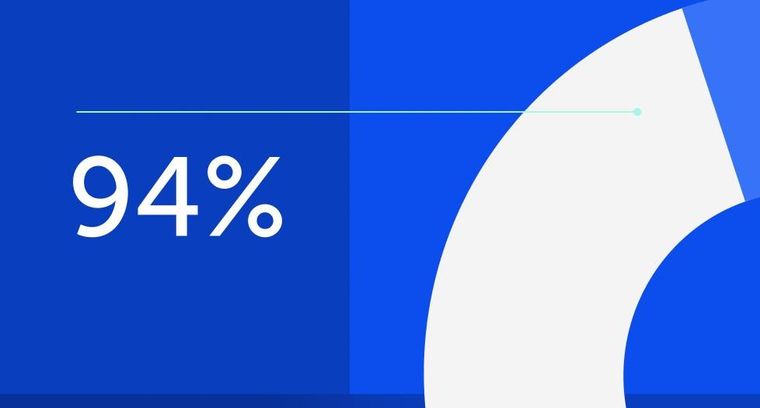
94% of researchers rate our articles as excellent or good
Learn more about the work of our research integrity team to safeguard the quality of each article we publish.
Find out more
REVIEW article
Front. Microbiol., 12 June 2024
Sec. Food Microbiology
Volume 15 - 2024 | https://doi.org/10.3389/fmicb.2024.1406904
This article is part of the Research TopicThe Weissella and Periweissella genera: Taxonomy, Detection, Safety and Their Application in Food and HealthView all articles
This review aims to comprehensively chronicle the biosynthesis, classification, properties, and applications of bacteriocins produced by Weissella genus strains, particularly emphasizing their potential benefits in food preservation, human health, and animal productivity. Lactic Acid Bacteria (LAB) are a class of microorganisms well-known for their beneficial role in food fermentation, probiotics, and human health. A notable property of LAB is that they can synthesize antimicrobial peptides known as bacteriocins that exhibit antimicrobial action against both closely related and other bacteria as well. Bacteriocins produced by Weissella spp. are known to exhibit antimicrobial activity against several pathogenic bacteria including food spoilage species, making them highly invaluable for potential application in food preservation and food safety. Importantly, they provide significant health benefits to humans, including combating infections, reducing inflammation, and modulating the gut microbiota. In addition to their applications in food fermentation and probiotics, Weissella bacteriocins show promising prospects in poultry production, processing, and improving animal productivity. Future research should explore the utilization of Weissella bacteriocins in innovative food safety measures and medical applications, emphasizing their potential to combat antibiotic-resistant pathogens, enhance gut microbiota composition and function, and synergize with existing antimicrobial therapies.
Graphical Abstract. Schematic representation of Weissella bacteriocin production, classification and applications.
Bacteriocins are bioactive peptides or proteins produced through ribosomal synthesis by bacteria. They are recognized for their ability to hinder the growth of closely related bacterial species, which is why they are referred to as bacteriocins (Daw and Falkiner, 1996). Several bacteria produce these antimicrobial peptides as part of their survival strategy. Depending on their size and mode of action, bacteriocins can either be lethal to bacteria (bactericidal) or inhibit their growth (bacteriostatic) (Nes et al., 2007). Bacteriocins mostly possess anti-microbial action either by inducing pore formation in the cell wall of the target organism or inhibiting cell wall biosynthesis, consequently resulting in death (Zacharof and Lovitt, 2012; Malik et al., 2021). The immunity of bacteriocin-producing organisms against their bacteriocins is facilitated by immunity proteins (Cotter et al., 2005). According to the reported studies (Klaenhammer, 1993; Kotelnikova and Gelfand, 2002; Chavan and Riley, 2007), bacteriocin synthesis typically occurs during the early stationary or late exponential phases of development. It is also commonly regulated by stress signaling and quorum sensing mechanisms. It is regarded as an adaptive probiotic trait causing the clampdown of gut-based pathogens (Dobson et al., 2012; O'Shea et al., 2012).
Antimicrobial peptides have gained interest recently as novel antimicrobials to fight dangerous bacteria, particularly those resistant to traditional antibiotics. Such bioactive substances might serve as models for creating new entities in the hunt for new bio-preservatives or drugs (Goh and Philip, 2015). Earlier reports have revealed the diverse capabilities of LAB isolates including probiotic potential, pathogen growth inhibition, mycotoxin degradation (Naidu et al., 1999; Todorov et al., 2020). Additionally, the rising interest in their use as natural preservatives for preserving foods has been fueled by the surge in demand for natural foods free of chemical preservatives (Gupta and Tiwari, 2014). Many bacteriocins produced by Lactobacillus, Enterococcus and Leuconostoc species have already been studied earlier (Yang et al., 2018; Cherukuri et al., 2019). However, the information on bacteriocins from Weissella species is limited and can be investigated further for possible applications (Cleveland et al., 2001). Additionally, some Weissella strains were reported to produce exopolysaccharides (EPS), which have different techno-functional and biological properties including prebiotic capabilities (Kavitake et al., 2016, 2020; Devi et al., 2021; Teixeira et al., 2021).
Weissella genus has consequently been acknowledged for its remarkable ability to endure the gastrointestinal tract (GIT), producing antimicrobial substances effective against numerous pathogens, and promoting the production of compounds that improve the gut microbiome. This review aims at providing up to date information on bacteriocins derived from the Weissella genus. It discusses production, classification, physico-chemical and functional properties, inhibitory mechanisms, and their diverse applications of bacteriocins from Weissella across various fields.
Bacteriocin production, control, self-immunity, transport, and modification are all regulated by the biosynthetic genes known as bacteriocin genes (Sahl and Bierbaum, 1998; McAuliffe et al., 2001; Dimov et al., 2005). The fundamental route involves the creation of pre-bacteriocin and further separation of the pre-peptide at a particular processing site, eliminating the leader sequence and leading translocation of pro-bacteriocin outside the cell membrane (Abdulkarim et al., 2020; Simons et al., 2020). Various genes play a vital role in bacteriocin biosynthesis processes (Table 1). These genes are generally organized as operons which can be found on conjugative transposable elements (nisin), over the host chromosomes (subtilin), and on the plasmids (cytolysin) (Banerjee and Hansen, 1988; Ike et al., 1990; Immonen and Saris, 1998). For instance, in the synthesis of most common bacteriocins, structural genes are responsible for the encoding of lantibiotics, which are usually referred to as lan, which denotes lantibiotics and the latter alphabet denotes functional aspect. This configuration of minimum genetic machinery includes genes required for modification (lanM), ABC-type peptide translocators (lanT), proteolytic processing (lanP), immunity (lanI), and regulation (lanR) (Klaenhammer, 1993; McAuliffe et al., 2001). The entire mechanism of bacteriocin production and release is based on signal transduction systems, where the general secretory path (GSP) and the signal peptide type sequence (SP) are crucial in processing and releasing bacteriocins (Kotelnikova and Gelfand, 2002). There are three major components, the inductor peptide (IP), response regulatory protein (RR), and sensor Histidine Protein Kinase (HPK). Moreover, genes with related functions are in close proximity to the cluster (Li et al., 2017).
Bacteriocins have the ability to operate as signaling, killing, and colonizing peptides. Cell-to-cell contact between members of the same or different species may arise from the interaction with the GI tract, enabling both cooperative and hostile microbial interactions (Perez et al., 2018). Furthermore, mechanistic activity of enterocin B and enterocin ST5Ha against H3N2, H1N138, and HSV type 137 respectively was regarded similar to class II bacteriocins. Moreover, bacteriocin Subtilosin A does not function prior to HSV type 1 and 2 viral protein synthesis at lower concentrations but has a virucidal action toward assembly or release of concerned proteins (Elalem, 2021).
Studies predict bacteriocin enters the target cell via cell surface receptors and creates ion-permeable channels in the cytoplasmic membrane, degrading cellular DNA non-specifically. This consequently inhibits the synthesis of proteins and peptidoglycan by specifically cleaving 16s rRNA, or causing cell lysis (Riley and Wertz, 2002). Similarly, a bacteriocin named Plantaricin CYLB47 targets Staphylococcus aureus and Pseudomonas aeruginosa by the mechanism of action of lantibiotics (type A lantibiotics that kill byforming pores, or type B lantibiotics that impede the biosynthesis of peptidoglycans) (Thuy et al., 2024). Limited studies mention an in-depth information with regard to few lactic acid bacteria, their gene structures, routes, and mechanisms of action of Class I, II, III, and IV bacteriocins from different ethnic background (Sharma et al., 2021). Specific to Weissella strains, bacteriocins 7293A, and B plausibly worked on the cytoplasmic membrane of target cells by creating hydrophilic portions that allowed essential cellular molecules to be effluxed and ultimately led to cell death (Papagianni and Papamichael, 2011; Woraprayote et al., 2015).
Target organisms naturally have a tendency for an environmental adaptation. After prolonged exposure, they acquire components that make them resistant to bacteriocins. Few ways of resistance development are, modifications in the fatty acid composition of the membrane; increase in the d-alanine content of teichoic acid wall; increase in the l-lysine content and modification of phospholipids and lastly changes in the structural genetic elements. By combining the bacteriocin with other antimicrobial substances like essential oil and organic acids and peptides increased the susceptibility of the target organisms. Other methods for effective outcomes include encapsulation, irradiation, and synergistic combinations of bacteriocins (Skaugen et al., 2003). One promising area of bioengineering has been the rational design of bacteriocins to enhance their functionality. Modification at selected residues of bactofencin and lacticin 3147 A increased their antibacterial efficacy (Kumariya et al., 2019).
LABs are gram-positive, non-aerobic and non-spore forming but aerotolerant cocci or cocco-bacilli bacteria. These are known for lactic acid production by fermenting carbohydrates. Belonging to the firmicutes phylum, they include most bacteria having probiotic properties (Liptáková et al., 2017; Bintsis, 2018). LABs are found ubiquitously in environments of milk, meat, fermented vegetables, and beverages. They were first discovered in milk, soil, water, manure, and sewage (Liu et al., 2014). Humans and animals also harbor LAB which has different functional roles such as inhibition of pathogen growth, extending the shelf life of foods, and enhancing the nutritive qualities of the product (Martinez et al., 2013). They have also been used as enhancing agents to improve flavor and texture (Silva et al., 2018). Some LAB like Streptococcus thermophilus and Lactobacillus lactis, possess the inhibiting capability for pathogenic bacterial growth, avoid food spoilage along with the simultaneous advantage of preserving the nutritive qualities of food material consequently giving it an extended shelf life. Additionally, they tend to produce growth-inhibitory substances like bacteriocins, hydrogen peroxide, diacyls, and others. Based on the type of products produced after the fermentation of carbohydrates, LAB can be classified into two types: homofermentative and heterofermentative microorganisms. Homofermentative LAB chiefly utilizes sugars to bring forth lactic acid, while heterofermentative produces lactic acid, alcohols, acetic acid, and carbon dioxide (Riley and Wertz, 2002; Teixeira et al., 2021). Additionally, LAB is better known for the production of bacteriocins of various kinds; a few of them are mentioned in Table 2.
