- College of Animal Science, Inner Mongolia Agricultural University, Hohhot, China
With the high intensification of poultry breeding, a series of diseases caused by pathogenic bacteria threaten the health of poultry and human. Among them, poultry diseases induced by Escherichia coli cause significant economic loss every year. The aim of this study was to investigate the effects of dietary supplementation with Artemisia annua L. polysaccharide (AAP) on the growth performance and intestinal barrier function of broilers with Escherichia coli (E. coli) challenge. A total of 256 one-day-old chicks were randomly assigned to four treatment groups: control group (fed basal diet), AAP group (fed basal diet supplemented with AAP), E. coli group (fed basal diet and orally administered E. coli), AAP + E. coli group (fed basal diet supplemented with AAP and orally administered E. coli). Dietary AAP supplementation elevated the BW, ADG and ADFI in non-challenged broilers. AAP also increased the apparent metabolic rate of EE and Ca in E. coli-challenged broilers. Moreover, AAP not only enhanced the serum IgA content but also decreased the serum and jejunum content of IL-6, as well as the jejunum level of IL-1β in non-challenged broilers. AAP also down-regulates the mRNA level of inflammatory factors (IL-1β, IL-6, and TNF-α) by inhibiting the mRNA expression of TLR4 and MyD88 in intestinal NF-κB signaling pathway of E. coli-challenged broilers. Meanwhile, AAP up-regulates the activity and mRNA level CAT by down-regulating the mRNA level of Keap1 in intestinal Nrf2 signaling pathway of E. coli-challenged broilers, and decreased serum MDA concentration. AAP significantly elevated the mRNA level of CAT, SOD and Nrf2 in jejunal of non-challenged broilers. Interestingly, AAP can improve intestinal physical barrier by down-regulating serum ET content, increasing the jejunal villus height/crypt depth (VH/CD) and ZO-1 mRNA level in broilers challenged by E. coli. AAP also elevated the VH/CD and the mRNA level of Occludin, ZO-1, Mucin-2 in non-challenged broilers. Importantly, AAP reshaped the balance of jejunum microbiota in E. coli-challenged broilers by altering α diversity and community composition. In summary, AAP ameliorated the loss of growth performance in broilers challenged with E. coli, probably by regulating the intestinal permeability and mucosa morphology, immune function, antioxidant ability, and microbiota.
1 Introduction
The health of the gastrointestinal tract is crucial for the overall health and production performance of poultry. Firstly, it is a part of the digestive system responsible for breaking down food, absorbing nutrients, and excreting undigested food. The intestinal tract is composed of the mucosal layer and epithelial cells, which effectively block the invasion of pathogenic bacteria through tight junctions and adherent junctions between the cells. In addition, the presence of gut-associated lymphoid tissue (GALT) and immune cells further enhances the defensive ability of the intestinal tract. GALT is the main part of intestinal lymphoid tissue and can produce antibodies and cytokines to mount immune responses against invading pathogens. Immune cells, including lymphocytes, macrophages, and other white blood cells, play a crucial role in the intestinal tract. These cells possess the ability to recognize and attack invading pathogens, thus protecting poultry from infection (Berkes et al., 2003; Yegani and Korver, 2008).
When the gastrointestinal tract is infected with pathogenic bacteria such as Escherichia coli (E. coli), the intestinal barrier may be disrupted, leading to the invasion of the bacteria into the body and triggering an inflammatory response and infection. The negative effects of E. coli on poultry are primarily manifested in intestinal inflammation, disrupted digestive function, decreased growth performance, and increased mortality. These consequences not only impact the health and production performance of the animals but also result in substantial economic loss for the poultry farming industry (Croxen et al., 2013; Gomes et al., 2016). In recent years, research on mitigating the negative impacts of avian E. coli has garnered increasing attention. It has been reported that dietary live yeast and mannan-oligosaccharide supplementation can effectively attenuate E. coli-induced intestinal disruption in broilers by reducing intestinal inflammation and barrier dysfunction (Wang et al., 2016). Furthermore, Kumari et al. (2023) discovered that added drinking water with Aloe vera leaf extract could mitigate the detrimental effects of E. coli-challenge on broilers by enhancing antioxidant level and bolstering cellular immune response. In addition, studies showed that dietary hydrolyzed wheat gluten supplementation ameliorated intestinal barrier dysfunction of broilers challenged with E. coli O78 (Wu et al., 2022b).
In recent years, plant-derived polysaccharides have been widely studied in poultry production, which can relieve various stress and improve intestinal barrier function (Guo et al., 2022b). It has been reported that dietary Gan Cao (Glycyrrhiza uralensis Fisch) polysaccharide improves growth performance, immune function, gut microflora and intestinal health of broiler chickens (Wu et al., 2022c; Zhang et al., 2022c). Qiao et al. (2022b) also found that polysaccharides derived from Astragalus membranaceus and Glycyrrhiza uralensis improved the growth performance of broilers by enhancing intestinal health and modulating gut microbiota. Moreover, dietary Astragalus polysaccharide supplementation has been found to alleviate necrotic enteritis in broiler chickens by balancing Th17/Treg response and regulating gut microbiota composition (Song et al., 2022). Besides, dietary Caulis Spatholobi polysaccharide alleviated the immunosuppression induced by cyclophosphamide in broilers though regulating immunity, intestinal mucosal barrier function, and intestinal microbiota (Cui et al., 2022). Previous studies demonstrated that dietary supplementation Artemisia polysaccharides have beneficial effects on broilers. It was found that dietary supplementation with Artemisia argyi polysaccharide improved immune and antioxidative functions in broilers (Zhang et al., 2022b). It has been reported that Artemisia ordosica polysaccharide ameliorated LPS-induced growth inhibition and intestinal injury in broilers through enhancing immune regulation and antioxidant capacity (Xing et al., 2023).
Artemisia annua L. (A. annua) is a species of Artemisia within the Asteraceae family, is widely distributed (Ding et al., 2020) and is renowned for its rich variety of active substances, including sesquiterpenoids, flavonoids, coumarins, and volatile oils (Das et al., 2020; Fu et al., 2020), consequently, it possesses numerous biological functions, including anti-inflammatory, antioxidant, antibacterial, antiviral, antitumor anti-malarial and immune regulatory activity (Graham et al., 2019; Stan, 2020; Han et al., 2022; Chebbac et al., 2023; Wu et al., 2023). In poultry, previous studies have shown that A. annua aqueous extract can promote intestine immunity and antioxidant function (Guo et al., 2022a), inhibit the reproduction of E. coli and promote the proliferation of Lactobacillusla in broilers (Guo et al., 2023). In addition, adding A. annua and its extracts into diets can effectively relieve heat stress, immune stress and oxidative stress in broilers (Song et al., 2017, 2018; Wan et al., 2017, 2018; Choi et al., 2020). Addition of A. annua extract to Nile tilapia diets modulated the intestinal microbiota and altered the abundance of specific bacteria (Fusobacteriaceae, Stenotrophomonas, and Clostridium), thereby improving growth performance (Soares et al., 2022). Notably, the polysaccharide, being a bioactive substance in A. annua, has been found to possess antitumor activity (Yan et al., 2019), along with antioxidant and immunomodulatory properties, as confirmed by in vitro experiments (Zhang et al., 2022a). The stable structures and properties of polysaccharides, as macromolecular compounds, contribute to their notably high safety profile. With a mature extraction and purification technology specific to Artemisia (Xing et al., 2020; Zhang et al., 2022b), polysaccharides are poised for large-scale production and application. However, it has not been reported whether A. annua polysaccharide is beneficial to broilers so far. In this study, oral administration of E. coli was used to establish a challenge model, in order to investigate the effect of dietary supplementation of A. annua polysaccharide on growth performance and intestinal barrier function of broilers challenged with E. coli, and provide theoretical basis for the application of A. annua polysaccharide in poultry production.
2 Materials and methods
2.1 Animal ethics statement
All animal experiments were approved by the Inner Mongolia Agricultural University Animal Care and Use Committee, Hohhot, P. R. China (approval number: NND2021090).
2.2 Preparation of Artemisia annua L. polysaccharide
Artemisia annua L. (A. annua) plants were collected from Hohhot, Inner Mongolia, China. The collected plants were washed with distilled water and air-dried in the shade at room temperature. A quantity of 500 g of A. annua powder was taken and subjected to ultrasound treatment using petroleum ether for 30 min to remove fat. The resulting powder was then passed through a 60-mesh sieve. After the natural volatilization of petroleum ether, A. annua polysaccharide extraction was performed using a complex enzyme with a solid–liquid ratio of 1:30. The enzyme mixture consisted of cellulase, pectinase, and papain, added at proportions of 23.2, 19.7, and 15.6%, respectively. The mixture was incubated in a constant temperature shaker at 50°C and 150 rpm for 3 h. Subsequently, it was transferred to a 70°C water bath for 1 h to inactivate the enzymes. The resulting mixture was filtered and concentrated, followed by the addition of anhydrous ethanol into the concentrate at a 4:1 volume ratio. The solution was refrigerated in a 4°C refrigerator for 48 h, then the solution was centrifuged at 3000 × g for 5 min, and the precipitate was collected. The collected precipitate was washed with anhydrous ethanol and acetone, each three times. Subsequent to the washing, the precipitate was freeze-dried to obtain the A. annua polysaccharide. The total soluble sugar content of AAP was measured at 397.42 mg/g, while its molecular weight was identified as 14.639 kDa. AAP was consisted of rhamnose, arabinose, galactose, glucose, mannose, galacturonic acid, and glucuronic acid, with a molar ratio of 2.93:3.75:7.44:58.47:20.87:5.49:1.07. According to the previous study, the appropriate dosage of AAP is 750 mg/kg (Zhang, 2023).
2.3 Bacterial strains and culture
Escherichia. coli O78 (CVCC1490) was obtained from CVCC (China Veterinary Culture Collection Center). The strain was cultured at 37°C in Luria-Bertani (LB) broth (Guangdong Huankai Microbial Sci. & Tech. Co., Ltd., Guangzhou, China) for 24 h to reach a final concentration of 1.0 × 1012 CFU/mL in sterile LB liquid medium.
2.4 Experimental design and diets
A total of 256 Arbor Acres broilers were purchased from a commercial hatchery (Hohhot, China). These birds were randomly divided into four treatments according to their initial body weight including the control group (fed basal diet), the AAP group (fed basal diet supplemented with 750 mg/kg AAP), E. coli group (fed basal diet and orally administered E. coli (3.2 × 1011 CFU/kg body weight)), and AAP + E. coli group (fed basal diet supplemented with 750 mg/kg AAP and orally administered E. coli (3.2 × 1011 CFU/kg body weight)), respectively. Each treatment group had eight replicates with eight birds per replicate, half male and half female. The trial included the pre-feeding period (d 1 to 14), and the formal trial period (d 15 to 42). During d 15 to 20 (E. coli challenge phase I), the E. coli treatment groups were given oral administration of 0.5 mL E. coli saline suspension (2.5 × 1011 CFU/mL) in the morning, the control group was given the same amount of normal saline. During the earlier period (d 1 to 21), the chicks are characterized by an underdeveloped immune system and limited resistance, rendering them vulnerable to external pathogens. Additionally, their intestinal microflora is in the establishment stage, and is susceptible to environmental influence. This phase is provided an opportune time for the observation and analysis of the infection process, pathogenic mechanisms, and the broiler’s resistance to E. coli. As broilers progress into the growth period (d 22 to 42), their physiological and immune function gradually improve. This developmental stage presents an opportunity for deeper exploration of the infection characteristics and pathogenicity of E. coli at different growth stages. By conducting E. coli challenge experiments during this phase, valuable insights into the interplay between E. coli infection and broiler physiology can be gained, contributing to a more nuanced understanding of host-pathogen interactions in poultry production systems. Therefore, during d 36 to 42 (E. coli challenge phase II), the E. coli treatment groups were given oral administration of 2 mL E. coli saline suspension (3.2 × 1011 CFU/mL) in the morning, the control group was given the same amount of normal saline. By conducting E. coli challenges in two stages, the dynamics of E. coli infection in broilers can be comprehensively investigated, thus enhancing the understanding of its pathogenicity. The workflow is shown in Figure 1. Diets were formulated to meet the nutritional recommendations of the Feeding Standard of Chicken, China (NY/T 33-2004) (Table 1). The birds had ad libitum access to food and water. At the end of the experiment, eight birds were randomly selected from each treatment (one chicken per replicate) and slaughtered on d 21 and d 42, respectively. Blood was collected from wing veins, and the serum was separated and preserved at −20°C. Additionally, the jejunal tissues and chyme were promptly collected and stored at −80°C for further analysis.
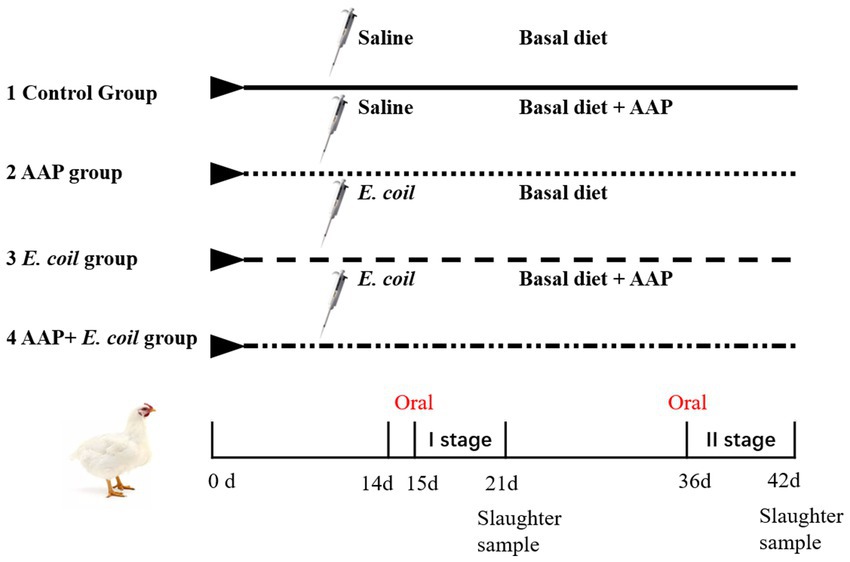
Figure 1. Flowchart of the broiler experiment. AAP Artemisia annua L. polysaccharide group, E. coli Escherichia. coli challenge group, AAP + E. coli Artemisia annua L. polysaccharide + E. coli challenge group.
2.5 Growth performance and nutrient apparent metabolic rate
On d 1, 14, 21, 35, and 42 of the experiment, the body weight (BW) and feed intake of experiment birds in each replicate were meticulously measured and recorded. Subsequently, the average daily gain (ADG), average daily feed intake (ADFI), and feed conversion ratio (FCR) were calculated for each stage of the experiment. During d 19 to 21 and d 40 to 42 of the experiment, feces was methodically collected from each replicate, and the fecal weight and feed intake of each replicate were meticulously recorded after continuous fecal collection for three consecutive days. The apparent nutrient retention was measured via the total feces collection method, and then calculating the apparent metabolic rate of feed dry matter (DM), crude protein (CP), crude fat (ether extract, EE), calcium (Ca), and phosphorus (P). To provide a more accurate representation of growth, the values were corrected for mortality rate.
2.6 Sample collection
On d 21 and 42, one bird was randomly selected from each replicate pen and weighed accurately. Blood samples were then collected from the wing vein and centrifuged (3,000 × g, 10 min) at 4°C. The serum was then carefully harvested and stored at −20°C until further analysis. The birds were humanely euthanized by cervical dislocation. The jejunum’s middle segment was carefully excised, rinsed with sterile cold saline, placed in a sterile tube, and then flash-frozen in liquid nitrogen. The samples were stored at −80°C for mRNA expression analysis. Additionally, 1 cm section of the jejunum was gently washed with 0.9% (w/vol) physiological saline and fixed in a 10% formalin solution for further morphological examination.
2.7 Intestinal permeability
The serum diamine oxidase (DAO, product code: A088-1, assay range: 0 U/L–100 U/L) was tested using commercial assay kits, read absorbance OD at 340 nm, the difference in inter-assay is less 10% (Jiancheng Bioengineering Institute, Co. Ltd., Nanjing, China). The serum endotoxins (ET, catalogue number: JYM0109Ch, assay range: 1.5 ng/mL–100 ng/mL) and D-lactate (D-LA, catalogue number: JYM0160Ch, assay range: 0.8 ng/mL–50 ng/mL) were tested using ELISA kits, read absorbance OD at 450 nm, the difference in intra-assay and inter-assay is less than 9 and 15%, respectively, (Wuhan Gene Beauty Biotechnology Co. Ltd., China).
2.8 Preparation of intestinal homogenate
The jejunal tissues were processed using a hand-held homogenizer (FA6/10, FLUKO, Shanghai, China) at 4°C in ice-cold 0.9% NaCl solution (wt/vol, 1:9) and then centrifuged at 4000 × g for 15 min at 4°C. The resulting supernatant was collected for further analysis. The protein content of the homogenate was determined using the Coomassie Brilliant Blue assay (product code: A045-2, absorbance OD at 595 nm) according to the manufacturer’s instructions for the commercial kits (Nanjing Jiancheng Institute of Bioengineering, Nanjing, China).
