- Water, Energy and Environmental, Engineering Research Unit, University of Oulu, Oulu, Finland
Mining activities, even in arctic regions, create waste materials releasing metals and metalloids, which have an impact on the microorganisms inhabiting their surroundings. Some species can persist in these areas through tolerance to meta(loid)s via, e.g., metabolic transformations. Due to the interaction between microorganisms and meta(loid)s, interest in the investigation of microbial communities and their possible applications (like bioremediation or biomining) has increased. The main goal of the present study was to identify, isolate, and characterize microorganisms, from subarctic mine sites, tolerant to the metalloid antimony (Sb) and the metal copper (Cu). During both summer and winter, samples were collected from Finnish mine sites (site A and B, tailings, and site C, a water-treatment peatland) and environmental parameters were assessed. Microorganisms tolerant to Sb and Cu were successfully enriched under low temperatures (4°C), creating conditions that promoted the growth of aerobic and fermenting metal(loid) tolerating or anaerobic metal(loid) respiring organism. Microbial communities from the environment and Sb/Cu-enriched microorganisms were studied via 16S rRNA amplicon sequencing. Site C had the highest number of taxa and for all sites, an expected loss of biodiversity occurred when enriching the samples, with genera like Prauserella, Pseudomonas or Clostridium increasing their relative abundances and others like Corynebacterium or Kocuria reducing in relative abundance. From enrichments, 65 putative Sb- and Cu-metabolizing microorganisms were isolated, showing growth at 0.1 mM to 10 mM concentrations and 0°C to 40°C temperatures. 16S rRNA gene sequencing of the isolates indicated that most of the putative anaerobically Sb-respiring tolerators were related to the genus Clostridium. This study represents the first isolation, to our knowledge, of putative Sb-metabolizing cold-tolerant microorganisms and contributes to the understanding of metal (loid)-tolerant microbial communities in Arctic mine sites.
Introduction
Mining activities generate substantial amounts of waste materials and those can result in the release of metals and metalloids to ecosystems. Even in (sub-)arctic sites such as Svalbard, Alaska or Finland, metal(loid)s are present as a consequence of the mining industry (Perryman et al., 2020; Rudnicka-Kępa and Zaborska, 2021; Haddaway et al., 2022). Although metal(loid)s in waste materials might be potentially valuable resources (Falagán et al., 2017), when those pollutants end up in nature, they can negatively impact plants, animals or microbial communities inhabiting those sites (Ledin, 1996). Some microorganisms with the ability of tolerate metal(loid)s can colonize and inhabit highly contaminated places by using strategies such as metabolic pathways or resistance mechanisms (Newsome and Falagán, 2021). Because of the ability of certain microorganisms to transform metal(loid)s, there is an interest in studying microbial communities from mining contaminated sites and their potential industrial applications (Levett et al., 2021; Staicu and Stolz, 2021). However, there are still many gaps to fill in the knowledge on microorganisms from polluted places and their interaction with elements in nature, especially in extreme places, like arctic regions.
One example of mining-impacted sites are mine tailings, the waste or the material left over after the valuable component has been extracted (Roche et al., 2017). Because of the chemical properties of mine tailings, their environmental impact depends on their potential to release metal(loids)s into natural ecosystems (Jiang et al., 2021; Cacciuttolo et al., 2023). Another example of a place highly impacted by mining activities are the systems utilized for the purification of mining discharges, like peatlands used for passive mine water treatment purposes (Palmer et al., 2015). Due to the characteristics of peatlands, e.g., high water tables or large effective surface area, different metal(loids)s can accumulate in these environments through different pathways such as atmospheric deposition or contaminated groundwater (Steinnes and Friedland, 2006; Palmer et al., 2015; Eberle et al., 2021). Both tailings and mine water-treatment peatlands represent an ecological challenge due to the presence of toxic elements, but it could also serve as a potential sources of valuable minerals which, if not reutilized, result in economic loss (Falagán et al., 2017; Sethurajan et al., 2018; Aznar-Sánchez et al., 2019).
Microorganisms can be used for several industrial purposes. For instance, microorganisms can be used in bioremediation, a method to biologically remove contaminants from ecosystems (Dixit et al., 2015; Malla et al., 2018). This technique has been previously studied and applied in mining-affected environments in an attempt to return the ecosystems to their natural state (Newsome and Falagán, 2021; Bala et al., 2022; Riseh et al., 2023; Sánchez-Castro et al., 2023). Microorganisms can also be used for biomining processes, the extraction of metals of interest from their ores aided through biological processes such as bioleaching or bioaccumulation (Jerez, 2017). Microbial contribution in the obtention of metal(loid)s has been demonstrated, including recovery from mine waste materials (Gao et al., 2021; Chen W. et al., 2022). In the mine areas we had access to in our study, antimony (Sb) and copper (Cu) are of particular interest. Antimony is an element that has been listed as a priority pollutant internationally (United States Environmental Protection Agency, 1997; European Parliament, Council of the European Union, 2020) and a critical raw material for the EU (Grohol et al., 2023). The awareness towards Sb has grown in the past years because of its detrimental effects to humans and the environment (Bolan et al., 2022; Periferakis et al., 2022). Industrial operations, such as mining, contribute to the release of Sb to surrounding native areas (Qi et al., 2022). However, Sb is a non-renewable and valuable element whose importance is expected to continue increasing because of its role in the energy transition (Li et al., 2022). Another example of precious raw material is Cu. Nowadays, one of the concerns in the mining industry is that usually small traces of Cu, which were not extracted in the first place, are left in the material after the extractive operations (Loredo Portales et al., 2015; Falagán et al., 2017). Thus, efforts are needed to remove Sb, from mine sites to reduce contamination of natural ecosystems and to develop alternative supplies of Sb and Cu, like recovering less available and less concentrated Sb and Cu from mine waste (Falagán et al., 2017; Sun et al., 2018; Grohol et al., 2023).
Different approaches have been described for Sb bioremediation like antimonite [Sb(III)] oxidation which decreases the Sb toxicity (Hamamura et al., 2013; Li et al., 2019) or antimonate [Sb(V)] reduction and subsequent precipitation (Wang et al., 2013, 2018; Zhu et al., 2018). However, regardless of its promising significance, little is known about microorganisms that participate in Sb(V) reduction when comparing to, e.g., Sb(III) oxidation (Li et al., 2019). In fact, only a few Sb(V) reducing microbes have been isolated (Chen Y. et al., 2022), with the first organisms described only a decade ago (Kulp et al., 2014). The relationship between Sb and microorganisms has been studied for other applications as well. For example, it has been proven that microorganisms can contribute in the recovery of Sb from mining ores through bioleaching (Hafeez et al., 2017; Aghazadeh et al., 2023). However, Cu is the metal which has been most extensively researched for biomining. For instance, Cu biomining systems have been applied internationally (Jerez, 2017; Yin et al., 2018; Vera et al., 2022). In addition, Cu-recovery from waste materials has also been developed (Falagán et al., 2017; Chen W. et al., 2022). Several species isolated from mine sites have been reported for their bioleaching activities of Cu (Kumar and Nagendran, 2007; Govarthanan et al., 2014), and most of them are known to be able to withstand very high Cu concentrations (Dopson et al., 2003; Orell et al., 2010). Nonetheless, despite the mentioned studies assessing Sb and Cu-tolerant microorganisms, only a few species have been isolated from cold environments or reported growth at low temperatures. From our knowledge, no psychrophilic microorganism [with an optimal growth temperature below 15°C (Morita, 1975)] or psychrotolerant microorganism [capable of growing at temperatures up to 0°C but with optimum growth temperature at 20°C or higher (Morita, 1975)] with Sb-resistance has been isolated. In addition, only a small number of studies have focused on characterizing psychrotolerant Cu-resistant microorganisms (Barahona et al., 2014; Muñoz-Villagrán et al., 2022). Therefore, with the rise of mining operations in Northern regions such as Finland, one of the top countries in the world in terms of mineral exploration (Tuusjärvi et al., 2014; Mejía and Aliakbari, 2023), research should focus on isolating, identifying and characterizing psychrophilic and psychrotolerant Sb and Cu-metabolizing microorganisms, as they must withstand the challenging temperatures of the mining industry in the Arctic as well as metal(loid) concentration to be viable for in-situ systems applications.
The goal of this study was to assess the diversity of microorganisms from mining-impacted sites in subarctic regions and isolate microorganisms that have potential for bioremediation and biomining applications. The aims of our study were to (1) compare microbial communities from different mining environments and the environmental parameters shaping the microbial community structure, (2) enrich and isolate microorganisms from environmental samples which have tolerance for our metals of interest, Sb and Cu, under cold conditions (4°C), (3) characterize the isolated microorganisms, and (4) asses their potential for further biotechnology applications. To address these aims, we obtained samples from mining contaminated sites in Northern and Central Finland in different seasons and studied their microbial communities via 16S rRNA gene sequencing and the influence of environmental parameters on them. Antimony and Cu tolerant microorganisms were selectively enriched in cold, in-situ relevant temperatures and subsequently isolated and characterized. Our study adds to the understanding of cold-adapted microorganisms from mining impacted environments and their potential use in biotechnology.
Materials and methods
Sampling and physicochemical analyses of environmental samples
Samples were obtained from three Finnish mine areas: one tailing from an abandoned mine (site A), one tailing from an active mine (site B) and one peatland used for mine water treatment in an active mine (site C) (Figure 1). Sites were selected based on the chemical element composition, with special emphasis on the Sb and Cu concentrations.
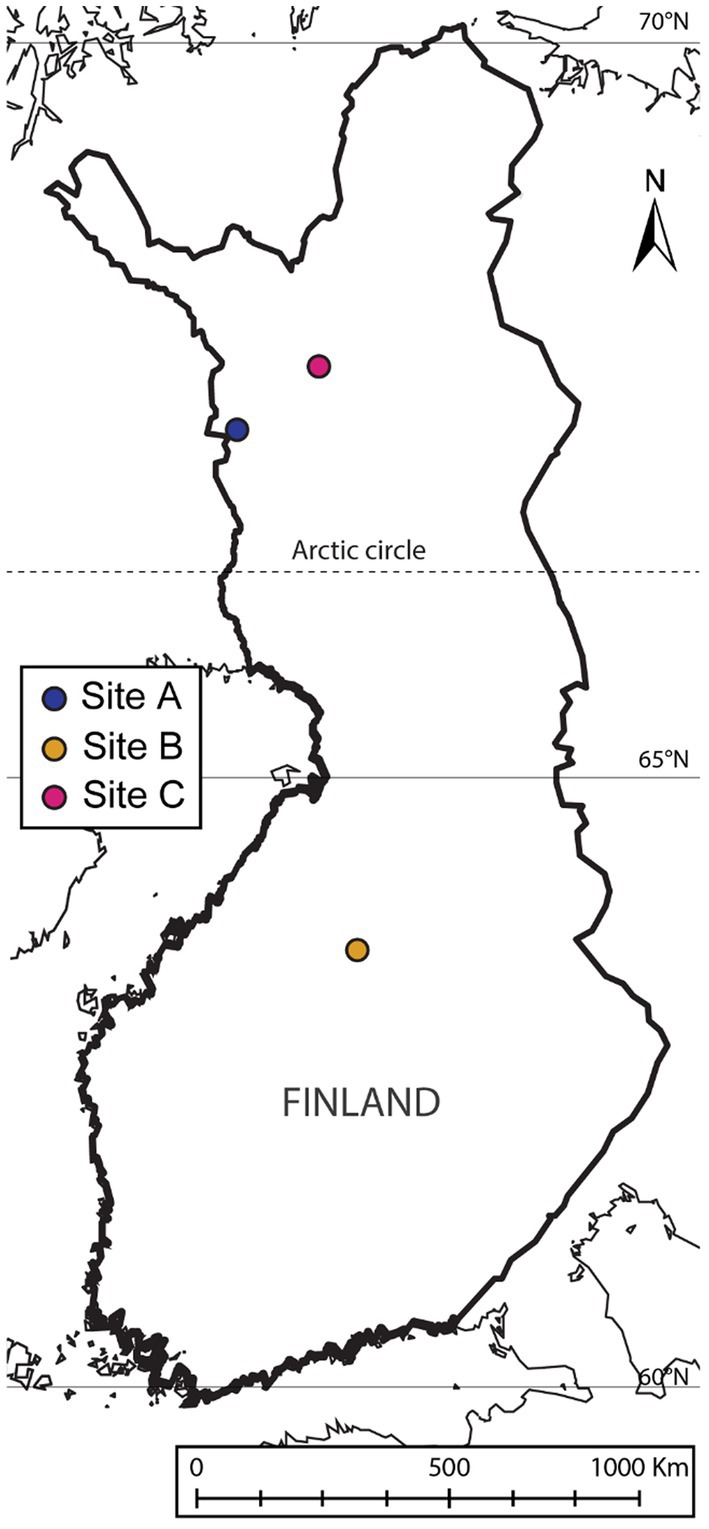
Figure 1. Sampling locations. Figure made with ArcGisPro [using basemap “Light Gray Canvas Map” (source: Esri, DeLorme, HERE, MapmyIndia) and layers “World Latitude and Longitude Grids” and “World GeoReference Lines” (Esri Data and Maps)].
