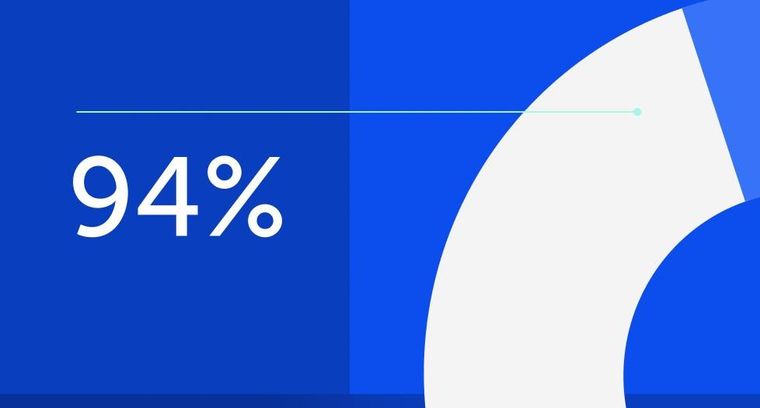
94% of researchers rate our articles as excellent or good
Learn more about the work of our research integrity team to safeguard the quality of each article we publish.
Find out more
REVIEW article
Front. Microbiol., 05 July 2024
Sec. Antimicrobials, Resistance and Chemotherapy
Volume 15 - 2024 | https://doi.org/10.3389/fmicb.2024.1384164
This article is part of the Research TopicEngineering Antibodies and Bacteriophages to Manage and Diagnose Microbial InfectionsView all 8 articles
Amid the growing challenge of antibiotic resistance on a global scale, there has been a notable resurgence in bacteriophage-based treatments, signaling a shift in our approach to managing infections. Bacteriophages (BPs), bacterial predators of nature, present a promising alternative for tackling infections caused by antibiotic-resistant pathogens. This review delves into the intricate relationship between bacteriophages and resistant bacteria, exploring various treatment strategies. Drawing upon both preclinical and clinical studies, the review highlights the effectiveness of bacteriophage therapy, particularly when integrated synergistically with conventional antibiotics. It discusses various treatment approaches for systemic and localized infections, demonstrating the adaptability of bacteriophage therapy across different clinical scenarios. Furthermore, the formulation and delivery of bacteriophages shed light on the various methods used to encapsulate and administer them effectively. It also acknowledges the challenge of bacterial resistance to bacteriophages and the ongoing efforts to overcome this hurdle. In addition, this review highlights the importance of the bacteriophage sensitivity profile (phagogram), which helps tailor treatment regimens to individual patients and specific pathogens. By surpassing the limitations of traditional antibiotics, bacteriophage-based therapies offer a personalized and potent solution against antibiotic resistance, promising to reshape the future of infectious disease management.
Antibiotics play a vital role in curing bacteria-caused minor and life-threatening infections. Nonetheless, bacteria have become incredibly resilient and are capable of using numerous approaches to survive the attack by most antibiotics (Roach and Donovan, 2015). Globally, there is a growing rate of antibiotic resistance (AR) and the rise of multidrug-resistant superbugs, largely caused by the non-judicious usage of antibiotics. In addition to jeopardizing healthy living, AR has a huge economic impact on both developed and developing countries (Aslam et al., 2021). As per the World Health Organization (WHO), AR is responsible for causing approximately 700,000 deaths every year. Moreover, the number of AR-caused deaths is estimated to rise rapidly in the upcoming years (Ling et al., 2022). On average, patients suffering from AR-associated infections require approximately 13 days of hospital stay, imposing a cost of approximately $29,000 per patient treated for such resistant bacterial infections. This can lead to a total annual potential productivity loss of approximately $35 billion (Khurshid et al., 2017; Aslam et al., 2018; Khurshid et al., 2020). These statistics indicate the global impact of AR and the urgency to find novel and effective antimicrobial therapies to control the AR crisis (Baloch et al., 2018; Aslam et al., 2021; Ling et al., 2022).
Bacteriophages (BPs) or phages are viruses that are natural bacterial killers. Approximately, an incredible number (1031) of BPs are present in the world, which outnumber bacteria by an estimated tenfold (Skurnik, 2022). In recent times, BP therapy has gained a lot of attention as a powerful and potential therapeutic antibacterial approach (Taati Moghadam et al., 2020; Wei et al., 2020; Tamma and Suh, 2021). BPs are more specific to their targets than antibiotics, and therefore, BPs can eradicate the target pathogenic bacteria without altering the commensal microflora. On the other hand, broad-spectrum antibiotics kill pathogenic bacteria in infected individuals and disrupt microbes and normal bacterial flora (Rai and Kumar, 2022). Antibiotic-resistant bacterial pathogens are also accountable for causing severe nosocomial infections, particularly in individuals with life-threatening conditions. Furthermore, various pathogens can escape the activity of antibiotics by hiding in safe niches within the host; for instance, S. aureus has the ability to endure in the intracellular environment including abscesses or biofilms, which leads to persistent infections. The deficiency of new antibiotics and the rise of multidrug-resistant bacterial strains necessitate the development of alternative therapies, for example, BP-based therapies (Schmelcher and Loessner, 2021).
