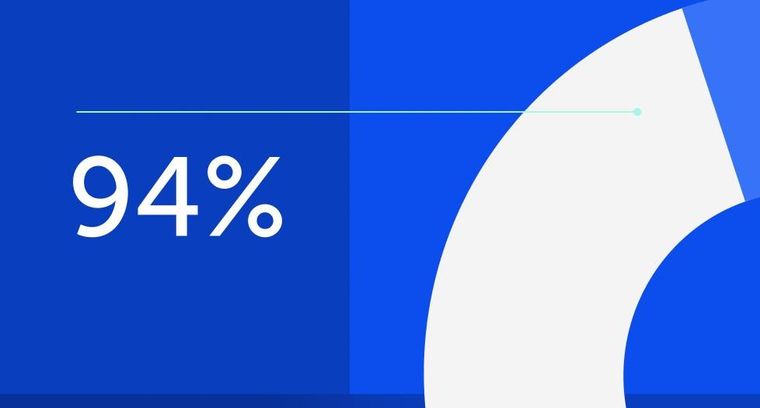
94% of researchers rate our articles as excellent or good
Learn more about the work of our research integrity team to safeguard the quality of each article we publish.
Find out more
REVIEW article
Front. Microbiol., 08 July 2024
Sec. Microbiotechnology
Volume 15 - 2024 | https://doi.org/10.3389/fmicb.2024.1396116
This article is part of the Research TopicPharmaceutically Active Micropollutants – How Serious is the Problem and is there a Microbial Way Out?View all 6 articles
Veterinary medications are constantly being used for the diagnosis, treatment, and prevention of diseases in livestock. However, untreated veterinary drug active compounds are interminably discharged into numerous water bodies and terrestrial ecosystems, during production procedures, improper disposal of empty containers, unused medication or animal feed, and treatment procedures. This exhaustive review describes the different pathways through which veterinary medications enter the environment, discussing the role of agricultural practices and improper disposal methods. The detrimental effects of veterinary drug compounds on aquatic and terrestrial ecosystems are elaborated with examples of specific veterinary drugs and their known impacts. This review also aims to detail the mechanisms by which microbes degrade veterinary drug compounds as well as highlighting successful case studies and recent advancements in microbe-based bioremediation. It also elaborates on microbial electrochemical technologies as an eco-friendly solution for removing pharmaceutical pollutants from wastewater. Lastly, we have summarized potential innovations and challenges in implementing bioremediation on a large scale under the section prospects and advancements in this field.
Pharmaceutical compounds that treat irregularities in animals are veterinary pharmaceutics. Veterinary drugs include biological products, hygienic goods, and products used to treat internal and external parasites as well as disease-transmitting vectors in animals. Veterinary drugs can also refer to any chemical or combination of compounds used in the diagnosis, treatment, or prevention of animal illness. Veterinary medicines are frequently used to treat illness and safeguard an animal’s health. Animals raised for food are also fed dietary boosting feed additives like growth promoters to accelerate their growth rates. When veterinary drugs are used in fish farms, for example, they are released into the environment both directly and indirectly when animal dung containing excreted products are spread on the ground. Every year, the production and use of pharmaceuticals increases significantly worldwide. The global consumption of different active compounds is expected to reach 100,000 tons or more per year. The concentration of antibiotics in wastewater is determined to be less than 1–150 μg/L, which may be increased further by nonmedical usage of antibiotics, such as agricultural, animal feeding activities, and so on (Singh and Saluja, 2021). According to long-term and multicentric research conducted by Klein et al. (2018), antibiotic consumption grew by 65% from 2000 to 2015, with a worrisome increase from 21 billion to over 35 billion specified daily doses. Concern over the destiny and impacts of these substances on the environment has increased along with this expansion. Low quantities of pharmaceutical chemicals are being found in ground and surface waters more often (Dar et al., 2019). Even though pharmaceutical ambient concentrations have been observed to date being far lower than the intended therapeutic dosages, it’s a global concern that these chemicals may have a negative effect on aquatic life and human health (Menzir and Adeladlw, 2022). Active raw components may be discharged during the production of an active pharmaceutical component and formulation of the completed drug product into the air, water, or land in the form of solid waste (Figure 1). Drugs end up in the environment during production primarily through the removal of functional pharmaceutical ingredients and coating equipment, mixing, compressing, as well as packaging tablets (Larsson, 2014; Singh and Saluja, 2021). Prior to the discharge of wastewater or sewage solids to surface waterways, effluent cleaning facilities, or ground, it is considered that the majority of drug residues are removed by biological and chemical degradation processes such biotransformation, mineralization, hydrolysis, and photolysis (Oluwole et al., 2020; Samal et al., 2022).
Veterinarians, animal owners, or domestic consumers are the main veterinary medication end users (Mutua et al., 2020). Veterinary pharmaceuticals may need to be disposed of at any point in their lifespan. Like with human drugs, it is probably reasonable to predict that some prescribed or over-the-counter veterinary medications may go unused by the intended patient. Disposal of veterinary pharmaceuticals should cautiously be taken into account by end users. Things like broken and expired veterinary medicines along with disposable boxes and packages, contaminated surgical instruments, gloves and bandages should also be taken into consideration (Rutala and Weber, 2015; Menzir and Adeladlw, 2022).
Previous studies on antibiotics degradation promisingly showed that living organisms such as bacteria, fungi, algae and plants are good for successful mitigation of these compounds. But not all the antibiotics can be degraded using a single microorganism because each veterinary pharmaceutical compound (e.g., antibiotics) varies in size from molecular to biological aspect like inoculum size, organism strain and different culture conditions. However, present mitigation processes like microbial fuel cell, saw dust and biochar have the hopeful potential in the possible removal of these pharmaceutics (Patwardhan et al., 2022). Many studies suggest that more efficient elimination of recalcitrant antibiotics can be achieved by combining different processes (Sodhi and Singh, 2022).
The aim of the present review is to understand the types and sources of veterinary pharmaceuticals, their fate in different environmental niches as well as their potential of causing antimicrobial resistance. We have also highlighted here the microbial way out for their removal through in situ and ex situ remediation or enzymatic degradation. The review also includes a brief on the upcoming cutting-edge technology of microbial electrochemical method for wastewater treatment, and the future challenges, making the paper a cornerstone resource for researchers in their work.
Veterinary pharmaceuticals can enter the environment through various pathways. Their fate involves processes such as degradation, transport and accumulation, impacting ecosystems and potentially leading to long-term environmental implications.
Large amounts of farmyard manure (animal urine and feces together with contaminated bedding material) and/or slurry (urine, feces, and washing-down water) are generated on livestock farms where animals are housed. Both can be used as an immediate organic matter supplement and fertilizer or kept in manure pits for later use (Velagaleti et al., 2003). Although there is no information on the fate of the degradation products, sulfonamides, aminoglycosides, lactams, and macrolides that have half-lives of 30 days are expected to be considerably degraded during manure/slurry storage. Ivermectin, tetracyclines, and quinolones, on the other hand, have longer half-lives and are therefore probably more persistent (Boxall et al., 2004). On the other hand, enormous amounts of farmyard manure and/or slurry are produced on livestock farms. Typically, this is collected and stored in manure pits for later application or applied to the ground right away. Slurry can be kept for a long time in storage. The time for storage of manure and slurry on an average is usually between 0 and 48 months with an average of 6 months (Gros et al., 2019).
Aerobic soil biodegradation is the primary method by which veterinary drugs are broken down in soils. Different medications degrade at different degrees in soil, and their t ½ can range from days to months. In a study conducted by Topp et al. (2016), the half-life of azithromycin in soil was 12.8 days with the application of azithromycin at concentration 10 mg/kg soil. According to Liu et al. (2015), chlortetracycline was degraded in 4.7 days for 100 mg/kg of soil. A study conducted on soil from wheat field in china by Duan et al. (2017), reported that ofloxacin was degraded 85.6 and 87.3% with 10% compost and 10% compost with 2% of biochar. Oxytetracycline was degraded 86.6, 89.6, 93.7, and 95.4% at concentrations of 1, 3.6, 10, and 30 mg/kg of soil (Ma et al., 2016). Environmental factors that affect the degradation of veterinary medicines include temperature, pH, soil type, soil-organic carbon, nutrient conditions, and the presence of degrading bacteria that have evolved to destroy classes of medicine (Ingerslev et al., 2001). Other modes of chemical breakdown and depletion, such as soil photolysis and hydrolysis, may be significant as well, subject to the nature of the chemical. Since photodegradation is only likely to take place in the top layer of soil, farming practices including the depth and time of any plowing will have an impact on how long photodegradable compounds survive in the environment. In the topsoil layers, breakdown of byproducts of both photolytic and hydrolytic breakdown procedure may go through aerobic biodegradation (Ingerslev et al., 2001). A veterinary drug may deteriorate, leach into groundwater, be transferred to surface waters, or partition to soil particles once it has reached the soil. The weather at the time of application will have an impact on behavior to some extent. The ability of veterinary drugs to bind to soils varies greatly. As a result, there are significant differences in the mobility of several veterinary pharmaceuticals. Variations in soil-organic carbon cannot account for the large differences in distribution of chemical in various soils. In the case of carbadox, the highest known organic-carbon normalized sorption coefficient is around two times higher than the lowest documented value. The fact that several veterinary medications are ionizable around their pKa values while in the natural pH range of soils, helps to explain these significant discrepancies in sorption behavior. Therefore, drugs can exist in the environment as negatively charged, neutrally charged, zwitter ionic charged, and positively charged organisms. Depending on the species, interactions with soil may take the form of hydrophobic interactions, hydrogen bonds at the surface, electrostatic attraction, or surface bridging.