2.9 Immune indexes in serum and tissue
The concentration of interleukin-1 beta (IL-1β, catalogue number: JYM0041Ch, assay range: 1.5 pg/mL–100 pg/mL), interleukin-6 (IL-6, catalogue number: JYM0028Ch, assay range: 1 pg/mL–100 pg/mL), tumor necrosis factor-α (TNF-α, catalogue number: JYM0033Ch, assay range: 1.2 pg/mL–100 pg/mL), immunoglobulin A (IgA, catalogue number: JYM0012Ch, assay range: 1 μg/mL–70 μg/mL), immunoglobulin G (IgG, catalogue number: JYM0001Ch, assay range: 0.3 μg/mL–20 μg/mL), immunoglobulin M (IgM, catalogue number: JYM0060Ch, assay range: 8 ng/mL–450 ng/mL), and secretory immunoglobulin A (sIgA, catalogue number: JYM0036Ch, assay range: 15 pg/mL–1000 pg/mL) in the serum and intestinal tissue homogenate supernatant was analyzed using ELISA kits (Wuhan Gene Beauty Biotechnology Co. Ltd., China) following the manufacturer’s instructions, read absorbance OD at 450 nm, the difference in intra-assay and inter-assay is less than 9 and 15%, respectively.
2.10 Antioxidant indexes in serum and tissue
The total antioxidant capacity (TAC, product code: A015-2-1, assay range: 0.5 mM–2 mM, absorbance OD at 405 nm), the activity of total superoxide dismutase (SOD, product code: A001-1, absorbance OD at 550 nm), glutathione peroxidase (GPx, product code: A005, absorbance OD at 412 nm), and catalase (CAT, product code: A007-1-1, absorbance OD at 405 nm), and the concentration of glutathione (GSH, product code: A006-2-1, absorbance OD at 405 nm) and malondialdehyde (MDA, product code: A003-1, absorbance OD at 532 nm) in the serum and intestinal tissue were determined by a spectrophotometric method according to the instructions of the commercial kits (Nanjing Jiancheng Institute of Bioengineering, Nanjing, China).
2.11 Intestinal morphology
A small portion of the jejunum tissues were fixed in 10% formalin and then embedded in paraffin, then sliced into thin sections with a thickness of 7 μm using a rotary microtome (YD-1508R Rotary Slicer, Yidi Medical Equipment Factory, Jinhua, Zhejiang, China), and stained with hematoxylin and eosin. The 10 intact villi of each tissue were precisely measured for villus height (VH) and crypt depth (CD) by high-resolution photography under 100× magnification using a light microscope (Olympus SZX10, Tokyo, Japan), and the average values for each tissue for each tissue were calculated.
2.12 Total RNA extraction and reverse transcription
Total RNA from jejunal tissue samples was obtained using Trizol reagent (TaKaRa Biotechnology Co. Ltd., Dalian, China). The purity and quantity of the total RNA were assessed with a spectrophotometer (Pultton P200CM, San Jose, CA, United States). Subsequently, the DNA of total RNA was removed by incubation for 2 min at 42°C with a gDNA digester (Yeasen Biotechnology Co., Ltd. Shanghai, China). Total RNA was reverse transcribed to cDNA on Labcycler (SensoQuest GmbH, Göttingen, Germany) using Hifair® II SuperMix plus (Yeasen Biotechnology Co., Ltd. Shanghai, China). The reactions were incubated for 5 min at 85°C, 30 min at 42°C, and 5 min at 85°C.
2.13 Quantitative real-time PCR
Real-time PCR was performed using LightCycler® 96 instrument and application software analysis system (LightCycler® 96 Instrument, Roche Diagnostics, Indiana, United States) with a Hieff® qPCR SYBR® Green Master Mix (No Rox) Kit (Yeasen Biotechnology Co., Ltd. Shanghai, China). The reactions were: 95°C for 30 s (hold stage), followed by 40 cycles of 95°C for 5 s, 60°C for 30 s, and 72°C for 20 s (PCR stage), then 95°C for 15 s, 60°C for 1 min, 95°C for 15 s (melt-curve stage). All samples were run in duplicate in 10 μL reaction volume and melt curve analysis was performed to ensure the specificity of the PCR-amplified product. The mRNA expression of each gene was normalized to that of 𝛽-actin. The fold change relative to the control group was analyzed according to the 2−ΔΔCT method. The specific sequences of primers are listed in Table 2.
2.14 16S rRNA amplicon sequencing and bioinformatics
Microbial community genomic DNA was extracted from jejunum content samples using the E.Z.N.A.® soil DNA Kit (Omega Bio-tek, Norcross, GA, United States) according to manufacturer’s instructions. The DNA extract was checked on 1% agarose gel, and DNA concentration and purity were determined with NanoDrop 2000 UV–vis spectrophotometer (Thermo Scientific, Wilmington, United States). The hypervariable region V3-V4 of the bacterial 16S rRNA gene were amplified with primer pairs 338F (5’-ACTCCTACGGGAGGCAGCAG-3′) and 806R (5’-GGACTACHVGGGTWTCTAAT-3′) by an ABI GeneAmp® 9,700 PCR thermocycler (ABI, CA, United States). The PCR amplification of 16S rRNA gene was performed as follows: initial denaturation at 95°C for 3 min, followed by 27 cycles of denaturing at 95°C for 30 s, annealing at 55°C for 30 s and extension at 72°Cfor 45 s, and single extension at 72°C for 10 min, and end at 10°C. The PCR mixtures contain 5 × Pro Taq 10 μL, forward primer (5 μM) 0.8 μL, reverse primer (5 μM) 0.8 μL, template DNA 10 ng/μL, and finally ddH2O up to 20 μL. PCR reactions were performed in triplicate. The PCR product was extracted from 2% agarose gel and purified using the AxyPrep DNA Gel Extraction Kit (Axygen Biosciences, Union City, CA, United States) according to manufacturer’s instructions and quantified using Quantus™ Fluorometer (Promega, United States). Purified amplicons were pooled in equimolar and paired-end sequenced on an Illumina MiSeq PE300 platform/NovaSeq PE250 platform (Illumina, San Diego, United States) according to the standard protocols by Majorbio Bio-Pharm Technology Co. Ltd. (Shanghai, China). The raw 16S rRNA gene sequencing reads were demultiplexed, quality-filtered by fastp version 0.20.0 and merged by Flash version 1.2.7. Operational taxonomic units (OTUs) with 97% similarity cutoff were clustered using Uparse version 7.1, and chimeric sequences were identified and removed. The taxonomy of each OTU representative sequence was analyzed by RDP Classifier version 2.2 against the 16S rRNA database (Silva v138) using confidence threshold of 0.7. α diversity, β diversity, community composition, and analysis of different communities are carried out on the I-Sanger Cloud Platform provided by Majorbio Bio-Pharm Technology Co., Ltd. (Qiao et al., 2022b).
2.15 Statistical analysis
Data were analyzed by one-way ANOVA with the general linear model procedure of SAS version 9.2 (SAS Institute Inc., Cary, NC), and a pen of broilers (a replicate) served as the experimental unit for all data. The differences among treatments were tested by Duncan’s multiple comparison analysis and were considered significant at p < 0.05. The results were expressed as the mean and standard error of the mean (SEM).
3 Results
3.1 Growth performance and nutrient apparent metabolic rate
As shown in Table 3, compared with the control group, dietary AAP significantly increased BW of broilers on d 42 (p < 0.01). E. coli-challenged broilers had significantly decreased BW on d 21, d 35, and d 42, ADG and ADFI on d 36–42 (p < 0.05). And there was no difference between AAP + E. coli group and control group. In addition, dietary AAP supplementation significantly enhanced ADG compared to the control group during d 36–42 (p < 0.01). However, there was no difference in FCR among treatment groups.
As described in Figure 2, compared with the control group, dietary AAP supplementation significantly increased the apparent metabolic rate of CP on d 21 and 42 (p < 0.05). The apparent metabolic rate of P on d 21, EE and Ca on d 42 in E. coli-challenged broilers was significantly lower than that of control group (p < 0.05). And there was no difference between AAP + E. coli group and the control group.
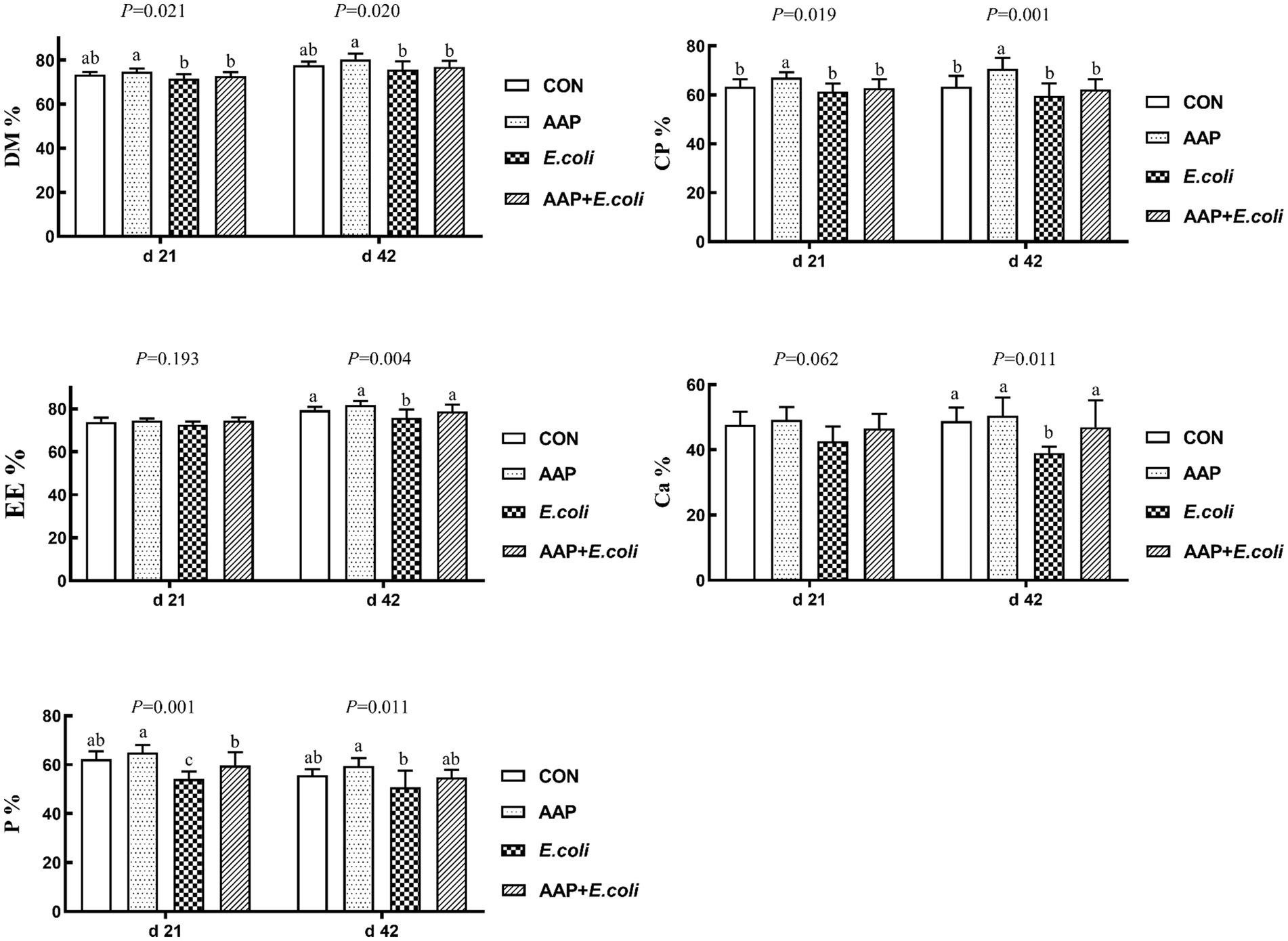
Figure 2. Effects of AAP on apparent nutrient metabolic rate of broilers challenged by E. coli. CON control group, AAP Artemisia annua L. polysaccharide group, E. coli Escherichia. coli group, AAP + E. coli Artemisia annua L. polysaccharide + Escherichia. coli group, DM dry matter, CP crude protein, EE ether extract, Ca calcium, P phosphorus. a,b,cDifferent letters in the same period indicate significant differences between groups (p < 0.05).
3.2 Intestinal permeability
As summarized in Table 4, compared with the control group, E. coli-challenged broilers had significantly increased DAO activity on d 21 and 42, and ET content on d 42 (p < 0.05), and there was no difference between the AAP + E. coli group and the control group.
3.3 Intestinal morphology
As illustrated in Figure 3, compared with the control group, E. coli-challenged broilers had reduced VH on d 21 (p = 0.055) and 42 (p < 0.05), and VH/CD d 21 and 42 (p < 0.01), but there was no difference between the AAP + E. coli group and the control group. Moreover, dietary AAP supplementation significantly enhanced VH/CD on d 42 (p < 0.01).
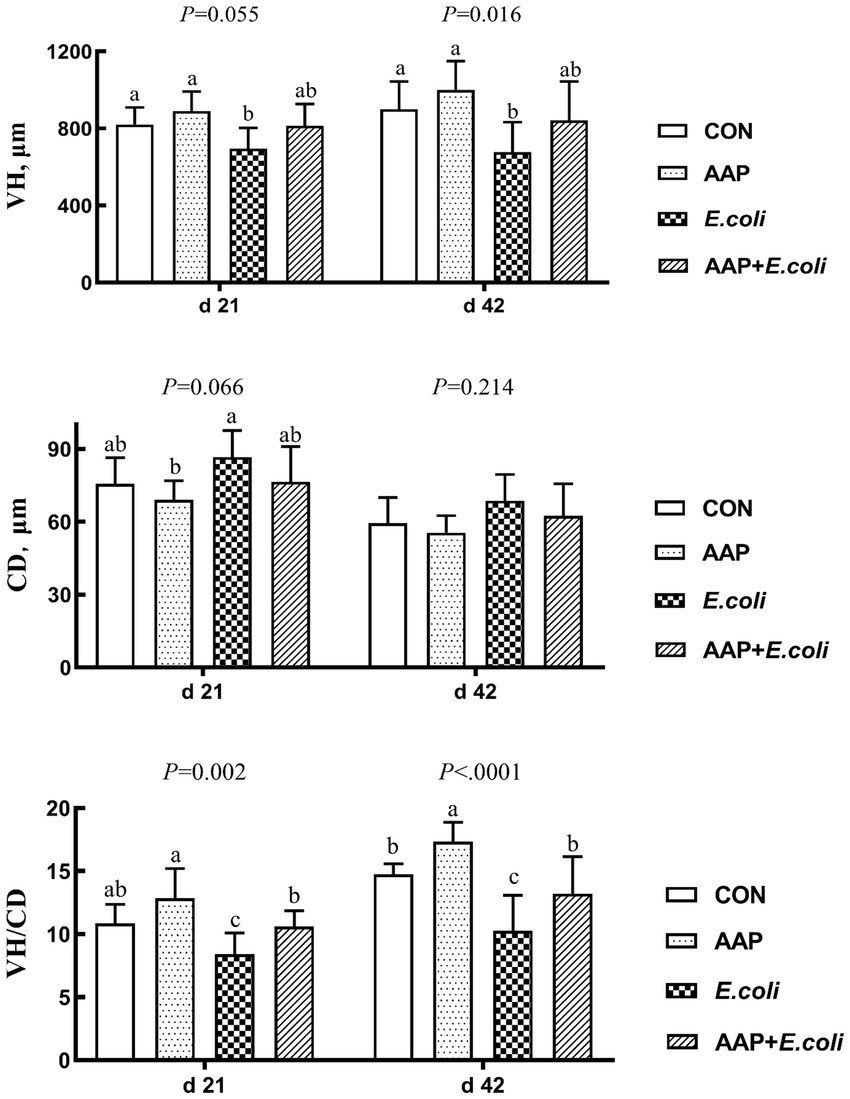
Figure 3. Effects of AAP on intestinal morphology of broilers challenged by E. coli. CON control group, AAP Artemisia annua L. polysaccharide group, E. coli Escherichia. coli group, AAP + E. coli Artemisia annua L. polysaccharide + Escherichia. coli group, VH villus height, CD crypt depth, VH/CD villus height to crypt depth ratio. a–cDifferent letters in the same period indicate significant differences between groups (p < 0.05).
3.4 Immune indexes in serum and tissue
As shown in Table 5, dietary AAP supplementation significantly increased serum IgA content on d 21 (p < 0.01). Compared with the control group, E. coli-challenged broilers significantly reduced serum IgM content on d 21 (p < 0.05) and increased IL-6 content on d 42 (p < 0.01), and there was no difference between the AAP + E. coli group and the control group.
As presented in Table 6, compared with the control group, E. coli-challenged broilers had significantly decreased jejunum IgG content (p < 0.05), but there was no difference between the AAP + E. coli group and the control group on d 21. The jejunum IgM content of E. coli group and AAP + E. coli group was significantly lower than that of the control group on d 21 (p < 0.01). Compared with the control group, E. coli-challenged broilers markedly increased jejunum IL-1β and IL-6 content (p < 0.05), but there was no difference between the AAP + E. coli group and the control group on d 42.
3.5 Antioxidant indexes in serum and tissue
As shown in Table 7, compared with the control group, E. coli-challenged broilers had significantly reduced serum GPx activity on d 21 (p < 0.05), but there was no difference between the AAP + E. coli group and the control group. Compared with the control group, dietary AAP inclusion had notably decreased serum MDA concentration on d 21 (p < 0.01).
As presented in Table 8, compared with the control group, E. coli-challenged broilers had significantly reduced jejunum TAC, CAT, SOD activity (p < 0.05), but there was no difference between the AAP + E. coli group and the control group on d 42. Moreover, the jejunum MDA content of E. coli-challenged broilers trended to be higher than that of the control group on d 21 (p = 0.098) and 42 (p = 0.052).