This review aims to explore the potential of BPs as an alternative to antibiotics in combating antimicrobial resistance. In addition, the advantages of BP-based therapies over antibiotics, phage-antibiotic synergy, the potential of antimicrobial BP-derived proteins, multiple formulation strategies of BP therapy, preclinical and clinical progress of BP-based therapeutics against clinically significant pathogens, and factors that need to be considered in BP therapy have been highlighted in this review.
Antibiotic resistance (AR) is a global phenomenon that even precedes the development and usage of antibiotics by human beings. Microorganisms can generate numerous biologically active molecules across diverse environments that possess antibacterial properties; some of these molecules have been further developed and are currently used as antibiotics. The levels of these molecules within natural environments are frequently under the clinically relevant thresholds, which indicates that resistance does not solely occur to evade their toxic activity. In nature, the interplay between antibiotics and AR mechanisms plays the role of a communication channel between the microbial community members instead of only antimicrobial agents. In addition, these molecules induce genotypic and phenotypic responses and shape the composition of the community. Therefore, genes conferring AR to modern antibiotics were also detected in ancient microbial communities (Perron et al., 2015). Along with the treatment of human infectious diseases, antibiotics are also utilized to mediate livestock growth and treat fish and crop diseases in aquaculture and agriculture (Ventola, 2015). As high as 180 mg of active antibiotic agent is used per kilogram of produced meat in the United States, an even higher proportion has been reported in other countries (Price et al., 2017; Gordillo Altamirano and Barr, 2019). A major problem of using antibiotics in agriculture is the release of millions of tons of antibiotics into environmental reservoirs and water effluents (Manyi-Loh et al., 2018). A lack of proper water treatment facilities further exacerbates this problem. Moreover, pharmaceutical waste and reduced distance between cities and farmlands also play a role in elevating the extents of antibiotic release and environmental persistence. Indeed, this continuous introduction of environmental microorganisms to a range of antibiotics has resulted in increased evolution and extended the range of AR genes in natural reservoirs. AR can also occur due to the genetic information transfer between organisms through transduction, conjugation, or transformation (Yelin and Kishony, 2018) (Figure 1).
Figure 1. Mechanisms of antibiotic resistance. Reproduced with permission from Elsevier (Yelin and Kishony, 2018).
Antibiotic-resistant bacteria found in the guts of animals exposed to antibiotics are a major source of horizontal transfer determinants. In addition, bacterial cells can attain non-genetically encoded, transient resistance through various mechanisms including persistence, metabolic dormancy, swarming adaptation, and growth in biofilms (Xu et al., 2022). Numerous resistance mechanisms can hinder the effectiveness of antibiotics at every step of their passage through the bacterial cell. First, bacteria can modify the structure of their cell envelope to repudiate the entry of the antibiotics or can generate efflux pumps to expel antibiotics (Yelin and Kishony, 2018) (Figure 1). Bacteria can inhibit the production of enzymes needed for the activation of antibiotics, or they can also destroy or alter antibiotics by producing enzymes including β-lactamases. Bacteria can hide, modify, or quantitatively alter the intended targets of the drug. Finally, bacteria can cause the activation of alternative metabolic signaling pathways to evade the toxic effects of antibiotics (Munita and Arias, 2016). Since antibiotic-resistant bacteria are rising at an alarming rate, alternative therapies are urgently required for the control of bacterial infections (Alaoui Mdarhri et al., 2022).
Bacteriophages (BPs) are viruses that can infect bacterial hosts (Skurnik, 2022). Typically, BPs share all the common features of viruses, and they cannot replicate by themselves. They need bacterial hosts for reproduction. BPs are small (50–200 nm), and they transmit genetic instructions for effective and fast replication. Generally, BPs are specific to a particular bacterial host; any one BP might infect multiple different species within a genus and many or most strains within a species. However, occasionally, it may affect very few or only one specific isolate of a species (Aslam et al., 2021). Several BPs infect bacteria from diverse genera and usually can do so only when they are phylogenetically closely associated (Tamma and Suh, 2021). The host range of BP can be broad (numerous distinct species or even distinct genera) or very narrow (where only a limited number of bacterial isolates assist replication). Indeed, the host preference or host range largely determines the therapeutic potential of BPs (Rai and Kumar, 2022).
A BP delivers the genomic content into a bacterium during a lytic infection cycle by attaching with the receptor(s) on its surface, and subsequently, it goes through viral replication in the cytosol through bacterial transcription, translation, and replication. After the generation of new BP particles, they evade through the cytoplasm by causing bacterial lysis (Figure 2). This mechanism is subsequently repeated by the new BP particles as they cause infection of additional susceptible cells. It has been reported that this mechanism plays a beneficial role in BP therapy, where lytic BPs are used as self-amplifying drugs that can locate and eradicate susceptible cells, which might be more effective as compared with antibiotics, since antibiotics are not capable of self-amplification (Kortright et al., 2019).
