- 1College of Animal Science and Technology/Laboratory of Functional Microbiology and Animal Health, Henan University of Science and Technology, Luoyang, China
- 2Luoyang Key Laboratory of Live Carrier Biomaterial and Animal Disease Prevention and Control, Luoyang, China
- 3The Key Lab of Animal Disease and Public Health, Henan University of Science and Technology, Luoyang, China
- 4Animal Diseases and Public Health Engineering Research Center of Henan Province, Luoyang Polytechnic, Luoyang, China
Increases in the virulence and survival of some pathogens in the presence of subinhibitory concentrations of antibiotics have been reported. However, research on the effects of subinhibitory concentrations of antimicrobial substances derived from traditional Chinese medicine on pathogens is still insufficient. Glabridin is a well-known active isoflavone found in licorice roots that possesses a wide range of biological activities. Therefore, in this study, Listeria monocytogenes (L. monocytogenes) exposed to subinhibitory concentrations of glabridin was used as the research object. The minimum inhibitory concentration (MIC) was determined for L. monocytogenes. We investigated the impacts of subinhibitory concentrations of glabridin on the morphology, motility, biofilm formation, adherence, and survival of L. monocytogenes. The results indicated that the MIC of glabridin for L. monocytogenes was 31.25 μg/mL. At 1/8, 1/4, or 1/2 of the MIC, glabridin did not affect the growth, morphology, flagellar production, or biofilm formation of L. monocytogenes. However, subinhibitory concentrations of glabridin inhibited bacterial swimming and swarming motility and decreased the hemolytic activity of L. monocytogenes. Glabridin reduced the hemolytic activity of L. monocytogenes culture supernatants. The results also showed that subinhibitory concentrations of glabridin had no toxic effect on RAW264.7 cells but decreased the intracellular growth of L. monocytogenes in RAW264.7 cells. Furthermore, subinhibitory concentrations of glabridin triggered ROS production but did not induce MET formation in macrophages. In addition, glabridin did not enhance the capacity of L. monocytogenes to trigger METs or the extracellular killing of macrophages by METs. Thus, we conclude that subinhibitory concentrations of glabridin reduce L. monocytogenes motility and hemolytic activity but do not exhibit antimicrobial activity. Glabridin could be an interesting food additive as a bacteriostatic agent with anti-Listeria activity.
1 Introduction
Listeria monocytogenes (L. monocytogenes) is a gram-positive, facultative intracellular bacterial pathogen. It is ubiquitous and widely distributed in soil, vegetation, animal-derived food products, silage, excrement, sewage, and water (Hadjilouka et al., 2016). L. monocytogenes is the only Listeria species known to be highly pathogenic in humans and is considered to be the most frequently reported foodborne pathogen (Zhang et al., 2024). The occurrence of mild febrile gastroenteritis is one of the most common symptoms in healthy individuals following the consumption of L. monocytogenes-contaminated food products. In particular, L. monocytogenes leads to more serious invasive diseases, including septicemia, encephalitis, meningitis, peritonitis, recurrent spontaneous abortion (Xu et al., 2024), and even death, in elderly, neonatal, or pregnant patients, as well as chemotherapy-treated patients, transplant recipients, and immunocompromised patients (Budvytyte et al., 2024; Vazquez et al., 2024). The expression of many virulence factors by L. monocytogenes potentially contributes to its ability to enter into host cells, survive, and spread to infect adjacent cells (Osek and Wieczorek, 2022). Although large outbreaks of listeriosis have been reported in some countries and regions, these cases account for only a small proportion of all listeriosis cases (Mohapatra et al., 2024), and the case-fatality rate is usually as high as 20–30% in humans (Huang et al., 2023). Approximately 1,600 cases of human listeriosis are reported each year in the United States, 20% of which result in death (Pogreba-Brown et al., 2022). Therefore, L. monocytogenes remains a serious public hygiene problem with a heavy economic burden throughout the world, especially in countries with no network management system for complete food hygiene surveillance.
Over the past century, more than 200 antibiotics have been widely used to defend against pathogenic infections and are considered a key therapeutic modality for almost all diseases to date (Mehdi et al., 2018). L. monocytogenes is usually susceptible to a wide range of antibiotics in human and veterinary medicine, and the administration of penicillin, ampicillin, and gentamicin is used as the first choice (Arslan and Ozdemir, 2020). The abuse or overuse of antibiotics has led to a rapid increase in the prevalence of antibiotic-resistant bacteria (Mesa-Ramos et al., 2024). Although current antibiotic strategies are still effective options for the treatment of listeriosis (Kumaraswamy et al., 2018), the emergence of multidrug-resistant L. monocytogenes isolates from river water, traditionally consumed food products, and human clinical specimens has been documented since the isolation of the first multiresistant strain of L. monocytogenes in France in 1988 (Poyart-Salmeron et al., 1990; Harakeh et al., 2009; Soni et al., 2013), and the prevalence rate is increasing in China and other countries (Lachtara et al., 2023; Zhang et al., 2024). Therefore, developing new drugs and alternative intervention approaches for the treatment of listeriosis has become imperative.
Licorice, called Gan-Cao, which has a 2000-year history of application in China, belongs to the genus Glycyrrhiza (family: Fabaceae/Leguminosae). It is derived from the roots and stolons of Glycyrrhiza uralensis Fisch., Glycyrrhiza inflata Bat., and Glycyrrhiza glabra L. Studies have shown that licorice is used in flavoring and sweetening agents, demulcents, expectorants, and anti-allergic and anti-inflammatory agents (Ji et al., 2024). It has widespread pharmacological effects on human diseases, including atherosclerosis, immunodeficiency, hormone deficiency, endocrine disruption, cancer, and skin diseases (Kwon et al., 2020). The extracts of traditional Chinese herbal medicines contain many effective ingredients. A large number of the active components, including glycyrrhizin acid, glucose, ammonia, polyphenols, flavonoids, isoflavonoids, chalcones, triterpenoids, and saponins, have been isolated from licorice (Pastorino et al., 2018).
Licorice has become the subject of intense global research efforts in recent years (Kuang et al., 2018). At present, these studies have focused mainly on herbal monomers with active components of Chinese herbal extracts. More than 300 flavonoids of licorice, including liquiritin, glycyrrhizic acid, glabridin, liquiritigenin, and isoliquiritigenin, have been identified. Glabridin [(R)-4-(3,4-dihydro-8,8-dimethyl)-2H, 8H-benzo [1,2-b:3,4-b′]dipyran-3yl-1,3-benzenediol, C20H20O4], a polyphenolic flavonoid compound in licorice, is considered one of the key biologically active components. Since the structure of glabridin was first described by Saitoh et al. (1976), it has been found to possess multiple beneficial properties, including antibacterial, antifungal, antiparasitic, antioxidation, anti-inflammatory, antiatherogenic, antifatigue, anticancer, antiobesity, antidiabetes, estrogenic, bone protection, cardiovascular protection, hepatoprotection, and neuroprotective effects (Zhang J. et al., 2023).
Although antibiotics are small molecules with positive and direct therapeutic activity in killing microbes or inhibiting microbial growth, they also have negative effects on bacteria. Exposure to subinhibitory concentrations of antibiotics significantly increases biofilm formation in several pathogenic species (Bernardi et al., 2021). Recently, glabridin was reported to exhibit antimicrobial activity against L. monocytogenes, with a minimum inhibitory concentration (MIC) of 12.5 μg/mL (Bombelli et al., 2023). However, little is known about the effects of subinhibitory concentrations of the Chinese herbal medicines on bacteria. Considering the above findings, we were interested in determining whether subinhibitory concentrations of glabridin affect the characteristics of L. monocytogenes in this study. For this purpose, we analyzed the effects of subinhibitory concentrations of glabridin on the morphology, motility, biofilm production, adherence, and survival of L. monocytogenes.
2 Materials and methods
2.1 Bacterial strain and growth conditions
The L. monocytogenes 10,403 s strain was used in this study and was grown in brain heart infusion (BHI, Haibo, Qingdao, China) broth media supplemented with 1% (w/v) peptone, 1.25% (w/v) dehydrated calf brain infusion, 0.5% (w/v) dehydrated beef heart infusion, 0.5% (w/v) NaCl, 0.2% (w/v) glucose, and 0.25% (w/v) Na2PO4 under sterile conditions. The medium was filter-sterilized with a 0.2 μm filter (Beyotime Biotechnology, Shanghai, China). Before exposure to glabridin or macrophages, the bacterial cultures were incubated in sterile 50 mL closed conical tubes (Guangzhou Jet Bio-Filtration Co., Ltd., Guangzhou, China) at 37°C under static conditions or with shaking at 180 rpm.
2.2 Determination of the MIC and minimum bactericidal concentration (MBC)
Glabridin (molecular weight of 324.40 g/moL) from Glycyrrhiza glabra L. root (licorice) was obtained from Luoyang Lansealy Technology Co., Ltd. (Luoyang, China). The antibacterial activity of glabridin against L. monocytogenes 10,403 s was determined using broth microdilution methods (MIC and MBC), as previously described (Fang et al., 2018). Briefly, 2-fold serial dilutions of glabridin ranging from 1.95 to 500 μg/mL were prepared and then added to wells containing bacteria (1 × 106 CFUs/ml) in a 96-well plate. The plates were incubated overnight at 37°C. Finally, the MIC was defined as the lowest sample concentration that prevented microbial growth according to the OD600 nm. A 1:10 dilution of each concentration in fresh broth was spread onto BHI (Haibo, Qingdao, China) agar plates to determine the MBC. After an incubation at 37°C for 24 h, the MBC was recorded as the minimum concentration at which no apparent growth on agar subculture occurred. Triplicates of this experiment were conducted.
2.3 Determination of the growth level
Growth curves were generated to determine the effect of subinhibitory concentrations of glabridin on L. monocytogenes growth, as previously described (Jiang et al., 2019). Briefly, cultures of L. monocytogenes at the exponential phase in the BHI medium were collected by centrifugation and washed once with fresh BHI medium. Then, the cell’s initial concentration was adjusted to a concentration of 1 × 106 CFUs/ml, and the cells were incubated in BHI media supplemented with subinhibitory concentrations (1/8, 1/4, or 1/2 of the MIC) or the MIC of glabridin with shaking at 37°C. The optical density at 600 nm was measured each hour. Moreover, viable bacteria were counted by serial dilution, plating onto BHI agar, and incubation at 37°C for 24 h.
Moreover, the colony morphology of L. monocytogenes was evaluated on BHI agar plates. Cultures of L. monocytogenes at the exponential phase in BHI medium were collected by centrifugation and washed once with PBS (10 mM, pH 7.4). Ten microliters (~104 CFUs) of the culture solution were dripped on BHI agar supplemented with subinhibitory concentrations (1/8, 1/4, or 1/2 of the MIC) or the MIC of glabridin. Following an overnight incubation at 37°C, the colony morphology was observed and photographed. The colony growth diameter was measured using a micrometer.
2.4 Cellular morphology
Cultures of L. monocytogenes at the exponential phase in BHI medium supplemented with subinhibitory concentrations (1/8, 1/4, or 1/2 of the MIC), the MIC, or MBC of glabridin were collected by centrifugation and washed once with PBS (10 mM, pH 7.4) to observe cellular morphology. Then, the cell suspensions were uniformly spread onto sterile glass coverslips. Bacteria were stained with Gram stain and examined under a light microscope.
Moreover, scanning electron microscopy (SEM) was performed via an earlier procedure (Jalil Sarghaleh et al., 2023). Cultures of L. monocytogenes at the exponential phase in BHI medium supplemented with subinhibitory concentrations (1/8, 1/4, or 1/2 of the MIC), the MIC, or MBC of glabridin were collected by centrifugation and washed once with PBS (10 mM, pH 7.4). Then, the cell suspensions were uniformly spread onto sterile glass coverslips. A 2.5% (v/v) glutaraldehyde solution (Servicebio, Wuhan, China) was used for stabilization and incubated with the samples at 4°C for 2 h. After washing with distilled water, ethanol gradients (25, 50, 75, 95, and 100%) were used for the final dehydration. After air drying at room temperature, critical point drying was performed with liquid CO2. The samples were covered with a layer of gold and then observed under a JEOL scanning electron microscope (JSM-IT200 InTouchScope™, JEOL, Tokyo, Japan).
2.5 Motility assay
The motility phenotype was assayed on soft BHI agar as described previously (Roy et al., 2022), with minor modifications. Briefly, 0.25% (w/v) and 0.5% (w/v) agar were mixed with BHI for the swimming and swarming studies, respectively. Cultures (~104 CFUs) at the exponential phase were point inoculated onto BHI agar plates supplemented with subinhibitory concentrations (1/8, 1/4, or 1/2 of the MIC) of glabridin. The culture medium was incubated at 30°C for 24 h, and the migration zone (mm) was determined to report the bacterial motility in the agar by measuring the diameter using a micrometer. Each group was independently tested three times.
2.6 Transmission electron microscopy (TEM)
TEM experiments were performed to investigate the flagella presence as described previously (Wang et al., 2024). Bacteria from 0.25% BHI agar plates supplemented with subinhibitory concentrations (1/8, 1/4, or 1/2 of the MIC) of glabridin were fixed with a 3% paraformaldehyde solution on an electron microscopy grid. Then, the grids were washed three times. The bacteria were stained with phosphotungstic acid (2%) for 10 s at room temperature, the excess liquid was gently removed using filter paper, and the grids were air-dried. The dried grids were observed under a Hitachi transmission electron microscope (HT7700, Hitachi High-Tech Corporation, Tokyo, Japan).
2.7 Biofilm formation assays
The effect of subinhibitory concentrations of glabridin on the formation of biofilms was determined using a crystal violet assay according to the procedure described by Djordjevic et al. (2002), with slight modifications. Assays were performed in sterile 96-well plates. Briefly, overnight cultures were added to the wells with 100 μL of fresh medium supplemented with subinhibitory concentrations (1/8, 1/4, or 1/2 of the MIC), the MIC or MBC of glabridin. The plates were placed in an incubator at 37°C for 48 h. Subsequently, the supernatant was discarded, and then the wells were gently washed three times with aseptic PBS. The plates were blotted dry on a sterilized paper towel after the last wash and allowed to dry naturally for 1 h. Then, the biofilms were stained with 2% (m/v) crystal violet for 45 min. The wells were washed with distilled water to remove the unbound dye. After drying at 37°C for 10 min, the cell-bound dye was dissolved in 200 μL of 95% (w/w) ethanol. The quantitative evaluation of biofilm formation was performed on a microplate reader (Infinite M Nano, TECAN, Switzerland) by measuring the absorbance of the solution at 570 nm.
Moreover, the biofilm formation of L. monocytogenes was investigated using confocal laser scanning microscopy (CLSM) (Adamus-Bialek et al., 2015). Initially, overnight cultures were seeded onto 20-mm glass cover slips in 12-well plates in the absence or presence of glabridin. After an incubation at 37°C for 48 h to allow biofilm formation, the culture medium was discarded, and then the wells were gently washed three times with aseptic PBS. Subsequently, the biofilms were stained with SYTO Green (KeyGEN BioTECH, Nanjing, China) and PI (Beyotime Biotechnology, Shanghai, China), a green and red fluorescent nucleic acid stain, for up to 20 min at room temperature in the dark. The samples were visualized under a confocal laser scanning microscope (FV3000, Olympus, Japan) at an excitation wavelength of 500 nm and emission wavelength of 535 nm, according to the manufacturer’s instructions.
SEM was used to visualize the morphologies of the L. monocytogenes biofilms, as previously described (Wang et al., 2022), with slight modifications. The fresh cultures were placed on 20-mm glass cover slips in the wells of 12-well plates. After the incubation, the attached cells were washed three times with PBS to remove the planktonic cells. The samples were fixed with a 2.5% (v/v) glutaraldehyde solution (Servicebio, Wuhan, China) at 4°C for 10 h. After washes with distilled water, the samples were subjected to dehydration with an increasing series of ethanol solutions (25, 50, 75, 95, and 100%). After air drying at room temperature, critical point drying was performed with liquid CO2. Subsequently, the samples were covered with a layer of gold using a sputter coater and examined using a JEOL scanning electron microscope (JSM-IT200 InTouchScope™, JEOL, Tokyo, Japan).