Wastewater treatment plant effluent, are the main sources of contamination by veterinary medicines mostly antibiotic in the environmental compartments mainly surface and underground water (Ezzariai et al., 2018). Due to water shortages driven upon by pollution, urbanization, climate change, and regional droughts, wastewater is frequently utilized to irrigate agricultural land in arid and semi-arid countries. As a result, wastewater is now a useful resource for irrigating agricultural land. Veterinary antibiotics can enter into the ecosystem by way of untreated wastewater discharge into water bodies as lakes and rivers, livestock grazing on land that has been sprayed with manure and slurry, or direct cattle deposition. The pathway of recalcitrant veterinary pharmaceuticals’ entry into environment can be understood with Figure 2. Antibiotics have the potential to build up in soil and then be absorbed by crops when untreated wastewater or animal dung is utilized for agricultural irrigation or fertilization (Pan and Chu, 2017). Monensin concentrations of up to 183 μg/kg have been found in fertilized soils, which could be sources of contamination for surface and groundwater. Antibiotic ionophore values of up to 9 μg/L have also been found in these soils (Delgado et al., 2023). Another route is aquaculture, or fish farming, which often involves the use of antibiotics to prevent or treat bacterial infections in fish populations (Carvalho and Santos, 2016). Antibiotics used in fish farms can enter the surrounding water bodies directly through excretion from treated fish, uneaten medicated feed, or improper disposal of unused medications (de Ilurdoz et al., 2022). Veterinary pharmaceutical residues undergo biotic (biodegradation) or abiotic (hydrolysis or photolysis) processes in water, which can lead to their deterioration and/or transformation. During dry seasons, these degradation mechanisms are more effective because a rise in temperature encourages microbial activity and photolysis (Charuaud et al., 2019). When parent veterinary pharmaceutical compounds degrade, several transformation products may be produced, for example in enrofloxacin and marbofloxacin, photolysis of the fluoroquinolones results in the synthesis of 5 and 2 photo-transformation products, respectively (Sturini et al., 2010).
The use of antibiotics serves as growth promoters in animal farming, such as cattle, pigs, and poultry, as well as to enhance feeding efficiency, particularly in the agriculture and livestock sectors (Cowieson and Kluenter, 2019). Some major veterinary antibiotics and their mode of action, source and proposed use in animal diseases are presented in Table 1. The increased use of all existing antimicrobials for the well-being of humans, animals, and agriculture results in their recurrent and frequent discharge into the environment and ecological systems (Nielsen et al., 2022).
The emergence of antibiotic resistance in every organism is the primary failure of all antibiotics and other pharmaceutical companies. Human and animal health is put at risk due to horizontal gene transfer (resistance) in the bacterial population (Kivits et al., 2018). The antibiotic resistant genes and antibiotic resistant bacteria reduce the curative ability of antibacterial substances toward human and animal infections. As a result, the residues of antibiotics in surface water have the potential to disrupt essential bacterial cycles/mechanisms/processes that are critical to aquatic balance, agricultural balance, and animal productivity (Ribeiro et al., 2018). Secondary sources include wastewater treatment plant effluents, sewage leaks, and agricultural waste, as these chemicals are not completely metabolized and may escape decomposition. Antibiotics have been found in hospital wastewater and wastewater treatment plant effluent, biosolids from wastewater treatment plants, soil, surface and groundwaters, sediments, biota, and drinking water (Barancheshme and Munir, 2018; Williams and Kookana, 2018). Antibiotic overuse, underuse, and abuse are shaping and causing novel incidences of microbial resistance, particularly among bacterial species. The logic for this scenario is long-term antibiotic exposure at low concentrations. Antibiotic resistance is characterized by a rise in the minimum inhibitory concentration of antibiotics against microorganisms. It is a process by which bacteria may withstand the stress of antibiotics. Antibiotic resistance can be carried out by microbes by evading drug-target interactions, efflux of antibiotics from the cell (efflux pump TolC in E. coli), and modification of antibiotics and their metabolites by enzymes (e.g., ADP-ribosyl transferases and glycosyl transferases). These antibiotic modifying enzymes can transfer functional groups that covalently change the antibiotics via acetylation, phosphorylation, adenylation, nucleotidylation, ribosylation, and glycosylation with the help of a co-substrate, ATP (Camotti Bastos et al., 2018).
These implications can result in changes to cell surface receptors, redox processes, hydrolysis, and other such aspects, all leading to MDRS. Under strong antibiotic stress, several types of resistance genes are activated, which eventually becomes a part of regular bacterial machinery and hence generates resistant enzymes even under control environments. The antibiotic fosfomycin, which is used to treat multi-drug resistant bacterial infections, is an active antibiotic against E. coli, Staphylococcus aureus, Streptococcus pneumonia, Enterococcus faecalis, and E. faecium, but it is less effective against Klebsiella pneumonia and Enterobacter cloacae, this lower susceptibility is attributable to mutations in the GipT and UhpT transporters, as well as changes in the antibiotic target MurA enzyme and antibiotic modification by proteins FosA, FosB, or FosX (Lucas et al., 2018; Scortti et al., 2018). Veterinary pharmaceutics like lincomycin and ofloxacin have high risk at the concentrations detected in effluents and medium risk in their receiving water bodies (Liu et al., 2023), resulting in potential hazard to the healthy aquatic ecosystem. Antimicrobial resistant bacteria have grown excessively due to unrestricted practice and for their mitigation, use of alternate strategies other than antibiotics are being explored extensively, like plant based natural compounds (Mathur et al., 2013; Singh et al., 2016), probiotic strains (Rastogi et al., 2021) or use of bacteriophages (Singh et al., 2022; Padmesh et al., 2023).
Antibiotics can undergo both biological and non-biological degradation in a variety of settings, to differing degrees. Photolysis, hydrolysis, oxidative degradation, and ionizing radiation degradation are examples of non-biological degradation, whereas microorganisms, algae, and plants are examples of biodegradation in the natural environment (Li et al., 2019).
Natural biodegradation greatly contributes to the breakdown of antibiotics; bacteria, algae, and plants play a major role in this process. Antibiotic pollution can be made harmless by microorganisms by altering the structure and physicochemical characteristics of antibiotics and breaking down antibiotic residues from macromolecular compounds to small molecule compounds and finally to H2O and CO2. Numerous microbial species, including photosynthetic bacteria, lactic acid bacteria, actinomycetes, yeast, fermentation filamentous bacteria, Bacillus subtilis, and nitrated bacteria, have been found to be capable of breaking down antibiotics in the environment. Tetracycline was reported to be 78% destroyed by a yeast strain that was obtained from the pharmaceutical sewage plant’s discharge sample (Zheng et al., 2022). It has been discovered that crop plants (Cucumis sativus, Lactuca sativa, and Phaseolus vulgaris) may convert approximately 25% of stored enrofloxacin into ciprofloxacin through metabolism (Migliore et al., 2003). In a study conducted by Baena-Nogueras et al. (2017) it was reported that half life of sulfamethazine after biodegradation was 24 days in fresh water and that of Sulphamethoxazole was 13 days post biodegradation in sea water (Baena-Nogueras et al., 2017). Fluoroquinolone was reported to have half life of 10.4 days in river after biodegradation (Caracciolo et al., 2018). Koba et al. (2017) reported the half life of Trimethoprim in soil after biodegradation 13–84% of the initial concentration was degraded. The primary environmental factors that impact the microbial breakdown of antibiotics include temperature, pH, oxygen concentration, and the type of environment. Strong specificity and low cost allow microbial breakdown a feasible remedy for antibiotic contamination.
Veterinary pharmaceuticals can be difficult to treat due to their enormous amount, complex and extremely durable chemical structure, and harmful nature (Kaczala and Blum, 2016). Physical and chemical remediation methods, such as coagulation/flocculation, membrane filtration, adsorption, ion extraction and photodegradation are currently available (Mahmood et al., 2022). However, even sophisticated oxidation procedures such as the use of ozone, hydrogen peroxide, and UV radiation are not always appropriate as they are expensive and may cause secondary pollution (Cuerda-Correa et al., 2019). Table 2 below summarizes the advantages and disadvantages of traditional methods of antibiotic removal from environment. The rising detection of pharmaceuticals and their metabolites in the environment highlights the need for more efficient and low-cost remediation approaches such as bioremediation (Letsoalo et al., 2023).
The use of organisms that occur naturally or genetically designed microorganisms (bacteria and fungus) to consume and break down contaminants in contaminated media such as water, soil, and sediment is known as bioremediation or biological remediation (Singh and Singh, 2022). The definition of bioremediation according to USPEA (United States Environmental Protection Agency) is “an engineered technique that transforms environmental conditions (physical, chemical, biochemical, or microbiological) to promote microbes to destroy or detoxifying organic and inorganic contaminants in the environment.” The process is a well-established technology that has been used for decades to effectively degrade various forms of complex molecules, like chlorine-based solvents or hydrocarbons from petroleum in groundwater and soil. Bioremediation is broadly classified into two categories namely in-situ and ex-situ bioremediation (Figure 3).
Antibiotic degradation is aided by microorganisms found in soil, sewage treatment facilities, and surface water (Patel et al., 2019). Bioremediation by a microbe is an attribute to a special metabolic potential possessed by the organism. Oxidation is a predominant and essential pathway for antibiotic degradation as well as photolysis, characterized by the degradation of the compound upon absorption of light energy, is also another significant mechanism in the breakdown of antibiotics. However, the success of degradation processes is dependent on the chemical nature of the pharmaceutical compound. For instance, tetracycline is smaller than cephalexin and thus more easily broken down by photolysis and UV (Azimi and Nezamzadeh-Ejhieh, 2015), whereas fluoroquinolones are resistant to hydrolysis but susceptible to UV. A number of microbes from all three major categories of bacteria, fungi and algae have been explored extensively for antibiotic bioremediation. For example, members of three prominent groups of filamentous fungi, the ascomycetes, basidiomycetes, and zygomycetes have been prominent in breaking down pollutants. However, it’s the white rot fungi that has been found to be most promising in pharmaceutical bioremediation (Amobonye et al., 2023). The major categories of microorganisms viz. bacteria, fungi and algae employed for pharmaceutical bioremediation (Table 3) are discussed below.
