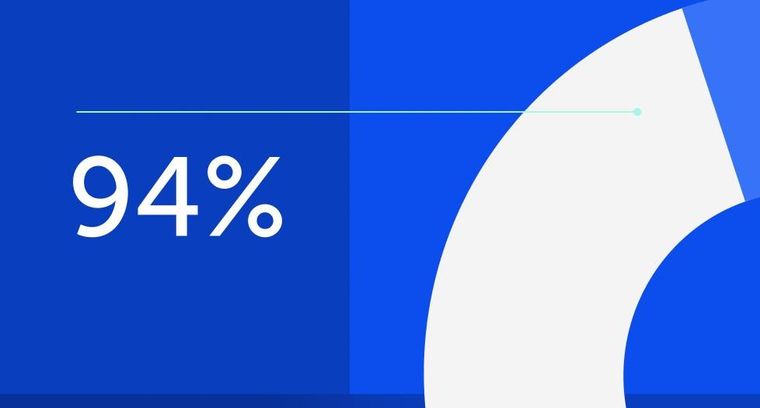
94% of researchers rate our articles as excellent or good
Learn more about the work of our research integrity team to safeguard the quality of each article we publish.
Find out more
REVIEW article
Front. Microbiol., 06 May 2024
Sec. Microbial Physiology and Metabolism
Volume 15 - 2024 | https://doi.org/10.3389/fmicb.2024.1386179
This article is part of the Research TopictRNA and Protein Synthesis in MicroorganismsView all 10 articles
Throughout the tree of life, cells and organisms enter states of dormancy or hibernation as a key feature of their biology: from a bacterium arresting its growth in response to starvation, to a plant seed anticipating placement in fertile ground, to a human oocyte poised for fertilization to create a new life. Recent research shows that when cells hibernate, many of their essential enzymes hibernate too: they disengage from their substrates and associate with a specialized group of proteins known as hibernation factors. Here, we summarize how hibernation factors protect essential cellular enzymes from undesired activity or irreparable damage in hibernating cells. We show how molecular hibernation, once viewed as rare and exclusive to certain molecules like ribosomes, is in fact a widespread property of biological molecules that is required for the sustained persistence of life on Earth.
Do you know that we live on a dormant planet? It is currently estimated that at least 60% of the global microbial biomass exists in various forms of dormancy (Blagodatskaya and Kuzyakov, 2013; Rittershaus et al., 2013). Some bacteria can use dormancy to remain viable for astonishing stretches of time, in some cases exceeding 250 million years (Vreeland et al., 2000; Morono et al., 2020). Furthermore, dormancy is not limited to the realm of microbes. For a multitude of multicellular organisms, including bears, arctic squirrels, raccoons, snakes, snails, spiders, and many others, hibernation serves as an integral stage in the normal life cycle (Nedergaard and Cannon, 1990; Lee, 2008; Mohr et al., 2020). Organisms that do not hibernate as a whole can induce dormancy in some parts of their bodies. For instance, in human bodies, oocytes can remain dormant for over 30 years, exhibiting no overt signs of life while being fully capable of initiating a new life in response to fertilization. Therefore, for many organisms the most prevalent form of life is lack of any activity.
Decades ago, clues began to emerge from early studies of central biomolecules that dormant cells contain dormant molecular machines (Figure 1). However, many of these clues were collected independently, by scientists who had little awareness of studies by others while being focused on their preferred biological assembly—whether it be the ribosome, RNA polymerase, proteasome, ATP synthetase, or others (McCarthy, 1960; Pullman and Monroy, 1963; Hille, 1974; Patterson et al., 1984; Gutteridge et al., 1986; Chu-Ping et al., 1992; Fernández-Tornero, 2018). Nevertheless, when combined, research spanning from the late 1950s to the present day shows compelling evidence of the existence of a common molecular mechanism that allows organisms to survive in a state of dormancy. We refer to this mechanism as “hibernation of biological molecules.” This concept refers to a self-preservation strategy that is conserved from the simplest bacteria to humans, which consists in the production of a special class of proteins, known as hibernation factors that either inhibit or protect essential biological molecules from degradation in response to starvation and stress. And it is only through the presence of these hibernating proteins that organisms can endure extended periods of decreased metabolic activity without digesting and degrading their most essential molecular structures that are indispensable for a cell to be alive.
Since our planet is teeming with dormant life, we anticipate the studies of hibernating biological molecules to bring a lot of value to the clinic and the commercial world. By elucidating how hibernating molecules enable pathogenic bacteria to withstand stress and assaults from antibiotics, we hope to learn how to effectively combat chronic infections by subverting pathogens’ mechanisms of molecular hibernation—something that has already been accomplished to some degree (Song et al., 2019). By studying how this process protects cells and tissues, including agricultural seeds, transplant tissues, or human oocytes, we hope to learn how to enhance biological dormancy processes to slow aging and preserve valuable biological specimens in a safer and longer-lasting form. Underlying all these practical applications, the study of molecular hibernation promises to illuminate the underpinnings of a fundamental mechanism sustaining life on Earth. With the rapid advances in imaging and other tools to study hibernation, we anticipate uncovering an intricate process that prepares a living cell for dormancy—allowing biological molecules to perform their most common activity, which paradoxically involves no activity at all.
In 1958, James Watson worked with Alfred Tissières to study ribosomal particles, which had been recently discovered by George Palade (Tissières et al., 1959). To characterize the chemical properties of ribosomes and the conditions contributing to their stability in vitro, they isolated ribosomes from actively growing Escherichia coli cultures. However, their experimental procedure involved placing E. coli culture on ice and pelleting the cells before isolating the ribosomes. As a result, E. coli cells were inadvertently exposed to cold shock, hypoxia, and nutrient depletion. Under these conditions, Tissières and Watson observed 100S particles in addition to individual 70S ribosomes. They correctly concluded that 100S particles represent ribosome dimers, but they were unaware of their biological significance (Tissières et al., 1959). Thus, unknowingly, Tissières and Watson became perhaps the first people on Earth to observe hibernating molecules. Their accidental observation initiated a long-standing tradition of discovering hibernating molecules by chance, as a byproduct of their isolation from cells or tissues that were placed on ice and centrifuged in nutrient-free buffers (Yusupov and Spirin, 1988; Ben-Shem et al., 2011; Anger et al., 2013; Brown et al., 2018; Barandun et al., 2019; Ehrenbolger et al., 2020; Nicholson et al., 2022).
Two years later, Brian McCarthy showed that E. coli 100S ribosomes appear only during stationary phase or nutrient depletion, when E. coli cells reduce the rate of protein synthesis (McCarthy, 1960). Upon nutrient replenishment, cells promptly resumed protein synthesis, and the presence of 100S ribosomes was no longer observed. These experiments put forth the idea that environmental stress might govern the formation of 100S ribosomes, which were only detectable during periods of protein synthesis arrest: laying an early foundation for our current understanding of ribosome hibernation. Additionally, McCarthy was the first to hypothesize that cells may possess the ability to “switch off” their ribosomes in response to stress (McCarthy, 1960).