The properties of LAB including synthesis of bacteriocins make it quite applicable as a natural preservative in food-based industries (Mokoena, 2017). Bacteriocin-producing LAB has been exploited as bio-preservatives and has contributed immensely to a diversity of food fermentations (Malik et al., 2021). A significant portion of bacteriocin research has revolved around the synthesis and examination of peptides derived out of various LAB species, including Lactococcus spp., Leuconostoc spp., Enterococcus spp., and Pediococcus spp. to explore the prospected use of these peptides as naturally originated food preservatives (Deegan et al., 2006; Chavan and Riley, 2007; Cheikhyoussef et al., 2009). Pediocin CP2, a natural antimicrobial peptide produced by Pediococcus acidilactici MTCC 5101 was studied for cytotoxicity toward the following cell lines: Sp2/0-Ag14 (a spleen lymphoblast), MCF7 (a mammary gland cancer), HeLa (a cervical adenocarcinoma), and HepG2 (a hepatocarcinoma cell line). The MTT and DNA fragmentation assays demonstarted the anti-cancer property of its recombinant version (Kumar et al., 2012). Bovicin HC5, which is produced by Streptococcus bovis HC5, was tested for its cytotoxic effects on following eukaryotic cell lines: HepG2 (a human liver hepatocellular carcinoma cell line), MCF-7 (a human breast adenocarcinoma cell line), and Vero cells (a monkey kidney epithelial cell line) (Paiva et al., 2012). AS-48 bacteriocin, obtained from Enterococcus faecalis strain UGRA10 has already become a commercial option for pharmacological development to prevent and cure infections, including those caused by multidrug-resistant microorganisms, especially in the skin and soft tissues (Cebrián et al., 2019).
The initial scheme of classification grew with new bacteriocins being identified and characterized. Eventually, based on the structural transformations, bacteriocins were categorized under new sub-classes (Montville and Kaiser, 1993; Kaiser, 2014; Zimina et al., 2020). Currently, four main classes have been figured out and recognized after reviewing the literature for several years (Figure 1). Lantibiotics, also called Class I bacteriocins, are a group of peptides with low molecular mass (< 5 kDa) that exhibit heat stability. These peptides are differentiated by the constitution of specific amino acids like lanthionine or β-methyl lanthionine that are the result of post-translational modifications (Ng et al., 2020). The Class I category can be classified into three more subgroups: Class Ia (lantibiotics), Class Ib (labyrinthopeptins), and Class Ic (sanctibiotics). Among these, nisin is the most studied and established bacteriocin belonging to the Class I category (Cuozzo et al., 2001; LeLay et al., 2016). Class II bacteriocins are non-modified smaller peptides (< 10 kDa) that are thermo-stable. They are divided into further subclasses: Class IIa, known as pediocin-like; Class IIb, consisting of two-chain peptides; and Class IIc, which includes single-chain peptides that are non-pediocin-like (Yang et al., 2018). The class II bacteriocins in the LAB, sakacin A (SakA), pediocin PA-1 (PedPA-1), enterocins P, Q, and L50 (enterocins), plantaricins EF and JK (plantaricins), and garvicin ML (GarML), differ significantly from one another in terms of their physicochemical characteristics and range of inhibition. They are also known to have a potential role in modifying gut microbiota to improve host health (Umu et al., 2016). On the other hand, Class III bacteriocins include larger proteins (>30 kDa) that are thermo-labile. Finally, Class IV bacteriocins encompass cyclic peptides with N and C termini that are covalently linked (Meade et al., 2020).
Bacteriocins initially have a very low molecular weight, but eventually, they go through post-translational modifications and thus are susceptible to proteolytic enzymes (Lajis, 2020). In general, they are abundantly constituted of lysyl and arginyl residues, making them amphipathic molecules by nature. Structural studies reveal that they are unstructured in aqueous solutions but once coming in exposure to structure favoring solvents (e.g., anionic phospholipid membranes or trifluoroethanol), they form a helical structure (Zacharof and Lovitt, 2012). Bacteriocins are typically titled after the species or genus of the producer strain. For instance, strains of Lactiplantibacillus plantarum (formerly Lactobacillus plantarum) are known to produce plantaricin, Lactococcus spp. produce lacticin and nisin, Carnobacterium spp. produce carnocin, Enterococcus spp. produce enterocin, Leuconostoc spp. produce leucocin, and Pediococcus spp. produce pediocin. These naming conventions help identify and differentiate the bacteriocins produced by specific bacterial strains (O'sullivan et al., 2002; Zouhir et al., 2010; Zheng et al., 2020).
There exists a distinct category of bacteriocins known as high molecular weight (HMW) bacteriocins, have been observed to have a structure like that of phage tails. The resemblance between HMW bacteriocins and bacteriophages has been accepted by examining their morphological characteristics through electron microscopy, immunological cross-reactivity, complementing properties, and DNA hybridization traits (Klaenhammer, 1993).
Weissella spp. comes under Gram-positive, non-motile, catalase-negative, and non-sporing group bacteria. Currently, the Weissella genus encompasses approximately 22 officially recognized species and most of these species are obtained from widely screened sources, with a particular emphasis on fermented foods (Kavitake et al., 2016, 2020). As members of LAB, Weissella spp. has specific growth requirements due to their natural habitat in environments rich in nutrients. They can be found in various sources such as vegetables, raw milk fish, meat, sewage, soil, blood, human and animal gastrointestinal tract, human oral cavity, and urogenital tract (Abriouel et al., 2015). Few Weissella spp. have been reported to be a cause of disease occurrences like sepsis, otitis, endocarditis and fish mortality (Harlan et al., 2011; Welch and Good, 2013; Kamboj et al., 2015). Infections in the human population resulting from Weissella spp. are infrequently documented, typically occurring for individuals who are susceptible to infections (Lee et al., 2012). Notably, the Weissella genus comprises both beneficial and harmful strains. However, it also plays a significant part in food fermentation and is identified as a potent probiotic culture with numerous health benefits (Fusco et al., 2015). Weissella spp. is commonly found in naturally fermented foods and actively contributes to the unique qualities of fermented products. These bacteria possess numerous technological and functional properties that have the potential to improve the safety, nutritional worth, and sensory construct of food (Fusco et al., 2018).
In addition to their recognized role through traditional fermentations, certain strains of Weissella spp. are gaining attention as putative probiotics. Specifically, the development of W. cibaria strains is being explored due to their significant probiotic potential in managing periodontal disease (Fusco et al., 2015). Despite the characterization of numerous strains, only a few bacteriocins from Weissella spp. have been identified, as outlined in Table 3. Furthermore, there are scarce reports on bacteriocin production by the commonly encountered Weissella confusa (Goh and Philip, 2015). It was not until 2007 that W. cibaria was identified as the first bacteriocin-producing strain within the genus, exhibiting inhibitory effects against a few gram-positive bacteria (Srionnual et al., 2007). Furthermore, W. cibaria became the center of interest due to its potential antimicrobial and antifungal properties. This eventually raised interest in other Weissella strains. In recent times, there have been emerging applications of bacteriocins like weissellin A and bacteriocin D1501 of W. hellenica D1501 which have led to the improvement of fermented foods by raising their shelf life (Papagianni, 2012; Chen et al., 2014b; Tenea and Lara, 2019). Moreover, there have been limited reports regarding the mechanism of these antimicrobial substances obtained from Weissella spp. (Sturino, 2018).
Bacteriocin isolation and purification from Weissella spp. was primarily done after obtaining the desired pure colony and optimization of culture conditions for maximum production (Fusco et al., 2015; Goh and Philip, 2015). It was demonstrated that W. hellenica QU 13 could possess regulated production of Weissellicins Y and M depending on the nutritional conditions, and varying environmental circumstances (Masuda et al., 2016). To study production of Weissellicin L from W. hellenica 4-7, GYP (Glucose Yeast Peptone) medium was studied and optical density of the inoculated culture at 600 nm was measured. Qualitatively, bacteriocin activity was further expressed as arbitrary activity units (AU; reciprocal of the highest dilution at which activity was still obtained) (Leong et al., 2013). In order to assess how well various culture mediums facilitate W. hellenica BCC 7293 to produce bacteriocin, TGE (Trypton Glucose Extract Broth; 1% tryptone, 1% yeast extract, 1% glucose, 0.2% Tween 80), TSB (Tryptic Soya Broth), MRS, APT (All Purpose Tween 80), CYG (Casein Yeast Glucose; 1.5% casein sodium salt, 1.5% yeast extract, and 1.0% glucose), WYG (Whey Yeast Glucose; 1.5% whey protein isolate, 1.5% yeast extract, and 1.0% glucose) broths were compared for quantitave production of bacteriocin (Woraprayote et al., 2015). Upon incubation, the cell free culture supernatant harvest was taken further for extraction. Moreover, methods of purification have also been further chosen according to the yield obtained after modification in methodologies (Sharma et al., 2019). For instance, changes in concentrations of reagents such as molarities of buffers, precipitating agents, etc. (Cheigh et al., 2002; Fahim et al., 2017). Primarily used culture medium is De Man, Rogosa, and Sharpe broth (MRS), but Luria Bertani (LB) broth was also used sometimes (Goh and Philip, 2015). Enrichment of the media using different supplements has been in trend after surveying the factors leading to less production of the active principle. It has been an efficient solution to move toward an expected yield (Goh and Philip, 2015; Ma et al., 2020). Monitoring of the Colony Forming Units (CFU) and optical density of the bacteria within different time durations has been a major approach to determining the growth pattern of the organism (Loutfi et al., 2020). Apart from the amount of yield, the activity of obtained bacteriocin and further validation of its potency is a significant step in studies. Every method followed has been with respect to the molecular, chemical, and structural properties of the active component. According to the studies, bacteriocins from Weissella spp. have been crucial enough for novel research regarding food safety (Delgado et al., 2005). Considering the methods followed till now, the most conventional has been an initial lab-scale production followed by scaled-up production under optimized conditions (Mahrous et al., 2013). Steps involved are the removal of cellular debris by centrifugation; protein precipitation using appropriate salt and solvent; removal of respective salt or solvent by centrifugation or dialysis; removal of excessive precipitating agent; estimation of total protein content, validation of activity in crude sample expected to contain the active protein; ultra-purification of the crude sample to get the purified component and further application based approaches as depicted in Figure 3 (Chen et al., 2014b; Goh and Philip, 2015; Goyal et al., 2018). Maximum bacteriocin production has been recorded in the early stationary phase using MRS, Glucose Yeast Peptone (GYP), or in some cases All Purpose Tween (APT) culture media (Yang et al., 2018).
