- 1Key Laboratory of Industrial Biotechnology of the Ministry of Education, School of Biotechnology, Jiangnan University, Wuxi, China
- 2National Engineering Research Center of Cereal Fermentation and Food Biomanufacturing, Jiangnan University, Wuxi, China
The coevolution of bacteria and bacteriophages has created a great diversity of mechanisms by which bacteria fight phage infection, and an equivalent diversity of mechanisms by which phages subvert bacterial immunity. Effective and continuous evolution by phages is necessary to deal with coevolving bacteria. In this study, to better understand the connection between phage genes and host range, we examine the isolation and genomic characterization of two bacteriophages, JNUWH1 and JNUWD, capable of infecting Escherichia coli. Sourced from factory fermentation pollutants, these phages were classified within the Siphoviridae family through TEM and comparative genomic analysis. Notably, the phages exhibited a viral burst size of 500 and 1,000 PFU/cell, with latent periods of 15 and 20 min, respectively. They displayed stability over a pH range of 5 to 10, with optimal activity at 37°C. The complete genomes of JNUWH1 and JNUWD were 44,785 bp and 43,818 bp, respectively. Phylogenetic analysis revealed their close genetic relationship to each other. Antibacterial assays demonstrated the phages’ ability to inhibit E. coli growth for up to 24 h. Finally, through laboratory-driven adaptive evolution, we successfully identified strains for both JNUWH1 and JNUWD with mutations in receptors specifically targeting lipopolysaccharides (LPS) and the lptD gene. Overall, these phages hold promise as additives in fermentation products to counter E. coli, offering potential solutions in the context of evolving bacterial resistance.
1 Introduction
Bacteriophages represent an intriguing avenue in the ongoing battle to control bacterial infections. These viruses, which specifically target bacteria, have long been recognized for their potential in combatting bacterial infections (Chang et al., 2022; Sanmukh et al., 2023). Since their discovery in the early 20th century, phages have garnered increasing attention for their unique ability to infect and lyse bacterial cells (Chen et al., 2023). This natural phenomenon has been the driving force behind endeavors to harness phages as potential therapeutic agents against bacterial pathogens (Sharma et al., 2017). The dynamic co-evolution between bacteria and phages has not only shaped the intricate resistance systems of bacteria but has also propelled the adaptation and diversification of phages (Koonjan et al., 2020; Venturini et al., 2022).
This study delves into the isolation and analysis of two bacteriophages, JNUWH1 and JNUWD, with the capability to infect E. coli. Phages isolated from natural environments often demonstrate low burst sizes and weak inhibitory effects, and they tend to have limited application potential (Chevallereau et al., 2021). However, in fermentation systems, extremely low Multiplicity of Infection (MOI, MOI < 0.001) can lead to system collapse. These two bacteriophages were isolated from collapsed fermentation medium. They exhibit short replication cycles and high burst volume of cleavage, as well as genetic stability in the infection cycle. Therefore, research directed toward these phages holds significant relevance (Tabib-Salazar et al., 2017; Chen et al., 2023; Wei et al., 2023; Xiao et al., 2023; Zhang et al., 2023). Our research aims to illuminate the genetic makeup and taxonomic classification of these phages. Through this exploration, we seek to not only contribute to the broader understanding of phage diversity but also to highlight their potential applications (Wei et al., 2023).
This study stands at the intersection of historical phage research and contemporary applications. Studying phages’ biological and receptors characteristics can help us understand the life process of phages and help solve the huge economic losses caused by phage infection in the fermentation process. In addition, through this work, we strive to contribute to a growing body of knowledge that also has the potential to revolutionize approaches to bacterial control and treatment (Duan et al., 2022), even though the phages in this study have not yet been found to lyse pathogenic microorganisms.
2 Materials and methods
2.1 Phage isolation and purification
The samples were obtained from two synthetic biology products factories in China’s Jiangsu and Hubei provinces that specialize in industrial microbes. The fermentation broth, potentially harboring bacteriophages, underwent centrifugation (10 min at 12,000 g, 4°C) and filtration (through a 0.22 μm syringe filter, Nylon) for enrichment. Subsequently, 300 μL of E. coli BL21 from a 5-h culture and 100 μL of the fermented liquid after filtration were combined in 10 mL LB broth, followed by an overnight incubation at 37°C with agitation (180 rpm). Subsequent validation of phage presence was accomplished through continuous monitoring of OD600 values during the growth of E. coli. The bacteriophage was purified using a double-layer agar method, wherein 100 μL of the bacteriophage suspension, diluted to 103 pfu/ml, was mixed with 300 μL of host bacterial suspension in 5 mL of semi-solid LB medium (containing agar powder at a concentration of 0.5%). This mixture was then poured into a solid LB medium (containing agar powder at a concentration of 1%) and allowed to incubate overnight at 37°C. Following this, a singular plaque containing a purified phage was selected. Individual plaques were carefully picked and introduced into E. coli medium for the purpose of amplification. The resulting lysate underwent a series of centrifugation and filtration processes, culminating in its preservation in buffer at 4°C to ensure optimal stability for subsequent experiments (Luong et al., 2020).
The phages JNUWH1 and JNUWD were subjected to purification for TEM analysis and purified phage lysates were stained with 1% (w/v) uranyl acetate for 2 min prior to examination using a transmission electron microscope (Hitachi H-7650) (Wei et al., 2023).
2.2 Host range determination of phage JNUWH1 and JNUWD
The bacteriostatic effect of phages was assessed against 15 colistin-resistant E. coli strains using an OD-based turbidimetric method (OD600) (Xiao et al., 2023). The cultures were treated with 20 μL of purified phages at 107 PFU/mL, and the control group contained only bacteria. A control (bacteria only) and experimental (bacteria with phage) group were set up for each bacterial strain tested and incubated in a petri dish at 37°C for 8 h, and OD600 readings were taken every 30 min to determine whether the bacteria were lysed by comparing the changes of OD600 in the experimental group compared to the control group. In addition, for the strains whose growth was inhibited, host range was further verified by observing whether plaques formed after dropping bacteriophages on the double-layer plate of E. coli (Chen et al., 2023).
2.3 One-step growth curve of phage JNUWH1 and JNUWD
The one-step growth experiment was carried out according to established protocol with minor modifications (Koonjan et al., 2020). The host strain E. coli BL21 was grown to an early logarithmic stage (OD600 = 0.45–0.55) and diluted with LB broth to 106 CFU/mL. The diluted bacteriophage was mixed with the bacterial culture at MOI 1, incubated at 37°C, and samples were taken every 5 min, and then centrifuged. The supernatant was diluted to an appropriate concentration, and the host E. coli was mixed with phage through a double-layer plate and cultured at 37°C for 6 h. The phage titer was determined by measuring the number of single plaques.
2.4 Biological characteristics of phage JNUWH1 and JNUWD
For thermal stability testing, the method of Chen et al. (2023) was used where phages (106 PFU/mL) were incubated at different temperatures for 60 min. For pH stability, phages (107 PFU/mL) were exposed to varying pH (2–12) at 4°C for 60 min. Phage titers were measured at 60 min using the double-layer agar method.
To calculate the adsorption rate, the strain E. coli to be detected in this study was cultured until reaching the early logarithmic growth phase (OD600 = 0.45–0.55). Subsequently, the bacteriophage was combined with the bacterial culture at a multiplicity of infection (MOI) of 1, followed by incubation at 37°C. The control group (S0) underwent the same procedure using LB medium without bacteria. Samples were collected after 15 min and subsequently centrifuged. The resulting supernatant was further diluted to an appropriate concentration. E. coli BL21 was then mixed with the bacteriophage using a double-layer plate method and incubated at 37°C for 6 h. The phage titer (S) was determined by quantifying the number of single plaques formed. The calculation method for bacteriophage adsorption rate was defined as “1 - S/S0”.