Figure 2. Replication cycles of bacteriophages. Reproduced with permission from Elsevier (Alaoui Mdarhri et al., 2022).
In the case of lysogeny or a lysogenic cycle, BPs incorporate their nucleic acids into the chromosomes of a cell instead of causing cell lysis. Furthermore, viral proteins integrate viral DNA into the bacterial chromosome; as a result, the virus exists as prophage DNA, which is replicated each time the cell divides. It has been observed that in some cases, viral prophages are maintained as circularized; small plasmids of DNA within the cell are also stably inherited. A single infected cell in this way can rapidly propagate the BP DNA without leading to cell lysis (Louten, 2022).
Various conditions including food poisoning, colorectal cancer, ulcerative colitis, and Crohn’s disease can disturb the intestinal flora. The upper digestive tract primarily contains Gram-positive facultative anaerobes and bacterial concentration in this tract at a rate of 103–104 CFU/mL. Nonetheless, in the colon, this concentration can increase up to 1011–1012 CFU/mL, which mostly contains various anaerobic bacteria including Enterobacter, Enterococcus, Clostridium, Eubacterium, Bifidobacterium, and Bacillus. Most of the studies on polymer-encapsulated BPs have explored their potential in treating gastrointestinal tract (GIT) infections. Various factors regarding GIT need to be considered when developing oral BP formulations for animal or human use, such as the impact of various digestive ferments (including trypsinogen, amylase, lipase, protease, and pepsin), harsh pH conditions, effects of bile salts, and varying retention times of different intestinal segments, including the ileum and duodenum. The main goal of polymeric encapsulation is to protect BPs from the adverse gastric environment to evade inactivation and reduction of BP titer (Silva Batalha et al., 2021). Indeed, polymeric carriers could be developed to withstand various pH values, for instance, pH of small intestine (pH 5.5–6.5), stomach (pH 1–3), and colon (pH 6.5–7.2). Several chemically modified biopolymers (such as polyamino acids, polycarbonates, polyamides, and aliphatic polyesters) are available commercially and licensed for preparing their coatings or microspheres (Ling et al., 2022). Alginates are widely utilized to encapsulate various BPs for oral delivery. Cross-linked gels are formed upon exposure of alginate to two valence cations, and extrusion (a physical encapsulation method) can be used for microencapsulation. Further addition of calcium carbonate in alginate gel beads can significantly enhance the acid-resistance capacity of encapsulated BPs (Colom et al., 2017). Polymer-encapsulated BPs with anti-acid agents are more stable in acidic environment than free BPs. Whey protein, chitosan, pectin, guar gum, and neutral gum can also be utilized to ameliorate the stability of alginate microparticles in acidic conditions. The gut flora can generate a range of substances such as β-xylosidase, urea dehydroxygenase, nitroreductase, β-galactosidase, α-arabinosidase, and β-glucosidase to use the undigested substrate in the small intestine. Multiple enzyme-sensitive polymers such as pectin-, methylcellulose-, hydroxypropyl-, and cellulose-derived biopolymers can react with the aforementioned enzymes and release on the target site, which can eventually result in the target site regulation of specific flora (Colom et al., 2017; Ling et al., 2022).
It is essential to exactly match the pathogens and BPs in BP-based therapies. Nonetheless, in vivo lysis properties and in vitro screening of BPs might not always match. Sometimes BP monotherapy is not adequate to fulfill the clinical requirements, even though BPs have the capacity to overcome antibiotic-induced AR. Therefore, a BP cocktail-based therapy is important to overcome the limitation of the narrow antibiogram of BP monotherapy. BP cocktails can be used to target multiple strains or a single bacterial strain of a single bacterial species, or even several bacterial species. Nevertheless, the purification and preparation processes of such cocktails are complex due to their complex composition. Moreover, the pharmacodynamic and pharmacokinetic properties of BPs derived from cocktails are erratic (Monteiro et al., 2019). Thus, the relative efficacy of each cocktail constituent should be assessed, and BPs with low-efficacy ought to be discarded (Abedon, 2017). Interestingly, BP cocktails could be further designed in a way that each BP is sequentially active, rather than being active simultaneously with the other BPs in a cocktail. Henceforth, even if resistance occurs because of continuous administration, new non-resistant BPs will continue to exert their effects. Studies with animal models demonstrated that the sequential BP cocktail administration can result in outstanding results in overcoming BP resistance and decreasing bacterial populations. BP cocktails are already available in Georgia and Russia as over-the-counter medicines to treat bacterial infections. Pyo BP developed by Eliava biopreparations is also used to treat suppurative and intestinal diseases caused by Proteus mirabilis, Streptococcus pyogenes, Pseudomonas aeruginosa, Proteus vulgaris, and Escherichia coli (Ling et al., 2022) [Eliava Institute of Bacteriophage (Russia), Microbiology and Virology in Tbilisi (Georgia) Microbiology].