While the mechanism behind the formation of E. coli 100S ribosomes would remain a mystery for over 3 decades, important strides were made during that time in other experimental systems, including unfertilized eggs of animal species. By that time, it was well established that oocytes can endure dormancy for several decades while remaining capable of rapid reactivation upon fertilization. Seeking to understand this remarkable capacity for self-preservation, early studies in the 1970s detected “factors” of unknown molecular identity that bind to ribosomes in unfertilized eggs and inhibit protein synthesis in vitro (Gambino et al., 1973; Hille, 1974; Huang and Warner, 1974). Thus, as early as 1973, it became clear that ribosomes can associate with unidentified factors that seem to inhibit protein synthesis in dormant, unfertilized eggs.
The first ribosome hibernation factor was identified in 1990 by Wada et al. (1990). Using two-dimensional protein gels to characterize the composition of E. coli ribosomes under different growth conditions, they discovered an additional protein that binds to ribosomes from the 100S fraction of stationary E. coli cells in a stoichiometric manner. They named this tiny protein, comprising just 55 amino acids, ribosome-modulating factor (RMF). They showed that RMF gene is transcriptionally activated in stationary phase cultures and is necessary for the formation of 100S ribosomes in E. coli. Based on these findings, Wada and colleagues were the first to propose that RMF triggers the formation of 100S ribosomes as a strategy to store and protect ribosomes from nucleolytic degradation. Therefore, the research conducted by Wada’s group established that, in metabolically inactive cells, nearly all (or all) ribosomes associate with a dedicated hibernation factor.
Shortly after the identification of RMF, two additional ribosome hibernation factors, HPF and RaiA, were identified in E. coli (Figure 1; Table 1). HPF and RaiA share the same fold and likely originated through gene duplication to fulfill redundant roles in ribosome hibernation (Agafonov et al., 1999, 2001; Maki et al., 2000). In 2004, RaiA became the first ribosome hibernation factor to be structurally characterized while bound to ribosomes. The pioneering study by Jamie Cate and colleagues showed that RaiA occupies the active sites of the small ribosomal subunit, including the tRNA-binding sites and mRNA-binding channel (Figure 2; Vila-Sanjurjo et al., 2004). This structural study revealed a common characteristic that was later identified in virtually all known ribosome hibernation factors: not only do these factors bind to ribosomes in metabolically inactive cells, but also they occupy the active centers of ribosomes, making these centers inaccessible for other biological molecules.
Following the discovery of ribosome hibernation factors in bacteria, functionally similar proteins were soon identified in eukaryotic species. The first of these factors, Stm1, was identified in 2011 as a protein that binds to essentially all cellular ribosomes in yeast S. cerevisiae in response to sudden glucose starvation (Ben-Shem et al., 2011). Later, the Stm1 homolog, Serbp1, was found to bind to ribosomes in cold-shocked cells of humans and Drosophila melanogaster (Anger et al., 2013). Subsequent studies revealed five more families of ribosome hibernation factors. These included (i) proteins Lso2/CCDC124 in humans, yeasts and parasitic fungi microsporidia (Wang et al., 2018; Ehrenbolger et al., 2020; Wells et al., 2020), (ii) proteins IFRD1/IFRD2 in rabbit reticulate extracts and Drosophila cells (Brown et al., 2018; Hopes et al., 2022), (iii, iv) proteins MDF1 and MDF2 in metabolically inactive spores of fungal parasites microsporidia (Barandun et al., 2019), and (v) protein Dap1b in frogs/Dapl1 in xenopus that participates in ribosome hibernation in oocytes of frogs or zebrafish (Leesch et al., 2023).
All these ribosome hibernation factors in eukaryotes were shown to act in essentially the same manner as bacterial hibernation factors—in terms of their ability to occupy the functional centers of all or most ribosomes in metabolically inactive or stressed cells (Figure 2). However, strikingly, none of the ribosome hibernation factors of eukaryotes bore resemblance to those of bacteria, indicating an independent evolutionary origin of ribosome hibernation factors in the two domains of life.
Combined with the studies of bacterial hibernation factors, these findings have taught us two important lessons. Firstly, it has become evident that the majority of characterized species harbor more than one family of hibernation factors. The best-studied organism E. coli stands out as the current leader in this regard, harboring at least four families of ribosome hibernation factors, including proteins RMF, HPF/RaiA, and putative hibernation factors Sra (Wada et al., 1990) and YqjD/ElaB/YgaM (Yoshida et al., 2012). The reasons underlying the necessity for multiple families of these proteins remain unclear. It also remains unclear whether each family of factors exhibits a preference for specific environments or stressors.
The second lesson learned is that, despite the essential role of ribosome hibernation in cell survival, these proteins are not universally conserved across the evolutionary tree. Instead, bacteria and eukaryotes possess distinct sets of ribosome hibernation factors—including Stm1/Serbp1 (Ben-Shem et al., 2011), Lso2/CCDC124 (Wang et al., 2018), IFRD1/IFRD2 (Brown et al., 2018), MDF1 (Barandun et al., 2019), MDF2 (Barandun et al., 2019), and Dap1b (Leesch et al., 2023) in eukaryotes, and HPF/RaiA (Agafonov et al., 1999, 2001; Maki et al., 2000), RMF (Wada et al., 1990), and Balon (Helena-Bueno et al., 2024) in bacteria.
It remains unclear how conserved each family of ribosome hibernation factors is across species: whether they are broadly conserved across bacteria or eukaryotes, or if certain lineages of bacteria and eukaryotes encode their own sets of hibernation factors. One difficulty in addressing this question lies in the much higher rate of sequence evolution for these proteins compared to other ribosome-binding proteins in a cell. This heightened rate of evolution makes it challenging to employ traditional approaches of homology search, such as simple Blast or even Markov Models-based search of homology, for the identification of their protein homologs across species (Helena-Bueno et al., 2024). However, the characteristic domain specificity of hibernation factors suggests that they emerged shortly after the split of bacteria and archaea-eukaryotes. If our current dating of this evolutionary event is accurate, then living cells have been enjoying ribosome hibernation for approximately 3.5 billion years.