3.6 Intestinal tight junction protein-related mRNA expression
As shown in Figure 4, compared with the control group, dietary AAP supplementation extremely significantly increased jejunum mRNA expression levels of Occludin (d 21), ZO-1 (d 42) and Mucin-2 (d 42) (p < 0.01). Besides, compared with the control group, E. coli-challenged broilers noticeably reduced jejunum mRNA expression level of Claudin-1 (d 21), Occludin (d 21 and 42), ZO-1 (d 42) and Mucin-2 (d 21 and 42) (p < 0.05), but there was no difference between AAP + E. coli group and control group.
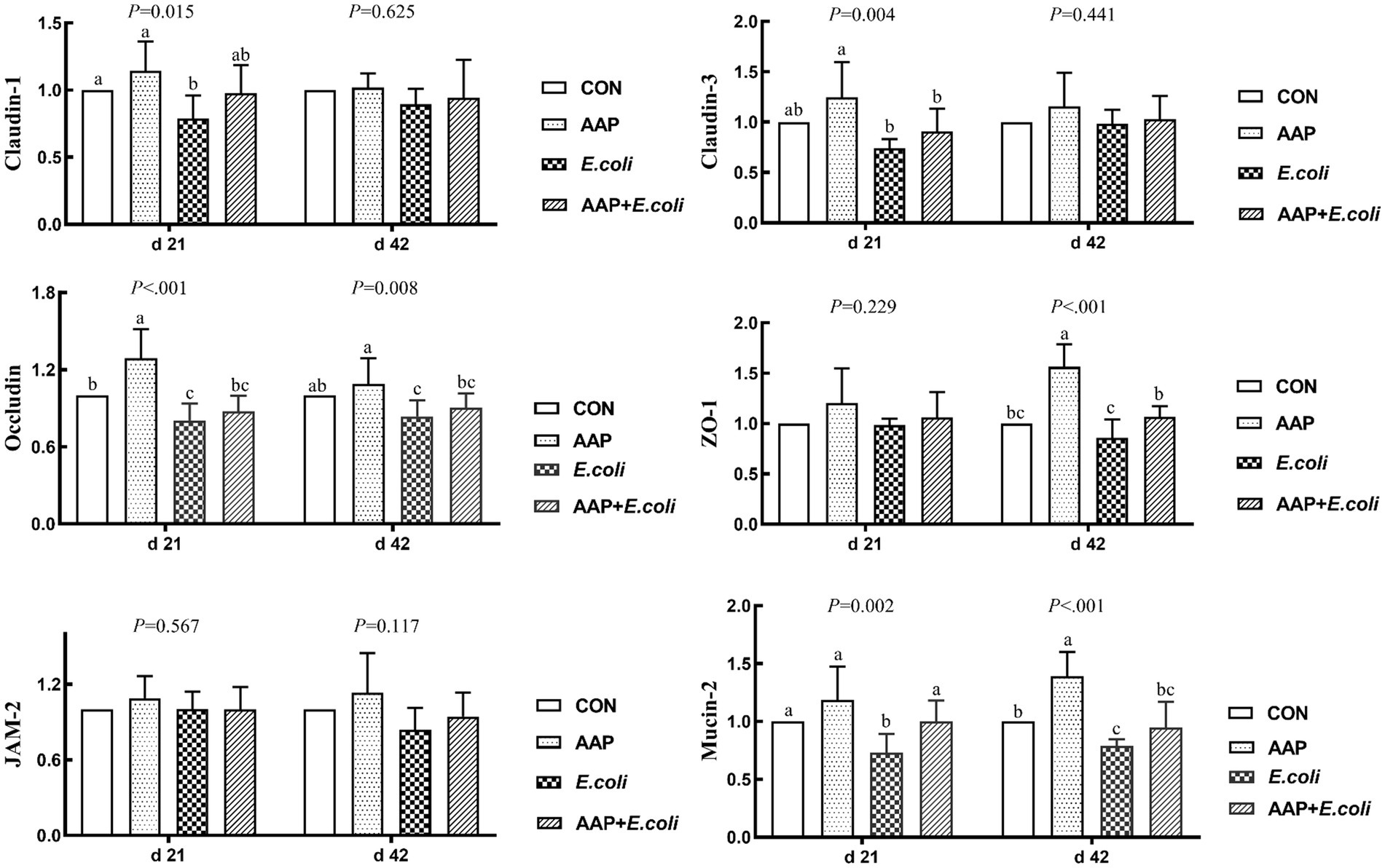
Figure 4. Effects of AAP on tight junction protein mRNA expression level of broilers challenged by E. coli. CON control group, AAP Artemisia annua L. polysaccharide group, E. coli Escherichia. coli group, AAP + E. coli Artemisia annua L. polysaccharide + Escherichia. coli group, ZO-1 zonula occludens-1, JAM-2 junctional adhesion molecule-2. a–cDifferent letters in the same period indicate significant differences between groups (p < 0.05).
3.7 Intestinal proinflammatory factor-related mRNA expression
As indicated in Figure 5, compared with the control group, E. coli-challenged broilers had extremely significantly increased jejunum mRNA expression level of IL-1β and TLR4 (p < 0.01), while there was no difference between the AAP + E. coli group and the control group on d 21 and 42. Compared with the control group, E. coli group and AAP + E. coli group significantly increased jejunum mRNA expression level of IL-6, but which was markedly lower in AAP + E. coli group than that in the E. coli group on d 21 and 42 (p < 0.01). Compared with the control group, E. coli-challenged broilers extremely significantly increased jejunum mRNA expression level of TNF-α (d 21) and MyD88 (d 42) (p < 0.01), but there was no difference between the AAP + E. coli group and the control group.
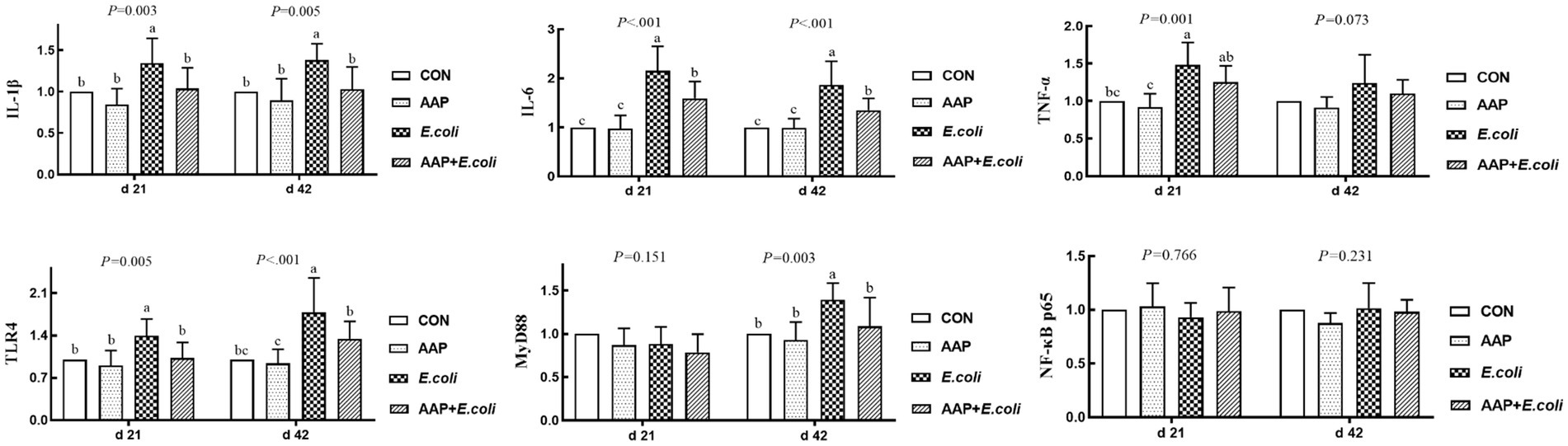
Figure 5. Effects of AAP on inflammatory-related mRNA expression level of broilers challenged by E. coli. CON control group, AAP Artemisia annua L. polysaccharide group, E. coli Escherichia. coli group, AAP + E. coli Artemisia annua L. polysaccharide + Escherichia. coli group, IL-1β interleukin 1 beta, IL-6 interleukin 6, TNF-α tumor necrosis factor α, TLR4 toll like receptor 4, MyD88 myeloid differentiation primary response 88, NF-κB p65 nuclear factor kappa B p65. a–cDifferent letters in the same period indicate significant differences between groups (p < 0.05).
3.8 Intestinal antioxidant-related mRNA expression
As shown in Figure 6, compared with the control group, E. coli-challenged broilers dramatically decreased jejunum mRNA expression level of CAT and Nrf2 (p < 0.01), but there was no difference between the AAP + E. coli group and the control group on d 21. Dietary AAP supplementation extremely significantly increased jejunum mRNA expression level of CAT (d 42), SOD (d 42; p = 0.079) and Nrf2 (d 21 and 42) (p < 0.01). Besides, compared with the control and AAP group, E. coli group and AAP + E. coli group markedly significantly increased the jejunum mRNA expression level of Keap1 on d 21 and 42 (p < 0.01), however, AAP + E. coli group had significantly lower jejunum mRNA expression level of Keap1 than E. coli group on d 42 (p < 0.01).
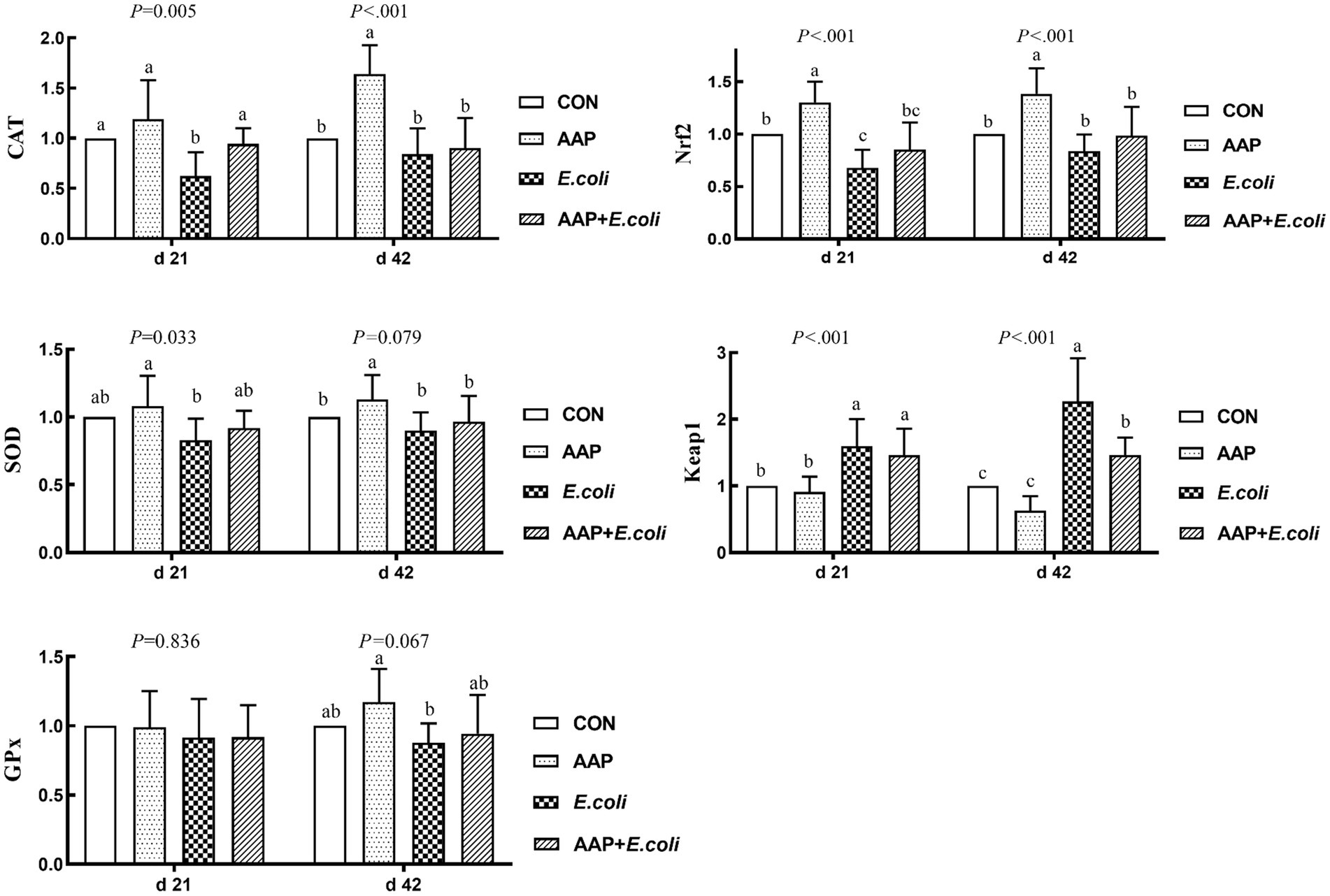
Figure 6. Effects of AAP on antioxidant-related mRNA expression level of broilers challenged by E. coli. CON control group, AAP Artemisia annua L. polysaccharide group, E. coli Escherichia. coli group, AAP + E. coli Artemisia annua L. polysaccharide + Escherichia. coli group, CAT catalase, SOD total superoxide dismutase, GPx glutathione peroxidase, Nrf2 nuclear factor erythroid-2-related factor 2, Keap1 kelch like ECH associated protein 1. a–cDifferent letters in the same period indicate significant differences between groups (p < 0.05).
3.9 Intestinal microbial analysis
The bacterial α diversity indices are presented in Figures 7, 8. There was no significant difference in α diversity indices (Sobs, Chao, Simpson, Shannon, Ace, and Coverage) on d 21 (Figures 7A–F; p > 0.05). However, the Simpson indexes (Figure 7C; p = 0.075) and Ace indexes (Figure 7E; p = 0.074) of the E. coli group tended to be significantly higher and lower, respectively, than those of the other groups. On d 42, E. coli-challenged broilers significantly increased the indexes of Sobs, Chao, Shannon and Ace (Figures 8A,B,D,E; p < 0.05), and significantly decreased the indexes of Simpon and Coverage (Figures 8C,F; p < 0.05). However, there was no difference between the AAP + E. coli group and the control group.
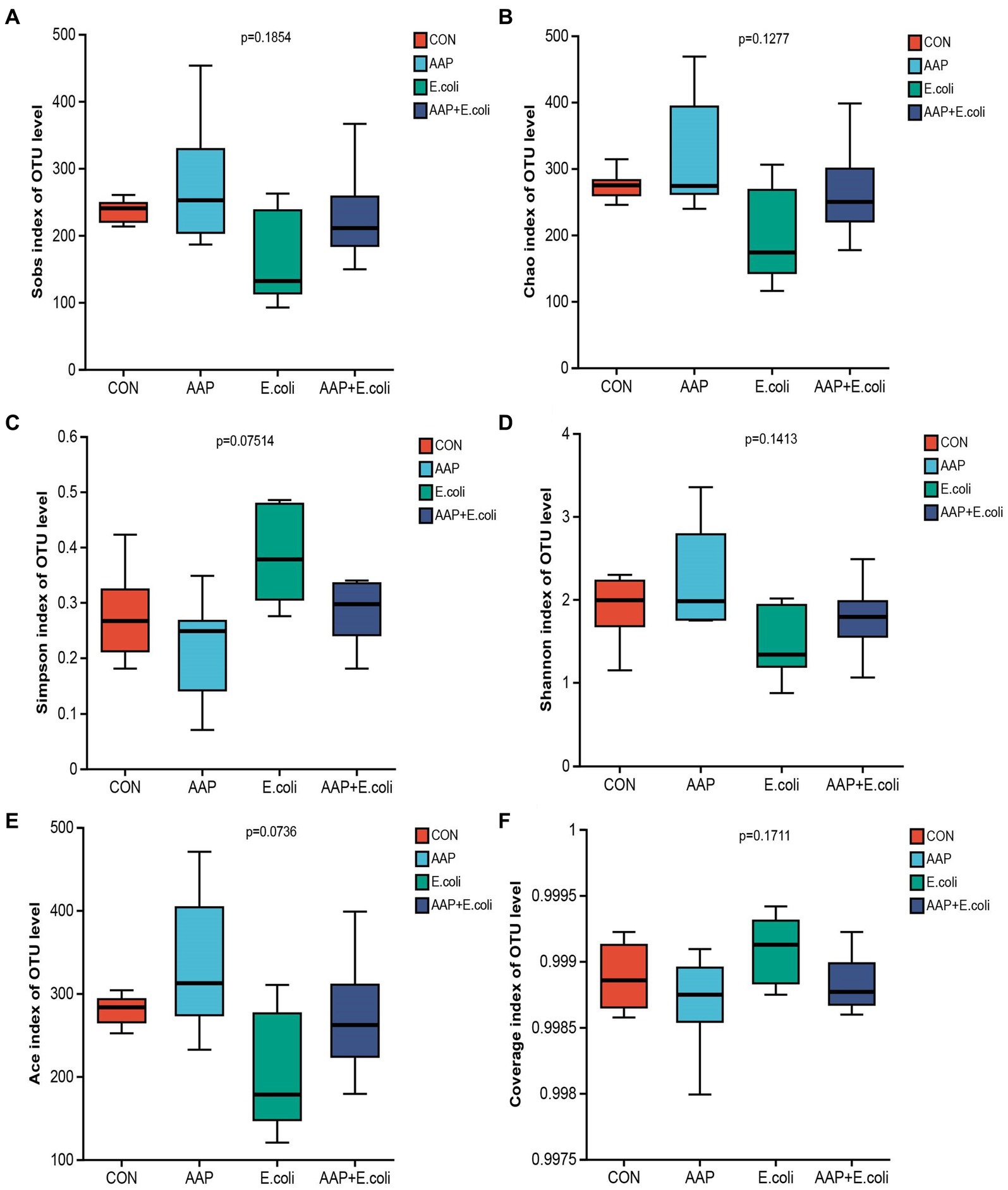
Figure 7. Effects of AAP on α diversity index of jejunal microbiota of broilers on d 21 challenged by E. coli. CON control group, AAP Artemisia annua L. polysaccharide group, E. coli Escherichia. coli group, AAP + E. coli Artemisia annua L. polysaccharide + Escherichia. coli group. Sobs index, Chao index, Simpson index, Shannon index, Ace index, Coverage index (A–F).