The major two problems of the low therapeutic efficacy of oral BPs include inadequate retention time in the intestine and poor stability of BPs in gastric acid environment, which requires repeated administration of free BPs. There are several disadvantages of frequent or repeated administration including expensive, time-consuming, and poor patient compliance (Singla et al., 2016). These problems can be avoided by using positively charged liposomes. Liposomes can act as proton barriers and provide protection to BPs from gastric acids (Otero et al., 2019). Furthermore, the intestinal retention time of BPs can also be extended because of their positively charged surfaces (Cui et al., 2017; Kuerban et al., 2020). Enterobacteria BPs encapsulated in cationic liposomes were developed in a study conducted by Colom et al. (2017).
There are several advantages of cationic liposome-loaded BPs because of their positive charge on their surfaces including enhanced mucus adhesion and prevention of inactivation of BPs in gastric acid conditions, which further extends the intestinal residence time of BPs (Ma et al., 2016). Moreover, smaller particles of liposome-encapsulated BPs can enhance the chances of cell uptake in the host cytoplasm via membrane fusion or endocytosis (Ma et al., 2016; Fukuta et al., 2017), which can then inactivate various intracellular pathogens. Functionalization of liposomes is also possible to increase the clinical applications of BPs by altering the charge distribution of liposomes to extend the retention time, encapsulating multiple probes to observe the pharmacokinetics of liposome-loaded BPs, enhancing the circulation time in vivo, delaying the release of contents, and attaching specific targeting ligands to the surfaces of liposomes. Nonetheless, liposomes can attach and even fuse under specific conditions, which is undesirable for clinical applications and storage (Nieth et al., 2015; Ling et al., 2022).
Oral BPs have also been tested as a topical treatment against infectious gut microbiota. Typically, BPs do not enter into systemic circulation from the GIT. Moreover, intestinal carbohydrates and bile salts might block the bivalent metal ions that are essential for the replication of BPs. Thus, oral BPs encounter challenges in achieving a systemic therapeutic effect. On the other hand, parenteral administration of BPs is a more efficient way to permit BPs to exhibit a systemic therapeutic role. Intravenous administration of BPs has some drawbacks including poor compliance, maintenance of a costly cold chain, and chances of cross-contamination. However, these aforementioned problems can be solved by microneedle-mediated transdermal delivery of BPs. In this regard, a hollow polycarbonate microneedle array has been developed by the researchers. The bottom diameter and average height of this device were 750 μm and 995 μm, respectively, and the hollow aperture was 100 μm. It was observed that this device can fully perforate the skin and deliver BPs. Bioavailability can reach up to 100% through this transdermal delivery device. The width and depth of the residual skin pores are 600 μm and 210 μm, respectively, which can be closed rapidly following treatment. In addition, the functions of the epithelial barriers are not disrupted in this process. Nonetheless, it is hard for microneedles to transport stock solutions through all skin layers. It has been reported that microneedles do not have the capacity to cross all skin layers, resulting in a liquid pool on the skin surface (Ling et al., 2022).
In recent times, there has been growing interest in electrospun biopolymer fibers as delivery devices or reliable carriers for bioactive agents. BPs can also be fixed internally or wrapped on their surfaces (Korehei and Kadla, 2014; Díaz et al., 2018). There are several advantages of producing fibers via the electrospinning method, such as porosity, larger surface area, softness, and flexibility. Thus, these fibers can be used locally in the form of packaging materials or wound dressings and bandages with antibacterial properties. In addition to targeting inactivated bacterial pathogens, the bioactive surfaces are also effective in BP-mediated immobilization, identification, and detection of targeted microbes. It is possible to achieve a controlled release of BP particles with electrospun fibers by selecting appropriate materials. Interestingly, the controlled release kinetics of BPs can also be achieved by mixing different fiber polymers with different molecular weights (Ling et al., 2022).
In general, antibiotics can act against a wide range of microbial species and therefore can be used to treat a broad range of bacterial pathogens. However, numerous bacteria have already developed many mechanisms for obtaining AR. In this regard, genetic alterations including horizontal gene transfer (HGT) and mutation also play a role. In addition, various physiological factors including eradication of the antibiotics and enzymatic modification can play a role in altering efflux pump and cell permeability (Munita and Arias, 2016). Several resistance mechanisms developed by bacteria can be passed to others via HGT. In contrast, distantly related bacteria (non-host) are not likely to have ever been exposed to the BP of interest because of the host specificity of BPs. As a result, these bacteria are not likely to play a role in resistance genes to non-hosts through HGT (Cohan et al., 2020). Similarly, CRISPR-based BP immunity is unlikely to protect other taxa and therefore does not play a role in spreading resistance through HGT (Cohan et al., 2020). Thus, the probability of spread and development of BP resistance is markedly lower than that of the development of AR. Moreover, a synergistic effect can be achieved by the combined use of BPs and antibiotics (Gu Liu et al., 2020), since targeted bacterial strains struggle to simultaneously counter two different types of stress (Burmeister et al., 2020; Khan et al., 2022). A comparative advantage of BP-based therapies over antibiotics in the treatment of infections is presented in Table 1.