The precipitation step has been accordingly optimized depending upon the variations in saturation percentages of the salt or solvent. Ammonium sulfate, acetone, ethanol, trichloroacetic acid, trifluoroacetic acid, or isopropanol are commonly used depending on the properties of the targeted component (Goh and Philip, 2015). In some cases, surfactants have also been known to be contributing for studying the stability and growth responses (Goyal et al., 2018; Wang et al., 2018). Removal of excessive components and impurities up to an extent is further done by dialyzing the sample using buffers of appropriate pH and concentration. Moreover, for storage and washing purposes sodium phosphate buffer and Tris-HCl buffer were most commonly used (Banerjee et al., 2013). The next step of purification is done by gel permeation chromatography and further appropriate gel electrophoresis methods like SDS-PAGE, Native-PAGE, or even modified tricine-PAGE for molecular mass characterization. Ultra-purification was achieved using pre-equilibrated Sepharose or Sephadex columns and purity check using methods like HPLC or LCMS as per the requirement (Wu et al., 2015). After the completion of purification, application-based approaches have been used for food-grade development toward food safety and similar processed products (Pal and Ramana, 2010; Papagianni and Papamichael, 2011; Chen et al., 2013; Bancalari et al., 2020).
Advanced identification techniques for bacteriocins involve a combination of biochemical, genetic, and bioinformatic approaches (Pingitore et al., 2007; Durgadevi et al., 2020).
Mass spectrometry (MS) upon the conventional chromatographic method allows the characterization of bacteriocins based on their mass-to-charge ratio (m/z). Matrix-assisted laser desorption/ionization time-of-flight (MALDI-TOF) MS and electrospray ionization (ESI) MS are efficient techniques for bacteriocin analysis, providing information on peptide mass, sequence, and post-translational modifications. With advancements in sequencing technologies, whole-genome sequencing (WGS) has become a powerful tool for identifying bacteriocin genes.
These tools utilize algorithms for sequence alignment, motif prediction, and structural modeling to annotate bacteriocin genes and predict their biological activity. Genomic analytical tools can then be used to analyze the genomic data and predict potential bacteriocin-encoding genes based on sequence homology, conserved domains, and structural features.
X-ray crystallography, nuclear magnetic resonance (NMR) spectroscopy, and cryo-electron microscopy (cryo-EM) can be used to determine the three-dimensional structure of bacteriocins. Structural studies provide insights into the molecular mechanisms at the genetic level including potential interactions with target cells and membrane permeabilization.
Machine learning algorithms can be trained on large datasets of known bacteriocins to predict novel candidates based on sequence features, physicochemical properties, and activity spectra. Data mining approaches can be used to uncover pattern and relation among bacteriocin databases. This can further be validated by high-throughput methods, such as microfluidics-based assays or automated liquid handling systems, to evaluate the potency and spectrum of bacteriocins.
Bacteriocins are diverse in terms of environment, structure, and function, although they have an apparent similarity to yeast and paramecium killing factors. Due to such properties, it has been regarded as a potentially sustainable food preservation agent (Ng et al., 2020). Applications of bacteriocins have been tested and are in the process of evaluating and assessing their application as narrow-spectrum antibiotics. They have drawn immense attention with regard to medicine, as they are hands-on at nanomolar concentrations causing no toxicity to humans. Moreover, their specific mechanisms of low propensity and extremely specific activity to cause resistivity are profitable properties. Many of these have been used as prospective therapeutic agents (Mathur et al., 2017). Bacteriocins have been thus used and consequently known to battle skin infections along with oral, gastrointestinal, respiratory, and urogenital tract infections (Hammami et al., 2013; Sidek et al., 2018).
The bacteriocins obtained from the Weissella genus display a wide range of physicochemical properties, making them highly diverse, adaptable, and applicable (Lee et al., 2002; Abriouel et al., 2015; Fusco et al., 2015). These bacteriocins possess several recognized properties, including:
Weissella bacteriocins demonstrate antimicrobial activity against various foodborne pathogens and other disease-causing microorganisms. They have shown efficacy in combating bacteria, viruses, fungi, tumors, obesity-related factors, inflammation, and oxidative stress (Meade et al., 2020). The bacteriocins classified under class II are expected to affect plasma and disturb the membrane integrity of pathogens causing cell death by an efflux of requisite cellular metabolites. The thermal stability and acid resistance of this have been a potential aspect at the industrial level for acidic food preservation (Ahmed et al., 2022). Weissella sp. GMP12 is able to inhibit Staphylococcus aureus ATCC 6,538 with bacteriocin activity of 3,693.60 AU and Salmonella spp. 230C with bacteriocin activity 2,254.17. Moreover, Weissella sp. GMP12 inhibits Klebsiella sp. CK2 with bacteriocin activity is 3,165.51 AU (Yaafi'Al-Hammam et al., 2023).
Weissella bacteriocins exhibit stability under different temperature conditions and pH ranges. Their optimal activity is typically observed at acidic pH levels between 2 and 6 (Adesina and Enerijiofi, 2016). Masuda et al. (2012) reported the effects of pH and temperature on the activities of purified bacteriocins Weissellicins Y and M at an adjusted pH range of 3.0–11.0 incubation temperature 80, 100, and 121°C for 30 min. Weissellicin M has been observed to be significantly more stable against pH and heat than many other bacteriocins reported earlier.
These bacteriocins are proteolytically degradable, indicating that they are susceptible to enzymatic destruction. They have, however, performed positively to different enzymatic treatments, including lipase, oxidase, catalase, lysozyme, and amylase (Tenea and Lara, 2019).
Weissella bacteriocins have been linked to probiotic attributes owing to their capacity to maintain functionality within the gastrointestinal tract (GIT) (Sharma et al., 2018). These bacteriocins are deemed harmless and hold promise for advantageous use (Dobson et al., 2012; Yang et al., 2014; Fusco et al., 2015; Todorov et al., 2020). Studies also signify that bacteriocin like substance produced by Weissella sp. GMP12 has the potential to be used as a probiotic starter culture in food fermentations (Yaafi'Al-Hammam et al., 2023). All of these characteristics demonstrate the adaptability and potential usefulness of Weissella bacteriocins in a stretch of industries, for instance food preservation, disease prevention, and probiotic formulations. Further research and exploration of aforementioned properties can eventually support the evolution of innovative plans of action for utilizing Weissella bacteriocins in different industries.
Data about bacteriocin-based therapeutic applications have been independently found in several resources for several years. To sort out this kind of informative deficiency, a basal literature area in BACTIBASE has been developed, which works by grouping papers focusing on bacteriocins and similar substances (Bacteriocin-like substances-BLIS), that have been consequently established as leading therapeutic agents (Newstead et al., 2020). There are reports supporting the potential of bacteriocins for pathogen inhibition in a variety of food matrices such as cheese, meat, and vegetables. Several bacteriocins from validated or notable probiotic bacterial strains have been prospectively evaluated for utilization as potent therapeutic agents for in-vitro studies and countable ones for in-vivo as well. W. hellenica BCC 7239 is known to produce bacteriocins 7293A and 7293B which inhibits pathogens like P. aeruginosa, E. coli, A. hydrophila and S. Typhimurium in meat and related products (da Costa et al., 2019). Evaluation of the pharmacokinetics and pharmacodynamics of several bacteriocins proved respective therapeutic efficacies (such as lantibiotic MU1140) (Soltani et al., 2020). Moreover, extensive improvement has been achieved with regard to knowledge about bacteriocin structure and function, regulation, immunity, additional elements affecting cell survival, production of bacteriocin, and its activity thereafter (Meade et al., 2020). Applications of bacteriocins have been reported in various fields as mentioned in Figure 2 and elaborated below:
W. confusa, W. hellenica, and W. cibaria species are studied for some essential properties like the production of extracellular polysaccharides and non-digestible oligosaccharides, which can be further utilized as a probiotic constituent of food, fodder, cosmetics and pharmaceutics (Björkroth et al., 2002; Cole et al., 2006). Bacteriocin A3 produced by W. confusa A3 showed strong inhibition against Micrococcus luteus ATCC10240, Escherichia coli UT181, Bacillus cereus ATCC14579, Enterococcus faecium C1, Pseudomonas aeruginosa PA7, Lactococcus lactis A1. This supported the ability of the aforementioned bacteriocins to possess antimicrobial activity against Gram-positive and Gram-negative bacteria as well (Goh and Philip, 2015). Weissellicin D produced by W. hellenica D1501 has been reported to inhibit food spoilage bacteria as well as pathogens like Escherichia coli, Listeria monocytogenes, Staphylococcus aureus, yeasts, and molds in soymilk (Chen et al., 2014a,b). Weissellicin A from Weissella paramesenteroides DX isolated from European-type fermented sausages has been known to be heat resistant and controls the growth of Listeria monocytogenes, Listeria innocua, and Clostridium sporogenes (Papagianni and Papamichael, 2011; Papagianni and Sergelidis, 2013).
Weissella spp. has also been found to be useful in the form of probiotics, due to their antimicrobial activity. Some of them are W. cibaria, W. paramesenteroides, and W. hellenica (Fusco et al., 2015). For instance, W. cibaria is proposed as an oral probiotic, inhibiting Streptococcus mutans which are responsible for glucan biofilm formation (Kang et al., 2009). Weissellicin 110 from Weissella cibaria 110 and Weissella cibaria KMITL-QU 21 have been known to be acting against potential pathogens (Srionnual et al., 2007; Swetwiwathana et al., 2008). Moreover, bacteriocins from strains like Weissella cibaria FMF4B16 and Weissella cibaria N23 have been known to inhibit pathogenic bacteria like Aspergillus niger MUCL 28699 and related potentially pathogenic Weissella spp. strains (Ndagano et al., 2011; Pringsulaka et al., 2012). Also, W. hellenica DS-12 from flounder intestine is evidently in use as a fish probiotic, due to its inhibitory activity against pathogens found in fishes, such as Aeromonas sp., Edwardsiella sp., Pasteurella sp., and Vibrio sp. (Cai et al., 1998). The pro-technological and probiotic potential of Weissella spp. therefore strikes off the potential of other human pathogens. Thus, a safety evaluation recommended for every strain which is potentially a starter culture or a probiotic (Ballongue, 2004; Ogier and Serror, 2008).