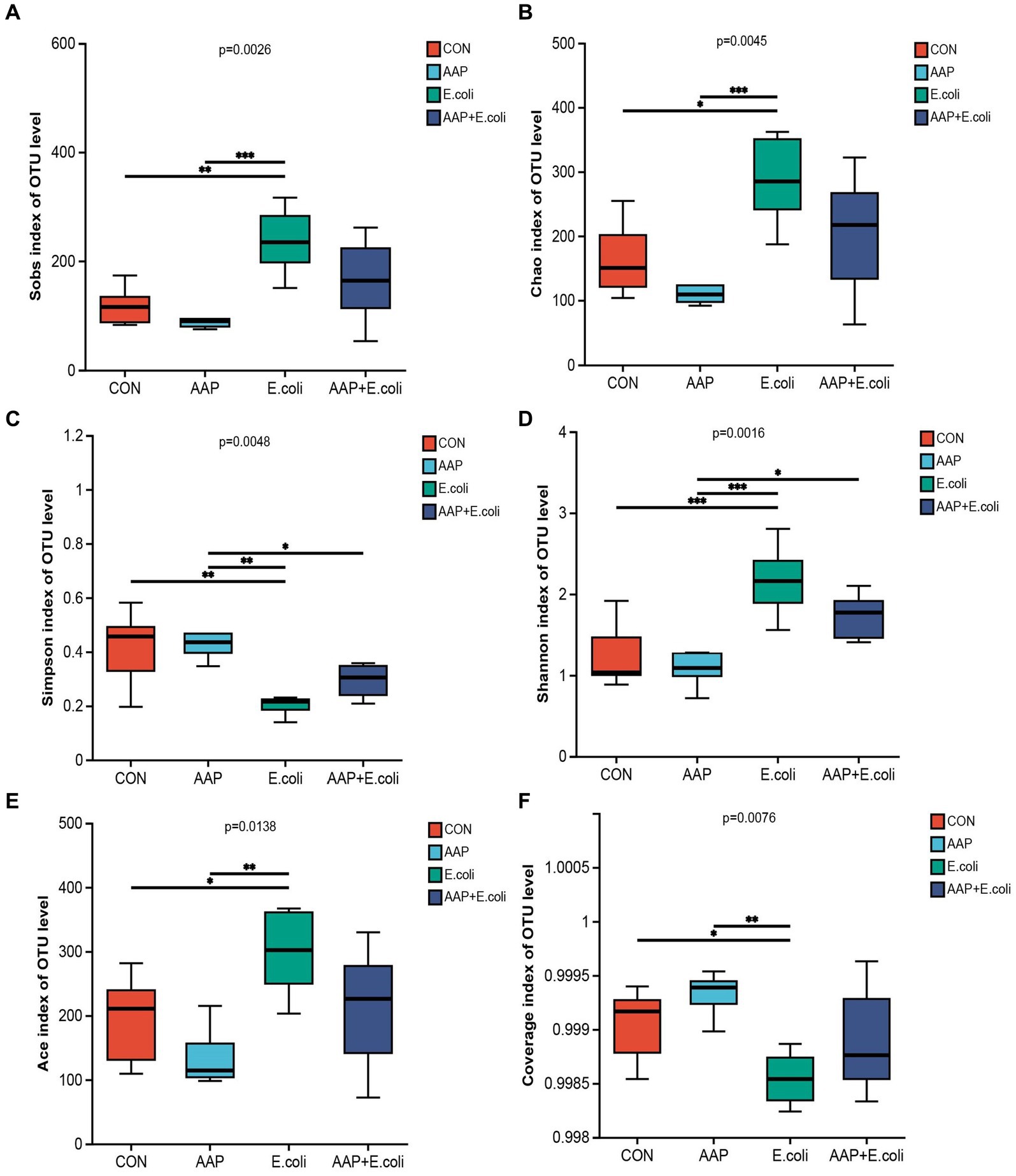
Figure 8. Effects of AAP on α diversity index of jejunal microbiota of broilers on d 42 challenged by E. coli. CON control group, AAP Artemisia annua L. polysaccharide group, E. coli Escherichia. coli group, AAP + E. coli Artemisia annua L. polysaccharide + Escherichia. coli group. Sobs index, Chao index, Simpson index, Shannon index, Ace index, Coverage index (A–F). Symbol “*,” “**,” “***” indicates the significant difference based on p < 0.05, p < 0.01, p < 0.001.
On d 21, the Venn diagram (Figure 9A) showed that a total of 322 OTU is shared among the 4 treatment groups. In addition, the unique OUT numbers corresponding to the control group, AAP group, E. coli group, and AAP + E. coli group were, respectively, 71, 248, 39, and 105 on d 21. Principal co-ordinates analysis (PCoA) (Figure 9B) showed that the microbial community composition changed among the four treatment groups on d 21. The composition of the jejunal microbiota is shown in Figure 9 on d 21. At the phylum level, the dominant bacteria were Firmicutes, Proteobacteria, Cyanobacteria, Patescibacteria, and Actinobacteria (Figure 9C). The abundance of Desulfobacterota in phylum level in AAP group and AAP + E. coli group was significantly higher than that in control group and E. coli group (Figure 9D; p < 0.05). At the genus level, the dominant bacteria were Lactobacillus, Streptococcus, Enterococcus, Novosphingobium, and Romboutsia (Figure 9E). Compared with the control group and E. coli group, the AAP group and AAP + E. coli group significantly decreased the abundance of norank_f_Obscuribacteraceae, Ralstonia, Mitsuokella, Megasphaera, Megamonas and Bifidobacterium, and significantly increased the abundance of Aerococcus, Desulfovibrio and Candidatus_Saccharimonas at the genus level (Figure 9F; p < 0.05). Besides, the abundance of Enterorhabdus in the AAP + E. coli group was significantly higher than that in the other groups (Figure 9F; p < 0.05).
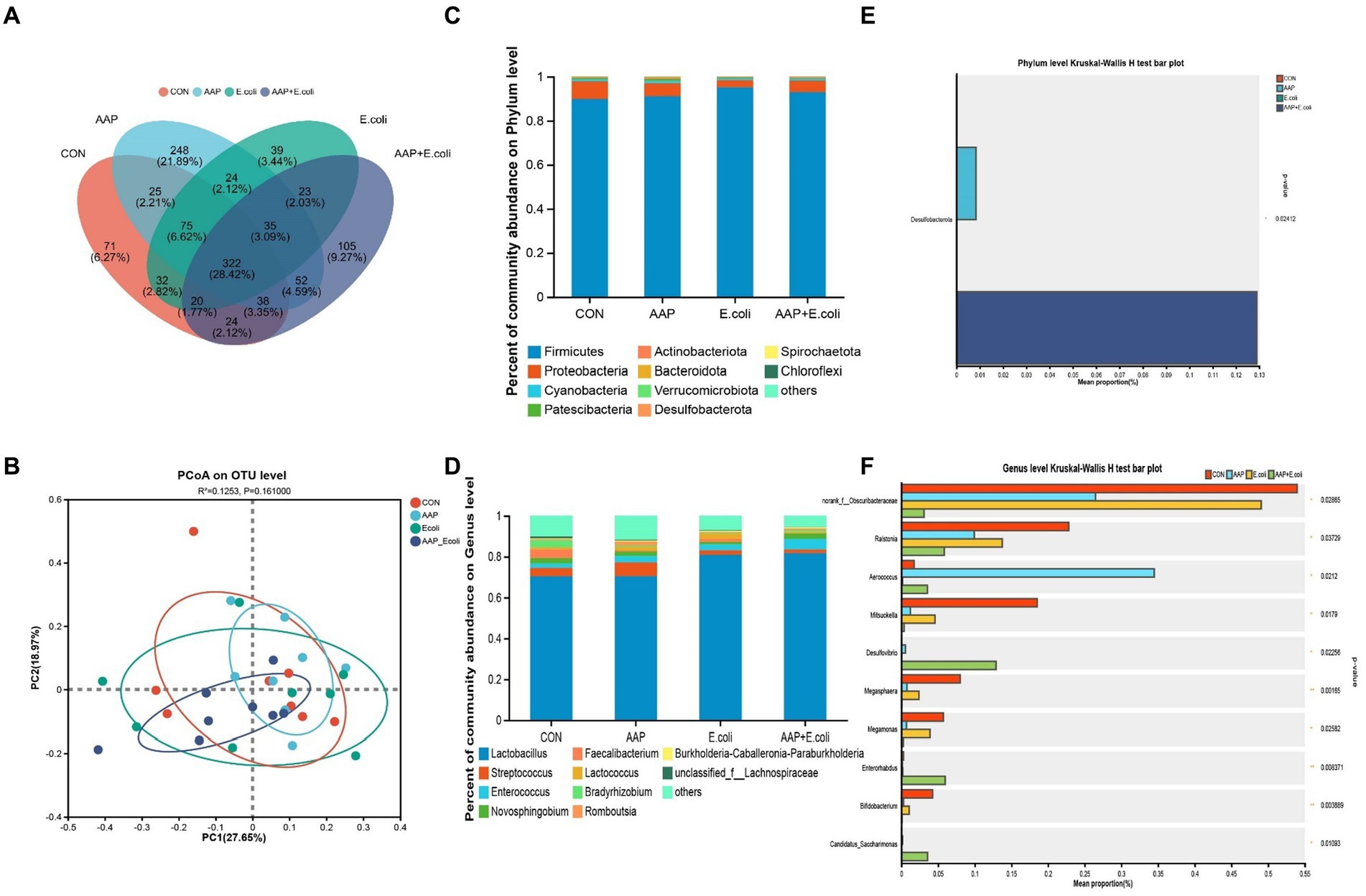
Figure 9. Effects of AAP on microbial composition of jejunal microbiota of broilers on d 21 challenged by E. coli. CON control group, AAP Artemisia annua L. polysaccharide group, E. coli Escherichia. coli group, AAP + E. coli Artemisia annua L. polysaccharide + Escherichia. coli group. Venn diagram based on OTU (A). Principal coordinate analysis (PCoA) plot (B). Jejunal microbiota composition at the phylum level, and alterations of the abundance of bacterial phylum found in the jejunum of broilers (C,D). Jejunal microbiota composition at the genus level, and alterations of the abundance of bacterial genus found in the jejunum of broilers (E,F).
On d 42, the Venn diagram (Figure 10A) showed that a total of 227 OTU is shared among the 4 treatment groups. In addition, the unique OUT numbers corresponding to the control group, AAP group, E. coli group, and AAP + E. coli group were, respectively, 183, 24, 111, and 81 on d 42. Principal co-ordinates analysis (PCoA) (Figure 10B) showed that the microbial community composition changed dramatically among the four treatment groups on d 42. The composition of the jejunal microbiota is shown in Figure 10 on d 42. At the phylum level, the dominant bacteria were Firmicutes, Proteobacteria, Actinobacteria, Cyanobacteria, and Bacteroidota (Figure 10C). The abundance of Bacteroidota in AAP + E. coli group was significantly higher than the other treatment groups at the phylum level (Figure 10D; p < 0.05). At the genus level, the dominant bacteria were Lactobacillus, Streptococcus, Enterococcus, Lactococcus, and Turicibacter (Figure 10E). Compared with the control group and AAP group, the E. coli group and AAP + E. coli group significantly decreased the abundance of Lactobacillus, and significantly increased the abundance of Romboutsia, Turicibacter, Christensenellaceae_R-7_group, UCG-005, Eisenbergiella, unclassified_f_Lachnospiraceae, norank_f_norank_o_Clostridia_UCG-014, Ruminococcus_torques_group and unclassified_f_Peptostreptococcaceae at the genus level (Figure 10F; p < 0.05). Among them, the abundance of Christensenellaceae_R-7_group, UCG-005, Eisenbergiella, unclassified_f_Lachnospiraceae, norank_f_norank_o_Clostridia_UCG-014, Ruminococcus_torques_group and unclassified_f_Peptostreptococcaceae in AAP + E. coli group was significantly higher than that in E. coli group (Figure 10F; p < 0.05).
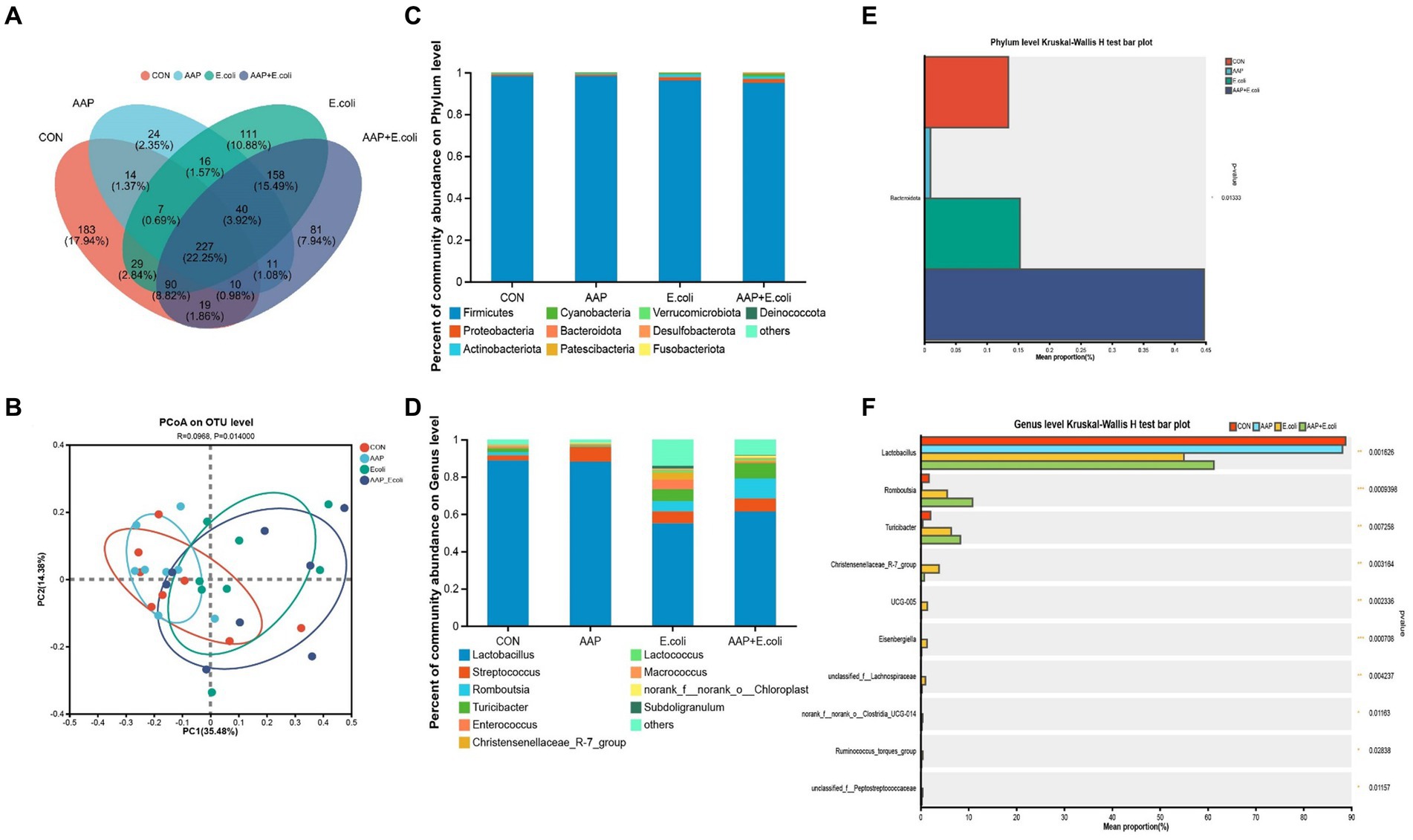
Figure 10. Effects of AAP on microbial composition of jejunal microbiota of broilers on d 42 challenged by E. coli. CON control group, AAP Artemisia annua L. polysaccharide group, E. coli Escherichia. coli group, AAP + E. coli Artemisia annua L. polysaccharide + Escherichia. coli group. Venn diagram based on OTU (A). Principal coordinate analysis (PCoA) plot (B). Jejunal microbiota composition at the phylum level, and alterations of the abundance of bacterial phylum found in the jejunum of broilers (C,D). Jejunal microbiota composition at the genus level, and alterations of the abundance of bacterial genus found in the jejunum of broilers (E,F).