Summer sampling of site B was conducted at the end of August (24.08.2021) and sites A and C beginning of September (01.09.2021), while winter sampling for all sites took place in February (16.02.2022 for A, 08.02.2022 for B, and 15.02.2022 for C). Environmental samples were collected at different depths within the soil profile by excavating holes using a shovel, with one hole in site A and C and two holes in site B. During winter sampling, an ice drill was used to penetrate the superficial frozen layer. All samples were transported for no more than three hours in a cold box at 4°C until they were frozen and kept at −20°C until further analysis. Three subsamples were taken for all sites/depths/sampling occasions: one of the subsamples was used to determine pH, one subsample was used to determine elemental composition at a commercial laboratory (Eurofins Ahma Oy) and one subsample was used for DNA and RNA extraction and, in the case of the summer samples, as inoculant for the enrichment experiments. The week following each sampling total elemental concentrations of Sb, Cu, arsenic (As), sulfur (S) and iron (Fe) were determined after acidic microwave digestion by inductively coupled plasma mass spectrometry (ICP-MS) in a commercial laboratory (Eurofins Ahma Oy). The pH was measured in a 1:2 (w/v) aqueous solution using a pH meter (InoLab® Multi 9,420 IDS).
Enrichment and isolation of Sb- and Cu- tolerant microorganisms
Microbes from the environmental samples collected in summer were cultivated in three different growth media to selectively enrich Sb and Cu utilizing microorganisms: aerobic Sb or Cu tolerators, fermenting Sb or Cu tolerators and anaerobically Sb or Cu respiring microorganisms. Media were prepared using an artificial porewater solution containing essential minerals, trace elements, and vitamins [modified from Balch et al. (1979) and Kuhner et al. (1996)]. Media for aerobic and fermenting Sb and Cu tolerators was supplemented with 1:10 diluted nutrient broth as carbon source and media for anaerobic Sb and Cu respires with 1:100 diluted nutrient broth as well as sodium acetate and sodium lactate (5 mM each) as carbon source. The pH of all growth media was adjusted to 6.5. Anoxic media for fermenting tolerators and anaerobic respirers were prepared using modified Hungate techniques (Wüst et al., 2009). After autoclaving the media, 0.2 μm-filtered stock solutions of Sb or Cu were added to reach final concentrations of 1 mM. Antimonite (supplemented as Sb2O3) was used for the cultures of aerobic antimony-tolerant microorganisms, while antimonate (supplemented as H6KO6Sb) was added as a potential electron acceptor for fermenting antimony-tolerators and anaerobical antimonate respirers. Copper (supplemented as CuSO4) was utilized for the medium of aerobic and fermenting copper-tolerators as well as anaerobic copper- or sulfate-reducer, which would potentially use copper [as Cu(II)] or sulfate (SO42−) as an electron acceptor. To prepare the sample for inoculation, 1 g of content from each of the environmental samples were placed in test tubes with 9 mL of distilled water (1:10 dilution) and shaken for ten minutes.
After the media were prepared, two approaches were used: enrichment culturing and dilution-to extinction protocols. For the enrichment culturing, 0.5 mL of each of the environmental solutions were transferred to 15 mL media contained in either 50 mL falcon tubes (aerobic) or sealed glass tubes (anoxic). For the dilution-to-extinction approach, 96-well plates were inoculated with different dilutions (10−3, 10−4 and 10−5) of the environmental suspension. In each well, 250 μL of enriched growth medium was inoculated with 25 μL from the dilution series and one well with sterile water to serve as negative controls. Aerobic plates were incubated in ambient air, and anoxic plates were incubated under anoxic conditions in air-tight containers with AnaeroGen pouches (Thermo Fisher Scientific, Walthan, MA, United States) to generate an anoxic atmosphere (Supplementary Figure S1). For each target group (aerobic Sb or Cu tolerators, fermenting Sb or Cu tolerators, Sb or Cu respirers) 8 tubes (1 per environmental sample) and 4 plates (1/2 plate per environmental sample) were prepared. All tubes and plates were incubated at 4°C for three weeks or until turbidity was observed and transferred to new tubes or plates. After three rounds of transfers, 35 tubes and 85 wells on the plates presented turbidity (from now on called enrichments) and the supplemented Sb(III), Sb(V) and Cu(II) were dissolved in the media. and 100 μL of growth medium was transferred to solid media using the spread plate technique. Plates were incubated at 4°C for three weeks or until colony growth was observed (Supplementary Table S4), and colonies were transferred onto fresh plates at least three times using the streak plate technique in order to isolate them. Isolates were tested in 96-well plates at different temperatures and concentrations to study their growth. The media used for the plates varied according to the isolates and was prepared using the same conditions as mentioned before. Growth was tested at five temperatures (0°C, 5°C, 10–15°C, 20°C, 30°C) in with final concentrations of 100 μM of Sb(V) or 1 mM of Cu(II) in the medium. Impact of concentration was tested with three concentrations of Sb and Cu (“low” = 100 μM, “medium” = 1 mM and “high” = 10 mM) at room temperature. In each case, isolates and a negative control (without microbial inoculation) were inoculated in triplicates. Microbial growth was measured regularly on the spectrophotometer (absorbance 600 nm).
Sequencing and sequencing analyses
To study microbial communities present in the environment and enrichment, nucleic acids from the samples were co-extracted using an optimized protocol that mitigates inhibitor effects (Lim et al., 2016). For environmental samples, extraction occurred within one month of sampling, targeting for DNA and RNA to evaluate total and active microbial communities respectively, as done in previous ecological studies (Baldrian et al., 2012; Zhang et al., 2014, 2021). Conversely, extraction of enrichment samples was conducted immediately prior to their transfer to solid media, focusing solely on DNA. Each of the co-extracted samples was divided into two eppendorfs that were stored at −20°C and − 80°C to later on purify the DNA or RNA. To remove RNA from the DNA samples, a RNase treatment was performed using a diluted RNase (100 μg/mL) (Thermo Scientific™) and to eliminate genomic DNA from the RNA samples a DNase treatment was done using DNase Max Kit (Thermo Scientific™). Complementary DNA (cDNA) [qScript™ cDNA synthesis Kit (Quantabio)] was generated in the case of RNA samples. The V3-V4 region of the 16S rRNA gene was amplified from DNA and cDNA using universal primers 341F (CCTACGGGNGGCWGCAG) and 805R (GACTACHVGGGTATCTAATCC) (Herlemann et al., 2011) and sequenced on an Illumina MiSeq platform with 300 bp paired-end reads at Macrogen Europe. Regarding the isolates, DNA was extracted from the isolates using NucleoSpin DNA Microbial 740235.50 from MACHEREY-NAGEL©. After the extraction, DNA was amplified via 16S rRNA gene PCR using universal primers 27F and 1492R 27F (AGAGTTTGATCCTGGCTCAG) and 1492R (TACGGYTACCTTGTTACGACTT) (Lane, 1991) and sequenced via sanger sequencing at a commercial laboratory (Macrogen Europe). Raw sequence data of the environmental and enrichment samples were deposited into the NCBI Sequence Read Archive (SRA) under BioProject accession PRJNA1079661 and sequencing data of the isolates into NCBI GenBank under project accession PP448086-PP448136.
For the environmental and enrichment samples, diversity profiling analysis were performed with QIIME 2 2022.2 (Bolyen et al., 2019). The demultiplexed raw reads were primer trimmed, quality filtered, denoised and merged with DADA2 [Divisive Amplicon Denoising Algorithm 2 (Callahan et al., 2016)] with truncation length of 270 and 220 and trimmed length of 16 and 21 for the forward and reverse read sequence, respectively. Taxonomy was assigned to the ASVs (Amplicon Sequence Variants) using the classifier SILVA 138 (Quast et al., 2012). Phyla names were given according to the most updated taxonomy classification rule (Oren and Garrity, 2021). For each sample, phyla and genera above and below 5% abundance were considered “high” and “low” relative abundance, respectively. Rarefaction depth was settled at 21177 reads to ensure all samples were included in the analysis (reads per sample ranged from 21177 to 58267). Artifacts from QIIME2 were exported to R (version 4.2.3 2023-03-15) and a phyloseq object was created via the R package QIIME2R and phyloseq (v0.99.20 and v1.42 respectively). Enrichment and environmental sequencing data was analyzed with R packages ape (v5.7–1), ddplyr (v1.1.1), lme4 (v1.1–32), tidyr (v1.3.0), vegan (v2.6–4) and tidyverse (v2.0.0). R package microbiome (v1.21.1) and picante (v1.8.2) were used to study Faith Phylogenetic Diversity, Observed ASVs (species richness), Rarity (log_modulo_skewness) (species richness of the less abundant species) and Shannon Diversity. Forward and reverse sequences of the isolate samples were aligned with MEGA X software version 10.0.5. Neighbor-sequence alignment of the isolates was done in SILVA ACT (FastTree program, 0.95 min. Identity with query sequence) (Quast et al., 2012). The alignment was exported and a phylogenetic tree was constructed with maximum likelihood and using the Maximum Likelihood method and Tamura-Nei model (Tamura and Nei, 1993) and Neighbor-Join and BioNJ algorithms in MEGA X (Kumar et al., 2018). Most abundant ASVs of the enrichments as well as isolate sequences were also classified according to next-cultured relative in BLAST search using the NCBI database (Altschul et al., 1990). In addition, Isolate sequences were aligned with their respective enrichment and environmental sample to search for possible matches (identity >99%). For the heatmap, the tree was exported from SILVA ACT and modified (branch.length = ‘none’) in R [ggtree (v.3.6.2)]. Isolate sequences were also aligned with their respective enrichment and environmental sample to search for possible matches (identity >99%). Statistical analyses of the environmental parameters of the samples were done in R. T-test was used to find significantly different values from the mean of each parameter and ANOVA test was used to find significantly differences in the values obtained from each site. Significant p-values were considered from * ≤ 0.1, ** ≤ 0.01, and *** ≤ 0.001. All figures were generated in R [packages ggplot 2 (v3.4.2), ggVennDiagram (1.2.2)] and edited with Adobe Illustrator 2022.
Results
Sampling and physicochemical analyses of environmental samples
A total of 18 environmental samples were obtained in the summer (8) and winter (10) (Table 1). Differences in the environmental parameters of the samples were statistically tested (Supplementary Table S1). When comparing each sampling site, no significant differences were found in pH and As concentrations, but significant differences were found in Sb, Cu, Fe and S concentrations. The pH was near-neutral in site A and C, while more variation was found in each of the cores of site B. Antimony was nearly only found in site C with concentrations 75 times higher than the mean of the other sites, copper concentrations were significantly higher in site B, with an average three times higher than the mean of the other sites and As concentrations were relatively similar in all sites.
Microbial communities from mining environments
DNA and RNA were extracted from 18 environmental samples for 16S rRNA amplicon sequencing. A total of 5,619 ASVs were detected in all environmental samples. Most of the ASVs were detected exclusively in site C (79%), followed by site A (7.9%) and site B (7.1%). Some ASVs were detected in multiple sites, such as A and C (4.5%), A and B (0.89%) and site B and C (0.11%). In addition, 18 ASVs were detected in all sampling sites (0.32%) (Supplementary Figure S2).
Differences were found in the microbial community structure of the environmental samples when analyzed by nonmetric multidimensional scaling (NMDS) (Figure 2). Samples from site A and B form a tight cluster, whereas samples from site C exhibit a more dispersed pattern with a slight seasonal clustering effect.
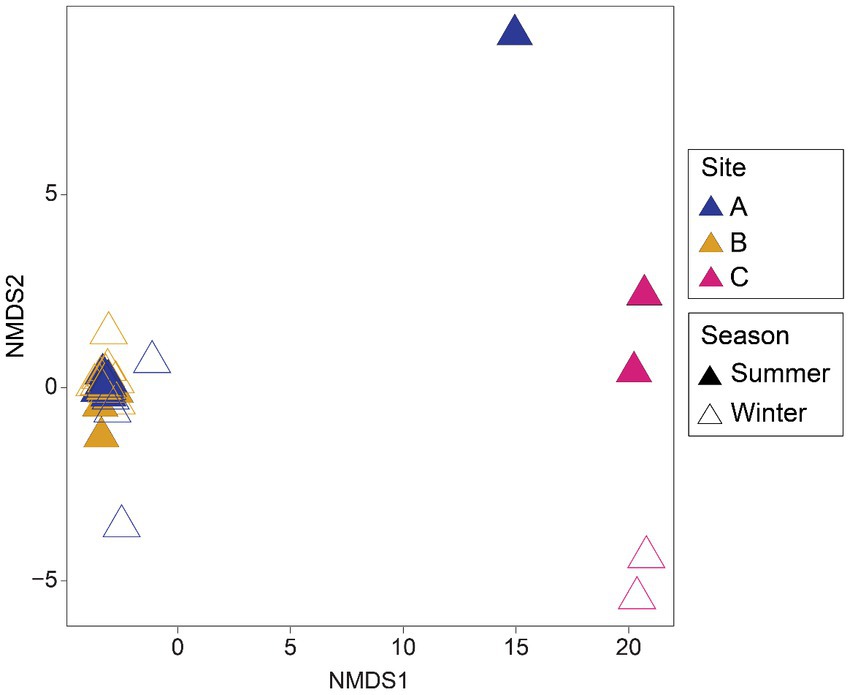
Figure 2. Nonmetric multidimensional scaling (NMDS) ordination of microbial assemblages from environmental samples by sites and seasons of sampling. Plot based on Bray-Curtis distances calculated from rarefied ASV tables.