Phage-antibiotic synergy (PAS) is defined as the phenomenon where the combined effect of BPs and antibiotics is greater compared with the sum of either BPs or antibiotics alone (Chaudhry et al., 2017; Simon et al., 2021). It has been reported that certain antibiotics induce the generation of BPs via a bacterial host and form larger plaques (Figure 3) in the occurrence of antibiotics (Kamal and Dennis, 2015; Li et al., 2021). In addition, the release of progeny BPs from bacterial cells can occur due to the sublethal concentrations of some antibiotics. PAS can decrease the concentration of antibiotics utilized in therapy and ultimately decrease the emergence of AR in bacterial populations (Jo et al., 2016b). The killing mechanism of bacteria differs between BPs and antibiotics (Chaudhry et al., 2017). PAS involves several mechanisms that enhance phage production and/or antibiotic efficacy. Antibiotics such as β-lactams and quinolones induce cell filamentation, potentially aiding phage lysis enzyme (Knezevic et al., 2013). Some antibiotics, such as linezolid and tetracycline, increase plaque size and phage amplification, while others such as cefotaxime enhance phage burst size leading to higher phage concentrations (Ryan et al., 2012).
Subinhibitory antibiotic concentrations inhibit the emergence of phage and/or antibiotic-resistant mutants (Kebriaei et al., 2020). Phage therapy can sensitize bacteria to antibiotics, lowering the MIC and increasing susceptibility (Gu Liu et al., 2020). BPs can also degrade bacterial polysaccharides, aiding antibiotic diffusion. These mechanisms collectively underscore the potential of combined phage-antibiotic therapies against drug-resistant infections. Therefore, it has been suggested that BPs ought to be combined with antibiotics for even better control of bacteria than BPs alone (Torres-Barceló and Hochberg, 2016). The use of BPs and antibiotics is predominantly suggested for infections caused by Gram-positive bacteria such as multidrug-resistant Enterococcus strains and methicillin-resistant Staphylococcus aureus (MRSA). Nonetheless, in terms of killing S. aureus, PAS was found to be effective against the antibiotic-susceptible strains compared to the antibiotic-resistant ones (Jo et al., 2016a), which further indicates that antibiotics can cause alterations in bacterial membrane proteins that can cause alteration in the BP receptors. In a study, Simon et al. showed synergism between the lytic S. aureus BP Sb-1 at BP multiplicity of infection of 10−1 and 10 and oxacillin at concentrations varying from 5 to 100 μg/mL for most studied isolates of S. aureus. The combination of oxacillin and BP Sb-1 markedly reduced the level of bacteria than oxacillin alone (Simon et al., 2021). A study conducted by Malik S showed that combining an E. coli phage cocktail with antibiotics reduced MIC values, notably from 8 to 2 μg/mL for amikacin and from 32 to 8 μg/mL for fosfomycin. These findings underscore how phages serve as adjuvants, bolstering antibiotic effectiveness against drug-resistant E. coli strains (Malik et al., 2021).
Daptomycin (an antibiotic) is often used to treat infections caused by Enterococcus faecium, since 80–90% of Enterococcus faecium strains are resistant against ampicillin and vancomycin. It has also been reported that PAS synergy including daptomycin was linked with lower BP resistance. In another study, Morrisette et al. (2022) observed bactericidal activity with E. faecium BP cocktail and daptomycin in most regimens. In addition, daptomycin introduced to the BP averted BP resistance against daptomycin-resistant E. faecium (Łusiak-Szelachowska et al., 2022). Elevated BP titers and larger plaques were noticed when used in increasing antibiotic concentrations.
A study observed that the combination of low-dose meropenem and B. cepacia BP elevated the survival rate of Galleria mellonella larvae (Kamal and Dennis, 2015). In another study, Aghaee et al. used a combination of antibiotics and BPs against the P. aeruginosa strain PA14 isolated from a burn (Aghaee et al., 2021). This strain was treated with antibiotics at a sub-minimum inhibitory concentration (MIC) and MIC levels, a combination of BPs, a mixture of two BPs, and a single BP. Subsequently, the four lytic BPs were selected based on their performance in a primary efficiency of plating test. Moreover, out of these four primarily sequenced BPs, those with different infection properties and genetic features were selected. Interestingly, all designated BPs formed plaques on P. aeruginosa. Collectively, these findings suggested that a combination of one antibiotic and two BPs showed the highest killing potential against the strain of P. aeruginosa. In another study involving Citrobacter amalonaticus, synergistic effects were observed with the BP MRM57 at concentrations of 103 and 106 plaque forming units (PFU/ml), combined with a sublethal dose of antibiotics with a distinct mode of action (including tigecycline, cefepime-tazobactam, meropenem, gentamicin, fosfomycin, colistin, and carbenecillin) at 1/10 × MIC (Łusiak-Szelachowska et al., 2022; Manohar et al., 2022).