Bacteriocin-producing bacteria (BPB) have been known to provide many contributions to get beneficial outputs from livestock usage by increasing the productivity of animals. This is obtained by the inhibition of a particular group of organisms (Castillo-González et al., 2014). There are instances to increase feed efficacy, due to the synthesis of bacteriocins by reduction in carbon produced in the form of methane (Rabelo, 2016). The pre-harvest application of BPB for the safety of food is reasoned as one of the vital interventions causing a decrease in the gut-centered colonization of livestock by food-borne pathogenic organisms; for instance, during the processing of feeds like silage, immense contribution has been reported by LAB (Diez-Gonzalez, 2007; Bemena et al., 2014). In this regard, the anti-pathogenic characteristics of Weissella bacteriocins against several pathogens could be the prominent option for animal productivity applications (Mantovani et al., 2011).
Nisin significantly reduced the quantity of Listeria bacteria in scald water from a poultry processing factory, according to Mahadeo and Tatini (1994). There was an instantaneous 2-log (100-fold) decline following therapy, indicating a significant reduction. Moreover, after 48 h of chilling, Listeria was completely eradicated. This proved how effective nisin is at improving food safety while processing poultry. Salmonella typhimurium was experimentally contaminated chicken parts' skin. Natrajan and Sheldon (2000a) reported that nisin, EDTA (a chelating agent), and Tween 80 (a surfactant) were administered under acidic conditions via an alginate or agarose-based gel onto the skin of the chicken parts. After 72 h of storage at 4°C, this treatment significantly decreased the pathogen's numbers by up to 3 log10 (1,000-fold). Studies also showed that immersing chilled grill drumsticks in a solution containing nisin could extend their shelf life (Natrajan and Sheldon, 2000b). In addition, they were kept on nisin-treated tray pads and in a Polyvinyl chloride (PVC) overwrap treated with nisin. The chicken pieces' shelf life was increased by 0.6 to 2.2 days using this combined method.
Bacteriocin, Nisin-based formulation was employed to combat mastitis in lactating cows caused by bacterial pathogens like Staphylococcus aureus, Streptococcus uberis, and Streptococcus dysgalactiae (Cao et al., 2007; Wu et al., 2007; Field et al., 2021). BPB that produces bacteriocins can help stimulate animal productivity. By inhibiting specific groups of harmful organisms, they create a more favorable environment for livestock, promoting better health and growth. This can result in increased meat and milk production, which is essential for the livestock industry (Bemena et al., 2014; Bennett et al., 2022). Some BPB can synthesize bacteriocins that target methanogenic bacteria, which are responsible for producing methane during the digestive process in the stomachs of ruminant animals. By reducing the populations of these methane-producing bacteria, BPB can reduce methane emissions from livestock, which is a potent greenhouse gas and a significant contributor to climate change, thereby environmentally beneficial. Additionally, reduced methane production may lead to improved feed efficiency, as less energy is lost in the form of methane (Moumen et al., 2016).
Weissellicin Y, Weissellicin M, and Weissellicin L bacteriocins derived from Weissella strains have been found to have specific inhibitory abilities against Listeria monocytogenes ATCC 19111. This suggests their potential use in food safety and preservation to prevent the growth of Listeria bacteria that cause foodborne illnesses. Weissella strains have been shown to exhibit chemo-preventive and anti-tumor effects. This suggests their potential use in cancer prevention and treatment (Park et al., 2012; Kwak et al., 2014). Bacteriocins which include compounds like Weissellicin Y and M are reported to have antiviral properties. This has led to their consideration for pharmaceutical and food-based applications. Their high margin of safety makes them attractive for these purposes. Additionally, addressing viral resistance is an ongoing concern, and the use of bacteriocins could help tackle this issue. Reports suggest antiviral activity of bacteriocin enterocin CRL35, obtained from Enterococcus faecium CRL35 33 was found to act on the intracellular replication of Herpes Simplex (HSV) type 1 and 2.This was further investigated, suggesting that enterocin CRL35 acts on glycoprotein synthesis of the replication of the virus. Similarly, another study reveals that enterocin ST4V generated by Enterococcus mundtii ST4V, inhibited the polio virus by 50% and HSV types 1 and 2 by 99.9% and measles virus by 95%. Synthesis of bacteriocin mutacin 1,140 was associated with Streptococcus mutans strain's ability to colonize the oral cavity (Elalem, 2021). In vitro studies on Staphylococcus aureus demonstrate the potent antibacterial action of mersacidin. Lantibiotics like Pumilicin 4 and haloduracin produced by Bacillus sphaericus, have been found to be more stable than nisin at physiological pH values, particularly interesting for medical applications (Malik et al., 2021). There are bacteriocins that can be produced in vitro and prove helpful for intestinal tract survival is supported by intestinal bacteria like Fusobacterium mortiferum isolated from chicken ceca (Yusuf and Hamid, 2013).
Through their effects on gut microbial communities, bacteriocins may influence the host's immune system in addition to helping the producer survive and colonize in the gut and inhibiting closely related competitor strains or pathogens (Makras and De Vuyst, 2006). When probiotic strains that do not generate bacteriocins are combined with one that does, for example, Lactobacillus salivarius strain that produces salivaricin P becomes prevalent in the ileum of pigs (Millette et al., 2008; Walsh et al., 2008; Dobson et al., 2012).
The review covers the biosynthesis, classification, and naming of bacteriocins within LAB, with an emphasis on Weissella spp. Their applications are a testament to the multifaceted benefits of agriculture and animal husbandry as well. However, there is a clear research gap in this area because there hasn't been much research done especially on bacteriocins from the Weissella genus. This review seeks to clarify this issue and motivate scientists to focus on future investigation of these Weissella bacteriocins, thus broadening their possible applications. The production and purification methods of Weissella bacteriocins are discussed, highlighting their unique properties. These bacteriocins have strong antimicrobial activity against various pathogenic bacteria, making them valuable for food preservation and food safety. Weissella spp. also has probiotic potential, promoting healthy microbiota in the human gut and enhancing immunity, another crucial role exists in poultry processing. Although probiotic characterization has been validated, future research may involve rigorous testing of Weissella bacteriocins to ensure their safety for consumption in both humans and animals, as well as obtaining regulatory approvals for their use in various applications. Approaches may focus on developing Weissella based probiotics with enhanced bacteriocin production, efficacy, and stability. Biotechnological advances may lead to the development of efficient production systems for Weissella bacteriocins. This could involve the dynamics of genetic engineering to enhance production or the use of fermentation processes to scale up production for commercial applications of bacteriocins. These findings underscore the versatility of these microorganisms and their bioactive compounds in various fields, including food science, medicine, and health promotion.
JS: Conceptualization, Writing – original draft, Writing – review & editing, Visualization. PD: Writing – original draft, Writing – review & editing, Visualization. GR: Writing – original draft, Writing – review & editing, Supervision. AJ: Writing – original draft, Writing – review & editing. DK: Writing – original draft, Writing – review & editing, Conceptualization, Supervision. PS: Conceptualization, Supervision, Visualization, Writing – original draft, Writing – review & editing.
The author(s) declare that no financial support was received for the research, authorship, and/or publication of this article.
JS, DK, and PD are grateful to the Indian Council of Medical Research (ICMR), SERB and DST for providing financial assistance in the form of ICMR-Senior Research Fellowship (OMI-Fellowship/13/2022/ECD), SERB-National Post Doctoral Fellowship (PDF/2021/000551) and WISE KIRAN Scheme (DST/ WOS-A/LS -259/2019 (G) respectively.
The authors declare that the research was conducted in the absence of any commercial or financial relationships that could be construed as a potential conflict of interest.
All claims expressed in this article are solely those of the authors and do not necessarily represent those of their affiliated organizations, or those of the publisher, the editors and the reviewers. Any product that may be evaluated in this article, or claim that may be made by its manufacturer, is not guaranteed or endorsed by the publisher.
Abdulkarim, I. H., Mohammed, S. S. D., and Orukotan, A. A. (2020). Gene identification for bacteriocin production by lactic acid bacteria isolated from selected fermented foods. Asian J. Biochem. Genet. Molec. Biol. 3, 1–12. doi: 10.9734/ajbgmb/2020/v3i430090
Abriouel, H., Lerma, L. L., Casado Muñoz, M. D. C., Montoro, B. P., Kabisch, J., Pichner, R., et al. (2015). The controversial nature of the Weissella genus: technological and functional aspects versus whole genome analysis-based pathogenic potential for their application in food and health. Front. Microbiol. 6:1197. doi: 10.3389/fmicb.2015.01197
Adesina, I. A., and Enerijiofi, K. E. (2016). Effect of pH and heat treatment on bacteriocin activity of Pediococcus pentosaceus IO1, Tetragenococcus halophilus PO9 and Lactobacillus cellobiosus BE1. SAU Sci. Tech J. 1, 113–118.