2.8 Biofilm eradication assays
The evaluation of the biofilm-eradicating ability of glabridin was performed according to the previously described methods with further modifications (Shen et al., 2021). Briefly, overnight cultures were added to 96-well plates and incubated for 48 h at 37°C to form a biofilm. After the incubation, the culture medium was removed and replaced with sterile BHI medium by washing three times with PBS. Subsequently, subinhibitory concentrations (1/8, 1/4, or 1/2 of the MIC), the MIC, and MBC of glabridin were added to the wells. The plates were incubated at 37°C for 1 h. After the incubation, the methods mentioned above (crystal violet assay, CLSM, and, SEM) were used to evaluate biofilm eradication.
2.9 Hemolytic activity assay
Hemolytic activity was assayed using the blood-plate method as previously described (Kawacka et al., 2022). Briefly, 5.0% of sterile sheep blood was mixed with BHI to prepare a blood agar plate. L. monocytogenes cultures with logarithmic growth (~104 CFUs) at the exponential phase were point-inoculated onto BHI agar plates supplemented with subinhibitory concentrations (1/8, 1/4, and 1/2 of MIC) of glabridin. The culture medium was incubated at 37°C for 24 h. The plates were then visually examined for clear zones or no zones around colonies. The experiments were independently repeated three times.
Moreover, the hemolytic activity was determined by performing a microdilution method according to a previous study (Yue et al., 2023). Briefly, L. monocytogenes cultures with logarithmic growth (~106 CFUs) were treated with subinhibitory concentrations (1/8, 1/4, or 1/2 of the MIC) of glabridin at 37°C and 120 rpm for 12 h. Bacterial cultures were centrifuged at 12,000 rpm for 10 min. Then, the supernatant was filtered through a 0.22 μm pore filter (Guangzhou Jet Bio-Filtration Co., Ltd., Guangzhou, China). After filtration, 100 μL of the cell-free supernatant was mixed with 100 μL of a 5.0% (v/v) red blood cell suspension in a 96-well plate and incubated for 1 h at 37°C. Subsequently, the tested samples were gathered by centrifugation at 1000 rpm for 5 min. Finally, absorbance measurements were performed at 540 nm with a microplate reader. BHI and 1% Triton X-100 were served as negative and positive controls, respectively. The percentage of hemolysis (%) = (OD540 nm of test well – OD540 nm of negative control)/(OD540 nm of positive control – OD540 nm of negative control) × 100%.
2.10 Real-time PCR
L. monocytogenes cultures with logarithmic growth were initially treated with subinhibitory concentrations (1/8, 1/4, or 1/2 of the MIC) of glabridin at 37°C and 120 rpm for 12 h. The samples were centrifuged at 12,000 rpm for 5 min, and the supernatant was discarded. Total RNA was extracted using a TRNpure Total RNA Kit (Nobelab Biotech, Beijing, China) based on the manufacturer’s manual. The RNA concentration, purity, and integrity were assessed using a NanoDrop 2000c (Thermo, Massachusetts, United States). Then, the cDNA strand was synthesized using an EasyScript® All-in-One First-Strand cDNA Synthesis SuperMix for qPCR (One-Step gDNA Removal) (TransGen, Beijing, China), according to the manufacturer’s protocol. Reactions (20 μL) containing 100 ng of RNA, the mix, and primers were prepared. The thermal cycling conditions were 42°C for 15 min and 85°C for 5 s using a T100 Thermal Cycler (T100™; Bio-Rad Laboratories, California, United States). cDNA was quantified using a NanoDrop 2000c. Beacon Designer (version 8.0) software was used to design specific primers for the hly (GenBank: DQ054589.1; forward: 5′-TGCAAGTCCTAAGACGCCA-3′, reverse: 5′-CACTGCATCTCCGTGGTATACTAA-3′) gene (Tamburro et al., 2015). The 16S rRNA (GenBank: CP002002.1; forward: 5′-TTAGCTAGTTGGTAGGGT-3′, reverse: 5′-AATCCGGACAACGCTTGC-3′) gene is considered a housekeeping gene for L. monocytogenes (Fraser et al., 2003). The amplification profile was as follows: predenaturation at 94°C for 30 s and 40 cycles of 5 s at 94°C and 30 s at 60°C. Reactions (20 μL) containing PerfectStart® Green qPCR SuperMix (TransGen, Beijing, China), cDNA, and specific primers were performed in a CFX96 real-time system (Bio-Rad Laboratories, California, United States). All samples were prepared in triplicate. The mRNA expression level of the hly gene was normalized to the expression level of 16S rRNA. The expression levels in the untreated group were normalized to 1 for comparison with those in the treated group. The relative expression levels of the hly gene were analyzed using the 2−ΔΔCT relative expression quantification method: ΔΔCt = (mean Ct value of hly in the sample – mean Ct value of 16S rRNA in the sample) – (mean Ct value of hly in the control – mean Ct value of 16S rRNA in the control).
2.11 Eukaryotic cell line and culture conditions
RAW264.7 cells were purchased from Procell Life Science and Technology Co., Ltd. (Wuhan, China). The RAW264.7 cells and Caco-2 cells were stored in the Luoyang Key Laboratory of Live Carrier Biomaterial and Animal Disease Prevention and Control. The cells were grown in DMEM (HyClone, United States) supplemented with 10% heat-inactivated (56°C, 30 min) fetal bovine serum (FBS; Clark Bioscience, Dalian, United States) and maintained at 37°C with 5% CO2 in a humidified incubator. Then, 100 U/mL penicillin and 100 μg/mL streptomycin (Beijing Solarbio Science & Technology Co., Ltd., Beijing, China) were added when needed. The cells were passaged in 25 cm2 flasks (Guangzhou Jet Bio-Filtration Co., Ltd., Guangzhou, China). After the cells were counted using a hemocytometer, they were seeded into 24-well plates (Guangzhou Jet Bio-Filtration Co., Ltd., Guangzhou, China) for subsequent procedures.
2.12 Cytotoxicity test
The cytotoxicity of glabridin was evaluated using a lactate dehydrogenase (LDH) assay kit as previously described (Hou et al., 2023). Briefly, RAW264.7 cells and Caco-2 cells were seeded at a density of 2 × 105 cells per well in a 24-well cell culture plate, respectively. The cells were incubated in DMEM supplemented with subinhibitory concentrations (1/8, 1/4, or 1/2 of the MIC), the MIC, or MBC of glabridin for 6 h. The cells treated with 0.2% Triton X-100 or DMEM only were used as the positive and negative controls, respectively. The cell culture supernatants were centrifuged and collected. The levels of LDH release were measured using an LDH Cytotoxicity Assay Kit (Beyotime Biotechnology, Shanghai, China) in accordance with the manufacturer’s instructions. The absorbance at 490 nm was read using a microplate reader. The results are shown as (OD490 nm of sample – OD490 nm of negative control)/(OD490 nm of positive control – OD490 nm of negative control) × 100%.
2.13 Intracellular growth assay in macrophages
The intracellular growth assay was performed as previously described (Meng et al., 2015; Zhou et al., 2017). RAW264.7 cells were seeded at a density of 2 × 105 cells per well in 24-well plates with antibiotic-free medium. The cells were incubated with subinhibitory concentrations (1/8, 1/4, or 1/2 of the MIC) of glabridin, infected with bacteria at an MOI of 10:1, and then incubated at 37°C with 5% CO2 for 1 h. The cells were rinsed three times with PBS and incubated with 100 μg/mL gentamicin for 1 h to kill the extracellular bacteria. Then, the cells were washed three times with PBS to remove the killed extracellular bacteria. The infected RAW264.7 cells were then incubated with fresh DMEM supplemented with subinhibitory concentrations (1/8, 1/4, or 1/2 of the MIC) of glabridin and 10% FBS for 3h. The cells were subsequently lysed with 0.1% Triton X-100 in PBS for 5 min. The number of viable intracellular bacteria was determined by plating serial dilutions of the resulting lysates on BHI agar plates. The experiments were repeated in three independent assays.
2.14 MET formation in macrophages
Macrophage extracellular traps (METs) were visualized as previously described (Cogen et al., 2010; Brinkmann et al., 2012). RAW264.7 cells (2 × 105 cells per well) were plated onto laser confocal Petri dishes in DMEM without antibiotics or fetal calf serum. The cells were incubated with subinhibitory concentrations (1/8, 1/4, or 1/2 of the MIC) of glabridin, infected with L. monocytogenes at an MOI of 10:1, and then incubated at 37°C with 5% CO2 for 2 h. The cells were stained with Hoechst 33342 (Beyotime Biotechnology, Shanghai, China) and observed via confocal laser scanning microscopy (FV3000, Olympus, Japan) at 350 nm excitation and 460 nm emission. Images of random fields were acquired from each group (Zhang X. W. et al., 2023). The MET formation in the PMA-treated macrophages was set as 100%. The data are presented as percentages of the PMA-treated group.
2.15 Intracellular ROS generation
Intracellular ROS generation was detected according to a previously described procedure (Liu et al., 2014). RAW264.7 cells (2 × 105 cells per well) were seeded onto laser confocal Petri dishes in DMEM without antibiotics or fetal calf serum. The cells were incubated with subinhibitory concentrations (1/8, 1/4, or 1/2 of the MIC) of glabridin, infected with L. monocytogenes at an MOI of 10:1, and then incubated at 37°C with 5% CO2 for 2 h. DCFH-DA (5 μM, Beyotime, Shanghai, China) was added, and the cells were continuously incubated in the dark. The samples were observed under a confocal laser scanning microscope at 488 nm excitation and 525 nm emission.
2.16 MET-mediated extracellular killing assay in macrophages
The MET-mediated extracellular killing assay was performed as described previously (Liu et al., 2014). Briefly, 2 × 105 RAW264.7 cells per well were seeded into a 24-well plate. The cells were incubated with subinhibitory concentrations (1/8, 1/4, or 1/2 of the MIC) of glabridin, infected with L. monocytogenes at an MOI of 10:1, and then incubated at 37°C with 5% CO2 for 2 h. Cytochalasin D (10 μg/mL; Beijing Solarbio Science & Technology Co., Ltd., Beijing, China) or 100 U/mL protease-free DNase I (Sangon Biotech (Shanghai) Co., Ltd., Shanghai, China) was added to inhibit phagocytosis or MET formation at 20 min before infection with L. monocytogenes. Pretreatment with cytochalasin D and DNase I was used as the 100% survival control group. The experiments were repeated five times. Bacteria were serially diluted and evenly plated onto BHI agar to determine the number of CFUs.
2.17 Statistical analysis
All experiments were performed in three independent replicates. The data are presented as means ± SEMs. Statistical significance was determined using two-tailed Student’s t-test. p values indicated in the figures (*p < 0.05 and **p < 0.01) were considered significant.
3 Results
3.1 Effect of subinhibitory concentrations of glabridin on the growth of L. monocytogenes
As determined using the microdilution method, the MIC value for L. monocytogenes was 31.25 μg/mL of glabridin. The results indicated that the MBC of glabridin against L. monocytogenes was 125 μg/mL. The optical densities of the bacterial suspension were measured at 600 nm to determine the effect of subinhibitory concentrations of glabridin on the growth of L. monocytogenes. Following an overnight incubation in BHI medium, the growth profiles of glabridin-treated and -untreated L. monocytogenes were similar (Supplementary Figure S1A). As shown in Figure 1, the growth of L. monocytogenes was not significantly affected by glabridin concentrations of 3.91, 7.81, or 15.63 μg/mL, which are equal to 1/8, 1/4, or 1/2 of the MIC of glabridin, respectively (Figure 1A). Moreover, the colony morphologies of the glabridin-treated and -untreated L. monocytogenes strains were compared. The detailed results are presented in Figure 1B. The colony morphology of the glabridin-treated L. monocytogenes after incubation with subinhibitory concentrations was similar to that of the glabridin-untreated L. monocytogenes in BHI agar plates (Figure 1B). As the glabridin concentration increased, no significant differences in colony size were observed (Supplementary Figure S1B). The colonies of all groups appeared as milky white, opaque colonies with smooth surfaces. Therefore, our results suggested that subinhibitory concentrations of glabridin did not affect the growth of L. monocytogenes.
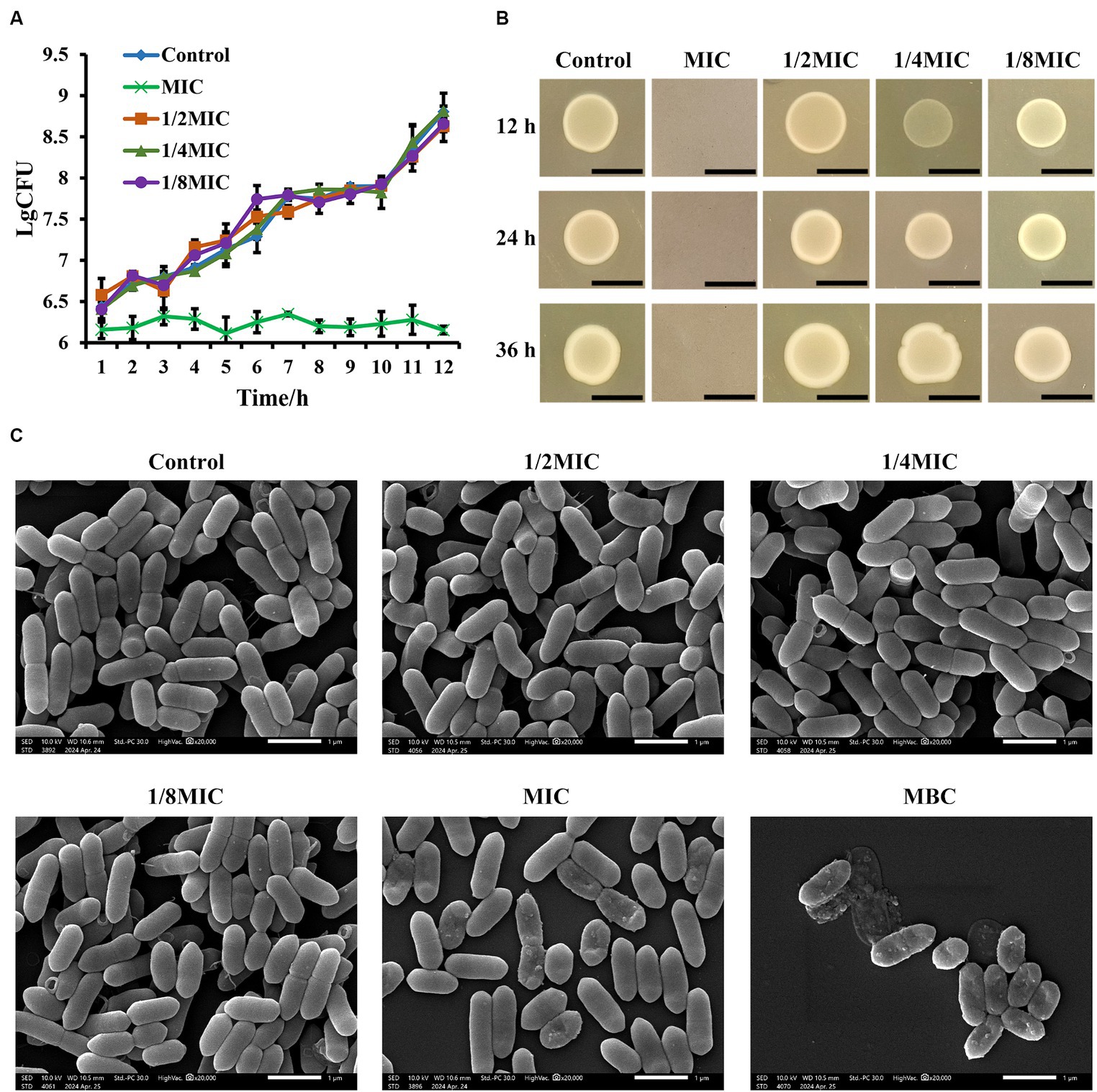
Figure 1. Effect of subinhibitory concentrations of glabridin on the growth and morphology of L. monocytogenes. (A) Growth curves of L. monocytogenes in subinhibitory concentrations (1/8, 1/4, or 1/2 of the MIC) or the MIC of glabridin. There was no statistically significant difference between bacterial growth of the glabridin-treated and -untreated cultures after overnight incubation in BHI medium. (B) Colony morphology of L. monocytogenes in subinhibitory concentrations of glabridin. L. monocytogenes treated with subinhibitory concentrations (1/8, 1/4, or 1/2 of the MIC) or the MIC of glabridin were grown in BHI agar, and no difference in colony morphology was observed. Scale bar: 5 mm. (C) Scanning electron microscopy images of the cellular morphology of L. monocytogenes treated with subinhibitory concentrations (1/8, 1/4, or 1/2 of the MIC), the MIC or MBC of glabridin. L. monocytogenes were grown in BHI medium and observed under a Hitachi scanning electron microscope. Scale bar: 1 μm. The experiments were repeated three times.