The discovery of ribosome hibernation factors made it possible to assess the physiological impacts of ribosome hibernation on cellular dormancy, metabolism, and survival. Studies have shown that the deletion of genes encoding RaiA/HPF and RMF leads to accelerated rRNA decay in metabolically inactive cells of E. coli, Staphylococcus aureus, and Mycobacterium smegmatis (Trauner et al., 2012; Lipońska and Yap, 2021; Prossliner et al., 2021). Furthermore, the absence of hibernation factors in stationary phase bacterial cultures was shown to cause ribosomal dissociation into individual subunits, followed by nucleolytic degradation of ribosomal subunits by the RNA-degrading enzyme RNase R (Lipońska and Yap, 2021). Remarkably, these ribosomes tend to accumulate rRNA nicks precisely at the sites where hibernation factors bind, leading to the conclusion that the primary role of ribosome hibernation factors is to safeguard the vulnerable active centers of the ribosome against cellular nucleases (Prossliner et al., 2021). The protective role of hibernation factors in maintaining ribosome integrity (as opposed to ribosome inhibition) has also been shown in resting Bacillus subtilis cells where lack of HPF results in a significant loss of ribosomal proteins uS2 and uS3 during stationary phase (Feaga et al., 2020). This effect is likely attributed to the degradation of the small ribosomal subunit by RNAse R (Dimitrova-Paternoga et al., 2024).
When dormant cells are transferred back to the normal growth environment, ribosomes quickly exit hibernation. Although the exact mechanism of this process is yet to be determined, studies of the hibernation factors HPF/RaiA, RMF, and Stm1 have shown that ribosomes may exit hibernation with the assistance of ribosome-recycling factors, including proteins RRF and HflX in bacteria (Basu and Yap, 2017; Basu et al., 2020), and Pelota/Dom34 and Rli1/Hbs1 in eukaryotes (Van Den Elzen et al., 2014). These factors split ribosomes into individual subunits and appear to release hibernation factors from the ribosomes, enabling them to engage in protein synthesis.
Shortly after the identification of hibernation factors in both bacteria and eukaryotes, it became evident that they impact the ability of cells to survive stress. Genetic knockouts of RMF in E. coli not only accelerate rRNA decay but cause a 100-fold decline in cellular survival rate after a 5-day toxic exposure to acid (El-Sharoud and Niven, 2007). The survival of RMF-deficient cells largely depends on the time that cells spend in stationary phase or under starvation. While RMF was dispensable for a relatively short-term stress of up to 4 days, the impact of RMF on the ability of cells to recover increased dramatically with longer periods of stress. A similar, time-dependent impact was observed in other model organisms, including Stm1(Serbp1)-deficient and Lso2-deficient yeasts (Van Dyke et al., 2013; Wang et al., 2018) and HPF/RaiA-depleted E. coli (Prossliner et al., 2021), S. aureus (Lipońska and Yap, 2021), and M. smegmatis (Trauner et al., 2012). Collectively, these data revealed that the fitness cost of hibernation factors depends on the duration of metabolically inactive states. The longer cells remain metabolically inactive in the absence of hibernation factors, the less likely they are to recover. Given that many cells can remain dormant for several years, hibernation factors are therefore a matter of life or death during such states of long-term dormancy.
Importantly, a growing body of evidence suggests that, aside from their role in long-term cell survival, hibernation factors may participate in short-term cellular responses. These responses include transient exposure to oxidants, zinc depletion, osmotic shock, cold shock, as well as gradual fluctuations of nutrients that are sensed by mTOR signaling or eEF2K signaling pathways (Li et al., 2018; Smith et al., 2021; Saba et al., 2023; Shetty et al., 2023). For example, eEF2K signaling appears to control ribosome availability in mammalian neurons by triggering transient hibernation of a subset of cellular ribosomes and inducing a reversible deposition of excessive amounts of ribosomes into large macromolecular condensates known as p-bodies (Smith et al., 2021).
While foundational work suggested that ribosomes hibernate solely in association with hibernation factors, more recent research has shown that, for many biological species, this view is incomplete. Ribosomes isolated from ice-cold human blood samples and Drosophila eggs were shown to associate not only with the hibernation factor Serbp1 but with two additional molecules, the translation elongation factor eEF2 and deacylated tRNA (Figure 3) (Anger et al., 2013; Leesch et al., 2023). Similarly, ribosomes isolated from oocytes of frogs and zebrafish associate not only with the hibernation factors Serbp1(Hapb4) and Dapl1 in xenopus (frog) and dap1b in zebrafish, but also with eEF2 and the translation factor eIF5a (Leesch et al., 2023). In bacteria, the elongation factor EF-Tu has been shown to bind hibernating ribosomes in metabolically inactive B. subtilis cells during spore formation (Pereira et al., 2015), and in the γ-proteobacterium Psychrobacter urativorans where stress conditions trigger EF-Tu binding in addition to the hibernation factors HPF/RaiA and Balon (Helena-Bueno et al., 2024). The common thread connecting all these non-hibernation factors that bind dormant ribosomes is the fact that they are all accessory factors in normal protein synthesis, making their binding to hibernating ribosomes highly unexpected (Figure 3).
Figure 3. Hibernation factors frequently bind ribosomes in cooperation with factors of protein synthesis.
While the biological role(s) of these protein synthesis factors in ribosome hibernation remains unclear, a few lessons can be derived from structural studies that show the location of these factors in hibernating ribosomes. Just as during the normal cycle of protein synthesis, proteins eEF2 and EF-Tu bind to the sarcin-ricin loop and the L7/12-stalk (or P-stalk in eukaryotes) of hibernating ribosomes, whereas tRNA molecules associate with the ribosomal L1-stalk. It is therefore possible that these proteins may help to shield additional vulnerable active sites of hibernating ribosomes from undesired activity or damage. In light of these findings, the prevailing narrative has gradually shifted from viewing idle ribosomes as vacant to appreciating that idle ribosomes can bind a wide array of cellular proteins to actively enter an assisted state of hibernation.
The large size of ribosomal particles, which enabled their early discovery by George Palade in 1955, has also enabled their observation through transmission microscopy in dormant cells. Among the earliest snapshots of this kind were the ones obtained in 1972 for oocytes and follicular cells of lizards Lacerta sicula (Taddei, 1972). These snapshots revealed that, during the periods of winter rest, the ribosomes of these lizards aggregate into so called “ribosome bodies.” Each of these bodies is made of crystalline sheets comprising thousands of ribosome tetramers that aggregate into a periodic pattern. During spring, these ribosome bodies are disassembled into individual ribosomes, so that ribosome bodies are completely absent in summer. Similar patterns were also observed in oocytes from mice (Burkholder et al., 1971) and an ascidian Ciona intestinalis (Kessel, 1966), as well as in cold-shocked chick embryos (Morimoto et al., 1972). There, ribosomes were found to be orderly packed on the inner side of their cell membranes, further illustrating that ribosomes can produce complex and periodical arrangements in vivo. In her Nobel Prize lecture, Ada Yonath, a pioneer in ribosome structural studies, revealed that early imaging studies of ribosomes through transmission electron microscopy served as a major motivation for her to attempt to crystallize ribosomes: the fact that ribosomes could form crystal-like aggregates in a cell suggested the possibility of their crystallization in a test tube (Yonath, 2010).