2.5 Genome sequencing and bioinformatic analysis
For next-generation sequencing library preparation, phage genomic DNA (200 μg) was precisely fragmented using Covaris S220 (Duty Factor 10%, Peak/Displayed Power 175 W, Cycles/Burst 200, Duration 50 s, Temperature 4–8°C), resulting in fragments averaging 300-350 bp. Following this, the End Prep Enzyme Mix (#ND608, Vazyme, Nanjing, China) was applied for accurate end-repair, 5′ phosphorylation, and 3′ adenylation, enabling adaptor attachment at both ends. The adaptor-ligated DNA underwent precise size selection with DNA Cleanup beads (#N411, Vazyme, Nanjing, China). Subsequently, each sample underwent 8 cycles of PCR amplification, designed for flowcell annealing, with a primer featuring a six-base index for multiplexing. The PCR products were then thoroughly purified and validated using an Agilent 2,100 Bioanalyzer. The qualified libraries were subjected to pair-end (PE150) sequencing on the Illumina Novaseq 6,000 System. The reads underwent rigorous quality control, followed by assembly using Velvet, with gap-filling conducted through SSPACE (version 3.0) and GapFiller (version 1–10) (Zerbino and Birney, 2008; Zerbino et al., 2009; Boetzer et al., 2011; Boetzer and Pirovano, 2012; Hunt et al., 2014). Gene identification used the software program Prodigal (version 3.02) (Stanke et al., 2006; Tillich et al., 2017), while tRNAs were detected via tRNAscan-SE2.0 with default settings, and other RNA types were identified using Rfam (version 1.1.2). Coding genes were annotated through BLAST against the NCBI nr database, followed by functional annotation using the Gene Ontology (GO) database, and pathway annotation with the Kyoto Encyclopedia of Genes and Genomes (KEGG) database (Ogata et al., 1999; Harris et al., 2004). The alignment between two genomes was conducted using the nucmer algorithm with specific parameters (−-maxgap = 200, −-mincluster = 50), ensuring a maximum gap of 200 base pairs and a minimum of 50 matches per cluster, subsequent data refinement, employing delta-filter with stringent criteria (60% minimum similarity, 500 bp minimum match length), yielded a refined delta file (Kurtz et al., 2004).
vContact2 v0.11.3 has been used to predict species relationships of phages (Jang et al., 2019), and PhageTerm v4.1 software was used to determine the genome ends (Garneau et al., 2017). The evolutionary relationship between the two phages within was investigated by performing a BLASTn1 search against the nr database for the tail fiber protein and terminase large subunit. Identified homologous sequences were then subjected to phylogenetic analysis. Utilizing the Maximum Likelihood method based on the Tamura and Nei (1993), the evolutionary history was inferred. For robust results, we initiated the heuristic search by automatically generating initial trees. This was accomplished by employing both the Neighbor-Join and BioNJ algorithms on a pairwise distance matrix, which was estimated using the Maximum Composite Likelihood (MCL) approach. The topology with the highest log likelihood value was selected. Positions with less than 95% site coverage were excluded, allowing for fewer than 5% alignment gaps, missing data, and ambiguous bases at any position. These evolutionary analyses were conducted using MEGA7 (Kumar et al., 2016). Unless otherwise specified, default parameters were used.
2.6 Laboratory adaptive evolution
By drawing on previous studies using laboratory adaptation, bacteria with phage resistance were evolved by mixing bacteriophages with their host at MOI 1 and cultured them until the OD600 drops, which means that the bacteria were lysed. After the lysate was further cultured for 24 h at 37°C, the single colonies were separated by streaking and the phage resistance was verified by double-plate method, as described in section 2.1 (Huang et al., 2022). To validate the phage-resistant bacterial strains, they were initially cultured in 10 mL of LB liquid medium at 37°C for 8 h. Subsequently, the cultures were transferred to 50 mL of LB liquid medium and incubated overnight under the same conditions. After incubation, the cultures were centrifuged at 8000 rpm for 10 min, and the supernatant was discarded. The resulting bacterial pellet was flash-frozen in liquid nitrogen and sent to GENEWIZ (Suzhou China) for resequencing. The resequencing was conducted according to the protocols specified by the company.
2.7 Strain and plasmid construction
In the process of evolution, we found that some candidate genes that may affect the interaction between bacteriophages and bacteria may stop the phage adsorption process by knockout, and supplementing these knockout genes by plasmids can further prove the necessity of these genes in the phage adsorption process. The method used for gene knockout and integration involved the CRISPR-Cas9 system (Li et al., 2021). Regarding plasmid construction, the amplified target gene sequence and the plasmid vector were each digested with BamHI (Takara, Dalian, China) and HindIII (Takara, Dalian, China) enzymes separately. Following digestion, the linearized plasmid vector and the target gene fragment were ligated together using T4 DNA ligase (Vazyme, Nanjing, China), facilitating the integration of the target gene into the plasmid vector. The constructed plasmid was introduced into corresponding E. coli by chemical transformation method.
3 Result
3.1 Morphological features of JNUWH1 and JNUWD
Bacteriophage JNUWD was isolated and purified from fermentation vessels at a biotechnology company in Jiangsu China that employs E. coli as the microbial host for production. The phage JNUWH1 was obtained from a contaminated fermentation vessel in an academic laboratory environment different from the one where JNUWD was found. The two phages had been repeatedly responsible for fermentation failures due to persistent contamination. Transmission Electron Microscopy (TEM) analysis confirmed the classification of both JNUWD and JNUWH1 as long-tailed lytic bacteriophages. Phage JNUWH1 has a 70 nm diameter head and a 185 nm long tail (Figure 1A) and phage JNUWD has a 50 nm diameter head and a 160 nm long tail (Figure 1B). The two phages exhibit similar bull-eyed plaque morphologies characterized, indicating a high lytic potential. Based on the turbidity of the plaque, both phages have bull-eyed plaques, and these represent depolymerase activity, representing the importance in biofilm removal (Sanmukh et al., 2023; Figure 1C).