In carrying antibiotics bioremediation, the bacterial population attains antibiotic resistant genes and some studies have shown positive correlation of antibiotic degradation potential with development of resistant genes (Zad et al., 2018). Bacteria are mostly employed in bioremediation. Bacteria utilized in bioremediation can live in harsh conditions; as they have an intrinsic ability to withstand and grow in adverse conditions (Ünal Turhan et al., 2019). Degradation of ciprofloxacin carried out by the mixture of Bacteroidia and Proteobacteria was proposed as well as dealkylation, deamination and C-F bond cleavage were some of the mechanisms by which ciprofloxacin was biodegraded (Liao et al., 2016; Sodhi and Singh, 2022). Chen and Xie (2018) proposed the microbial degradation of sulfonamide drugs in a sewage treatment plant. Several genera of microorganisms have been isolated from and characterized with efficient potential of sulfonamide drugs (Yang et al., 2021). The reactions by which these sulfonamide antibiotics degraded are oxidation, hydroxylation and acetylation. In a work by Zhang et al. (2020) using a model bacterium Lelliottia aquatilis and amoxicillin as a model β-Lactam antibiotic, improved detoxification of antibiotic wastewater was reported using a novel strategy of co-substrate combined with biological process. They have shown amplified activity of key enzymes, β-Lactamase, amidases, transaminase, and amide hydrolase, resulting in increased removal (95%) of amoxicillin with an increase of 60% from control (Zhang et al., 2020). A Paracoccus spp. isolated from sludge is studied for degradation of Penicillin G. Effective biotransformation of tetracycline by a novel strain Stenotrophomona smaltophilia DT1 (Leng et al., 2016) and Klebsiella sp. SQY5 (Shao et al., 2019) is reported.
In a recent study on bacteria mediated removal of antibiotics from wastewater sludge was studied for seven common antibiotics, namely oxytetracycline, tetracycline, chlortetracycline, amoxicillin, sulfamethazine, sulfamethoxazole andsulfadimethoxine. Twenty-four effective antibiotic degrading genera from both aerobic and anaerobic groups were identified from sludge, which had some well-known groups like Acinetobacter, Bacillus, Burkholderia, Corynebacterium, Klebsiella, Mycobacterium, Pseudomonas. However, they have also reported genera belonging to Achromobacter, Acidovorax, Castellaniella, Comamonas, Dechloromonas, Gordonia, Novosphingobium, Pandoraea, Sphingomonas, Treponema and Xanthobacter and some more as potential antibiotic degraders (Yang et al., 2020; Pathak et al., 2023). Batch fermentation using two different strains was found to be more effective than using a single strain. Additionally, these bacteria not only directly degraded antibiotics but also influenced the microbial community composition in the sludge, enhancing antibiotic degradation capacity (Yang et al., 2020).
The biodegradation of veterinary medicines by fungal laccases enzyme, with the most remarkable outcomes obtained with Trametes laccases. T. versicolor laccases, for example, have been demonstrated to bioremediate the antibiotics tetracycline, chlortetracycline, doxycycline, and oxytetracycline (Suda et al., 2012). Trametes polyzona, another species of the same genus, was also shown to breakdown tetracycline, as well as several β-lactam and quinolone antibiotics (Lueangjaroenkit et al., 2019). In a study by Tormo-Budowski et al. (2021), T. versicolor immobilized on lignocellulosic substrate in a stirred tank bioreactor also substantially eliminated 16 PhACs in synthetic wastewater and those naturally occurring in veterinary hospital wastewater, with removal rates of 95.7 and 85.0%, respectively; however, it increased the general toxicity of wastewater matrices after treatment. The scientists then used a similar set up in a trickled bed bioreactor which led to significant removal of veterinary pharmaceutical compounds and detoxify the wastewater too (Tormo-Budowski et al., 2021). Another white rot fungus, P. chrysosporium, was used in a plate bioreactor and was used in both sequence batch and continuous modes to eradicate carbamazepine at substantial clearance rates ranging from 60 to 80%. The study also found that the bioreactor was successful even after running continuously for over 100 days. Apart from white-rot fungus, Penicillium oxalicum, an ascomycete, was employed for diclofenac biological remediation in a bench bioreactor, with cytochrome P450 enzyme activation playing important roles in its bioremediation endeavor (Olicón-Hernández et al., 2017). Similarly, a trickle-bed bioreactor based on various fungal biomass immobilized on rice husks was recently shown to eliminate 88.6 and 89.8% of PhACs in synthetic and real wastewater, respectively, and it was also demonstrated that adsorption was an important physical phenomenon in the trickle-bed bioreactor’s veterinary drug elimination effectiveness (Tormo-Budowski et al., 2021). The study conducted by Lucas et al. (2016), assessed the efficacy of a fungal treatment as a substitute veterinary wastewater treatment method for the removal of 47 veterinary antibiotics that fall into seven categories: β-lactams, trimethoprim, tetracyclines, macrolides, metronidazoles, sulfonamides, and fluoroquinolones. Following the fungal treatment, 77% of antibiotics were eliminated, which is more as compared to traditional treatment methods.
In this context, a wide range of fungal enzymes, including laccases, peroxidases, cytochrome P450 mixed function oxidases, lipases, and esterases, have been identified for their functions in degrading veterinary medicines. Fungal enzymes have been characterized as having extraordinary potential in the degradation of veterinary active compounds found in a variety of waste streams under different conditions. As a result, they provide a more cost-effective and ecologically friendly alternative to traditional treatment procedures (Vaksmaa et al., 2023). This amazing capacity has been attributed to their resilience, which enables them to breakdown complicated chemical structures into simpler and less hazardous compounds that may be digested further by other microbes (Rathore et al., 2022). The fungal enzymes have been found to aid in the biodegradation of nonpolar and poorly soluble pharmaceutically active veterinary compounds and other xenobiotics in organic solvents (Espinosa-Ortiz et al., 2021). These enzymes alter and detoxify medicines by reduction, oxidation, hydroxylation, dehalogenation, dehydrogenation, deamination, formylation, and other processes (Ferrando-Climent et al., 2015; Narayanan et al., 2022).
Aquatic environments are the principal producers of algae. Because of their short development cycle, high sensitivity to aquatic pollutants, and ability to begin stress response mechanisms, they can be employed as ecological indicators to eliminate pharmaceutical pollutants (Rodríguez Sánchez et al., 2015). Recently, algae-based techniques have been widely used for the treatment of antibiotic-containing wastewater, providing numerous benefits such as efficient CO2 fixation, low environmental impact, solar energy-driven activity, and the production of a potential raw material for the generation of biofuel or other high-value by-products (Xiong et al., 2021). For example, Nannochloris can entirely eliminate triclosan from water after 7 days of culture (Bai and Acharya, 2016), and it can efficiently digest diphenhydramine, memantine, and trihexyphenidyl, with average degradation rates of 88, 59, and 83%, respectively (Gojkovic et al., 2019). Organic pharmaceutical pollutants such as pesticides and antibiotics might be removed from groundwater using microalgae-mediated technology (up to 65%) (Ferrando and Matamoros, 2020). Similarly, Wang et al. (2021) introduced green alga Scenedesmus obliquus into wastewater to examine the erythromycin degradation routes. After 5 days of cultivation, the main mechanisms of erythromycin degradation in the microalgae-mediated system were biodegradation (including bioadsorption), hydrolysis, and photolysis, with biodegradation responsible for the highest proportion of removal (57.87%), followed by hydrolysis (34.13%), and photolysis (5%). It has previously been reported that Chlamydomonas sp. Tai-03 can entirely remove ciprofloxacin, with biodegradation accounting for up to 65.05% of clearance (Xie et al., 2020). According to Sutherland and Ralph, algae from the genera Scenedesmus, Chlorella, and Chlamydomonas are the species most explored and widely used for antibiotic bioremediation. Microalgae exhibit three primary pathways, bioadsorption, bio-uptake, and biodegradation for the bioremediation of pharmaceutical compounds, through which complex compounds undergo catalytic metabolic degradation. Furthermore, because microalgal cells are negatively charged and hydrophilic, lipophilic cationic chemicals show strong bioadsorption affinity with microalgae (Sutherland and Ralph, 2019). Ndlela et al. (2021) have demonstrated that Chlorella protothecoides and Chlorella vulgaris are effective in removing sulfamethoxazole (77.3 and 46.5%) and ofloxacin (43.5 and 55.1%) from samples containing 10 and 100 ppb concentrations, respectively.
Enzyme-mediated bioremediation is the process of cleaning polluted locations by using naturally existing enzymes found in microorganisms or plants to break down or eliminate harmful, unwanted, and resistant environmental contaminants (Karigar and Rao, 2011). Enzyme mediated bioremediation plays a significant part in the breakdown of antibiotics, which is crucial for the development of antibacterial resistance mechanisms. A correlation exists between the development of antibiotic-degraded enzymes, antibiotic-resistant genes, and antibiotic-resistant microorganisms. Study by Wencewicz (2019) shows that antibiotic resistance genes that express beta-lactamase enzymes and modify aminoglycosides existed long before the environment was exposed to synthetic antibiotics for medicinal purposes (Wang et al., 2020).