Ahmed, S., Singh, S., Singh, V., Roberts, K. D., Zaidi, A., and Rodriguez-Palacios, A. (2022). The Weissella genus: Clinically treatable bacteria with antimicrobial/probiotic effects on inflammation and cancer. Microorganisms 10:2427. doi: 10.3390/microorganisms10122427
Ballongue, J. E. A. N. (2004). Bifidobacteria and probiotic action. Food Sci. Technol. NY. Marcel Dekker 139, 67–124. Available online at: https://www.cabidigitallibrary.org/doi/full/10.5555/20053043844
Bancalari, E., Alinovi, M., Bottari, B., Caligiani, A., Mucchetti, G., and Gatti, M. (2020). Ability of a wild Weissella strain to modify viscosity of fermented milk. Front. Microbiol. 10:3086. doi: 10.3389/fmicb.2019.03086
Banerjee, S., and Hansen, J. N. (1988). Structure and expression of a gene encoding the precursor of subtilin, a small protein antibiotic. J. Biol. Chem. 263, 9508–9514. doi: 10.1016/S0021-9258(19)76571-5
Banerjee, S. P., Dora, K. C., and Chowdhury, S. (2013). Detection, partial purification and characterization of bacteriocin produced by Lactobacillus brevis FPTLB3 isolated from freshwater fish: Bacteriocin from Lb. brevis FPTLB3. J. Food Sci. Technol. 50, 17–25. doi: 10.1007/s13197-011-0240-4
Bemena, L. D., Mohamed, L. A., Fernandes, A. M., and Lee, B. H. (2014). Applications of bacteriocins in food, livestock health and medicine. Int. J. Curr. Microbiol. Appl. Sci. 3, 924–949. doi: 10.13140/RG.2.1.3426.2488
Bennett, S., Fliss, I., Said, L. B., Malouin, F., and Lacasse, P. (2022). Efficacy of bacteriocin based formula for reducing Staphylococci, Streptococci, and total bacterial counts on teat skin of dairy cows. J. Dairy Sci. 105, 4498–4507. doi: 10.3168/jds.2021-21381
Bintsis, T. (2018). Lactic acid bacteria as starter cultures: an update in their metabolism and genetics. AIMS Microbiol. 4:665. doi: 10.3934/microbiol.2018.4.665
Björkroth, K. J., Schillinger, U., Geisen, R., Weiss, N., Hoste, B., Holzapfel, W. H., et al. (2002). Taxonomic study of Weissella confusa and description of Weissella cibaria sp. nov., detected in food and clinical samples. Int. J. System. Evolut. Microbiol. 52, 141–148. doi: 10.1099/00207713-52-1-141
Blom, H., Katla, T., Holck, A., Sletten, K., Axelsson, L., and Holo, H. (1999). Characterization, production, and purification of leucocin H, a two-peptide bacteriocin from Leuconostoc MF215B. Curr. Microbiol. 39, 43–48. doi: 10.1007/PL00006825
Cai, Y., Benno, Y., Nakase, T., and Oh, T. K. (1998). Specific probiotic characterization of Weissella hellenica DS-12 isolated from flounder intestine. J. Gen. Appl. Microbiol. 44, 311–316. doi: 10.2323/jgam.44.311
Cao, L. T., Wu, J. Q., Xie, F., Hu, S. H., and Mo, Y. (2007). Efficacy of nisin in treatment of clinical mastitis in lactating dairy cows. J. Dairy Sci. 90, 3980–3985. doi: 10.3168/jds.2007-0153
Castillo-González, A. R., Burrola-Barraza, M. E., Domínguez-Viveros, J., and Chávez-Martínez, A. (2014). Rumen microorganisms and fermentation. Arch. Med. Vet. 46, 349–361. doi: 10.4067/S0301-732X2014000300003
Cebrián, R., Rodríguez-Cabezas, M. E., Martín-Escolano, R., Rubiño, S., Garrido-Barros, M., Montalbán-López, M., et al. (2019). Preclinical studies of toxicity and safety of the AS-48 bacteriocin. J. Adv. Res. 20, 129–139. doi: 10.1016/j.jare.2019.06.003
Chavan, M. A., and Riley, M. A. (2007). “Molecular evolution of bacteriocins in gram-negative bacteria,” in Bacteriocins: Ecology and evolution (Berlin, Heidelberg: Springer Berlin Heidelberg), 19–43. doi: 10.1007/978-3-540-36604-1_3
Cheigh, C. I., Choi, H. J., Park, H., Kim, S. B., Kook, M. C., Kim, T. S., et al. (2002). Influence of growth conditions on the production of a nisin-like bacteriocin by Lactococcus lactis subsp. lactis A164 isolated from kimchi. J. Biotechnol. 95, 225–235. doi: 10.1016/S0168-1656(02)00010-X
Cheikhyoussef, A., Pogori, N., Chen, H., Tian, F., Chen, W., Tang, J., et al. (2009). Antimicrobial activity and partial characterization of bacteriocin-like inhibitory substances (BLIS) produced by Bifidobacterium infantis BCRC 14602. Food Control 20, 553–559. doi: 10.1016/j.foodcont.2008.08.003
Chen, C., Chen, X., Jiang, M., Rui, X., Li, W., and Dong, M. (2014a). A newly discovered bacteriocin from Weissella hellenica D1501 associated with Chinese Dong fermented meat (Nanx Wudl). Food Control 42, 116–124. doi: 10.1016/j.foodcont.2014.01.031
Chen, C., Rui, X., Lu, Z., Li, W., and Dong, M. (2014b). Enhanced shelf-life of tofu by using bacteriocinogenic Weissella hellenica D1501 as bioprotective cultures. Food Control 46, 203–209. doi: 10.1016/j.foodcont.2014.05.004
Chen, Y. S., Wu, H. C., Kuo, C. Y., Chen, Y. W., Ho, S., and Yanagida, F. (2018). Leucocin C-607, a novel bacteriocin from the multiple-bacteriocin-producing Leuconostoc pseudomesenteroides 607 isolated from persimmon. Probiotics Antimicrob. Proteins 10, 148–156. doi: 10.1007/s12602-017-9359-6
Chen, Y. S., Yu, C. R., Ji, S. H., Liou, M. S., Leong, K. H., Pan, S. F., et al. (2013). Enterocin T, a novel class IIa bacteriocin produced by Enterococcus sp. 812. Arch. Microbiol. 195, 655–660. doi: 10.1007/s00203-013-0917-3
Cherukuri, P. J., Narayanan, R., and Akkina, R. C. (2019). Production and preliminary characterization of bacteriocin from Enterococcus Faecium against Listeria Monocytogenes. Asian J. Microbiol. Biotechnol. Environ. Sci. 21, 1033–1040. Available online at: http://www.envirobiotechjournals.com/AJMBES/Issue42019/AJ-36.pdf
Cleveland, J., Montville, T. J., Nes, I. F., and Chikindas, M. L. (2001). Bacteriocins: safe, natural antimicrobials for food preservation. Int. J. Food Microbiol. 71, 1–20. doi: 10.1016/S0168-1605(01)00560-8
Cole, K., Farnell, M. B., Donoghue, A. M., Stern, N. J., Svetoch, E. A., Eruslanov, B. N., et al. (2006). Bacteriocins reduce Campylobacter colonization and alter gut morphology in turkey poults. Poult. Sci. 85, 1570–1575. doi: 10.1093/ps/85.9.1570
Cotter, P. D., Hill, C., and Ross, R. P. (2005). Bacteriocins: developing innate immunity for food. Nat. Rev. Microbiol. 3, 777–788. doi: 10.1038/nrmicro1273
Cuozzo, S. A., Sesma, F. J., Holgado, A. A. P. D. R., and Raya, R. R. (2001). “Methods for the detection and concentration of bacteriocins produced by lactic acid bacteria,” in Food Microbiology Protocols (Humana Press), 141–146. doi: 10.1385/1-59259-029-2:141
da Costa, R. J., Voloski, F. L., Mondadori, R. G., Duval, E. H., and Fiorentini, Â. M. (2019). Preservation of meat products with bacteriocins produced by lactic acid bacteria isolated from meat. J. Food Qual. 2019, 1–12. doi: 10.1155/2019/4726510
Daw, M. A., and Falkiner, F. R. (1996). Bacteriocins: nature, function and structure. Micron 27, 467–479. doi: 10.1016/S0968-4328(96)00028-5
Deegan, L. H., Cotter, P. D., Hill, C., and Ross, P. (2006). Bacteriocins: biological tools for bio-preservation and shelf-life extension. Int. Dairy J. 16, 1058–1071. doi: 10.1016/j.idairyj.2005.10.026
Delgado, A., Brito, D., Fevereiro, P., Tenreiro, R., and Peres, C. (2005). Bioactivity quantification of crude bacteriocin solutions. J. Microbiol. Methods 62, 121–124. doi: 10.1016/j.mimet.2005.01.006
Devi, P. B., Kavitake, D., Jayamanohar, J., and Shetty, P. H. (2021). Preferential growth stimulation of probiotic bacteria by galactan exopolysaccharide from Weissella confusa KR780676. Food Res. Int. 143:110333.
Diez-Gonzalez, F. (2007). Applications of bacteriocins in livestock. Curr. Issues Intest. Microbiol. 8:15. Available online at: https://www.caister.com/backlist/ciim/v/v8/03.pdf
Dimov, S., Ivanova, P., and Harizanova, N. (2005). Genetics of bacteriocins biosynthesis by lactic acid bacteria. Biotechnol. Biotechnol. Equip. 19, 4–10. doi: 10.1080/13102818.2005.10817270
Dobson, A., Cotter, P. D., Ross, R. P., and Hill, C. (2012). Bacteriocin production: a probiotic trait? Appl. Environ. Microbiol. 78, 1–6. doi: 10.1128/AEM.05576-11
Durgadevi, R., Abirami, G., Swasthikka, R. P., Alexpandi, R., Pandian, S. K., and Ravi, A. V. (2020). Proteomic analysis deciphers the multi-targeting antivirulence activity of tannic acid in modulating the expression of MrpA, FlhD, UreR, HpmA and Nrp system in Proteus mirabilis. Int. J. Biol. Macromol. 165, 1175–1186. doi: 10.1016/j.ijbiomac.2020.09.233
Elalem, N. M. (2021). The diversity of bacteriocin and its antiviral potential: an overview. Egypt. J. Med. Microbiol. 30, 175–180. doi: 10.21608/ejmm.2021.203649
Ennahar, S., Sashihara, T., Sonomoto, K., and Ishizaki, A. (2000). Class IIa bacteriocins: Biosynthesis, structure and activity. FEMS Microbiol. Rev. 24, 85–106. doi: 10.1111/j.1574-6976.2000.tb00534.x
Etayash, H., Norman, L., Thundat, T., Stiles, M., and Kaur, K. (2014). Surface-conjugated antimicrobial peptide leucocin a displays high binding to pathogenic gram-positive bacteria. ACS Appl. Mater. Inter. 6, 1131–1138. doi: 10.1021/am404729c
Fahim, H. A., El Rouby, W. M., El-Gendy, A. O., Khairalla, A. S., Naguib, I. A., and Farghali, A. A. (2017). Enhancement of the productivity of the potent bacteriocin avicin A and improvement of its stability using nanotechnology approaches. Sci. Rep. 7, 1–13. doi: 10.1038/s41598-017-10157-9
Field, D., Considine, K., O'Connor, P. M., Ross, R. P., Hill, C., and Cotter, P. D. (2021). Bio-engineered nisin with increased anti-Staphylococcus and selectively reduced anti-Lactococcus activity for treatment of bovine mastitis. Int. J. Mol. Sci. 22:3480. doi: 10.3390/ijms22073480
Field, D., Cotter, P., Hill, C., and Ross, R. P. (2007). Bacteriocin biosynthesis, structure, and function. Res. Applic. Bacter. 4, 5–41.
