- School of Life Sciences, University of Technology Sydney, Sydney, NSW, Australia
Non-Tuberculous mycobacteria (NTM) are opportunistic environmental bacteria. Globally, NTM incidence is increasing and modeling suggests that, without new interventions, numbers will continue to rise. Effective treatments for NTM infections remain suboptimal. Standard therapy for Mycobacterium avium complex, the most commonly isolated NTM, requires a 3-drug regime taken for approximately 18 months, with rates of culture conversion reported between 45 and 70%, and high rates of relapse or reinfection at up to 60%. New therapeutic options for NTM treatment are urgently required. A survey of ongoing clinical trials for new NTM therapy listed on ClinicalTrials.Gov using the terms ‘Mycobacterium avium’, ‘Mycobacterium abscessus’, ‘Mycobacterium intracellulare’, ‘Non tuberculous Mycobacteria’ and ‘Nontuberculous Mycobacteria’ and a selection criterion of interventional studies using antibiotics demonstrates that most trials involve dose and combination therapy of the guideline based therapy or including one or more of; Amikacin, Clofazimine, Azithromycin and the anti-TB drugs Bedaquiline and Linezolid. The propensity of NTMs to form biofilms, their unique cell wall and expression of both acquired and intrinsic resistance, are all hampering the development of new anti-NTM therapy. Increased investment in developing targeted treatments, specifically for NTM infections is urgently required.
1 Introduction
Non-Tuberculous Mycobacteria (NTM) are environmental bacteria found commonly in soil and water, both natural and municipal sources. This group includes all members of the mycobacteria family excluding Mycobacterium tuberculosis complex (TB) and Mycobacterium leprae, the causative agents of tuberculosis and leprosy, respectively. NTMs represent a vast group of bacteria with over 190 distinct species (Armstrong et al., 2023). The most common causing disease in humans are the Mycobacterium avium complex (MAC), comprised of Mycobacterium avium and Mycobacterium intracellulare, then Mycobacterium abscessus (MAB) (Falkinham, 2013a). Obtaining accurate data on the extent of NTM infection is challenging, as NTMs are not notifiable diseases in many states or countries. Data from Queensland, Australia where NTMs are a notifiable disease, demonstrates that the number of NTM cases more than doubled in the last 10 years from 672 in 2012 (Thomson et al., 2017) to 1,490 cases in 2022 (Queensland Health, 2024). Current projections suggest NTM infections will triple by 2040 (Ratnatunga et al., 2020). Isolation of NTM are now eight times more common in Queensland than M. tuberculosis, with 191 cases of TB reported in 2023 versus 1,565 NTM isolated (Queensland Health, 2024). While isolation of M. tuberculosis indicates clinical disease, the clinical relevance of an NTM isolation and the decision to treat is more contentious. Clinical, microbiological, and radiological guidelines developed by the ATS/IDSA are used to clarify the likelihood of NTM pulmonary disease versus colonization (Griffith et al., 2007; Schiff et al., 2019).
Increases in NTM infections have also been identified on multiple continents including North America, Europe, and Asia (Andréjak et al., 2010; Lai et al., 2010; Moore et al., 2010; Park et al., 2010; Taiwo and Glassroth, 2010; Donohue and Wymer, 2016; Diel et al., 2017a). South Korea reported a 5-fold increase in NTM infections between 2007 and 2016 from 6.7 cases per 100,000 to 39.6 per 100,000 with MAC and M. abscessus being the most frequently reported (Lee et al., 2019). Japan has also seen a rapid increase in NTM cases 5.7 cases per 100,000 in 2007 to 14.7 cases per 100,000 by 2014, with MAC again, being the most prevalent NTM identified (Namkoong et al., 2016). This incidence rate was higher than the rate of TB in Japan in 2014 at 12.9 cases per 100,000 population (Namkoong et al., 2016).
Multiple theories have been posed to explain the increased numbers of NTMs reported in recent years. Improvements in diagnostic techniques is making accurate diagnosis easier, with faster and more specific tests able to identify NTM infections. The number of individuals who are immunocompromised, and therefore vulnerable to NTM infection is on the rise. A NHIS survey reported an increase from 2.7% in 2013 to 6.6% in 2021 in immunocompromised individuals (Martinson and Lapham, 2024). Individuals with cystic fibrosis (CF), who thankfully show an increase in life expectancy are unfortunately, also at increased risk of developing an NTM infection (Ruseckaite et al., 2022).
The changing climate may be another reason for infection rates rising. A retrospective study by Sherrard et al. compared NTM infection rates between those living in tropical and subtropical regions of Queensland (Sherrard et al., 2017). Those living in tropical regions were 2.5 times more likely to be impacted by an NTM infection (Sherrard et al., 2017). Similarly, a large geographic study conducted across the United States found areas with a higher risk of NTM infection had greater levels of precipitation, evapotranspiration and higher average temperatures (Adjemian et al., 2012). In the tropical state of Hawaii, NTM cases per capita were four times higher than mainland states (Adjemian et al., 2012) with five novel mycobacterial species recently identified in Hawaiian soils (Hennessee et al., 2009). It is also possible that the tropical climate leads to more outdoor activities, such as hiking and swimming which increase chances of exposure to these bacteria (Koschel et al., 2006). With the changing climate, and expansion of tropical regions, this may increase the risk of NTM infection.
Even with increasing instances of infection, NTMs also have the significant issue of misdiagnosis. Clinically, NTM infections manifest with non-specific symptoms such as chronic cough, weight loss, fatigue and fever (Griffith et al., 2007). Diagnosis requires both clinical and microbiologic confirmation, including pulmonary symptoms, nodular or cavity opacities observed through imaging and positive cultures from sputum, bronchial wash or lavage or lung biopsy with mycobacterial histopathologic features (Griffith et al., 2007). NTMs can be misdiagnosed as TB, especially in areas where TB is endemic (Baldwin et al., 2019). One final and important distinction is that a positive NTM isolation does not always signify infection or disease. This further complicates the choice to begin treatment, or continue monitoring the patient, individual patient risk factors then need to be considered.
The general population is frequently exposed to M. avium and other NTMs during everyday activities. A common source of exposure occurs from showerheads, as bacteria found in the municipal water are aerosolized and inhaled (Gebert et al., 2018). NTMs are also found in soils when gardening and can be isolated from hospital equipment (Falkinham, 2013b). Despite the population being frequently exposed to NTM, only a very small percentage develop infection, with immunocompromised individuals at increased risk. NTM infection has been identified in 3.7–24% of CF patients with MAC and MAB being the most prevalent (Olivier et al., 2003; Levy et al., 2008; Roux et al., 2009; Hill et al., 2012). NTM infection in CF patients results in declined lung functionality and overall reduced life expectancy (Malouf and Glanville, 1999). HIV positive patients are also susceptible to NTM infections (Chai et al., 2022). Bacteria can disseminate from the lungs to anywhere in the body, with bone marrow, liver, spleen and lymph nodes the most common (Horsburgh, 1991; von Reyn et al., 2002). Inexplicably, another group with increased susceptibility to NTM infection, are slender, tall immunocompetent women between the ages of 50–80 (Chan and Iseman, 2010; Takayama et al., 2022). Often referred to as ‘Lady Windemere’ syndrome, studies have shown that women meeting these criteria are more likely to develop NTM infections. While chronic cough suppression has been hypothesized as a risk factor for their developing MAC disease no direct evidence explaining this increased susceptibility has been established. Some theories include decreased estrogen in post-menopausal women, or abnormalities in fibrillin which result in expression of immunosuppressive TGF-β may lead to greater susceptibility to NTM infections (Chan and Iseman, 2010).
These significant global increases make having an effective treatment for NTM disease increasingly important. Current treatment regimens are not optimal for efficient and sustained clearance of NTM infection. More research to elucidate antibiotics which are effective against NTM is essential. This review aims to highlight the significant challenges related to treatment of NTM infection, with compounding factors such as limited clinical trials, the paucity of NTM drug discovery pipelines and intrinsic and adaptive drug resistance of the pathogen all contributing to this challenge. It will also discuss opportunities of using new therapeutics to tackle this significant and growing problem.
2 Current treatment regime
2.1 Challenges of current treatment options
The current treatment for NTM infection is a minimum of three antibiotics. Most regimes include ethambutol and rifampicin with a macrolide backbone of either clarithromycin or azithromycin(Griffith et al., 2007). Due to the genetic variability of NTMs strains there is not one standardised treatment plan. Treatment is continued 12 months post a negative sputum culture(Griffith et al., 2007). However, studies report between 10 and 60% of patients experience relapse or reinfection within 6–12 months of initial therapy completion (Lee et al., 2015; Koh et al., 2017; Kwon et al., 2019). One possible reason for the poor outcome is the pharmacokinetic interactions between antibiotics. A study by van Ingen et al. identified that rifampicin significantly reduced peak serum concentrations of macrolides, when used concurrently, clarithromycin and azithromycin concentrations were decreased by up to 68 and 23%, respectively (Van Ingen et al., 2012). If this decrease in serum concentration reduced macrolide killing efficacy in the lung is unknown. Macrolides have strong tissue penetration therefore it is possible therapeutic doses were still being achieved, however, the lack of synergy between these antibiotics may be reducing the efficacy of this treatment regime (Zuckerman et al., 2011).