Microbial diversity was significantly higher in samples from site C than from the other two (Supplementary Tables S2, S3). Observed ASVs from site C were 28 times higher than the average of sites A and B, Shannon diversity was two times higher, rarity index 49 times higher and phylogenetic diversity 19 times higher. In contrast, no significant differences were found for microbial diversity in different seasons (summer vs. winter), depth (frozen vs. surface vs. middle vs. bottom) or nucleic acid type (DNA vs. RNA) at each site. Differences between the sites were also reflected in the taxonomic composition of the microbial communities (Figure 3 and Supplementary Figure S3). In site A and B, Actinobacteriota was the dominant phylum (44% in both sites), followed by Bacillota (24 and 28%, respectively) and Pseudomonadota (24 and 25%, respectively), while in site C Pseudomonadota was the most abundant phylum (36%), followed by Bacteroidota (14%) and Chloroflexota (9%). Genera like Corynebacterium and Kocuria were only found in seven RNA samples from sites A and B but not in site C. The representation of the low-abundant phyla was <3% in samples from sites A and B and 13% in samples from site C. When looking at genus level, differences in sites A and B were slightly more pronounced than at phylum level. Dominant genera in sites A and B were Prauserella (22%), Escherichia-Shigella (16 and 20% respectively) and Alteribacillus (11 and 12%) but most of genera were of low abundance (40 and 36%). In contrast, in site C almost all the genera (93%) were those with low relative abundances.
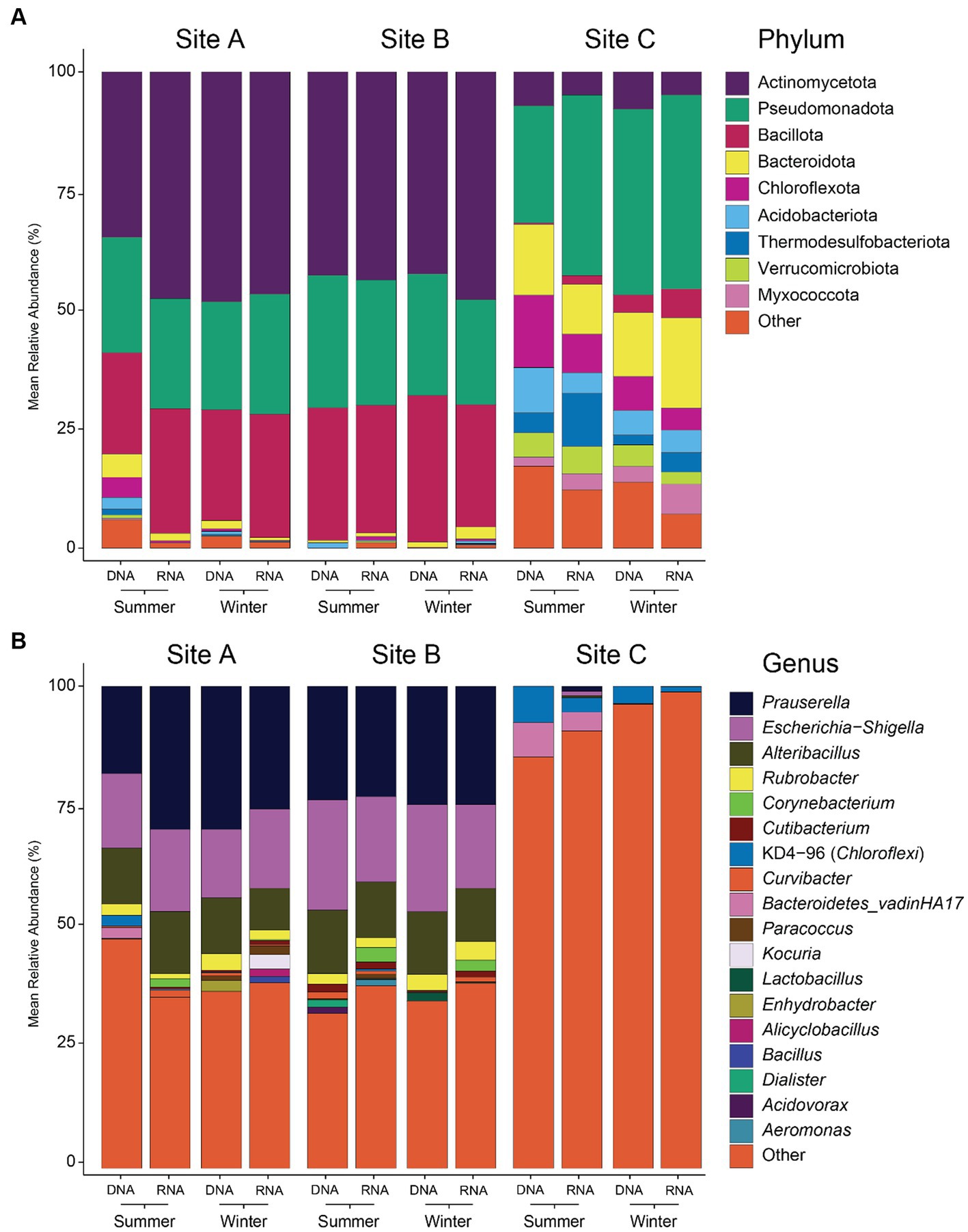
Figure 3. Microbial composition of environmental samples at phylum (A) and genus (B) level. Phyla and genera with a mean relative abundance of less than 5% were grouped in “Other.” Taxonomy classification based on 16S rRNA amplicon sequencing and SILVA database.
Enrichment of Sb-/Cu- tolerant microbes from mining environments
Environmental samples from the summer were inoculated to tubes and 96-well plates with different enrichment media. A total of 771 ASVs were obtained from sequencing the enrichment samples, but none were found in all enrichments. Some ASVs identified in the enrichments (122, 16%) were also present in the environmental samples, while the majority of ASVs (649, 84%) were exclusively detected in the enrichments. ASVs shared between environmental and enrichment samples belonged mostly to phylum Bacillota, Pseudomonadota and Actinomycetota (Supplementary Figure S4).
Microbial community structure (Figure 4) revealed a subtle distinction between the environmental and enrichment samples from site C. In addition, there appeared to be an overlap between some environmental and enrichment samples from site A and B.
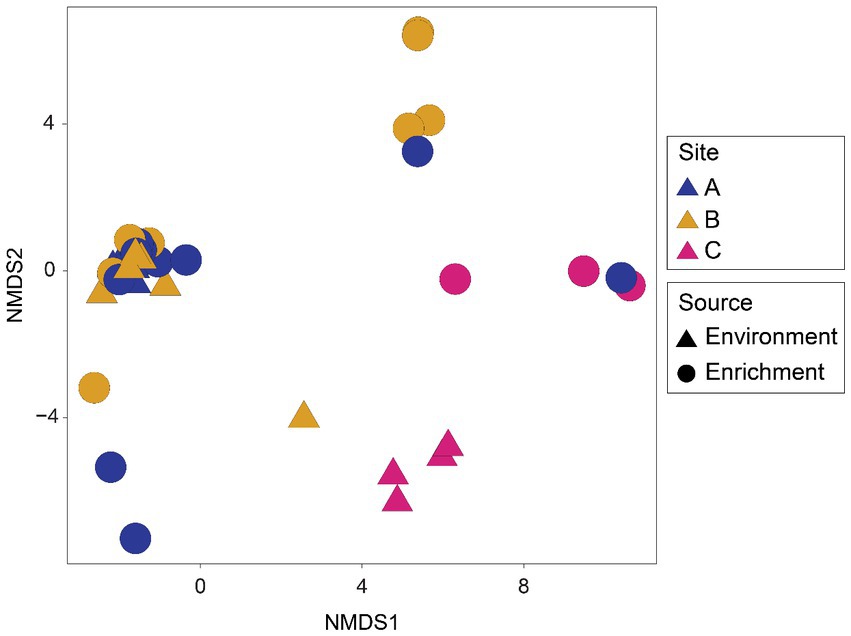
Figure 4. Nonmetric multidimensional scaling (NMDS) ordination of microbial communities from environmental (DNA and summer) and enrichment samples. Plot based on Bray-Curtis distances calculated from rarefied ASV tables.
Considering all the enrichment samples (except the anaerobic Sb-cultures, whose sequencing was not carried out), differences were found in the microbial composition of the enrichments according to different sites (Figure 5 and Supplementary Figure S3). In enrichments from sites A and B, Actinobacteriota was the dominant phylum (35 and 36% respectively), followed by Bacillota (32 and 31%, respectively) and Pseudomonadota (27 and 26%, respectively), while in enrichments from site C the dominant phylum was Pseudomonadota (46%), followed by Bacteroidota (21%), and Bacillota (20%). On genus level, enrichments from sites A and B were dominated by Prauserella (12 and 16%, respectively) and Escherichia-Shigella (8 and 16%, respectively) but most of the taxa belong to genera with relative abundances <1% (22 and 28%, respectively). In contrast, the most abundant genus in enrichments from site C was Pseudomonas (17%), followed by Clostridium (11%) and Yersinia (9%) and most genera had a relative abundance <1% (22%). Corynebacterium, which was abundant in environmental samples, was only found in small proportions in enrichments from sites A and B while Kocuria was not detected in any of the samples.
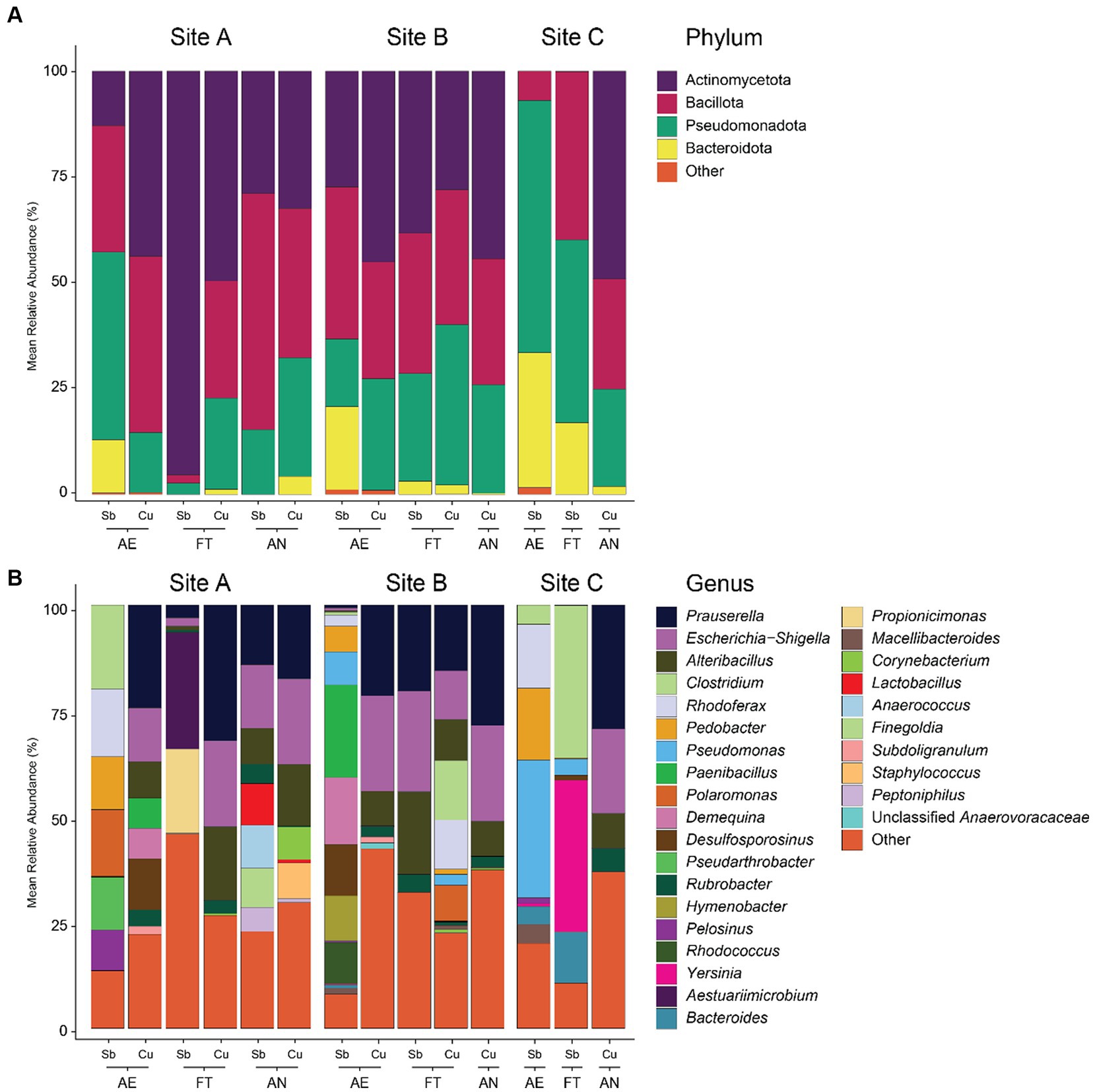
Figure 5. Microbial community composition of enrichment samples at phylum (A) and genus (B) level. Aerobic tolerators are described as AE, fermenting tolerators as FT and anaerobically respiring as AN. Antimonite (1 mM) was used for the cultures of aerobic antimony-tolerant microorganisms, antimonate (1 mM) for the fermenting antimony-tolerators and anaerobical antimonate respirers and copper sulfate (1 mM) for the aerobic and fermenting copper-tolerators as well as anaerobic copper- or sulfate-reducer. Phyla and genera with a mean relative abundance of less than 5% were grouped in “Other.” The genera “Clostridium_sensu_stricto_13” and “Clostridium_sensu_stricto_9” were grouped together as “Clostridium.” Taxonomy classification based on 16S rRNA amplicon sequencing and SILVA database. The microbial composition presented in this figure represents the results of three rounds of enrichment transfers, with each round involving 3 weeks of culturing.