Enzymes are smaller than microbial cells, which makes it easier for them to come into touch with pollutants (Sharma et al., 2018). This helps with faster movement, more interaction with pollutants, and more focused, rapid, and efficient degradation or reduction to an acceptable or less dangerous form. The involvement of several enzymes (oxygenase, laccases, peroxidases, haloalkane dehalogenases, carboxylesterases, phosphodiesterase, lipases, and cellulases) in the breakdown routes of different pollutants is extensively described by Mansour et al. (2012). More operational condition flexibility is available when enzymes are applied rather than microorganisms or plants. They can be used in more severe environmental settings that are unsuitable for microbial populations, such as pollutant concentration, pH, temperature, and salinity (Cho et al., 2015). Compared to microbial or whole cell therapy, mass transfer limitation is significantly decreased with enzymatic treatment. Furthermore, enzymatic processes are simpler to manage than microbial remediation. Using enzymes for bioremediation is faster, less expensive, more accessible, and more focused than using plants or microorganisms. For microbial entire cells to develop at their best, air and nutrients must be introduced. Solvents and/or surfactants can improve bioavailability and immobilization (Demarche et al., 2012). From an enzymatic standpoint, it is also more practical than using whole cells. Additionally, compared to chemical and some forms of microbial remediation, enzyme-mediated biodegradation greatly minimizes the creation of hazardous byproducts. A further advancement in the miniaturization of analytical equipment with higher qualitative and quantitative quantification of chemicals of interest at site has been made by enzyme-based biosensors (Rocchitta et al., 2016).
Microbial electrochemical technologies stand out among cutting-edge water treatment methods as an effective and environmentally safe way to remove pharmaceutical pollutants in wastewater (Morris and Jin, 2007; McCormick et al., 2013). The advantageous features of microbiology, electrochemistry, and material science are combined in microbial electrochemical technologies, which offer viable methods for a variety of environmental engineering applications, such as resource recovery, wastewater treatment, bioremediation, and environmental monitoring. Microbial electrochemical technologies are often used in the bio-electro-Fenton process, microbial fuel cell, microbial electrolysis cells, and microbial desalination cell. METs have shown promise in recent years for the removal of stubborn pollutants and the rehabilitation of the environment because of their sustainable, effective, and energy-efficient characteristics (Xu et al., 2022). Microbial fuel cells (MFCs) harness the remarkable power of microbial communities to transform organic or inorganic compound oxidation into electricity through bio-electrochemical processes. This technology essentially taps into the natural metabolic activities of microbes, converting their energy-generating capabilities into a sustainable source of electric current (Gul et al., 2021; Nawaz et al., 2022). The primary source of METs, electrochemical active bacteria, can oxidize a variety of organic and inorganic materials found in sewage, organic waste, and wastewater used for dyeing. METs are a potential method for getting rid of drugs. Proteobacteria, Bacteroidetes, and Firmicutesemerge as the predominant organisms in mostmicrobial bioelectrochemical reactors (Hassan et al., 2021). Pseudomonas and Klebsiella are also a dominant genus in biocathodes for nitrofurazon degradation (Kong et al., 2017).
The first report on the use of METs for the removal of pharmaceutical pollutants, specifically antibiotics, has been reported by Wen et al. (2011). A specially designed two chambered microbial fuel cell was used for metronidazole degradation in wastewater treatment, and 85% removal of antibiotic was achieved within 24 h (Song et al., 2013). Still, several issues require attention. The main emphasis of future studies should be to support the environmentally friendly growth of METs during the treatment of pharmaceutical wastewater like constructing modeling tools to assess bio electrochemical systems. Other major concerns are to forecast treatment efficiencies of wastewaters containing pharmaceuticals under various operating conditions (Ortiz-Martínez et al., 2015) or combining METs with additional technologies, like a moving bed biofilm reactor (MBBR), to enhance the efficiency of pharmaceutical degradation (Chen F. et al., 2020; Chen M. et al., 2020). As pharmaceutical compounds can be highly variable in terms of their complex structure, continued research to determine their removal limits across different operational conditions becomes important. Some experimental electrochemical systems have shown promise of almost complete mineralization of antibiotic substances, e.g., microbial fuel cell coupled wetland constructs and microbial electrolytic cells and microbial fuel cell systems (Hassan et al., 2021). While significant progress has been made in understanding the antibiotic removal capacity of microbial electrochemical systems, it is still not enough considering the fast-growing environmental pollution.
Microorganisms are at the forefront in bioremediation and environmental clean-up studies due to several documented benefits like ease of handling and cost-effectiveness. However, its robust implementation is still lacking, maybe due to insufficient scaling up of proven technologies and reluctance from industries. The special issue by Hlihor and Cozma (2023) highlights many such points as well as complex issues of application of microbial bioremediation in general. Therefore, bioremediation data must be vigorously searched in order to better understand degradative routes. New methods may be utilized to easily fit these data into simulation and numerical modeling, as well as data assembly, repositioning, exploration, and transmission, all of which need standard procedures. When combined with other physical and chemical processes, bioremediation can give a holistic strategy to remove various veterinary pharmaceuticals from the environment. In a recent review by Xu et al. (2024), the microbial enzyme mediated degradation of veterinary medicines is explored, with very high degradation efficiency in some cases (Bilal et al., 2019; Mathur et al., 2021). Approaches like immobilization and bioengineering techniques are extensively being explored with respect to microbial enzymes (Xu et al., 2024). Moreover, the application of microorganisms with genetic modification in the future to enhance bioremediation potential will be an effective technique. Studies have suggested that certain antibiotic resistance genes (ARGs), particularly those related to aminoglycoside modification and β-lactamase enzymes, have existed in environmental microbial communities prior to the use of synthetic antibiotics for medical purposes (D’Costa et al., 2011). This aspect needs to be explored more and understanding of naturally occurring genes can be identified. Over and above that, employing simultaneous gene transfer and multiplication of microorganisms with genetic modification will be a promising strategy.
Through newly found metabolic pathways, enzymes, genes, or operons; genetically designed microbes may become more efficient to bioremediate certain contaminants. Omics has set foot with great significance in the sphere of microbial remediation of veterinary pharmaceutical industry. While the field of genomics metabolomics, and proteomics in biological remediation help in the discovery of potential solutions to specific pollutants, the next frontier in bioremediation research is to find and compare gene and protein sequences that are successful at eliminating contaminants. Genetically modified organisms have the potential to clean up a wide spectrum of veterinary waste effluents and contaminated soil. In one such example, Crofts et al. (2018) have synthesized a genetically engineered Escherichia coli with ability to simultaneously express a β-lactamase and penicillin amidase or the penicillin utilization operon (put) operon. This modification enabled the strain to thrive by utilizing penicillin or benzyl penicilloic acid as growth substrates. This approach was utilized only after the genomic and transcriptomic studies of penicillin catabolism pathways unveiling the upregulation of β-lactamase, amidase, and the phenylactic acid catabolome (Crofts et al., 2018). Thus, there is a need for additional studies on the important genomic and proteomic codes of bioremediators found in nature. For this purpose, utilizing a systems biology approach also provides essential initial insights for the metabolic engineering of microbes, aimed at augmenting their bioremediation potential (Dangi et al., 2019). Understanding the variety and evolutionary links between various biological remediators can aid in the development of an even more effective bioremediation mechanism in the future. Similarly, for microbial electrochemical approach, it’s imperative to delve deeper into the dynamics of the interaction between these microorganisms and the electrodes, as well as between the electrodes and antibiotics. For instance, exploring modified electrodes could potentially boost gene expression linked to electron transfer mechanisms, thereby enhancing the efficiency of electron transfer involved in the degradation of antibiotics through redox reactions. So, with a good understanding of type of microbe, and metabolic redox reactions being carried out by them along with data on associated antibiotic-resistant bacteria and antibiotic resistance genes, we can innovate an effective solution for mitigating environmental antibiotic contamination (Kumar et al., 2019; Roy et al., 2022).
The comprehensive management of veterinary pharmaceuticals requires a multi-pronged approach, combining regulatory measures with advanced treatment technologies. Microbe-based bioremediation, particularly using microbial consortia, shows promise as a sustainable and effective strategy. Accurately identifying the different biodegradation pathways that microorganisms use is necessary to improve comprehension. The latest developments in immobilization have made it possible to repurpose enzymes or whole cells that are involved in the breakdown of pharmaceuticals. The passage of intermediates and metabolites into and out of the immobilized support system is impacted by a few mass transfer limitations, though. Modern innovations like metabolic or genetic engineering and immobilization can greatly boost veterinary pharmaceutical biodegradation thanks to the identification of microbial degradation pathways. Numerous kinetic studies on the biodegradation of pharmaceuticals have been carried out. Therefore, more study that takes into account other process factors is needed to improve the estimation of the kinetic mechanism and degradation rate. However, further research and the development of hybrid technologies are essential to address the limitations and enhance the efficiency of existing methods.
HS: Data curation, Formal analysis, Investigation, Methodology, Visualization, Writing – original draft. SP: Data curation, Formal analysis, Investigation, Methodology, Validation, Writing – original draft. AS: Data curation, Conceptualization, Supervision, Validation, Writing – review & editing. AN: Data curation, Formal analysis, Methodology, Writing – original draft. SS: Data curation, Resources, Writing – original draft. RD: Data curation, Formal analysis, Writing – original draft.
The author(s) declare that no financial support was received for the research, authorship, and/or publication of this article.
The authors declare that the research was conducted in the absence of any commercial or financial relationships that could be construed as a potential conflict of interest.
The reviewer DG declared a past co-authorship with the author AS to the handling editor.
All claims expressed in this article are solely those of the authors and do not necessarily represent those of their affiliated organizations, or those of the publisher, the editors and the reviewers. Any product that may be evaluated in this article, or claim that may be made by its manufacturer, is not guaranteed or endorsed by the publisher.