2.2 Determination of the current treatment regime
The first “American Thoracic Society (ATS) declaration for Nontuberculous Mycobacteria identification and treatment” in 1990 recommended a four drug regimen including 300 mg isoniazid, 600 mg rifampin, and 25 mg/kg ethambutol for the first 2 months followed by 15 mg/kg for the remainder of treatment with streptomycin for the first three to 6 months of therapy (Wallace et al., 1990). This regimen was supported by data from two non-comparative clinical trials (Ahn et al., 1986; Seibert and Bass, 1989). In 1997, the regime was revised to incorporate the macrolides, clarithromycin or azithromycin, now a staple for MAC treatment (American Thoracic Society, 1997).
The ATS/IDSA recommendation for NTM treatment regime was based on data from a number of studies (Table 1) demonstrating that the inclusion of between 250 mg to 750 mg clarithromycin twice daily, to the ethambutol, rifampin and streptomycin regime was highly successful with conversion rates of between 78 and 92%, a low relapse rates of 18% and increased survival (Shafran et al., 1996; Wallace et al., 1996a). Subsequent studies investigating dosing frequency demonstrated a thrice weekly regimen was as effective while reducing toxicity to patients (Griffith et al., 1998, 2000, 2001). Recent studies have seen a shift from rifabutin to rifampin, as rifabutin was associated with higher rates of adverse events (Griffith et al., 2000, 2001). Rifabutin is still recommended as part of the ATS declaration, but is only used in more severe cases of MAC infection.
While the majority of studies demonstrate that the 3-drug regimen induces sputum conversion, the rate of initial conversion, and the rate of relapse, can vary significantly. Studies reported rates of sputum conversion between 47 and 92%, and a relapse rate of 18–39%, with a gradual decrease in rates of conversion evident over time (Table 1). This may be due growing rates of antibiotic resistance, especially against macrolides. Early studies, published in the late 90’s were conducted when clarithromycin had been approved for use for less than a decade and resistance was uncommon (Gardner et al., 2005), whereas resistance was reported as an issue by 2003, and this may have contributed to the reduced conversion rates recorded (Kobashi and Matsushima, 2003).
In 2020 an updated report on the guidelines for NTM treatment was published by the ATS, European Respiratory Society (ERS), European Society of Clinical Microbiology and Infectious Diseases (ESCMID) and the Infectious Disease Society of America (IDSA). The three-drug regime of ethambutol, rifampicin and a macrolide for treatment of M. avium was again endorsed, with the addition of amikacin liposome inhalation suspension (ALIS), approved by the FDA in 2018, for serious infection (U.S. Food and Drug Administration, 2018). If amikacin or streptomycin do not induce negative conversion within 6 months the less toxic ALIS is recommended. While new clinical evidence was used to inform additions to treatment, the report acknowledges the need for ‘well designed’ clinical trials to justify further alterations to this current, best available, treatment regime.
This same report, also defined the treatment regime for MAB. While it acknowledges that the optimal drugs, regimes and duration of treatment for M. abscessus is not known, a combination of at least three drugs including a macrolide, amikacin, imipenem, cefoxitin and tigecycline was recommended (Daley et al., 2020). If the strain is macrolide susceptible at least three drugs should be used, in macrolide resistant strains, treatments should include at least four drugs (Daley et al., 2020). M. abscessus is particularly challenging to treat, with success rates ranging between 41 and 46% for all subspecies (Diel et al., 2017b; Kwak et al., 2019). To date, no antibiotic regime based on in vitro research has effectively produced long term sputum conversion for patients with M. abscessus (Griffith et al., 2007). For all NTMs developing new drugs, with shorter, less toxic, treatment regimens are a research priority.
3 Clinical trials
As indicated, additional clinical trials, to determine the optimum treatment regime for each NTM infection, are urgently required. Although in vitro and in vivo studies are essential to understand drug and bacterial interactions, translation of promising in vitro data into positive clinical correlations has often not been forthcoming (Griffith et al., 2007). Between 85 and 90% of drugs fail the clinical trial phase (Ledford, 2011; Sun et al., 2022). Of those which succeed, only half are approved for clinical use (Ledford, 2011). Unmanageable toxicity accounts for 30% failure, while lack of clinical efficacy is responsible for 40–50% of clinical trial failures (Sun et al., 2022). Factors that influence this lack of efficacy include, variability in metabolic pathways and drug metabolism, complexity and regulation between species and animal models which may not accurately reflect human disease (Pound et al., 2004; Brubaker and Lauffenburger, 2020). For mycobacterial infections most drug discovery research is focused on TB, with few drugs in the NTM development pipeline designed specifically for NTM disease (Wu et al., 2018; Dartois and Dick, 2022). Currently, drugs that show promise in TB are usually then tested on NTM diseases. A focus on progressing drugs that target NTMs to clinical trials is required to effectively address this.
An assessment of registered clinical trials for NTMs on ClinicalTrials.Gov, and searching common NTM using the terms ‘Non tuberculous mycobacteria’, ‘Nontuberculous mycobacteria’, ‘Mycobacterium. avium’, ‘Mycobacterium. intracellulare’ and ‘Mycobacterium. abscessus’ and the selection criteria of clinical trial which (1) listed the use of an antibiotic, (2) was an interventional study and (3) listed an NTM under ‘condition’, identified 58 studies. Of these, 10 are listed as recruiting, 36 were completed with results to be released, and six provided results. One study was terminated, four were active not recruiting, with one with status unknown (Figure 1). The majority of these studies were testing a new antibiotic as monotherapy, or as an addition to the guideline-based therapy (GBT).
The trials with available data fall into two broad categories; studies looking at culture conversion following antibiotic use, or studies assessing pharmacokinetic drug interactions of antibiotics.
Of the trials assessing culture conversion, three utilized liposomal amikacin for inhibition (LAI), combined with standard therapy. The addition of LAI to a GBT regime significantly improved culture conversion, rising to 26.7–33.3% within 12 months compared to 9–13.7% for standard therapies (Olivier et al., 2017; Griffith et al., 2018; Winthrop et al., 2021). Although superiority of a LAI containing regime is shown it is notable that these conversion rates of GBT only are lower than the rates referenced above. The inclusion criteria for these studies were patients already receiving at least 6 months of GBT, within the last 12 month. Studies have shown that conversion within 6 months is predictive of treatment success, therefore it is not unexpected that these patients may have decreased conversion rates (Moon et al., 2019). Amikacin is an aminoglycoside primarily used for treatment of NTM infection when macrolide resistant forms are present. Side effects with amikacin are a significant issue, as amikacin treatment can cause irreversible ototoxicity and vestibular toxicity (Griffith et al., 2007). In the studies testing the addition of LAI (Table 2) it was administered as an inhalant. Adverse events, including COPD and bronchiectasis exacerbation, pneumonia, haemoptysis and worsening MAC infection remained high (Olivier et al., 2017; Griffith et al., 2021; Winthrop et al., 2021). LAI treatment was also successful in increasing conversion to culture negative for rapidly growing NTMs, with 50% conversion at 12 months, a 33% relapse rates and few serious adverse events (Siegel et al., 2023). An additional study adding the antibiotic tigecycline to GBT regimes for treatment of patients with M. abscessus and M. chelonae showed 60% improvement on culture conversion rates (Wallace et al., 2014).
Comorbidities and drug interactions with patients on treatment for other diseases such as CF and HIV pose additional challenges for treating NTM infections. The pharmacokinetic study, NCT01894776, revealed an antagonistic relationship between the antiretroviral drug, maraviroc and rifabutin. This antagonistic relationship, result in a suboptimal treatment responses for HIV+ patients (Abel et al., 2008). The relationship with treatments commonly prescribed for comorbidities such as CF and HIV in individuals being treated for NTM infection needs to be considered. This was recognized in 2015 by The US Cystic Fibrosis Foundation and the European Cystic Fibrosis Society in their joint statement that NTM disease poses a major threat to patients with CF (Floto et al., 2016). All current treatment guideline recommendations are based on data from patients without CF or extrapolated from studies with TB (Peloquin, 1997; Griffith et al., 2007). Additional studies for NTM infections to optimize treatment regimens need to consider the potential of antagonistic drug interactions.
3.1 Optimizing treatment regimes
Optimizing the current treatment regime and investigating new or modified antibiotic regimens is critical to increase cure rates and reduce relapse. The FORMat study (NCT04310930) (Table 3), is a multi-arm study ‘Finding the Optimal Regimen for Mycobacterium abscessus Treatment’. Other ongoing studies are introducing a new drug into the GBT, or studying the efficacy and safety of an isolated drug, which if successful these could lead to marked changes in therapy. The trial NCT05496374, is testing an aminobenzimidazole bacterial DNA gyrase -SPR720, which has demonstrated good activity against mycobacteria (Talley et al., 2021). If successful, SPR720 could be the first novel NTM antibiotic approved for use. The paucity of registered clinical trials for new NTM therapy is a major area of concern.