In the enrichments, ASVs with >10% mean relative abundance were considered dominant taxa. Almost half of the 25 dominant ASVs (12) were detected in the environmental samples, and three of them were considered abundant in at least one of the environmental samples (Figure 6). Dominant ASVs (based on SILVA taxonomy classification) were plotted in a heatmap, and a phylogenetic tree was added to infer phylogeny. Next-cultured relative from BLAST search is found in Supplementary Table S5.
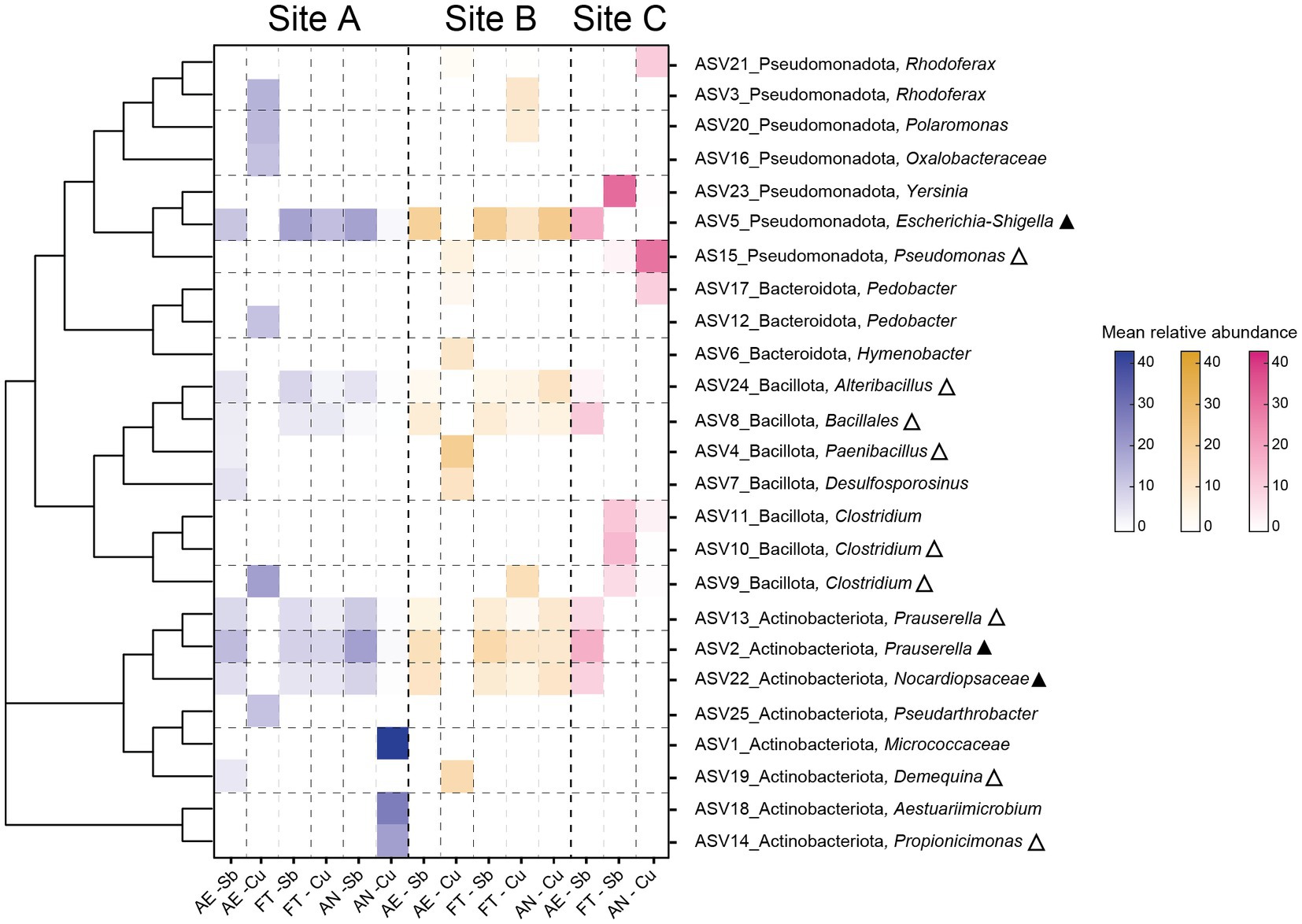
Figure 6. Heat map representing dominant ASVs (>10% mean relative abundance) in the enrichments. Aerobic tolerators are described as AE, fermenting tolerators as FT and anaerobically respiring as AN. Triangles next to the taxa indicate that the ASV is also present in at least one of the environmental samples (blank triangle) or that the ASV is dominant in at least one of the environmental samples (filled triangle).
Isolation of putative Sb and Cu-metabolizing isolates
A total of 65 putative Sb and Cu-metabolizing microbes were isolated from the enriched samples. Based on the media of isolation, 26 isolates were aerobic -tolerators, 9 Sb-fermenting tolerators, 18 anaerobic Sb-respirers and 12 aerobic Cu-tolerators. No fermenting Cu tolerator or anaerobic Cu- or sulfate-reducer was obtained. Sequences of 13 isolates were not obtained due to low results of amplification and/or bad sequencing quality. 52 isolates were successfully identified based on their 16S rRNA gene sequences and mapped onto a phylogenetic tree (Figure 7). Most isolates sequences (31) revealed less than 99.2% identity to cultured representatives and few of them (4) showed less than 97.5% (Supplementary Table S6). The majority of isolates were associated with phyla Pseudomonadota (26), Bacillota (13), Actinomycetota (7) and Bacteroidota (6). Many of the Bacillota isolates came from the anaerobic enrichments [fermenting Sb-tolerators (1) and anaerobic Sb-respirers (11)] and most of the Pseudomonadota isolates came from the aerobic enrichments [aerobic Sb-tolerators (14) and aerobic Cu-tolerators (6)]. Bacteroidota were only isolated in aerobic Sb-tolerator enrichments.
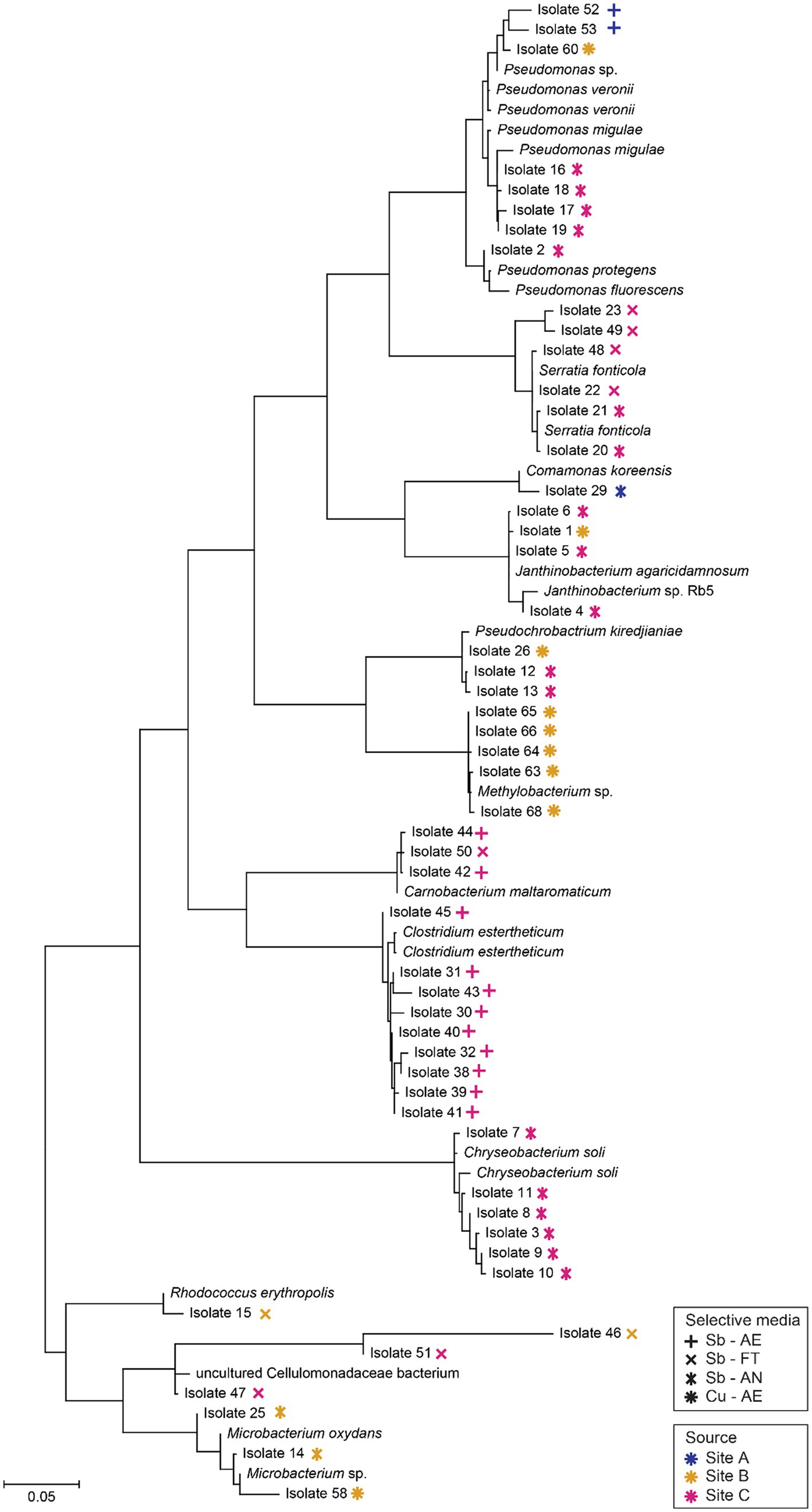
Figure 7. Phylogenetic tree of 16S rRNA gene sequences of putative Sb-/Cu-metabolizing bacterial isolates (according to their enriched media and site of origin) and their closest neighbors. Sequencing alignment was done in SILVA and tree was constructed in MEGA X.
Regarding the alignments between the isolates and environmental samples, ten isolates from site C matched at least one of the ASVs of their respective environmental sample, but no matches were found between the isolates from sites A and B and their environmental sample. Concerning the alignments between the isolates and the enrichment samples, nine isolates matched at least one of the ASVs from their respective aerobic Sb-tolerators enrichment and six isolates from the fermenting Sb-tolerators enrichment. No isolates matched an ASV from both, their respective enrichment and environmental sample (Supplementary Table S6).
The impact of temperature and Sb or Cu concentration on growth was determined for 63 isolates. While all the isolates demonstrated at least partial growth at the lowest temperature and the majority (70%) exhibited growth at the highest temperature, neither the lowest nor the highest temperatures were optimal for any of them. Most of the aerobic isolates (76%) had an optimum temperature of 20°C, in contrast with the anaerobic isolates, most of which (95%) had an optimum temperature below 20°C. All isolates were capable of growing in the smallest Sb or Cu concentration (0.1 mM) and most of them (81%) grew as well in the highest concentration (10 mM), but the preferable Sb or Cu concentration for the majority of them (79%) was the lowest one.
