12.015
Schmitt, J., and Flemming, H. C. (1998). FTIR-spectroscopy in microbial and material analysis. Int. Biodeterior. Biodegrad. 41, 1–11. doi: 10.1016/S0964-8305(98)80002-4
Seiferth, O., Wolter, K., Dillmann, B., Klivenyi, G., Freund, H. J., Scarano, D., et al. (1999). IR investigations of CO2 adsorption on chromia surfaces: Cr2O3 (0001)/Cr(110) versus polycrystalline α-Cr2O3. Surf. Sci. 421, 176–190. doi: 10.1016/S0039-6028(98)00857-7
Selvakumar, P., Subramanian, D., Natarajan, J., and Solai Ramatchandirane, P. (2021). Analysis of chromate transporters in bacterial species for Cr(VI) reduction isolated from tannery effluent contaminated site of Dindigul District, Tamil Nadu, India. Geomicrobiol. J. 38, 598–606. doi: 10.1080/01490451.2021.1906359
Sevak, P., Pushkar, B., and Mazumdar, S. (2023). Mechanistic evaluation of chromium bioremediation in Acinetobacter junii strain b2w: a proteomic approach. J. Environ. Manage. 328:116978. doi: 10.1016/j.jenvman.2022.116978
Sharma, P., Singh, S. P., Parakh, S. K., and Tong, Y. W. (2022). Health hazards of hexavalent chromium (Cr (VI)) and its microbial reduction. Bioengineered 13, 4923–4938. doi: 10.1080/21655979.2022.2037273
Sharma, S., and Malaviya, P. (2016). Bioremediation of tannery wastewater by chromium resistant novel fungal consortium. Ecol. Eng. 91, 419–425. doi: 10.1016/j.ecoleng.2016.03.005
Shroff, K. A., and Vaidya, V. K. (2012). Effect of pre-treatments on the biosorption of Chromium (VI) ions by the dead biomass of Rhizopus arrhizus. J. Chem. Technol. Biotechnol. 87, 294–304. doi: 10.1002/jctb.2715
Singh, R., Kumar, M., and Bishnoi, N. R. (2016). Development of biomaterial for chromium(VI) detoxification using Aspergillus flavus system supported with iron. Ecol. Eng. 91, 31–40. doi: 10.1016/j.ecoleng.2016.01.060
Socrates, G. (2001). Infrared and Raman Characteristic Group Frequencies: Tables and Charts, 3rd Edn. New York, NY: John Wiley and Sons Ltd.
Srinath, T., Verma, T., Ramteke, P. W., and Garg, S. K. (2002). Chromium (VI) biosorption and bioaccumulation by chromate resistant bacteria. Chemosphere 48, 427–435. doi: 10.1016/S0045-6535(02)00089-9
Su, Y. Q., Yuan, S., Guo, Y. C., Tan, Y. Y., Mao, H. T., Cao, Y., et al. (2021). Highly efficient and sustainable removal of Cr (VI) in aqueous solutions by photosynthetic bacteria supplemented with phosphor salts. Chemosphere 283:131031. doi: 10.1016/j.chemosphere.2021.131031
Tan, H., Wang, C., Zeng, G., Luo, Y., Li, H., Xu, H., et al. (2020). Bioreduction and biosorption of Cr(VI) by a novel Bacillus sp. CRB-B1 strain. J. Hazard Mater 386:121628. doi: 10.1016/j.jhazmat.2019.121628
Tangahu, B., Berlianto, M., and Kartika, A. A. (2020). Deconcentration of chromium contained in wastewater using a bacteria and microalgae consortia with a high rate algal reactor System. J. Ecol. Eng. 21, 272–284. doi: 10.12911/22998993/126878
Thatoi, H., Das, S., Mishra, J., Rath, B. P., and Das, N. (2014). Bacterial chromate reductase, a potential enzyme for bioremediation of hexavalent chromium: a review. J. Environ. Manage. 146, 383–399. doi: 10.1016/j.jenvman.2014.07.014
Tremmel, S., Beyermann, M., Oschkinat, H., Bienert, M., Naumann, D., Fabian, H., et al. (2005). 13C-labeled tyrosine residues as local IR probes for monitoring conformational changes in peptides and proteins. Angew. Chem. Int. Ed. Engl. 44, 4631–4635. doi: 10.1002/anie.200500547
Tripathi, M., Upadhyay, S. K., Kaur, M., and Kaur, K. (2018). Toxicity concerns of hexavalent chromium from tannery waste. J. Biotechnol. Bioeng. 2, 40–44. doi: 10.22259/2637-5362.0202007
Tumolo, M., Ancona, V., De Paola, D., Losacco, D., Campanale, C., Massarelli, C., et al. (2020). Chromium pollution in european water, sources, health risk, and remediation strategies: an overview. Int. J. Environ. Res. Public Health 17:438. doi: 10.3390/ijerph17155438
Upadhyay, N., Vishwakarma, K., Singh, J., Mishra, M., Kumar, V., Rani, R., et al. (2017). Tolerance and reduction of chromium(VI) by Bacillus sp. MNU16 isolated from contaminated coal mining soil. Front. Plant Sci. 8:778. doi: 10.3389/fpls.2017.00778