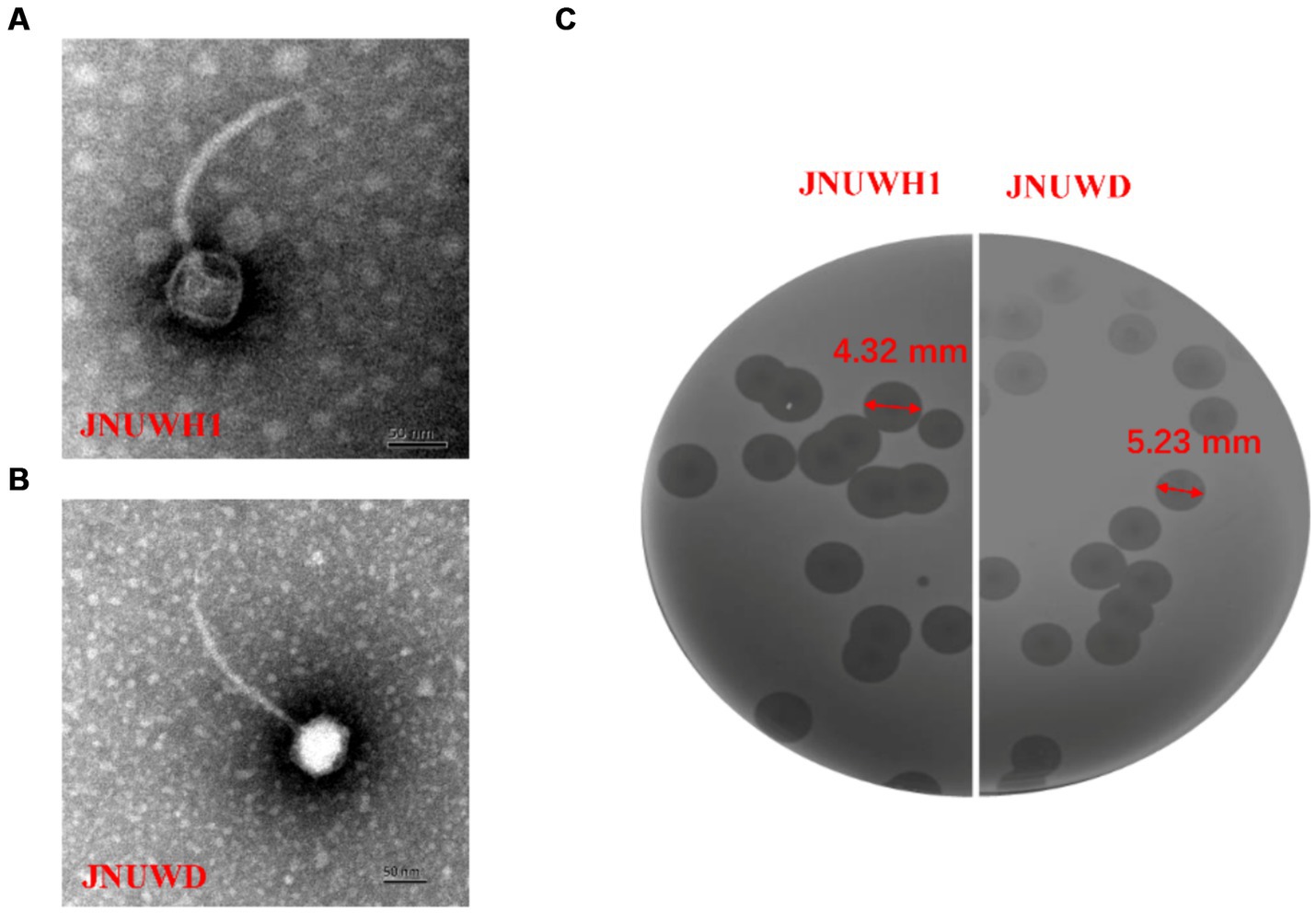
Figure 1. Escherichia phage JNUWH1 and JNUWD. (A) Transmission electron micrograph of phage JNUWH1; (B) transmission electron micrograph of phage JNUWD; (C) plaques formed on the lawn of E. coli BL21.
3.2 Biological characteristics of phage JNUWH1 and JNUWD
In assessing the thermal stability of the two bacteriophages, JNUWH1 demonstrated notable stability at temperatures below 60°C, while for JNUWD, temperatures below 50°C within a one-hour timeframe had a limited impact on phage stability (Figure 2A). Optimal infection temperatures for both bacteriophages were observed at 40°C (Figure 2A). This may be explained by the heightened activity of the host bacteria at this temperature, which provides an optimal environment for the bacteriophage life cycle to progress. In terms of pH sensitivity, both bacteriophages exhibited low stability when subjected to incubation in different buffering solutions for 1 h, particularly at pH levels below 5. Notably, there was a significant reduction in JNUWH1 infectivity observed at pH levels exceeding 11, whereas JNUWD exhibited instability at pH levels as high as 10 (Figure 2B). This disparity may be attributed to the differential pH stability of capsid proteins and receptor-binding proteins associated with the tail fibers, influencing the interaction dynamics of the bacteriophages (Leprince and Mahillon, 2023; Supplementary Figure S1).
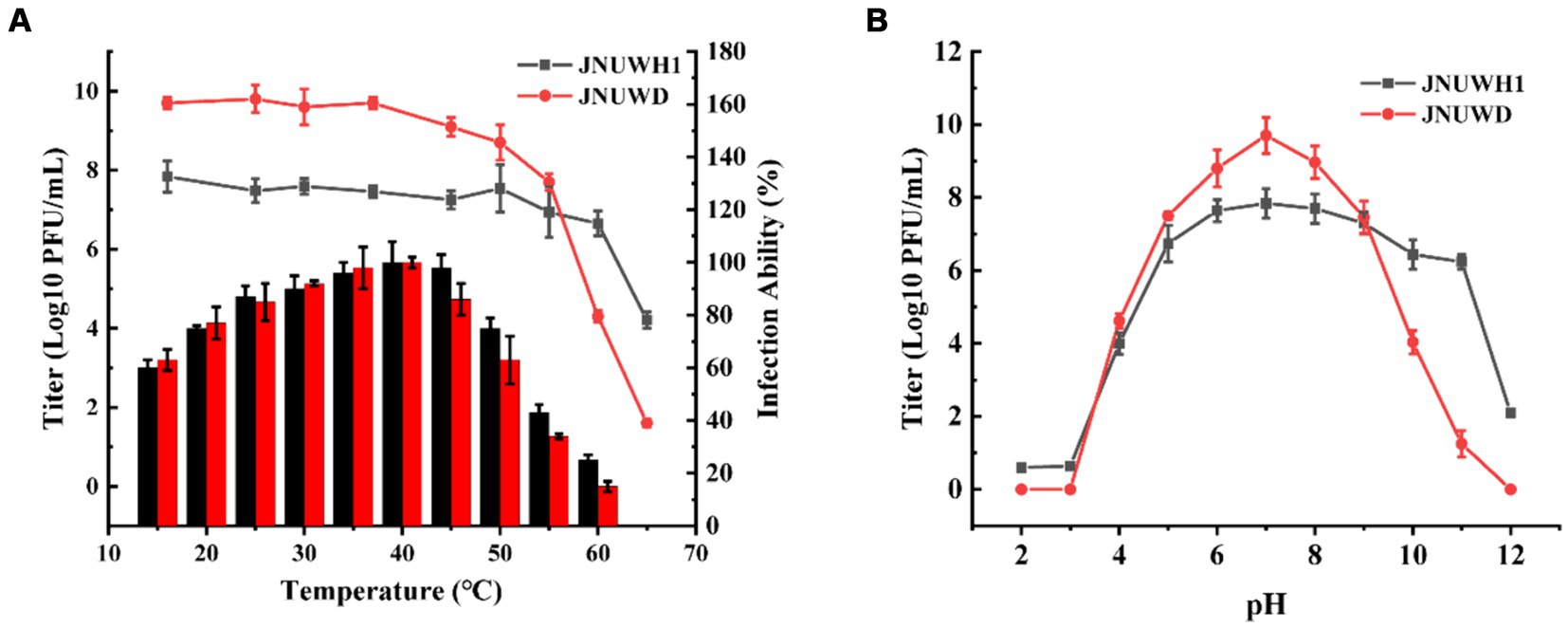
Figure 2. Biological characteristics of phage JNUWH1 and JNUWD. (A) Thermostability and Optimal infection temperature of phage JNUWH1 and JNUWD, the line graph represents the temperature stability, and the bar graph represents the optimal infection temperature. (B) pH stability of JNUWH1 and JNUWD. The error bars indicate the standard deviation. The result was calculated based on three repeated experiments.
3.3 One step curve and lysis spectrum of phages
It was observed that both bacteriophages exhibited efficacy across a spectrum of MOI levels (Figures 3A,B). Under MOIs of 0.01, 0.1, 1.0, and 10, the growth of E. coli BL21 gradually declined within a timeframe of 40–60 min. After 24 h there was no proliferation of the host bacteria in the cultures. The one-step growth curves of E. coli indicate that the latent periods for JNUWH1 and JNUWD are 20 and 15 min, respectively, with corresponding burst periods of 55 and 40 min and the viral burst size of the phages were 500 and 1,000 PFU/cell. The final titers of both bacteriophages exceeded 109 pfu/mL (Figure 3C). These data underscore the potent inhibitory capacity of these two virulent bacteriophages toward E. coli growth. Consequently, in industrial fermentation processes, proactive measures are imperative to prevent and mitigate the impact of such bacteriophages.