3.2 Effect of subinhibitory concentrations of glabridin on the morphology of L. monocytogenes
Gram staining and SEM were performed to determine the effect of subinhibitory concentrations of glabridin on the morphology of L. monocytogenes. The light microscopic examination of bacterial staining indicated that L. monocytogenes treated with 1/8, 1/4, or 1/2 of the MIC for glabridin had the same cellular morphology as the glabridin-untreated L. monocytogenes (Supplementary Figure S1C). The microscopic appearance of the glabridin-treated L. monocytogenes was further observed via SEM. SEM images of L. monocytogenes before and after treatment with subinhibitory concentrations of glabridin (1/8, 1/4, or 1/2 of the MIC) are shown in Figure 1C. The structure of L. monocytogenes is naturally rod-shaped. Treatment of the bacteria with glabridin (1/8, 1/4, or 1/2 of the MIC) did not cause changes in the surface morphology (Figure 1C). Under a light microscope, the results revealed that the number of bacteria in the group treated with the MIC of glabridin was significantly lower than the number of bacteria in the groups treated with subinhibitory concentrations of glabridin (Supplementary Figure S1C). However, SEM confirms the shrunken, crumpled appearance of the membranes of some bacteria in the group treated with the MIC of glabridin (Figure 1C). Moreover, the bacteria were disrupted after the application of the MBC of glabridin (Figure 1C). Therefore, in the following experiments in this study, subinhibitory concentrations of glabridin, 1/8, 1/4, or 1/2 of the MIC, were used since they did not affect the growth or morphology of L. monocytogenes.
3.3 Subinhibitory concentrations of glabridin inhibit the motility of L. monocytogenes
Flagellum-mediated motility is involved in the bacterial pathogenicity, biofilm formation, drug resistance, and chronic infections. Thus, we examined whether subinhibitory concentrations of glabridin affect the motility of L. monocytogenes in vitro. A semisoft agar assay with 0.3% (w/v) and 0.5% (w/v) agar was used to monitor the swimming and swarming of L. monocytogenes, respectively. In the swimming test, we observed a significant decrease in the mobility of L. monocytogenes exposed to glabridin (1/4 or 1/2 of the MIC) through soft agar (Figure 2A). In addition, the quantitative experimental results showed that the swimming expansion rate of L. monocytogenes colonies in the presence of 1/8 of the MIC of glabridin did not differ from that of glabridin-untreated L. monocytogenes colonies (Figure 2B). As depicted in Figure 2, for the swarming test, the motility of L. monocytogenes decreased with increasing concentrations of glabridin (1/8, 1/4, or 1/2 of the MIC) in 0.5% BHI agar, and the effect was concentration-dependent (Figure 2A). The zone size of L. monocytogenes exposed to glabridin at a concentration of 1/2 of the MIC was significantly lower than that at a concentration of 1/8 of the MIC (Figure 2B). Therefore, subinhibitory concentrations of glabridin inhibited bacterial swimming and swarming motility in L. monocytogenes.
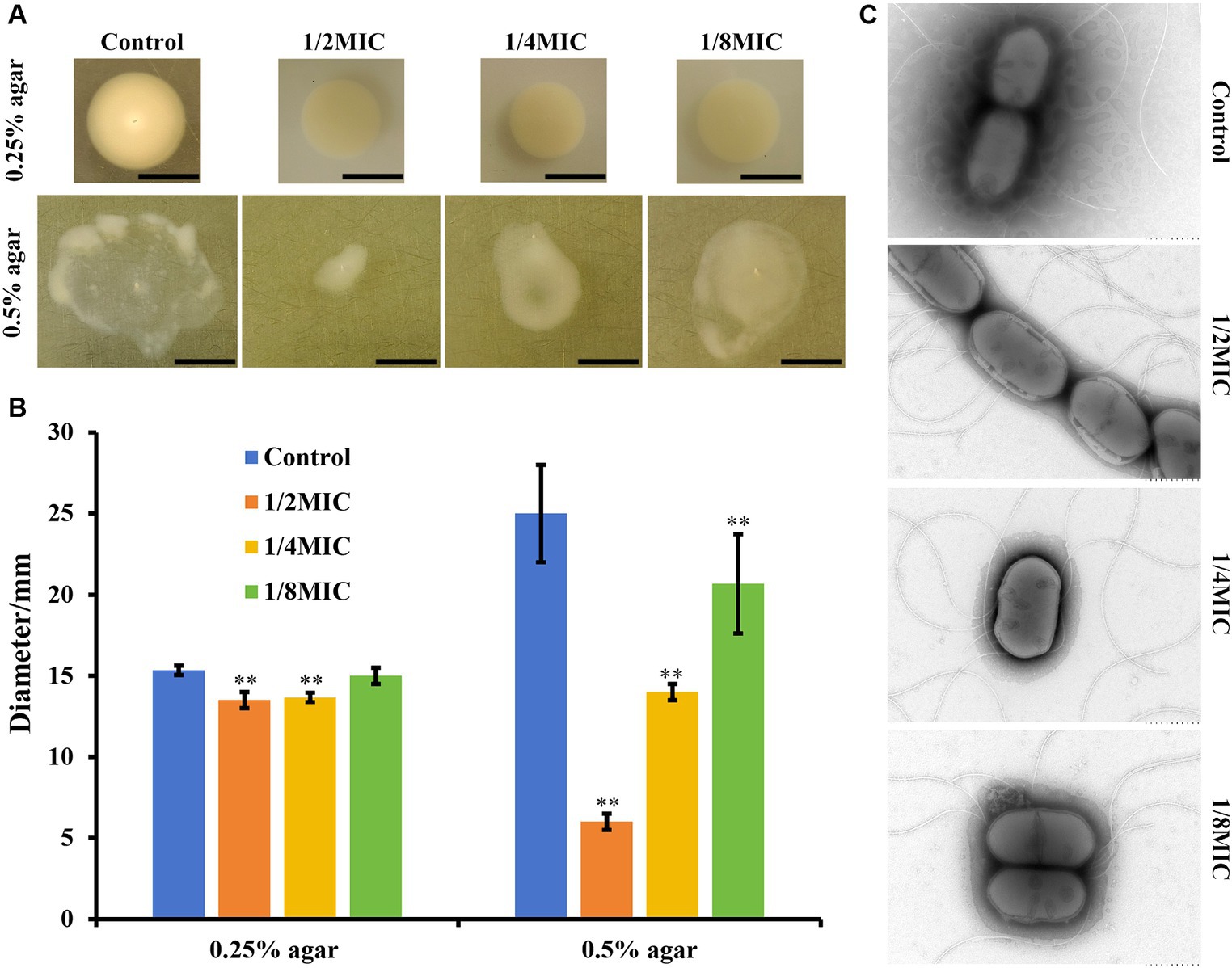
Figure 2. Motility of L. monocytogenes in subinhibitory concentrations (1/8, 1/4, or 1/2 of the MIC) of glabridin in semisoft agar plates. (A) The bacterial cultures (~104 CFUs) at the exponential phase were spotted on the center of freshly prepared BHI with 0.3% (w/v) and 0.5% (w/v) agar for the swimming and swarming studies, respectively. Subinhibitory concentrations (1/8, 1/4, or 1/2 of the MIC) of glabridin were added. After an incubation for 24 h at 30°C, the plates were imaged and measured using calipers. (B) Representative images and the diameter of swimming and swarming zones. **p < 0.01 indicates statistical significance compared with the control. (C) Transmission electron microscopic visualization of the effects of glabridin on flagella production. L. monocytogenes was grown in 0.3% BHI agar plates in the absence or presence of subinhibitory concentrations (1/8, 1/4, or 1/2 of the MIC) of glabridin. Bacteria were visualized using transmission electron microscopy with negative staining. Scale bar: 500 nm.
3.4 Effect of subinhibitory concentrations of glabridin on flagellar production in L. monocytogenes
TEM was used to further determine whether subinhibitory concentrations of glabridin adversely affected flagella synthesis. Interestingly, the results showed that L. monocytogenes exposed to different concentrations of glabridin were able to produce flagellar filaments, as shown by negative staining of the glabridin-untreated L. monocytogenes (Figure 2C). No significant difference was found. Therefore, the results clearly showed that subinhibitory concentrations of glabridin did not interfere with flagellar production in L. monocytogenes.
3.5 Effect of subinhibitory concentrations of glabridin on L. monocytogenes biofilms
Bacteria were grown on polystyrene in BHI plates supplemented with subinhibitory concentrations (1/8, 1/4, or 1/2 of the MIC) of glabridin to study whether subinhibitory concentrations of glabridin influence biofilm formation of L. monocytogene in vitro. Biofilm formation was quantified after 48 h by performing crystal violet staining. As shown in Figure 3A, L. monocytogenes produced a biofilm in the absence of glabridin. Although a declining trend in biomass was observed after the bacteria were exposed to subinhibitory concentrations of glabridin, a statistically significant difference was not observed after culture in the absence or presence of glabridin (Supplementary Figure S2A). The corresponding fluorescence microscopy images are shown in Figure 3A. Consistent with the above results, L. monocytogenes showed a similar phenomenon in terms of the biofilm biomass with or without glabridin treatment (Figure 3A). Since a green color is used to stain cells, the cells treated with or without glabridin were clearly green and observed in the form of dots. Moreover, very small amounts of biofilm production were observed after treatment with glabridin at the MIC but not at the MBC (Figure 3A). SEM imaging revealed biofilm morphologies and supported the CLSM results. Based on the SEM images, many microcolonies that held together were observed in the absence of glabridin, whereas no change occurred after treatment with subinhibitory concentrations (1/8, 1/4, or 1/2 of the MIC) of glabridin (Figure 3B). However, compared with those in the control group, a few cells were attached to the glass coupons after treatment with glabridin at the MIC, and the bacteria exhibited a normal morphology. Interestingly, the bacteria were disrupted after the application of glabridin at the MBC (Figure 3B), and the bacterial morphology was obviously wrinkled.
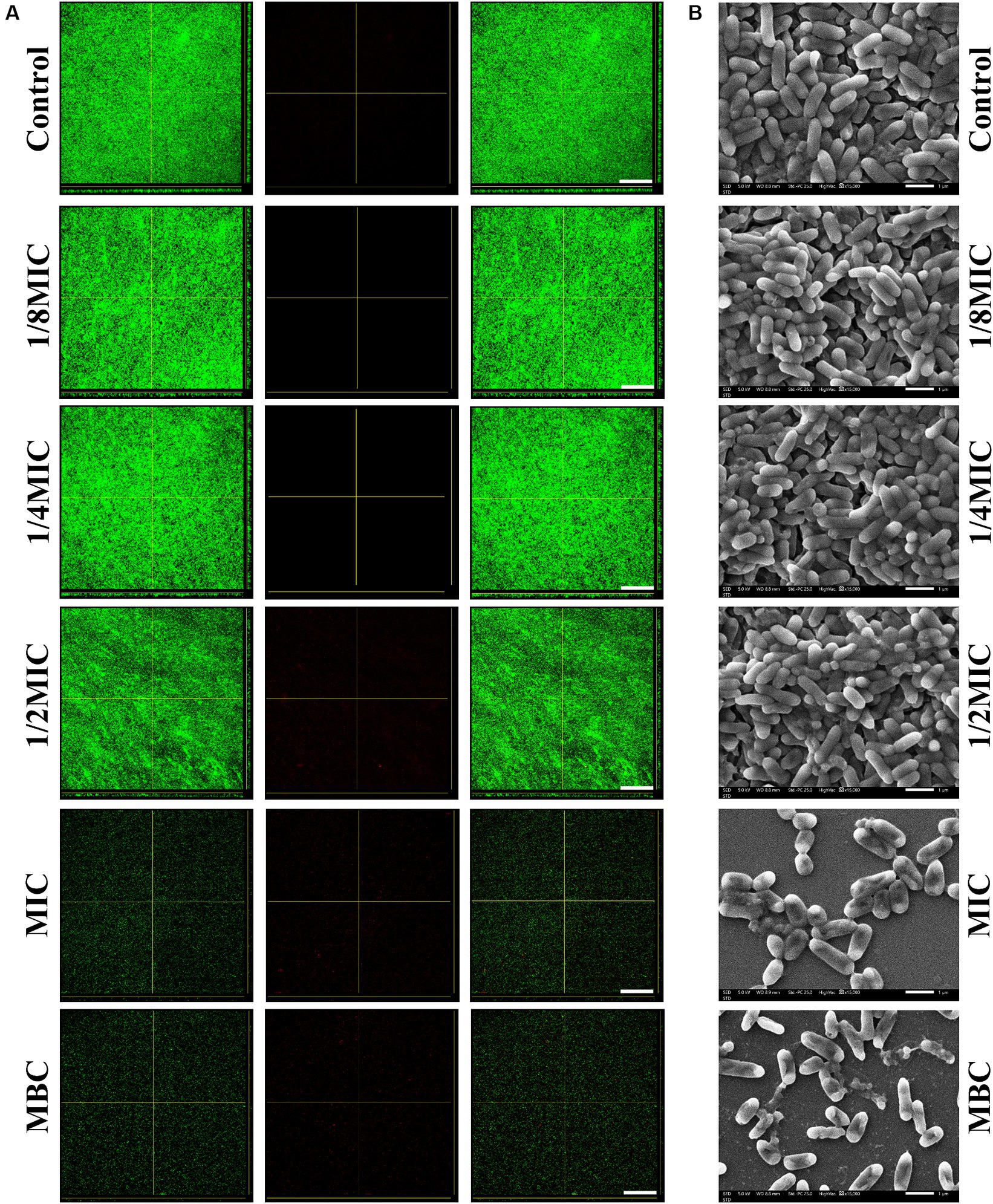
Figure 3. Biofilm formation of L. monocytogenes in the presence of subinhibitory concentrations (1/8, 1/4, or 1/2 of the MIC) of glabridin. (A) Confocal laser scanning microscopy images of biofilms of L. monocytogenes treated with subinhibitory concentrations (1/8, 1/4, or 1/2 of the MIC), the MIC or MBC of glabridin after 48 h of incubation at 37°C. Scale bar: 200 μm. (B) Scanning electron microscopy images of biofilms of L. monocytogenes treated with subinhibitory concentrations (1/8, 1/4, or 1/2 of the MIC), the MIC or MBC of glabridin after 48 h of incubation at 37°C. Scale bar: 1 μm.
According to the crystal violet staining results, none of the subinhibitory concentrations (1/8, 1/4, or 1/2 of the MIC) or the MIC of glabridin could eradicate the complete biofilms of L. monocytogenes (Figure 4A; Supplementary Figure S2B). What is more, at the MBC, glabridin did not eradicate the formed biofilms. Furthermore, CLSM images indicated that subinhibitory concentrations (1/8, 1/4, or 1/2 of the MIC) or the MIC of glabridin had no obvious effect on the biofilms (Figure 4B). Then, SEM images of the bacterial biofilms were recorded. The thick and dense layer of bacteria in the control group was smooth and intact (Figure 4B), similar to that observed after treatment with subinhibitory concentrations (1/8, 1/4, or 1/2 of the MIC) or the MIC of glabridin (Figure 4B). When the bacteria were exposed to glabridin at the MBC, the bacteria exhibited obvious wrinkling (Figure 4B). These results suggested that subinhibitory concentrations of glabridin did not alter L. monocytogenes biofilms.