Following the initial observation of ribosome aggregates in vivo, these studies were largely forgotten and rarely discussed in the literature. However, with the advent of cryo-electron tomography, interest in this supramolecular organization of hibernating ribosomes has been reignited, bringing this intriguing ribosome behavior back into the spotlight of ribosome biology. Specifically, in the last year, cytosolic ribosomes of yeasts cell have been shown to associate with the outer membrane of mitochondria during glucose starvation (Gemin et al., 2023). Similarly, in metabolically inactive spores of parasitic fungi microsporidia, ribosomes were shown to assemble into helical sheets comprising dozens or hundreds of ribosomes per sheet (Sharma et al., 2024). Currently, the biological significance and mechanisms of these assemblies of hibernating ribosomes remain unclear. However, it is clear that hibernating ribosomes not only associate with special hibernation proteins but also undergo changes oligomeric status and intracellular localization, where they tend to associate with membranes and with each other.
How is the stoichiometry between ribosomes and hibernation factors regulated in metabolically active cells vs. dormant cells? Through quantifying ribosome levels in E. coli growing in different media, scientists from the so-called “Copenhagen School” in the early 1970s have found that in rich media, E. coli has a division time of 24 min and contains approximately 72,000 ribosomes per cell (Burton, 1998). In minimal media, the same strain had a division time of 100 min and contained only 6,800 ribosomes per cell. However, comparison of these cells revealed that more rapidly dividing E. coli tended to be much larger in size. As a result, the concentration of ribosomes within the cytoplasm of actively growing E. coli remains largely invariant, constituting nearly one-third of the dry cellular weight in both active and slow-growing E. coli cultures (Burton, 1998).
Furthermore, recent quantitative proteomics studies have estimated that an actively growing E. coli cell contains approximately 28,000–36,000 ribosomes and comparable numbers of hibernation factors, including 2,000 copies of HPF and 7,900 copies of RaiA, although only 199 copies of RMF (Schmidt et al., 2016). Thus, actively growing cells seem to bear a large pool of available hibernation factors, sufficient to bind approximately a third of all cellular ribosomes (Table 2). Recent studies suggest that dedicated mechanisms exist to constrain hibernation factors in an inactive state in actively growing cells to prevent them from interfering with protein synthesis. For example, S. aureus produces YwlG under normal growth conditions, which acts as an inhibitor that sequesters the hibernation factor HPF (Ranava et al., 2022). Similarly, Saccharomyces cerevisiae has been shown to inactivate the hibernation factor Serbp1/Stm1 via phosphorylation by the TOR kinase (Shetty et al., 2023). Furthermore, mutations of ribosome-binding residues of Serbp1 that are phosphorylated by TORC1 cause a delay in the resumption of protein synthesis in nutrient-deprived cells once they are transferred to fresh media (Shetty et al., 2023). This suggests that TORC1-mediated regulation of ribosome activity might not be limited to inducing dormancy, but also play a role in the reactivation of ribosomes upon nutrient replenishment.
Table 2. Estimated protein copy numbers in Escherichia coli during active growth compared to the stationary phase.
When cells exhaust the available nutrients and transition to stationary phase, the synthesis of ribosomes slows down and cells degrade a significant fraction of their ribosomes shortly after nutrient depletion (Adachi and Sells, 1979; Davis et al., 1986). In E. coli, where cells are estimated to translate an average protein in just 20 s, the transition to ribosome hibernation is known to take about 1 min. Mass spectrometry studies show that after 24 h in stationary phase, E. coli cells contain about 2,000 ribosomes, whereas the amount of hibernation factors HPF, RaiA and RMF increases to 4,000, 11,700, and 3,500, respectively (Schmidt et al., 2016). Thus, hibernation factors greatly outnumber ribosomes in these conditions, explaining their ability to bind essentially all cellular ribosomes during prolonged periods of metabolic inactivity. Notably, hibernation factors also substantially outnumber other factors involved in ribosome-associated stress responses. For example, stationary phase E. coli cells contain just 2 detectable copies of the protein RelA that is involved in cellular responses to stress or nutrient depletion (Schmidt et al., 2016), which corresponds stoichiometrically to just 0.01% of cellular ribosomes (Table 2).
Importantly, states of molecular hibernation are not limited to ribosomes but also extend to other essential enzymes (Figure 4). Another well-characterized case of hibernating enzymes includes RNA polymerases. In both eukaryotes (S. cerevisiae) and bacteria (M. smegmatis), RNA polymerases have been shown to hibernate by forming inactive dimers or octamers (Fernández-Tornero, 2018; Kouba et al., 2020; Pei et al., 2020; Aibara et al., 2021; Heiss et al., 2021; Morichaud et al., 2023). In yeasts, hibernation of RNA polymerase I was explored both in vitro and in vivo (Torreira et al., 2017). This hibernation is induced through the formation of dimers, where the flexible stalk of RNA polymerase I from one molecule is inserted into the DNA-binding channel of another molecule within the dimer. As a result, RNA polymerase I becomes inactive. When dormant yeast cells are brought back to an optimal environment, RNA polymerase I dimers get disassembled with the help of the protein Rrn3 that prevents the stalk from acting as a DNA tunnel-binding factor (alongside other activities of Rrn3) (Torreira et al., 2017).
Furthermore, recent studies have revealed that bacteria M. smegmatis and B. subtilis possess a dedicated hibernation factor for RNA polymerase, protein HelD (Kouba et al., 2020; Pei et al., 2020). When cells experience starvation and stress, HelD acts in a similar fashion to ribosome hibernation factors by occupying the active sites of RNA polymerase, such as DNA- and RNA-binding channels (Figure 4). Thus, at least two molecular machines of a living cell were shown to hibernate in association with dedicated and genetically encoded hibernation factor proteins.
A few other enzymes, such as plant catalases or Rubisco enzyme, were shown to enter a hibernation-like state in response to associating with small molecules endogenously produced by plant cells in response to cold shock or darkness (Patterson et al., 1984; Gutteridge et al., 1986). Most extensively, this process has been studied for Rubisco, the plant enzyme responsible for converting carbon dioxide into organic compounds. During night time, when the light becomes unavailable for promoting the photosynthesis, Rubisco markedly reduced its activity. In some plant species, this inhibition was shown to be caused by the intracellular accumulation of the small molecule 2-carboxy-D-arabinitol 1-phosphate (CA1P) during darkness and low light, progressively binding to the active site of Rubisco (Moore and Seemann, 1994; Liu et al., 2022; Orr et al., 2023). As light increases, CA1P is removed from Rubisco by a specialized protein called Rubisco activase, while a specific phosphatase known as CA1Pase inactivates CA1P through dephosphorylation, producing a CA molecule that cannot bind to Rubisco (Orr et al., 2023). In addition to CA1P, several other small molecules are currently being investigated as condition-specific endogenous inhibitors of plant Rubisco. These include phosphorylated sugar molecules known as XuBP (Salvucci and Crafts-Brandner, 2004), PDBP (Bracher et al., 2017), and CTBP (Parry et al., 2008). While it is unknown how many enzymes in nature undergo a similar hibernation mechanism, this example illustrates that, in addition to genetically encoded hibernation factor proteins, some enzymes can hibernate by associating with endogenously produced small molecules.