The linear discriminant analysis (LDA = 3) effect size (LEfSe) algorithm was used to analyze the taxonomic abundance of microbiota. The results for d 21 are shown in Figures 11A,B. c_Vampirivibrionia, f_Obscuribacteraceae, g_norank_f_Obscuribacteraceae, o_Obscuribacterales, c_Negativicutes, o_Veillonellales-Selenomonadales, f_Veillonellaceae, o_Streptosporangiales, g_Ureibacillus, and f_Selenomonadaceae were enhanced in the control group. f_Streptococcaceae, f_Aerococcaceae, g_Aerococcus, and g_Harryflintia were enhanced in AAP group. g_Candidatus_Saccharimonas, g_Aureimonas, and g_norank_f_Lachnospiraceae were enhanced in AAP + E. coli group. The results for d 42 are shown in Figures 12A,B. g_Lactobacillus and f_Lactobacillaceae were enhanced in the control group. o_Lactobacillales and c_Bacilli were enhanced in AAP group. c_Clostridia, g_Ruminococcus_gauvreauii_group, o_Oscillospirales, o_Lachnospirales, f_Lachnospiraceae, o_Christensenellales, f_Christensenellaceae, g_Christensenellaceae_R-7_group, g_Dubosiella, f_Ruminococcaceae, f_Oscillospiraceae, g_Sellimonas, g_UCG-005, and g_Eisenbergiella were enhanced in E. coli group (LDA ≥ 4). o_Peptostreptococcales-Tissierellales, f_Peptostreptococcaceae, g_Romboutsia, o_Erysipelotrichales, f_Erysipelotrichaceae, g_Turicibacter, g_Brevibacterium, f_Brevibacteriaceae, and f_Geodermatophilaceae were enhanced in AAP + E. coli group (LDA ≥ 4).
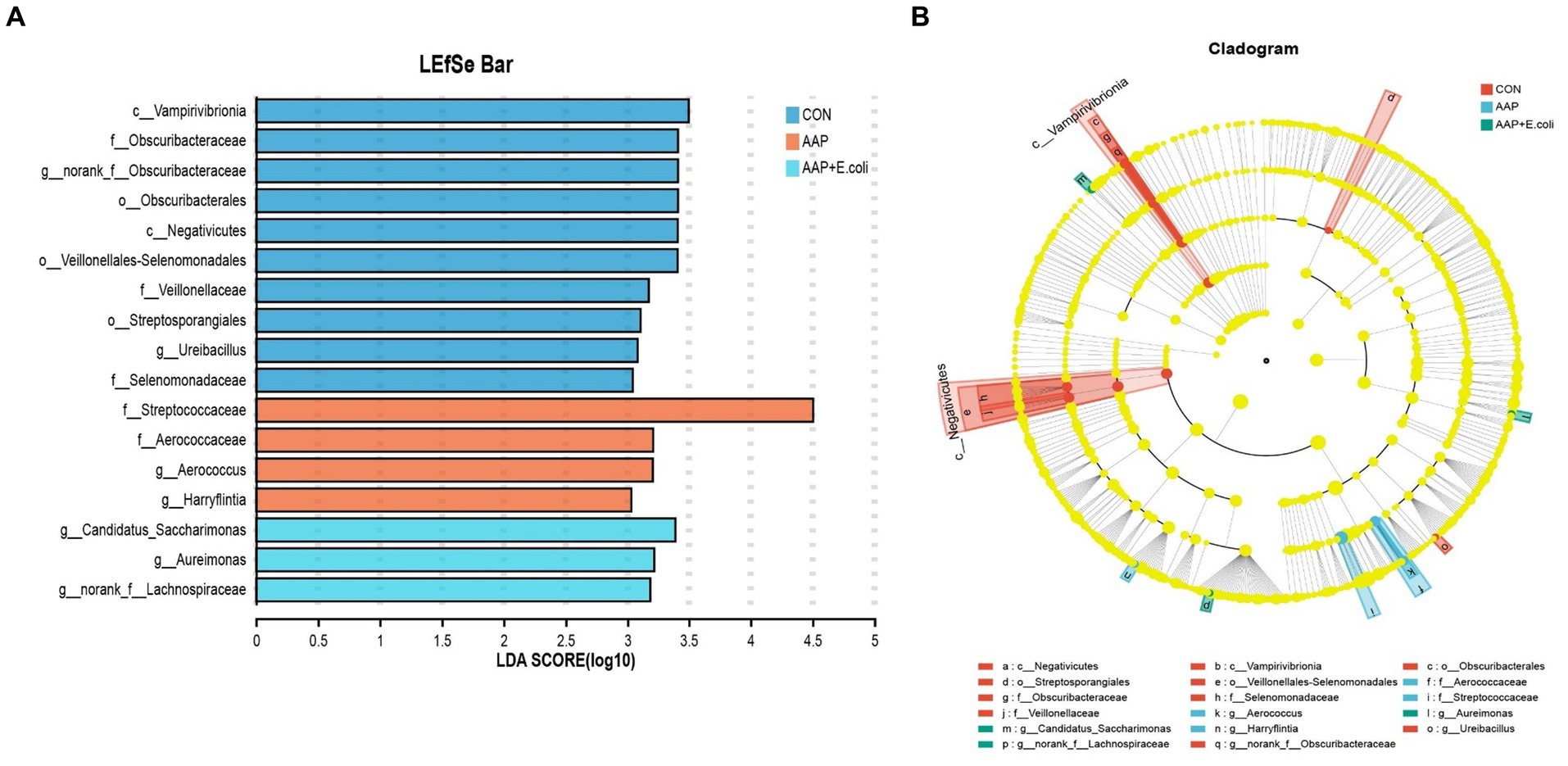
Figure 11. Linear discriminant analysis (LDA) effect size (LEfSe) analysis of jejunal microbiota on d 21. CON control group, AAP Artemisia annua L. polysaccharide group, E. coli Escherichia. coli group, AAP + E. coli Artemisia annua L. polysaccharide + Escherichia. coli group. LDA bar chart (A). LDA cladogram (B). LDA scores generated for the differentially abundant microbiota (LDA > 3, p < 0.05).
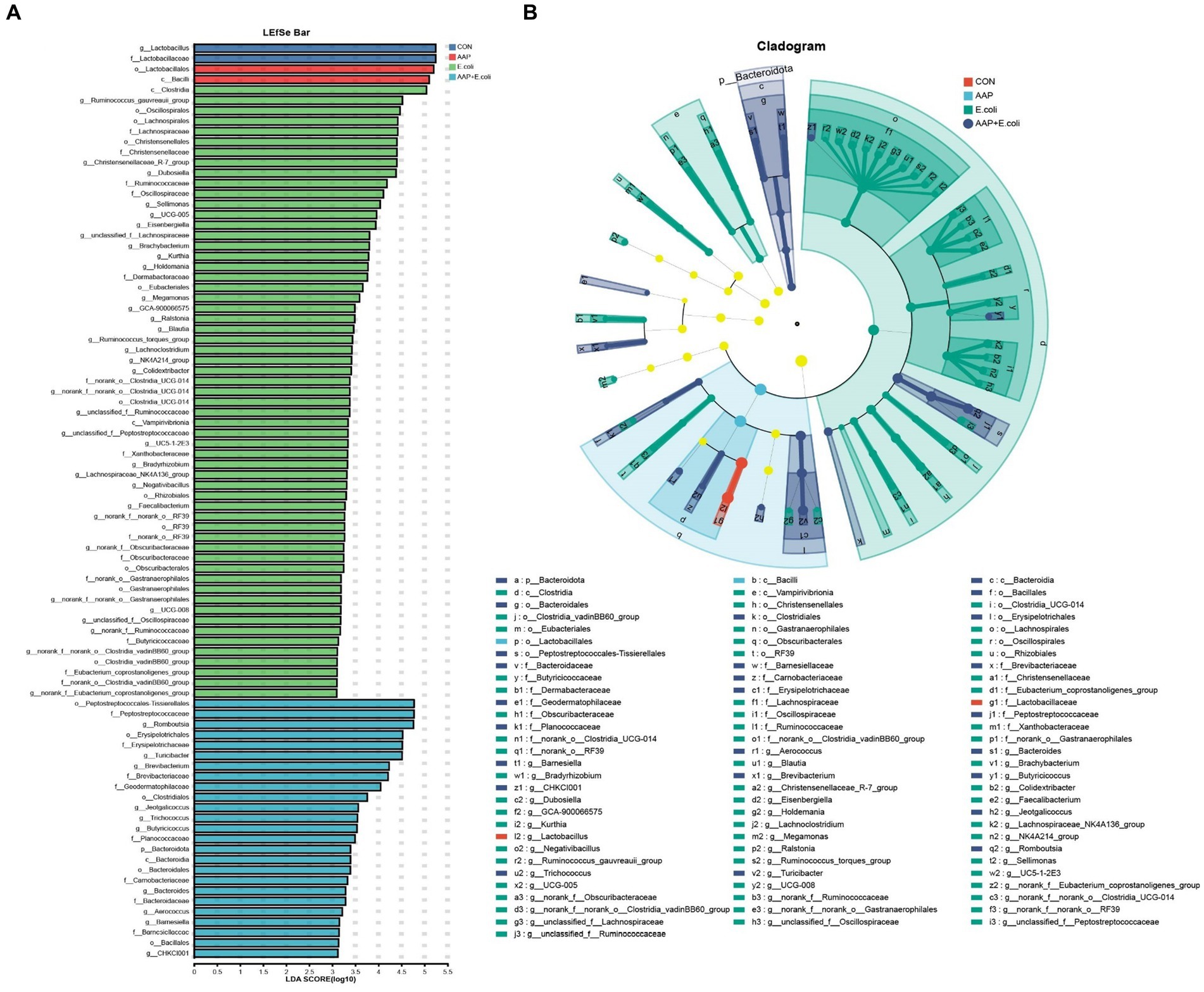
Figure 12. Linear discriminant analysis (LDA) effect size (LEfSe) analysis of jejunal microbiota on d 42. CON control group, AAP Artemisia annua L. polysaccharide group, E. coli Escherichia. coli group, AAP + E. coli Artemisia annua L. polysaccharide + Escherichia. coli group. LDA bar chart (A). LDA cladogram (B). LDA scores generated for the differentially abundant microbiota (LDA > 3, p < 0.05).
The co-occurrence network diagram of intestinal microbial at phylum and genus levels is depicted in Figure 13. The results for d 21 are shown in Figures 13A,B. At the phylum level, p_Acidobacteriota, p_Deinococcota, and p_WPS-2 were only enriched in the AAP group (Figure 13A). p_unclassified_k_norank_d_Bacteria were only enriched in the AAP + E. coli group (Figure 13A). At the genus level, g_norank_f_Caulobacteraceae, g_unclassified_f_Lachnospiraceae, g_Lachnoclostridium, g_Eisenbergiella, and g_UCG-005 were only enriched in the control group (Figure 13B). g_Christensenellaceae_R-7_group, g_Clostridium_sensu_stricto_1, g_Gemmobacter, g_norank_f_norank_o_Clostridia_UCG-014, and g_unclassified_o_Saccharimonadales were only enriched in the AAP group (Figure 13B). g_Subdoilgranulum were only enriched in the E. coli group (Figure 13B). The results for d 42 are shown in Figures 13C,D. At the phylum level, p_Fibrobacterota and p_Spirochaetota were only enriched in the control group (Figure 13C). p_Acidobacteriota and p_Halanaerobiaeota were only enriched in the AAP + E. coli group (Figure 13C). At the genus level, g_bacteroides and g_Candidatus_Arthromitus were only enriched in the AAP + E. coli group (Figure 13D). g_Blautia, g_Bradyrhizobium, g_Lachnoclostridium, g_UCG-005, g_NK4A214_group, g_Subdoligranulum, g_Veillonella, g_Eienbergiella, g_Ruminococcus_torques_group, g_unclassified_f_Ruminococcaceae, g_unclassified_f_Lachnospiraceae, g_unclassified_f_Peptostreptococcaceae, and g_norank_f_norank_o_Clostridia_UCG-014 were only enriched in the E. coli group (Figure 13D).
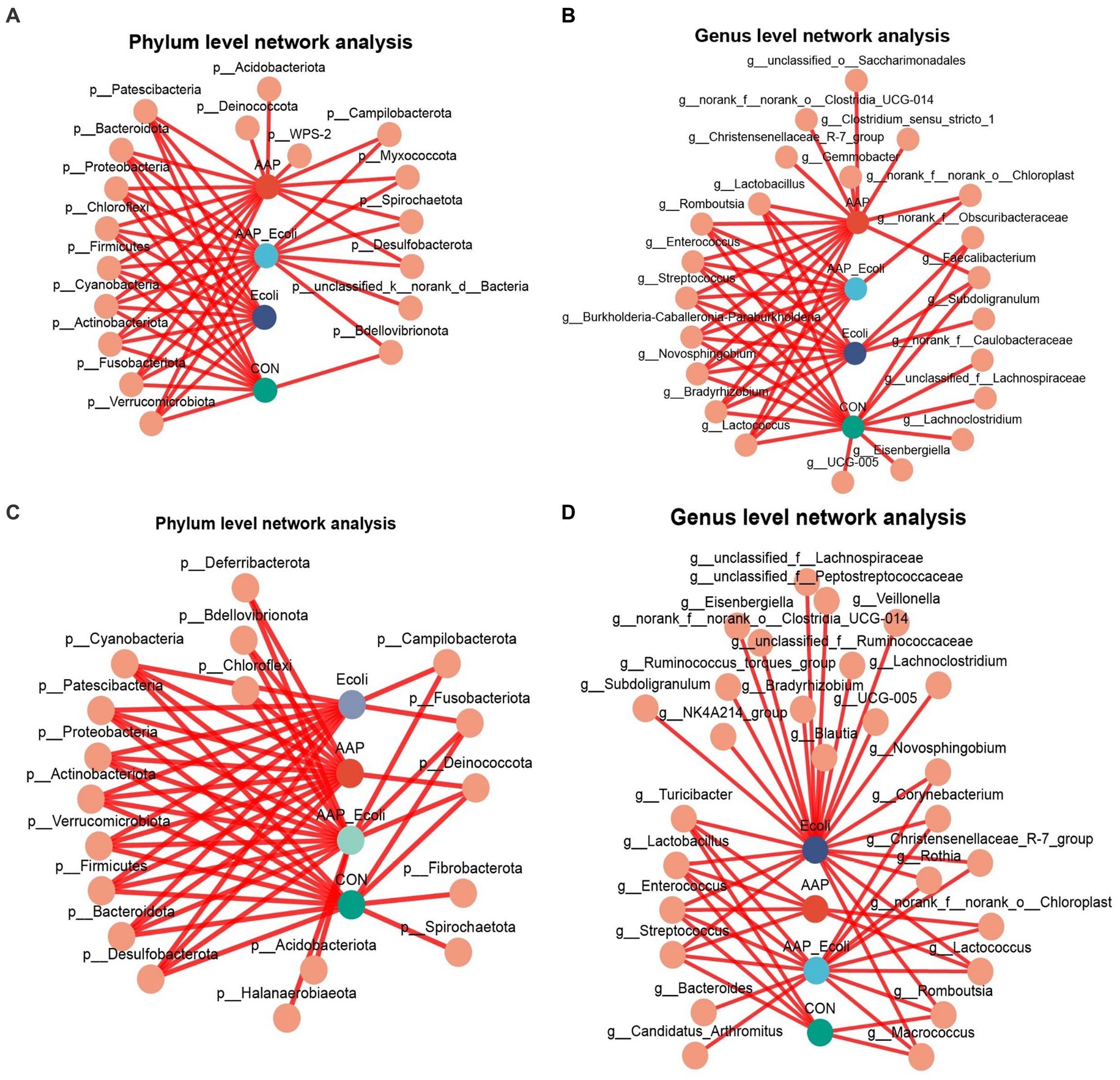
Figure 13. Co-occurrence network analysis of jejunal microbiota community at phylum and genus level. CON control group, AAP Artemisia annua L. polysaccharide group, E. coli Escherichia. coli group, AAP + E. coli Artemisia annua L. polysaccharide + Escherichia. coli group. d 21 phylum and genus level (A,B). d 42 phylum and genus level (C,D).
3.10 Association between intestinal microbiota and oxidative stress status, and inflammatory markers
The correlation between intestinal microbial proportions at the genus level and intestinal oxidative status, as well as pro/anti-inflammatory markers among four groups, is illustrated in Figure 14. The results for d 21 are shown in Figure 14A. The proportion of Lactobacillus had a positive correlation with Keap1 mRNA expression level (p < 0.05), and had a negative correlation with CAT mRNA expression level (p < 0.05). The proportion of norank_f_Obscuribacteraceae had a positive correlation with IL-1β content and MyD88 mRNA expression level (p < 0.01), and had a negative correlation with GPx activity (p < 0.05). The proportion of Lachnoclostridium had a positive correlation with IL-6 content (p < 0.01). The proportion of Eisenbergiella had a negative correlation with GSH content (p < 0.01). The proportion of Streptococcus had a positive correlation with CAT activity (p < 0.05), and had a negative correlation with IL-6 and Keap1 mRNA expression level (p < 0.05). The proportion of Romboutsia had a positive correlation with NF-κB p65 mRNA expression level (p < 0.05), and had a negative correlation with TNF-α content (p < 0.05). The proportion of Enterococcus had a positive correlation with CAT activity (p < 0.05). The proportion of Bradyrhizobium had a positive correlation with MyD88 mRNA expression level (p < 0.01). The proportion of norank_f_Caulobacteraceae had a positive correlation with IL-1β and IL-6 content, MyD88 mRNA expression level (p < 0.01), and had a negative correlation with Keap1 mRNA expression level (p < 0.05). The proportion of Novosphingobium and Lactococcus had a negative correlation with Keap1 mRNA expression level (p < 0.05). The proportion of Christensenellaceae_R-7_group had a negative correlation with GPx activity (p < 0.05). The proportion of unclassified_f_Lachnospiraceae had a positive correlation with IL-6 content (p < 0.05).