4 Challenges to developing new therapies
4.1 Antibiotic resistance
Antibiotic resistance is one factor that makes effective treatment of NTM disease challenging. Resistance to NTMs can be either acquired or intrinsic. Unlike most resistance mechanisms which occur from horizontal transfer of mutations, mycobacterial resistance to antibiotics occurs most commonly through spontaneous mutations on chromosomal genes (Nasiri et al., 2017). Single point mutations on the 23 s rRNA gene (rrl) in positions 2058 or 2059 are the most commonly identified source of macrolide resistance (Meier et al., 1996; Wallace et al., 1996b). A mutation in the RNA polymerase beta subunit gene rPOB is responsible for 95% of rifampicin resistance and mutations on the emb CAB operon result in ethambutol resistance (Obata et al., 2006; Lingaraju et al., 2016).
Mutations can arise from a variety of different sources. NTM produce enzymes which can modify or neutralize antibiotics. This is achieved by preventing binding to the drug target or increasing susceptibility to hydrolysis by the bacteria, a classic example of this are beta lactamases (Luthra et al., 2018). Multiple NTM species also contain efflux pumps that contribute to decreased efficacy of antibiotics, by pumping antibiotics out of the cell into the external environment. Prolonged exposure of efflux pumps to NTM antibiotics, facilitated by long treatment times contribute to increased antibiotic resistance (Viveiros et al., 2003). Efflux pump inhibitors, such Verapamil can improve antibiotic success. Verapamil is a Ca2+ blocker, that decreases resistance to key NTM and TB drugs including rifampicin, isoniazid, streptomycin and bedaquiline (Song and Wu, 2016; Rodrigues et al., 2017). Finally, mutations in the transcription regulator whiB7, have a significant effect on susceptibility to mycobacterial infection, being associated with both hyper susceptibility and resistance (Warit et al., 2015; The Cryptic Consortium, 2022). Present in both pathogenic and non-pathogenic mycobacteria, whiB7 works on multiple pathways including antibiotic export and modifications of antibiotics and their targets (Burian et al., 2012; Cushman et al., 2021). WhiB7 transcription has been demonstrated to increase in the presence of antibiotics such as aminoglycosides and macrolides and confer various degrees of resistance (Burian et al., 2012). There have also been some reported instances where a frameshift mutation in whiB7 resulted in hyper susceptibility to clarithromycin (Warit et al., 2015). Using combinations of antibiotics lowers the instance of mutations, however, acquired resistance is not the only hurdle to effective treatment. Intrinsic resistance, such as the thick waxy cell wall and the proclivity to form biofilms also pose a significant treatment challenge.
Another challenge to effective treatment against NTMs is the difficulty in correlating in vitro drug susceptibility testing with clinical outcomes. Drug sensitivity testing for macrolides and amikacin demonstrate good clinical correlation, whereas minimum inhibitory concentration testing for rifampicin and ethambutol, do not show good correlation with clinical response (Daley, 2017).
4.2 Biofilms
In the environment M. avium often form biofilms, such as on PVC plastics in plumbing (Yamazaki et al., 2006b; Falkinham, 2011). Their slow growth rate, ability to adhere to other planktonic bacteria and to plastics, all facilitate their persistence in waterways (Falkinham, 2018a). Recent murine studies have also shown that M. avium’s successful persistence in vivo is largely dependent on its ability to colonize and establish biofilms within the lung (Yamazaki et al., 2006a). Once established, it is incredibly difficult to kill bacteria in this form. The MBCs of M. avium in biofilms are four to six times higher than in planktonic form treated with a single antibiotic (Batista et al., 2023). As the majority of chronic and recurrent bacterial infections are caused by bacteria in biofilm, being able to penetrate, and break-down, the biofilm will be crucial to the success of new therapeutics (Mah, 2012).
4.3 Colony morphology
The smooth or rough appearance of NTM colony morphology can affect treatment. Although relevant in many species, colony morphology of M. abscessus is a key factor in treatment success. A retrospective multicentre cohort study conducted in Sweden between 2009 and 2020 found rough colony morphology was associated with worse treatment outcomes in patients with M. abscessus. Only 30% of patients achieved a clinical cure compared to 86% of patients with smooth morphology (Hedin et al., 2023). Mortality was also greater at 50% compared to 7% (Hedin et al., 2023). One key factor affecting colony morphology is the presence of glycopeptidolipids (GPL), with smooth colonies exhibiting higher GPL expression (Hedin et al., 2023). GPL also have a role in biofilm formation, with the smooth morphology M. abscessus forming biofilms by spreading out across the entire surface (Oschmann-Kadenbach et al., 2024). The exact mechanism for the poorer clinical outcomes with rough variants is unknown, however rough M. abscessus replicate more actively within macrophages and can spread more effectively between cells (Hedin et al., 2023). The rough variants also exhibit more cording, a virulence factor in mycobacterial infections known to inhibit macrophage phagocytosis (Howard et al., 2006; Hedin et al., 2023).
4.4 Cell wall
The unusual cell wall structure of mycobacteria also provides significant intrinsic resistance and a distinct survival advantage, enhancing viability and pathogenesis. Mycobacteria cell walls are highly lipid dense, lipids make up 60% of the cell wall mass (Kurz and Rivas-Santiago, 2020). This includes the outer lipids as well as mycolic acids. Mycolic acids are long chain acids comprising 60–90 carbon chains (Marrakchi et al., 2014; Minnikin and Brennan, 2020). Their structure contributes significantly to the low permeability of the mycobacterial cell wall (Liu et al., 1996). Mycolic acids attach to polysaccharides and then peptidoglycan and provide strength to the wall (Figure 2).
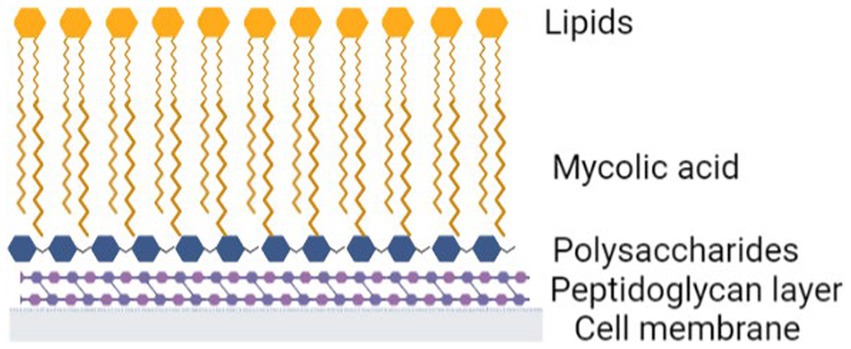
Figure 2. Mycobacteria cell wall. Created with Biorender.com
The cell wall is hydrophobic, while the majority of antibiotics are hydrophilic, and therefore cannot penetrate an intact cell wall (Falkinham, 2018b). Beta-lactams (β-lactams) and glycopeptides belong to a class of antibiotics which inhibit cell wall synthesis of both gram negative and positive bacteria (Batt et al., 2020). β-lactams, like penicillin and cephalosporins, work by irreversibly binding to the active sites of penicillin binding proteins and inhibiting 3 → 4 peptide crosslinking, hindering peptidoglycan synthesis of the cell wall (Kurz and Bonomo, 2012; Batt et al., 2020). Unfortunately, mycobacteria, have 3 → 3 peptide crosslinking, so are not inhibited by β-lactams(Kumar et al., 2012; Kurz and Bonomo, 2012). Glycopeptides, like vancomycin and teicoplanin also target peptidoglycan structures, inhibiting cell wall synthesis by binding to the C terminal acyl- D-ala-D-ala residues in peptidoglycan precursors (Reynolds, 1989). The thick waxy exterior of the NTMs hydrophobic outer layer, reduces the capacity of these glycopeptides to access the peptidoglycan layer thereby reducing the overall effectiveness of the antibiotic. Interestingly, mycobacteria have also developed additional strategies to survive cell wall disrupting antibiotics and can replicate in a cell wall deficient state.