Discussion
Biotechnology potential of putative Sb-/Cu-tolerant cold-adapted microorganisms
There is an increasing interest in studying microbial communities from mining contaminated sites because of their ability to transform metal(loid)s and their potential in industrial applications (Levett et al., 2021; Newsome and Falagán, 2021; Staicu and Stolz, 2021). One approach of Sb-bioremediation consists of Sb(V) reduction and subsequent precipitation of the formed Sb(III) (Wang et al., 2013, 2018; Zhu et al., 2018). However, only few Sb-resistant species have been isolated, and much more is known about Sb(III)-oxidizing than about of Sb(V)-respiring microorganisms (Li et al., 2019; Shi et al., 2019; Chen Y. et al., 2022). This lack of knowledge is especially the case for Sb-resistant psychrophilic and psychrotolerant microorganism, which are of particular interest for their in-situ applicability in bioremediation systems of Arctic mining environments. In this study, 65 putative Sb- and Cu- metabolizing microorganisms from subarctic mine sites were enriched and isolated at 4°C in three different media selecting for: aerobic tolerators, fermenting tolerators and anaerobically respiring microorganisms. Considering that previous studies have determined the values of Sb(III) and Cu(II) that inhibit microbial growth of model bacteria such as Escherichia coli (Yamamoto and Ishihama, 2005; An and Kim, 2009) to be >0.1 mM for Sb(V) and > 1 mM for Cu(II), it is therefore asserted that all the microorganisms isolated in this study exhibit a certain degree of resistance to Sb or Cu, as they were all capable of growing at concentrations higher than 0.1 mM and a portion of them even at 10 mM. While no tests were conducted to assess the metabolic capabilities of the candidates, few hints suggest their potential for Sb/Cu-metabolizing abilities. For instance, the color of certain colonies changed after being inoculated to plate or a halo surrounding colonial growth appeared after inoculation (Supplementary Figure S1). Also, although the final enrichments for putative Sb-fermenting tolerators and putative anaerobically Sb-respiring microorganisms were supplemented with Sb(V), no growth was observed when supplementing them with Sb(III) (Supplementary Table S4). In addition, the metal(loid)s supplemented in the media, including the Sb(V) which took longer than the rest and whose particles were initially visible, were dissolved in all the liquid culture after weeks of inoculation, indicating there is a biochemical change occurring in the cultures. Therefore, while not the primary focus of this paper, several indications throughout the enrichment and isolation processes suggest the potential of the isolated microbes to utilize Sb(V) as an electron acceptor or Cu(II) as part of their metabolism. Considering the challenging environmental conditions of Arctic mines in terms of temperature and metal(loid) concentrations, microorganisms isolated in this study emerge as promising candidates for bioremediation applications within in-situ systems of (sub-)Arctic mines. Further tests with the isolates are needed to confirm their metabolizing capabilities and feasibility in the system but findings from this study represent the first step in the process to establish a metal(loid) immobilization system under low temperatures.
New metal(loid)-tolerance insights into known environmental microorganisms
At taxonomic level, most of the putative anaerobic Sb(V)-respiring isolates were related to Clostridium estertheticum, an anaerobic bacterium which was firstly isolated from vacuum-packed beef (Collins et al., 1992) and was classified as a true psychrophile when isolated from an Antarctic microbial mat (Spring et al., 2003). When the genome of C. estertheticum was studied (Yu et al., 2016), metal-resistance genes were found. It is particularly interesting the identification of arsBDR and pstB, genes which confer resistance to As and Sb (Carlin et al., 1995; Li et al., 2016), two metalloids that are often associated in the nature. However, from our knowledge, no C. estertheticum has been isolated from Sb-respiring cultures. Apart from the genus Clostridium, the other putative anaerobic Sb-respiring isolates were related to the genus Pseudomonas. Pseudomonas spp. have been reported for their ability to reduce a wide variety of metals (Blake et al., 1993), potentially including the reduction of Sb(V) (Lai et al., 2016; Sun et al., 2021). In particular, one of the isolates was related to Pseudomonas veronii, a metal tolerant species of this genus, which has been studied for bioremediation (Malla et al., 2018) and whose genome has been found to have the heavy metal resistance gene arsBDR (Morales et al., 2016). Furthermore, As-tolerant Pseudomonas strains have been previously isolated from same location as site C (Ziegelhöfer and Kujala, 2021). However, unlike the strains presented in this study, the previously reported Pseudomonas showed an optimum growth at 20–28°C, implying this might be a different isolated strain as the optimum temperatures for the Pseudomonas presented here was 10°C. Finally, two isolates were related to Carnobacterium maltaromaticum with a high identity percentage. This species has been described as a facultatively anaerobic fermenting bacteria (Rainey et al., 2009) and its tolerance to other metals such as Cd have been assessed (Butrimienė et al., 2022), but not its interactions with Sb. Overall, these results open a gap for further bioremediation studies as the isolates, particularly those from the anaerobic Sb-respiring tolerators, might have the potential to be used in bioremediation by using Sb(V) as an electron acceptor.
Given the limited number of species that have been described for Cu-reducing capabilities (Kimber et al., 2023), the anaerobic Cu-enrichment cultures prove to be especially relevant. Even though growth was observed in different Cu-enrichment cultures (Supplementary Table S4), only few Cu microorganisms were isolated on plates, which might be explained by “great plate count anomaly” in which the number of microorganisms is reduced when transferring them to solid media (Staley and Konopka, 1985). Overall, most of the Cu-isolates were psychrotolerant which is expected as usually, more psychrotolerant than psychrophilic species are isolated from permanently cold environments (Männistö and Puhakka, 2002). Since temperature can affect the behavior of microbial communities in the environment, becoming the limiting factor in bioleaching applications (Dopson et al., 2007; Xiao et al., 2017), efforts are being made in order to characterize bioleaching bacteria which can carry out its activity at low temperature and being therefore a good candidate for mining areas with low mean temperatures (Muñoz-Villagrán et al., 2022). While some of the isolated strains were able to grow at temperatures near 0°C, their Cu-resistance was low (1 mM) in comparison with strains isolated for biomining in previous studies (>100 mM) (Orell et al., 2010; Barahona et al., 2020). It is therefore suggested that the putative Cu-metabolizing microorganisms isolated might not be good candidates for bioleaching applications. Nevertheless, because of the well-known advantages of using microbial consortia for bioleaching (Brune and Bayer, 2012; Liu et al., 2020; Bobadilla-Fazzini and Poblete-Castro, 2021), microorganisms from this study could still have potential applications, particularly due to their cold-tolerance or in other systems with lower Cu-concentrations.
Majority of environmental microorganisms are missed by selectively enrichment approach
In the present study, microorganisms isolated differed from the diversity found in the enrichment samples and environment. Differences were found in the microbial community structures of the environmental and enrichment samples (Figure 4). The most evident observation of this dissimilarity came with the total number of ASVs from the environment, which was seven times larger than the total number ASVs from the enrichments (Supplementary Figure S2). Certain phyla like Bacillota might be favored during the enrichment stage as, e.g., its abundance highly increased in site C. As expected, the abundances of Chloroflexota and Acidobacteriota, phyla which have been reported for their slow growth and difficulties for isolation (Campanharo et al., 2016; Salam et al., 2021; Sansupa et al., 2021), were reduced in the enrichments. Genera like Pseudomonas or Clostridium, which were not abundant in the environment of site C, dominated some of the enrichment samples from this site. These results were expected Pseudomonas is a fast-growing, metabolically versatile genus (Jones et al., 2021). In fact, Pseudomonas has been reported in previous studies (Ponce-Soto et al., 2015) for increasing its abundance during enrichment experiments, including in experiments from cold-metal contaminated sites (Ziegelhöfer and Kujala, 2021). Some genera like Corynebacterium or Kocuria, which were relatively high in the environmental samples from sites A and B, had reduced relative abundances in the enrichments. Both genera include several species which have been described for their slow growth, especially under cold conditions (Bernard and Funke, 2015; Youn and Seo, 2022). In both the enrichments and the environment, few taxa resulted in unclassified at genus level, including some of the most abundant ASVs of the enrichments (Figure 5), indicating that previously uncultured taxa have been selectively enriched. Our findings seem to confirm the main goal of the enrichment experiment, to selectively enrich microorganisms of interest (regardless of their abundance in the environment) by creating conditions that are favorable for their growth while not supporting growth of non-target groups was accomplished.
Microbial communities from sub(-arctic) mine sites
Mine sites, especially those in extreme environments like arctic regions, pose challenges due to their environmental conditions, which significantly influence the growth of microorganisms. Metal(loid)s and temperature are an example of selective pressure in the environment (Ledin, 1996; Bruins et al., 2000; Hao et al., 2021; Wang et al., 2021; Rijkers et al., 2022). Therefore, the environmental low temperatures and metal(loid) concentration which characterizes the sites for this study are particularly challenging for microbial communities. In the present study, sites A and B were mine tailings and site C was a peatland treating mining-affected waters. Peatlands are carbon-rich wetland ecosystems where peat, an organic-rich soil is formed (Lourenco et al., 2023). It is well known that peatlands are the habitat of a wide diversity of microorganisms with different metabolic activities, including those affected by metal(loid)s (Preston et al., 2012; Andersen et al., 2013; Kujala et al., 2020). Chodak and Niklińska (2010) found that in post-mining environments, the texture of the soil was the main factor shaping microbial communities, where loamy sands supported more microbial activity than sandy soils. Microbial composition exhibited significant variations primarily across different sites. Specifically, microbial communities from site C (water treatment peatland) exhibited markedly higher diversity than those from sites A and B (tailings). Differences between sites A and B, as opposed to site C, were also distinguished when observing the taxonomy. For example, sites A and B exhibited a dominance of three distinct phyla: Actinomycetota, Pseudomonadota, and Bacillota. In contrast, site C showed a much more even distribution of phyla in the samples, including the high representation of Chloroflexota and Bacteroidota, which is consistent with results from earlier studies on frozen peatlands (Liu et al., 2022). At the genus level, differences between sites A and B were slightly more pronounced yet notably similar when compared to the genera observed in site C. In site A and B the dominant genera were Prauserella, Escherichia-Shigella and Alteribacillus. In contrast, nearly all detected taxa in site C were represented by low-relative abundance genera, which aligns with the high values obtained in the rarity index, the indicator for the species richness of less abundant species, in site C (Supplementary Table S3). Contrary to expectations based on previous studies (Baldrian et al., 2012; Zhang et al., 2014), total (DNA) and active (RNA) microbial communities showed no variations across all samples or when grouped by sites (Supplementary Table S2 and Figure 3). In addition, difference between DNA- and RNA-based community composition appeared to be insignificant, as evidenced by the results of the CCA (Supplementary Figure S5). Considering RNA as a better approach to describe changes in active microbial communities due to, for instance, its lower stability in the environment compared to DNA (Jia et al., 2020), our results suggest certain degree of consistency for both, active and total microbial communities.
Toxic metal(loid)s were detected in the samples; in particular, site C and site B, had significantly higher values of Sb and Cu, respectively, (Table 1). When plotting the chemical elements on the CCA (Supplementary Figure S5), Sb appeared to be an important factor shaping microbial communities. However, high concentrations of Sb were only found in site C, the site from a different mining environment and soil texture. Consequently, we do believe changes in the microbial communities were not solely due to the Sb but to the texture of the soil. However, the higher diversity and Sb concentrations of site C might have played a role as most of the Sb-tolerant isolates (79.3%) originated from site C (Supplementary Table S6). In addition, higher levels of Cu were only found in site B, the one from where all of the Cu-tolerant microorganisms were isolated. However, Cu concentrations in the present study were not as high as other places from where Cu-reducing microorganisms have been isolated (Kimber et al., 2023). These results suggest that metal(loid) concentrations present in the environment might play a significant role in the isolation of tolerant microorganisms. Also, to observe the effect of the temperature on microbial communities, samples were collected from the same sites during summer and winter to observe changes in microbial community in two opposite arctic seasons. However, no significant differences in the microbial community composition were found between the summer and winter samples (Figures 2, 3). Our results support the notion that some microorganisms can be persistent and active under different temperature conditions. Gonzalez and Aranda (2023) described that in environments under changing conditions, microorganisms develop different growth states. This strategy is the reason of why certain thermophiles have been found in cold soils (Marchant et al., 2008). Thus, even if our results do not show changes in the microbial taxa detected in the summer and the winter, further studies on their growing rates could better explain the persistence of the microorganisms throughout the year.
Conclusion and limitations of the study
This study provides valuable insights into the biotechnological potential of putative Sb and Cu-tolerant cold-adapted microorganisms isolated from subarctic mine sites. The presented putative anaerobic Sb(V)-respiring microorganisms represents, to our knowledge, the first potentially Sb-metabolizing cold-tolerant microorganisms isolated. With the increasing development of mining operations in Northern regions like Finland, recognized as one of the leading countries globally in mineral exploration (Tuusjärvi et al., 2014; Mejía and Aliakbari, 2023), the microorganisms isolated in this study, emerge as promising candidates for bioremediation applications within in-situ systems of (sub-)Arctic mines as the isolation of metal(loid)- and cold-tolerant microorganisms are the first step in the process to establish a metal(loid) immobilization system under low temperatures. While the putative Cu-metabolizing isolates, may not be optimal candidates for conventional bioleaching applications due to their lower Cu-resistance compared, they still exhibit Cu-tolerance and cold adaptation. A different approach during the Cu-enrichment, e.g., lowering the pH of the medium or sampling in sites where Cu concentrations were higher, could have promoted the growth strains with a higher Cu tolerance. Furthermore, changes in the microbial communities from the environment to the isolates reflect the successful implementation of an enrichment protocol and suggest that high abundances in the environment do not correspond to chances of isolation. However, considering the limitations of amplicon-sequencing (Alteio et al., 2021), it is important to remark that even if some ASVs were not detected in the environment, it does not necessarily mean that the ASV was not present. Overall, the presented findings contribute to the understanding of metal(loid)-tolerant microbial communities in subarctic mine sites and open avenues for future biotechnology applications of cold-adapted metal(loid)-tolerant microorganisms.