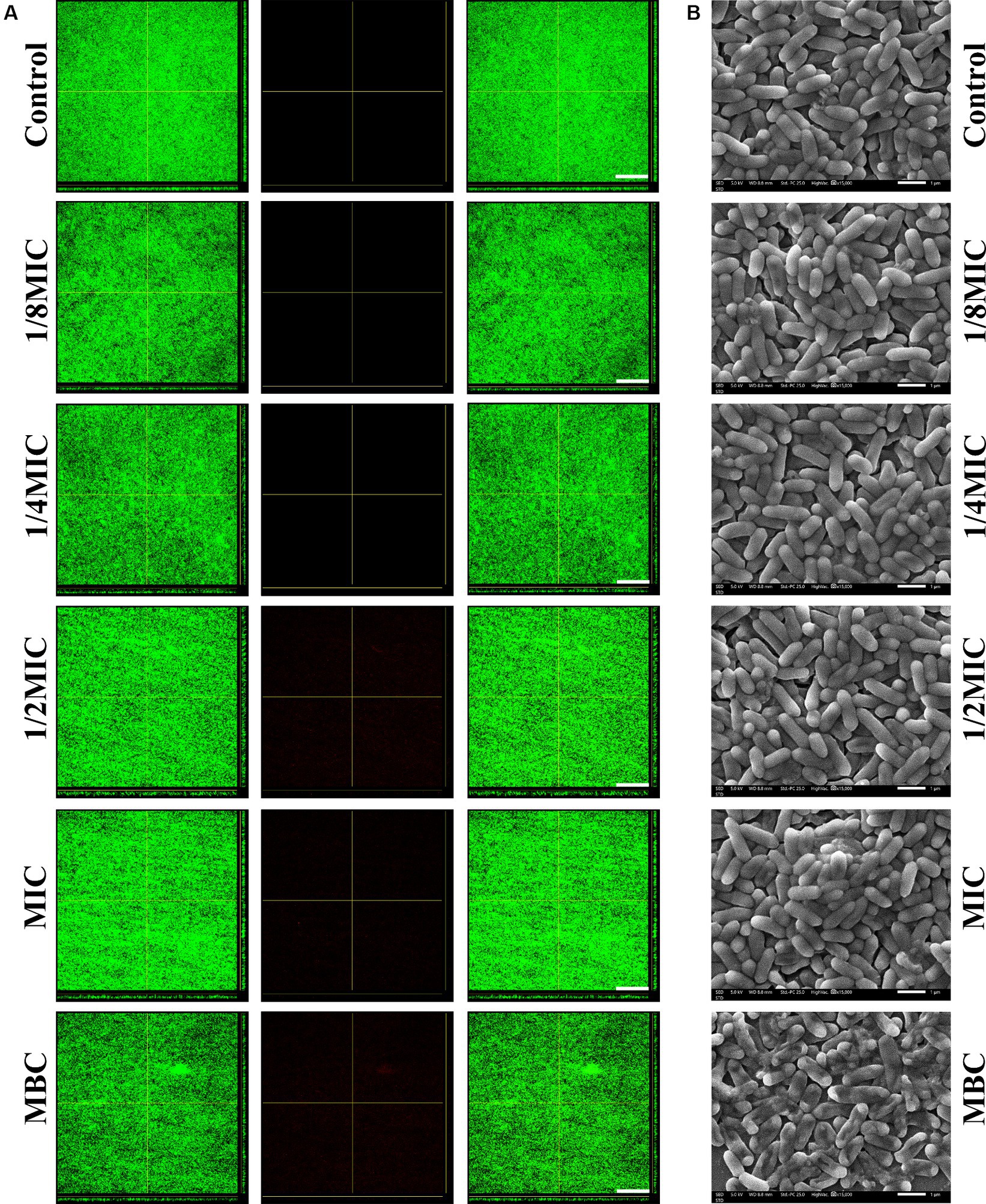
Figure 4. Eradication of L. monocytogenes mature biofilms in the presence of subinhibitory concentrations (1/8, 1/4, or 1/2 of the MIC) of glabridin. (A) Confocal laser scanning microscopy examination for eradication of L. monocytogenes mature biofilms by glabridin. The 48 h mature biofilms on cover slide were cultured with or without subinhibitory concentrations (1/8, 1/4, or 1/2 of the MIC), the MIC or MBC of glabridin, then examined by confocal laser scanning microscopy. Scale bar: 200 μm. (B) Scanning electron microscopy examination for eradication of L. monocytogenes mature biofilms by glabridin. The 48 h mature biofilms on cover slide were cultured with or without subinhibitory concentrations (1/8, 1/4, or 1/2 of the MIC), the MIC or MBC of glabridin, then fixed and examined by scanning electron microscopy. Scale bar: 1 μm.
3.6 Subinhibitory concentrations of glabridin decrease the hemolytic activity of L. monocytogenes
A bacterial plate assay was used to determine hemolytic activity. As shown in Figure 5A, L. monocytogenes 10403S without glabridin treatment exhibited hemolytic activity on blood agar plates. No difference in the hemolytic ability was observed between untreated L. monocytogenes and L. monocytogenes treated with glabridin at 1/8 of the MIC. However, none of the L. monocytogenes strains cultured in the presence of glabridin at 1/4 or 1/2 of the MIC showed hemolytic activity (Figure 5A). The microdilution method also indicated that the addition of glabridin at 1/4 or 1/2 of the MIC resulted in a dramatic decrease in hemolytic activity (Figure 5B). Moreover, the expression of the hly gene was significantly higher in L. monocytogenes treated with glabridin at 1/4 or 1/2 of the MIC than in untreated L. monocytogenes. The expression of the hly gene in L. monocytogenes treated with glabridin at 1/4 or 1/2 of the MIC decreased significantly by more than 5-fold compared with that in L. monocytogenes treated with glabridin at 1/8 of the MIC (p < 0.001), as shown in Figure 5C. Therefore, these results clearly showed that subinhibitory concentrations of glabridin decreased the hemolytic activity of L. monocytogenes.
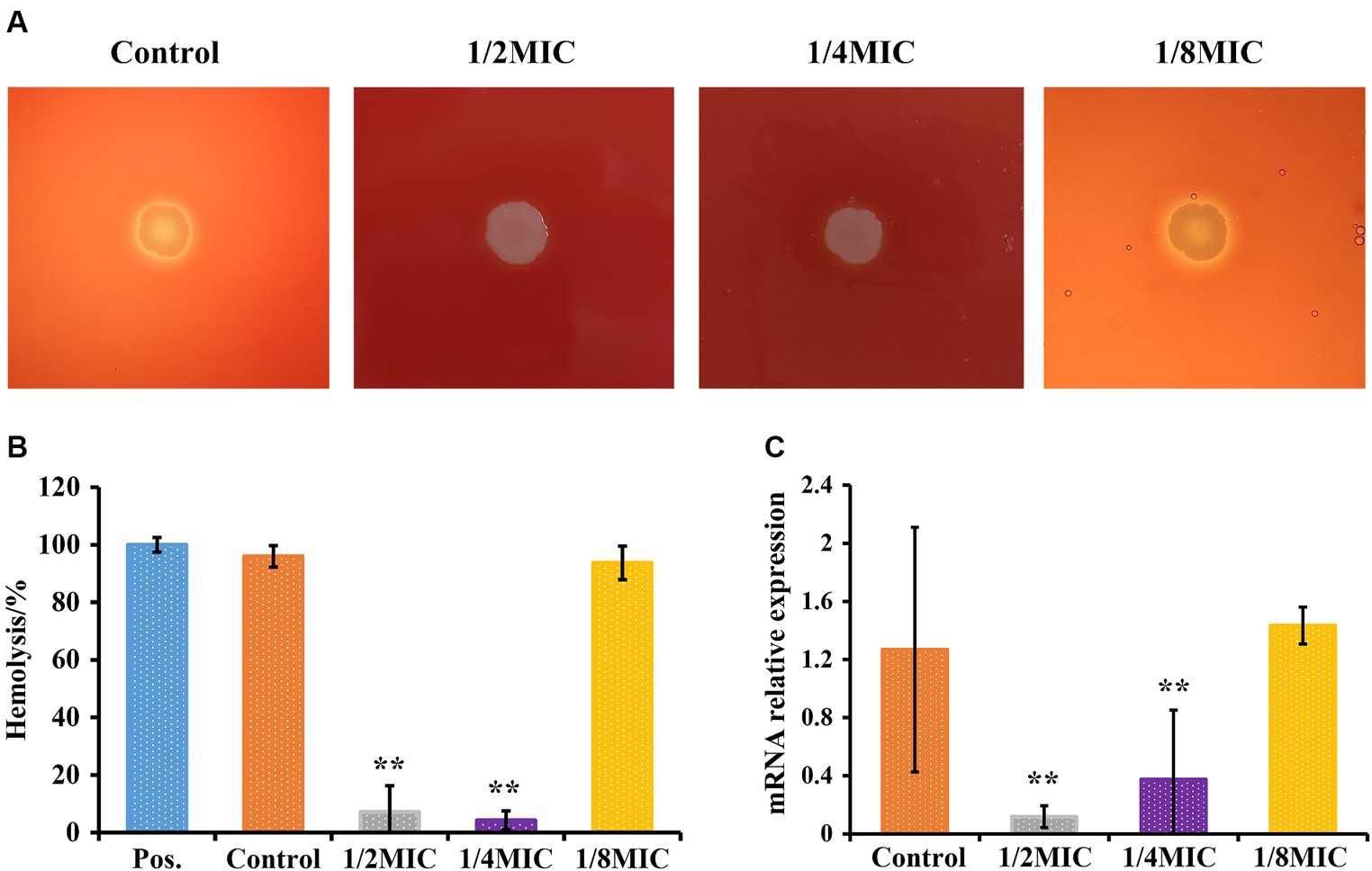
Figure 5. Effect of glabridin at subinhibitory concentrations on the hemolytic activity of L. monocytogenes. (A) The hemolytic activity of L. monocytogenes was analyzed using blood agar plates. L. monocytogenes was point inoculated onto BHI agar plates supplemented with 5.0% of sterile sheep blood and subinhibitory concentrations (1/8, 1/4, or 1/2 of the MIC) of glabridin. (B) The hemolytic activity of L. monocytogenes was analyzed by performing a microdilution method. L. monocytogenes were cultured at 37°C for 12 h exposure to subinhibitory concentrations (1/8, 1/4, or 1/2 of the MIC) of glabridin. Filtered supernatants were added at a 1:1 ratio to a suspension of red blood cells, followed by incubation for 1 h at 37°C. The optical density at 540 nm was measured. Triton X-100 was used as the positive control (Pos.). (C) Expression level of the hly gene in L. monocytogenes by RT-PCR. Each group was independently repeated three times. L. monocytogenes were cultured and exposed to subinhibitory concentrations (1/8, 1/4, or 1/2 of the MIC) of glabridin at 12 h of incubation at 37°C. mRNA was extracted. The abundances of the hly transcripts were quantified. Data were normalized to the 16S rRNA levels. The experiments were repeated three times. Statistical analysis was carried out using a Student’s t-test. **p < 0.01 (p < 0.05 or less was regarded as statistically significant).
3.7 Subinhibitory concentrations of glabridin are not toxic to Caco-2 and RAW264.7 cells
The cytotoxic effects of subinhibitory concentrations of glabridin on epithelial cells and macrophages were investigated via the LDH method following 6 h of treatment. As shown in Figure 6, different concentrations of glabridin (1/8, 1/4, or 1/2 of the MIC) had no toxic effect on Caco-2 and RAW264.7 cells (Figure 6). The results showed that subinhibitory concentrations of glabridin did not induce the release of LDH from Caco-2 and RAW264.7 cells. Concentrations of 1/8, 1/4, or 1/2 of the MIC were used for additional cell studies.
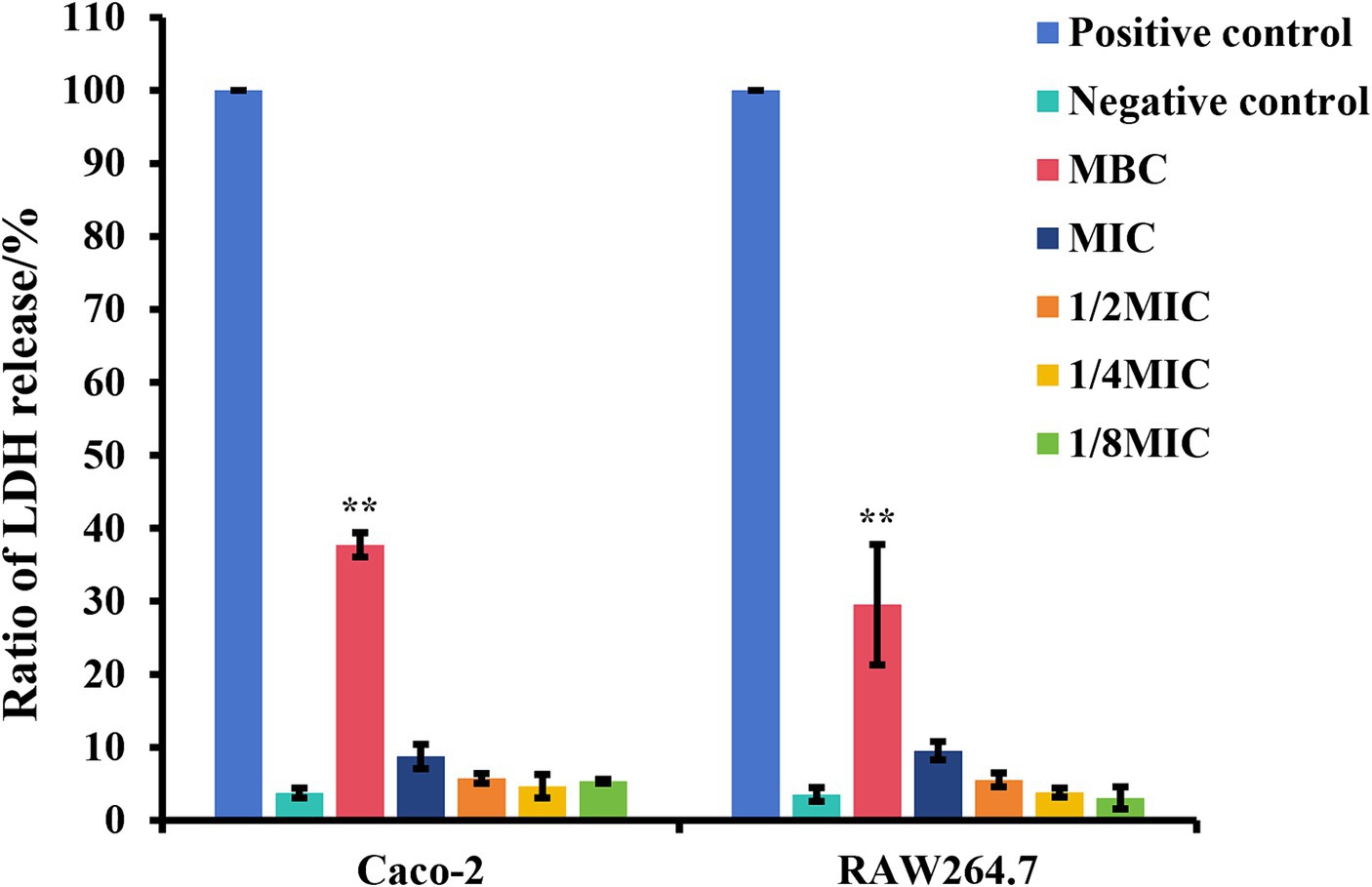
Figure 6. The ratio of lactate dehydrogenase (LDH) release of Caco-2 and RAW264.7 cells in the presence of glabridin at subinhibitory concentrations. Cytotoxicity was determined by the LDH method following a 6 h incubation with subinhibitory concentrations (1/8, 1/4, or 1/2 of the MIC), the MIC or MBC of glabridin. 0.2% Triton X-100 or DMEM only was used as the positive control and negative control, respectively. The experiments were repeated three times.
3.8 Subinhibitory concentrations of glabridin decrease the intracellular growth of L. monocytogenes in RAW264.7 cells
Gentamicin survival assays were performed to evaluate the influence of glabridin at subinhibitory concentrations on the intracellular growth of L. monocytogenes in RAW264.7 cells, as previously described. The results of the intracellular growth assay are illustrated in Supplementary Figure S3. After exposure to subinhibitory concentrations of glabridin at 1/4 or 1/2 of the MIC, the number of intracellular bacteria in RAW264.7 cells was much lower than that in the glabridin-untreated L. monocytogenes (Supplementary Figure S3). Glabridin inhibited the intracellular growth of L. monocytogenes in a concentration-dependent manner. However, treatment with glabridin at 1/8 of the MIC did not affect the intracellular growth of L. monocytogenes in RAW264.7 cells. This result suggested that subinhibitory concentrations of glabridin could improve L. monocytogenes clearance in RAW264.7 cells.