5 NTMs and cell wall deficiency
When faced with antibiotic or nutrient stress NTMs are able to alter their morphology, forgoing their normal protective cell wall to grow in a cell wall deficient (CWD) state, previously called L-forms (Claessen and Errington, 2019). Without the cell wall, bacteria display as cocci (Mickiewicz et al., 2019). Cell wall deficiency enables NTMs to survive cell-wall targeting antibiotics and continue to replicate. CWD bacteria were first observed by Klieneberger in Streptobacillus moniliformis (Klieneberger, 1935). Initially speculated to be symbiosis between bacterial colonies, this theory was later abandoned by the author (Klieneberger, 1935; Klieneberger-Nobel, 1949). The discovery spurred on significant research into the phenomenon. Subsequent studies demonstrated that bacteria remain viable in these forms and revert back to their cell wall competent forms when the stress is removed (Mickiewicz et al., 2019). Studies in zebrafish have demonstrated that CWD can occur both in vitro and in vivo (Mickiewicz et al., 2019). The initial switch to a CWD form can occur rapidly in response to environmental factors. Multiple mycobacterial species, including M. avium and M. tuberculosis, can form viable CWD bacteria under chemical or cryogenic stress (Hines and Styer, 2003; Slavchev et al., 2016). It was initially thought that genetic alteration resulted in this morphological change, however, this was subsequently shown not to be the case, suggesting that changes in morphology were responsible for the increased antibiotic resistance (Slavchev et al., 2016). The potential to exploit the capacity of the bacteria to grow in a CWD state, as a treatment option is worth exploring. While in a CWD state the bacteria are resistant to cell wall inhibiting antibiotics, they may however, become more susceptible to other antibiotics, including those that are usually unable to penetrate the cell wall. Targeting synergy with new drugs combinations which induce CWD may provide alternatives to current treatment methods.
6 New options for NTM therapy
Treatment options for NTMs have largely been based on repurposing anti-TB antibiotics. This offers advantages in lower costs and less time to market. Increased instances of multi drug resistant (MDR) and extremely drug resistant (XDR) cases of TB have resulted in a greater focus on new pharmaceuticals with over 35 antibiotic candidates for TB treatment in discovery phase and approximately 30 in clinical trials (Wu et al., 2018). Exploring the options of repurposing antibiotics recently approved for TB, like bedaquiline and linezolid, use may identify new treatment options for NTM infections as well.
6.1 Bedaquiline
Bedaquiline was the first anti-TB drug approved for use by the FDA since rifampicin in 1971, approved against MDR and XDR-TB (World Health Organization, 2013; Nguyen et al., 2016). Bedaquiline acts by inhibiting ATP synthase and stopping mycobacterial F-ATP function, an affect specific to mycobacteria (Kundu et al., 2016). Low MICs have been shown when using this drug with good tolerability. The most significant adverse effect is a prolonging of QT intervals (Shulha et al., 2019) which needs to be monitored in patients. In TB studies, synergy has been shown between bedaquiline and other drugs including cephalosporins, linezolid and pyrazinamide (Khoshnood et al., 2021). While most data from this drug is on use against TB, one study has examined its effect on M. avium. This study found that the MIC90 was 0.015 μg/mL with 87% of isolates tested susceptible to bedaquiline at concentrations ≤0.008 μg/mL (Brown-Elliott et al., 2017), similar to the ranges those used for M. tb (Ismail et al., 2018). The inclusion of bedaquiline into XDR-TB treatment regimens lowered the risk of death compared to regimes not containing it (Schnippel et al., 2018). At least one listed clinical trial is currently recruiting to assess the effect of bedaquiline against NTM infection (Table 3).
6.2 Linezolid
Another antibiotic recently accepted for therapy against MDR and XRD TB is linezolid (Diekema and Jones, 2000). It acts by binding to 23 s and 50s ribosomal subunits of rRNA to inhibit bacterial protein synthesis at the initiation step (Diekema and Jones, 2000). The downside of linezolid is its high toxicity. A recent systematic review of 367 patients with MDR and XDR TB, found over 55% experienced some form of adverse effect (Zhang et al., 2015). Therapy was discontinued in 35% of these patients, most commonly occurring within the first 2 months (Zhang et al., 2015). Lower doses and shorter durations of antibiotics, by utilizing drugs with synergistic effects, could permit effective use of linezolid at reduced concentrations and thereby reduce toxicity. One study examining refractory NTM infection in 16 patients, demonstrated complete remission in 50% of patients and improvement in a further 25% but adverse reactions remained problematic (Chetchotisakd and Anunnatsiri, 2014). Newer drugs in this class of oxazolidinones are being developed for both TB and NTM infection, with the aim of having an improved safety and toxicity profile (Negatu et al., 2023). The outcome of ongoing studies to assess the effectiveness linezolid and bedaquiline to treat NTM infections are eagerly anticipated (Table 3).
6.3 Phage therapy
Bacteriophages, or phages, are viruses which infect and replicate exclusively within bacterial cells. They are highly diverse and found in abundance in the environment. Phages are host specific and often only infect a specific bacteria species or strain. Phages are absorbed by the bacterial host where they spread their genetic material, replicate and finally lyse the bacteria (Young et al., 2000). The use of phage therapy against NTM infection was first described in 2017 for two patients with CF and M. abscessus infection (Dedrick et al., 2019). The inclusion of phages, along with continued use of antibiotics, while not leading to complete clearance of the bacteria did generally result in improved clinical responses (Dedrick et al., 2019). A study across a small patient subpopulation receiving this therapy found phages successfully improved conditions in 75% of patients with a rough colony morphology (Dedrick et al., 2023). Several other studies using phages in humans also reported reducing NTM infection (Dedrick et al., 2019, 2021; Nick et al., 2022). Based on current research phages appear to be safe and well tolerated by patients. While resistance to bacteriophages has not been reported, host neutralization of phages may limit their effectiveness. There is also a considerable amount of personalization required for this therapy, making it more difficult to implement as a large-scale solution to NTM infection. Phages may be a useful tool, especially in combination with antibiotics. For large scale implementation, new antibiotics specific to NTM infections, are urgently required.
Expanding research to explore new antibiotics and therapeutic avenues that may demonstrate greater effectiveness against NTM disease is urgently required. Focusing on newly licensed anti-TB antibiotics, such as bedaquiline and linezolid in NTMs is one avenue, but new specific treatments will be required to manage the increasing public health threat posed by NTM disease.
Author contributions
LC: Writing – original draft, Writing – review, editing. BS: Conceptualization, Funding acquisition, Supervision, Writing – original draft, Writing – review, editing.
Funding
The author(s) declare financial support was received for the research, authorship, and/or publication of this article. LC was supported by an Australian Government Research Training Program Stipend.
Conflict of interest
The authors declare that the research was conducted in the absence of any commercial or financial relationships that could be construed as a potential conflict of interest.
Publisher’s note
All claims expressed in this article are solely those of the authors and do not necessarily represent those of their affiliated organizations, or those of the publisher, the editors and the reviewers. Any product that may be evaluated in this article, or claim that may be made by its manufacturer, is not guaranteed or endorsed by the publisher.