Al-Gheethi, A. A. S., Norli, I., Lalung, J., Azlan, A. M., Nur Farehah, Z. A., and Kadir, M. O. (2014). Biosorption of heavy metals and cephalexin from secondary effluents by tolerant bacteria. Clean Technol. Environ. Policy 16, 137–148. doi: 10.1007/s10098-013-0611-9
Ali, M. E. M., Abd El-Aty, A. M., Badawy, M. I., and Ali, R. K. (2018). Removal of pharmaceutical pollutants from synthetic wastewater using chemically modified biomass of green alga Scenedesmus obliquus. Ecotoxicol. Environ. Safety 151, 144–152. doi: 10.1016/j.ecoenv.2018.01.012
Amobonye, A., Aruwa, C. E., Aransiola, S., Omame, J., Alabi, T. D., and Lalung, J. (2023). The potential of fungi in the bioremediation of pharmaceutically active compounds: a comprehensive review. Front. Microbiol. 14:1207792. doi: 10.3389/fmicb.2023.1207792
Anusha, P., and Natarajan, D. (2020). Bioremediation potency of multi metal tolerant native bacteria Bacillus cereus isolated from bauxite mines, kolli hills, Tamilnadu- A lab to land approach. Biocatal. Agric. 25, 101581–101595. doi: 10.1016/j.bcab.2020.101581
Azimi, S., and Nezamzadeh-Ejhieh, A. (2015). Enhanced activity of clinoptilolite-supported hybridized PbS–CdS semiconductors for the photocatalytic degradation of a mixture of tetracycline and cephalexin aqueous solution. J. Mol. Catal. A Chem. 408, 152–160. doi: 10.1016/j.molcata.2015.07.017
Baena-Nogueras, R. M., González-Mazo, E., and Lara-Martín, P. A. (2017). Degradation kinetics of pharmaceuticals and personal care products in surface waters: photolysis vs biodegradation. Sci. Total Environ. 590-591, 643–654. doi: 10.1016/j.scitotenv.2017.03.015
Bai, X., and Acharya, K. (2016). Removal of trimethoprim, sulfamethoxazole, and triclosan by the green alga Nannochloris sp. J. Hazard. Mater. 315, 70–75. doi: 10.1016/j.jhazmat.2016.04.067
Barancheshme, F., and Munir, M. (2018). Strategies to combat antibiotic resistance in the wastewater treatment plants. Front. Microbiol. 8:2603. doi: 10.3389/fmicb.2017.02603
Bhattacharya, A., and Khare, S. K. (2022). Utilizing the ß-lactam hydrolyzing activity of ß-lactamase produced by Bacillus cereus EMB20 for remediation of ß-lactam antibiotics. Int Biodeterior Biodegradation. 168, 105363–105363. doi: 10.1016/j.ibiod.2021.105363
Bilal, M., Ashraf, S. S., Barceló, D., and Iqbal, H. M. N. (2019). Biocatalytic degradation/redefining “removal” fate of pharmaceutically active compounds and antibiotics in the aquatic environment. Sci. Total Environ. 691, 1190–1211. doi: 10.1016/j.scitotenv.2019.07.224
Boxall, A. B. A., Fogg, L. A., Blackwell, P. A., Kay, P., Pemberton, E. J., and Croxford, A. (2004). Veterinary medicines in the environment. Rev. Environ. Contam. Toxicol. 180, 1–91. doi: 10.1007/0-387-21729-0_1
Camotti Bastos, M., Rheinheimer dos Santos, D., Aubertheau, É., de Castro Lima, J. A. M., Le Guet, T., Caner, L., et al. (2018). Antibiotics and microbial resistance in Brazilian soils under manure application. Land Degrad. Dev. 29, 2472–2484. doi: 10.1002/ldr.2964
Caracciolo, A. B., Grenni, P., Rauseo, J., Ademollo, N., Cardoni, M., Rolando, L., et al. (2018). Degradation of a fluoroquinolone antibiotic in an urbanized stretch of the river Tiber. Microchem. J. 136, 43–48. doi: 10.1016/j.microc.2016.12.008
Carvalho, I. T., and Santos, L. (2016). Antibiotics in the aquatic environments: a review of the European scenario. Environ. Int. 94, 736–757. doi: 10.1016/j.envint.2016.06.025
Charuaud, L., Jarde, E., Jaffrezic, A., Thomas, M.-F., and Le Bot, B. (2019). Veterinary pharmaceutical residues from natural water to tap water: sales, occurrence and fate. J. Hazard. Mater. 361, 169–186. doi: 10.1016/j.jhazmat.2018.08.075
Chen, M., Ren, L., Qi, K., Li, Q., Lai, M., Li, Y., et al. (2020). Enhanced removal of pharmaceuticals and personal care products from real municipal wastewater using an electrochemical membrane bioreactor. Bioresour. Technol. 311:123579. doi: 10.1016/j.biortech.2020.123579
Chen, J., and Xie, S. (2018). Overview of sulfonamide biodegradation and the relevant pathways and microorganisms. Sci. Total Environ. 640-641, 1465–1477. doi: 10.1016/j.scitotenv.2018.06.016
Chen, F., Zeng, S., Luo, Z., Ma, J., Zhu, Q., and Zhang, S. (2020). A novel MBBR–MFC integrated system for high-strength pulp/paper wastewater treatment and bioelectricity generation. Sep. Sci. Technol. 55, 2490–2499. doi: 10.1080/01496395.2019.1641519
Cho, J. Y., Chung, B. Y., and Hwang, S. A. (2015). Detoxification of the veterinary antibiotic chloramphenicol using electron beam irradiation. Environ. Sci. Pollut. Res. 22, 9637–9645. doi: 10.1007/s11356-015-4123-5
Chu, L., Wang, J., He, S., Chen, C., László, W., and Erzsébet, T. (2021). Treatment of pharmaceutical wastewater by ionizing radiation: Removal of antibiotics, antimicrobial resistance genes and antimicrobial activity. J. Hazard. Mater. 415, 125724–125724. doi: 10.1016/j.jhazmat.2021.125724
Cowieson, A. J., and Kluenter, A.-M. (2019). Contribution of exogenous enzymes to potentiate the removal of antibiotic growth promoters in poultry production. Anim. Feed Sci. Technol. 250, 81–92. doi: 10.1016/j.anifeedsci.2018.04.026
Crofts, T. S., Wang, B., Spivak, A., Gianoulis, T. A., Forsberg, K. J., Gibson, M. K., et al. (2018). Shared strategies for β-lactam catabolism in the soil microbiome. Nat. Chem. Biol. 14, 556–564. doi: 10.1038/s41589-018-0052-1
Cuerda-Correa, E. M., Alexandre-Franco, M. F., and Fernández-González, C. (2019). Advanced oxidation processes for the removal of antibiotics from water. An overview. Water 12:102. doi: 10.3390/w12010102
D’Costa, V., King, C., Kalan, L., Morar, M., Sung, W. W. L., Schwarz, C., et al. (2011). Antibiotic resistance is ancient. Nature 477, 457–461. doi: 10.1038/nature10388
Dalecka, B., Juhna, T., and Rajarao, G. K. (2020). Constructive use of filamentous fungi to remove pharmaceutical substances from wastewater. J. Water Process Eng. 33, 100992–100998. https://doi:10.1016/j.jwpe.2019.100992. doi: 10.1016/j.jwpe.2019.100992
Dangi, A. K., Sharma, B., Hill, R. T., and Shukla, P. (2019). Bioremediation through microbes: systems biology and metabolic engineering approach. Crit. Rev. Biotechnol. 39, 79–98. doi: 10.1080/07388551.2018.1500997
Dar, M. A., Maqbool, M., and Rasool, S. (2019). Pharmaceutical wastes and their disposal practice in routine. Int. J. Inf. Comput. Sci. 6, 78–92.