3.9 Effect of subinhibitory concentrations of glabridin on the ability of L. monocytogenes to trigger MET formation
We incubated RAW264.7 cells with glabridin (at 1/8, 1/4, or 1/2 of the MIC) for 2 h to examine the effect of glabridin on MET formation in macrophages. The fluorescence imaging analysis revealed that compared with the control treatment, treatment with PMA, an inducer of METs, could stimulate macrophages to release METs (Figure 7). The untreated macrophages did not release extracellular DNA. However, treatment with glabridin (1/8, 1/4, or 1/2 of the MIC) did not induce MET formation in macrophages (Figure 7). However, the presence of glabridin at the MBC significantly induced the release of extracellular DNA from macrophages (Supplementary Figure S4). In addition, we also observed intracellular ROS generation in macrophages exposure to glabridin (1/8, 1/4, or 1/2 of the MIC) (Figure 7). No significant difference in ROS production was detected among the glabridin treatments.
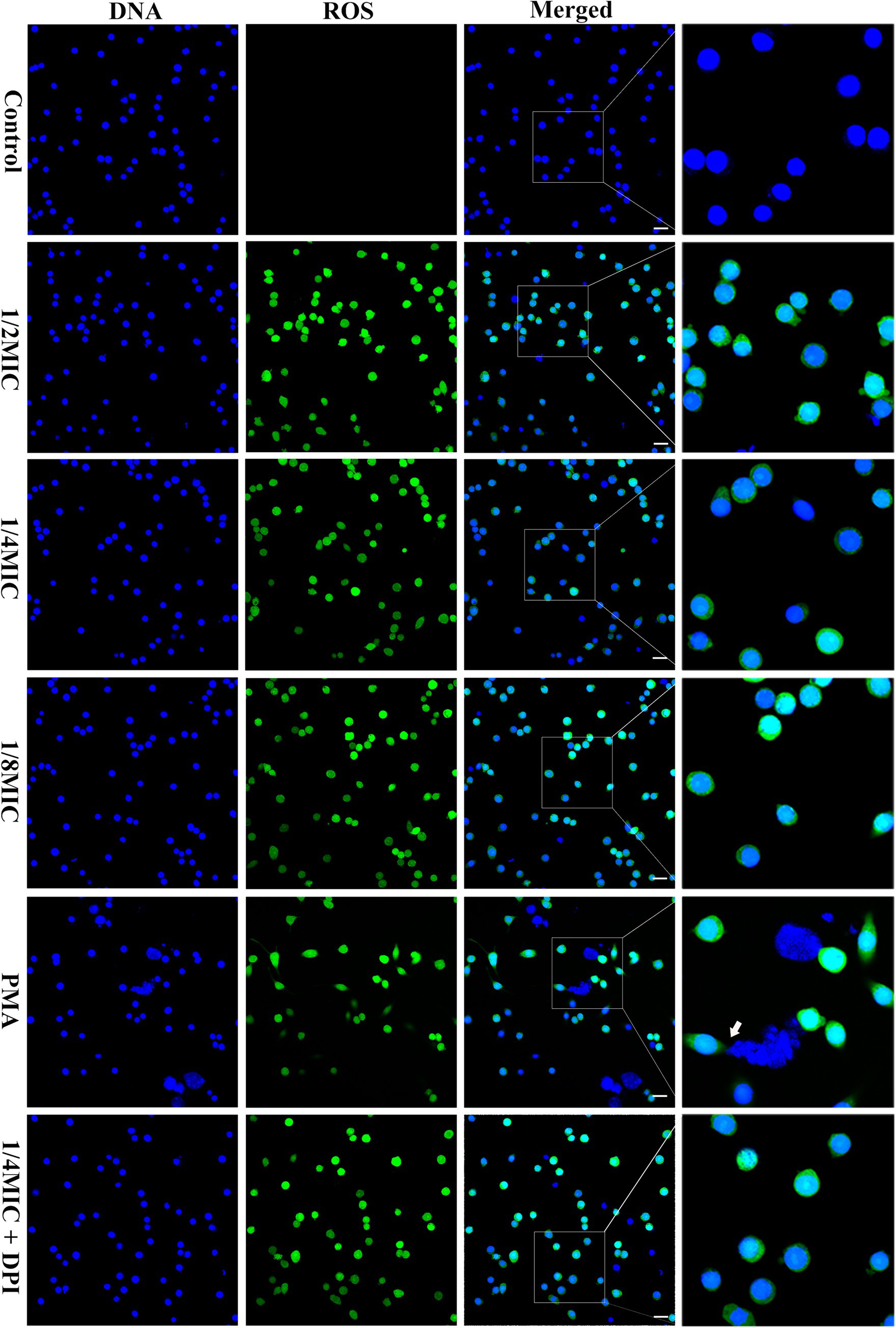
Figure 7. Glabridin and L. monocytogenes induced METs and intracellular ROS in RAW264.7 cells. RAW264.7 cells were infected with L. monocytogenes (MOI 10) and glabridin. After incubation for 2 h, Hoechst 33342 was added to stain the extracellular DNA. MET formation was observed using confocal laser scanning microscopy. PMA was used as the only positive control. Intracellular ROS levels in RAW264.7 cells were observed with DCFH-DA staining. The experiments were repeated three times. Scale bars: 20 μm. The white arrow represents METs.
Next, we asked ourselves whether subinhibitory concentrations of glabridin would have any effect on the ability of L. monocytogenes-stimulated macrophages to release METs. For this experiment, we incubated RAW264.7 cells with L. monocytogenes treated with glabridin for 2 h. Figure 8 shows that treatment with L. monocytogenes alone induced MET release from macrophages (Figure 8A). A similar phenomenon was also observed in which L. monocytogenes induced the release of extracellular reticulation structures after exposure to glabridin; however, the extent of MET formation was not significantly different from that of L. monocytogenes alone (Figure 8B). Based on these results, we showed that subinhibitory concentrations of glabridin did not affect the L. monocytogenes-induced MET release from macrophages.
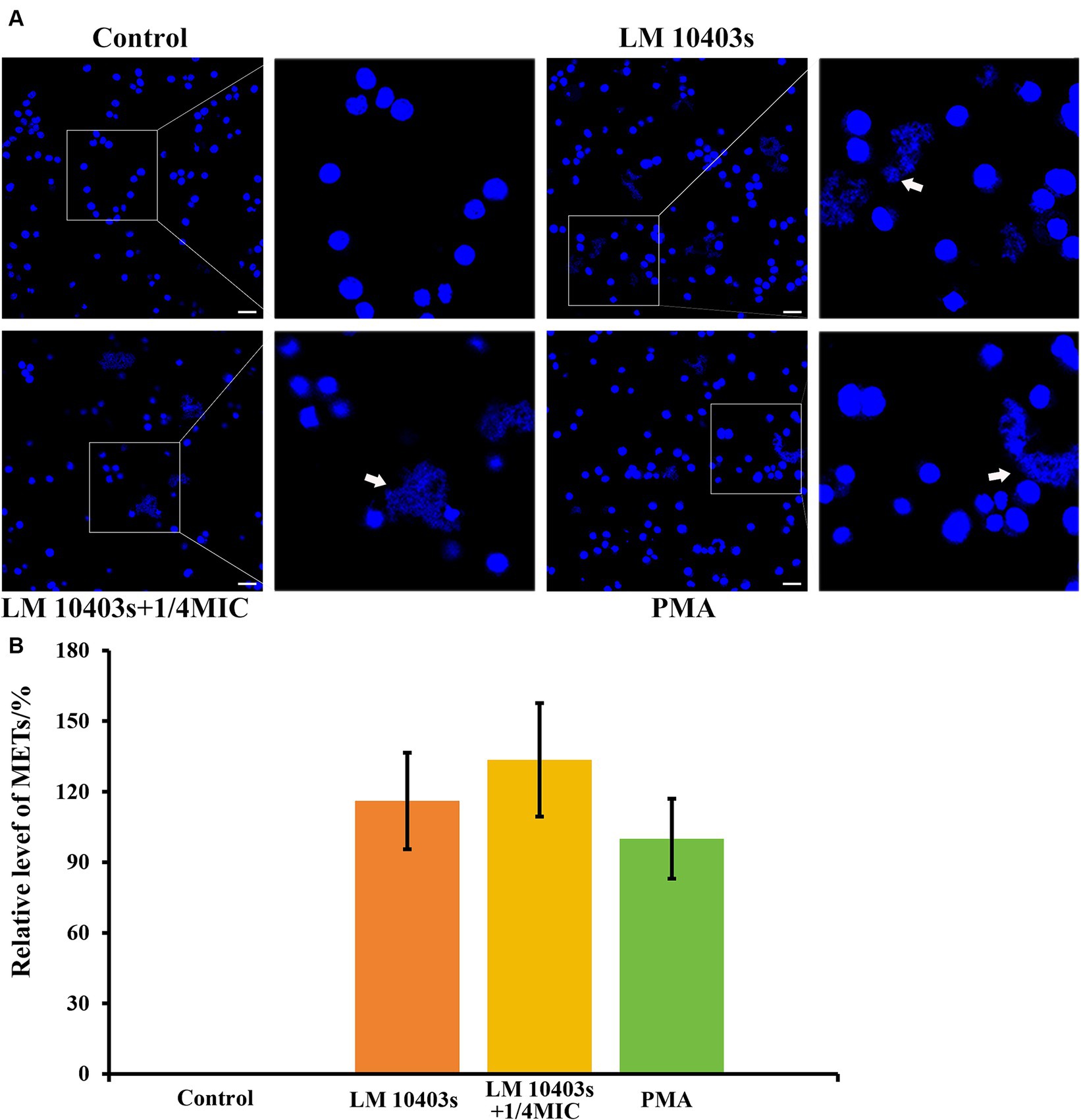
Figure 8. Effect of glabridin at subinhibitory concentrations on L. monocytogenes induced METs in RAW264.7 cells. RAW264.7 cells were infected with L. monocytogenes (MOI 10) and glabridin. After incubation for 2 h, Hoechst 33342 was added to stain the extracellular DNA. (A) MET formation was observed using confocal laser scanning microscopy. (B) Quantification of the MET formation by counting the percentage of RAW264.7 cells with METs. PMA was used as the only positive control. The MET formation in the PMA-treated macrophages was set as 100%. Data are represented as percentage of the PMA-treated group. The experiments were repeated three times. Scale bars: 20 μm. The white arrow represents METs.
3.10 Effect of subinhibitory concentrations of glabridin on the MET-mediated killing of extracellular bacteria by macrophages
The ET-mediated extracellular bactericidal effect is a novel defense mechanism of innate immune cells. L. monocytogenes was co-incubated with RAW264.7 cells in the presence of glabridin to determine whether subinhibitory concentrations of glabridin contribute to MET-mediated killing of extracellular bacteria by macrophages. We found that after a co-incubation with RAW264.7 cells and L. monocytogenes at 37°C for 2 h, L. monocytogenes was killed by macrophages compared with the control group (Figure 9). Efficient bacterial killing by macrophages was observed after the addition of glabridin. Cytochalasin D (10 μg/mL) was used to block phagocytosis to assess the effect of glabridin on extracellular L. monocytogenes clearance by METs. The results showed that the survival of L. monocytogenes treated with 1/4 of the MIC glabridin was similar to that of L. monocytogenes not treated with glabridin (Figure 9). This result showed that glabridin had no effect on the ability of macrophages to kill L. monocytogenes via METs.
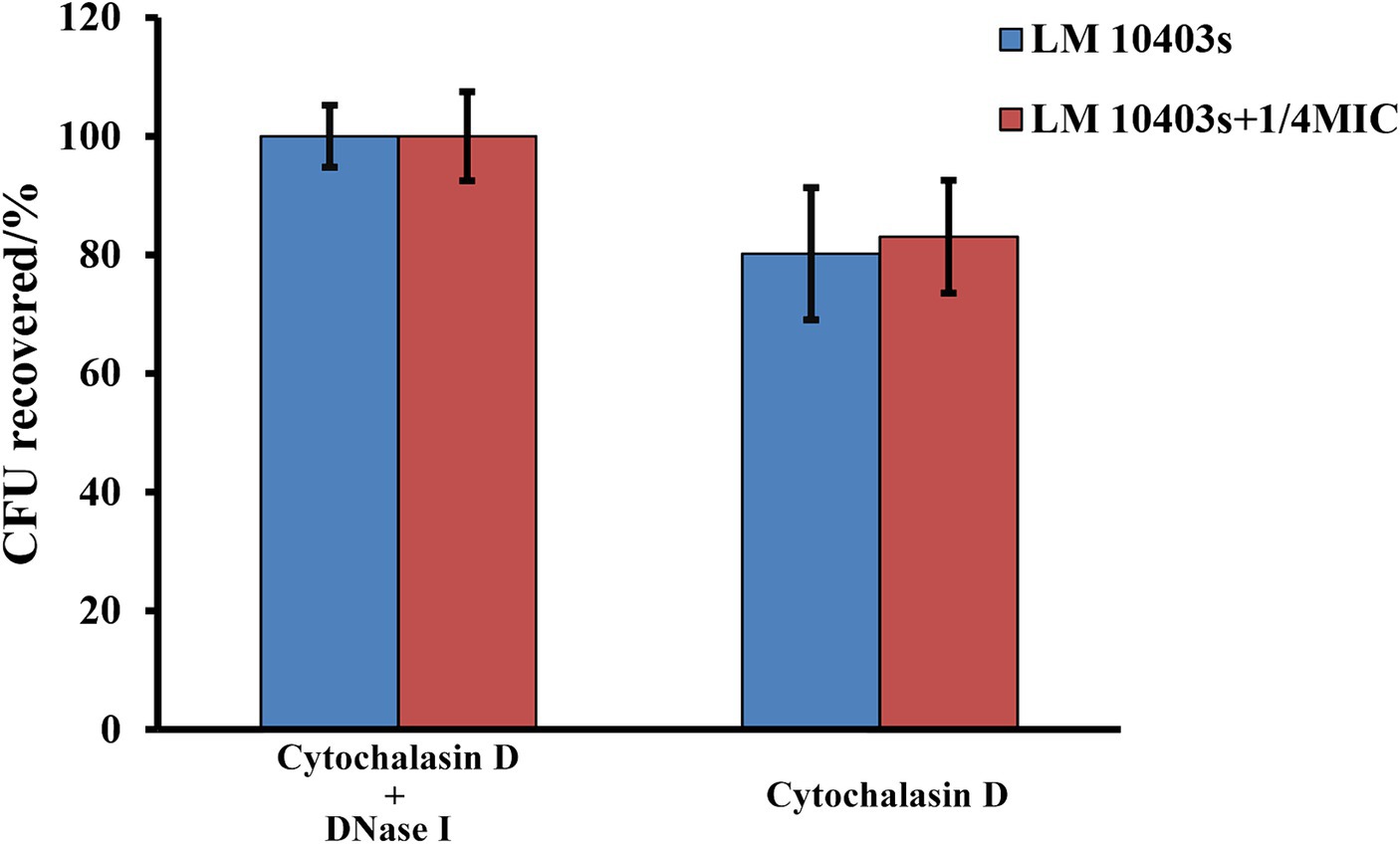
Figure 9. Effect of glabridin at subinhibitory concentrations on MET-mediated extracellular killing of macrophages against L. monocytogenes. RAW264.7 cells infected by L. monocytogenes were treated with subinhibitory concentrations of glabridin. Cytochalasin D and DNase I were added to inhibit phagocytosis and MET formation. The number of viable bacteria in the RAW264.7 cells was determined by CFU quantification. Pretreatment with cytochalasin D and DNase I was used as the 100% survival control group. The experiments were repeated three times.
4 Discussion
Research on the function of traditional Chinese medicine has become one of the hotspots in the research and development of antibacterial drugs. Licorice is a medicinal herb with a 2000-year history of application in traditional Chinese medicine. The most studied and documented benefits of glabridin from licorice are its anti-inflammatory properties, and its antibacterial activities have also been described, albeit less frequently, in the reported literature (Simmler et al., 2013; Ji et al., 2024). L. monocytogenes is a dangerous foodborne pathogen that poses a serious threat to public health and causes substantial economic losses for society. Subinhibitory concentrations of antibiotics are known to elicit multiple responses in bacteria. This study was performed to determine the effect of subinhibitory concentrations of glabridin on L. monocytogenes.