BP genomes encode several important enzymes and proteins to eradicate bacteria during infection. Polysaccharide depolymerizing enzymes (PDEs) and virus-associated peptidoglycan hydrolases (VAPGHs) are two such groups of proteins which are required for BPs to adsorb with and infect host bacteria (Roach et al., 2017). VAPGHs are typically found in the outermost layer of the protein shells of BPs, which is a component of the viral structure and plays a role in the local peptidoglycan layer degradation so that the tail tube structures of BPs release their genomes into the host. BP-encoded PDEs can cause the degradation of polysaccharide components found on the bacterial cell membrane surface, for instance, lipopolysaccharides derived from Gram-negative bacteria. Furthermore, PDEs also help BPs reach the host receptors by digesting the polysaccharides present on the bacterial cell membrane, which can further degrade biofilms (Maciejewska et al., 2018). There is a growing interest regarding BP-derived lysins or cell wall hydrolases as potential alternatives to antimicrobials. Lysins are also considered promising clinical candidates because of their differential virulence, low drug resistance, and rapid bactericidal action (Sharma et al., 2018).
BP-derived lysins possess enzymatic functions and can destroy non-divided bacterial cells, which can be a therapeutic option against recurrent or drug-resistant infections. Ectolysins and endolysins are the two major lysine enzymes. Ectolysins play a role in decomposing peptidoglycans externally to bacteria during BP DNA invasion. Endolysins also decompose the peptidoglycans of the cell wall at the end of the replication of BPs (Schmelcher et al., 2012). Various lysine enzymes such as amidases, peptidases, and glycosidases can split distinct chemical bonds according to the selectivity of lysins. The addition of a small quantity of lysin can rapidly decrease the optical density of turbid-suspended Gram-positive bacterial cultures (>108 CFU/mL) within minutes. Moreover, lysine does not exert any effect on eukaryotic cells since murein hydrolases are only present in bacteria. For instance, p128, a chimeric recombinant exolysin derived from BP K, completely inactivated prokaryotic cells and did not exhibit any cytotoxicity on eukaryotic cell lines including Hep 2 and Vero even at a high concentration of 2.5 mg/mL (Ling et al., 2022). The biofilm and capsule structure of bacteria play an important role in resistance that averts the actions of antibiotics. The capsule structures of numerous bacteria can assist pathogens to escape phagocytosis and mediate cell invasion, intravascular survival, and epithelial colonization. Similarly, the biofilm structure can protect the bacterial population against immune functions. Thus, it is possible to remove or target these structures by using BP-derived depolymerases. Moreover, it was confirmed that the synergistic effect of amoxicillin and depolymerase could destroy the biofilms of Klebsiella pneumoniae (Ling et al., 2022).
In mouse models, Waters et al. reported the full removal of a cystic fibrosis strain of P. aeruginosa in a study, where two BP doses were intranasally administered to infected mice 48/60 or 24/36 h following infection. Interestingly, after providing treatment at 144/156 h post-infection, a significant reduction was observed in 30% of mice, while complete eradication was observed in 70% of mice. It was also reported that BP therapy markedly ameliorated the survival rate of CF-like lung disease in mice following intranasal administration 2 h post-infection. Administering a higher dose of BP 4 days before the bacterial challenge completely protected the mice, which further suggests that prophylactic therapy with BP can avert chronic infections (Waters et al., 2017). In a different study, Semler et al. assessed various routes of BP delivery in a murine model of Burkholderia cepacia complex respiratory infection. Bacterial load was reduced by 100-fold when BP was administered through nebulization, whereas no reduction was detected following intraperitoneal administration (Semler et al., 2014). Collectively, these findings regarding curative and prophylactic treatment of respiratory infections with BP suggest that respiratory infections might be a potential target for effective BP therapy (Kortright et al., 2019).
Therapies with BP in the treatment of GIT bacterial infections can significantly decrease or even prevent virulent bacteria colonization without affecting the natural gut microbiota. Four days following an adherent-invasive E. coli challenge, Galtier et al. (2017) noticed that a preventative BP treatment averted the advancement of colitis symptoms and decreased colonization of bacteria in the gut of mice treated with dextran sodium sulfate. Prophylactic therapy with BP administered 2 h before bacterial challenge led to a 100% mortality rate in an insect model of Clostridium difficile colonization, whereas coadministration of BP and bacteria resulted in a 72% reduction in the mortality rate. Moreover, BP treatment 2 h after bacterial challenge led to a 30% reduction in the mortality rate (Nale et al., 2016). In the small intestine of infant mouse models, Yen et al. (2017) reported that prophylactic therapy with a BP cocktail decreased colonization of Vibrio cholerae when BPs were provided 3 and 6 h before bacterial challenge. Nonetheless, BP-resistant bacterial mutants were recovered following treatment, and reduced outcomes were observed with BP therapy when taken over 6 h prior to bacterial challenge as well as when a higher dose of V. cholerae was used to challenge mice (Yen et al., 2017). Indeed, the findings of the prophylactic therapy of GIT infections with BP are promising; however, more studies are required on the treatment after bacterial challenge, since prophylactic therapies are always not feasible in clinical settings (Kortright et al., 2019).