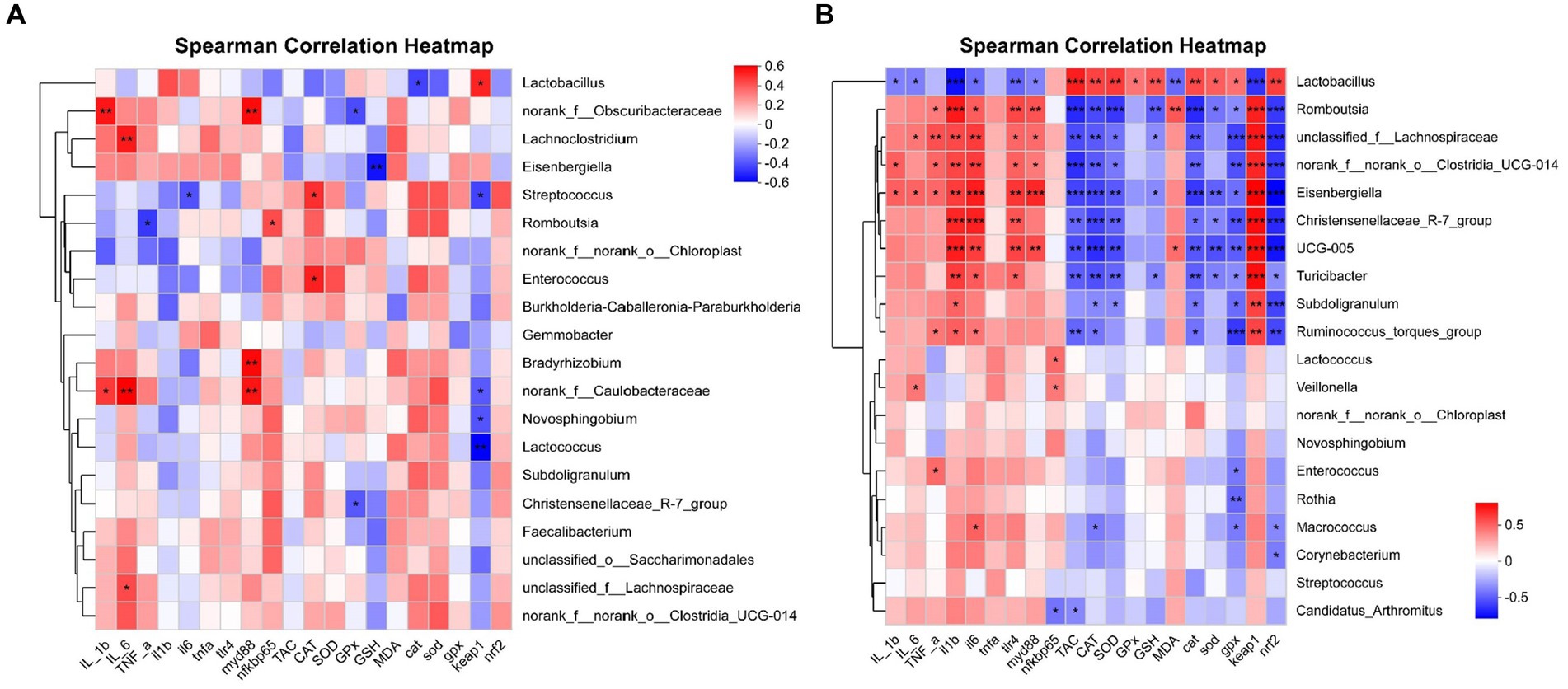
Figure 14. Correlation analysis of jejunal microbial proportions at the genus level with intestinal oxidative status and pro/anti-inflammatory markers among four groups, red represents a positive correlation, while blue represents a negative correlation. IL_1b interleukin-1 beita content, IL_6 interleukin-6 content, TNF-a tumor necrosis factor α content, il1b interleukin 1 beta gene expression, il6 interleukin 6 gene expression, tnfa tumor necrosis factor α gene expression, tlr4 toll like receptor 4 mRNA, myd88 myeloid differentiation primary response 88 gene expression, nfkbp65 nuclear factor kappa B p65 gene expression, TAC total antioxidant capacity, CAT catalase activity, SOD total superoxide dismutase activity, GPx glutathione peroxidase activity, GSH glutathione content, MDA malondialdehyde content, cat catalase gene expression, sod total superoxide dismutase gene expression, gpx glutathione peroxidase gene expression, nrf2 nuclear factor erythroid-2-related factor 2 gene expression, keap1 kelch like ECH associated protein 1 gene expression. *p < 0.05, **p < 0.01, and *** p < 0.001 indicate a significant correlation d 21 (A) and d 42 (B).
The results for d 42 are shown in Figure 14B. The proportion of Lactobacillus had a positive correlation with TAC, CAT, SOD, and GPx activity, GSH content, CAT, SOD, GPx, and Nrf2 mRNA expression level (p < 0.05), and had a negative correlation with IL-1β, IL-6 and MDA content, IL-1β, IL-6, TLR4, MyD88, and Keap1 mRNA expression level (p < 0.05). The proportion of Romboutsia had a positive correlation with TNF-α and MDA content, and IL-1β, IL-6, TLR4, MyD88, and Keap1 mRNA expression level (p < 0.05), and had a negative correlation with TAC, CAT, and SOD activity, GSH content, CAT, SOD, GPx, and Nrf2 mRNA expression level (p < 0.05). The proportion of unclassified_f_Lachnospiraceae had a positive correlation with IL-6 and TNF-α content, and IL-1β, IL-6, TLR4, MyD88, and Keap1 mRNA expression level (p < 0.05), and had a negative correlation with TAC, CAT, and SOD activity, GSH content, CAT, GPx, and Nrf2 mRNA expression level (p < 0.05). The proportion of norank_f_norank_o_Clostridia_UCG-014 had a positive correlation with IL-1β and TNF-α content, and IL-1β, IL-6, TLR4, MyD88, and Keap1 mRNA expression level (p < 0.05), and had a negative correlation with TAC, CAT, and SOD activity, CAT, GPx, and Nrf2 mRNA expression level (p < 0.05). The proportion of Eisenbergiella had a positive correlation with IL-1β, IL-6 and TNF-α content, and IL-1β, IL-6, TLR4, MyD88, and Keap1 mRNA expression level (p < 0.05), and had a negative correlation with TAC, CAT, and SOD activity, GSH content, CAT, SOD, GPx, and Nrf2 mRNA expression level (p < 0.05). The proportion of Christensenellaceae_R-7_group had a positive correlation with IL-1β, IL-6, TLR4, and Keap1 mRNA expression level (p < 0.05), and had a negative correlation with TAC, CAT, and SOD activity, CAT, SOD, GPx, and Nrf2 mRNA expression level (p < 0.05). The proportion of UCG-005 had a positive correlation with MDA content, and IL-1β, IL-6, TLR4, MyD88, and Keap1 mRNA expression level (p < 0.05), and had a negative correlation with TAC, CAT, and SOD activity, CAT, SOD, GPx, and Nrf2 mRNA expression level (p < 0.05). The proportion of Turicibacter had a positive correlation with IL-1β, IL-6, TLR4, and Keap1 mRNA expression level (p < 0.05), and had a negative correlation with TAC, CAT, and SOD activity, GSH content, CAT, SOD, GPx, and Nrf2 mRNA expression level (p < 0.05). The proportion of Subdoligranulum had a positive correlation with IL-1β and Keap1 mRNA expression level (p < 0.05), and had a negative correlation with CAT and SOD activity, CAT, GPx, and Nrf2 mRNA expression level (p < 0.05). The proportion of Ruminococcus_torques_group had a positive correlation with TNF-α content, IL-1β, IL-6, and Keap1 mRNA expression level (p < 0.05), and had a negative correlation with TAC and CAT activity, CAT, GPx, and Nrf2 mRNA expression level (p < 0.05). The proportion of Lactococcus had a positive correlation with NF-κB p65 mRNA expression level (p < 0.05). The proportion of Veillonella had a positive correlation with IL-6 content and NF-κB p65 mRNA expression level (p < 0.05). The proportion of Enterococcus had a positive correlation with TNF-α content, and had a negative correlation with GPx mRNA expression level (p < 0.05). The proportion of Rothia had a negative correlation with GPx mRNA expression level (p < 0.01). The proportion of Macrococcus had a positive correlation with IL-6 mRNA expression level (p < 0.05), and had a negative correlation with CAT activity, GPx and Nrf2 mRNA expression level (p < 0.05). The proportion of Corynebacterium had a negative correlation with Nrf2 mRNA expression level (p < 0.05). The proportion of Candidatus_Arthromitus had a negative correlation with TAC ability, NF-κB p65 mRNA expression level (p < 0.05).
4 Discussion
Previous studies found that Artemisia plant polysaccharides (such as Artemisia argyi and Artemisia ordosica) improved growth performance of broilers (Zhang et al., 2022b; Du et al., 2023). In the present study, dietary supplementation with AAP significantly increased BW on d 42, ADFI and ADG on d 36–42 in broilers. This is similar to our previous study, which found that dietary supplementation with Artemisia annua L. water extract increased the final body weight and feed efficiency of broilers (Guo et al., 2023). Furthermore, we found that oral administration of E. coli significantly reduced BW, ADG, and ADFI, and increased FCR of broilers in the present study. This is consistent with the study of Wu et al. (2021), who found that E. coli-challenge decreased ADG, ADFI, and BW in broilers. It is noteworthy that, in the present experiment, BW, ADG, ADFI and FCR of broilers in AAP + E. coli group were not different from those in control group, suggesting that dietary supplementation with AAP alleviated the decline in growth performance caused by E. coli-challenge. Moreover, Jahanian et al. (2021) observed that E. coli O78:K80-infected broilers significantly decreased ADFI and ADG during the trial period, however, dietary addition of silymarin significantly improved ADG in finisher and throughout the trial period. In the current study, dietary inclusion AAP increased growth performance of broilers, which might be due to its stimulant effect on appetite, improvement action on nutrient digestibility, and the consequent decrease in the gastrointestinal retention of food in birds. Simultaneously, we found that the apparent metabolic rate of EE (d 42), Ca (d 42) and P (d 21) in AAP + E. coli group was significantly higher than that in E. coli group. The nutrient metabolic rate is an important indicator for evaluating the digestion and absorption of nutrients in poultry. Its level is directly impacted by the growth performance of poultry and reflected the diet’s nutritional value. Previous experiment showed that Astragalus polysaccharides and Glycyrrhiza polysaccharides increased diets’ apparent metabolic rate in broilers (Qiao et al., 2022a). Previous studies have shown that Radix rehmanniae preparata polysaccharide supplementation can enhance mineral absorption in broilers (Yang et al., 2023), thus interact with Ca and P to promote the absorption of both. Therefore, we preliminarily speculated that dietary AAP could improve the growth performance of broilers by increasing the nutrient apparent metabolic rate.
Intestinal permeability is one of the indicators that indirectly reflect the damage of the intestinal mucosal barrier. Among them, the representative indicators include DAO, D-LA and ET (Guo et al., 2022b). In the present study, we observed that the activity of DAO and ET (d 42) in serum of broilers was significantly higher than that in the control group after oral administration of E. coli, indicating E. coli caused intestinal damage in broilers. As reported by Wu et al. (2021), E. coli-challenged broilers significantly increased the serum concentration of DAO and LPS compared with the unchallenged birds over the whole infection period (d 14 and 21). However, in our study, there was no difference in DAO activity between AAP + E. coli group and the control group, indicating that dietary supplementation AAP alleviated the damage caused by E. coli-challenge. In addition, intestinal permeability is closely related to intestinal villi morphology. When the intestine is damaged, the intestinal villi shrink or fall off, and the permeability of the intestine increases (Song et al., 2023; Zhang et al., 2023). In the present study, we found that E. coli-challenged broilers significantly reduced the VH and VH/CD of jejunum villi, but there was no difference between the AAP + E. coli group and the control group, indicating that dietary inclusion of AAP alleviated the damage of intestinal villi morphology caused by E. coli-challenge. This result is consistent with the finding reported by Liu et al. (2018), who found that dietary supplementation Achyranthes bidentata polysaccharide could significantly increase the VH and VH/CD of broilers caused by E. coli K88-challenge, indicating that polysaccharides could improve the intestine villus morphology of the birds. Tight junction protein is an important indicator of intestinal permeability. In the current study, we found that oral administration of E. coli significantly reduced mRNA expression level of Claudin-1 and Occludin in broilers, which was consistent with the mentioned increase in the level of DAO and D-LA, suggesting that E. coli-challenge leads to increase in intestinal permeability and damage of tight junction proteins, ultimately resulting in impairment of intestinal mucosal integrity. Similar to the findings reported by Wu et al. (2022b), who found that the ileum mRNA level of Occludin, Claudin and ZO-1 were significantly decreased in broilers by E. coli-challenge, however, dietary hydrolyzed wheat gluten supplementation ameliorated the decrease of tight junction protein mRNA expression caused by E. coli-challenge. Besides, it was reported that dietary supplementation of Astragalus membranaceus polysaccharides and Glycyrrhiza uralensis polysaccharides significantly increased the intestinal ZO-1, Claudin-1 and Occludin mRNA expression level of broilers (Qiao et al., 2022b). It is noteworthy that the AAP + E. coli group showed no difference in tight junction protein mRNA level compared with the control group in the current study. Similar outcomes were observed by Liu et al. (2023) that dietary addition of Enteromorpha prolifera polysaccharides upregulated the mRNA expression level of Occludin, ZO-1 in jejunum of broilers under heat stress. Thus, dietary supplementation AAP mitigated the decrease in tight junction protein mRNA expression level caused by E. coli-challenge. Mucin-2 is an important component of the intestinal mucous layer and plays an important role in resistance to pathogen invasion. It was previously reported that dietary supplementation of plant polysaccharides significantly increased the mRNA level of intestinal Mucin-2 in broilers (Qiao et al., 2022b). In the present study, we found that dietary supplementation with AAP effectively mitigated the decrease of Mucin-2 mRNA expression level in the intestine of broilers caused by E. coli-challenge. Moreover, it has been reported that prebiotics can alleviate the decrease of Mucin-2 mRNA expression level in broilers caused by E. coli-challenge (Huang et al., 2019).
The immune function of the body is essential for maintaining the health of the body. In this study, we observed that dietary inclusion AAP significantly increased the serum IgA content of broilers. Immunoglobulins are an important part of the body’s immune system and play an important role in resisting pathogen invasion. Both IgG and IgA are secreted by B lymphocytes as a part of humoral immunity. The present study showed that dietary supplementation AAP alleviated the decrease of serum IgM and jejunum IgG content in broilers caused by E. coli-challenge. In addition, Zheng et al. (2023) found that dietary Yupingfeng polysaccharides increased serum IgA, IgM and IgG level. It is well known that polysaccharides can enhance the secretion of B lymphocytes (Wu et al., 2022a), which is consistent with the results of the present investigation. Besides, it was reported that dietary addition of Achyranthes bidentata polysaccharides alleviated the decrease of sIgA content in intestinal mucosa caused by E. coli K88-challenge (Liu et al., 2018). Furthermore, in our study, we found that E. coli-challenged broilers significantly increased serum IL-6 and jejunum IL-1β and IL-6 content on d 42, however, dietary supplementation with AAP could effectively alleviate the increase of the proinflammatory cytokines caused E. coli-challenge. Furthermore, inflammatory factors like IL and TNF activate cell membrane receptors, initiating signal cascades that activate transcription factors, leading to increased gene transcription. They also affect mRNA-binding proteins, altering mRNA stability and degradation, influencing mRNA expression. Additionally, they modulate translation regulators, impacting mRNA translation efficiency and protein synthesis rate (Stumpo et al., 2010). In the current study, oral administration of E. coli significantly increased the mRNA level of IL-1β, IL-6 and TNF-α in jejunum, and dietary supplementation of AAP alleviated the up-regulation of mRNA level, and the trend was consistent with the content of inflammatory factors. Previous research found that dietary Astragalus polysaccharide significantly decreased the mRNA level of TNF-α and IL-1β in chickens challenged by E. coli (Su et al., 2019). Hence, dietary supplementation AAP alleviated the upregulation of inflammatory factors’ mRNA caused by E. coli-challenge. Presumably, the upregulation of pro-inflammatory factors may be regulated through signaling pathways such as TLR4 and NF-κB. In the current study, oral administration of E. coli significantly increased the mRNA level of TLR4 and MyD88 in jejunum, and dietary supplementation of AAP alleviated the upregulation of mRNA expression level. These results are a line with those found by Wu et al. (2022b), who reported that dietary hydrolyzed wheat gluten supplementation down-regulated the elevation of ileal TLR4 mRNA level caused by E. coli O78-challenge in broilers. It might be related to the anti-inflammatory activity of arabinose, galactose and fucoidan in plant-derived polysaccharides (Wang et al., 2023). In addition, in vitro experiment found the E. coli-induced intestinal barrier dysfunction was alleviated by seaweed polysaccharide supplementation via the inhibition of the NF-κB pathway (TLR4, MyD88, IκBα and p65 mRNA level) and inflammatory cytokines (IL-6 and TNF-α) production of IPEC-J2 cells (Guo et al., 2021). Another in vitro experiment showed that AAP significantly inhibited the production of IL-6 and TNF-α in murine RAW 264.7 macrophages stimulated by lipopolysaccharide (LPS) (Zhang et al., 2022a). Remarkably, our previous study found that Artemisia ordosica polysaccharides decreased LPS-induced over-production of IL-1β and IL-6 through suppressing TLR4/NF-κB pathway, and alleviated LPS-induced decreasing of TAC, CAT and GPx activity by activating Nrf2/Keap1 pathway, which ultimately improved jejunum morphology (Xing et al., 2023). LPS on the cell wall of E. coli is one of the main pathogenic components, and can be specifically recognized by TLR4 on the surface of the cell membrane, induce intestinal epithelial cells to release a large number of inflammatory factors, and stimulate the body to produce excessive ROS, thus generating oxidative stress.