Fusco, V., Abriouel, H., Benomar, N., Kabisch, J., Chieffi, D., Cho, G. S., et al. (2018). “Opportunistic food-borne pathogens,” in Food Safety and Preservation (Academic Press), 269–306. doi: 10.1016/B978-0-12-814956-0.00010-X
Fusco, V., Quero, G. M., Cho, G. S., Kabisch, J., Meske, D., Neve, H., et al. (2015). The genus Weissella: taxonomy, ecology and biotechnological potential. Front. Microbiol. 6:155. doi: 10.3389/fmicb.2015.00155
Goh, H. F., and Philip, K. (2015). Purification and characterization of bacteriocin produced by Weissella confusa A3 of dairy origin. PLoS ONE 10:e0140434. doi: 10.1371/journal.pone.0140434
Gonzalez, B., Arca, P., Mayo, B., and Suárez, J. E. (1994). Detection, purification, and partial characterization of plantaricin C, a bacteriocin produced by a Lactobacillus plantarum strain of dairy origin. Appl. Environ. Microbiol. 60, 2158–2163. doi: 10.1128/aem.60.6.2158-2163.1994
Goyal, C., Malik, R. K., and Pradhan, D. (2018). Purification and characterization of a broad spectrum bacteriocin produced by a selected Lactococcus lactis strain 63 isolated from Indian dairy products. J. Food Sci. Technol. 55, 3683–3692. doi: 10.1007/s13197-018-3298-4
Gupta, A., and Tiwari, S. K. (2014). Plantaricin LD1: A bacteriocin produced by food isolate of Lactobacillus plantarum LD1. Appl. Biochem. Biotechnol. 172, 3354–3362. doi: 10.1007/s12010-014-0775-8
Hammami, R., Fernandez, B., Lacroix, C., and Fliss, I. (2013). Anti-infective properties of bacteriocins: an update. Cell. Molec. Life Sci. 70, 2947–2967. doi: 10.1007/s00018-012-1202-3
Harlan, N. P., Kempker, R. R., Parekh, S. M., Burd, E. M., and Kuhar, D. T. (2011). Weissella confusa bacteremia in a liver transplant patient with hepatic artery thrombosis. Transpl. Infect. Dis. 13, 290–293. doi: 10.1111/j.1399-3062.2010.00579.x
Holo, H., Jeknic, Z., Daeschel, M., Stevanovic, S., and Nes, I. F. (2001). Plantaricin W from Lactobacillus plantarum belongs to a new family of two-peptide lantibiotics. Microbiology 147, 643–651. doi: 10.1099/00221287-147-3-643
Ike, Y., Clewell, D. B., Segarra, R. A., and Gilmore, M. S. (1990). Genetic analysis of the pAD1 hemolysin/bacteriocin determinant in Enterococcus faecalis: Tn917 insertional mutagenesis and cloning. J. Bacteriol. 172, 155–163. doi: 10.1128/jb.172.1.155-163.1990
Immonen, T., and Saris, P. E. J. (1998). Characterization of the nisFEG Operon of the Nisin Z Producing Lactococcus Luctis Subsp. Lactis N8 Strain. DNA Sequence 9, 263–274. doi: 10.3109/10425179809008466
Kaiser, A. L. (2014). “Antimicrobial proteins: classification, nomenclature, diversity, and relationship to bacteriocins,” in Bacteriocins of Lactic Acid Bacteria, 1–12.
Kamboj, K., Vasquez, A., and Balada-Llasat, J. M. (2015). Identification and significance of Weissella species infections. Front. Microbiol. 6:1204. doi: 10.3389/fmicb.2015.01204
Kang, H. K., Oh, J. S., and Kim, D. (2009). Molecular characterization and expression analysis of the glucansucrase DSRWC from Weissella cibaria synthesizing a α (1 → 6) glucan. FEMS Microbiol. Lett. 292, 33–41. doi: 10.1111/j.1574-6968.2008.01460.x
Kaur, R., and Tiwari, S. K. (2018). Membrane-acting bacteriocin purified from a soil isolate Pediococcus pentosaceus LB44 shows broad host-range. Biochem. Biophys. Res. Commun. 498, 810–816. doi: 10.1016/j.bbrc.2018.03.062
Kavitake, D., Devi, P. B., and Shetty, P. H. (2020). Overview of exopolysaccharides produced by Weissella genus-A review. Int. J. Biol. Macromol. 164, 2964–2973. doi: 10.1016/j.ijbiomac.2020.08.185
Kavitake, D., Devi, P. B., Singh, S. P., and Shetty, P. H. (2016). Characterization of a novel galactan produced by Weissella confusa KR780676 from an acidic fermented food. Int. J. Biol. Macromol. 86, 681–689. doi: 10.1016/j.ijbiomac.2016.01.099
Klaenhammer, T. R. (1993). Genetics of bacteriocins produced by lactic acid bacteria. FEMS Microbiol. Rev. 12, 39–85. doi: 10.1016/0168-6445(93)90057-G
Klaenhammer, T. R., Ahn, C., and Muriana, P. M. (1994). Lactacin F, a small hydrophobic heat-stable bacteriocin from Lactobacillus johnsonii. Bacterioc Lactic Acid Bacter. 15, 377–396. doi: 10.1007/978-1-4615-2668-1_14
Kotelnikova, E. A., and Gelfand, M. S. (2002). Bacteriocin production by Gram-positive bacteria and the mechanisms of transcriptional regulation. Russ. J. Genet. 38, 628–641. doi: 10.1023/A:1016035700012
Kumar, B., Balgir, P. P., Kaur, B., Mittu, B., and Chauhan, A. (2012). In vitro cytotoxicity of native and rec-pediocin CP2 against cancer cell lines: a comparative study. Pharmac. Analyt. Acta 1, 3–8. doi: 10.4172/2153-2435.1000183
Kumariya, R., Garsa, A. K., Rajput, Y. S., Sood, S. K., Akhtar, N., and Patel, S. (2019). Bacteriocins: classification, synthesis, mechanism of action and resistance development in food spoilage causing bacteria. Microb. Pathog. 128, 171–177. doi: 10.1016/j.micpath.2019.01.002
Kwak, S. H., Cho, Y. M., Noh, G. M., and Om, A. S. (2014). Cancer preventive potential of kimchi lactic acid bacteria (Weissella cibaria, Lactobacillus plantarum). J. Cancer Prev. 19:253. doi: 10.15430/JCP.2014.19.4.253
Lajis, A. F. B. (2020). Biomanufacturing process for the production of bacteriocins from Bacillaceae family. Bioresour. Bioproc. 7, 1–26. doi: 10.1186/s40643-020-0295-z
Lee, J. S., Lee, K. C., Ahn, J. S., Mheen, T. I., Pyun, Y. R., and Park, Y. H. (2002). Weissella koreensis sp. nov., isolated from kimchi. Int. J. System. Evolut. Microbiol. 52, 1257–1261. doi: 10.1099/00207713-52-4-1257
Lee, K. W., Park, J. Y., Jeong, H. R., Heo, H. J., Han, N. S., and Kim, J. H. (2012). Probiotic properties of Weissella strains isolated from human faeces. Anaerobe 18, 96–102. doi: 10.1016/j.anaerobe.2011.12.015
LeLay, C., Coton, E., Le Blay, G., Chobert, J. M., Haertl,é, T., Choiset, Y., et al. (2016). Identification and quantification of antifungal compounds produced by lactic acid bacteria and propioni bacteria. Int. J. Food Microbiol. 239, 79–85. doi: 10.1016/j.ijfoodmicro.2016.06.020
Leong, K. H., Chen, Y. S., Lin, Y. H., Pan, S. F., Yu, B., Wu, H. C., et al. (2013). Weissellicin L, a novel bacteriocin from siansianzih isolated Weissella hellenica 4-7. J. Appl. Microbiol. 115, 70–76. doi: 10.1111/jam.12218
Li, S. W., Chen, Y. S., Lee, Y. S., Yang, C. H., Srionnual, S., Wu, H. C., et al. (2017). Comparative genomic analysis of bacteriocin producing Weissella cibaria 110. Appl. Microbiol. Biotechnol. 101, 1227–1237. doi: 10.1007/s00253-016-8073-8
Liptáková, D., Matejčeková, Z., and Valík, L. (2017). Lactic acid bacteria and fermentation of cereals and pseudocereals. Ferment. Proc. 10:65459. doi: 10.5772/65459
Liu, W., Pang, H., Zhang, H., and Cai, Y. (2014). “Biodiversity of lactic acid bacteria,” in Lactic Acid Bacteria: Fundamentals and Practice, eds. W. Liu, H. Pang, H. Zhang, and Y. Cai (Amsterdam, The Netherlands: Springer), 103–203. doi: 10.1007/978-94-017-8841-0_2
Loutfi, H., Pellen, F., Le Jeune, B., Lteif, R., Kallassy, M., Le Brun, G., et al. (2020). Real-time monitoring of bacterial growth kinetics in suspensions using laser speckle imaging. Sci. Rep. 10, 1–10. doi: 10.1038/s41598-019-57281-2
Ma, J., Yu, W., Hou, J., Han, X., Shao, H., and Liu, Y. (2020). Characterization and production optimization of a broad-spectrum bacteriocin produced by Lactobacillus casei KLDS 1.0338 and its application in soybean milk biopreservation. Int. J. Food Proper. 23, 677–692. doi: 10.1080/10942912.2020.1751656
Mahadeo, M., and Tatini, S. R. (1994). The potential use of nisin to control Listeria monocytogenes in poultry. Lett. Appl. Microbiol. 18, 323–326. doi: 10.1111/j.1472-765X.1994.tb00879.x
Mahrous, H., Mohamed, A., Abd El-Mongy, M., El-Batal, A. I., and Hamza, H. A. (2013). Study bacteriocin production and optimization using new isolates of Lactobacillus spp. isolated from some dairy products under different culture conditions. Food Nutr. Sci. 4:342. doi: 10.4236/fns.2013.43045
Makras, L., and De Vuyst, L. (2006). The in vitro inhibition of Gram-negative pathogenic bacteria by Bifidobacteria is caused by the production of organic acids. Int. Dairy J. 16, 1049–1057. doi: 10.1016/j.idairyj.2005.09.006
Malik, A., Yuliantie, E., Suprahman, N. Y., Linardi, T., Widiyanti, A. W., Haldy, J., et al. (2021). Construction and Functional Analysis of the Recombinant Bacteriocins Weissellicin-MBF from Weissella confusa MBF8-1. Curr. Pharm. Biotechnol. 22, 115–122. doi: 10.2174/1389201021666200611111040
Mandal, V., Sen, S. K., and Mandal, N. C. (2011). Isolation and characterization of pediocin NV 5 producing Pediococcus acidilactici LAB 5 from vacuum-packed fermented meat product. Indian J. Microbiol. 51, 22–29. doi: 10.1007/s12088-011-0070-0
Mantovani, H. C., Cruz, A. M. O., and Paiva, A. D. (2011). “Bacteriocin activity and resistance in livestock pathogens,” in Science Against Microbial Pathogens: Communicating Current Research and Technological Advances, ed. A., Méndez-Vilas (Badajoz, Spain: Formatex Research Center), 853–863.