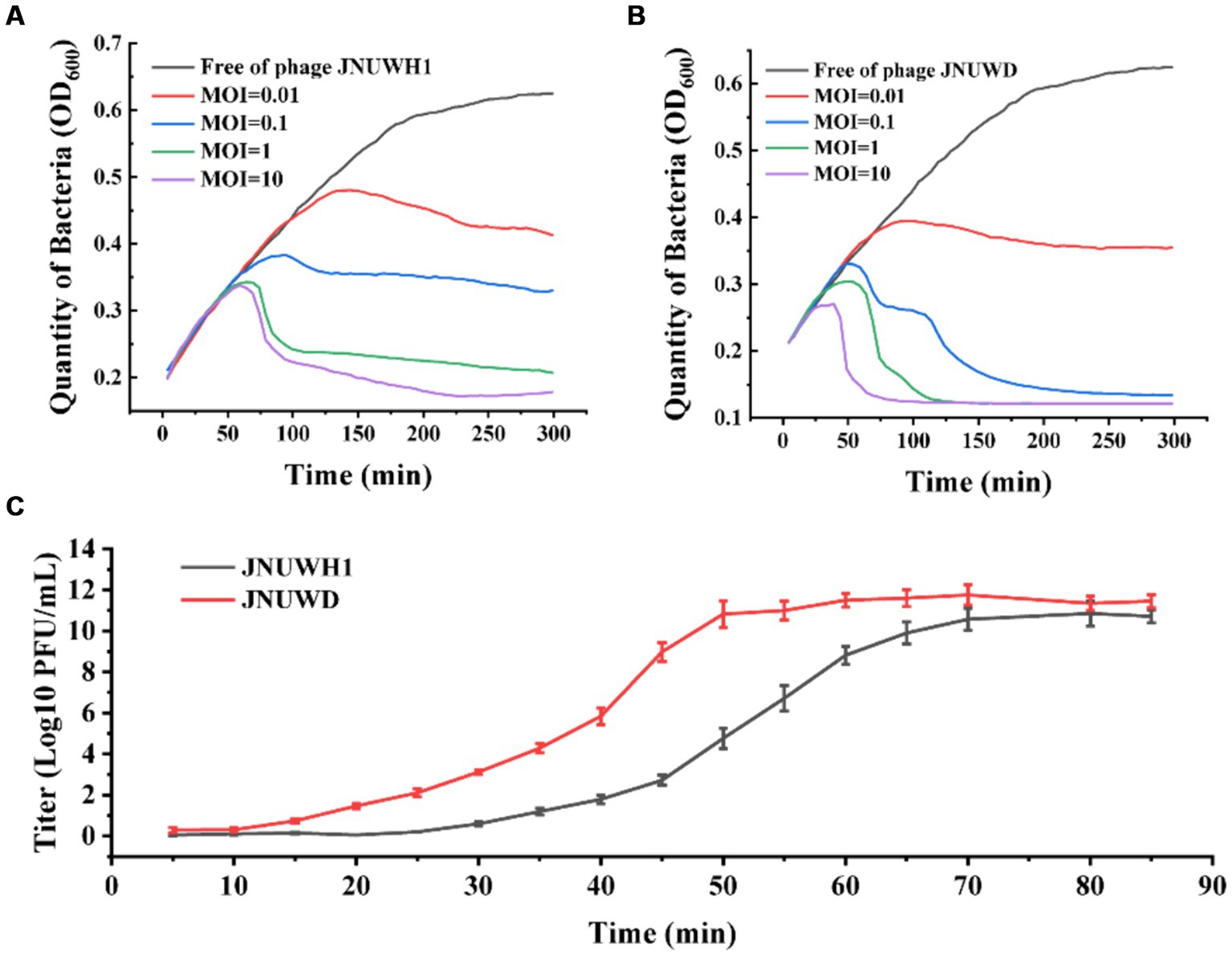
Figure 3. The infection curve and the one-step growth curves of JNUWH1 and JNUWD. (A) The horizontal axis represents the coincubation time of JNUWH1 at different MOIs. (B) The horizontal axis represents the coincubation time of JNUWD at different MOIs (C) One step growth curves of JNUWH1 and JNUWD in the presence of the E. coli BL21 host. The error bars indicate standard deviation. The result was calculated based on three repeated experiments.
We evaluated the host range of the two bacteriophages against fifteen strains of E. coli from diverse sources. Measurements of OD600 indicated that bacteriophage JNUWH1 and JNUWD demonstrated inhibitory activity only against three specific strains of E. coli (Table 1). The two types of bacteriophages lacked infectivity against the tested pathogenic E. coli (Supplementary Table S1).
3.4 Genomic characteristics and taxonomy
The genome of JNUWH1 (NCBI accession No. OR544901) is 44,051 bp in length, with the GC content of 43.67%. Bioinformatic analysis identified 68 open reading frames (ORFs), with 35 hypothetical proteins of unknown functions (Figure 4A). The genome of JNUWD (NCBI accession No. OR544902) spans 43,278 bp, exhibiting the GC content of 43.87%. The phage genome encompasses 66 predicted ORFs, harboring genes responsible for various biological functions (Figure 4B), with 35 ORFs annotated as hypothetical proteins. The substantial proportion of putative ORFs with no assigned function underscores the importance of future research to uncover the roles of these hypothetical proteins. The two genomes contain many genes encoding proteins involved in phage cleavage cycle, e.g., helicase and ssDNA binding protein, and proteins that may be involved in the bacteriophage resistance to bacteria, e.g., endonuclease, exonuclease, and methyltransferase. PhageTerm analysis reveals these phages’ genome ends have direct terminal repeats. Based on tRNA prediction programs, it was revealed in the JNUWH1 and JNUWD genome, sequences encoding tRNAs with CCT anticodons were identified.
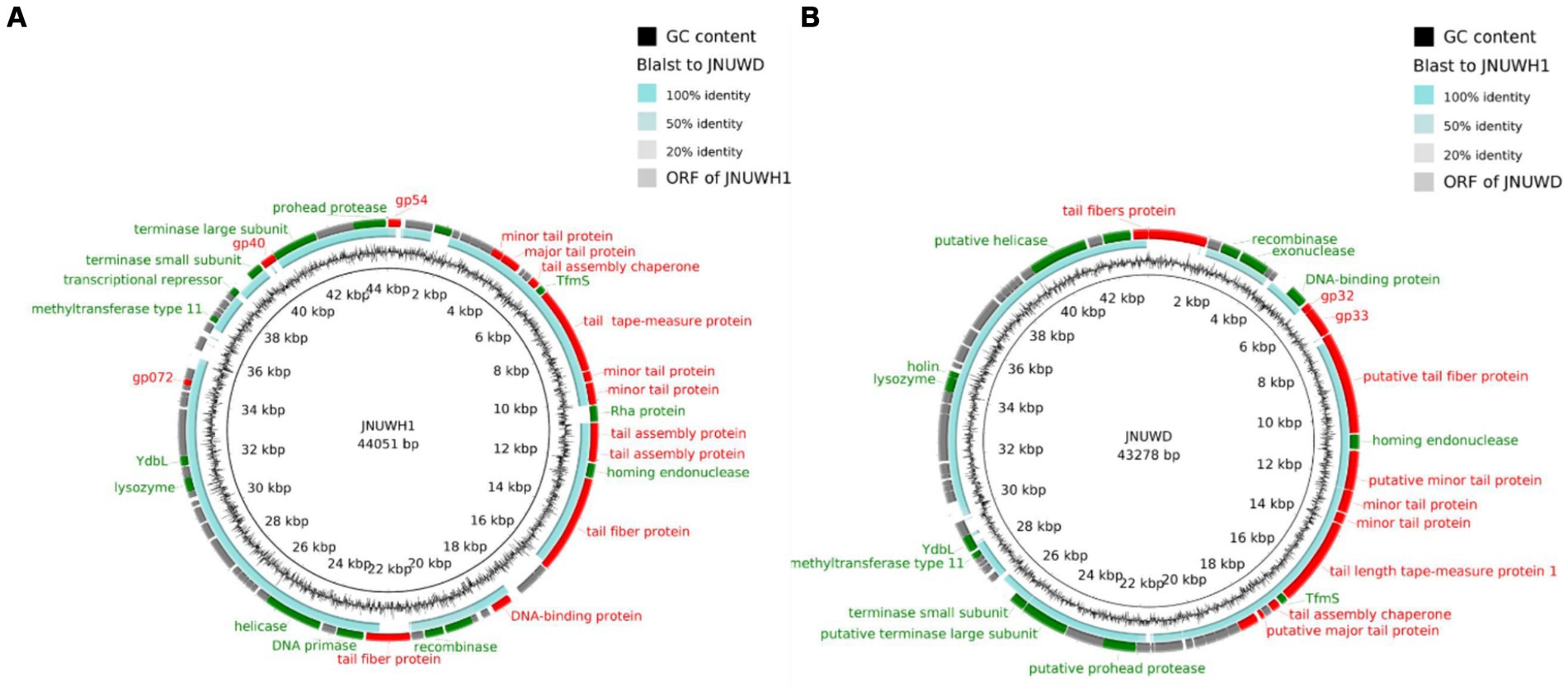
Figure 4. (A) Genome map and functional protein identity of phage JNUWH1. (B) Genome map and functional protein identity of phage JNUWD. The arrows indicate the direction of transcription of each gene. Structurally related ORFs are marked in red, DNA-or enzyme-related ORFs are marked in green, and hypothetical proteins are marked in gray.