We do not know how many enzymes can hibernate. But the growing evidence suggests that molecular hibernation may be a common quality among essential enzymes to enter, withstand, and emerge from a wide variety of cellular stresses. For instance, dedicated inhibitors that are induced by stress and starvation—among other factors—have been identified not only for ribosomes and RNA polymerases, but also for the ATP synthase of eukaryotic mitochondria (protein IF1) (Pullman and Monroy, 1963; Gu et al., 2019) and proteasomes (protein PI31) (Chu-Ping et al., 1992; Jespersen et al., 2022), although their role in molecular hibernation is still a matter of debate. Nevertheless, given a growing number of instances of apparent molecular hibernation, it is very plausible that living cells employ an array of undiscovered and intricate mechanisms to prepare their most precious molecules for extended periods of inactivity—in a state of a delicate balance between being alive and dead that we call hibernation.
In the story of the Tower of Babel, God decides to punish arrogant people by inventing new languages to prevent them from understanding each other. It seems that a similar issue exists in the field of ribosome hibernation, where homologs of the same protein have different names in different organisms or even in the same organism (with Mycobacterium smegmatis being the prime example). To help our readers, we have created a catalog of the most common names of proteins from the HPF/RaiA family. We show that this craving for pseudonyms arises from independent discoveries of the same protein in different species or is driven by the fact that some species encode more than one isoform of HPF/RaiA factors, creating a need to discriminate between them.
KH-B: Conceptualization, Writing – original draft, Writing – review & editing. LIC: Conceptualization, Writing – original draft, Writing – review & editing. SVM: Conceptualization, Writing – review & editing.
The author(s) declare that financial support was received for the research, authorship, and/or publication of this article. This work was funded by the Newcastle University Overseas Fellowship (to KH-B), the Biotechnology and Biological Sciences Research Council (BB/T008695/1 to LIC), and the Royal Society (RGS/R2/202003 to SVM).
The authors thank the members of Melnikov lab (all at Newcastle University, United Kingdom) as well as Matthieu G. Gagnon (UTMB, United States), Sarah Loerch (UC Santa Cruz, United States), and Carlos Fernández-Tornero (CIB Margarita Salas, Spain) for their critical comments. We also thank our reviewers, including Zachary T. Campbell (University of Wisconsin, United States), for their time and input, which have significantly contributed to improving the quality of our work. Finally, we are grateful to Ron Milo and Rob Phillips whose resource Cell Biology by the Numbers had inspired us to think in numbers while writing this review.
The authors declare that the research was conducted in the absence of any commercial or financial relationships that could be construed as a potential conflict of interest.
All claims expressed in this article are solely those of the authors and do not necessarily represent those of their affiliated organizations, or those of the publisher, the editors and the reviewers. Any product that may be evaluated in this article, or claim that may be made by its manufacturer, is not guaranteed or endorsed by the publisher.
Adachi, K., and Sells, B. H. (1979). The effect of magnesium starvation on the dissociation of ribosomal proteins from Escherichia coli K-12 ribosomes. Biochim. Biophys. Acta 563, 163–170. doi: 10.1016/0005-2787(79)90017-0
Agafonov, D. E., Kolb, V. A., Nazimov, I. V., and Spirin, A. S. (1999). A protein residing at the subunit interface of the bacterial ribosome. Proc. Natl. Acad. Sci. 96, 12345–12349. doi: 10.1073/pnas.96.22.12345
Agafonov, D. E., Kolb, V. A., and Spirin, A. S. (2001). Ribosome-associated protein that inhibits translation at the aminoacyl-tRNA binding stage. EMBO Rep. 2, 399–402. doi: 10.1093/embo-reports/kve091
Aibara, S., Dienemann, C., and Cramer, P. (2021). Structure of an inactive RNA polymerase II dimer. Nucleic Acids Res. 49, 10747–10755. doi: 10.1093/nar/gkab783
Anger, A. M., Armache, J. P., Berninghausen, O., Habeck, M., Subklewe, M., Wilson, D. N., et al. (2013). Structures of the human and drosophila 80S ribosome. Nature 497, 80–85. doi: 10.1038/nature12104
Barandun, J., Hunziker, M., Vossbrinck, C. R., and Klinge, S. (2019). Evolutionary compaction and adaptation visualized by the structure of the dormant microsporidian ribosome. Nat. Microbiol. 4, 1798–1804. doi: 10.1038/s41564-019-0514-6
Basu, A., Shields, K. E., and Yap, M. N. (2020). The hibernating 100S complex is a target of ribosome-recycling factor and elongation factor G in Staphylococcus aureus. J. Biol. Chem. 295, 6053–6063. doi: 10.1074/jbc.RA119.012307
Basu, A., and Yap, M. N. (2017). Disassembly of the Staphylococcus aureus hibernating 100S ribosome by an evolutionarily conserved GTPase. Proc. Natl. Acad. Sci. 114, E8165–E8173. doi: 10.1073/pnas.1709588114
Ben-Shem, A., Garreau de Loubresse, N., Melnikov, S., Jenner, L., Yusupova, G., and Yusupov, M. (2011). The structure of the eukaryotic ribosome at 3.0 Å resolution. Science 334, 1524–1529. doi: 10.1126/science.1212642
Blagodatskaya, E., and Kuzyakov, Y. (2013). Active microorganisms in soil: critical review of estimation criteria and approaches. Soil Biol. Biochem. 67, 192–211. doi: 10.1016/j.soilbio.2013.08.024
Bracher, A., Whitney, S. M., Hartl, F. U., and Hayer-Hartl, M. (2017). Biogenesis and metabolic maintenance of rubisco. Annu. Rev. Plant Biol. 68, 29–60. doi: 10.1146/annurev-arplant-043015-111633
Brown, A., Baird, M. R., Yip, M. C., Murray, J., and Shao, S. (2018). Structures of translationally inactive mammalian ribosomes. eLife 7:e40486. doi: 10.7554/eLife.40486
Burkholder, G. D., Comings, D. E., and Okada, T. A. (1971). A storage form of ribosomes in mouse oocytes. Exp. Cell Res. 69, 361–371. doi: 10.1016/0014-4827(71)90236-9
Burton, R. F. (1998). Biology by numbers: An encouragement to quantitative thinking. Cambridge: Cambridge University Press.