References
Abel, S., Jenkins, T. M., Whitlock, L. A., Ridgway, C. E., and Muirhead, G. J. (2008). Effects of Cyp3A4 inducers with and without Cyp3A4 inhibitors on the pharmacokinetics of maraviroc in healthy volunteers. Br. J. Clin. Pharmacol. 65, 38–46. doi: 10.1111/j.1365-2125.2008.03134.x
Adjemian, J., Olivier, K. N., Seitz, A. E., Falkinham, J. O. 3rd, Holland, S. M., and Prevots, D. R. (2012). Spatial clusters of nontuberculous mycobacterial lung disease in the United States. Am. J. Respir. Crit. Care Med. 186, 553–558. doi: 10.1164/rccm.201205-0913OC
Ahn, C. H., Ahn, S. S., Anderson, R. A., Murphy, D. T., and Mammo, A. (1986). A four-drug regimen for initial treatment of cavitary disease caused by Mycobacterium avium complex. Am. Rev. Respir. Dis. 134, 438–441
American Thoracic Society (1997). Diagnosis and treatment of disease caused by nontuberculous mycobacteria. This official statement of the American Thoracic Society was approved by the Board of Directors, march 1997. Medical section of the American Lung Association. Am. J. Respir. Crit. Care Med. 156, S1–S25. doi: 10.1164/ajrccm.156.2.atsstatement
Andréjak, C., Thomsen, V., Johansen, I. S., Riis, A., Benfield, T. L., Duhaut, P., et al. (2010). Nontuberculous pulmonary mycobacteriosis in Denmark: incidence and prognostic factors. Am. J. Respir. Crit. Care Med. 181, 514–521. doi: 10.1164/rccm.200905-0778OC
Armstrong, D. T., Eisemann, E., and Parrish, N. (2023). A brief update on mycobacterial taxonomy, 2020 to 2022. J. Clin. Microbiol. 61:e0033122. doi: 10.1128/jcm.00331-22
Baldwin, S. L., Larsen, S. E., Ordway, D., Cassell, G., and Coler, R. N. (2019). The complexities and challenges of preventing and treating nontuberculous mycobacterial diseases. PLoS Negl. Trop. Dis. 13:e0007083. doi: 10.1371/journal.pntd.0007083
Batista, S., Fernandez-Pittol, M., Nicolás, L. S., Martínez, D., Rubio, M., Garrigo, M., et al. (2023). Vitro effect of three-antibiotic combinations plus potential Antibiofilm agents against biofilm-producing Mycobacterium avium and Mycobacterium intracellulare clinical isolates. Antibiotics 12:1409. doi: 10.3390/antibiotics12091409
Batt, S. M., Burke, C. E., Moorey, A. R., and Besra, G. S. (2020). Antibiotics and resistance: the two-sided coin of the mycobacterial cell wall. Cell Surf 6:100044. doi: 10.1016/j.tcsw.2020.100044
Brown-Elliott, B. A., Philley, J. V., Griffith, D. E., Thakkar, F., and Wallace, R. J. (2017). In vitro susceptibility testing of Bedaquiline against Mycobacterium avium complex. Antimicrob. Agents Chemother. 61:e01798. doi: 10.1128/AAC.01798-16
Brubaker, D. K., and Lauffenburger, D. A. (2020). Translating preclinical models to humans. Science 367, 742–743. doi: 10.1126/science.aay8086
Burian, J., Ramón-García, S., Howes, C. G., and Thompson, C. J. (2012). WhiB7, a transcriptional activator that coordinates physiology with intrinsic drug resistance in Mycobacterium tuberculosis. Expert Rev. Anti-Infect. Ther. 10, 1037–1047. doi: 10.1586/eri.12.90
Chai, J., Han, X., Mei, Q., Liu, T., Walline, J. H., Xu, J., et al. (2022). Clinical characteristics and mortality of non-tuberculous mycobacterial infection in immunocompromised vs. Immunocompetent hosts. Front. Med. (Lausanne) 9:884446. doi: 10.3389/fmed.2022.884446
Chan, E. D., and Iseman, M. D. (2010). Slender, older women appear to be more susceptible to nontuberculous mycobacterial lung disease. Gend. Med. 7, 5–18. doi: 10.1016/j.genm.2010.01.005
Chetchotisakd, P., and Anunnatsiri, S. (2014). Linezolid in the treatment of disseminated nontuberculous mycobacterial infection in anti-interferon-gamma autoantibody-positive patients. Southeast Asian J. Trop. Med. Public Health 45, 1125–1131
Claessen, D., and Errington, J. (2019). Cell Wall deficiency as a coping strategy for stress. Trends Microbiol. 27, 1025–1033. doi: 10.1016/j.tim.2019.07.008
Cushman, J., Freeman, E., Mccallister, S., Schumann, A., Hutchison, K. W., and Molloy, S. D. (2021). Increased whiB7 expression and antibiotic resistance in Mycobacterium chelonae carrying two prophages. BMC Microbiol. 21:176. doi: 10.1186/s12866-021-02224-z
Daley, C. L. (2017). Mycobacterium avium complex disease. Microbiol. Spectr. 5:2017. doi: 10.1128/microbiolspec.TNMI7-0045-2017
Daley, C. L., Iaccarino, J. M., Lange, C., Cambau, E., Wallace, R. J. Jr., Andrejak, C., et al. (2020). Treatment of nontuberculous mycobacterial pulmonary disease: an official Ats/Ers/Escmid/Idsa clinical practice guideline. Eur. Respir. J. 56:2000535. doi: 10.1183/13993003.00535-2020
Dartois, V., and Dick, T. (2022). Drug development challenges in nontuberculous mycobacterial lung disease: Tb to the rescue. J. Exp. Med. 219:e20220445. doi: 10.1084/jem.20220445
Dedrick, R. M., Freeman, K. G., Nguyen, J. A., Bahadirli-Talbott, A., Smith, B. E., Wu, A. E., et al. (2021). Potent antibody-mediated neutralization limits bacteriophage treatment of a pulmonary Mycobacterium abscessus infection. Nat. Med. 27, 1357–1361. doi: 10.1038/s41591-021-01403-9
Dedrick, R. M., Guerrero-Bustamante, C. A., Garlena, R. A., Russell, D. A., Ford, K., Harris, K., et al. (2019). Engineered bacteriophages for treatment of a patient with a disseminated drug-resistant Mycobacterium abscessus. Nat. Med. 25, 730–733. doi: 10.1038/s41591-019-0437-z
Dedrick, R. M., Smith, B. E., Cristinziano, M., Freeman, K. G., Jacobs-Sera, D., Belessis, Y., et al. (2023). Phage therapy of Mycobacterium infections: compassionate use of phages in 20 patients with drug-resistant mycobacterial disease. Clin. Infect. Dis. 76, 103–112. doi: 10.1093/cid/ciac453
Diekema, D. I., and Jones, R. N. (2000). Oxazolidinones: a review. Drugs 59, 7–16. doi: 10.2165/00003495-200059010-00002
Diel, R., Jacob, J., Lampenius, N., Loebinger, M., Nienhaus, A., Rabe, K. F., et al. (2017a). Burden of non-tuberculous mycobacterial pulmonary disease in Germany. Eur. Respir. J. 49:1602109. doi: 10.1183/13993003.02109-2016
Diel, R., Ringshausen, F., Richter, E., Welker, L., Schmitz, J., and Nienhaus, A. (2017b). Microbiological and clinical outcomes of treating non-Mycobacterium avium complex nontuberculous mycobacterial pulmonary disease: a systematic review and Meta-analysis. Chest 152, 120–142. doi: 10.1016/j.chest.2017.04.166
Donohue, M. J., and Wymer, L. (2016). Increasing prevalence rate of nontuberculous mycobacteria infections in five states, 2008-2013. Ann. Am. Thorac. Soc. 13, 2143–2150. doi: 10.1513/AnnalsATS.201605-353OC
Falkinham, J. O. (2011). Nontuberculous mycobacteria from household plumbing of patients with nontuberculous mycobacteria disease. Emerg. Infect. Dis. 17, 419–424. doi: 10.3201/eid1703.101510
Falkinham, J. O. (2013a). Ecology of nontuberculous mycobacteria--where do human infections come from? Semin. Respir. Crit. Care Med. 34, 95–102. doi: 10.1055/s-0033-1333568
Falkinham, J. O. (2013b). Reducing human exposure to Mycobacterium avium. Ann. Am. Thorac. Soc. 10, 378–382. doi: 10.1513/AnnalsATS.201301-013FR
Falkinham, J. O. (2018a). Mycobacterium avium complex: adherence as a way of life. AIMS Microbiol. 4, 428–438. doi: 10.3934/microbiol.2018.3.428
Falkinham, J. O. (2018b). Challenges of Ntm drug development. Front. Microbiol. 9:1613. doi: 10.3389/fmicb.2018.01613
Floto, R. A., Olivier, K. N., Saiman, L., Daley, C. L., Herrmann, J.-L., Nick, J. A., et al. (2016). Us Cystic Fibrosis Foundation and European cystic fibrosis society consensus recommendations for the management of non-tuberculous mycobacteria in individuals with cystic fibrosis. Thorax 71, i1–i22. doi: 10.1136/thoraxjnl-2015-207360
Gardner, E. M., Burman, W. J., Degroote, M. A., Hildred, G., and Pace, N. R. (2005). Conventional and molecular epidemiology of macrolide resistance among new Mycobacterium avium complex isolates recovered from Hiv-infected patients. Clin. Infect. Dis. 41, 1041–1044. doi: 10.1086/433187
Gebert, M. J., Delgado-Baquerizo, M., Oliverio, A. M., Webster, T. M., Nichols, L. M., Honda, J. R., et al. (2018). Ecological analyses of mycobacteria in showerhead biofilms and their relevance to human health. MBio 9, e01614–e01618. doi: 10.1128/mBio.01614-18
Griffith, D. E., Aksamit, T., Brown-Elliott, B. A., Catanzaro, A., Daley, C., Gordin, F., et al. (2007). An official Ats/Idsa statement: diagnosis, treatment, and prevention of nontuberculous mycobacterial diseases. Am. J. Respir. Crit. Care Med. 175, 367–416. doi: 10.1164/rccm.200604-571ST
Griffith, D. E., Brown, B. A., Cegielski, P., Murphy, D. T., and Wallace, R. J. (2000). Early results (at 6 months) with intermittent clarithromycin-including regimens for lung disease due to Mycobacterium avium complex. Clin. Infect. Dis. 30, 288–292. doi: 10.1086/313644
Griffith, D. E., Brown, B. A., Girard, W. M., Griffith, B. E., Couch, L. A., and Wallace, R. J. Jr. (2001). Azithromycin-containing regimens for treatment of Mycobacterium avium complex lung disease. Clin. Infect. Dis. 32, 1547–1553. doi: 10.1086/320512