According to previous reports, the MICs of glabridin against pathogens vary from 1.562 to 31.3 μg/mL (Okada et al., 1989; Simmler et al., 2013; Zhang J. et al., 2023). The pathogens tested were Enterococcus faecalis, Fusobacterium nucleatum, Bacillus subtilis, Staphylococcus aureus (S. aureus), Streptococcus mutans (S. mutans), Porphyromonas gingivalis (P. gingivalis), P. endodontalis, Prevotella intermedia, Mycobacterium smegmatis, Candida albicans (C. albicans), C. glabrata, C. utilis, Fusarium graminearum, Saccharomyces cerevisiae, Mucor pusillus, Aspergillus niger, Eimeria tenella, and Corynespora cassiicola (Simmler et al., 2013; Zhang J. et al., 2023). Although the antimicrobial spectrum of glabridin in vitro has been relatively little studied, the active substances can promote non-specific immune function and enhance cellular immunity (Chirumbolo, 2016), which is then able to exert an anti-inflammatory effect on pathogen infection. The anti-inflammatory effects of glabridin have also been evaluated in vitro in microglia, promyelocytic cells, and dendritic cells, as well as in vivo in mice (Simmler et al., 2013). Glabridin ameliorates dextran sulfate sodium-induced colitis in mice by reducing colonic myeloperoxidase activity and the production of inflammatory mediators, such as nitric oxide, prostaglandin E2, and proinflammatory cytokines (Kwon et al., 2008).
In the present study, glabridin from Xinjiang licorice exhibited antimicrobial activity against L. monocytogenes, and the MIC and MBC were 31.25 μg/mL and 125 μg/mL, respectively. Okada et al. (1989) reported that glabridin had no antibacterial activity against the gram-negative bacteria, E. coli or Pseudomonas aeruginosa. However, treatment with glabridin at the MIC (12.5 μg/mL) exhibits antibacterial activity against Helicobacter pylori by inhibiting protein synthesis, DNA gyrase, and dihydrofolate reductase (Asha et al., 2013). Bombelli et al. (2023) reported that the MIC and MBC of glabridin against L. monocytogenes EGDe are 12.5 and 25 μg/mL, respectively. They also confirmed that temperature, pH, and oxygen were factors that influenced the antibacterial activity of glabridin against L. monocytogenes. Overall, glabridin exhibits only weak and non-specific antibiotic properties, and the antimicrobial properties of glabridin are mainly bacteriostatic. In addition to this particular activity, the antimicrobial mechanism of glabridin is still unclear. Compared with the control, treatment with glabridin at 1/8, 1/4, or 1/2 of the MIC did not limit the growth of L. monocytogenes in BHI at any time point during the incubation. In fact, glabridin at the MBC was sufficient to stimulate the disruption of the membrane integrity of L. monocytogenes (Bombelli et al., 2024). We then established subinhibitory concentrations of 1/8, 1/4, or 1/2 of the MIC of glabridin (i.e., 3.91, 7.81, and 15.63 μg/mL, respectively) that were used in subsequent experiments.
Previous studies have shown that swarming, swimming, twitching, gliding, and sliding are modes of bacterial movement (Kearns, 2010; Zegadlo et al., 2023). Swimming and swarming motility are two active forms of movement found in most bacteria, as observed in L. monocytogenes (Borges et al., 2012). In our study, we found a decrease in the swimming and swarming motility of L. monocytogenes in the presence of glabridin. Previous studies have shown that flagella are important drivers of bacterial motility. Interestingly, our study revealed that the number of flagella of L. monocytogenes did not change significantly in the presence of subinhibitory concentrations of glabridin. Therefore, from the TEM results, the reduced swimming and swarming motility were clearly not related to the flagellar production in L. monocytogenes cultured in the presence of glabridin. This result can be attributed to the loss of functionality of the flagellum (Inamuco et al., 2012). Swarming motility refers to multicellular surface movement in a fixed direction on a semisolid surface by the rotation of flagella. Swimming motility refers to individual movement in a fixed direction on a semisolid medium in aqueous environments by the rotation of flagella (Kearns, 2010; Wu et al., 2022). However, the mechanism by which glabridin affects swimming and swarming motility but not flagellar production is unclear. As shown in Figure 2, the effect of glabridin on the swarming motility of L. monocytogenes was greater than that on the swimming motility. This result may be related to the fact that bacterial swarming motility consumes more energy for flagellar activity than swimming motility (Poudel et al., 2019). However, extracellular ATP levels in L. monocytogenes were slightly decreased in the presence of glabridin, but the difference was not statistically significant. Furthermore, subinhibitory concentrations of antimicrobial agents reduce microbial pathogenicity by inhibiting the production of virulence factors because toxin secretion is usually co-regulated by swarming motility (Kearns, 2010). However, more experimental work is needed in order to support such claims.
Biofilms are complex surface-attached communities composed of extracellular polymeric substances (Muhammad et al., 2020). Most bacteria naturally grow in biofilms, which render the infection difficult to treat due to their high resistance to antibiotics and host immunity. Compared with their planktonic counterparts, bacteria in biofilms present up to a 1,000-fold increase in resistance to antimicrobial agents (Summer et al., 2022). Approximately 60% of foodborne illness outbreaks and 80% of human infections are likely related to microbial biofilms (Zafer et al., 2024). Biofilms could be promising therapeutic targets for the treatment of pathogenic bacterial infections. L. monocytogenes has a high capacity for biofilm formation on food and food-processing surfaces in food-processing environments. Plant-derived antimicrobial substances are generally considered safe, effective, and environmentally friendly for the prevention of foodborne diseases. These compounds have a negative effect on the biofilm formation of L. monocytogenes. Gallic acid treatment (at 5.8 mM) and resveratrol treatment (0.2–0.8 mM) reduced the overall biomass of L. monocytogenes biofilms (Ferreira and Domingues, 2016). Previous studies have shown that the minimum biofilm eradication concentrations of glabridin were 25, 50, and 25 μg/mL for S. mutans, S. aureus, and P. gingivalis biofilms, respectively (Tsukatani et al., 2022), but this effect has not been reported for L. monocytogenes. In our study, we found that 125 μg/mL glabridin exhibited a poor ability to eradicate L. monocytogenes biofilms. Furthermore, the spectrum of antibiofilm activities of glabridin has been described in relatively few articles. The formation of MRSA 4423 biofilms was completely reduced in the presence of glabridin at 6.25 μg/mL (1/2 of the MIC) (Gangwar et al., 2020). Moreover, 12 μg/mL (MIC) significantly inhibited the formation of Aspergillus fumigatus biofilms (Gao H. et al., 2022), and 12.5 μg/mL (2× MIC) significantly reduced the viability of S. mutans biofilms following a 2 h treatment (Vaillancourt et al., 2021). At 25 μg/mL, 11.2% of E. faecalis embedded in a biofilm was killed following 15 min of contact (Marcoux et al., 2020), whereas at 1/4 of the MIC (3.125 μg/mL), biofilm formation was not attenuated after 30 min of contact (Grenier et al., 2020). Moreover, 1/4 of the MIC (128 μg/mL) and 1/2 of the MIC significantly decreased biofilm formation by multidrug-resistant Acinetobacter baumannii by 19.98 and 27.43%, respectively (Lin et al., 2023).
Antibiotics have been shown to decrease the abundance of microorganisms in biofilms. Subinhibitory concentrations of allicin have been shown to inhibit the formation of C. albicans, S. epidermidis, and uropathogenic E. coli biofilms (Yang et al., 2016). However, exposure to subinhibitory concentrations of antibiotics has been found to induce biofilm formation in several pathogenic species (Jolivet-Gougeon and Bonnaure-Mallet, 2014). The MIC for Clostridioides difficile (C. difficile) increased following exposure to subinhibitory concentrations of metronidazole. Doan et al. (2022) also reported that the production of C. difficile biofilms was induced by exposure to subinhibitory concentrations of metronidazole. He et al. (2022) reported that subinhibitory concentrations of ethanol (1/4 of the MIC, 2.5%; 1/2 of the MIC, 5.0%) were able to stimulate biofilm formation in Salmonella enteritidis. Park et al. (2020) documented that subinhibitory concentrations of chlorhexidine and mupirocin promoted biofilm formation in the clinical methicillin-resistant S. aureus isolates. Accordingly, this study evaluated the effect of subinhibitory concentrations of glabridin on L. monocytogenes. At 1/8, 1/4, or 1/2 of the MIC, glabridin had no effect on biofilm formation by L. monocytogenes. Flagella are very important for biofilm formation in several bacterial species. As an adhesive structure, flagella participate in the initial phase of L. monocytogenes biofilm formation (Vatanyoopaisarn et al., 2000). In this study, the flagella of L. monocytogenes did not change upon exposure to subinhibitory concentrations of glabridin. In addition, exposure to subinhibitory concentrations of antibiotics can stimulate the formation of factors such as toxins and adhesins (Gao P. et al., 2022).
Listeriolysin O (LLO), encoded by the hly gene, is recognized as a Listeria-specific hemolysin. Screening natural compounds as inhibitors of LLO is a hot topic in the research of antimicrobial agents against L. monocytogenes infection. Fisetin, a natural flavonoid from vegetables and fruits, significantly decreases the hemolytic activity of an L. monocytogenes culture supernatant (Wang et al., 2015). Glabridin is an isoflavan isolated from licorice root extract. Consistent with previous studies, our results indicate that glabridin was more effective at inhibiting the hemolytic activity of L. monocytogenes. Jiang et al. (2022) reported that the hemolytic activity of L. monocytogenes 10403S was almost completely inhibited upon exposure to 1/4 of the MIC of phenyllactic acid. Previous studies have shown that LLO is an essential virulence determinant for L. monocytogenes to escape from phagosomes (Nguyen et al., 2019). In our study, we analyzed the expression of the hly gene in L. monocytogenes exposed to glabridin using qRT-PCR. The qRT-PCR results revealed that the expression of the hly gene in L. monocytogenes treated with glabridin at 1/2 and 1/of the MIC was reduced significantly by 2.9- and 2.4-fold, respectively, compared to that in the untreated L. monocytogenes. Similarly, LLO secretion by L. monocytogenes 10403S was reduced by subinhibitory concentrations of phenyllactic acid (Jiang et al., 2022). In addition, Hou et al. (2023) reported that a cinnamon twig extract can effectively inhibit the hemolysis of LLO without inhibiting the growth of L. monocytogenes.
Pathogenic bacterial adhesion to host cell surfaces is the first and crucial step for the successful establishment of survival and replication during infection (Birhanu et al., 2018). However, the presence of glabridin at subinhibitory concentrations did not alter the adherence of L. monocytogenes to epithelial cells in this study (Supplementary Figure S5). Although the primary function of flagella is not to mediate adhesion, an impaired motility function conferred by flagella results in the reduced adhesion of pathogens (Zhai et al., 2024). One possibility is that the subinhibitory concentration of glabridin did not damage the flagella production in L. monocytogenes. The survival and replication of cells are well established as important virulence factors for pathogens. Macrophages play an important role in anti-inflammatory processes and pathogen clearance and serve as a crucial role in shaping both innate and adaptive immune responses. Rosenblat et al. (1999) confirmed that an incubation of macrophages (J774.1A) with glabridin (20 μM) for 20 h at 37°C was not cytotoxic to the cells. Consistent with this finding, we showed that subinhibitory concentrations (1/8, 1/4, or 1/2 of the MIC) of glabridin had no toxic effect on RAW264.7 cells in the present study. Notably, we observed that glabridin inhibited the intracellular growth of L. monocytogenes in a concentration-dependent manner. A previous study by Rosenblat’s laboratory showed that 60 and 40% of the glabridin was localized in the macrophage membrane and the cytosol, respectively (Rosenblat et al., 1999). Although subinhibitory concentrations of glabridin did not directly affect the growth rate of L. monocytogenes, these concentrations were more effective at inhibiting the hemolytic activity of L. monocytogenes. Hemolysin is responsible for the lysis of macrophage lysosomes (Okunnu and Berg, 2019). LLO produced by L. monocytogenes leads to lysis of the phagosomal membrane in macrophages, subsequently allowing L. monocytogenes to escape into the cytoplasm, where it is able to replicate and participate in cell–cell spread (Portnoy et al., 1988; Gedde et al., 2000; Nguyen et al., 2019). Moreover, glabridin has a notably distinctive mechanism of inhibiting the inflammatory response (Shin et al., 2023). Shin et al. (2023) reported that synthetic glabridin derivatives exert strong anti-inflammatory effects on LPS-induced RAW264.7 cells by inhibiting the MAPK and NF-κB pathways. Kang et al. (2005) documented that glabridin inhibits NO production and iNOS gene expression by blocking NF-κB/Rel activation in RAW264.7 cells. Glabridin reverses changes in amino acid, energy, and lipid metabolism during the LPS-induced inflammatory process in RAW264.7 cells (Liu et al., 2017). In addition, tiliroside, a flavonoid present in edible plants, may inhibit a major signaling pathway upstream of glycolytic enzymes (Zhuang et al., 2021). These effects may be beneficial for reducing macrophage damage and subsequent bacterial dissemination.
ETs are composed of extracellular DNA supplemented with various protein substances and have been shown to be a unique defense strategy for the immune system (Liao et al., 2022). Vertebrate innate immune cells, including neutrophils, mast cells, eosinophils, and macrophages, are capable of forming ETs, as are invertebrate and plant cells. Previous studies have shown that various pathogens and their products and chemical reagents can stimulate immune cells to release METs (Cubillo-Martinez et al., 2022). In our present study, we observed that subinhibitory concentrations of glabridin did not trigger extracellular DNA release from RAW264.7 cells. In fact, glabridin at the MBC was sufficient to stimulate macrophages to release METs. NADPH oxidase-dependent ROS production is thought to be important for ET formation (Azzouz and Palaniyar, 2022). Several studies have shown that the NADPH oxidase-independent pathway is another key mechanism of NET formation based on the death of neutrophils (Khan and Palaniyar, 2017). Here, we found that glabridin-induced ROS production was similar to that induced by PMA, and ROS production was detected in the presence or absence of DPI (an NADPH oxidase inhibitor).
L. monocytogenes has been shown to trigger the formation of METs in macrophages (Cheng et al., 2015). Next, we examined the effect of glabridin on MET formation induced by L. monocytogenes. The amount of METs formed by macrophages infected with L. monocytogenes was not altered by the addition of glabridin. No obvious synergistic effects of glabridin and L. monocytogenes on enhancing MET formation were detected. The mechanisms by which glabridin works positively or negatively on NADPH oxidase and ROS have not yet been clearly described. Rosenblat et al. (1999) reported that glabridin has no direct inhibitory effect on the active NADPH oxidase; however, it can inhibit the cellular processes that lead to NADPH oxidase activation. Glabridin attenuates ROS production induced by 2-deoxy-D-ribose in the mouse osteoblastic cell line MC3T3-E1 (Kim et al., 2013), UVB-irradiation of human keratinocytes, and NaIO3 in retinal pigment epithelial ARPE-19 cells (Veratti et al., 2011). Thus, glabridin prevents direct and indirect DNA damage to avoid activating cell death pathways (Aung et al., 2020). ETosis is a form of inflammatory cell death that occurs following ET formation in immune cells. However, Zhang et al. (2022) reported that intracellular ROS levels were dose-dependently increased in the prostate cancer cell lines DU-145 and LNCaP after glabridin treatment. ETs are considered a novel bactericidal mechanism that differ from phagocytosis (Arabi et al., 2023). However, glabridin did not affect the killing of extracellular bacteria by macrophages via METs, as no obvious synergistic effect on MET formation induced by L. monocytogenes was detected.
5 Conclusion
In summary, we found that subinhibitory concentrations of glabridin did not affect the growth, morphology, flagellar production, or biofilm formation of L. monocytogenes but inhibited motility and decreased hemolytic activity. At subinhibitory concentrations, glabridin did not affect the ability of L. monocytogenes adhere to Caco-2 cells but decreased its intracellular growth in RAW264.7 cells. Glabridin induced ROS production in macrophages but did not affect MET formation, the capacity of L. monocytogenes to trigger METs, or extracellular killing by METs. These findings provide valuable insights into the further application of glabridin as an alternative antimicrobial agent to inhibit L. monocytogenes.