Data availability statement
The datasets presented in this study can be found in online repositories. The names of the repository/repositories and accession number(s) can be found at: NCBI – PRJNA1079661, https://www.ncbi.nlm.nih.gov/bioproject/?term=PRJNA1079661.
Author contributions
FP-F: Conceptualization, Data curation, Formal analysis, Investigation, Methodology, Software, Visualization, Writing – original draft, Writing – review & editing. SL: Conceptualization, Methodology, Resources, Software, Supervision, Validation, Writing – original draft, Writing – review & editing. KK: Conceptualization, Funding acquisition, Investigation, Methodology, Project administration, Resources, Supervision, Validation, Writing – original draft, Writing – review & editing.
Funding
The author(s) declare that financial support was received for the research, authorship, and/or publication of this article. The authors would like to acknowledge the Research Council of Finland for the support (grant number: 322753) in the project “Capturing the unknown microbial players and genes involved in the cycling of arsenic and antimony in Northern peatland soils” and the K. H. Renlund Foundation in “Exploiting metal-cycling microbes for sustainable mining.” Also, to Finnish Environment Institute (SYKE) for providing laboratory access to the first experimental stages of this study. In addition, researcher Tiina Laamanen and Joni Koivula for their contribution in the sampling campaigns as well as Grethel Andino Ruiz and Sara Rinne for their help in the laboratory experiments.
Conflict of interest
The authors declare that the research was conducted in the absence of any commercial or financial relationships that could be construed as a potential conflict of interest.
Publisher’s note
All claims expressed in this article are solely those of the authors and do not necessarily represent those of their affiliated organizations, or those of the publisher, the editors and the reviewers. Any product that may be evaluated in this article, or claim that may be made by its manufacturer, is not guaranteed or endorsed by the publisher.
Supplementary material
The Supplementary material for this article can be found online at: https://www.frontiersin.org/articles/10.3389/fmicb.2024.1386120/full#supplementary-material
SUPPLEMENTARY TABLE S1 | One-sample t-test results for environmental parameters across different samples. 95% Confidence Interval represented as CI and Degrees of Freedom as Df.
SUPPLEMENTARY TABLE S2 | ANOVA results for diversity metrics of environmental samples grouped by nucleic acid type (DNA vs RNA), site (A vs B vs C), season (summer vs winter) and depth (frozen vs surface vs middle vs bottom). Metrics were measured including all study sites, including only site A, including only site B and including only site C. Top of Form.
SUPPLEMENTARY TABLE S3 | Diversity measures of the microbial communities in environmental samples.
SUPPLEMENTARY TABLE S4 | Growth (turbidity) of enrichment tubes after first (1), second (2), and third (3) inoculations. Turbidity levels are indicated as *** for highly turbid, ** for medium turbid, and - for no turbidity. Sb-fermenting tolerators and anaerobically respiring tolerators Sb-enrichments went through a second round of enrichment from the first inoculation (marked as 2a and 3a).
SUPPLEMENTARY TABLE S5 | Next-related sequences of the most abundant ASVs detected in the enrichment samples. Relative abundances in the enrichments are separated by their site of origin (A, B or C) and the type of enrichment, where Aerobic tolerators are described as AE, fermenting tolerators as FT and anaerobically respiring as AN.
SUPPLEMENTARY TABLE S6 | Identification of isolates using different enrichment and isolation media. Identification was made using BLAST based on the NCBI database. Growth at different temperatures and concentrations is given in categories related to their growth curves where (-) no growth observed (<5% of maximum), (+) low growth (<25% of maximum), (++) substantial growth (<75% of maximum), (+++) high growth (<85% of maximum), and (++++) maximum growth (>85% of maximum). Relative abundance indicates the abundance of the abundance of one or more than one ASV from the respective environmental and enrichment sample which matched the isolate sequence (>99% identity). ᵃ=there was more than one match but on the table is only included the one with the highest relative abundance.
SUPPLEMENTARY FIGURE S1 | Pictures of enrichments and isolate cultures. One aerobic Sb-enrichment sample from the third inoculation (A), isolates in anaerobic jars (B), colonies of isolate 68 after a month of inoculation (C) colony of isolate 23 and its halo marked with an arrow (D).
SUPPLEMENTARY FIGURE S2 | Venn Diagram of ASVs detected in all the stages of the study, where big circles represent ASVs detected in the environmental samples from each site, medium circles the enrichment samples and small circles the isolated colonies. Blue circles are ASVs from site A, yellow circles B and pink circles C. Inside of each circle there is the total number of ASVs detected, and the overlap region of two or the three circles indicates the shared detected ASVs.
SUPPLEMENTARY FIGURE S3 | Microbial composition of environmental, enrichments and control samples at genus level. Genera with a mean relative abundance of less than 10% were grouped in “Other”. Taxonomy classification based on 16S rRNA amplicon sequencing using SILVA database.
SUPPLEMENTARY FIGURE S4 | Heat map representing ASVs (relative abundance >0.05) shared between environmental (Env) and enrichment (Enr) samples and its phylogenetic relationships. Site A (blue) is plotted on the first and second group of columns, B (yellow) in third and fourth and C (pink) in fifth and sixth. A cladogram of the ASVs and the results of the SILVA alignment was added next to the heatmap to infer phylogeny relationships.
SUPPLEMENTARY FIGURE S5 | Canonical Correspondence Analysis (CCA) of environmental samples from different sites and seasons. Texture (tailings vs peat), genetic (DNA vs RNA) and season (summer vs winter) were processed as binary values in the metadata.
References
Aghazadeh, S., Abdollahi, H., Gharabaghi, M., and Mirmohammadi, M. (2023). Bioleaching of zinc, copper and antimony from a tetrahedrite concentrate using acidophilic microorganisms. Hydrometallurgy 219:106075. doi: 10.1016/j.hydromet.2023.106075
Alteio, L. V., Séneca, J., Canarini, A., Angel, R., Jansa, J., Guseva, K., et al. (2021). A critical perspective on interpreting amplicon sequencing data in soil ecological research. Soil Biol. Biochem. 160:108357. doi: 10.1016/j.soilbio.2021.108357
Altschul, S. F., Gish, W., Miller, W., Myers, E. W., and Lipman, D. J. (1990). Basic local alignment search tool. J. Mol. Biol. 215, 403–410. doi: 10.1016/S0022-2836(05)80360-2
An, Y.-J., and Kim, M. (2009). Effect of antimony on the microbial growth and the activities of soil enzymes. Chemosphere 74, 654–659. doi: 10.1016/j.chemosphere.2008.10.023
Andersen, R., Chapman, S. J., and Artz, R. R. E. (2013). Microbial communities in natural and disturbed peatlands: A review. Soil Biol. Biochem. 57, 979–994. doi: 10.1016/j.soilbio.2012.10.003
Aznar-Sánchez, J. A., Velasco-Muñoz, J. F., Belmonte-Ureña, L. J., and Manzano-Agugliaro, F. (2019). Innovation and technology for sustainable mining activity: A worldwide research assessment. J. Clean. Prod. 221, 38–54. doi: 10.1016/j.jclepro.2019.02.243
Bala, S., Garg, D., Thirumalesh, B. V., Sharma, M., Sridhar, K., Inbaraj, B. S., et al. (2022). Recent strategies for bioremediation of emerging pollutants: A review for a green and sustainable environment. Toxics 10:484. doi: 10.3390/toxics10080484
Balch, W. E., Fox, G. E., Magrum, L. J., Woese, C. R., and Wolfe, R. S. (1979). Methanogens: reevaluation of a unique biological group. Microbiol. Rev. 43, 260–296. doi: 10.1128/mr.43.2.260-296.1979
Baldrian, P., Kolařík, M., Štursová, M., Kopecký, J., Valášková, V., Větrovský, T., et al. (2012). Active and total microbial communities in forest soil are largely different and highly stratified during decomposition. ISME J. 6, 248–258. doi: 10.1038/ismej.2011.95
Barahona, S., Castro-Severyn, J., Dorador, C., Saavedra, C., and Remonsellez, F. (2020). Determinants of copper resistance in Acidithiobacillus ferrivorans ACH isolated from the Chilean Altiplano. Genes 11:844. doi: 10.3390/genes11080844
Barahona, S., Dorador, C., Zhang, R., Aguilar, P., Sand, W., Vera, M., et al. (2014). Isolation and characterization of a novel Acidithiobacillus ferrivorans strain from the Chilean Altiplano: attachment and biofilm formation on pyrite at low temperature. Res. Microbiol. 165, 782–793. doi: 10.1016/j.resmic.2014.07.015
Bernard, K. A., and Funke, G. (2015). “Corynebacterium” in Bergey’s manual of systematics of Archaea and Bacteria. ed. W. B. Whitman (New York, NY: Wiley).
Blake, R. C., Choate, D. M., Bardhan, S., Revis, N., Barton, L. L., and Zocco, T. G. (1993). Chemical transformation of toxic metals by a Pseudomonas strain from a toxic waste site. Environ. Toxicol. Chem. 12, 1365–1376. doi: 10.1002/etc.5620120806
Bobadilla-Fazzini, R. A., and Poblete-Castro, I. (2021). Biofilm formation is crucial for efficient copper bioleaching from bornite under mesophilic conditions: unveiling the lifestyle and catalytic role of sulfur-oxidizing bacteria. Front. Microbiol. 12:761997. doi: 10.3389/fmicb.2021.761997
Bolan, N., Kumar, M., Singh, E., Kumar, A., Singh, L., Kumar, S., et al. (2022). Antimony contamination and its risk management in complex environmental settings: A review. Environ. Int. 158:106908. doi: 10.1016/j.envint.2021.106908
Bolyen, E., Rideout, J. R., Dillon, M. R., Bokulich, N. A., Abnet, C. C., Al-Ghalith, G. A., et al. (2019). Reproducible, interactive, scalable and extensible microbiome data science using QIIME 2. Nat. Biotechnol. 37, 852–857. doi: 10.1038/s41587-019-0209-9
Bruins, M. R., Kapil, S., and Oehme, F. W. (2000). Microbial resistance to metals in the environment. Ecotoxicol. Environ. Saf. 45, 198–207. doi: 10.1006/eesa.1999.1860
Brune, K. D., and Bayer, T. S. (2012). Engineering microbial consortia to enhance biomining and bioremediation. Front. Microbiol. 3:203. doi: 10.3389/fmicb.2012.00203
Butrimienė, R., Kalnaitytė, A., Januškaitė, E., Bagdonas, S., Jurgelėnė, Ž., Butkauskas, D., et al. (2022). Interactions of semiconductor cd-based quantum dots and cd 2+ with gut bacteria isolated from wild Salmo trutta fry. PeerJ 10:e14025. doi: 10.7717/peerj.14025
Cacciuttolo, C., Cano, D., and Custodio, M. (2023). Socio-environmental risks linked with mine tailings chemical composition: promoting responsible and safe mine tailings management considering copper and gold mining experiences from Chile and Peru. Toxics 11:462. doi: 10.3390/toxics11050462
Callahan, B. J., McMurdie, P. J., Rosen, M. J., Han, A. W., Johnson, A. J. A., and Holmes, S. P. (2016). DADA2: high-resolution sample inference from Illumina amplicon data. Nat. Methods 13, 581–583. doi: 10.1038/nmeth.3869
Campanharo, J. C., Kielak, A. M., Castellane, T. C. L., Kuramae, E. E., and Lemos, E. G. D. M. (2016). Optimized medium culture for Acidobacteria subdivision 1 strains. FEMS Microbiol. Lett. 363:fnw 245. doi: 10.1093/femsle/fnw245
Carlin, A., Shi, W., Dey, S., and Rosen, B. P. (1995). The ars operon of Escherichia coli confers arsenical and antimonial resistance. J. Bacteriol. 177, 981–986. doi: 10.1128/jb.177.4.981-986.1995
Chen, Y., O’Loughlin, E., and Kwon, M. J. (2022). Antimony redox processes in the environment: A critical review of associated oxidants and reductants. J. Hazard. Mater. 431:128607. doi: 10.1016/j.jhazmat.2022.128607
Chen, W., Yin, S., Zhou, G., Li, Z., and Song, Q. (2022). Copper recovery from tailings through bioleaching and further application of bioleached tailings residue: comprehensive utilization of tailings. J. Clean. Prod. 332:130129. doi: 10.1016/j.jclepro.2021.130129
Chodak, M., and Niklińska, M. (2010). Effect of texture and tree species on microbial properties of mine soils. Appl. Soil Ecol. 46, 268–275. doi: 10.1016/j.apsoil.2010.08.002
Collins, M. D., Rodrigues, U. M., Dainty, R. H., Edwards, R. A., and Roberts, T. A. (1992). Taxonomic studies on a psychrophilic Clostridium from vacuum-packed beef: description of Clostridium estertheticum sp. nov. FEMS Microbiol. Lett. 96, 235–239. doi: 10.1111/j.1574-6968.1992.tb05423.x
Dixit, R., Wasiullah, M., Malaviya, D., Pandiyan, K., Singh, U., Sahu, A., et al. (2015). Bioremediation of heavy metals from soil and aquatic environment: An overview of principles and criteria of fundamental processes. Sustain. For. 7, 2189–2212. doi: 10.3390/su7022189
Dopson, M., Baker-Austin, C., Koppineedi, P. R., and Bond, P. L. (2003). Growth in sulfidic mineral environments: metal resistance mechanisms in acidophilic micro-organisms. Microbiology 149, 1959–1970. doi: 10.1099/mic.0.26296-0
Dopson, M., Halinen, A., Rahunen, N., Özkaya, B., Sahinkaya, E., Kaksonen, A. H., et al. (2007). Mineral and iron oxidation at low temperatures by pure and mixed cultures of acidophilic microorganisms. Biotechnol. Bioeng. 97, 1205–1215. doi: 10.1002/bit.21312
Eberle, A., Besold, J., León Ninin, J. M., Kerl, C. F., Kujala, K., and Planer-Friedrich, B. (2021). Potential of high pH and reduced sulfur for arsenic mobilization: insights from a Finnish peatland treating mining waste water. Sci. Total Environ. 758:143689. doi: 10.1016/j.scitotenv.2020.143689
European Parliament, Council of the European Union (2020). The quality of water intended for human consumption. The member states: Official journal of the European Union. Available at: http://data.europa.eu/eli/dir/2020/2184/oj
Falagán, C., Grail, B. M., and Johnson, D. B. (2017). New approaches for extracting and recovering metals from mine tailings. Miner. Eng. 106, 71–78. doi: 10.1016/j.mineng.2016.10.008
Gao, X., Jiang, L., Mao, Y., Yao, B., and Jiang, P. (2021). Progress, challenges, and perspectives of bioleaching for recovering heavy metals from mine tailings. Adsorp. Sci. Technol. 2021, 1–13. doi: 10.1155/2021/9941979
Gonzalez, J. M., and Aranda, B. (2023). Microbial growth under limiting conditions: future perspectives. Microorganisms 11:1641. doi: 10.3390/microorganisms11071641
Govarthanan, M., Lee, G.-W., Park, J.-H., Kim, J. S., Lim, S.-S., Seo, S.-K., et al. (2014). Bioleaching characteristics, influencing factors of cu solubilization and survival of Herbaspirillum sp. GW103 in cu contaminated mine soil. Chemosphere 109, 42–48. doi: 10.1016/j.chemosphere.2014.02.054
Grohol, M., and Veeh, C.Directorate-General for Internal Market, Industry, Entrepreneurship and SMEs (2023). Study on the critical raw materials for the EU 2023: Final report. LU: Publications Office of the European Union. Available at: https://data.europa.eu/doi/10.2873/725585 (accessed January 31, 2024).