Griffith, D. E., Brown, B. A., Murphy, D. T., Girard, W. M., Couch, L., and Wallace, R. J. Jr. (1998). Initial (6-month) results of three-times-weekly azithromycin in treatment regimens for Mycobacterium avium complex lung disease in human immunodeficiency virus-negative patients. J. Infect. Dis. 178, 121–126. doi: 10.1086/515597
Griffith, D. E., Eagle, G., Thomson, R., Aksamit, T. R., Hasegawa, N., Morimoto, K., et al. (2018). Amikacin liposome inhalation suspension for treatment-refractory lung disease caused by Mycobacterium avium complex (Convert). A prospective, open-label, randomized study. Am. J. Respir. Crit. Care Med. 198, 1559–1569. doi: 10.1164/rccm.201807-1318OC
Griffith, D. E., Thomson, R., Flume, P. A., Aksamit, T. R., Field, S. K., Addrizzo-Harris, D. J., et al. (2021). Amikacin liposome inhalation suspension for refractory Mycobacterium avium complex lung disease: sustainability and durability of culture conversion and safety of long-term exposure. Chest 160, 831–842. doi: 10.1016/j.chest.2021.03.070
Hedin, W., Fröberg, G., Fredman, K., Chryssanthou, E., Selmeryd, I., Gillman, A., et al. (2023). A rough Colony morphology of Mycobacterium abscessus is associated with Cavitary pulmonary disease and poor clinical outcome. J. Infect. Dis. 227, 820–827. doi: 10.1093/infdis/jiad007
Hennessee, C. T., Seo, J. S., Alvarez, A. M., and Li, Q. X. (2009). Polycyclic aromatic hydrocarbon-degrading species isolated from Hawaiian soils: Mycobacterium crocinum sp. nov., Mycobacterium pallens sp. nov., Mycobacterium rutilum sp. nov., Mycobacterium rufum sp. nov. and Mycobacterium aromaticivorans sp. nov. Int. J. Syst. Evol. Microbiol. 59, 378–387. doi: 10.1099/ijs.0.65827-0
Hill, U. G., Floto, R. A., and Haworth, C. S. (2012). Non-tuberculous mycobacteria in cystic fibrosis. J. R. Soc. Med. 105 Suppl 2, S14–S18. doi: 10.1258/jrsm.2012.12s003
Hines, M. E., and Styer, E. L. (2003). Preliminary characterization of chemically generated Mycobacterium avium subsp. paratuberculosis cell wall deficient forms (spheroplasts). Vet. Microbiol. 95, 247–258. doi: 10.1016/S0378-1135(03)00185-8
Horsburgh, C. R. (1991). Mycobacterium avium complex infection in the acquired immunodeficiency syndrome. N. Engl. J. Med. 324, 1332–1338. doi: 10.1056/NEJM199105093241906
Howard, S. T., Rhoades, E., Recht, J., Pang, X., Alsup, A., Kolter, R., et al. (2006). Spontaneous reversion of Mycobacterium abscessus from a smooth to a rough morphotype is associated with reduced expression of glycopeptidolipid and reacquisition of an invasive phenotype. Microbiology (Reading) 152, 1581–1590. doi: 10.1099/mic.0.28625-0
Ismail, N. A., Omar, S. V., Joseph, L., Govender, N., Blows, L., Ismail, F., et al. (2018). Defining Bedaquiline susceptibility, resistance, cross-resistance and associated genetic determinants: a retrospective cohort study. EBioMedicine 28, 136–142. doi: 10.1016/j.ebiom.2018.01.005
Khoshnood, S., Goudarzi, M., Taki, E., Darbandi, A., Kouhsari, E., Heidary, M., et al. (2021). Bedaquiline: Current status and future perspectives. J. Glob. Antimicrob. Resist. 25, 48–59. doi: 10.1016/j.jgar.2021.02.017
Klieneberger, E. (1935). The natural occurrence of pleuropneumonia-like organism in apparent symbiosis with Strrptobacillus moniliformis and other bacteria. J. Pathol. 40, 93–105. doi: 10.1002/path.1700400108
Klieneberger-Nobel, E. (1949). Oriǵin, development and significance of L-forms in bacterial cultures. Microbiology 3, 434–443,
Kobashi, Y., and Matsushima, T. (2003). The effect of combined therapy according to the guidelines for the treatment of Mycobacterium avium complex pulmonary disease. Intern. Med. 42, 670–675. doi: 10.2169/internalmedicine.42.670
Kobashi, Y., Matsushima, T., and Oka, M. (2007). A double-blind randomized study of aminoglycoside infusion with combined therapy for pulmonary Mycobacterium avium complex disease. Respir. Med. 101, 130–138. doi: 10.1016/j.rmed.2006.04.002
Koh, W. J., Moon, S. M., Kim, S. Y., Woo, M. A., Kim, S., Jhun, B. W., et al. (2017). Outcomes of Mycobacterium avium complex lung disease based on clinical phenotype. Eur. Respir. J. 50:1602503. doi: 10.1183/13993003.02503-2016
Koschel, D., Pietrzyk, C., Sennekamp, J., and Müller-Wening, D. (2006). Schwimmbadlunge - Exogen-allergische Alveolitis oder Mykobakteriose? Pneumologie (Stuttgart, Germany) 60, 285–289. doi: 10.1055/s-2006-932160
Kumar, P., Arora, K., Lloyd, J. R., Lee, I. Y., Nair, V., Fischer, E., et al. (2012). Meropenem inhibitsD,D‐carboxypeptidase activity in Mycobacterium tuberculosis. Mol. Microbiol. 86, 367–381. doi: 10.1111/j.1365-2958.2012.08199.x
Kundu, S., Biukovic, G., Grüber, G., and Dick, T. (2016). Bedaquiline targets the ε subunit of mycobacterial F-Atp synthase. Antimicrob. Agents Chemother. 60, 6977–6979. doi: 10.1128/AAC.01291-16
Kurz, S. G., and Bonomo, R. A. (2012). Reappraising the use of β-lactams to treat tuberculosis. Expert Rev. Anti-Infect. Ther. 10, 999–1006. doi: 10.1586/eri.12.96
Kurz, S. G., and Rivas-Santiago, B. (2020). Time to expand the picture of mycobacterial lipids: spotlight on nontuberculous mycobacteria. Am. J. Respir. Cell Mol. Biol. 62, 275–276. doi: 10.1165/rcmb.2019-0324ED
Kwak, N., Dalcolmo, M. P., Daley, C. L., Eather, G., Gayoso, R., Hasegawa, N., et al. (2019). Mycobacterium abscessus pulmonary disease: individual patient data meta-analysis. Eur. Respir. J. 54:1801991. doi: 10.1183/13993003.01991-2018
Kwon, B. S., Shim, T. S., and Jo, K. W. (2019). The second recurrence of Mycobacterium avium complex lung disease after successful treatment for first recurrence. Eur. Respir. J. 53:1801038. doi: 10.1183/13993003.01038-2018
Lai, C. C., Tan, C. K., Chou, C. H., Hsu, H. L., Liao, C. H., Huang, Y. T., et al. (2010). Increasing incidence of nontuberculous mycobacteria, Taiwan, 2000-2008. Emerg. Infect. Dis. 16, 294–296. doi: 10.3201/eid1602.090675
Ledford, H. (2011). Translational research: 4 ways to fix the clinical trial. Nature 477, 526–528. doi: 10.1038/477526a
Lee, B. Y., Kim, S., Hong, Y., Lee, S. D., Kim, W. S., Kim, D. S., et al. (2015). Risk factors for recurrence after successful treatment of Mycobacterium avium complex lung disease. Antimicrob. Agents Chemother. 59, 2972–2977. doi: 10.1128/AAC.04577-14
Lee, H., Myung, W., Koh, W. J., Moon, S. M., and Jhun, B. W. (2019). Epidemiology of nontuberculous mycobacterial infection, South Korea, 2007-2016. Emerg. Infect. Dis. 25, 569–572. doi: 10.3201/eid2503.181597
Levy, I., Grisaru-Soen, G., Lerner-Geva, L., Kerem, E., Blau, H., Bentur, L., et al. (2008). Multicenter cross-sectional study of nontuberculous mycobacterial infections among cystic fibrosis patients, Israel. Emerg. Infect. Dis. 14, 378–384. doi: 10.3201/eid1403.061405
Lingaraju, S., Rigouts, L., Gupta, A., Lee, J., Umubyeyi, A. N., Davidow, A. L., et al. (2016). Geographic differences in the contribution of ubiA mutations to high-level ethambutol resistance in Mycobacterium tuberculosis. Antimicrob. Agents Chemother. 60, 4101–4105. doi: 10.1128/AAC.03002-15
Liu, J., Barry, C. E., Besra, G. S., and Nikaido, H. (1996). Mycolic acid structure determines the fluidity of the mycobacterial cell wall. J. Biol. Chem. 271, 29545–29551. doi: 10.1074/jbc.271.47.29545
Luthra, S., Rominski, A., and Sander, P. (2018). The role of antibiotic-target-modifying and antibiotic-modifying enzymes in Mycobacterium abscessus drug resistance. Front. Microbiol. 9:2179. doi: 10.3389/fmicb.2018.02179
Mah, T. F. (2012). Biofilm-specific antibiotic resistance. Future Microbiol. 7, 1061–1072. doi: 10.2217/fmb.12.76
Malouf, M. A., and Glanville, A. R. (1999). The spectrum of mycobacterial infection after lung transplantation. Am. J. Respir. Crit. Care Med. 160, 1611–1616. doi: 10.1164/ajrccm.160.5.9808113
Marrakchi, H., Lanéelle, M. A., and Daffé, M. (2014). Mycolic acids: structures, biosynthesis, and beyond. Chem. Biol. 21, 67–85. doi: 10.1016/j.chembiol.2013.11.011
Martinson, M. L., and Lapham, J. (2024). Prevalence of immunosuppression among us adults. JAMA 331, 880–882. doi: 10.1001/jama.2023.28019
Meier, A., Heifets, L., Wallace, R. J. Jr., Zhang, Y., Brown, B. A., Sander, P., et al. (1996). Molecular mechanisms of clarithromycin resistance in Mycobacterium avium: observation of multiple 23S rdna mutations in a clonal population. J. Infect. Dis. 174, 354–360. doi: 10.1093/infdis/174.2.354
Mickiewicz, K. M., Kawai, Y., Drage, L., Gomes, M. C., Davison, F., Pickard, R., et al. (2019). Possible role of L-form switching in recurrent urinary tract infection. Nat. Commun. 10:4379. doi: 10.1038/s41467-019-12359-3
Minnikin, D. E., and Brennan, P. J. (2020). “Lipids of clinically significant mycobacteria” in Health consequences of microbial interactions with hydrocarbons, oils, and lipids. ed. H. Goldfine (Cham: Springer International Publishing).