Martinez, F. A. C., Balciunas, E. M., Converti, A., Cotter, P. D., and de Souza Oliveira, R. P. (2013). Bacteriocin production by Bifidobacterium spp. a review. Biotechnol. Adv. 31, 482–488. doi: 10.1016/j.biotechadv.2013.01.010
Masuda, Y., Perez, R. H., Zendo, T., and Sonomoto, K. (2016). Nutrition adaptive control of multiple bacteriocin production by Weissella hellenica QU 13. J. Appl. Microbiol. 120, 70–79. doi: 10.1111/jam.12997
Masuda, Y., Zendo, T., Sawa, N., Perez, R. H., Nakayama, J., and Sonomoto, K. (2012). Characterization and identification of weissellicin Y and weissellicin M, novel bacteriocins produced by Weissella hellenica QU 13. J. Appl. Microbiol. 112, 99–108. doi: 10.1111/j.1365-2672.2011.05180.x
Mathur, H., Field, D., Rea, M. C., Cotter, P. D., Hill, C., and Ross, R. P. (2017). Bacteriocin-antimicrobial synergy: a medical and food perspective. Front. Microbiol. 8:1205. doi: 10.3389/fmicb.2017.01205
McAuliffe, O., Ross, R. P., and Hill, C. (2001). Lantibiotics: structure, biosynthesis and mode of action. FEMS Microbiol. Rev. 25, 285–308. doi: 10.1111/j.1574-6976.2001.tb00579.x
Meade, E., Slattery, M. A., and Garvey, M. (2020). Bacteriocins, potent antimicrobial peptides and the fight against multi drug resistant species: resistance is futile? Antibiotics 9:32. doi: 10.3390/antibiotics9010032
Millette, M., Cornut, G., Dupont, C., Shareck, F., Archambault, D., and Lacroix, M. (2008). Capacity of human nisin-and pediocin-producing lactic acid bacteria to reduce intestinal colonization by vancomycin-resistant enterococci. Appl. Environ. Microbiol. 74, 1997–2003. doi: 10.1128/AEM.02150-07
Mokoena, M. P. (2017). Lactic acid bacteria and their bacteriocins: Classification, biosynthesis and applications against uropathogens: a mini-review. Molecules 22:1255. doi: 10.3390/molecules22081255
Montville, T. J., and Kaiser, A. L. (1993). “Antimicrobial proteins: classification, nomenclature, diversity, and relationship to bacteriocins,” in Bacteriocins of lactic acid bacteria (Academic Press), 1–22. doi: 10.1016/B978-0-12-355510-6.50009-9
Moumen, A., Azizi, G., Chekroun, K. B., and Baghour, M. (2016). The effects of livestock methane emission on the global warming: a review. Int. J. Global Warming 9, 229–253. doi: 10.1504/IJGW.2016.074956
Mulders, J. W., Boerrigter, I. J., Rollema, H. S., siezen, R. J., and de Vos, W. M. (1991). Identification and characterization of the lantibiotic nisin Z, a natural nisin variant. Eur. J. Biochem. 201, 581–584. doi: 10.1111/j.1432-1033.1991.tb16317.x
Naidu, A. S., Bidlack, W. R., and Clemens, R. A. (1999). Probiotic spectra of lactic acid bacteria (LAB). Crit. Rev. Food Sci. Nutr. 39, 13–126. doi: 10.1080/10408699991279187
Natrajan, N., and Sheldon, B. W. (2000a). Efficacy of nisin-coated polymer films to inactivate Salmonella typhimurium on fresh broiler skin. J. Food Prot. 63, 1189–1196. doi: 10.4315/0362-028X-63.9.1189
Natrajan, N., and Sheldon, B. W. (2000b). Inhibition of Salmonella on poultry skin using protein-and polysaccharide-based films containing a nisin formulation. J. Food Prot. 63, 1268–1272. doi: 10.4315/0362-028X-63.9.1268
Ndagano, D., Lamoureux, T., Dortu, C., Vandermoten, S., and Thonart, P. (2011). Antifungal activity of 2 lactic acid bacteria of the Weissella genus isolated from food. J. Food Sci. 76, M305–M311. doi: 10.1111/j.1750-3841.2011.02257.x
Nes, I. F., Yoon, S. S., and Diep, D. B. (2007). Ribosomally synthesiszed antimicrobial peptides (bacteriocins) in lactic acid bacteria: a review. Food Sci. Biotechnol. 16, 675–690.
Newstead, L. L., Varjonen, K., Nuttall, T., and Paterson, G. K. (2020). Staphylococcal-produced bacteriocins and antimicrobial peptides: their potential as alternative treatments for Staphylococcus aureus infections. Antibiotics 9:40. doi: 10.3390/antibiotics9020040
Ng, Z. J., Zarin, M. A., Lee, C. K., and Tan, J. S. (2020). Application of bacteriocins in food preservation and infectious disease treatment for humans and livestock: a review. RSC Adv. 10, 38937–38964. doi: 10.1039/D0RA06161A
Noda, M., Miyauchi, R., Danshiitsoodol, N., Matoba, Y., Kumagai, T., and Sugiyama, M. (2018). Expression of genes involved in bacteriocin production and self-resistance in Lactobacillus brevis 174A is mediated by two regulatory proteins. Appl. Environ. Microbiol. 84, e02707–17. doi: 10.1128/AEM.02707-17
Ogier, J. C., and Serror, P. (2008). Safety assessment of dairy microorganisms: the Enterococcus genus. Int. J. Food Microbiol. 126, 291–301. doi: 10.1016/j.ijfoodmicro.2007.08.017
O'Shea, E. F., Cotter, P. D., Stanton, C., Ross, R. P., and Hill, C. (2012). Production of bioactive substances by intestinal bacteria as a basis for explaining probiotic mechanisms: Bacteriocins and conjugated linoleic acid. Int. J. Food Microbiol. 152, 189–205. doi: 10.1016/j.ijfoodmicro.2011.05.025
O'sullivan, L., Ross, R. P., and Hill, C. (2002). Potential of bacteriocin-producing lactic acid bacteria for improvements in food safety and quality. Biochimie 84, 593–604. doi: 10.1016/S0300-9084(02)01457-8
Paiva, A. D., de Oliveira, M. D., de Paula, S. O., Baracat-Pereira, M. C., Breukink, E., and Mantovani, H. C. (2012). Toxicity of bovicin HC5 against mammalian cell lines and the role of cholesterol in bacteriocin activity. Microbiology 158, 2851–2858. doi: 10.1099/mic.0.062190-0
Pal, A., and Ramana, K. V. (2010). Purification and characterization of bacteriocin from Weissella paramesenteroides DFR-8, an isolate from cucumber (Cucumis sativus). J. Food Biochem. 34, 932–948. doi: 10.1111/j.1745-4514.2010.00340.x
Papagianni, M. (2012). Effects of dissolved oxygen and pH levels on weissellin A production by Weissella paramesenteroides DX in fermentation. Bioprocess Biosyst. Eng. 35, 1035–1041. doi: 10.1007/s00449-012-0689-1
Papagianni, M., and Papamichael, E. M. (2011). Purification, amino acid sequence and characterization of the class IIa bacteriocin weissellin A, produced by Weissella paramesenteroides DX. Bioresour. Technol. 102, 6730–6734. doi: 10.1016/j.biortech.2011.03.106
Papagianni, M., and Sergelidis, D. (2013). Effects of the presence of the curing agent sodium nitrite, used in the production of fermented sausages, on bacteriocin production by Weissella paramesenteroides DX grown in meat simulation medium. Enzyme Microb. Technol. 53, 1–5. doi: 10.1016/j.enzmictec.2013.04.003
Park, J. A., Tirupathi Pichiah, P. B., Yu, J. J., Oh, S. H., Daily, I. I. I. J. W., et al. (2012). Anti-obesity effect of kimchi fermented with Weissella koreensis OK1-6 as starter in high-fat diet-induced obese C57BL/6J mice. J. Appl. Microbiol. 113, 1507–1516. doi: 10.1111/jam.12017
Perez, R. H., Zendo, T., and Sonomoto, K. (2018). Circular and leaderless bacteriocins: biosynthesis, mode of action, applications, and prospects. Front. Microbiol. 9:2085. doi: 10.3389/fmicb.2018.02085
Pingitore, E. V., Salvucci, E., Sesma, F., and Nader-Macias, M. E. (2007). Different strategies for purification of antimicrobial peptides from lactic acid bacteria (LAB). Commun. Curr. Res. Educ. Top. Trends Appl. Microbiol. 1, 557–568.
Pringsulaka, O., Thongngam, N., Suwannasai, N., Atthakor, W., Pothivejkul, K., and Rangsiruji, A. (2012). Partial characterisation of bacteriocins produced by lactic acid bacteria isolated from Thai fermented meat and fish products. Food Control 23, 547–551. doi: 10.1016/j.foodcont.2011.08.029
Rabelo, C. H. S. (2016). Effect of Lactobacillus and Bacillus subtilis on the fermentative process of corn silage and performance of beef cattle and sheep. Universidade Estadual Paulista Júlio De Mesquita Filho, 1, 123.