Haddaway, N. R., Smith, A., Taylor, J. J., Andrews, C., Cooke, S. J., Nilsson, A. E., et al. (2022). Evidence of the impacts of metal mining and the effectiveness of mining mitigation measures on social–ecological systems in Arctic and boreal regions: a systematic map. Environ. Evid. 11:30. doi: 10.1186/s13750-022-00282-y
Hafeez, I., Azam, M., Amin, A., Mahmood, Z., Aamir, M., and Akram, A. (2017). Microbial extraction of antimony from stibnite of Qillah Abdullah. Am. Sci. Res. J. Eng. Technol. Sci. 28, 117–127, Available at: https://asrjetsjournal.org/index.php/American_Scientific_Journal/article/view/2552/1048
Hamamura, N., Fukushima, K., and Itai, T. (2013). Identification of antimony- and arsenic-oxidizing bacteria associated with antimony mine tailing. Microb. Environ. 28, 257–263. doi: 10.1264/jsme2.ME12217
Hao, X., Zhu, J., Rensing, C., Liu, Y., Gao, S., Chen, W., et al. (2021). Recent advances in exploring the heavy metal(loid) resistant microbiome. Comput. Struct. Biotechnol. J. 19, 94–109. doi: 10.1016/j.csbj.2020.12.006
Herlemann, D. P. R., Labrenz, M., Jürgens, K., Bertilsson, S., Waniek, J. J., and Andersson, A. F. (2011). Transitions in bacterial communities along the 2000 km salinity gradient of the Baltic Sea. ISME J. 5, 1571–1579. doi: 10.1038/ismej.2011.41
Jerez, C. A. (2017). Biomining of metals: how to access and exploit natural resource sustainably. Microb. Biotechnol. 10, 1191–1193. doi: 10.1111/1751-7915.12792
Jia, X., Dini-Andreote, F., and Falcão Salles, J. (2020). Comparing the influence of assembly processes governing bacterial community succession based on DNA and RNA data. Microorganisms 8:798. doi: 10.3390/microorganisms8060798
Jiang, L., Sun, H., Peng, T., Ding, W., Liu, B., and Liu, Q. (2021). Comprehensive evaluation of environmental availability, pollution level and leaching heavy metals behavior in non-ferrous metal tailings. J. Environ. Manag. 290:112639. doi: 10.1016/j.jenvman.2021.112639
Jones, M. L., Rivett, D. W., Pascual-García, A., and Bell, T. (2021). Relationships between community composition, productivity and invasion resistance in semi-natural bacterial microcosms. eLife 10:e71811. doi: 10.7554/eLife.71811
Kimber, R. L., Elizondo, G., Jedyka, K., Boothman, C., Cai, R., Bagshaw, H., et al. (2023). Copper bioreduction and nanoparticle synthesis by an enrichment culture from a former copper mine. Environ. Microbiol. 25, 3139–3150. doi: 10.1111/1462-2920.16488
Kuhner, C. H., Drake, H. L., Alm, E., and Raskin, L. (1996). Methane production and oxidation by soils from acidic forest wetlands of east-Central Germany. Washington, DC: American Society for Microbiology.
Kujala, K., Besold, J., Mikkonen, A., Tiirola, M., and Planer-Friedrich, B. (2020). Abundant and diverse arsenic-metabolizing microorganisms in peatlands treating arsenic-contaminated mining wastewaters. Environ. Microbiol. 22, 1572–1587. doi: 10.1111/1462-2920.14922
Kulp, T. R., Miller, L. G., Braiotta, F., Webb, S. M., Kocar, B. D., Blum, J. S., et al. (2014). Microbiological reduction of Sb(V) in anoxic freshwater sediments. Environ. Sci. Technol. 48, 218–226. doi: 10.1021/es403312j
Kumar, R. N., and Nagendran, R. (2007). Influence of initial pH on bioleaching of heavy metals from contaminated soil employing indigenous Acidithiobacillus thiooxidans. Chemosphere 66, 1775–1781. doi: 10.1016/j.chemosphere.2006.07.091
Kumar, S., Stecher, G., Li, M., Knyaz, C., and Tamura, K. (2018). MEGA X: molecular evolutionary genetics analysis across computing platforms. Mol. Biol. Evol. 35, 1547–1549. doi: 10.1093/molbev/msy096
Lai, C. Y., Wen, L. L., Zhang, Y., Luo, S. S., Wang, Q. Y., Luo, Y. H., et al. (2016). Autotrophic antimonate bio-reduction using hydrogen as the electron donor. Water Res. 88, 467–474. doi: 10.1016/j.watres.2015.10.042
Lane, D. J. (1991). “16S/23S rRNA sequencing” in Nucleic acid techniques in bacterial systematics. eds. E. Stackebrandt and M. Goodfellow (Chichester: John Wiley and Sons Ltd), 115–175.
Ledin, M. (1996). The environmental impact of mine wastes -- roles of microorganisms and their significance in treatment of mine wastes. Earth Sci. Rev. 41, 67–108. doi: 10.1016/0012-8252(96)00016-5
Levett, A., Gleeson, S. A., and Kallmeyer, J. (2021). From exploration to remediation: A microbial perspective for innovation in mining. Earth Sci. Rev. 216:103563. doi: 10.1016/j.earscirev.2021.103563
Li, J., Wang, Q., Oremland, R. S., Kulp, T. R., Rensing, C., and Wang, G. (2016). Microbial antimony biogeochemistry: enzymes, regulation, and related metabolic pathways. Appl. Environ. Microbiol. 82, 5482–5495. doi: 10.1128/AEM.01375-16
Li, J., Xu, D., and Zhu, Y. (2022). Global antimony supply risk assessment through the industry chain. Front. Energy Res. 10:1007260. doi: 10.3389/fenrg.2022.1007260
Li, J., Zhang, Y., Zheng, S., Liu, F., and Wang, G. (2019). Anaerobic bacterial immobilization and removal of toxic Sb(III) coupled with Fe(II)/Sb(III) oxidation and denitrification. Front. Microbiol. 10:360. doi: 10.3389/fmicb.2019.00360
Lim, N. Y. N., Roco, C. A., and Frostegård, Å. (2016). Transparent DNA/RNA co-extraction workflow protocol suitable for inhibitor-rich environmental samples that focuses on complete DNA removal for transcriptomic analyses. Front. Microbiol. 7:1588. doi: 10.3389/fmicb.2016.01588
Liu, Y., Wang, J., Hou, H., Chen, G., Liu, H., Liu, X., et al. (2020). Effect of introduction of exogenous strain Acidithiobacillus thiooxidans A01 on structure and function of adsorbed and planktonic microbial consortia during bioleaching of low-grade copper sulfide. Front. Microbiol. 10:3034. doi: 10.3389/fmicb.2019.03034
Liu, L., Wang, Z., Ma, D., Zhang, M., and Fu, L. (2022). Diversity and distribution characteristics of soil microbes across forest–peatland ecotones in the permafrost regions. Int. J. Environ. Res. Public Health 19:14782. doi: 10.3390/ijerph192214782
Loredo Portales, R., Cruz Jiménez, G., Castillo Michel, H., Rocha Amador, D. O., Vogel Mikuš, K., Kump, P., et al. (2015). Understanding copper speciation and mobilization in soils and mine tailings from “Mineral La Aurora” in central Mexico: Contributions from synchrotron techniques. BSGM. 67, 447–456. doi: 10.18268/BSGM2015v67n3a8
Lourenco, M., Fitchett, J. M., and Woodborne, S. (2023). Peat definitions: A critical review. Prog. Phys. Geogr. 47, 506–520. doi: 10.1177/03091333221118353
Malla, M. A., Dubey, A., Yadav, S., Kumar, A., Hashem, A., and Abd Allah, E. F. (2018). Understanding and designing the strategies for the microbe-mediated remediation of environmental contaminants using omics approaches. Front. Microbiol. 9:1132. doi: 10.3389/fmicb.2018.01132
Männistö, M. K., and Puhakka, J. A. (2002). Psychrotolerant and microaerophilic bacteria in boreal groundwater. FEMS Microbiol. Ecol. 41, 9–16. doi: 10.1111/j.1574-6941.2002.tb00961.x
Marchant, R., Franzetti, A., Pavlostathis, S. G., Tas, D. O., Erdbrűgger, I., Űnyayar, A., et al. (2008). Thermophilic bacteria in cool temperate soils: are they metabolically active or continually added by global atmospheric transport? Appl. Microbiol. Biotechnol. 78, 841–852. doi: 10.1007/s00253-008-1372-y
Mejía, J., and Aliakbari, E. (2023). Fraser Institute annual survey of mining companies 2022. Vancouver: Fraser Institute. Available at: https://www.fraserinstitute.org/studies/annual-survey-of-mining-companies-2022 (accessed March 22, 2024).