Chu-Ping, M., Slaughter, C. A., and DeMartino, G. N. (1992). Purification and characterization of a protein inhibitor of the 20S proteasome (macropain). Biochim. Biophys. Acta 1119, 303–311. doi: 10.1016/0167-4838(92)90218-3
Contreras, L. M., Sevilla, P., Cámara-Artigas, A., Hernández-Cifre, J. G., Rizzuti, B., Florencio, F. J., et al. (2018). The cyanobacterial ribosomal-associated protein LrtA from Synechocystis sp. PCC 6803 is an oligomeric protein in solution with chameleonic sequence properties. Int. J. Mol. Sci. 19:1857. doi: 10.3390/ijms19071857
Davis, B. D., Luger, S. M., and Tai, P. C. (1986). Role of ribosome degradation in the death of starved Escherichia coli cells. J. Bacteriol. 166, 439–445. doi: 10.1128/jb.166.2.439-445.1986
Dimitrova-Paternoga, L., Kasvandik, S., Beckert, B., Granneman, S., Tenson, T., Wilson, D. N., et al. (2024). Structural basis of ribosomal 30S subunit degradation by RNase R. Nature 626, 1133–1140. doi: 10.1038/s41586-024-07027-6
Ehrenbolger, K., Jespersen, N., Sharma, H., Sokolova, Y. Y., Tokarev, Y. S., Vossbrinck, C. R., et al. (2020). Differences in structure and hibernation mechanism highlight diversification of the microsporidian ribosome. PLoS Biol. 18:e3000958. doi: 10.1371/journal.pbio.3000958
El-Sharoud, W. M., and Niven, G. W. (2007). The influence of ribosome modulation factor on the survival of stationary-phase Escherichia coli during acid stress. Microbiology 153, 247–253. doi: 10.1099/mic.0.2006/001552-0
Feaga, H. A., Kopylov, M., Kim, J. K., Jovanovic, M., and Dworkin, J. (2020). Ribosome dimerization protects the small subunit. J. Bacteriol. 202, 10–128. doi: 10.1128/JB.00009-20
Fernández-Tornero, C. (2018). RNA polymerase I activation and hibernation: unique mechanisms for unique genes. Transcription 9, 248–254. doi: 10.1080/21541264.2017.1416267
Gambino, R., Metafora, S., Felicetti, L., and Raisman, J. (1973). Properties of the ribosomal salt wash from unfertilized and fertilized sea urchin eggs and its effect on natural mRNA translation. Biochim. Biophys. Acta 312, 377–391. doi: 10.1016/0005-2787(73)90382-1
Gemin, O, Gluc, M, Purdy, M, Rosa, H, Niemann, M, Peskova, Y, et al. (2023). Hibernating ribosomes tether to mitochondria as an adaptive response to cellular stress during glucose depletion. bioRxiv. [Preprint]. doi: 10.1101/2023.10.08.561365v1
Gu, J., Zhang, L., Zong, S., Guo, R., Liu, T., Yi, J., et al. (2019). Cryo-EM structure of the mammalian ATP synthase tetramer bound with inhibitory protein IF1. Science 364, 1068–1075. doi: 10.1126/science.aaw4852
Gutteridge, S., Parry, M. A., Burton, S., Keys, A. J., Mudd, A., Feeney, J., et al. (1986). A nocturnal inhibitor of carboxylation in leaves. Nature 324, 274–276. doi: 10.1038/324274a0
Heiss, F. B., Daiß, J. L., Becker, P., and Engel, C. (2021). Conserved strategies of RNA polymerase I hibernation and activation. Nat. Commun. 12:758. doi: 10.1038/s41467-021-21031-8
Helena-Bueno, K., Rybak, M. Y., Ekemezie, C. L., Sullivan, R., Brown, C. R., Dingwall, C., et al. (2024). A new family of bacterial ribosome hibernation factors. Nature 626, 1125–1132. doi: 10.1038/s41586-024-07041-8
Hille, M. B. (1974). Inhibitor of protein synthesis isolated from ribosomes of unfertilised eggs and embryos of sea urchins. Nature 249, 556–558. doi: 10.1038/249556a0
Hopes, T., Norris, K., Agapiou, M., McCarthy, C. G., Lewis, P. A., O’Connell, M. J., et al. (2022). Ribosome heterogeneity in Drosophila melanogaster gonads through paralog-switching. Nucleic Acids Res. 50, 2240–2257. doi: 10.1093/nar/gkab606
Huang, F. L., and Warner, A. H. (1974). Control of protein synthesis in brine shrimp embryos by repression of ribosomal activity. Arch. Biochem. Biophys. 163, 716–727. doi: 10.1016/0003-9861(74)90533-5
Jespersen, N., Ehrenbolger, K., Winiger, R. R., Svedberg, D., Vossbrinck, C. R., and Barandun, J. (2022). Structure of the reduced microsporidian proteasome bound by PI31-like peptides in dormant spores. Nat. Commun. 13:6962. doi: 10.1038/s41467-022-34691-x
Kessel, R. G. (1966). An electron microscope study of nuclear-cytoplasmic exchange in oocytes of Ciona intestinalis. J. Ultrastruct. Res. 15, 181–196. doi: 10.1016/S0022-5320(66)80103-X
Kouba, T., Koval’, T., Sudzinová, P., Pospíšil, J., Brezovská, B., Hnilicová, J., et al. (2020). Mycobacterial HelD is a nucleic acids-clearing factor for RNA polymerase. Nat. Commun. 11:6419. doi: 10.1038/s41467-020-20158-4
Kumar, A., Majid, M., Kunisch, R., Rani, P. S., Qureshi, I. A., Lewin, A., et al. (2012). Mycobacterium tuberculosis DosR regulon gene Rv0079 encodes a putative, dormancy associated translation inhibitor (DATIN). PLoS One 7:e38709. doi: 10.1371/journal.pone.0038709
Kumar, N., Sharma, S., and Kaushal, P. S. (2024). Cryo-EM structure of the mycobacterial 70S ribosome in complex with ribosome hibernation promotion factor RafH. Nat. Commun. 15:638. doi: 10.1038/s41467-024-44879-y
Lee, C. C. (2008). Is human hibernation possible? Annu. Rev. Med. 59, 177–186. doi: 10.1146/annurev.med.59.061506.110403
Leesch, F., Lorenzo-Orts, L., Pribitzer, C., Grishkovskaya, I., Roehsner, J., Chugunova, A., et al. (2023). A molecular network of conserved factors keeps ribosomes dormant in the egg. Nature 613, 712–720. doi: 10.1038/s41586-022-05623-y
Li, Y., Sharma, M. R., Koripella, R. K., Yang, Y., Kaushal, P. S., Lin, Q., et al. (2018). Zinc depletion induces ribosome hibernation in mycobacteria. Proc. Natl. Acad. Sci. 115, 8191–8196. doi: 10.1073/pnas.1804555115
Lipońska, A., and Yap, M. N. (2021). Hibernation-promoting factor sequesters Staphylococcus aureus ribosomes to antagonize RNase R-mediated nucleolytic degradation. MBio 12:e0033421. doi: 10.1128/mBio.00334-21
Liu, A. K., Pereira, J. H., Kehl, A. J., Rosenberg, D. J., Orr, D. J., Chu, S. K. S., et al. (2022). Structural plasticity enables evolution and innovation of RuBisCO assemblies. Sci. Adv. 8:eadc9440.