3.5 Phylogenetic and comparative genomic analysis
The genomes of bacteriophages JNUWH1 and JNUWD have 97.28% nucleic acid sequence similarity. Notably, 10 highly homologous sequences were identified and anchored, with structural genes, including tail fiber proteins, exhibiting remarkable similarity exceeding 95% (Supplementary Table S2). This comprehensive analysis sheds light on the evolutionary relationship and shared genetic elements between JNUWH1 and JNUWD, offering valuable insights into their biological implications and potential applications. Through vContact2 analysis, both investigated bacteriophages have been identified as members of the viral family Siphoviridae, consistent with the results obtained from TEM data. This taxonomic assignment is supported by shared genomic characteristics, conserved gene content, and phylogenetic proximity to known Siphoviridae family members (Brussow and Desiere, 2001).
The large terminase subunit protein exhibits relatively high conservation among the close relatives of JNUWD1 and JNUWH. For instance, the large terminase protein of Escherichia phage CY1_Cui-2023 (accession # OQ357823) shares 99.62 and 99.94% identical DNA sequences with those of JNUWD1 and JNUWH, respectively (Figure 5A). Even when compared to the distantly related large terminase phage proteins in the analysis, which share 93.46% identical residues with phage vB_EcoD_SU57 (Koonjan et al., 2020) (accession # MT511058) in comparison to both JNUWD1 and JNUWH, the level of similarity remains high. The large tail fiber protein, encoded by the genomes of JNUWD and JNUWH1, also shows surprising similarity among the cluster of phages most closely related to JNUWD and JNUWH1, with 89.91% identical DNA sequences. The highest similarity to JNUWD’s tail fiber protein DNA sequence is observed in Escherichia phage ZL19 (accession # OM258170.1), reaching 89.26%. Similarly, the highest similarity to JNUWH1’s tail fiber protein DNA sequence is found in Escherichia phage vB_EcoS-IME253 (accession # NC_047810.1), with a similarity of 94.07%. However, proteins from the more distantly related group of phages are not particularly similar (Figure 5B). For instance, phage vB_EcoD_SU57’s tail fiber (accession # MT511058) shares only 86.49 and 85.87% identity, with significant differences observed in the C-terminal end. It is noteworthy that the observed differences in tail fiber proteins between JNUWD and JNUWH1 are primarily concentrated at the C-terminal end. Despite their high relatedness at the genomic level, these differences suggest the potential for variations in host cell surface receptor specificity.
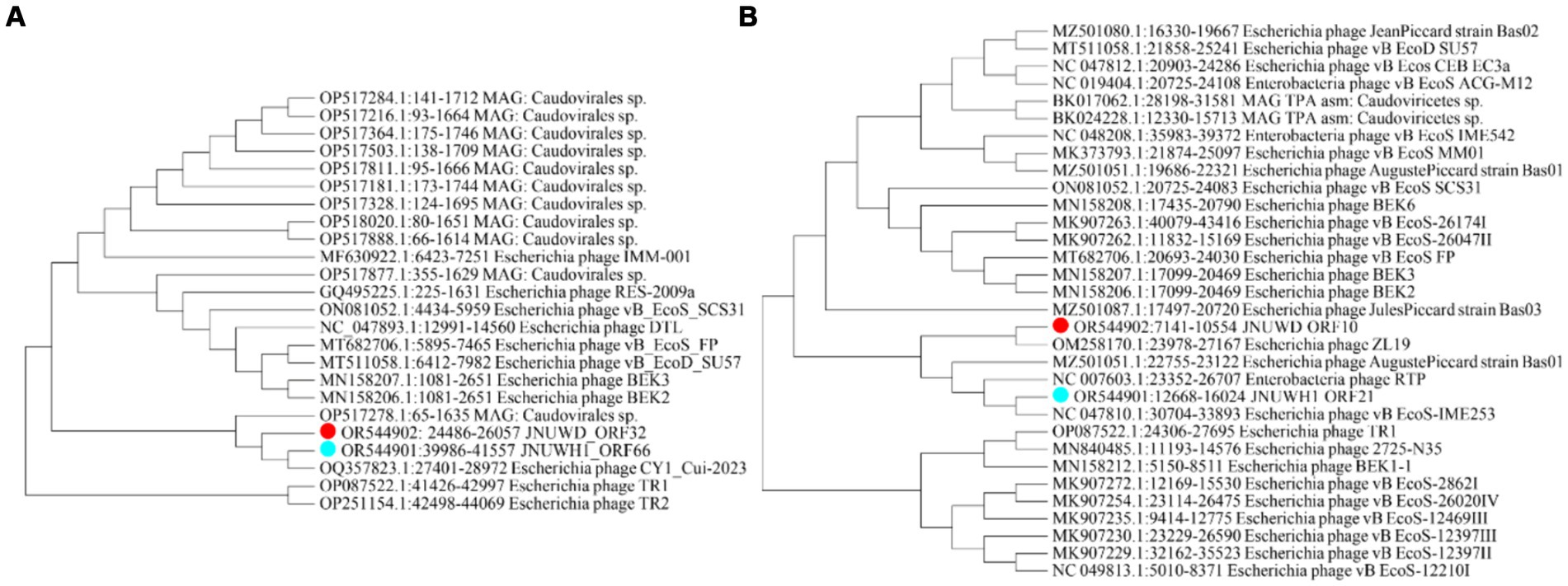
Figure 5. Molecular Phylogenetic analysis by Maximum Likelihood method. (A) Phylogenetic tree based on sequence of terminase large subunit. (B) Phylogenetic tree based on sequence of tail fiber protein.
3.6 Identification and confirmation of phage receptor-related genes
We sought to replicate natural evolutionary processes through co-cultivation of host E. coli and bacteriophages (Farquharson et al., 2021). This method was employed to selectively screen for phage receptor-associated proteins. Following comprehensive genomic resequencing, we established that the mutations in rfaD, waaG, and rfaQ conferred resistance to the JNUWH1 bacteriophage in the host E. coli. Notably, the genes rfaD, waaG, rfaQ are integral to lipopolysaccharide (LPS) biosynthesis, transfer, and assembly (Heinrichs et al., 1998; Washizaki et al., 2016). This substantiates that JNUWH1 recognizes LPS as its primary receptor. To further corroborate this finding, we conducted tests assessing the adsorption rate of the waaG-deficient, rfaD-deficient, and rfaQ-deficient strain (E. coli BL21 ΔwaaG, E. coli BL21 ΔrfaD, E. coli BL21 ΔrfaQ) against the JNUWH1 bacteriophage. The LPS deficient strain exhibited a resistance to JNUWH1, with an adsorption rate of 12.4–16.1%, in contrast to the wild-type E. coli BL21 which demonstrated an adsorption rate of 94.2% (Figure 6A). When the wild type genes in the mutant strain were complemented using pET-28a (pET28awaaG, pET28a rfaD, pET28arfaQ), it was observed that the strain regained sensitivity to the JNUWH1 bacteriophage. This underscores the inhibitory effect of LPS absence on the adhesion process during the invasion phase of JNUWH1.