Moon, S. M., Jhun, B. W., Baek, S. Y., Kim, S., Jeon, K., Ko, R. E., et al. (2019). Long-term natural history of non-cavitary nodular bronchiectatic nontuberculous mycobacterial pulmonary disease. Respir. Med. 151, 1–7. doi: 10.1016/j.rmed.2019.03.014
Moore, J. E., Kruijshaar, M. E., Ormerod, L. P., Drobniewski, F., and Abubakar, I. (2010). Increasing reports of non-tuberculous mycobacteria in England, Wales and Northern Ireland, 1995-2006. BMC Public Health 10:612. doi: 10.1186/1471-2458-10-612
Namkoong, H., Kurashima, A., Morimoto, K., Hoshino, Y., Hasegawa, N., Ato, M., et al. (2016). Epidemiology of pulmonary nontuberculous mycobacterial disease, Japan. Emerg. Infect. Dis. 22, 1116–1117. doi: 10.3201/eid2206.151086
Nasiri, M. J., Haeili, M., Ghazi, M., Goudarzi, H., Pormohammad, A., Imani Fooladi, A. A., et al. (2017). New insights in to the intrinsic and acquired drug resistance mechanisms in mycobacteria. Front. Microbiol. 8:681. doi: 10.3389/fmicb.2017.00681
Negatu, D. A., Aragaw, W. W., Cangialosi, J., Dartois, V., and Dick, T. (2023). Side-by-side profiling of Oxazolidinones to estimate the therapeutic window against mycobacterial infections. Antimicrob. Agents Chemother. 67:e0165522. doi: 10.1128/aac.01655-22
Nguyen, T. V., Cao, T. B., Akkerman, O. W., Tiberi, S., Vu, D. H., and Alffenaar, J. W. (2016). Bedaquiline as part of combination therapy in adults with pulmonary multi-drug resistant tuberculosis. Expert. Rev. Clin. Pharmacol. 9, 1025–1037. doi: 10.1080/17512433.2016.1200462
Nick, J. A., Dedrick, R. M., Gray, A. L., Vladar, E. K., Smith, B. E., Freeman, K. G., et al. (2022). Host and pathogen response to bacteriophage engineered against Mycobacterium abscessus lung infection. Cell 185, 1860–1874.e12. doi: 10.1016/j.cell.2022.04.024
Obata, S., Zwolska, Z., Toyota, E., Kudo, K., Nakamura, A., Sawai, T., et al. (2006). Association of rpoB mutations with rifampicin resistance in Mycobacterium avium. Int. J. Antimicrob. Agents 27, 32–39. doi: 10.1016/j.ijantimicag.2005.09.015
Olivier, K. N., Griffith, D. E., Eagle, G., Mcginnis, J. P., Micioni, L., Liu, K., et al. (2017). Randomized trial of liposomal amikacin for inhalation in nontuberculous mycobacterial lung disease. Am. J. Respir. Crit. Care Med. 195, 814–823. doi: 10.1164/rccm.201604-0700OC
Olivier, K. N., Weber, D. J., Wallace, R. J., Faiz, A. R., Lee, J. H., Zhang, Y., et al. (2003). Nontuberculous mycobacteria. I: multicenter prevalence study in cystic fibrosis. Am. J. Respir. Crit. Care Med. 167, 828–834. doi: 10.1164/rccm.200207-678OC
Oschmann-Kadenbach, A. M., Schaudinn, C., Borst, L., Schwarz, C., Konrat, K., Arvand, M., et al. (2024). Impact of Mycobacteroides abscessus colony morphology on biofilm formation and antimicrobial resistance. Int. J. Med. Microbiol. 314:151603. doi: 10.1016/j.ijmm.2024.151603
Park, Y. S., Lee, C. H., Lee, S. M., Yang, S. C., Yoo, C. G., Kim, Y. W., et al. (2010). Rapid increase of non-tuberculous mycobacterial lung diseases at a tertiary referral hospital in South Korea. Int. J. Tuberc. Lung Dis. 14, 1069–1071
Peloquin, C. A. (1997). Mycobacterium avium complex infection. Pharmacokinetic and pharmacodynamic considerations that may improve clinical outcomes. Clin. Pharmacokinet. 32, 132–144. doi: 10.2165/00003088-199732020-00004
Pound, P., Ebrahim, S., Sandercock, P., Bracken, M. B., and Roberts, I. (2004). Where is the evidence that animal research benefits humans? BMJ 328, 514–517. doi: 10.1136/bmj.328.7438.514
Queensland Health . (2024). Notifiable conditions anual reporting. Queensland Government. Available at: https://www.health.qld.gov.au/clinical-practice/guidelines-procedures/diseases-infection/surveillance/reports/notifiable/annual (Accessed February 27, 2024).
Ratnatunga, C. N., Lutzky, V. P., Kupz, A., Doolan, D. L., Reid, D. W., Field, M., et al. (2020). The rise of non-tuberculosis mycobacterial lung disease. Front. Immunol. 11:303. doi: 10.3389/fimmu.2020.00303
Reynolds, P. E. (1989). Structure, biochemistry and mechanism of action of glycopeptide antibiotics. Eur. J. Clin. Microbiol. Infect. Dis. 8, 943–950. doi: 10.1007/BF01967563
Rodrigues, L., Parish, T., Balganesh, M., and Ainsa, J. A. (2017). Antituberculosis drugs: reducing efflux=increasing activity. Drug Discov. Today 22, 592–599. doi: 10.1016/j.drudis.2017.01.002
Roux, A. L., Catherinot, E., Ripoll, F., Soismier, N., Macheras, E., Ravilly, S., et al. (2009). Multicenter study of prevalence of nontuberculous mycobacteria in patients with cystic fibrosis in France. J. Clin. Microbiol. 47, 4124–4128. doi: 10.1128/JCM.01257-09
Ruseckaite, R., Salimi, F., Earnest, A., Bell, S. C., Douglas, T., Frayman, K., et al. (2022). Survival of people with cystic fibrosis in Australia. Sci. Rep. 12:19748. doi: 10.1038/s41598-022-24374-4
Schiff, H. F., Jones, S., Achaiah, A., Pereira, A., Stait, G., and Green, B. (2019). Clinical relevance of non-tuberculous mycobacteria isolated from respiratory specimens: seven year experience in a Uk hospital. Sci. Rep. 9:1730. doi: 10.1038/s41598-018-37350-8
Schnippel, K., Ndjeka, N., Maartens, G., Meintjes, G., Master, I., Ismail, N., et al. (2018). Effect of bedaquiline on mortality in south African patients with drug-resistant tuberculosis: a retrospective cohort study. Lancet Respir. Med. 6, 699–706. doi: 10.1016/S2213-2600(18)30235-2
Seibert, A., and Bass, J. (1989). Four drug therapy of pulmonary disease due to Mycobacterium avium complex. Annual Meeting of American Thoracic Society, pp. 14–17.