Data availability statement
The raw data supporting the conclusions of this article will be made available by the authors, without undue reservation.
Ethics statement
Ethical approval was not required for the studies on humans in accordance with the local legislation and institutional requirements because only commercially available established cell lines were used. Ethical approval was not required for the studies on animals in accordance with the local legislation and institutional requirements because only commercially available established cell lines were used.
Author contributions
CL: Conceptualization, Methodology, Investigation, Writing – original draft, Writing – review & editing, Project administration, Funding acquisition. CY: Data curation, Methodology, Writing – review & editing. JG: Methodology, Writing – review & editing. MG: Methodology, Writing – review & editing.
Funding
The author(s) declare that financial support was received for the research, authorship, and/or publication of this article. This work was supported by the National Natural Science Foundation of China (32373015), National Natural Science Foundation of Henan Province (242300421107), Key Scientific Research Projects of Colleges and Universities in Henan Province (2023GGJS049), Science and Technology Research Project of Henan Province (222102110012), Youth Backbone Teachers Training Program of Henan Province (24A10015), and Youth Backbone Teachers Training Program of Henan University of Science and Technology (13450009).
Acknowledgments
We thank AJE for the language editing of the manuscript. We gratefully thank Junhui Niu, Jingzheng Hu, and Hanxiao Li for assisting in the biofilm formation and METs experiment.
Conflict of interest
The authors declare that the research was conducted in the absence of any commercial or financial relationships that could be construed as a potential conflict of interest.
Publisher’s note
All claims expressed in this article are solely those of the authors and do not necessarily represent those of their affiliated organizations, or those of the publisher, the editors and the reviewers. Any product that may be evaluated in this article, or claim that may be made by its manufacturer, is not guaranteed or endorsed by the publisher.
Supplementary material
The Supplementary material for this article can be found online at: https://www.frontiersin.org/articles/10.3389/fmicb.2024.1388388/full#supplementary-material
References
Adamus-Bialek, W., Kubiak, A., and Czerwonka, G. (2015). Analysis of uropathogenic Escherichia coli biofilm formation under different growth conditions. Acta Biochim. Pol. 62, 765–771. doi: 10.18388/abp.2015_1127
Arabi, T. Z., Fawzy, N. A., Abdul Rab, S., Alabdul Razzak, G., Sabbah, B. N., Alkattan, K., et al. (2023). NETs, infections, and antimicrobials: a complex interplay. Eur. Rev. Med. Pharmacol. Sci. 27, 9559–9568. doi: 10.26355/eurrev_202310_34129
Arslan, S., and Ozdemir, F. (2020). Prevalence and antimicrobial resistance of Listeria species and molecular characterization of Listeria monocytogenes isolated from retail ready-to-eat foods. FEMS Microbiol. Lett. 367:fnaa006. doi: 10.1093/femsle/fnaa006
Asha, M. K., Debraj, D., Prashanth, D., Edwin, J. R., Srikanth, H. S., Muruganantham, N., et al. (2013). In vitro anti-Helicobacter pylori activity of a flavonoid rich extract of Glycyrrhiza glabra and its probable mechanisms of action. J. Ethnopharmacol. 145, 581–586. doi: 10.1016/j.jep.2012.11.033
Aung, K. H., Liu, H., Ke, Z., Jiang, S., and Huang, J. (2020). Glabridin attenuates the retinal degeneration induced by sodium iodate in vitro and in vivo. Front. Pharmacol. 11:566699. doi: 10.3389/fphar.2020.566699
Azzouz, D., and Palaniyar, N. (2022). ROS and DNA repair in spontaneous versus agonist-induced NETosis: context matters. Front. Immunol. 13:1033815. doi: 10.3389/fimmu.2022.1033815
Bernardi, S., Anderson, A., Macchiarelli, G., Hellwig, E., Cieplik, F., Vach, K., et al. (2021). Subinhibitory antibiotic concentrations enhance biofilm formation of clinical Enterococcus faecalis isolates. Antibiotics 10:874. doi: 10.3390/antibiotics10070874
Birhanu, B. T., Park, N. H., Lee, S. J., Hossain, M. A., and Park, S. C. (2018). Inhibition of Salmonella Typhimurium adhesion, invasion, and intracellular survival via treatment with methyl gallate alone and in combination with marbofloxacin. Vet. Res. 49:101. doi: 10.1186/s13567-018-0597-8
Bombelli, A., Araya-Cloutier, C., Boeren, S., Vincken, J. P., Abee, T., and den Besten, H. M. W. (2024). Effects of the antimicrobial glabridin on membrane integrity and stress response activation in Listeria monocytogenes. Food Res. Int. 175:113687. doi: 10.1016/j.foodres.2023.113687
Bombelli, A., Araya-Cloutier, C., Vincken, J. P., Abee, T., and den Besten, H. M. W. (2023). Impact of food-relevant conditions and food matrix on the efficacy of prenylated isoflavonoids glabridin and 6,8-diprenylgenistein as potential natural preservatives against Listeria monocytogenes. Int. J. Food Microbiol. 390:110109. doi: 10.1016/j.ijfoodmicro.2023.110109
Borges, A., Saavedra, M. J., and Simoes, M. (2012). The activity of ferulic and gallic acids in biofilm prevention and control of pathogenic bacteria. Biofouling 28, 755–767. doi: 10.1080/08927014.2012.706751
Brinkmann, V., Goosmann, C., Kuhn, L. I., and Zychlinsky, A. (2012). Automatic quantification of in vitro NET formation. Front. Immunol. 3:413. doi: 10.3389/fimmu.2012.00413
Budvytyte, L., Schroeder, M., Graf, E., and Vaillant, J. J. (2024). Rapid progression of invasive Listeria monocytogenes infection in a patient with cirrhosis and primary Sclerosing cholangitis on Ustekinumab. Cureus 16:e58116. doi: 10.7759/cureus.58116
Cheng, W., Shi, X. C., Wang, C., An, Y. N., Zhang, Q. L., Jin, K. Q., et al. (2015). Macrophages catch and kill Listeria monocytogenes by producing extracellular traps. Chin. J. Vet. Sci. 35, 580–584. doi: 10.16303/j.cnki.1005-4545.2015.04.005
Chirumbolo, S. (2016). Commentary: the antiviral and antimicrobial activities of licorice, a widely-used Chinese herb. Front. Microbiol. 7:531. doi: 10.3389/fmicb.2016.00531
Cogen, A. L., Yamasaki, K., Muto, J., Sanchez, K. M., Crotty Alexander, L., Tanios, J., et al. (2010). Staphylococcus epidermidis antimicrobial delta-toxin (phenol-soluble modulin-gamma) cooperates with host antimicrobial peptides to kill group a Streptococcus. PLoS One 5:e8557. doi: 10.1371/journal.pone.0008557
Cubillo-Martinez, A. A., Pereyra, M. A., Garfias, Y., Guluarte, C., Zenteno, E., and Sanchez-Salgado, J. L. (2022). Extracellular traps involved in invertebrate immune mechanisms. Fish Shellfish Immunol. 121, 380–386. doi: 10.1016/j.fsi.2022.01.024
Djordjevic, D., Wiedmann, M., and McLandsborough, L. A. (2002). Microtiter plate assay for assessment of Listeria monocytogenes biofilm formation. Appl. Environ. Microbiol. 68, 2950–2958. doi: 10.1128/AEM.68.6.2950-2958.2002
Doan, T. H., Bernet-Camard, M. F., Hoys, S., Janoir, C., and Pechine, S. (2022). Impact of subinhibitory concentrations of metronidazole on morphology, motility, biofilm formation and colonization of Clostridioides difficile. Antibiotics 11:624. doi: 10.3390/antibiotics11050624
Fang, Z., Ban, L., Li, Y., Yuan, W., Liu, Z., Liu, T., et al. (2018). A quinoline-based FtsZ inhibitor for the study of antimicrobial activity and synergistic effects with beta-lactam antibiotics. J. Pharmacol. Sci. 137, 283–289. doi: 10.1016/j.jphs.2018.07.005
Ferreira, S., and Domingues, F. (2016). The antimicrobial action of resveratrol against Listeria monocytogenes in food-based models and its antibiofilm properties. J. Sci. Food Agric. 96, 4531–4535. doi: 10.1002/jsfa.7669
Fraser, K. R., Sue, D., Wiedmann, M., Boor, K., and O'Byrne, C. P. (2003). Role of sigmaB in regulating the compatible solute uptake systems of Listeria monocytogenes: osmotic induction of opuC is sigmaB dependent. Appl. Environ. Microbiol. 69, 2015–2022. doi: 10.1128/AEM.69.4.2015-2022.2003
Gangwar, B., Kumar, S., and Darokar, M. P. (2020). Glabridin averts biofilms formation in methicillin-resistant Staphylococcus aureus by modulation of the Surfaceome. Front. Microbiol. 11:1779. doi: 10.3389/fmicb.2020.01779
Gao, H., Peng, X., Zhan, L., Lin, J., Zhang, Y., Huan, Y., et al. (2022). The role of Glabridin in antifungal and anti-inflammation effects in aspergillus fumigatus keratitis. Exp. Eye Res. 214:108883. doi: 10.1016/j.exer.2021.108883
Gao, P., Wei, Y., Wan, R. E., Wong, K. W., Iu, H. T. V., Tai, S. S. C., et al. (2022). Subinhibitory concentrations of antibiotics exacerbate staphylococcal infection by inducing bacterial virulence. Microbiol. Spectr. 10:e0064022. doi: 10.1128/spectrum.00640-22
Gedde, M. M., Higgins, D. E., Tilney, L. G., and Portnoy, D. A. (2000). Role of listeriolysin O in cell-to-cell spread of Listeria monocytogenes. Infect. Immun. 68, 999–1003. doi: 10.1128/IAI.68.2.999-1003.2000
Grenier, D., Marcoux, E., Azelmat, J., Ben Lagha, A., and Gauthier, P. (2020). Biocompatible combinations of nisin and licorice polyphenols exert synergistic bactericidal effects against enterococcus faecalis and inhibit NF-kappaB activation in monocytes. AMB Express 10:120. doi: 10.1186/s13568-020-01056-w
Hadjilouka, A., Molfeta, C., Panagiotopoulou, O., Paramithiotis, S., Mataragas, M., and Drosinos, E. H. (2016). Expression of Listeria monocytogenes key virulence genes during growth in liquid medium, on rocket and melon at 4, 10 and 30 degrees C. Food Microbiol. 55, 7–15. doi: 10.1016/j.fm.2015.11.008
Harakeh, S., Saleh, I., Zouhairi, O., Baydoun, E., Barbour, E., and Alwan, N. (2009). Antimicrobial resistance of Listeria monocytogenes isolated from dairy-based food products. Sci. Total Environ. 407, 4022–4027. doi: 10.1016/j.scitotenv.2009.04.010
He, S., Zhan, Z., Shi, C., Wang, S., and Shi, X. (2022). Ethanol at subinhibitory concentrations enhances biofilm formation in Salmonella Enteritidis. Food Secur. 11:237. doi: 10.3390/foods11152237
Hou, X., Sheng, Q., Zhang, J., Du, R., Wang, N., Zhu, H., et al. (2023). The application of cinnamon twig extract as an inhibitor of Listeriolysin O against Listeria monocytogenes infection. Molecules 28:1625. doi: 10.3390/molecules28041625
Huang, C., Lu, T. L., and Yang, Y. (2023). Mortality risk factors related to listeriosis - a meta-analysis. J. Infect. Public Health 16, 771–783. doi: 10.1016/j.jiph.2023.03.013
Inamuco, J., Veenendaal, A. K., Burt, S. A., Post, J. A., Tjeerdsma-van Bokhoven, J. L., Haagsman, H. P., et al. (2012). Sub-lethal levels of carvacrol reduce Salmonella Typhimurium motility and invasion of porcine epithelial cells. Vet. Microbiol. 157, 200–207. doi: 10.1016/j.vetmic.2011.12.021
Jalil Sarghaleh, S., Alizadeh Behbahani, B., Hojjati, M., Vasiee, A., and Noshad, M. (2023). Evaluation of the constituent compounds, antioxidant, anticancer, and antimicrobial potential of Prangos ferulacea plant extract and its effect on Listeria monocytogenes virulence gene expression. Front. Microbiol. 14:1202228. doi: 10.3389/fmicb.2023.1202228
Ji, X., Liu, N., Huang, S., and Zhang, C. (2024). A comprehensive review of licorice: the preparation, chemical composition, bioactivities and its applications. Am. J. Chin. Med. 52, 667–716. doi: 10.1142/S0192415X24500289
Jiang, X., Jiang, C., Yu, T., Jiang, X., Kang, R., Ren, S., et al. (2022). Phenyllactic acid application to control Listeria monocytogenes biofilms and its growth in milk and spiced beef. Int. J. Food Microbiol. 381:109910. doi: 10.1016/j.ijfoodmicro.2022.109910
Jiang, X., Yu, T., Xu, Y., Wang, H., Korkeala, H., and Shi, L. (2019). Mdr L, a major facilitator superfamily efflux pump of Listeria monocytogenes involved in tolerance to benzalkonium chloride. Appl. Microbiol. Biotechnol. 103, 1339–1350. doi: 10.1007/s00253-018-9551-y
Jolivet-Gougeon, A., and Bonnaure-Mallet, M. (2014). Biofilms as a mechanism of bacterial resistance. Drug Discov. Today Technol. 11, 49–56. doi: 10.1016/j.ddtec.2014.02.003
Kang, J. S., Yoon, Y. D., Cho, I. J., Han, M. H., Lee, C. W., Park, S. K., et al. (2005). Glabridin, an isoflavan from licorice root, inhibits inducible nitric-oxide synthase expression and improves survival of mice in experimental model of septic shock. J. Pharmacol. Exp. Ther. 312, 1187–1194. doi: 10.1124/jpet.104.077107
Kawacka, I., Olejnik-Schmidt, A., and Schmidt, M. (2022). Nonhemolytic Listeria monocytogenes-prevalence rate, reasons underlying atypical phenotype, and methods for accurate hemolysis assessment. Microorganisms 10:483. doi: 10.3390/microorganisms10020483
Kearns, D. B. (2010). A field guide to bacterial swarming motility. Nat. Rev. Microbiol. 8, 634–644. doi: 10.1038/nrmicro2405
Khan, M. A., and Palaniyar, N. (2017). Transcriptional firing helps to drive NETosis. Sci. Rep. 7:41749. doi: 10.1038/srep41749
Kim, H. S., Suh, K. S., Ko, A., Sul, D., Choi, D., Lee, S. K., et al. (2013). The flavonoid glabridin attenuates 2-deoxy-D-ribose-induced oxidative damage and cellular dysfunction in MC3T3-E1 osteoblastic cells. Int. J. Mol. Med. 31, 243–251. doi: 10.3892/ijmm.2012.1172
Kuang, Y., Li, B., Fan, J., Qiao, X., and Ye, M. (2018). Antitussive and expectorant activities of licorice and its major compounds. Bioorg. Med. Chem. 26, 278–284. doi: 10.1016/j.bmc.2017.11.046
Kumaraswamy, M., Do, C., Sakoulas, G., Nonejuie, P., Tseng, G. W., King, H., et al. (2018). Listeria monocytogenes endocarditis: case report, review of the literature, and laboratory evaluation of potential novel antibiotic synergies. Int. J. Antimicrob. Agents 51, 468–478. doi: 10.1016/j.ijantimicag.2017.12.032
Kwon, H. S., Oh, S. M., and Kim, J. K. (2008). Glabridin, a functional compound of liquorice, attenuates colonic inflammation in mice with dextran sulphate sodium-induced colitis. Clin. Exp. Immunol. 151, 165–173. doi: 10.1111/j.1365-2249.2007.03539.x