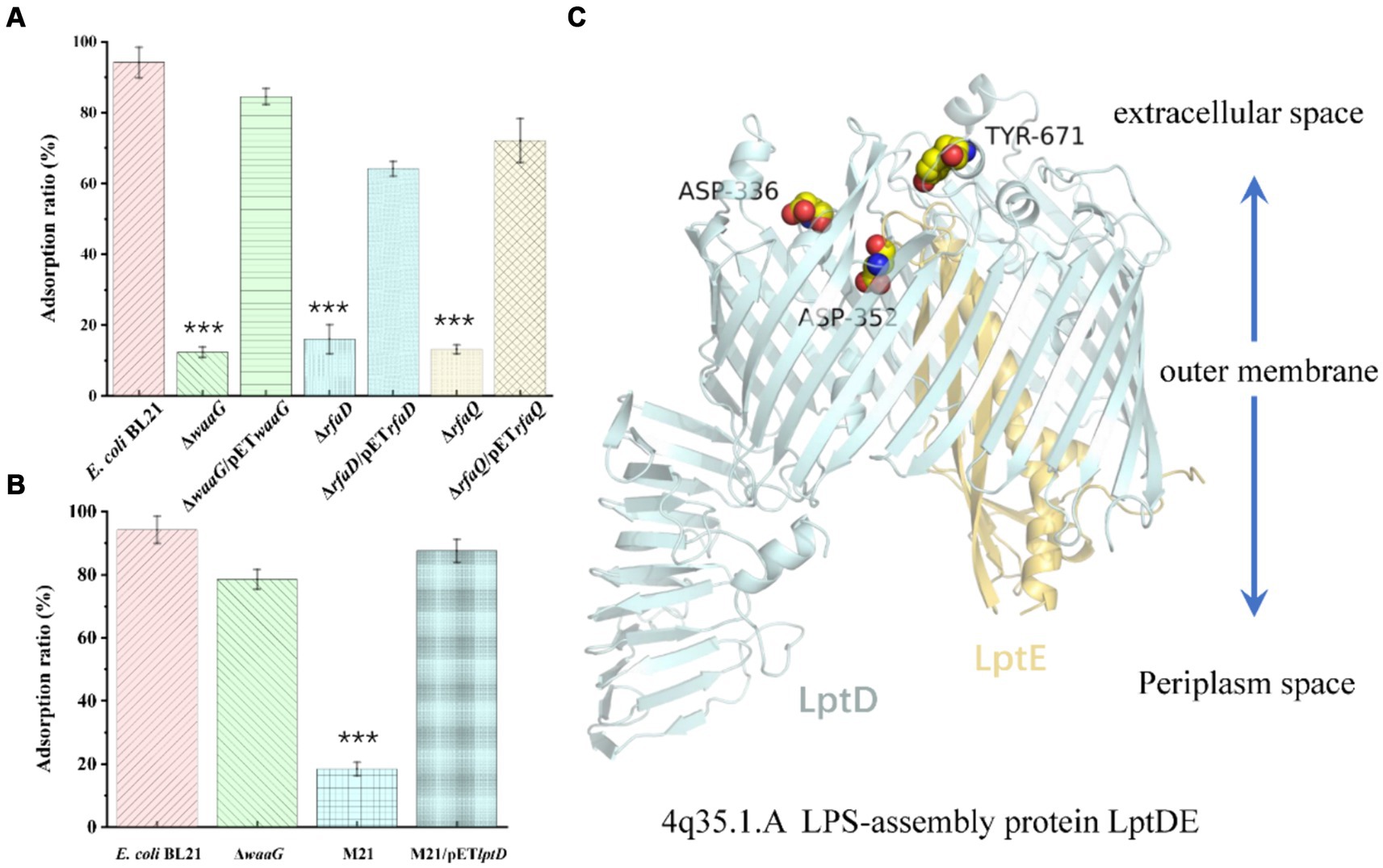
Figure 6. (A) Adsorption properties of phage JNUWH1 (B) Adsorption properties of phage JNUWD. (C) The site of mutation in the lptDE of mutant M21. The error bars indicate standard deviation. ***, p < 0.001. The result was calculated based on three repeated experiments.
Upon evolving E. coli to resist the JNUWD bacteriophage, we made an intriguing observation. Mutations in the lptD protein, which is involved in a variety of biological processes, including organic solvent tolerance, hydrophobic antibiotic resistance and membrane permeability (Frisinger et al., 2023), specifically Y671D/D336Y/D352Y, conferred resistance to the phage JNUWD. However, upon utilizing the waaG-deficient strain to ascertain whether LPS serves as the receptor for JNUWD, results were negative. Therefore, we hypothesized that the extracellular portion of LPS exposed does not act as a recognition receptor for JNUWD (Nakao et al., 2012; Washizaki et al., 2016). Upon evaluating the adsorption rate of the naturally evolved strain M21, we observed that mutations in the gene lptD, pertinent to outer membrane structure (Li et al., 2015; Skurnik et al., 2020), led to an adsorption rate of 18.4% (Figure 6B). We attempted to construct an lptD-deficient strain, however, the deletion of lptD led to growth limitations in E. coli (Frisinger et al., 2023), rendering successful knockout unattainable. Consequently, Therefore, we expressed the wild-type lptD gene by pET28a plasmid in the lab-evolved resistant strain M21. Our research revealed that the complemented strain exhibited restored sensitivity to the bacteriophage JNUWD, indicating a successful recovery of bacteriophage susceptibility. This supports our hypothesis that lptD plays a pivotal role as the receptor-associated gene for the JNUWD bacteriophage. According to the reported crystal structure of lptD (Protein Data Bank with the accession code 4Q35)(Qiao et al., 2014), the mutated points (Figure 6C) are positioned in regions of the lptD protein that are exposed to the extracellular space. This finding provides an explanation of protein structure that the mutations at these three points enable M21 to evade recognition by JNUWD.
4 Discussion
This study presents a comprehensive exploration of the genomic and biological characteristics of bacteriophages JNUWH1 and JNUWD. Their remarkable 97.28% homology, despite being isolated separately from distinct regions and at different times, raises intriguing questions about the convergence of phage evolution. This suggests a dynamic interplay between these phages and their bacterial hosts, driving the adaptation and evolution of phages in response to evolving bacterial resistance (Qiao et al., 2014). Their robust lytic activity and prolonged inhibition of bacteria hold great promise in clinical contexts (Chevallereau et al., 2021), especially in scenarios where traditional antibiotics face challenges.
Through advanced genomic techniques, we uncovered a high degree of extensive genomic modularity (Smug et al., 2023), exemplified by the identification of highly homologous sequences and the conservation of crucial structural genes. Notably, tail fiber proteins exhibited an exceptional degree of sequence similarity exceeding 95%, underscoring their functional significance in phage-host interactions.
From a taxonomic perspective, both phages were confidently classified within the Siphoviridae family, aligning with their shared genomic characteristics. Additionally, their biological traits, including thermal and pH stability, host range, and one-step growth curves, provide valuable insights into their potential applications in various industrial and clinical contexts. From our findings, it is evident that the isolated bacteriophages have a limited host range. Therefore, according to previous reports, replacing the cauda protein can expand or change the specific host selection, and in view of the potential application of JNUWH and JNUWD in anti-infection, we are inclined to explore ways to expand the host range, and possibly develop super phages with wider clinical applications (Chen et al., 2017).