BP therapy for a number of localized infections such as infected burns, urinary tract infections, and otitis has a great potential to completely avoid the usage of chemical antibiotics. Moreover, the usage of chemical antibiotics for hospital-acquired and surgical infections is often limited, since this usage can result in strains with extreme AR. Several studies reported positive outcomes in using bacteriophages (BPs) for infected ulcers (Fish et al., 2018; Morozova et al., 2018). Notably, integrating BPs into biodegradable wound dressings with sustained antimicrobial release, as demonstrated by Kvachadze et al. (2011), showed promising results. The capacity of BP to treat an E. coli-caused urinary tract infection was investigated in a murine model. Interestingly, 48 h after BP therapy, BP administered through the intraperitoneal route after 24 h of bacterial challenge reduced the bacterial load by 100-fold in the kidneys after 48 h of BP therapy (Dufour et al., 2016). Treatment with the same BP markedly reduced the bacterial load in an E. coli pneumonia model; however, the treatment was not effective in an E. coli model of sepsis (Kortright et al., 2019).
Various studies have already assessed the effectiveness of BP in treating systemic infections. In a study, Alemayehu et al. reported the efficacy of two phages (φNH-4 and φMR299-2) in combating clinical Pseudomonas strains in murine lungs and biofilm environments, bolstering the appeal of phage therapy as a strategy for managing multidrug-resistant Pseudomonas lung infections in CF patients (Alemayehu et al., 2012). In another study, the administration of a single intraperitoneal injection of the recently discovered phage EF-P29 showed effectiveness against vancomycin-resistant Enterococcus faecalis (VREF) strains, successfully combating bacteremia in murine models. This highlights the considerable potential of phage therapy in managing systemic VREF infections (Nasr Azadani et al., 2020). Multiple factors determine the favorable outcome of BP in the treatment of systemic infections, which needs to be rigorously assessed before broader usage of BP in sepsis treatment in humans (Kortright et al., 2019).
A study conducted by Fernando L et al. investigates the effectiveness of combining phage therapy with antibiotics against Acinetobacter baumannii infections. Using a murine model, researchers found that the combination treatment (PBS, phage øFG02, and ceftazidime) significantly reduced bacterial burden compared to controls or single treatments. Interestingly, phage (phage øFG02) therapy alone led to bacterial resistance, but these resistant strains became more susceptible to antibiotics. This suggests that phage therapy can sensitize bacteria to antibiotics, offering a promising strategy against antibiotic-resistant infections (Altamirano et al., 2022). On the other hand, no mortality was observed with the combination therapy of BP and enrofloxacin. In a similar study, Oechslin et al. reported that treatment with a combination of BP and ciprofloxacin led to a 10,000-fold decrease in bacterial load in comparison with the ciprofloxacin or BP treatment alone in rats with experimental P. aeruginosa endocarditis. Moreover, it was observed that the combination of BP and ciprofloxacin led to synergistic in vitro and in vivo killing of P. aeruginosa (Oechslin et al., 2017). Since combined BP therapy with chemical antibiotics has great potential, more studies are required to reveal the possible synergy between BP and antibiotics (Jennes et al., 2017; Hoyle et al., 2018; Kortright et al., 2019).
Eight clinical trials registered on clinicaltrials.gov focus on evaluating bacteriophage therapy for SSTIs, predominantly in phase I or phase I/II stages. Atopic dermatitis, venous leg ulcers, pressure ulcers, and diabetic foot ulcers are all included in these trials (Hitchcock et al., 2023).
A clinical trial has evaluated the effectiveness and safety of BPs in the treatment of burn wounds infected by P. aeruginosa (Jault et al., 2019). In this trial, a cocktail of 12 BPs with lytic action against P. aeruginosa was introduced to an alginate template that was directly applied to the wound, while a topical application of 1% sulfadiazine silver was used in the control group. Moreover, the average sterilization time for the control group and BP-treated group was 47 h and 144 h, respectively. It was observed that these unpredictably poor outcomes could occur owing to the administration of a lower BP dose than envisioned. A dose of 200–2,000 PFU was utilized rather than the expected 2 × 107 PFU dose, and the level of the BP cocktail dropped throughout the study. It was concluded that the lack of effectiveness of the therapy could be due to the unintended alteration in the treatment protocol (Kortright et al., 2019).
Another study, REVERSE, assessing a five-phage cocktail targeting multiple bacteria, showed promising safety results with efficacy data pending (Technophage, SA, 2022). The WPP-201 trial, a phase I study for venous leg ulcers, concluded that the phage therapy was safe, but no phase II efficacy study has been identified (Wolcott, 2011). These clinical trials represent significant steps in evaluating the efficacy and safety of bacteriophage therapy for various skin and wound infections. While some trials have shown promising safety results, efficacy data are still pending for many.