de Ilurdoz, M. S., Sadhwani, J. J., and Reboso, J. V. (2022). Antibiotic removal processes from water & amp; wastewater for the protection of the aquatic environment - a review. J. Water Process Eng. 45:102474. doi: 10.1016/j.jwpe.2021.102474
Delgado, N., Orozco, J., Zambrano, S., Casas-Zapata, J. C., and Marino, D. (2023). Veterinary pharmaceutical as emerging contaminants in wastewater and surface water: an overview. J. Hazard. Mater. 460:132431. doi: 10.1016/j.jhazmat.2023.132431
Demarche, P., Junghanns, C., Nair, R., and Agathos, S. N. (2012). Harnessing the power of enzymes for environmental stewardship. Biotechnol. Adv. 30, 933–953. doi: 10.1016/j.biotechadv.2011.05.013
Duan, M., Li, H., Gu, J., Tuo, X., Sun, W., Qian, X., et al. (2017). Effects of biochar on reducing the abundance of oxytetracycline, antibiotic resistance genes, and human pathogenic bacteria in soil and lettuce. Environ. Pollut. 224, 787–795. doi: 10.1016/j.envpol.2017.01.021
Espinosa-Ortiz, E. J., Rene, E. R., and Gerlach, R. (2021). Potential use of fungal-bacterial co-cultures for the removal of organic pollutants. Crit. Rev. Biotechnol. 42, 361–383. doi: 10.1080/07388551.2021.1940831
Ezzariai, A., Hafidi, M., Khadra, A., Aemig, Q., El Fels, L., Barret, M., et al. (2018). Human and veterinary antibiotics during composting of sludge or manure: global perspectives on persistence, degradation, and resistance genes. J. Hazard. Mater. 359, 465–481. doi: 10.1016/j.jhazmat.2018.07.092
Fakhri, H., Shahi, A., Suleyman, O., and Aydin, S. (2021). Bioaugmentation with immobilized endophytic Penicillium restrictum to improve quorum quenching activity for biofouling control in an aerobic hollow-fiber membrane bioreactor treating antibiotic-containing wastewater. Ecotoxicol. Environ. Safety, 210, 111831–111842. doi: 10.1016/j.ecoenv.2020.111831
Ferrando, L., and Matamoros, V. (2020). Attenuation of nitrates, antibiotics and pesticides from groundwater using immobilised microalgae-based systems. Sci. Total Environ. 703:134740. doi: 10.1016/j.scitotenv.2019.134740
Ferrando-Climent, L., Cruz-Morató, C., Marco-Urrea, E., Vicent, T., Sarrà, M., Rodriguez-Mozaz, S., et al. (2015). Nonconventional biological treatment based on Trametesversicolor for the elimination of recalcitrant anticancer drugs in hospital wastewater. Chemosphere 136, 9–19. doi: 10.1016/j.chemosphere.2015.03.051
Gojkovic, Z., Lindberg, R. H., Tysklind, M., and Funk, C. (2019). Northern green algae have the capacity to remove active pharmaceutical ingredients. Ecotoxicol. Environ. Saf. 170, 644–656. doi: 10.1016/j.ecoenv.2018.12.032
Gros, M., Mas-Pla, J., Boy-Roura, M., Geli, I., Domingo, F., and Petrović, M. (2019). Veterinary pharmaceuticals and antibiotics in manure and slurry and their fate in amended agricultural soils: findings from an experimental field site (Baix Empordà, NE Catalonia). Sci. Total Environ. 654, 1337–1349. doi: 10.1016/j.scitotenv.2018.11.061
Gul, H., Raza, W., Lee, J., Azam, M., Ashraf, M., and Kim, K. H. (2021). Progress in microbial fuel cell technology for wastewater treatment and energy harvesting. Chemosphere 281:130828. doi: 10.1016/j.chemosphere.2021.130828
Hassan, M., Zhu, G., Lu, Y., AL-Falahi, A. H., Lu, Y., Huang, S., et al. (2021). Removal of antibiotics from wastewater and its problematic effects on microbial communities by bioelectrochemical technology: current knowledge and future perspectives. Environ. Eng. Res. 26:190405. doi: 10.4491/eer.2019.405
Hlihor, R. M., and Cozma, P. (2023). Microbial bioremediation of environmental pollution. Processes 11:1543. doi: 10.3390/pr11051543
Ingerslev, F., Toräng, L., Loke, M.-L., Halling-Sørensen, B., and Nyholm, N. (2001). Primary biodegradation of veterinary antibiotics in aerobic and anaerobic surface water simulation systems. Chemosphere 44, 865–872. doi: 10.1016/s0045-6535(00)00479-3
Islas-Espinoza, M., Reid, B. J., Wexler, M., and Bond, P. L. (2012). Soil bacterial consortia and previous exposure enhance the biodegradation of sulfonamides from pig manure. Microb. Ecol. 64, 140–151. doi: 10.1007/s00248-012-0010-5
Kaczala, F., and Blum, E. S. (2016). The occurrence of veterinary Pharmaceuticals in the Environment: a review. Curr. Anal. Chem. 12, 169–182. doi: 10.2174/1573411012666151009193108
Karigar, C. S., and Rao, S. S. (2011). Role of microbial enzymes in the bioremediation of pollutants: a review. Enzyme Res. 2011:805187. doi: 10.4061/2011/805187
Kasonga, T. K., Coetzee, M. A., Kamika, I., and Momba, M. N. (2021). Assessing the fungal simultaneous removal efficiency of carbamazepine, diclofenac and ibuprofen in aquatic environment. Front. Microbiol. 12, 1–19. doi: 10.3389/fmicb.2021.755972
Kasonga, T. K., Coetzee, M. A., Van Zijl, C., and Momba, M. N. B. (2019). Data on UPLC/MS method validation for the biodegradation of pharmaceuticals and intermediates by a fungal consortium and on T47DK-Bluc reporter gene assay to assess the reduction of their estrogenic activity. Data Brief 25, 104336–104312. doi: 10.1016/j.dib.2019.104336
Kurt, A., Berna Kiril, M., Nihan, Ö., Özge, S., and Taner, Y. (2017). “Treatment of antibiotics in wastewater using advanced oxidation processes (AOPs)” in physico-chemical wastewater treatment and resource recovery (InTech EBooks). 175–190. doi: 10.5772/67538
Kivits, T., Broers, H. P., Beeltje, H., van Vliet, M., and Griffioen, J. (2018). Presence and fate of veterinary antibiotics in age-dated groundwater in areas with intensive livestock farming. Environ. Pollut. 241, 988–998. doi: 10.1016/j.envpol.2018.05.085
Klein, E. Y., Van, T. P., Martinez, E. M., Pant, S., Gandra, S., Levin, S. A., et al. (2018). Global increase and geographic convergence in antibiotic consumption between 2000 and 2015. Proc. Natl. Acad. Sci. USA 115, E3463–E3470. doi: 10.1073/pnas.1717295115
Koba, O., Golovko, O., Kodešová, R., Fér, M., and Grabic, R. (2017). Antibiotics degradation in soil: a case of clindamycin, trimethoprim, sulfamethoxazole and their transformation products. Environ. Pollut. 220, 1251–1263. doi: 10.1016/j.envpol.2016.11.007
Kong, D., Yun, H., Cui, D., Qi, M., Shao, C., Cui, D., et al. (2017). Response of antimicrobial nitrofurazone-degrading biocathode communities to different cathode potentials. Bioresour. Technol. 241, 951–958. doi: 10.1016/j.biortech.2017.06.056
Kumar, M., Jaiswal, S., Sodhi, K. K., Shree, P., Singh, D. K., Agrawal, P. K., et al. (2019). Antibiotics bioremediation: perspectives on its ecotoxicity and resistance. Environ. Int. 124, 448–461. doi: 10.1016/j.envint.2018.12.065
Larsson, J. (2014). Pollution from drug manufacturing: review and perspectives. Philos. Trans. R. Soc. Lond. Ser. B Biol. Sci. 369, –20130571. doi: 10.1098/rstb.2013.0571
Leng, Y., Bao, J., Chang, G., Zheng, H., Li, X., du, J., et al. (2016). Biotransformation of tetracycline by a novel bacterial strain Stenotrophomonasmaltophilia DT1. J. Hazard. Mater. 318, 125–133. doi: 10.1016/j.jhazmat.2016.06.053
Letsoalo, M. R., Sithole, T., Mufamadi, S., Mazhandu, Z., Sillanpaa, M., Kaushik, A., et al. (2023). Efficient detection and treatment of pharmaceutical contaminants to produce clean water for better health and environment. J. Clean. Prod. 387:135798. doi: 10.1016/j.jclepro.2022.135798
Li, Z., Qi, W., Feng, Y., Liu, Y., Ebrahim, S., and Long, J. (2019). Degradation mechanisms of oxytetracycline in the environment. J. Integr. Agricult. 18, 1953–1960. doi: 10.1016/s2095-3119(18)62121-5
Liao, X., Li, B., Zou, R., Dai, Y., Xie, S., and Yuan, B. (2016). Biodegradation of antibiotic ciprofloxacin: pathways, influential factors, and bacterial community structure. Environ. Sci. Pollut. Res. 23, 7911–7918. doi: 10.1007/s11356-016-6054-1
Liu, Y., Cai, D., Li, X., Wu, Q., Ding, P., Shen, L., et al. (2023). Occurrence, fate, and risk assessment of antibiotics in typical pharmaceutical manufactories and receiving water bodies from different regions. PLoS One 18:e0270945. doi: 10.1371/journal.pone.0270945
Liu, B., Li, Y., Zhang, X., Wang, J., and Gao, M. (2015). Effects of chlortetracycline on soil microbial communities: comparisons of enzyme activities to the functional diversity via biolog EcoPlates™. Eur. J. Soil Biol. 68, 69–76. doi: 10.1016/j.ejsobi.2015.01.002
Lucas, D., Badia-Fabregat, M., Vicent, T., Caminal, G., Rodríguez-Mozaz, S., Balcázar, J. L., et al. (2016). Fungal treatment for the removal of antibiotics and antibiotic resistance genes in veterinary hospital wastewater. Chemosphere 152, 301–308. doi: 10.1016/j.chemosphere.2016.02.113
Lucas, A. E., Ito, R., Mustapha, M. M., McElheny, C. L., Mettus, R. T., Bowler, S. L., et al. (2018). Frequency and mechanisms of spontaneous fosfomycin nonsusceptibility observed upon disk diffusion testing of Escherichia coli. J. Clin. Microbiol. 56, 10–1128. doi: 10.1128/jcm.01368-17
Lueangjaroenkit, P., Teerapatsakul, C., Sakka, K., Sakka, M., Kimura, T., Kunitake, E., et al. (2019). Two manganese peroxidases and a laccase of Trametespolyzona KU-RNW027 with novel properties for dye and pharmaceutical product degradation in redox mediator-free system. Mycobiology 47, 217–229. doi: 10.1080/12298093.2019.1589900
Ma, T., Pan, X., Chen, L., Liu, W., Christie, P., Luo, Y., et al. (2016). Effects of different concentrations and application frequencies of oxytetracycline on soil enzyme activities and microbial community diversity. Eur. J. Soil Biol. 76, 53–60. doi: 10.1016/j.ejsobi.2016.07.004
Mahmood, T., Momin, S., Ali, R., Naeem, A., and Khan, A. (2022). “Technologies for Removal of emerging contaminants from wastewater” in Wastewater Treatment (Intech Open).