In conclusion, phage convergence, evidenced by their high degree of homology despite their different origins, reveals the complex evolutionary dynamics of phage immunity to bacteriophage resistance. This study not only advances our understanding of phage biology but also highlights their potential as versatile tools in combating bacterial infections. The genetic flexibility observed at the molecular level opens new avenues for exploring phage-host interactions and their applications in biotechnology and medicine. In situations where conventional antibiotics encounter challenges, particularly in scenarios requiring novel therapeutic approaches, the screening of bacteriophages for clinical utility frequently reveals instances where phages targeting specific antibiotic-resistant bacteria exhibit inadequate inhibitory capabilities. Nonetheless, the robust lytic activity and prolonged inhibition of bacteria observed in phages also indicate their considerable clinical promise. Diversifying the host range of these phages exhibiting potent lytic activity presents a unique strategy in the advancement of clinical antibacterial phage therapy. This discovery lays the foundation for further investigation aimed at maximizing the clinical potential of these remarkable bacteriophages.
Data availability statement
The datasets presented in this study can be found in online repositories. The names of the repository/repositories and accession number(s) can be found at: https://www.ncbi.nlm.nih.gov/genbank/, No. OR544901 https://www.ncbi.nlm.nih.gov/genbank/, No. OR544902.
Author contributions
HZ: Conceptualization, Data curation, Formal analysis, Investigation, Methodology, Project administration, Software, Supervision, Validation, Visualization, Writing – original draft, Writing – review & editing. JY: Conceptualization, Formal analysis, Supervision, Validation, Visualization, Writing – review & editing. XP: Conceptualization, Data curation, Formal analysis, Methodology, Resources, Supervision, Writing – review & editing. YH: Data curation, Investigation, Methodology, Visualization, Writing – review & editing. ZZ: Conceptualization, Data curation, Software, Validation, Writing – review & editing. XZ: Conceptualization, Data curation, Formal analysis, Funding acquisition, Investigation, Project administration, Resources, Supervision, Visualization, Writing – review & editing. WZ: Conceptualization, Formal analysis, Project administration, Resources, Visualization, Writing – review & editing. ZR: Conceptualization, Funding acquisition, Project administration, Resources, Supervision, Visualization, Writing – review & editing.
Funding
The author(s) declare that financial support was received for the research, authorship, and/or publication of this article. This work was supported by grants from the National Natural Science Foundation of China (No. 32071470), the Postgraduate Research and Practice Innovation Program of Jiangsu Province (KYCX23_2501), and the Project Funded by the Priority Academic Program Development of Jiangsu Higher Education Institutions, Top-notch Academic Programs Project of Jiangsu Higher Education Institutions.
Conflict of interest
The authors declare that the research was conducted in the absence of any commercial or financial relationships that could be construed as a potential conflict of interest.
The author(s) declared that they were an editorial board member of Frontiers, at the time of submission. This had no impact on the peer review process and the final decision.
Publisher’s note
All claims expressed in this article are solely those of the authors and do not necessarily represent those of their affiliated organizations, or those of the publisher, the editors and the reviewers. Any product that may be evaluated in this article, or claim that may be made by its manufacturer, is not guaranteed or endorsed by the publisher.
Supplementary material
The Supplementary material for this article can be found online at: https://www.frontiersin.org/articles/10.3389/fmicb.2024.1407039/full#supplementary-material
Footnotes
References
Boetzer, M., Henkel, C. V., Jansen, H. J., Butler, D., and Pirovano, W. (2011). Scaffolding pre-assembled contigs using SSPACE. Bioinformatics 27, 578–579. doi: 10.1093/bioinformatics/btq683
Boetzer, M., and Pirovano, W. (2012). Toward almost closed genomes with GapFiller. Genome Biol. 13:R56. doi: 10.1186/gb-2012-13-6-r56
Brussow, H., and Desiere, F. (2001). Comparative phage genomics and the evolution of Siphoviridae: insights from dairy phages. Mol. Microbiol. 39, 213–223. doi: 10.1046/j.1365-2958.2001.02228.x
Chang, R. Y. K., Nang, S. C., Chan, H. K., and Li, J. (2022). Novel antimicrobial agents for combating antibiotic-resistant bacteria. Adv. Drug Deliv. Rev. 187:114378. doi: 10.1016/j.addr.2022.114378
Chen, M., Zhang, L., Abdelgader, S. A., Yu, L., Xu, J., Yao, H., et al. (2017). Alterations in gp37 expand the host range of a T4-like phage. Appl. Environ. Microbiol. 83:17. doi: 10.1128/aem.01576-17
Chen, Q., Dong, Z., Ding, T., Yang, Q., Liu, C., Yin, F., et al. (2023). Isolation and characterization of a novel Enterococcus phage Phi_Eg_SY1. Virus Res. 332:199132. doi: 10.1016/j.virusres.2023.199132
Chevallereau, A., Pons, B. J., van Houte, S., and Westra, E. R. (2021). Interactions between bacterial and phage communities in natural environments. Nat. Rev. Microbiol. 20, 49–62. doi: 10.1038/s41579-021-00602-y
Duan, Y., Young, R., and Schnabl, B. (2022). Bacteriophages and their potential for treatment of gastrointestinal diseases. Nat. Rev. Gastroenterol. Hepatol. 19, 135–144. doi: 10.1038/s41575-021-00536-z
Farquharson, E. L., Lightbown, A., Pulkkinen, E., Russell, T., Werner, B., and Nugen, S. R. (2021). Evaluating phage tail Fiber receptor-binding proteins using a luminescent flow-through 96-well plate assay. Front. Microbiol. 12:304. doi: 10.3389/fmicb.2021.741304
Frisinger, F. S., Jana, B., Ortiz-Marquez, J. C., van Opijnen, T., Donadio, S., and Guardabassi, L. (2023). LptD depletion disrupts morphological homeostasis and upregulates carbohydrate metabolism in Escherichia coli. FEMS Microbes 4:13. doi: 10.1093/femsmc/xtad013
Garneau, J. R., Depardieu, F., Fortier, L. C., Bikard, D., and Monot, M. (2017). PhageTerm: a tool for fast and accurate determination of phage termini and packaging mechanism using next-generation sequencing data. Sci. Rep. 7:8292. doi: 10.1038/s41598-017-07910-5
Harris, M. A., Clark, J., Ireland, A., Lomax, J., Ashburner, M., Foulger, R., et al. (2004). The gene ontology (GO) database and informatics resource. Nucleic Acids Res. 32, 258D–2261D. doi: 10.1093/nar/gkh036
Heinrichs, D. E., Yethon, J. A., and Whitfield, C. (1998). Molecular basis for structural diversity in the core regions of the lipopolysaccharides ofEscherichia coliand Salmonella enterica. Mol. Microbiol. 30, 221–232. doi: 10.1046/j.1365-2958.1998.01063.x
Huang, L., Guo, W., Lu, J., Pan, W., Song, F., and Wang, P. (2022). Enterococcus faecalis bacteriophage vB_EfaS_efap05-1 targets the surface polysaccharide and com EA protein as the receptors. Front. Microbiol. 13:866382. doi: 10.3389/fmicb.2022.866382