Vendruscolo, F., da Rocha Ferreira, G. L., and Antoniosi Filho, N. R. (2017). Biosorption of hexavalent chromium by microorganisms. Int. Biodet. Biodegr. 119, 87–95. doi: 10.1016/j.ibiod.2016.10.008
Venyaminov, S. Y., and Kalnin, N. N. (1990a). Quantitative IR spectrophotometry of peptide compounds in water (H2O) solutions. I. Spectral parameters of amino acid residue absorption bands. Biopolymers 30, 1243–1257. doi: 10.1002/bip.360301309
Venyaminov, S. Y., and Kalnin, N. N. (1990b). Quantitative IR spectrophotometry of peptide compounds in water (H2O) solutions. II. Amide absorption bands of polypeptides and fibrous proteins in α-, β-, and random coil conformations. Biopolymers 30, 1259–1271. doi: 10.1002/bip.360301310
Wang, Q., Song, X., Wei, C., Jin, P., Chen, X., Tang, Z., et al. (2022). In situ remediation of Cr(VI) contaminated groundwater by ZVI-PRB and the corresponding indigenous microbial community responses: a field-scale study. Sci. Total Environ. 805:150260. doi: 10.1016/j.scitotenv.2021.150260
Wang, Y., Liu, Y., Zheng, K., Xie, L., Ren, Q., Yang, Z., et al. (2021). The role of extracellular polymeric substances (EPS) in the reduction of Cr(VI) by Pannonibacter phragmitetus BB. J. Environ. Chem. Eng. 9:106163. doi: 10.1016/j.jece.2021.106163
Wilson, R. H., Smith, A. C., Kačuráková, M., Saunders, P. K., Wellner, N., Waldron, K. W., et al. (2000). The mechanical properties and molecular dynamics of plant cell wall polysaccharides studied by Fourier-transform infrared spectroscopy. Plant Physiol. 124, 397–405. doi: 10.1104/pp.124.1.397
Xia, S., Song, Z., Jeyakumar, P., Shaheen, S. M., Rinklebe, J., Ok, Y. S., et al. (2019). A critical review on bioremediation technologies for Cr(VI)-contaminated soils and wastewater. Crit. Rev. Environ. Sci. Technol. 49, 1027–1078. doi: 10.1080/10643389.2018.1564526
Yasir, M. W., Capozzi, S. L., Kjellerup, B. V., Mahmood, S., Mahmood, T., Khalid, A., et al. (2021). Simultaneous biotreatment of hexavalent chromium Cr(VI) and polychlorinated biphenyls (PCBs) by indigenous bacteria of Co-polluted wastewater. Int. Biodet. Biodegr. 161:105249. doi: 10.1016/j.ibiod.2021.105249
Youssef, M., Morin, A., Aubret, A., Sacanna, S., and Palacci, J. (2020). Rapid characterization of neutral polymer brush with a conventional zetameter and a variable pinch of salt. Soft Matter 16, 4274–4282. doi: 10.1039/C9SM01850F
Zhu, Y., Yan, J., Xia, L., Zhang, X., and Luo, L. (2019). Mechanisms of Cr(VI) reduction by Bacillus sp. CRB-1, a novel Cr(VI)-reducing bacterium isolated from tannery activated sludge. Ecotoxicol. Environ. Saf. 186:109792. doi: 10.1016/j.ecoenv.2019.109792
Keywords: chromium bioremediation, scanning electron microscopy, energy-dispersive X-ray spectroscopy, Fourier transform infrared spectroscopy, zeta potential measurements
Citation: Aké AHJ, Rochdi N, Jemo M, Hafidi M, Ouhdouch Y and El Fels L (2024) Cr(VI) removal performance from wastewater by microflora isolated from tannery effluents in a semi-arid environment: a SEM, EDX, FTIR and zeta potential study. Front. Microbiol. 15:1423741. doi: 10.3389/fmicb.2024.1423741
Received: 26 April 2024; Accepted: 03 June 2024;
Published: 01 July 2024.
Edited by:
Linduo Zhao, University of Illinois at Urbana-Champaign, United StatesReviewed by:
Wei Li, Lawrence Livermore National Laboratory (DOE), United StatesAmrik Bhattacharya, Amity University, India
Copyright © 2024 Aké, Rochdi, Jemo, Hafidi, Ouhdouch and El Fels. This is an open-access article distributed under the terms of the Creative Commons Attribution License (CC BY). The use, distribution or reproduction in other forums is permitted, provided the original author(s) and the copyright owner(s) are credited and that the original publication in this journal is cited, in accordance with accepted academic practice. No use, distribution or reproduction is permitted which does not comply with these terms.
*Correspondence: Loubna El Fels, bG91Ym5hLmVsZmVscyYjeDAwMDQwO3VjYS5hYy5tYQ==; bG91Ym5hLmVsZmVscyYjeDAwMDQwO2dtYWlsLmNvbQ==; Mohamed Hafidi, aGFmaWRpJiN4MDAwNDA7dWNhLmFjLm1h