Kwon, Y. J., Son, D. H., Chung, T. H., and Lee, Y. J. (2020). A review of the pharmacological efficacy and safety of licorice root from corroborative clinical trial findings. J. Med. Food 23, 12–20. doi: 10.1089/jmf.2019.4459
Lachtara, B., Wieczorek, K., and Osek, J. (2023). Antimicrobial resistance of Listeria monocytogenes serogroups IIa and IVb from food and food-production environments in Poland. J. Vet. Res. 67, 373–379. doi: 10.2478/jvetres-2023-0050
Liao, C., Mao, F., Qian, M., and Wang, X. (2022). Pathogen-derived nucleases: An effective weapon for escaping extracellular traps. Front. Immunol. 13:899890. doi: 10.3389/fimmu.2022.899890
Lin, H., Zhou, C., Yu, K. H., Lin, Y. S., Wang, L. B., Zhang, Y., et al. (2023). Glabridin functions as a quorum sensing inhibitor to inhibit biofilm formation and swarming motility of multidrug-resistant Acinetobacter baumannii. Infect. Drug Resist. 16, 5697–5705. doi: 10.2147/IDR.S417751
Liu, K., Pi, F., Zhang, H., Ji, J., Xia, S., Cui, F., et al. (2017). Metabolomics analysis to evaluate the anti-inflammatory effects of polyphenols: Glabridin reversed metabolism change caused by LPS in RAW 264.7 cells. J. Agric. Food Chem. 65, 6070–6079. doi: 10.1021/acs.jafc.7b01692
Liu, P., Wu, X., Liao, C., Liu, X., Du, J., Shi, H., et al. (2014). Escherichia coli and Candida albicans induced macrophage extracellular trap-like structures with limited microbicidal activity. PLoS One 9:e90042. doi: 10.1371/journal.pone.0090042
Marcoux, E., Lagha, A. B., Gauthier, P., and Grenier, D. (2020). Antimicrobial activities of natural plant compounds against endodontic pathogens and biocompatibility with human gingival fibroblasts. Arch. Oral Biol. 116:104734. doi: 10.1016/j.archoralbio.2020.104734
Mehdi, Y., Letourneau-Montminy, M. P., Gaucher, M. L., Chorfi, Y., Suresh, G., Rouissi, T., et al. (2018). Use of antibiotics in broiler production: global impacts and alternatives. Anim. Nutr. 4, 170–178. doi: 10.1016/j.aninu.2018.03.002
Meng, R., Zhao, Z., Guo, N., Liu, Z., Zhao, X., Li, W., et al. (2015). Effect of honokiol on exotoxin proteins listeriolysin O and p60 secreted by Listeria monocytogenes. J. Med. Microbiol. 64, 1474–1480. doi: 10.1099/jmm.0.000183
Mesa-Ramos, L., Palacios, O. A., Adame-Gallegos, J. R., Chavez-Flores, D., and Nevarez-Moorillon, G. V. (2024). Assessing antibiotic residues in sediments from mangrove ecosystems: a review. Mar. Pollut. Bull. 204:116512. doi: 10.1016/j.marpolbul.2024.116512
Mohapatra, R. K., Mishra, S., Tuglo, L. S., Sarangi, A. K., Kandi, V., Al Ibrahim, A. A., et al. (2024). Recurring food source-based Listeria outbreaks in the United States: An unsolved puzzle of concern? Health Sci. Rep. 7:e1863. doi: 10.1002/hsr2.1863
Muhammad, M. H., Idris, A. L., Fan, X., Guo, Y., Yu, Y., Jin, X., et al. (2020). Beyond risk: bacterial biofilms and their regulating approaches. Front. Microbiol. 11:928. doi: 10.3389/fmicb.2020.00928
Nguyen, B. N., Peterson, B. N., and Portnoy, D. A. (2019). Listeriolysin O: a phagosome-specific cytolysin revisited. Cell. Microbiol. 21:e12988. doi: 10.1111/cmi.12988
Okada, K., Tamura, Y., Yamamoto, M., Inoue, Y., Takagaki, R., Takahashi, K., et al. (1989). Identification of antimicrobial and antioxidant constituents from licorice of Russian and Xinjiang origin. Chem. Pharm. Bull. 37, 2528–2530. doi: 10.1248/cpb.37.2528
Okunnu, B. M., and Berg, R. E. (2019). Neutrophils are more effective than monocytes at Phagosomal containment and killing of Listeria monocytogenes. Immunohorizons 3, 573–584. doi: 10.4049/immunohorizons.1900065
Osek, J., and Wieczorek, K. (2022). Listeria monocytogenes-how this pathogen uses its virulence mechanisms to infect the hosts. Pathogens 11:1491. doi: 10.3390/pathogens11121491
Park, K. H., Jung, M., Kim, D. Y., Lee, Y. M., Lee, M. S., Ryu, B. H., et al. (2020). Effects of subinhibitory concentrations of chlorhexidine and mupirocin on biofilm formation in clinical meticillin-resistant Staphylococcus aureus. J. Hosp. Infect. 106, 295–302. doi: 10.1016/j.jhin.2020.07.010
Pastorino, G., Cornara, L., Soares, S., Rodrigues, F., and Oliveira, M. (2018). Liquorice (Glycyrrhiza glabra): a phytochemical and pharmacological review. Phytother. Res. 32, 2323–2339. doi: 10.1002/ptr.6178
Pogreba-Brown, K., Boyd, K., Schaefer, K., Austhof, E., Armstrong, A., Owusu-Dommey, A., et al. (2022). Complications associated with foodborne Listeriosis: a scoping review. Foodborne Pathog. Dis. 19, 725–743. doi: 10.1089/fpd.2022.0012
Portnoy, D. A., Jacks, P. S., and Hinrichs, D. J. (1988). Role of hemolysin for the intracellular growth of Listeria monocytogenes. J. Exp. Med. 167, 1459–1471. doi: 10.1084/jem.167.4.1459
Poudel, S., Giannone, R. J., Farmer, A. T., Campagna, S. R., Bible, A. N., Morrell-Falvey, J. L., et al. (2019). Integrated proteomics and Lipidomics reveal that the swarming motility of Paenibacillus polymyxa is characterized by phospholipid modification, surfactant deployment, and flagellar specialization relative to swimming motility. Front. Microbiol. 10:2594. doi: 10.3389/fmicb.2019.02594
Poyart-Salmeron, C., Carlier, C., Trieu-Cuot, P., Courtieu, A. L., and Courvalin, P. (1990). Transferable plasmid-mediated antibiotic resistance in Listeria monocytogenes. Lancet 335, 1422–1426. doi: 10.1016/0140-6736(90)91447-i
Rosenblat, M., Belinky, P., Vaya, J., Levy, R., Hayek, T., Coleman, R., et al. (1999). Macrophage enrichment with the isoflavan glabridin inhibits NADPH oxidase-induced cell-mediated oxidation of low density lipoprotein. A possible role for protein kinase C. J. Biol. Chem. 274, 13790–13799. doi: 10.1074/jbc.274.20.13790
Roy, P. K., Song, M. G., and Park, S. Y. (2022). The inhibitory effect of quercetin on biofilm formation of Listeria monocytogenes mixed culture and repression of virulence. Antioxidants 11:1733. doi: 10.3390/antiox11091733
Saitoh, T., Kinoshita, T., and Shibata, S. (1976). New Isoflavan and flavanone from licorice root. Chem. Pharm. Bull. 24, 752–755. doi: 10.1248/cpb.24.752
Shen, T., Chen, L., Liu, Y., Shi, S., Liu, Z., Cai, K., et al. (2021). Decanoic acid modification enhances the antibacterial activity of PMAP-23RI-Dec. Eur. J. Pharm. Sci. 157:105609. doi: 10.1016/j.ejps.2020.105609
Shin, J., Choi, L. S., Jeon, H. J., Lee, H. M., Kim, S. H., Kim, K. W., et al. (2023). Synthetic Glabridin derivatives inhibit LPS-induced inflammation via MAPKs and NF-kappaB pathways in RAW264.7 macrophages. Molecules 28:2135. doi: 10.3390/molecules28052135
Simmler, C., Pauli, G. F., and Chen, S. N. (2013). Phytochemistry and biological properties of glabridin. Fitoterapia 90, 160–184. doi: 10.1016/j.fitote.2013.07.003
Soni, D. K., Singh, R. K., Singh, D. V., and Dubey, S. K. (2013). Characterization of Listeria monocytogenes isolated from Ganges water, human clinical and milk samples at Varanasi, India. Infect. Genet. Evol. 14, 83–91. doi: 10.1016/j.meegid.2012.09.019
Summer, K., Browne, J., Hollanders, M., and Benkendorff, K. (2022). Out of control: the need for standardised solvent approaches and data reporting in antibiofilm assays incorporating dimethyl-sulfoxide (DMSO). Biofilms 4:100081. doi: 10.1016/j.bioflm.2022.100081
Tamburro, M., Sammarco, M. L., Ammendolia, M. G., Fanelli, I., Minelli, F., and Ripabelli, G. (2015). Evaluation of transcription levels of inlA, inlB, hly, bsh and prfA genes in Listeria monocytogenes strains using quantitative reverse-transcription PCR and ability of invasion into human CaCo-2 cells. FEMS Microbiol. Lett. 362:18. doi: 10.1093/femsle/fnv018
Tsukatani, T., Kuroda, R., and Kawaguchi, T. (2022). Screening biofilm eradication activity of ethanol extracts from foodstuffs: potent biofilm eradication activity of glabridin, a major flavonoid from licorice (Glycyrrhiza glabra), alone and in combination with varepsilon-poly-L-lysine. World J. Microbiol. Biotechnol. 38:24. doi: 10.1007/s11274-021-03206-z
Vaillancourt, K., LeBel, G., Pellerin, G., Ben Lagha, A., and Grenier, D. (2021). Effects of the licorice Isoflavans Licoricidin and Glabridin on the growth, adherence properties, and acid production of Streptococcus mutans, and assessment of their biocompatibility. Antibiotics 10:163. doi: 10.3390/antibiotics10020163
Vatanyoopaisarn, S., Nazli, A., Dodd, C. E., Rees, C. E., and Waites, W. M. (2000). Effect of flagella on initial attachment of Listeria monocytogenes to stainless steel. Appl. Environ. Microbiol. 66, 860–863. doi: 10.1128/AEM.66.2.860-863.2000
Vazquez, E., de Gregorio-Vicente, O., Soriano, V., Alvarez-Dominguez, C., Corral, O., and Moreno-Torres, V. (2024). Increased incidence and mortality from Listeria monocytogenes infection in Spain. Int. J. Infect. Dis. 145:107089. doi: 10.1016/j.ijid.2024.107089
Veratti, E., Rossi, T., Giudice, S., Benassi, L., Bertazzoni, G., Morini, D., et al. (2011). 18beta-glycyrrhetinic acid and glabridin prevent oxidative DNA fragmentation in UVB-irradiated human keratinocyte cultures. Anticancer Res. 31, 2209–2215
Wang, Y., Ling, N., Wang, Y., Ou, D., Liang, Z., Li, G., et al. (2024). Effect of ferric ions on Cronobacter sakazakii growth, biofilm formation, and swarming motility. Int. J. Food Microbiol. 408:110418. doi: 10.1016/j.ijfoodmicro.2023.110418
Wang, Z., Ma, Y., Li, Z., Wang, Y., Liu, Y., and Dong, Q. (2022). Characterization of Listeria monocytogenes biofilm formation kinetics and biofilm transfer to cantaloupe surfaces. Food Res. Int. 161:111839. doi: 10.1016/j.foodres.2022.111839
Wang, J., Qiu, J., Tan, W., Zhang, Y., Wang, H., Zhou, X., et al. (2015). Fisetin inhibits Listeria monocytogenes virulence by interfering with the oligomerization of listeriolysin O. J. Infect. Dis. 211, 1376–1387. doi: 10.1093/infdis/jiu520
Wu, B., Liu, X., Nakamoto, S. T., Wall, M., and Li, Y. (2022). Antimicrobial activity of Ohelo berry (Vaccinium calycinum) juice against Listeria monocytogenes and its potential for Milk preservation. Microorganisms 10:548. doi: 10.3390/microorganisms10030548
Xu, W., Peng, M. J., Lu, L. S., Guo, Z. J., Li, A. M., Li, J., et al. (2024). Clinical characteristics and fatality risk factors for patients with Listeria monocytogenes infection: a 12-year hospital-based study in Xi'an, China. Infect. Dis. Ther. 13, 1359–1378. doi: 10.1007/s40121-024-00986-3
Yang, X., Sha, K., Xu, G., Tian, H., Wang, X., Chen, S., et al. (2016). Subinhibitory concentrations of Allicin decrease Uropathogenic Escherichia coli (UPEC) biofilm formation, adhesion ability, and swimming motility. Int. J. Mol. Sci. 17:979. doi: 10.3390/ijms17070979
Yue, Y., Zhong, K., Wu, Y., and Gao, H. (2023). Pyrrole-2-carboxylic acid inhibits biofilm formation and suppresses the virulence of Listeria monocytogenes. Biofouling 39, 527–536. doi: 10.1080/08927014.2023.2235287
Zafer, M. M., Mohamed, G. A., Ibrahim, S. R. M., Ghosh, S., Bornman, C., and Elfaky, M. A. (2024). Biofilm-mediated infections by multidrug-resistant microbes: a comprehensive exploration and forward perspectives. Arch. Microbiol. 206:101. doi: 10.1007/s00203-023-03826-z
Zegadlo, K., Gieron, M., Zarnowiec, P., Durlik-Popinska, K., Krecisz, B., Kaca, W., et al. (2023). Bacterial motility and its role in skin and wound infections. Int. J. Mol. Sci. 24:1707. doi: 10.3390/ijms24021707
Zhai, Y., Tian, W., Chen, K., Lan, L., Kan, J., and Shi, H. (2024). Flagella-mediated adhesion of Escherichia coli O157:H7 to surface of stainless steel, glass and fresh produces during sublethal injury and recovery. Food Microbiol. 117:104383. doi: 10.1016/j.fm.2023.104383
Zhang, X. W., An, M. X., Huang, Z. K., Ma, L., Zhao, D., Yang, Z., et al. (2023). Lpp of Escherichia coli K1 inhibits host ROS production to counteract neutrophil-mediated elimination. Redox Biol. 59:102588. doi: 10.1016/j.redox.2022.102588
Zhang, P., Ji, L., Wu, X., Liping, C., Yan, W., and Dong, F. (2024). Prevalence, genotypic characteristics and antibiotic resistance of Listeria monocytogenes from retail foods in Huzhou, China. J. Food Prot. 87:307. doi: 10.1016/j.jfp.2024.100307
Zhang, F., Wang, F., Li, W., Liang, L., and Sang, X. (2022). The toxicity mechanism of glabridin in prostate cancer cells is involved in reactive oxygen species-dependent PI3K/Akt pathway: integrated utilization of bioinformatic analysis and in vitro test validation. Environ. Toxicol. 37, 2937–2946. doi: 10.1002/tox.23649
Zhang, J., Wu, X., Zhong, B., Liao, Q., Wang, X., Xie, Y., et al. (2023). Review on the diverse biological effects of Glabridin. Drug Des. Devel. Ther. 17, 15–37. doi: 10.2147/DDDT.S385981
Zhou, X., Zhang, B., Cui, Y., Chen, S., Teng, Z., Lu, G., et al. (2017). Curcumin promotes the clearance of Listeria monocytogenes both in vitro and in vivo by reducing Listeriolysin O oligomers. Front. Immunol. 8:574. doi: 10.3389/fimmu.2017.00574
Keywords: Listeria monocytogenes , glabridin, licorice, subinhibitory concentration, antimicrobial agent
Citation: Liao C, Yu C, Guo J and Guan M (2024) Subinhibitory concentrations of glabridin from Glycyrrhiza glabra L. reduce Listeria monocytogenes motility and hemolytic activity but do not exhibit antimicrobial activity. Front. Microbiol. 15:1388388. doi: 10.3389/fmicb.2024.1388388
Edited by:
Valério Monteiro-Neto, Federal University of Maranhão, BrazilReviewed by:
Paweł Krzyżek, Wroclaw Medical University, PolandEugene A. Rogozhin, Institute of Bioorganic Chemistry (RAS), Russia
Copyright © 2024 Liao, Yu, Guo and Guan. This is an open-access article distributed under the terms of the Creative Commons Attribution License (CC BY). The use, distribution or reproduction in other forums is permitted, provided the original author(s) and the copyright owner(s) are credited and that the original publication in this journal is cited, in accordance with accepted academic practice. No use, distribution or reproduction is permitted which does not comply with these terms.
*Correspondence: Chengshui Liao, bGlhb2NoZW5nc2h1aTMzQDE2My5jb20=