Maki, Y., and Yoshida, H. (2021). Ribosomal hibernation-associated factors in Escherichia coli. Microorganisms 10:33. doi: 10.3390/microorganisms10010033
Maki, Y., Yoshida, H., and Wada, A. (2000). Two proteins, YfiA and YhbH, associated with resting ribosomes in stationary phase Escherichia coli. Genes Cells 5, 965–974. doi: 10.1046/j.1365-2443.2000.00389.x
McCarthy, B. J. (1960). Variations in bacterial ribosomes. Biochim. Biophys. Acta 39, 563–564. doi: 10.1016/0006-3002(60)90221-3
Mohr, S. M., Bagriantsev, S. N., and Gracheva, E. O. (2020). Cellular, molecular, and physiological adaptations of hibernation: the solution to environmental challenges. Annu. Rev. Cell Dev. Biol. 36, 315–338. doi: 10.1146/annurev-cellbio-012820-095945
Moore, B. D., and Seemann, J. R. (1994). Evidence that 2-carboxyarabinitol 1-phosphate binds to ribulose-1, 5-bisphosphate carboxylase in vivo. Plant Physiol. 105, 731–737. doi: 10.1104/pp.105.2.731
Morichaud, Z., Trapani, S., Vishwakarma, R. K., Chaloin, L., Lionne, C., Lai-Kee-Him, J., et al. (2023). Structural basis of the mycobacterial stress-response RNA polymerase auto-inhibition via oligomerization. Nat. Commun. 14:484. doi: 10.1038/s41467-023-36113-y
Morimoto, T., Blobel, G., and Sabatini, D. D. (1972). Ribosome crystallization in chicken embryos: II. Conditions for the formation of ribosome tetramers in vivo. J. Cell Biol. 52, 355–366. doi: 10.1083/jcb.52.2.355
Morono, Y., Ito, M., Hoshino, T., Terada, T., Hori, T., Ikehara, M., et al. (2020). Aerobic microbial life persists in oxic marine sediment as old as 101.5 million years. Nat. Commun. 11:3626. doi: 10.1038/s41467-020-17330-1
Nicholson, D., Salamina, M., Panek, J., Helena-Bueno, K., Brown, C. R., Hirt, R. P., et al. (2022). Adaptation to genome decay in the structure of the smallest eukaryotic ribosome. Nat. Commun. 13:591. doi: 10.1038/s41467-022-28281-0
Orr, D. J., Robijns, A. K., Baker, C. R., Niyogi, K. K., and Carmo-Silva, E. (2023). Dynamics of rubisco regulation by sugar phosphate derivatives and their phosphatases. J. Exp. Bot. 74, 581–590. doi: 10.1093/jxb/erac386
Parry, M. A., Keys, A. J., Madgwick, P. J., Carmo-Silva, A. E., and Andralojc, P. J. (2008). Rubisco regulation: a role for inhibitors. J. Exp. Bot. 59, 1569–1580. doi: 10.1093/jxb/ern084
Patterson, B. D., Payne, L. A., Chen, Y. Z., and Graham, D. (1984). An inhibitor of catalase induced by cold in chilling-sensitive plants. Plant Physiol. 76, 1014–1018. doi: 10.1104/pp.76.4.1014
Pei, H. H., Hilal, T., Chen, Z. A., Huang, Y. H., Gao, Y., Said, N., et al. (2020). The δ subunit and NTPase HelD institute a two-pronged mechanism for RNA polymerase recycling. Nat. Commun. 11:6418. doi: 10.1038/s41467-020-20159-3
Pereira, S. F., Gonzalez, R. L. Jr., and Dworkin, J. (2015). Protein synthesis during cellular quiescence is inhibited by phosphorylation of a translational elongation factor. Proc. Natl. Acad. Sci. 112, E3274–E3281. doi: 10.1073/pnas.1505297112
Polikanov, Y. S., Blaha, G. M., and Steitz, T. A. (2012). How hibernation factors RMF, HPF, and YfiA turn off protein synthesis. Science 336, 915–918. doi: 10.1126/science.1218538
Prossliner, T., Gerdes, K., Sørensen, M. A., and Winther, K. S. (2021). Hibernation factors directly block ribonucleases from entering the ribosome in response to starvation. Nucleic Acids Res. 49, 2226–2239. doi: 10.1093/nar/gkab017
Pullman, M. E., and Monroy, G. C. (1963). A naturally occurring inhibitor of mitochondrial adenosine triphosphatase. J. Biol. Chem. 238, 3762–3769. doi: 10.1016/S0021-9258(19)75338-1
Ranava, D., Scheidler, C. M., Pfanzelt, M., Fiedler, M., Sieber, S. A., Schneider, S., et al. (2022). Bidirectional sequestration between a bacterial hibernation factor and a glutamate metabolizing protein. Proc. Natl. Acad. Sci. 119:e2207257119. doi: 10.1073/pnas.2207257119
Rittershaus, E. S., Baek, S. H., and Sassetti, C. M. (2013). The normalcy of dormancy: common themes in microbial quiescence. Cell Host Microbe 13, 643–651. doi: 10.1016/j.chom.2013.05.012
Saba, JA, Huang, Z, Schole, KL, Ye, X, Bhatt, SD, Li, Y, et al. (2023). LARP1 senses free ribosomes to coordinate supply and demand of ribosomal proteins. bioRxiv [Preprint]. doi: 10.1101/2023.11.01.565189v1
Salvucci, M. E., and Crafts-Brandner, S. J. (2004). Mechanism for deactivation of rubisco under moderate heat stress. Physiol. Plant. 122, 513–519. doi: 10.1111/j.1399-3054.2004.00419.x
Sato, A., Watanabe, T., Maki, Y., Ueta, M., Yoshida, H., Ito, Y., et al. (2009). Solution structure of the E. coli ribosome hibernation promoting factor HPF: implications for the relationship between structure and function. Biochem. Biophys. Res. Commun. 389, 580–585. doi: 10.1016/j.bbrc.2009.09.022
Schmidt, A., Kochanowski, K., Vedelaar, S., Ahrné, E., Volkmer, B., Callipo, L., et al. (2016). The quantitative and condition-dependent Escherichia coli proteome. Nat. Biotechnol. 34, 104–110. doi: 10.1038/nbt.3418
Sharma, M. R., Dönhöfer, A., Barat, C., Marquez, V., Datta, P. P., Fucini, P., et al. (2010). PSRP1 is not a ribosomal protein, but a ribosome-binding factor that is recycled by the ribosome-recycling factor (RRF) and elongation factor G (EF-G) 2. J. Biol. Chem. 285, 4006–4014. doi: 10.1074/jbc.M109.062299
Sharma, H., Jespersen, N., Ehrenbolger, K., Carlson, L. A., and Barandun, J. (2024). Ultrastructural insights into the microsporidian infection apparatus reveal the kinetics and morphological transitions of polar tube and cargo during host cell invasion. PLoS Biol. 22:e3002533. doi: 10.1371/journal.pbio.3002533