Morales, M., Sentchilo, V., Bertelli, C., Komljenovic, A., Kryuchkova-Mostacci, N., Bourdilloud, A., et al. (2016). The genome of the toluene-degrading Pseudomonas veronii strain 1YdBTEX2 and its differential gene expression in contaminated sand. PLoS One 11:e0165850. doi: 10.1371/journal.pone.0165850
Morita, R. Y. (1975). Psychrophilic bacteria. Bacteriol. Rev. 39, 144–167. doi: 10.1128/br.39.2.144-167.1975
Muñoz-Villagrán, C., Grossolli-Gálvez, J., Acevedo-Arbunic, J., Valenzuela, X., Ferrer, A., Díez, B., et al. (2022). Characterization and genomic analysis of two novel psychrotolerant Acidithiobacillus ferrooxidans strains from polar and subpolar environments. Front. Microbiol. 13:960324. doi: 10.3389/fmicb.2022.960324
Newsome, L., and Falagán, C. (2021). The microbiology of metal mine easte: bioremediation applications and implications for planetary health. GeoHealth 5:e2020GH000380. doi: 10.1029/2020GH000380
Orell, A., Navarro, C. A., Arancibia, R., Mobarec, J. C., and Jerez, C. A. (2010). Life in blue: copper resistance mechanisms of bacteria and archaea used in industrial biomining of minerals. Biotechnol. Adv. 28, 839–848. doi: 10.1016/j.biotechadv.2010.07.003
Oren, A., and Garrity, G. M. (2021). Valid publication of the names of forty-two phyla of prokaryotes. Int. J. Syst. Evol. Microbiol. 71:005056. doi: 10.1099/ijsem.0.005056
Palmer, K., Ronkanen, A.-K., and Kløve, B. (2015). Efficient removal of arsenic, antimony and nickel from mine wastewaters in northern treatment peatlands and potential risks in their long-term use. Ecol. Eng. 75, 350–364. doi: 10.1016/j.ecoleng.2014.11.045
Periferakis, A., Caruntu, A., Periferakis, A.-T., Scheau, A.-E., Badarau, I. A., Caruntu, C., et al. (2022). Availability, toxicology and medical significance of antimony. Int. J. Environ. Res. Public Health 19:4669. doi: 10.3390/ijerph19084669
Perryman, C. R., Wirsing, J., Bennett, K. A., Brennick, O., Perry, A. L., Williamson, N., et al. (2020). Heavy metals in the Arctic: distribution and enrichment of five metals in Alaskan soils. PLoS One 15:e0233297. doi: 10.1371/journal.pone.0233297
Ponce-Soto, G. Y., Aguirre-von-Wobeser, E., Eguiarte, L. E., Elser, J. J., Lee, Z. M.-P., and Souza, V. (2015). Enrichment experiment changes microbial interactions in an ultra-oligotrophic environment. Front. Microbiol. 6:246. doi: 10.3389/fmicb.2015.00246
Preston, M. D., Smemo, K. A., McLaughlin, J. W., and Basiliko, N. (2012). Peatland microbial communities and decomposition processes in the James Bay lowlands, Canada. Front. Microbiol. 3:70. doi: 10.3389/fmicb.2012.00070
Qi, Y., Wei, X., Zhao, M., Pan, W., Jiang, C., Wu, J., et al. (2022). Heavy metal pollution characteristics and potential ecological risk assessment of soils around three typical antimony mining areas and watersheds in China. Front. Environ. Sci. 10:913293. doi: 10.3389/fenvs.2022.913293
Quast, C., Pruesse, E., Yilmaz, P., Gerken, J., Schweer, T., Yarza, P., et al. (2012). The SILVA ribosomal RNA gene database project: improved data processing and web-based tools. Nucleic Acids Res. 41, D590–D596. doi: 10.1093/nar/gks1219
Rainey, F. A., Hollen, B. J., and Small, A. (2009). “Genus I. Clostridium Prazmowski 1880, 23AL” in Bergey’s manual of systematic bacteriology. eds. M. Goodfellow, P. Kämpfer, H. -J. Busse, M. E. Trujillo, K. -I. Suzuki, and W. Ludwig, et al. (New York, NY: Springer).
Rijkers, R., Rousk, J., Aerts, R., Sigurdsson, B. D., and Weedon, J. T. (2022). Optimal growth temperature of Arctic soil bacterial communities increases under experimental warming. Glob. Chang. Biol. 28, 6050–6064. doi: 10.1111/gcb.16342
Riseh, R. S., Vazvani, M. G., Hajabdollahi, N., and Thakur, V. K. (2023). Bioremediation of heavy metals by Rhizobacteria. Appl. Microbiol. Biotechnol. 195, 4689–4711. doi: 10.1007/s12010-022-04177-z
Roche, C., Thygesen, K., and Baker, E. (2017). Mine tailings storage: safety is no accident. A UNEP rapid response assessment. United Nations environment Programme and GRID-Arendal, Nairobi and Arendal. Available at: www.grida.no
Rudnicka-Kępa, P., and Zaborska, A. (2021). Sources, fate and distribution of inorganic contaminants in the Svalbard area, representative of a typical Arctic critical environment–a review. Environ. Monit. Assess. 193:724. doi: 10.1007/s10661-021-09305-6
Salam, N., Xian, W.-D., Asem, M. D., Xiao, M., and Li, W.-J. (2021). From ecophysiology to cultivation methodology: filling the knowledge gap between uncultured and cultured microbes. Mar. Life. Sci. Technol. 3, 132–147. doi: 10.1007/s42995-020-00064-w
Sánchez-Castro, I., Molina, L., Prieto-Fernández, M.-Á., and Segura, A. (2023). Past, present and future trends in the remediation of heavy-metal contaminated soil - remediation techniques applied in real soil-contamination events. Heliyon 9:e16692. doi: 10.1016/j.heliyon.2023.e16692
Sansupa, C., Fareed Mohamed Wahdan, S., Disayathanoowat, T., and Purahong, W. (2021). Identifying hidden viable bacterial taxa in tropical forest soils using amplicon sequencing of enrichment cultures. Biology 10:569. doi: 10.3390/biology10070569
Sethurajan, M., Van Hullebusch, E. D., and Nancharaiah, Y. V. (2018). Biotechnology in the management and resource recovery from metal bearing solid wastes: recent advances. J. Environ. Manag. 211, 138–153. doi: 10.1016/j.jenvman.2018.01.035
Shi, L.-D., Wang, M., Han, Y.-L., Lai, C.-Y., Shapleigh, J. P., and Zhao, H.-P. (2019). Multi-omics reveal various potential antimonate reductases from phylogenetically diverse microorganisms. Appl. Microbiol. Biotechnol. 103, 9119–9129. doi: 10.1007/s00253-019-10111-x
Spring, S., Merkhoffer, B., Weiss, N., Kroppenstedt, R. M., Hippe, H., and Stackebrandt, E. (2003). Characterization of novel psychrophilic clostridia from an Antarctic microbial mat: description of Clostridium frigoris sp. nov., Clostridium lacusfryxellense sp. nov., Clostridium bowmanii sp. nov. and Clostridium psychrophilum sp. nov. and reclassification of Clostridium laramiense as Clostridium estertheticum subsp. laramiense subsp. nov. Int. J. Syst. Evol. Microbiol. 53, 1019–1029. doi: 10.1099/ijs.0.02554-0
Staicu, L. C., and Stolz, J. F. (2021). Microbes vs. metals: harvest and recycle. FEMS Microbiol. Ecol. 97:fiab 056. doi: 10.1093/femsec/fiab056
Staley, J. T., and Konopka, A. (1985). Measurements of in situ activities of nonphotosynthetic microorganisms in aquatic and terrestrial habitats. Ann. Rev. Microbiol. 39, 321–346. doi: 10.1146/annurev.mi.39.100185.001541
Steinnes, E., and Friedland, A. J. (2006). Metal contamination of natural surface soils from long-range atmospheric transport: existing and missing knowledge. Environ. Rev. 14, 169–186. doi: 10.1139/a06-002
Sun, W., Ji, B., Khoso, S. A., Tang, H., Liu, R., Wang, L., et al. (2018). An extensive review on restoration technologies for mining tailings. Environ. Sci. Pollut. Res. 25, 33911–33925. doi: 10.1007/s11356-018-3423-y
Sun, W., Sun, X., Häggblom, M. M., Kolton, M., Lan, L., Li, B., et al. (2021). Identification of antimonate reducing bacteria and their potential metabolic traits by the combination of stable isotope probing and metagenomic-pangenomic analysis. Environ. Sci. Technol. 55, 13902–13912. doi: 10.1021/acs.est.1c03967
Tamura, K., and Nei, M. (1993). Estimation of the number of nucleotide substitutions in the control region of mitochondrial DNA in humans and chimpanzees. Mol. Biol. Evol. 10, 512–526. doi: 10.1093/oxfordjournals.molbev.a040023
Tuusjärvi, M., Mäenpää, I., Vuori, S., Eilu, P., Kihlman, S., and Koskela, S. (2014). Metal mining industry in Finland – development scenarios to 2030. J. Clean. Prod. 84, 271–280. doi: 10.1016/j.jclepro.2014.03.038
United States Environmental Protection Agency (1997). National Primary Drinking Water Standards. Washington DC: USEPA.
Vera, M., Schippers, A., Hedrich, S., and Sand, W. (2022). Progress in bioleaching: fundamentals and mechanisms of microbial metal sulfide oxidation – part A. Appl. Microbiol. Biotechnol. 106, 6933–6952. doi: 10.1007/s00253-022-12168-7
Wang, H., Chen, F., Mu, S., Zhang, D., Pan, X., Lee, D.-J., et al. (2013). Removal of antimony (Sb(V)) from Sb mine drainage: biological sulfate reduction and sulfide oxidation–precipitation. Bioresour. Technol. 146, 799–802. doi: 10.1016/j.biortech.2013.08.002
Wang, C., Morrissey, E. M., Mau, R. L., Hayer, M., Piñeiro, J., Mack, M. C., et al. (2021). The temperature sensitivity of soil: microbial biodiversity, growth, and carbon mineralization. ISME J. 15, 2738–2747. doi: 10.1038/s41396-021-00959-1
Wang, L., Ye, L., Chen, Y., and Jing, C. (2018). Antimony redox biotransformation in the subsurface: effect of indigenous Sb(V) respiring microbiota. Environ. Sci. Technol. 52, 1200–1207. doi: 10.1021/acs.est.7b04624
Wüst, P. K., Horn, M. A., and Drake, H. L. (2009). Trophic links between fermenters and methanogens in a moderately acidic fen soil. Environ. Microbiol. 11, 1395–1409. doi: 10.1111/j.1462-2920.2009.01867.x
Xiao, Y., Liu, X., Fang, J., Liang, Y., Zhang, X., Meng, D., et al. (2017). Responses of zinc recovery to temperature and mineral composition during sphalerite bioleaching process. AMB Expr 7:190. doi: 10.1186/s13568-017-0491-1
Yamamoto, K., and Ishihama, A. (2005). Transcriptional response of Escherichia coli to external copper. Mol. Microbiol. 56, 215–227. doi: 10.1111/j.1365-2958.2005.04532.x
Yin, S., Wang, L., Kabwe, E., Chen, X., Yan, R., An, K., et al. (2018). Copper bioleaching in China: review and prospect. Fortschr. Mineral. 8:32. doi: 10.3390/min8020032
Youn, H.-Y., and Seo, K.-H. (2022). Isolation and characterization of halophilic Kocuria salsicia strains from cheese brine. Food. Sci. Anim. Resour. 42, 252–265. doi: 10.5851/kosfa.2022.e1
Yu, Z., Gunn, L., Brennan, E., Reid, R., Wall, P. G., Gaora, P. Ó., et al. (2016). Complete genome sequence of Clostridium estertheticum DSM 8809, a microbe identified in spoiled vacuum packed beef. Front. Microbiol. 7:1764. doi: 10.3389/fmicb.2016.01764
Zhang, S.-Y., Xiao, X., Chen, S.-C., Zhu, Y.-G., Sun, G.-X., and Konstantinidis, K. T. (2021). High arsenic levels increase activity rather than diversity or abundance of arsenic metabolism genes in paddy soils. Appl. Environ. Microbiol. 87:e0138321. doi: 10.1128/AEM.01383-21
Zhang, Y., Zhao, Z., Dai, M., Jiao, N., and Herndl, G. J. (2014). Drivers shaping the diversity and biogeography of total and active bacterial communities in the South China Sea. Mol. Ecol. 23, 2260–2274. doi: 10.1111/mec.12739
Zhu, Y., Wu, M., Gao, N., Chu, W., An, N., Wang, Q., et al. (2018). Removal of antimonate from wastewater by dissimilatory bacterial reduction: role of the coexisting sulfate. J. Hazard. Mater. 341, 36–45. doi: 10.1016/j.jhazmat.2017.07.042
Ziegelhöfer, A., and Kujala, K. (2021). Assessing the diversity and metabolic potential of psychrotolerant arsenic-metabolizing microorganisms from a subarctic peatland used for treatment of mining-affected waters by culture-dependent and -independent techniques. Front. Microbiol. 12:648412. doi: 10.3389/fmicb.2021.648412
Keywords: metal, metalloid, cold, copper, antimony, enrichment, isolation, bioremediation
Citation: Prieto-Fernández F, Lambert S and Kujala K (2024) Assessment of microbial communities from cold mine environments and subsequent enrichment, isolation and characterization of putative antimony- or copper-metabolizing microorganisms. Front. Microbiol. 15:1386120. doi: 10.3389/fmicb.2024.1386120
Edited by:
Saskia Bindschedler, Université de Neuchâtel, SwitzerlandReviewed by:
Errol Duncan Cason, University of the Free State, South AfricaOlli H. Tuovinen, The Ohio State University, United States
Copyright © 2024 Prieto-Fernández, Lambert and Kujala. This is an open-access article distributed under the terms of the Creative Commons Attribution License (CC BY). The use, distribution or reproduction in other forums is permitted, provided the original author(s) and the copyright owner(s) are credited and that the original publication in this journal is cited, in accordance with accepted academic practice. No use, distribution or reproduction is permitted which does not comply with these terms.
*Correspondence: Francisca Prieto-Fernández, ZnJhbmNpc2NhLnByaWV0b2Zlcm5hbmRlekBvdWx1LmZp