Our study found that oral administration of E coli decreased the activity of serum GPx, jejunum TAC, CAT and SOD, and increased the content of MDA in jejunum, suggesting that E. coli-challenge destroyed the intestinal antioxidant system and caused oxidative stress to a certain extent. Also, dietary inclusion of AAP alleviated the decrease of antioxidant enzyme activity and the increase of MDA content caused by E. coli-challenge in the current study. These results resonate with those reported by Dong et al. (2019), who observed that dietary addition of Camellia oleifera seed extract alleviated the decrease of serum GPx and SOD activity and the increase of MDA content in broilers caused by E. coli K88-challenge. Moreover, dietary supplementation of AAP alleviated the decrease of mRNA expression level of CAT, SOD, GPx and Nrf2 and the increase of mRNA level of Keap1 in jejunum caused by E. coli-challenge in our study. This is consistent with the change of antioxidant enzyme activity. Intriguingly, our previous study found that A. annua aqueous extract promoted the intestinal immune and antioxidant function of broilers (Guo et al., 2022a). Based on the above-mentioned results, we preliminarily speculated that AAP might alleviate intestinal oxidative damage caused by E. coli-challenge through Nrf2 pathway. Moreover, it might also be associated to the in vitro antioxidant activity of AAP, which has been reported to have significant OH•, DPPH• and ABTS•+ free radical scavenging capacity (Zhang et al., 2022a). Therefore, dietary supplementation with AAP could alleviate intestinal oxidative damage caused by E. coli-challenge.
Intestinal microbiota is closely related to various physiological functions such as growth performance, metabolism and immunity in poultry (Díaz Carrasco et al., 2022). And we previously summarized that plant-derived polysaccharides could regulate intestinal health by improving intestinal microbial barrier (Guo et al., 2022b). So far, it has not been reported that A. annua polysaccharide regulates intestinal microflora. In the current study, 16S rRNA sequencing technology was used to analyze the jejunal microbiota of broilers to explore the effect of AAP on the taxonomic composition of the gut microbial community under E. coli challenge. We found that the jejunum microbial α diversity Simpson and Ace index in E. coli group had a trend of increasing and decreasing on d 21, respectively. The higher the Simpson index value, the lower the community diversity. The greater the Ace index, the richer the community species. Therefore, it indicated that E. coli-challenge decreased jejunum α diversity. However, on d 42 of the trial, different results were presented. Throughout the growth process of broiler chickens, significant changes are undergone by their intestinal structure, function, and digestive capacity with age. The two time points of d 21 and d 42 represented different growth stages, which are affected by the diversity of microorganisms. The increase in nutritional demand with age is affected by the nutritional sources and growth and reproduction of intestinal microorganisms. Difference between the earlier and later stages of intestinal development are led to significant difference in the α diversity of the jejunal microbial community. Mao et al. (2022) found that the α diversity of the ileal microbiota of broilers in d 21 and d 42 was different. In our study, the Sobs, Chao, Shannon and Ace index of broiler jejunum microbiota were increased significantly by E. coli-challenged, while Simpson and Coverage index were decreased on d 42. Contrary to our study, Pham et al. (2023) found that E. coli O78-challenge reduced the Chao and Ace index. Interestingly, there was no difference in the aforementioned α diversity index between the AAP + E. coli group and the control group. The results indicated that dietary AAP supplementation could improve α diversity of jejunum microbe in broilers challenged by E. coli.
The results of β diversity showed that the microbiota structure was significantly different among groups. The taxonomical composition analysis showed that Firmicutes were the most dominant phylum in jejunum of broilers, accounting for 89.82, 91.08, 95.13, and 92.92% on d 21, 98.24, 98.29, 96.17, and 95.06% on d 42 in groups control, AAP, E. coli, AAP + E. coli, respectively. However, the results demonstrated that no significant difference was observed among the four groups. In the current study, at the phylum level, we found a significant increase in the abundance of jejunum Desulfobacterota in the AAP group and the AAP + E. coli group on d 21. It is reported that Desulfobacterota is correlated with the level of inflammatory factors (Zhang et al., 2023). Different from previous period, at the phylum level, we found that the abundance of Bacteroidota in the jejunum contents of the AAP + E. coli group was significantly higher than that of other groups on d 42. Bacteroides is considered to be a beneficial bacterium, and its increased abundance might be due to a slowing down of intestinal absorption of AAP caused by E. coli-challenge, which promotes the growth of Bacteroides. The taxonomical composition analysis showed that Lactobacillus were the most dominant genus in the jejunum of broilers, accounting for 70.34, 70.38, 80.91, and 81.67% on d 21, 88.77, 88.13, 55.00, and 61.27% on d 42 in groups control, AAP, E. coli, AAP + E. coli, respectively. E. coli-challenge might stimulate the growth of Lactobacillus, but the difference is not significant on d 21. Interestingly, the results were different on d 42. The two time points of d 21 and d 42 represented different growth stages, which are likely accompanied by adjustments in the structure and function of the digestive system, thus influencing the microbial flora. In our study, the abundance of Lactobacillus decreased significantly under the condition of E. coli-challenge on d 42. Previously, Zeng et al. (2023) reported that E. coli K88-challenge reduced the number of Lactobacillus in jejunum, ileum and colon of piglets. Previous studies showed that Yupingfeng polysaccharides increased the abundance of Lactobacillus genus in duodenum of broilers (Zheng et al., 2023). Coincidentally, LEfSe analysis showed that addition of AAP increased the number of dominant bacteria Lactobacillales in the jejunum at the order level in our study. Furthermore, it was found that the proportion of Lactobacillus was negatively correlated with the level of inflammatory factors, but positively correlated with the level of antioxidants, indicating that the proliferation of Lactobacillus could be promoted by AAP and the inflammatory response could be reduced. Besides, at the genus level, we found that the abundance of Megasphaera and Megamonas in jejunum contents of AAP group and AAP + E. coli group was significantly reduced. Megasphaera and Megamonas can ferment polysaccharides to produce SCFAs, such as acetic acid, propionic acid and butyric acid, further inhibiting the growth of pathogenic microorganisms (Liu et al., 2023). In the current study, the abundance of Megasphaera and Megamonas was decreased, which might be partially consumed during the fermentation of polysaccharides. It has previously been reported that dietary supplementation with Antrodia cinnamomea polysaccharide mitigated the decrease in the abundance of Megamonas and Ruminococcus_torques_group in cecal microbiota of broilers challenged by LPS (Ye et al., 2022), similar to the findings in our experiment. Parabacteroides regulate intestinal immune response and are positively correlated with IgG (Qiao et al., 2022b). In the present study, the abundance of Parabacteroides in jejunum of broilers was significantly reduced under the condition of E. coli challenge, indicating that E. coli challenge disrupted the microbiological balance. Ralstonia is a gram-negative bacterium (Deng et al., 2021), and dietary supplementation with AAP significantly reduced its abundance in our study. Pham et al. (2023) also found that E. coli O78-challenge increased the abundance of cecum Oscillospira in broilers, which is similar to the results of this experiment. However, Qiao et al. (2022a) found that Oscillospira was positively correlated with GPx mRNA expression level, and dietary supplementation of polysaccharides derived from astragalus and glycyrrhiza increased Oscillospira abundance in conventional feeding. We found that Faecalibacterium and Negativibacillus are mainly enriched in E. coli group (Figure 12). Previous experiment found that dietary supplementation of polysaccharides derived from astragalus and glycyrrhiza decreased the relative abundance of Faecalibacterium, and was positively correlated with MDA, but negatively correlated with T-AOC, GPx, SOD1 mRNA expression, indicating that the polysaccharides could improve antioxidant function by modulating gut microbiota in broilers (Qiao et al., 2022a). Previous studies showed that dietary supplementation of astragalus polysaccharide decreased the abundance of Negativibacillus in cecum of broilers, thereby improving intestinal health (Wang et al., 2022). Similarly, dietary AAP reduced the abundance of Faecalibacterium and Negativibacillus in jejunum of broilers challenged by E. coli in our study. UCG-005 is closely related to inflammation (Song et al., 2023). In our experiment, we observed a positive correlation between the mRNA expression levels of IL-1β, IL-6, TLR4, and MyD88 and the proportion of UCG-005. Furthermore, UCG-005 was found to be enriched in the E. coli group, as depicted in Figures 12, 13D. Dietary AAP reduced the abundance of UCG-005 under E. coli-challenged, suggesting that AAP might reduce the expression of intestinal pro-inflammatory factors by inhibiting the proliferation of UCG-005. Blautia is related to the immune response of the gut, and is also involved in regulating the body’s physiological responses (Li et al., 2022). Compared with the E. coli group, the abundance of Blautia in the AAP + E. coli group was down-regulated, indicating that AAP plays a role in the regulation of intestinal homeostasis. Song et al. (2022) found that dietary supplementation of astragalus polysaccharide alleviated necrotic enteritis-induced intestinal inflammatory damage by increasing the abundance of Romboutsia in the ileum. In our experiment, we observed a positive correlation between the levels of IL-1β, IL-6, TLR4, and MyD88 and the proportion of Romboutsia. Additionally, it was noted that Romboutsia exhibited no enrichment in the AAP group (Figure 13D), suggesting a potential inhibitory effect of AAP on its proliferation.
In this study, we found that the abundance of various beneficial bacteria in the E. coli group was up-regulated, such as Blautia, Megamonas and Christensenellaceae R-7 group, which may inhibit the reproduction of E. coli by participating in some different metabolic pathways, such as TCA cycle, butyrate metabolism, propionic acid metabolism (Li et al., 2022). Furthermore, the expansion of numerous beneficial bacteria indicated that E. coli resulted in a microbial imbalance in the gut, and prompting the production of a large number of beneficial bacteria in the intestine to regain the balance of microbiota and inhibit the growth of pathogenic bacteria (Li et al., 2021). However, the abundance of these beneficial bacteria in the AAP + E. coli group was reduced, which might be attributed to the pre-protective effect of AAP to restore intestinal microbiota balance as soon as possible. Moreover, it might also regulate the balance of microecology through other ways. Our previous studies have shown that Artemisia ordosica polysaccharide can prevent intestinal inflammation in broilers by inhibiting TLR4/NF-κB and activating Nrf2/Keap1 pathway (Xing et al., 2023). Furthermore, plant polysaccharides can also promote intestinal peristalsis and defecation, help to expel harmful substances and metabolites in poultry, further reduce the intestinal burden, and is conducive to the growth and reproduction of beneficial bacteria (Sundar et al., 2020). In addition, it was reported that whether polysaccharides can play an antibacterial role is related to the molecular weight of polysaccharides, and large molecular weight polysaccharides can inhibit harmful bacteria such as E. coli (Wang et al., 2023). It might explain the relatively low abundance of bacteria detected in the AAP + E. coli group. We found that the microbiota changes of d 21 and d 42 were different (Figure 13), which might be related to the age and feed intake of broilers at different periods (Konieczka et al., 2017). In general, E. coli-challenge destroyed the balance of jejunum microbiota in broilers, and dietary addition of AAP could restore the balance of microbe.
5 Conclusion
In summary, based on growth performance, apparent nutrient metabolic rate, intestinal permeability, immune response, intestinal morphology, antioxidant function, jejunal microbiota, we conclude that E. coli-challenge impaired intestinal health, and eventually decreased the growth performance of broilers. Dietary AAP supplementation can effectively reduce intestinal damage, improve the intestinal barrier function, enhance immune and antioxidant function, re-shape intestinal microbiota. These results suggest that AAP may be an effective option for the prevention of E. coli in poultry breeding.
Data availability statement
The datasets presented in this study can be found in the NCBI repository, accession number PRJNA1098622.
Ethics statement
The animal study was approved by Inner Mongolia Agricultural University Animal Care and Use Committee, Hohhot, P. R. China (approval number: NND2021090). The study was conducted in accordance with the local legislation and institutional requirements.
Author contributions
SG: Conceptualization, Formal analysis, Methodology, Visualization, Writing – original draft. BS: Supervision, Validation, Writing – review & editing. YyX: Writing – review & editing. YqX: Resources, Validation, Writing – review & editing. XJ: Data curation, Validation, Writing – original draft. LH: Supervision, Writing – original draft. SZ: Investigation, Writing – review & editing. MQ: Writing – review & editing. SY: Validation, Writing – original draft.
Funding
The author(s) declare that financial support was received for the research, authorship, and/or publication of this article. This study was supported by the Inner Mongolia Autonomous Region Natural Science Foundation Project (2022MS03047).
Acknowledgments
We would like to thank the staff at our laboratory for their ongoing assistance.
Conflict of interest
The authors declare that the research was conducted in the absence of any commercial or financial relationships that could be construed as a potential conflict of interest.
Publisher’s note
All claims expressed in this article are solely those of the authors and do not necessarily represent those of their affiliated organizations, or those of the publisher, the editors and the reviewers. Any product that may be evaluated in this article, or claim that may be made by its manufacturer, is not guaranteed or endorsed by the publisher.
References
Berkes, J., Viswanathan, V. K., Savkovic, S. D., and Hecht, G. (2003). Intestinal epithelial responses to enteric pathogens: effects on the tight junction barrier, ion transport, and inflammation. Gut 52, 439–451. doi: 10.1136/gut.52.3.439
Chebbac, K., Benziane Ouaritini, Z., El Moussaoui, A., Chalkha, M., Lafraxo, S., Bin Jardan, Y. A., et al. (2023). Antimicrobial and antioxidant properties of chemically analyzed essential oil of Artemisia annua L. (Asteraceae) native to Mediterranean area. Life 13:807. doi: 10.3390/life13030807
Chinese Ministry of Agriculture . Feeding standard of chicken, China (NY/T 33-2004). (2004). Hunan Feed. G Liu, B Xiong, J Su, C Ji, Q Diao, and H Liu, et al. (Eds.) Beijing: Ministry of Agriculture of the People’s Republic of China 4, 19–27.