Mansour, H. B., Mosrati, R., Barillier, D., Ghedira, K., and Chekir-Ghedira, L. (2012). Bioremediation of industrial pharmaceutical drugs. Drug Chem. Toxicol. 35, 235–240. doi: 10.3109/01480545.2011.591799
Mathur, P., Sanyal, D., and Dey, P. (2021). The optimization of enzymatic oxidation of levofloxacin, a fluoroquinolone antibiotic for wastetwater treatment. Biodegradation 32, 467–485. doi: 10.1007/s10532-021-09946-x
Mathur, P., Singh, A., Srivastava, V. R., Singh, D., and Mishra, Y. (2013). Antimicrobial activity of indigenous wildly growing plants: potential source of green antibiotics. Afr. J. Microbiol. Res. 7, 3807–3815. doi: 10.5897/AJMR2012.2368
McCormick, A. J., Bombelli, P., Lea-Smith, D. J., Bradley, R. W., Scott, A. M., Fisher, A. C., et al. (2013). Hydrogen production through oxygenic photosynthesis using the cyanobacterium Synechocystis sp. PCC 6803 in a bio-photoelectrolysis cell (BPE) system. Energy Environ. Sci. 6:2682. doi: 10.1039/c3ee40491a
Menzir, A., and Adeladlw, T. A. (2022). A review on veterinary medical waste disposal and management. J. Pharmaceut. Drug Dev. 9, 102–128.
Migliore, L., Cozzolino, S., and Fiori, M. (2003). Phytotoxicity to and uptake of enrofloxacin in crop plants. Chemosphere 52, 1233–1244. doi: 10.1016/s0045-6535(03)00272-8
Miyata, M., Ihara, I., Yoshid, G., Toyod, K., and Umetsu, K. (2011). Electrochemical oxidation of tetracycline antibiotics using a Ti/IrO2 anode for wastewater treatment of animal husbandry. Water Sci. Technol. 63, 456–461. doi: 10.2166/wst.2011.243
Morris, J. M., and Jin, S. (2007). Feasibility of using microbial fuel cell technology for bioremediation of hydrocarbons in groundwater. J. Environ. Sci. Health A 43, 18–23. doi: 10.1080/10934520701750389
Mutua, F., Sharma, G., Grace, D., Bandyopadhyay, S., Shome, B., and Lindahl, J. (2020). A review of animal health and drug use practices in India, and their possible link to antimicrobial resistance. Antimicrob. Resist. Infect. Control 9:103. doi: 10.1186/s13756-020-00760-3
Narayanan, M., Kandasamy, S., He, Z., and Kumarasamy, S. (2022). “Ecological impacts of pesticides on soil and water ecosystems and its natural degradation process” in Pesticides in the Natural Environment, 23–49.
Nawaz, A., Haq, I., Qaisar, K., Gunes, B., Raja, S. I., Mohyuddin, K., et al. (2022). Microbial fuel cells: insight into simultaneous wastewater treatment and bioelectricity generation. Process Saf. Environ. Prot. 161, 357–373. doi: 10.1016/j.psep.2022.03.039
Ndlela, L. L., Schroeder, P., Genthe, B., and Cruzeiro, C. (2021). Removal of antibiotics using an algae-algae consortium (Chlorella protothecoides and Chlorella vulgaris). Toxics 11, 588–597. doi: 10.3390/toxics11070588
Nielsen, K. M., Kausrud, K. L., Simonsen, G. S., Steinbakk, M., Trosvik, P., Wester, A. L., et al. (2022). Surveillance of antimicrobial resistance in the environment - scientific opinion of the panel on microbial ecology, Norwegian scientific Committee for Food and Environment. VKM Report 2022, 1–128. Available at: https://hdl.handle.net/11250/3054642
O’Dowd, K., and Pillai, S. C. (2020). Photo-fenton disinfection at near neutral pH: process, parameter optimization and recent advances. J. Environ. Chem. Eng. 8, 104063–104063. doi: 10.1016/j.jece.2020.104063
Olicón-Hernández, D. R., González-López, J., and Aranda, E. (2017). Overview on the biochemical potential of filamentous fungi to degrade pharmaceutical compounds. Front. Microbiol. 8, 1–19. doi: 10.3389/fmicb.2017.01792
Oluwole, A. O., Omotola, E. O., and Olatunji, O. S. (2020). Pharmaceuticals and personal care products in water and wastewater: a review of treatment processes and use of photocatalyst immobilized on functionalized carbon in AOP degradation. BMC Chem. 14:62. doi: 10.1186/s13065-020-00714-1
Ortiz-Martínez, V. M., Salar-García, M. J., De Los Ríos, A. P., Hernández-Fernández, F. J., Egea, J. A., and Lozano, L. J. (2015). Developments in microbial fuel cell modeling. Chem. Eng. J. 271, 50–60. doi: 10.1016/j.cej.2015.02.076
Padmesh, S., Singh, A., Chopra, S., Sen, M., Habib, S., Shrivastava, D., et al. (2023). Isolation and characterization of novel lytic bacteriophages that infect multi drug resistant clinical strains of Escherichia coli. Environ. Sci. Pollut. Res. doi: 10.1007/s11356-023-28081-z
Pan, M., and Chu, L. M. (2017). Transfer of antibiotics from wastewater or animal manure to soil and edible crops. Environ. Pollut. 231, 829–836. doi: 10.1016/j.envpol.2017.08.051
Patel, M., Kumar, R., Kishor, K., Mlsna, T., Pittman, C. U., and Mohan, D. (2019). Pharmaceuticals of Emerging Concern in aquatic systems: chemistry, occurrence, effects, and removal methods. Chem. Rev. 119, 3510–3673. doi: 10.1021/acs.chemrev.8b00299
Pathak, A., Angst, D. C., León-Sampedro, R., and Hall, A. R. (2023). Antibiotic-degrading resistance changes bacterial community structure via species-specific responses. ISME J. 17, 1495–1503. doi: 10.1038/s41396-023-01465-2
Patwardhan, S. B., Pandit, S., Kumar Gupta, P., Kumar Jha, N., Rawat, J., Joshi, H. C., et al. (2022). Recent advances in the application of biochar in microbial electrochemical cells. Fuel 311:122501. doi: 10.1016/j.fuel.2021.122501
Rastogi, S., Mittal, V., and Singh, A. (2021). Selection of potential probiotic Bacteria from exclusively breastfed infant Faeces with antagonistic activity against multidrug-resistant ESKAPE pathogens. Probiotics Antimicrob. Protein 13, 739–750. doi: 10.1007/s12602-020-09724-w
Rathore, S., Varshney, A., Mohan, S., and Dahiya, P. (2022). An Chlamydomonasreinhardtiiinnovative approach of bioremediation in enzymatic degradation of xenobiotics. Biotechnol. Genet. Eng. Rev. 38, 1–32. doi: 10.1080/02648725.2022.2027628
Ribeiro, A. R., Sures, B., and Schmidt, T. (2018). Cephalosporin antibiotics in the aquatic environment: a critical review of occurrence, fate, ecotoxicity and removal technologies. Environ. Pollut. 241, 1153–1166. doi: 10.1016/j.envpol.2018.06.040
Rocchitta, G., Spanu, A., Babudieri, S., Latte, G., Madeddu, G., Galleri, G., et al. (2016). Enzyme biosensors for biomedical applications: strategies for safeguarding analytical performances in biological fluids. Sensors (Basel). 16, 780–801. doi: 10.3390/s16060780
Rodríguez Sánchez, D., Houde, M., Douville, M., Silva, S. C., and Verreault, J. (2015). Transcriptional and cellular responses of the green alga to perfluoroalkyl phosphonic acids. Aquat. Toxicol. 160, 31–38. doi: 10.1016/j.aquatox.2014.12.002
Roy, A. S., Sharma, A., Thapa, B. S., Pandit, S., Lahiri, D., Nag, M., et al. (2022). Microbiomics for enhancing electron transfer in an electrochemical system. Front. Microbiol. 13:868220. doi: 10.3389/fmicb.2022.868220
Rutala, W. A., and Weber, D. J. (2015). “Disinfection, sterilization, and control of hospital waste” in Mandell, Douglas, And Bennett’s principles and practice of infectious diseases (W. B. Saunders), 3294–3309.e4.
Samal, K., Mahapatra, S., and Hibzur Ali, M. (2022). Pharmaceutical wastewater as emerging contaminants (EC): treatment technologies, impact on environment and human health. Energy Nexus 6:100076. doi: 10.1016/j.nexus.2022.100076
Scortti, M., Han, L., Alvarez, S., Leclercq, A., Moura, A., Lecuit, M., et al. (2018). Epistatic control of intrinsic resistance by virulence genes in Listeria. PLoS Genet. 14:e1007525. doi: 10.1371/journal.pgen.1007525
Shao, S., Hu, Y., Cheng, J., and Chen, Y. (2019). Biodegradation mechanism of tetracycline (TEC) by strain Klebsiella sp. SQY5 as revealed through products analysis and genomics. Ecotoxicol. Environ. Saf. 185, 109676–109683. doi: 10.1016/j.ecoenv.2019.109676
Sharma, B., Dangi, A. K., and Shukla, P. (2018). Contemporary enzyme-based technologies for bioremediation: a review. J. Environ. Manag. 210, 10–22. doi: 10.1016/j.jenvman.2017.12.075
Silva, A., Delerue-Matos, C., Figueiredo, S., and Freitas, O. (2019). The use of algae and fungi for removal of pharmaceuticals by bioremediation and biosorption processes: a review. Water, 11, 1555–1555. doi: 10.3390/w11081555
Singh, A., Padmesh, S., Dwivedi, M., and Kostova, I. (2022). How good are bacteriophages as an alternative therapy to mitigate biofilms of nosocomial infections. Infect. Drug. Resist. 15, 503–532. doi: 10.2147/IDR.S348700
Singh, A., and Saluja, S. (2021). “Microbial degradation of antibiotics from effluents” in Environmental and microbial biotechnology (Singapore: Springer), 389–404.