Shafran, S. D., Singer, J., Zarowny, D. P., Phillips, P., Salit, I., Walmsley, S. L., et al. (1996). A comparison of two regimens for the treatment of Mycobacterium avium complex bacteremia in Aids: rifabutin, ethambutol, and clarithromycin versus rifampin, ethambutol, clofazimine, and ciprofloxacin. Canadian Hiv trials network protocol 010 study group. N. Engl. J. Med. 335, 377–384. doi: 10.1056/NEJM199608083350602
Sherrard, L. J., Tay, G. T., Butler, C. A., Wood, M. E., Yerkovich, S., Ramsay, K. A., et al. (2017). Tropical Australia is a potential reservoir of non-tuberculous mycobacteria in cystic fibrosis. Eur. Respir. J. 49:1700046. doi: 10.1183/13993003.00046-2017
Shulha, J. A., Escalante, P., and Wilson, J. W. (2019). Pharmacotherapy approaches in nontuberculous mycobacteria infections. Mayo Clin. Proc. 94, 1567–1581. doi: 10.1016/j.mayocp.2018.12.011
Siegel, S. A. R., Griffith, D. E., Philley, J. V., Brown-Elliott, B. A., Brunton, A. E., Sullivan, P. E., et al. (2023). Open-label trial of amikacin liposome inhalation suspension in Mycobacterium abscessus lung disease. Chest 164, 846–859. doi: 10.1016/j.chest.2023.05.036
Slavchev, G., Michailova, L., and Markova, N. (2016). L-form transformation phenomenon in Mycobacterium tuberculosis associated with drug tolerance to ethambutol. Int. J. Mycobacteriol. 5, 454–459. doi: 10.1016/j.ijmyco.2016.06.011
Song, L., and Wu, X. (2016). Development of efflux pump inhibitors in antituberculosis therapy. Int. J. Antimicrob. Agents 47, 421–429. doi: 10.1016/j.ijantimicag.2016.04.007
Sun, D., Gao, W., Hu, H., and Zhou, S. (2022). Why 90% of clinical drug development fails and how to improve it? Acta Pharm. Sin. B 12, 3049–3062. doi: 10.1016/j.apsb.2022.02.002
Taiwo, B., and Glassroth, J. (2010). Nontuberculous mycobacterial lung diseases. Infect. Dis. Clin. N. Am. 24, 769–789. doi: 10.1016/j.idc.2010.04.008
Takayama, Y., Kitajima, T., Honda, N., Sakane, N., Yumen, Y., Fukui, M., et al. (2022). Nutritional status in female patients with nontuberculous mycobacterial lung disease and its association with disease severity. BMC Pulm. Med. 22:315. doi: 10.1186/s12890-022-02109-5
Talley, A. K., Thurston, A., Moore, G., Gupta, V. K., Satterfield, M., Manyak, E., et al. (2021). First-in-human evaluation of the safety, tolerability, and pharmacokinetics of Spr720, a novel Oral bacterial Dna gyrase (GyrB) inhibitor for mycobacterial infections. Antimicrob. Agents Chemother. 65:e0120821. doi: 10.1128/AAC.01208-21
The Cryptic Consortium (2022). Genome-wide association studies of global Mycobacterium tuberculosis resistance to 13 antimicrobials in 10,228 genomes identify new resistance mechanisms. PLoS Biol. 20:e3001755. doi: 10.1371/journal.pbio.3001755
Thomson, R., Donnan, E., and Konstantinos, A. (2017). Notification of nontuberculous mycobacteria: an Australian perspective. Ann. Am. Thorac. Soc. 14, 318–323. doi: 10.1513/AnnalsATS.201612-994OI
U.S. Food and Drug Administration . (2018). FDA approves a new antibacterial drug to treat a serious lung disease using a novel pathway to spur innovation. Available at: https://www.fda.gov/news-events/press-announcements/fda-approves-new-antibacterial-drug-treat-serious-lung-disease-using-novel-pathway-spur-innovation#:~:text=The%20U.S.%20Food%20and%20Drug,not%20respond%20to%20conventional%20treatment%20 (Accessed February 29, 2024).
Van Ingen, J., Egelund, E. F., Levin, A., Totten, S. E., Boeree, M. J., Mouton, J. W., et al. (2012). The pharmacokinetics and pharmacodynamics of pulmonary Mycobacterium avium complex disease treatment. Am. J. Respir. Crit. Care Med. 186, 559–565. doi: 10.1164/rccm.201204-0682OC
Viveiros, M., Leandro, C., and Amaral, L. (2003). Mycobacterial efflux pumps and chemotherapeutic implications. Int. J. Antimicrob. Agents 22, 274–278. doi: 10.1016/S0924-8579(03)00208-5
Von Reyn, C. F., Arbeit, R. D., Horsburgh, C. R., Ristola, M. A., Waddell, R. D., Tvaroha, S. M., et al. (2002). Sources of disseminated Mycobacterium avium infection in Aids. J. Infect. 44, 166–170. doi: 10.1053/jinf.2001.0950
Wallace, R. J., Brown, B. A., Griffith, D. E., Girard, W. M., and Murphy, D. T. (1996a). Clarithromycin regimens for pulmonary Mycobacterium avium complex. The first 50 patients. Am. J. Respir. Crit. Care Med. 153, 1766–1772. doi: 10.1164/ajrccm.153.6.8665032
Wallace, R. J., Dukart, G., Brown-Elliott, B. A., Griffith, D. E., Scerpella, E. G., and Marshall, B. (2014). Clinical experience in 52 patients with tigecycline-containing regimens for salvage treatment of Mycobacterium abscessus and Mycobacterium chelonae infections. J. Antimicrob. Chemother. 69, 1945–1953. doi: 10.1093/jac/dku062
Wallace, R. J., Meier, A., Brown, B. A., Zhang, Y., Sander, P., Onyi, G. O., et al. (1996b). Genetic basis for clarithromycin resistance among isolates of Mycobacterium chelonae and Mycobacterium abscessus. Antimicrob. Agents Chemother. 40, 1676–1681. doi: 10.1128/AAC.40.7.1676
Wallace, R. J., O'Brien, R., Glassroth, J., Raleigh, J., and Dutt, A. (1990). Diagnosis and treatment of disease caused by nontuberculous mycobacteria. Am. Rev. Respir. Dis. 142, 940–953. doi: 10.1164/ajrccm/142.4.940
Warit, S., Phunpruch, S., Jityam, C., Jaitrong, S., Billamas, P., Chaiprasert, A., et al. (2015). Genetic characterisation of a whiB7 mutant of a Mycobacterium tuberculosis clinical strain. J. Glob. Antimicrob. Resist. 3, 262–266. doi: 10.1016/j.jgar.2015.07.004
Winthrop, K. L., Flume, P. A., Thomson, R., Mange, K. C., Yuen, D. W., Ciesielska, M., et al. (2021). Amikacin liposome inhalation suspension for Mycobacterium avium complex lung disease: a 12-month open-label extension clinical trial. Ann. Am. Thorac. Soc. 18, 1147–1157. doi: 10.1513/AnnalsATS.202008-925OC
World Health Organization (2013). The use of bedaquiline in the treatment of multidrug-resistant tuberculosis: interim policy guidance. Berlin: World Health Organization.
Wu, M. L., Aziz, D. B., Dartois, V., and Dick, T. (2018). Ntm drug discovery: status, gaps and the way forward. Drug Discov. Today 23, 1502–1519. doi: 10.1016/j.drudis.2018.04.001
Yamazaki, Y., Danelishvili, L., Wu, M., Hidaka, E., Katsuyama, T., Stang, B., et al. (2006a). The ability to form biofilm influences Mycobacterium avium invasion and translocation of bronchial epithelial cells. Cell. Microbiol. 8, 806–814. doi: 10.1111/j.1462-5822.2005.00667.x
Yamazaki, Y., Danelishvili, L., Wu, M., Macnab, M., and Bermudez, L. E. (2006b). Mycobacterium avium genes associated with the ability to form a biofilm. Appl. Environ. Microbiol. 72, 819–825. doi: 10.1128/AEM.72.1.819-825.2006
Young, I., Wang, I., and Roof, W. D. (2000). Phages will out: strategies of host cell lysis. Trends Microbiol. 8, 120–128. doi: 10.1016/S0966-842X(00)01705-4
Zhang, X., Falagas, M. E., Vardakas, K. Z., Wang, R., Qin, R., Wang, J., et al. (2015). Systematic review and meta-analysis of the efficacy and safety of therapy with linezolid containing regimens in the treatment of multidrug-resistant and extensively drug-resistant tuberculosis. J. Thorac. Dis. 7, 603–615. doi: 10.3978/j.issn.2072-1439.2015.03.10
Keywords: Non-tuberculous mycobacteria, Mycobacterium avium, Mycobacterium abscessus, clinical trials, antibiotics, treatment regime
Citation: Conyers LE and Saunders BM (2024) Treatment for non-tuberculous mycobacteria: challenges and prospects. Front. Microbiol. 15:1394220. doi: 10.3389/fmicb.2024.1394220
Edited by:
Selvakumar Subbian, The State University of New Jersey, United StatesReviewed by:
Yadong Zhang, Harvard Medical School, United StatesThanh Quang Nguyen, Gyeongsang National University, Republic of Korea
Lina Davies Forsman, Karolinska Institutet, Sweden
Copyright © 2024 Conyers and Saunders. This is an open-access article distributed under the terms of the Creative Commons Attribution License (CC BY). The use, distribution or reproduction in other forums is permitted, provided the original author(s) and the copyright owner(s) are credited and that the original publication in this journal is cited, in accordance with accepted academic practice. No use, distribution or reproduction is permitted which does not comply with these terms.
*Correspondence: Bernadette M. Saunders, QmVybmFkZXR0ZS5TYXVuZGVyc0B1dHMuZWR1LmF1