Reenen, V. (1998). Isolation, purification and partial characterization of plantaricin 423, a bacteriocin produced by Lactobacillus plantarum. J. Appl. Microbiol. 84, 1131–1137. doi: 10.1046/j.1365-2672.1998.00451.x
Riley, M. A., and Wertz, J. E. (2002). Bacteriocins: evolution, ecology, and application. Ann. Rev. Microbiol. 56, 117–137. doi: 10.1146/annurev.micro.56.012302.161024
Rodríguez, J. M., Martínez, M. I., and Kok, J. (2002). Pediocin PA-1, a wide-spectrum bacteriocin from lactic acid bacteria. Crit. Rev. Food Sci. Nutr. 42, 91–121. doi: 10.1080/10408690290825475
Sahl, H. G., and Bierbaum, G. (1998). Lantibiotics: Biosynthesis and biological activities of uniquely modified peptides from gram-positive bacteria. Ann. Rev. Microbiol. 52, 41–79. doi: 10.1146/annurev.micro.52.1.41
Sharma, B. R., Halami, P. M., and Tamang, J. P. (2021). Novel pathways in bacteriocin synthesis by lactic acid bacteria with special reference to ethnic fermented foods. Food Sci. Biotechnol. 31, 1–16. doi: 10.1007/s10068-021-00986-w
Sharma, S., Kandasamy, S., Kavitake, D., and Shetty, P. H. (2018). Probiotic characterization and antioxidant properties of Weissella confusa KR780676, isolated from an Indian fermented food. LWT 97, 53–60. doi: 10.1016/j.lwt.2018.06.033
Sharma, V., Ranveer, R. C., Jain, N., and Aseri, G. K. (2019). Bacteriocins: Production, different strategies of purification and applications. Int. J. Res. Pharmac. Sci. 10, 1808–1817. doi: 10.26452/ijrps.v10i3.1376
Sidek, N. L. M., Halim, M., Tan, J. S., Abbasiliasi, S., Mustafa, S., and Ariff, A. B. (2018). Stability of bacteriocin-like inhibitory substance (BLIS) produced by Pediococcus acidilactici kp10 at different extreme conditions. Biomed. Res. Int. 2018:5973484. doi: 10.1155/2018/5973484
Silva, C. C., Silva, S. P., and Ribeiro, S. C. (2018). Application of bacteriocins and protective cultures in dairy food preservation. Front. Microbiol. 9:594. doi: 10.3389/fmicb.2018.00594
Simons, A., Alhanout, K., and Duval, R. E. (2020). Bacteriocins, antimicrobial peptides from bacterial origin: Overview of their biology and their impact against multidrug-resistant bacteria. Microorganisms 8:639. doi: 10.3390/microorganisms8050639
Skaugen, M., Cintas, L. M., and Nes, I. F. (2003). “Genetics of bacteriocin production in lactic acid bacteria,” in Genetics of lactic acid bacteria (Boston, MA: Springer), 225–260. doi: 10.1007/978-1-4615-7090-5_8
Soltani, S., Hammami, R., Cotter, P. D., Rebuffat, S., Said, L. B., Gaudreau, H., et al. (2020). Bacteriocins as a new generation of antimicrobials: Toxicity aspects and regulations. FEMS Microbiol. Rev. 45:fuaa039. doi: 10.1093/femsre/fuaa039
Srionnual, S., Yanagida, F., Lin, L. H., Hsiao, K. N., and Chen, Y. S. (2007). Weissellicin 110, a newly discovered bacteriocin from Weissella cibaria 110, isolated from plaa-som, a fermented fish product from Thailand. Appl. Environ. Microbiol. 73, 2247–2250. doi: 10.1128/AEM.02484-06
Sturino, J. M. (2018). Literature-based safety assessment of an agriculture-and animal associated microorganism: Weissella confusa. Regulat. Toxicol. Pharmacol. 95, 142–152. doi: 10.1016/j.yrtph.2018.03.013
Swetwiwathana, A., Sawa, N., Zendo, T., Nakayama, J., and Sonomoto, K. (2008). “A newly discovered bacteriocin from Weissella cibaria KMITL-QU 21 associated in traditional Thai fermented meat-rice sausage (Sai-krog Isan),” in The 54th International Congress of Meat Science and Technology (ICoMST) Proceedings (in CD).
Teixeira, C. G., Fusieger, A., Milião, G. L., Martins, E., Drider, D., Nero, L. A., et al. (2021). Weissella: An emerging bacterium with promising health benefits. Probiotics Antimicrob. Proteins 13, 915–925. doi: 10.1007/s12602-021-09751-1
Tenea, G. N., and Lara, M. I. (2019). Antimicrobial compounds produced by Weissella confusa Cys2-2 strain inhibit Gram-negative bacteria growth. J. Food 17, 105–111. doi: 10.1080/19476337.2018.1561520
Thuy, T. T. D., Lu, H. F., Bregente, C. J. B., Huang, F. C. A., Tu, P. C., and Kao, C. Y. (2024). Characterization of the broad-spectrum antibacterial activity of bacteriocin-like inhibitory substance-producing probiotics isolated from fermented foods. BMC Microbiol. 24:85. doi: 10.1186/s12866-024-03245-0
Todorov, S. D., Kang, H. J., Ivanova, I. V., and Holzapfel, W. H. (2020). Bacteriocins from LAB and other alternative approaches for the control of Clostridium and Clostridiodes related gastrointestinal colitis. Front. Bioeng. Biotechnol. 8:581778. doi: 10.3389/fbioe.2020.581778
Umu, Ö. C., Bäuerl, C., Oostindjer, M., Pope, P. B., Hernández, P. E., Pérez-Martínez, G., et al. (2016). The potential of class II bacteriocins to modify gut microbiota to improve host health. PLoS ONE 11:e0164036. doi: 10.1371/journal.pone.0164036
Walsh, M. C., Gardiner, G. E., Hart, O. M., Lawlor, P. G., Daly, M., Lynch, B., et al. (2008). Predominance of a bacteriocin-producing Lactobacillus salivarius component of a five-strain probiotic in the porcine ileum and effects on host immune phenotype. FEMS Microbiol. Ecol. 64, 317–327. doi: 10.1111/j.1574-6941.2008.00454.x
Wang, Y., Qin, Y., Xie, Q., Zhang, Y., Hu, J., and Li, P. (2018). Purification and characterization of plantaricin LPL-1, a novel class IIa bacteriocin produced by Lactobacillus plantarum LPL-1 isolated from fermented fish. Front. Microbiol. 9:2276. doi: 10.3389/fmicb.2018.02276
Welch, T. J., and Good, C. M. (2013). Mortality associated with Weissellosis (Weissella sp.) in USA farmed rainbow trout: potential for control by vaccination. Aquaculture 388, 122–127. doi: 10.1016/j.aquaculture.2013.01.021
Woraprayote, W., Pumpuang, L., Tosukhowong, A., Roytrakul, S., Perez, R. H., Zendo, T., et al. (2015). Two putatively novel bacteriocins active against Gram-negative food borne pathogens produced by Weissella hellenica BCC 7293. Food Control 55, 176–184. doi: 10.1016/j.foodcont.2015.02.036
Wu, H. C., Srionnual, S., Yanagida, F., and Chen, Y. S. (2015). Detection and characterization of weissellicin 110, a bacteriocin produced by Weissella cibaria. Iran. J. Biotechnol. 13:63. doi: 10.15171/ijb.1159
Wu, J., Hu, S., and Cao, L. (2007). Therapeutic effect of nisin Z on subclinical mastitis in lactating cows. Antimicrob. Agents Chemother. 51, 3131–3135. doi: 10.1128/AAC.00629-07
Yaafi'Al-Hammam, M., Putra, M. P., Mardinsyah, A. H., Cahyati, G., and Puspita, I. D. (2023). The antibacterial activity of Lactobacillus sp. GMP1 and Weisella sp. GMP12 against some foodborne disease causing-bacteria. J. Pengol. Hasil Perik. Indonesia 26, 206–215. doi: 10.17844/jphpi.v26i2.44618
Yang, E., Fan, L., Yan, J., Jiang, Y., Doucette, C., Fillmore, S., et al. (2018). Influence of culture media, pH and temperature on growth and bacteriocin production of bacteriocinogenic lactic acid bacteria. AMB Express 8:10. doi: 10.1186/s13568-018-0536-0
Yang, S. C., Lin, C. H., Sung, C. T., and Fang, J. Y. (2014). Antibacterial activities of bacteriocins: application in foods and pharmaceuticals. Front. Microbiol. 5:241. doi: 10.3389/fmicb.2014.00241
Yusuf, M. A., and Hamid, T. H. (2013). Lactic acid bacteria: Bacteriocin producer: a mini review. IOSR J. Pharmacy 3, 44–50. doi: 10.9790/3013-034104450
Zacharof, M. P., and Lovitt, R. W. (2012). Bacteriocins produced by lactic acid bacteria a review article. Apcbee Proc. 2, 50–56. doi: 10.1016/j.apcbee.2012.06.010
Zendo, T., Koga, S., Shigeri, Y., Nakayama, J., and Sonomoto, K. (2006). Lactococcin Q, a novel two-peptide bacteriocin produced by Lactococcus lactis QU 4. Appl. Environ. Microbiol. 72, 3383–3389. doi: 10.1128/AEM.72.5.3383-3389.2006
Zheng, J., Wittouck, S., Salvetti, E., Franz, C. M., Harris, H. M., Mattarelli, P., et al. (2020). A taxonomic note on the genus Lactobacillus: Description of 23 novel genera, emended description of the genus Lactobacillus beijerinck 1901, and union of Lactobacillaceae and Leuconostocaceae. Int. J. Syst. Evol. Microbiol. 70, 2782–2858. doi: 10.1099/ijsem.0.004107
Zimina, M., Babich, O., Prosekov, A., Sukhikh, S., Ivanova, S., Shevchenko, M., et al. (2020). Overview of global trends in classification, methods of preparation and application of bacteriocins. Antibiotics 9:553. doi: 10.3390/antibiotics9090553
Keywords: Weissella genus, Weissella bacteriocins, biosynthesis, properties, food and health applications
Citation: Singh JK, Devi PB, Reddy GB, Jaiswal AK, Kavitake D and Shetty PH (2024) Biosynthesis, classification, properties, and applications of Weissella bacteriocins. Front. Microbiol. 15:1406904. doi: 10.3389/fmicb.2024.1406904
Received: 25 March 2024; Accepted: 29 May 2024;
Published: 12 June 2024.
Edited by:
Vincenzina Fusco, National Research Council (CNR), ItalyReviewed by:
Dão Neto, Federal Institute of Education, Science, and Technology of Paraná (IFPR), BrazilCopyright © 2024 Singh, Devi, Reddy, Jaiswal, Kavitake and Shetty. This is an open-access article distributed under the terms of the Creative Commons Attribution License (CC BY). The use, distribution or reproduction in other forums is permitted, provided the original author(s) and the copyright owner(s) are credited and that the original publication in this journal is cited, in accordance with accepted academic practice. No use, distribution or reproduction is permitted which does not comply with these terms.
*Correspondence: Digambar Kavitake, ZGlnYW1iYXJrYXZpdGFrZUBnbWFpbC5jb20=; Prathapkumar Halady Shetty, c2hldHR5LmZzdEBwb25kaXVuaS5lZHUuaW4=; cGtzaGFsYWR5QHlhaG9vLmNvLnVr
†ORCID: Digambar Kavitake orcid.org/0000-0002-3031-2174
Prathapkumar Halady Shetty orcid.org/0000-0002-7414-2859
Disclaimer: All claims expressed in this article are solely those of the authors and do not necessarily represent those of their affiliated organizations, or those of the publisher, the editors and the reviewers. Any product that may be evaluated in this article or claim that may be made by its manufacturer is not guaranteed or endorsed by the publisher.
Research integrity at Frontiers
Learn more about the work of our research integrity team to safeguard the quality of each article we publish.