Singh, A., and Singh, A. (2022). Microbial degradation and value addition to food and agriculture waste. Curr. Microbiol. 79:119. doi: 10.1007/s00284-022-02809-5
Singh, A., Tripathi, P., Srivastava, A., Ali, S. M., and Rekhi, L. (2016). Antibacterial activity of six indigenous Indian plants: Acacia nilotica (Fabaceae), Albiziasaman (Fabaceae), Azadirachtaindica (Meliaceae), Carica papaya (Caricaceae), Cymbopogoncitratus (Poaceae) and Mangiferaindica (Anacardiaceae). Afr. J. Biotechnol. 15, 666–669. doi: 10.5897/AJB2015.14834
Sodhi, K. K., and Singh, D. K. (2021). Insight into the fluoroquinolone resistance, sources, ecotoxicity, and degradation with special emphasis on ciprofloxacin. J. Water Process Eng. 43:102218. doi: 10.1016/j.jwpe.2021.102218
Sodhi, K. K., and Singh, C. K. (2022). Recent development in the sustainable remediation of antibiotics: a review. Total Environ. Res. Themes 3-4:100008. doi: 10.1016/j.totert.2022.100008
Song, H., Guo, W., Liu, M., and Sun, J. (2013). Performance of microbial fuel cells on removal of metronidazole. Water Sci. Technol. 68, 2599–2604. doi: 10.2166/wst.2013.541
Sturini, M., Speltini, A., Maraschi, F., Profumo, A., Pretali, L., Fasani, E., et al. (2010). Photochemical degradation of Marbofloxacin and Enrofloxacin in natural waters. Environ. Sci. Technol. 44, 4564–4569. doi: 10.1021/es100278n
Suda, T., Hata, T., Kawai, S., Okamura, H., and Nishida, T. (2012). Treatment of tetracycline antibiotics by laccase in the presence of 1-hydroxybenzotriazole. Bioresour. Technol. 103, 498–501. doi: 10.1016/j.biortech.2011.10.041
Sutherland, D. L., and Ralph, P. J. (2019). Microalgal bioremediation of emerging contaminants - opportunities and challenges. Water Res. 164:114921. doi: 10.1016/j.watres.2019.114921
Topp, E., Renaud, J., Sumarah, M., and Sabourin, L. (2016). Reduced persistence of the macrolide antibiotics erythromycin, clarithromycin and azithromycin in agricultural soil following several years of exposure in the field. Sci. Total Environ. 562, 136–144. doi: 10.1016/j.scitotenv.2016.03.210
Tormo-Budowski, R., Cambronero-Heinrichs, J. C., Durán, J. E., Masís-Mora, M., Ramírez-Morales, D., Quirós-Fournier, J. P., et al. (2021). Removal of pharmaceuticals and ecotoxicological changes in wastewater using Trametesversicolor: a comparison of fungal stirred tank and trickle-bed bioreactors. Chem. Eng. J. 410:128210. doi: 10.1016/j.cej.2020.128210
Ünal Turhan, E., Erginkaya, Z., Korukluoğlu, M., and Konuray, G. (2019). “Beneficial biofilm applications in food and agricultural industry” in Health and safety aspects of food processing technologies (Cham: Springer International Publishing), 445–469.
Vaksmaa, A., Guerrero-Cruz, S., Ghosh, P., Zeghal, E., Hernando-Morales, V., and Niemann, H. (2023). Role of fungi in bioremediation of emerging pollutants. Front. Mar. Sci. 10:1070905. doi: 10.3389/fmars.2023.1070905
Vasiliadou, I., Sánchez-Vázquez, R., Molina, R., Martínez, F., Melero, J., Bautista, L., et al. (2016). Biological removal of pharmaceutical compounds using white-rot fungi with concomitant FAME production of the residual biomass. J. Environ. Manag. 180, 228–237. doi: 10.1016/j.jenvman.2016.05.035
Velagaleti, R., Burns, P. K., and Gill, M. (2003). Analytical support for drug manufacturing in the United States—from active pharmaceutical ingredient synthesis to drug product shelf life. Drug Inf. J. 37, 407–438. doi: 10.1177/009286150303700407
Wang, X., Dou, X., Wu, J., and Meng, F. (2021). Attenuation pathways of erythromycin and biochemical responses related to algal growth and lipid synthesis in a microalga-effluent system. Environ. Res. Lett. 195:110873. doi: 10.1016/j.envres.2021.110873
Wang, Y., Liu, L., Luo, Y., Awasthi, M. K., Yang, J., Duan, Y., et al. (2020). Mulching practices alter the bacterial-fungal community and network in favor of soil quality in a semiarid orchard system. Sci. Total Environ. 725:138527. doi: 10.1016/j.scitotenv.2020.138527
Wang, S., and Wang, J. (2018). Biodegradation and metabolic pathway of sulfamethoxazole by a novel strain Acinetobacter sp. Appl. Microbiol. Biotechnol. 102, 425–432. doi: 10.1007/s00253-017-8562-4
Wen, Q., Kong, F., Zheng, H., Yin, J., Cao, D., Ren, Y., et al. (2011). Simultaneous processes of electricity generation and ceftriaxone sodium degradation in an air-cathode single chamber microbial fuel cell. J. Power Sources 196, 2567–2572. doi: 10.1016/j.jpowsour.2010.10.085
Wencewicz, T. A. (2019). Crossroads of antibiotic resistance and biosynthesis. J. Mol. Biol. 431, 3370–3399. doi: 10.1016/j.jmb.2019.06.033
Williams, M., and Kookana, R. S. (2018). “Fate and behavior of environmental contaminants arising from health-care provision” in Health care and environmental contamination (Elsevier B.V.), 21–40.
Xie, P., Chen, C., Zhang, C., Su, G., and Ho, S.-H. (2020). Revealing the role of adsorption in ciprofloxacin and sulfadiazine elimination routes in microalgae. Water Res. 172:115475. doi: 10.1016/j.watres.2020.115475
Xiong, Q., Hu, L.-X., Liu, Y.-S., Zhao, J.-L., He, L.-Y., and Ying, G.-G. (2021). Microalgae-based technology for antibiotics removal: from mechanisms to application of innovational hybrid systems. Environ. Int. 155:106594. doi: 10.1016/j.envint.2021.106594
Xu, X., Lin, X., Ma, W., Huo, M., Tian, X., Wang, H., et al. (2024). Biodegradation strategies of veterinary medicines in the environment: enzymatic degradation. Sci. Total Environ. 912:169598. doi: 10.1016/j.scitotenv.2023.169598
Xu, W., Zou, R., Jin, B., Zhang, G., Su, Y., and Zhang, Y. (2022). The ins and outs of pharmaceutical wastewater treatment by microbial electrochemical technologies. Sustain. Horizons 1:100003. doi: 10.1016/j.horiz.2021.100003
Yang, C.-W., Liu, C., and Chang, B.-V. (2020). Biodegradation of amoxicillin, Tetracyclines and sulfonamides in wastewater sludge. Water 12:2147. doi: 10.3390/w12082147
Yang, L.-H., Qiao, B., Xu, Q.-M., Liu, S., Yuan, Y., and Cheng, J.-S. (2021). Biodegradation of sulfonamide antibiotics through the heterologous expression of laccases from bacteria and investigation of their potential degradation pathways. J. Hazard. Mater. 416:125815. doi: 10.1016/j.jhazmat.2021.125815
Yu, Y., Zhou, Y., Wang, Z., Torres, O. L., Guo, R., and Chen, J. (2017). Investigation of the removal mechanism of antibiotic ceftazidime by green algae and subsequent microbic impact assessment. Sci. Rep. 7:4168. doi: 10.1038/s41598-017-04128-3
Zad, T. J., Astuti, M. P., and Padhye, L. P. (2018). Fate of environmental pollutants. Water Environ. Res. 90, 1104–1170. doi: 10.2175/106143018x15289915807191
Zhang, C., Dong, S., Chen, C., Zhang, Q., and Zhou, D. (2020). Co-substrate addition accelerated amoxicillin degradation and detoxification by up-regulating degradation related enzymes and promoting cell resistance. J. Hazard. Mater. 394:122574. https://doi:10.1016/j.jhazmat.2020.122574. doi: 10.1016/j.jhazmat.2020.122574
Keywords: veterinary medicine, animal drugs, pharmaceutically active compounds, micropollutants, microbial electrochemical technologies, biological remediation
Citation: Saeed H, Padmesh S, Singh A, Nandy A, Singh SP and Deshwal RK (2024) Impact of veterinary pharmaceuticals on environment and their mitigation through microbial bioremediation. Front. Microbiol. 15:1396116. doi: 10.3389/fmicb.2024.1396116
Received: 05 March 2024; Accepted: 27 June 2024;
Published: 08 July 2024.
Edited by:
Sikandar I. Mulla, REVA University, IndiaReviewed by:
Montserrat Sarra, Autonomous University of Barcelona, SpainCopyright © 2024 Saeed, Padmesh, Singh, Nandy, Singh and Deshwal. This is an open-access article distributed under the terms of the Creative Commons Attribution License (CC BY). The use, distribution or reproduction in other forums is permitted, provided the original author(s) and the copyright owner(s) are credited and that the original publication in this journal is cited, in accordance with accepted academic practice. No use, distribution or reproduction is permitted which does not comply with these terms.
*Correspondence: Aditi Singh, YXNpbmdoM0Bsa28uYW1pdHkuZWR1;;c2luZ2guYWRpdGkwMEBnbWFpbC5jb20=
†ORCID: Aditi Singh, https://orcid.org/0000-0002-9303-5960
Disclaimer: All claims expressed in this article are solely those of the authors and do not necessarily represent those of their affiliated organizations, or those of the publisher, the editors and the reviewers. Any product that may be evaluated in this article or claim that may be made by its manufacturer is not guaranteed or endorsed by the publisher.
Research integrity at Frontiers
Learn more about the work of our research integrity team to safeguard the quality of each article we publish.