Shetty, S., Hofstetter, J., Battaglioni, S., Ritz, D., and Hall, M. N. (2023). TORC1 phosphorylates and inhibits the ribosome preservation factor Stm1 to activate dormant ribosomes. EMBO J. 42:e112344. doi: 10.15252/embj.2022112344
Smith, P. R., Loerch, S., Kunder, N., Stanowick, A. D., Lou, T. F., and Campbell, Z. T. (2021). Functionally distinct roles for eEF2K in the control of ribosome availability and p-body abundance. Nat. Commun. 12:6789. doi: 10.1038/s41467-021-27160-4
Song, S., Gong, T., Yamasaki, R., Kim, J. S., and Wood, T. K. (2019). Identification of a potent indigoid persister antimicrobial by screening dormant cells. Biotechnol. Bioeng. 116, 2263–2274. doi: 10.1002/bit.27078
Taddei, C. (1972). Ribosome arrangement during oogenesis of Lacerta sicula Raf. Exp. Cell Res. 70, 285–292. doi: 10.1016/0014-4827(72)90138-3
Tissières, A., Watson, J. D., Schlessinger, D., and Hollingworth, B. R. (1959). Ribonucleoprotein particles from Escherichia coli. J. Mol. Biol. 1, 221–233. doi: 10.1016/S0022-2836(59)80029-2
Torreira, E., Louro, J. A., Pazos, I., Gonzalez-Polo, N., Gil-Carton, D., Duran, A. G., et al. (2017). The dynamic assembly of distinct RNA polymerase I complexes modulates rDNA transcription. eLife 6:e20832. doi: 10.7554/eLife.20832
Trauner, A., Lougheed, K. E., Bennett, M. H., Hingley-Wilson, S. M., and Williams, H. D. (2012). The dormancy regulator DosR controls ribosome stability in hypoxic mycobacteria. J. Biol. Chem. 287, 24053–24063. doi: 10.1074/jbc.M112.364851
Van Den Elzen, A. M., Schuller, A., Green, R., and Séraphin, B. (2014). Dom34-Hbs1 mediated dissociation of inactive 80S ribosomes promotes restart of translation after stress. EMBO J. 33, 265–276. doi: 10.1002/embj.201386123
Van Dyke, N., Chanchorn, E., and Van Dyke, M. W. (2013). The Saccharomyces cerevisiae protein Stm1p facilitates ribosome preservation during quiescence. Biochem. Biophys. Res. Commun. 430, 745–750. doi: 10.1016/j.bbrc.2012.11.078
Vila-Sanjurjo, A., Schuwirth, B. S., Hau, C. W., and Cate, J. H. (2004). Structural basis for the control of translation initiation during stress. Nat. Struct. Mol. Biol. 11, 1054–1059. doi: 10.1038/nsmb850
Vreeland, R. H., Rosenzweig, W. D., and Powers, D. W. (2000). Isolation of a 250 million-year-old halotolerant bacterium from a primary salt crystal. Nature 407, 897–900. doi: 10.1038/35038060
Wada, A., Yamazaki, Y., Fujita, N., and Ishihama, A. (1990). Structure and probable genetic location of a “ribosome modulation factor” associated with 100S ribosomes in stationary-phase Escherichia coli cells. Proc. Natl. Acad. Sci. 87, 2657–2661. doi: 10.1073/pnas.87.7.2657
Wang, Y. J., Vaidyanathan, P. P., Rojas-Duran, M. F., Udeshi, N. D., Bartoli, K. M., Carr, S. A., et al. (2018). Lso2 is a conserved ribosome-bound protein required for translational recovery in yeast. PLoS Biol. 16:e2005903. doi: 10.1371/journal.pbio.2005903
Wells, J. N., Buschauer, R., Mackens-Kiani, T., Best, K., Kratzat, H., Berninghausen, O., et al. (2020). Structure and function of yeast Lso2 and human CCDC124 bound to hibernating ribosomes. PLoS Biol. 18:e3000780. doi: 10.1371/journal.pbio.3000780
Yonath, A. (2010). Hibernating bears, antibiotics, and the evolving ribosome (Nobel lecture). Angew. Chem. Int. Ed. 49, 4340–4354. doi: 10.1002/anie.201001297
Yoshida, H., Maki, Y., Furuike, S., Sakai, A., Ueta, M., and Wada, A. (2012). YqjD is an inner membrane protein associated with stationary-phase ribosomes in Escherichia coli. J. Bacteriol. 194, 4178–4183. doi: 10.1128/JB.00396-12
Keywords: hibernation, stress, ribosome, Rubisco, RNA polymerase, dormancy
Citation: Helena-Bueno K, Chan LI and Melnikov SV (2024) Rippling life on a dormant planet: hibernation of ribosomes, RNA polymerases, and other essential enzymes. Front. Microbiol. 15:1386179. doi: 10.3389/fmicb.2024.1386179
Received: 14 February 2024; Accepted: 21 March 2024;
Published: 06 May 2024.
Edited by:
Jiqiang Ling, University of Maryland, College Park, United StatesReviewed by:
Alfredo Cabrera-Orefice, Goethe University Frankfurt, GermanyCopyright © 2024 Helena-Bueno, Chan and Melnikov. This is an open-access article distributed under the terms of the Creative Commons Attribution License (CC BY). The use, distribution or reproduction in other forums is permitted, provided the original author(s) and the copyright owner(s) are credited and that the original publication in this journal is cited, in accordance with accepted academic practice. No use, distribution or reproduction is permitted which does not comply with these terms.
*Correspondence: Sergey V. Melnikov, c2VyZ2V5Lm1lbG5pa292QG5jbC5hYy51aw==; Karla Helena-Bueno, ay5oZWxlbmEtYnVlbm8yQG5jbC5hYy51aw==; Lewis I. Chan, bC5pLmNoYW4xQG5ld2Nhc3RsZS5hYy51aw==
†These authors have contributed equally to this work
Disclaimer: All claims expressed in this article are solely those of the authors and do not necessarily represent those of their affiliated organizations, or those of the publisher, the editors and the reviewers. Any product that may be evaluated in this article or claim that may be made by its manufacturer is not guaranteed or endorsed by the publisher.
Research integrity at Frontiers
Learn more about the work of our research integrity team to safeguard the quality of each article we publish.