Choi, E. Y., Choi, J. O., Park, C. Y., Kim, S. H., and Kim, D. (2020). Water extract of Artemisia annua L. exhibits Hepatoprotective effects through improvement of lipid accumulation and oxidative stress-induced cytotoxicity. J. Med. Food 23, 1312–1322. doi: 10.1089/jmf.2020.4696
Croxen, M. A., Law, R. J., Scholz, R., Keeney, K. M., Wlodarska, M., and Finlay, B. B. (2013). Recent advances in understanding enteric pathogenic Escherichia coli. Clin. Microbiol. Rev. 26, 822–880. doi: 10.1128/CMR.00022-13
Cui, Y., Sun, W., Li, Q., Wang, K., Wang, Y., Lv, F., et al. (2022). Effects of caulis Spatholobi polysaccharide on immunity, intestinal mucosal barrier function, and intestinal microbiota in cyclophosphamide-induced immunosuppressive chickens. Front. Vet. Sci. 9:833842. doi: 10.3389/fvets.2022.833842
Das, S., Vörös-Horváth, B., Bencsik, T., Micalizzi, G., Mondello, L., Horváth, G., et al. (2020). Antimicrobial activity of different Artemisia essential oil formulations. Molecules 25:2390. doi: 10.3390/MOLECULES25102390
Deng, S., Xu, Q., Fu, Y., Liang, L., Wu, Y., Peng, F., et al. (2021). Genomic analysis of a novel phage infecting the Turkey pathogen Escherichia coli APEC O78 and its Endolysin activity. Viruses 13:1034. doi: 10.3390/v13061034
Díaz Carrasco, J. M., Redondo, L. M., Casanova, N. A., and Fernández Miyakawa, M. E., (2022). The role of farm environment and Management in Shaping the gut microbiota of poultry. Gut microbiota, immunity, and health in production animals. Cham: Springer International Publishing. 193–224
Ding, F., Ma, T., Hao, M., Wang, Q., Chen, S., Wang, D., et al. (2020). Mapping worldwide environmental suitability for Artemisia annua L. Sustain. For. 12:1309. doi: 10.3390/su12041309
Dong, Z. L., Wang, Y. W., Song, D., Wang, W. W., Liu, K. B., Wang, L., et al. (2019). Effects of microencapsulated probiotics and plant extract on antioxidant ability, immune status and caecal microflora in Escherichia coli K88-challenged broiler chickens. Food Agr. Immunol. 30, 1123–1134. doi: 10.1080/09540105.2019.1664419
Du, H., Xing, Y., Jin, X., Yan, S., and Shi, B. (2023). Effects of Artemisia ordosica polysaccharide on growth performance and antioxidant capacity in broilers. J. Appl. Anim. Res. 51, 92–101. doi: 10.1080/09712119.2022.2158093
Fu, C., Yu, P., Wang, M., and Qiu, F. (2020). Phytochemical analysis and geographic assessment of flavonoids, coumarins and sesquiterpenes in Artemisia annua L. based on HPLC-DAD quantification and LC-ESI-QTOF-MS/MS confirmation. Food Chem. 312:126070. doi: 10.1016/j.foodchem.2019.126070
Gomes, T. A., Elias, W. P., Scaletsky, I. C., Guth, B. E., Rodrigues, J. F., Piazza, R. M., et al. (2016). Diarrheagenic Escherichia coli. Braz. J. Microbiol. 47, 3–30. doi: 10.1016/j.bjm.2016.10.015
Graham, I. A., Czechowski, T., Rinaldi, M. A., Famodimu, M. T., Van Veelen, M., Larson, T. R., et al. (2019). Flavonoid versus artemisinin anti-malarial activity in Artemisia annua whole-leaf extracts. Front. Plant Sci. 10:984. doi: 10.3389/fpls.2019.00984
Guo, X., Chen, J., Yang, J., He, Q., Luo, B., Lu, Y., et al. (2021). Seaweed polysaccharide mitigates intestinal barrier dysfunction induced by enterotoxigenic Escherichia coli through NF-κB pathway suppression in porcine intestinal epithelial cells. J. Anim. Physiol. Anim. Nutr. 105, 1063–1074. doi: 10.1111/jpn.13540
Guo, S., Ma, J., Xing, Y., Shi, L., Zhang, L., Xu, Y., et al. (2022a). Artemisia annua L. aqueous extract promotes intestine immunity and antioxidant function in broilers. Front. Vet. Sci. 9:934021. doi: 10.3389/fvets.2022.934021
Guo, S., Ma, J., Xing, Y., Xu, Y., Jin, X., Yan, S., et al. (2023). Effects of Artemisia annua L. water extract on growth performance and intestinal related indicators in broilers. J. Poult. Sci. 60:2023024. doi: 10.2141/jpsa.2023024
Guo, S., Xing, Y., Xu, Y., Jin, X., Yan, S., and Shi, B. (2022b). Progress of studies on plant-derived polysaccharides affecting intestinal barrier function in poultry. Animals 12:3205. doi: 10.3390/ani12223205
Han, X., Chai, Y., Lv, C., Chen, Q., Liu, J., Wang, Y., et al. (2022). Sesquiterpenes from Artemisia annua and their cytotoxic activities. Molecules 27:5079. doi: 10.3390/molecules27165079
Huang, L., Luo, L., Zhang, Y., Wang, Z., and Xia, Z. (2019). Effects of the dietary probiotic, Enterococcus faecium NCIMB11181, on the intestinal barrier and system immune status in Escherichia coli O78-challenged broiler chickens. Probiotics Antimicrob. Proteins 11, 946–956. doi: 10.1007/s12602-018-9434-7
Jahanian, E., Mahdavi, A. H., and Jahanian, R. (2021). Silymarin improved the growth performance via modulating the microbiota and mucosal immunity in Escherichia coli-challenged broiler chicks. Livest. Sci. 249:104529. doi: 10.1016/j.livsci.2021.104529
Konieczka, P., Barszcz, M., Choct, M., and Smulikowska, S. (2017). The interactive effect of dietary n-6: n-3 fatty acid ratio and vitamin E level on tissue lipid peroxidation, DNA damage in intestinal epithelial cells, and gut morphology in chickens of different ages. Poult. Sci. 97, 149–158. doi: 10.3382/ps/pex274
Kumari, M., Gupta, R. P., Bagri, P., and Singh, R. (2023). Immunopathological studies on Escherichia coli infected broiler chickens fed on Aloe vera leaf extract. Vet. Immunol. Immunopathol. 258:110562. doi: 10.1016/j.vetimm.2023.110562
Li, S., Lin, R., Chen, J., Hussain, R., Zhang, S., Su, Y., et al. (2022). Integrated gut microbiota and metabolomic analysis reveals immunomodulatory effects of Echinacea extract and Astragalus polysaccharides. Front. Vet. Sci. 9:971058. doi: 10.3389/fvets.2022.971058
Li, Y., Xia, S., Jiang, X., Feng, C., Gong, S., Ma, J., et al. (2021). Gut microbiota and diarrhea: An updated review. Front. Cell. Infect. Microbiol. 11:625210. doi: 10.3389/fcimb.2021.625210
Liu, W., Liu, H., Wang, Y., Zhao, Z., Balasubramanian, B., and Jha, R. (2023). Effects of Enteromorpha prolifera polysaccharides on growth performance, intestinal barrier function and cecal microbiota in yellow-feathered broilers under heat stress. J. Anim. Sci. Biotechnol. 14:132. doi: 10.1186/s40104-023-00932-2
Liu, Z., Wang, X., Ou, S., Arowolo, M. A., Hou, D. X., and He, J. (2018). Effects of Achyranthes bidentata polysaccharides on intestinal morphology, immune response, and gut microbiome in yellow broiler chickens challenged with Escherichia coli K88. Polymers 10:1233. doi: 10.3390/polym10111233
Mao, J., Wang, Y., Wang, W., Duan, T., Yin, N., Guo, T., et al. (2022). Effects of Taraxacum mongolicum hand.-Mazz. (dandelion) on growth performance, expression of genes coding for tight junction protein and mucin, microbiota composition and short chain fatty acids in ileum of broiler chickens. BMC Vet. Res. 18:180. doi: 10.1186/s12917-022-03278-5
Pham, V. H., Abbas, W., Huang, J., Guo, F., Zhang, K., Kong, L., et al. (2023). Dietary coated essential oil and organic acid mixture supplementation improves health of broilers infected with avian pathogenic Escherichia coli. Anim. Nutr. 12, 245–262. doi: 10.1016/j.aninu.2022.09.010
Qiao, Y., Guo, Y., Zhang, W., Guo, W., Oleksandr, K., Bozhko, N., et al. (2022a). Effects of compound polysaccharides derived from Astragalus and Glycyrrhiza on growth performance, meat quality and antioxidant function of broilers based on serum metabolomics and Cecal microbiota. Antioxidants 11:1872. doi: 10.3390/antiox11101872
Qiao, Y., Liu, C., Guo, Y., Zhang, W., Guo, W., Oleksandr, K., et al. (2022b). Polysaccharides derived from Astragalus membranaceus and Glycyrrhiza uralensis improve growth performance of broilers by enhancing intestinal health and modulating gut microbiota. Poult. Sci. 101:101905. doi: 10.1016/j.psj.2022.101905
Soares, M. P., Cardoso, I. L., Araújo, F. E., De Angelis, C. F., Mendes, R., Mendes, L. W., et al. (2022). Influences of the alcoholic extract of Artemisia annua on gastrointestinal microbiota and performance of Nile tilapia. Aquaculture 560:738521. doi: 10.1016/j.aquaculture.2022.738521
Song, Z., Cheng, K., Zhang, L., and Wang, T. (2017). Dietary supplementation of enzymatically treated Artemisia annua could alleviate the intestinal inflammatory response in heat-stressed broilers. J. Therm. Biol. 69, 184–190. doi: 10.1016/j.jtherbio.2017.07.015
Song, Z. H., Cheng, K., Zheng, X. C., Ahmad, H., Zhang, L. L., and Wang, T. (2018). Effects of dietary supplementation with enzymatically treated Artemisia annua on growth performance, intestinal morphology, digestive enzyme activities, immunity, and antioxidant capacity of heat-stressed broilers. Poult. Sci. 97, 430–437. doi: 10.3382/ps/pex312
Song, B., He, J., Pan, X., Kong, L., Xiao, C., Keerqin, C., et al. (2023). Dietary Macleaya cordata extract supplementation improves the growth performance and gut health of broiler chickens with necrotic enteritis. J. Anim. Sci. Biotechnol. 14:113. doi: 10.1186/s40104-023-00916-2
Song, B., Li, P., Yan, S., Liu, Y., Gao, M., Lv, H., et al. (2022). Effects of dietary Astragalus polysaccharide supplementation on the Th17/Treg balance and the gut microbiota of broiler chickens challenged with necrotic enteritis. Front. Immunol. 13:781934. doi: 10.3389/fimmu.2022.781934
Stan, R. L. (2020). Artemisia Annua L. extract: a new Phytoproduct with sod-like and antitumour activity. Farmacia 68, 812–821. doi: 10.31925/farmacia.2020.5.6
Stumpo, D. J., Lai, W. S., and Blackshear, P. J. (2010). Inflammation: cytokines and RNA-based regulation. Wiley Interdiscip. Rev. RNA. 1, 60–80. doi: 10.1002/wrna.1
Su, L., Wang, J., Huang, J., Zhao, Y., Jiang, H., and Li, H. (2019). Suppresses of Astragalus polysaccharide on E. coli-induced injured intestinal microvascular through TLR4-NF-κB signal pathways in chickens. Braz. J. Poultry Sci. 21, 1–8. doi: 10.1590/1806-9061-2018-0945
Sundar, V., Senthil Kumar, K. A., Manickam, V., and Ramasamy, T. (2020). Current trends in pharmacological approaches for treatment and management of acute pancreatitis—a review. J. Pharm. Pharmacol. 72, 761–775. doi: 10.1111/jphp.13229
Wan, X., Ahmad, H., Zhang, L., Wang, Z., and Wang, T. (2018). Dietary enzymatically treated Artemisia annua L. improves meat quality, antioxidant capacity and energy status of breast muscle in heat-stressed broilers. J. Sci. Food Agric. 98, 3715–3721. doi: 10.1002/jsfa.8879
Wan, X., Zhang, J., He, J., Bai, K., Zhang, L., and Wang, T. (2017). Dietary enzymatically treated Artemisia annua L. supplementation alleviates liver oxidative injury of broilers reared under high ambient temperature. Int. J. Biometeorol. 61, 1629–1636. doi: 10.1007/s00484-017-1341-1
Wang, W., Li, Z., Han, Q., Guo, Y., Zhang, B., and D’Inca, R. (2016). Dietary live yeast and mannan-oligosaccharide supplementation attenuate intestinal inflammation and barrier dysfunction induced by Escherichia coli in broilers. Br. J. Nutr. 116, 1878–1888. doi: 10.1017/S0007114516004116
Wang, Q., Wang, X. F., Xing, T., Li, J. L., Zhu, X. D., Zhang, L., et al. (2022). The combined impact of xylo-oligosaccharides and gamma-irradiated astragalus polysaccharides on the immune response, antioxidant capacity, and intestinal microbiota composition of broilers. Poult. Sci. 101:101996. doi: 10.1016/j.psj.2022.101996
Wang, Z., Zheng, Y., Lai, Z., Hu, X., Wang, L., Wang, X., et al. (2023). Effect of monosaccharide composition and proportion on the bioactivity of polysaccharides: a review. Int. J. Biol. Macromol. 254:127955. doi: 10.1016/j.ijbiomac.2023.127955
Wu, Q. G., Huang, L. Y., Fan, M. H., Chou, G. X., and Wang, Y. L. (2023). Anti-inflammatory activities of monoterpene and Sesquiterpene glycosides from the aqueous extract of Artemisia annua L. Chem. Biodivers. 20:e202201237. doi: 10.1002/cbdv.202201237
Wu, Y., Li, N., Zhang, T., Che, Y., Duan, K., Wang, Y., et al. (2022a). Glycyrrhiza polysaccharides can improve and prolong the response of chickens to the Newcastle disease vaccine. Poult. Sci. 101:101549. doi: 10.1016/j.psj.2021.101549
Wu, Y., Wang, W., Kim, I. H., and Yang, Y. (2022b). Dietary hydrolyzed wheat gluten supplementation ameliorated intestinal barrier dysfunctions of broilers challenged with Escherichia coli O78. Poult. Sci. 101:101615. doi: 10.1016/j.psj.2021.101615
Wu, Y., Wu, C., Che, Y., Zhang, T., Dai, C., Nguyen, A. D., et al. (2022c). Effects of Glycyrrhiza polysaccharides on Chickens' intestinal health and homeostasis. Front. Vet. Sci. 9:891429. doi: 10.3389/fvets.2022.891429
Wu, Z., Yang, K., Zhang, A., Chang, W., Zheng, A., Chen, Z., et al. (2021). Effects of Lactobacillus acidophilus on the growth performance, immune response, and intestinal barrier function of broiler chickens challenged with Escherichia coli O157. Poult. Sci. 100:101323. doi: 10.1016/j.psj.2021.101323
Xing, Y. Y., Xu, Y. Q., Jin, X., Shi, L. L., Guo, S. W., Yan, S. M., et al. (2020). Optimization extraction and characterization of Artemisia ordosica polysaccharide and its beneficial effects on antioxidant function and gut microbiota in rats. RSC Adv. 10, 26151–26164. doi: 10.1039/d0ra05063f
Xing, Y., Zheng, Y., Yang, S., Zhang, L., Guo, S., Shi, L., et al. (2023). Artemisia ordosica polysaccharide ameliorated LPS-induced growth inhibition and intestinal injury in broilers through enhancing immune-regulation and antioxidant capacity. J. Nutr. Biochem. 115:109284. doi: 10.1016/j.jnutbio.2023.109284
Yan, L., Xiong, C., Xu, P., Zhu, J., Yang, Z., Ren, H., et al. (2019). Structural characterization and in vitro antitumor activity of a polysaccharide from Artemisia annua L. (Huang Huahao). Carbohydr. Polym. 213, 361–369. doi: 10.1016/j.carbpol.2019.02.081
Yang, B., Li, X., Baran, A. M., and Abdel-Moneim, A. E. (2023). Effects of dietary incorporation of Radix rehmanniae praeparata polysaccharide on growth performance, digestive physiology, blood metabolites, meat quality, and tibia characteristics in broiler chickens. Poult. Sci. 102:103150. doi: 10.1016/j.psj.2023.103150
Ye, J., Zhang, C., Fan, Q., Lin, X., Wang, Y., Azzam, M., et al. (2022). Antrodia cinnamomea polysaccharide improves liver antioxidant, anti-inflammatory capacity, and cecal flora structure of slow-growing broiler breeds challenged with lipopolysaccharide. Front. Vet. Sci. 9:994782. doi: 10.3389/fvets.2022.994782
Yegani, M., and Korver, D. R. (2008). Factors affecting intestinal health in poultry. Poult. Sci. 87, 2052–2063. doi: 10.3382/ps.2008-00091
Zeng, Y., Li, R., Dong, Y., Yi, D., Wu, T., Wang, L., et al. (2023). Dietary supplementation with Puerarin improves intestinal function in piglets challenged with Escherichia coli K88. Animals 13:1908. doi: 10.3390/ani13121908
Zhang, S. , (2023). Effects of Artemisia annua L. Polysaccharide on Immune and Antioxidant Functions of Broilers. Master’s Thesis. (In Chinese)
Zhang, L., Reddy, N., Khoo, C. S., and Koyyalamudi, S. R. (2022a). Structural characterization and in-vitro antioxidant and immunomodulatory activities of polysaccharide fractions isolated from Artemisia annua L. Molecules 27:3643. doi: 10.3390/molecules27113643
Zhang, J., Shu, D., Cheng, X., Tian, T., Xiao, K., Zhang, D., et al. (2023). Effect of plant polysaccharides (Poria cocos and Astragalus polysaccharides) on immune responses and intestinal microbiota of Dabry's sturgeons. Biosci. Microb. Food H. 42, 243–253. doi: 10.12938/bmfh.2022-089
Zhang, L., Xing, Y., Shi, L., Guo, S., Jin, X., Xu, Y., et al. (2022b). The effects of dietary supplementation of Artemisia argyi polysaccharide on immune and antioxidative functions in broilers. J. Appl. Anim. Res. 50, 587–597. doi: 10.1080/09712119.2022.2119982
Zhang, J., Yu, H., Zhang, H., Zhao, Q., Si, W., Qin, Y., et al. (2023). Dietary Epimedium extract supplementation improves intestinal functions and alters gut microbiota in broilers. J. Anim. Sci. Biotechnol. 14:14. doi: 10.1186/s40104-022-00812-1
Zhang, S., Zhu, C., Xie, H., Wang, L., and Hu, J. (2022c). Effect of Gan Cao (Glycyrrhiza uralensis Fisch) polysaccharide on growth performance, immune function, and gut microflora of broiler chickens. Poult. Sci. 101:102068. doi: 10.1016/j.psj.2022.102068
Zheng, W., Guan, Y., and Wu, B. (2023). Effects of Yupingfeng polysaccharides as feed supplement on immune function and intestinal microbiome in chickens. Microorganisms 11:2774. doi: 10.3390/microorganisms11112774
Glossary
Keywords: Artemisia annua L. polysaccharide, broiler, Escherichia coli, intestinal barrier function, microbiota
Citation: Guo S, Shi B, Xing Y, Xu Y, Jin X, Hong L, Zhang S, Qiao M and Yan S (2024) Artemisia annua L. polysaccharide improves the growth performance and intestinal barrier function of broilers challenged with Escherichia coli. Front. Microbiol. 15:1390815. doi: 10.3389/fmicb.2024.1390815
Edited by:
Yu Pi, Chinese Academy of Agricultural Sciences, ChinaReviewed by:
Kaimin Niu, Jiangxi Academy of Sciences, ChinaTolulope Adebowale, Chinese Academy of Sciences (CAS), China
Victoria Anthony Uyanga, Iowa State University, United States
Copyright © 2024 Guo, Shi, Xing, Xu, Jin, Hong, Zhang, Qiao and Yan. This is an open-access article distributed under the terms of the Creative Commons Attribution License (CC BY). The use, distribution or reproduction in other forums is permitted, provided the original author(s) and the copyright owner(s) are credited and that the original publication in this journal is cited, in accordance with accepted academic practice. No use, distribution or reproduction is permitted which does not comply with these terms.
*Correspondence: Binlin Shi, c2hpYmlubGluQHllYWgubmV0; c2hpYmxAaW1hdS5lZHUuY24=