Hunt, M., Newbold, C., Berriman, M., and Otto, T. D. (2014). A comprehensive evaluation of assembly scaffolding tools. Genome Biol. 15:R42. doi: 10.1186/gb-2014-15-3-r42
Jang, H. B., Bolduc, B., Zablocki, O., Kuhn, J. H., Roux, S., Adriaenssens, E. M., et al. (2019). Taxonomic assignment of uncultivated prokaryotic virus genomes is enabled by gene-sharing networks. Nat. Biotechnol. 37:632. doi: 10.1038/s41587-019-0100-8
Koonjan, S., Seijsing, F., Cooper, C. J., and Nilsson, A. S. (2020). Infection kinetics and phylogenetic analysis of vB_EcoD_SU57, a virulent T1-like Drexlerviridae Coliphage. Front. Microbiol. 11:565556. doi: 10.3389/fmicb.2020.565556
Kumar, S., Stecher, G., and Tamura, K. (2016). MEGA7: molecular evolutionary genetics analysis version 7.0 for bigger datasets. Mol. Biol. Evol. 33, 1870–1874. doi: 10.1093/molbev/msw054
Kurtz, S., Phillippy, A., Delcher, A. L., Smoot, M., Shumway, M., Antonescu, C., et al. (2004). Versatile and open software for comparing large genomes. Genome Biol. 5:R12. doi: 10.1186/gb-2004-5-2-r12
Leprince, A., and Mahillon, J. (2023). Phage adsorption to gram-positive Bacteria. Viruses-Basel 15:196. doi: 10.3390/v15010196
Li, Q. I., Sun, B., Chen, J., Zhang, Y., Jiang, Y. U., and Yang, S. (2021). A modified pCas/pTargetF system for CRISPR-Cas9-assisted genome editing in Escherichia coli. Acta Biochim. Biophys. Sin. 53, 620–627. doi: 10.1093/abbs/gmab036
Li, X., Gu, Y., Dong, H., Wang, W., and Dong, C. (2015). Trapped lipopolysaccharide and LptD intermediates reveal lipopolysaccharide translocation steps across the Escherichia coli outer membrane. Sci. Rep. 5:1883. doi: 10.1038/srep11883
Luong, T., Salabarria, A. C., Edwards, R. A., and Roach, D. R. (2020). Standardized bacteriophage purification for personalized phage therapy. Nat. Protoc. 15:2867–2890. doi: 10.1038/s41596-020-0346-0
Nakao, R., Ramstedt, M., Wai, S. N., and Uhlin, B. E. Enhanced biofilm formation by Escherichia coli LPS mutants defective in Hep biosynthesis. PLoS One 7:1241. doi: 10.1371/journal.pone.0051241
Ogata, H., Goto, S., Sato, K., Fujibuchi, W., Bono, H., and Kanehisa, M. (1999). KEGG: Kyoto encyclopedia of genes and genomes. Nucleic Acids Res. 27, 29–34. doi: 10.1093/nar/27.1.29
Qiao, S., Luo, Q., Zhao, Y., Zhang, X. C., and Huang, Y. (2014). Structural basis for lipopolysaccharide insertion in the bacterial outer membrane. Nature 511, 108–111. doi: 10.1038/nature13484
Sanmukh, S. G., Admella, J., Moya-Andérico, L., Fehér, T., Arévalo-Jaimes, B. V., Blanco-Cabra, N., et al. (2023). Accessing the in vivo efficiency of clinically isolated phages against Uropathogenic and invasive biofilm-forming Escherichia coli strains for phage therapy. Cells 12:344. doi: 10.3390/cells12030344
Sharma, S., Chatterjee, S., Datta, S., Prasad, R., Dubey, D., Prasad, R. K., et al. (2017). Bacteriophages and its applications: an overview. Folia Microbiol. (Praha) 62, 17–55. doi: 10.1007/s12223-016-0471-x
Skurnik, D., Kaito, C., Yoshikai, H., Wakamatsu, A., Miyashita, A., Matsumoto, Y., et al. (2020). Non-pathogenic Escherichia coli acquires virulence by mutating a growth-essential LPS transporter. PLoS Pathog. 16:e1008469. doi: 10.1371/journal.ppat.1008469
Smug, B. J., Szczepaniak, K., Rocha, E. P. C., Dunin-Horkawicz, S., and Mostowy, R. J. (2023). Ongoing shuffling of protein fragments diversifies core viral functions linked to interactions with bacterial hosts. Nature. Communications 14:236. doi: 10.1038/s41467-023-43236-9
Stanke, M., Schoffmann, O., Morgenstern, B., and Waack, S. (2006). Gene prediction in eukaryotes with a generalized hidden Markov model that uses hints from external sources. BMC Bioinformatics 7:62. doi: 10.1186/1471-2105-7-62
Tabib-Salazar, A., Liu, B., Shadrin, A., Burchell, L., Wang, Z., Wang, Z., et al. (2017). Full shut-off of Escherichia coli RNA-polymerase by T7 phage requires a small phage-encoded DNA-binding protein. Nucleic Acids Res. 45, 7697–7707. doi: 10.1093/nar/gkx370
Tamura, K., and Nei, M. (1993). Estimation of the number of nucleotide substitutions in the control region of mitochondrial-DNA in humans and chimpanzees. Mol. Biol. Evol. 10, 512–526. doi: 10.1093/oxfordjournals.molbev.a040023
Tillich, M., Lehwark, P., Pellizzer, T., Ulbricht-Jones, E. S., Fischer, A., Bock, R., et al. (2017). GeSeq - versatile and accurate annotation of organelle genomes. Nucleic Acids Res. 45, W6–W11. doi: 10.1093/nar/gkx391
Venturini, C., Petrovic Fabijan, A., Fajardo Lubian, A., Barbirz, S., and Iredell, J. (2022). Biological foundations of successful bacteriophage therapy. EMBO Mol. Med. 14:e12435. doi: 10.15252/emmm.202012435
Washizaki, A., Yonesaki, T., and Otsuka, Y. (2016). Characterization of the interactions between receptors, LPS and OmpC, and bacteriophage T4 long tail fibers. Microbiology 5, 1003–1015. doi: 10.1002/mbo3.384
Wei, B., Cong, C., Zheng, L., Chen, L., and Yan, X. (2023). Isolation, characterization and whole genome analysis of the novel genus Lederbergvirus, phage vB_EcoP_E212 infecting enterotoxigenic Escherichia coli K88. Virus Res. 331:199125. doi: 10.1016/j.virusres.2023.199125
Xiao, T., Zhu, X., Wang, W., Jia, X., Guo, C., Wang, X., et al. (2023). A novel lytic bacteriophage against colistin-resistant Escherichia coli isolated from different animals. Virus Res. 329:199090. doi: 10.1016/j.virusres.2023.199090
Zerbino, D. R., and Birney, E. (2008). Velvet: algorithms for de novo short read assembly using de Bruijn graphs. Genome Res. 18, 821–829. doi: 10.1101/gr.074492.107
Zerbino, D. R., McEwen, G. K., Margulies, E. H., and Birney, E. (2009). Pebble and rock band: heuristic resolution of repeats and scaffolding in the velvet short-read de novo assembler. PLoS One 4:e8407. doi: 10.1371/journal.pone.0008407
Keywords: bacteriophages, genomic analysis, Escherichia coli , Siphoviridae, LPS, lptD
Citation: Zhang H, You J, Pan X, Hu Y, Zhang Z, Zhang X, Zhang W and Rao Z (2024) Genomic and biological insights of bacteriophages JNUWH1 and JNUWD in the arms race against bacterial resistance. Front. Microbiol. 15:1407039. doi: 10.3389/fmicb.2024.1407039
Edited by:
Veronique Delesalle, Gettysburg College, United StatesReviewed by:
Swapnil Ganesh Sanmukh, Université Clermont Auvergne, FranceAlbert Vill, Yale University, United States
Karen K. Klyczek, University of Wisconsin–River Falls, United States
Copyright © 2024 Zhang, You, Pan, Hu, Zhang, Zhang, Zhang and Rao. This is an open-access article distributed under the terms of the Creative Commons Attribution License (CC BY). The use, distribution or reproduction in other forums is permitted, provided the original author(s) and the copyright owner(s) are credited and that the original publication in this journal is cited, in accordance with accepted academic practice. No use, distribution or reproduction is permitted which does not comply with these terms.
*Correspondence: Xian Zhang, enhAamlhbmduYW4uZWR1LmNu; Weiguo Zhang, emhhbmd3Z0BqaWFuZ25hbi5lZHUuY24=; Zhiming Rao, cmFvemhtQGppYW5nbmFuLmVkdS5jbg==;
†These authors share first authorship