A clinical trial was conducted by Sarker et al. (2016) to evaluate the effectiveness and safety of two different cocktails of BP that target pathogenic E. coli in diarrheal diseases. In this trial, only the individuals exhibiting acute onset of dehydrating diarrhea were included. The BP therapy involved oral administration of either a T4-like BP cocktail (T) consisting of 11 BPs and a commercial BP cocktail (M) from Russia containing at least 17 different BPs in oral rehydration solution, while the placebo group received a standard treatment with an oral rehydration solution. Any significant differences and adverse effects were not observed between the placebo and BP therapy groups. Furthermore, it was not clear whether the BPs showed lytic activities against the exact strains of E. coli responsible for causing the disease, and no further actions were taken before the BP administration to buffer the stomach. Thus, a substantial number of BP particles were possibly not able to endure the low-pH environment of the stomach, and the surviving particles were not able to increase because of the deficiency of a proper host (Sarker et al., 2017).
Only a few ongoing clinical trials focus on bacteremia and phage therapy. One of these trials is a phase I study assessing the safety, tolerability, and pharmacodynamics of three different doses of an E. coli phage genetically modified to deliver a CRISPR/Cas payload (SNIPR001). Participants receive the treatment twice a day for 7 days. The second trial, DiSArm, is a phase II study investigating the safety, tolerability, and efficacy of S. aureus-specific phage cocktail (AP-SA02) alongside antibiotics for individuals with S. aureus bacteremia (SNIPR, 2023; Armata Pharmaceuticals, Inc, 2024a).
The majority of the seven ongoing clinical trials target lung infections associated with cystic fibrosis (CF), such as those brought on by Pseudomonas aeruginosa. The Cystic Fibrosis Foundation is backing these trials since P. aeruginosa has a major negative effect on the health of CF sufferers. There are three CF trials recruiting subjects (Koff, 2023). The MUCOPHAGES experiment, which ended in April 2012, looked at how 10 bacteriophages target pseudomonas-affected 59 CF patients’ induced sputum. No results, though, have been made public (University Hospital, Montpellier, 2013). The same product, an inhaled P. aeruginosa phage cocktail known as AP-PA02, is the subject of two lung-targeted phage trials called Tailwind and SWARM-Pa. Phase II trials Tailwind and SWARM-Pa are seeking volunteers with chronic P. aeruginosa infections and non-CF bronchiectasis (Armata Pharmaceuticals, Inc, 2024b).
Wright et al. conducted a clinical trial to evaluate the preliminary effectiveness and safety of BP therapy in treating P. aeruginosa-caused chronic otitis. In this trial, a cocktail of 6 BPs with lytic properties was used to treat individuals with P. aeruginosa-caused chronic otitis (Wright et al., 2009). A marked cumulative reduction in the bacterial counts was observed in the BP treatment group, while no marked cumulative alteration was observed in bacterial counts in the placebo group. Interestingly, by day 42, three participants from each group experienced undetectable P. aeruginosa levels. In the BP treatment group, BPs were obtained for an average of 23 days from participants, which suggested that bacteria became BP resistant, BP was not able to reach the infection site, or BPs were cleared following the resolution of the infection. The BP therapy was found to be safe in otitis treatment, since no severe adverse events were observed in either group (Wright et al., 2009). A summary of various clinical and preclinical findings on the potential of BP-based therapeutics against infections is shown in Table 2.
Table 2. A summary of clinical and preclinical findings on the potential of bacteriophage-based therapeutics against infections.
In a systematic review of clinical trials, bacteriophage administration resulted in significant microbiological improvements, with 62% of articles reporting complete bacterial eradication or urine sterilization (Al-Anany et al., 2023). Additionally, clinical symptom improvement was observed in 97% of human patients treated with bacteriophages. The trials also reported low rates of relapse or re-infection post-treatment, with only 0.8% experiencing re-infection after 2–4 months. This suggests that bacteriophage therapy may offer durable protection against UTIs. Some studies investigated combination therapy involving both bacteriophages and antibiotics. These trials showed enhanced efficacy compared with bacteriophage therapy alone, highlighting the potential synergistic effects of combining these treatments (Terwilliger et al., 2021).
Six clinical trials evaluate the use of phage preparations to treat infections in the genitourinary tract, focusing mainly on asymptomatic UTIs, with one trial targeting bacterial vaginosis. Among these trials, two have been completed. In the first completed trial, Pyo phage, a commercially available treatment from the Eliava Institute in Georgia, was assessed for its efficacy in treating urinary tract infections in patients undergoing prostate transurethral resection. Participants were randomly assigned to receive intravesicular Pyo phage, standard care, or placebo bladder irrigation. The study found that the phage treatment was comparable to standard care or placebo, but low treatment titers may have affected efficacy (Leitner et al., 2017, 2021). The second completed trial, a phase Ib study, examined a CRISPR-Cas3-enhanced phage cocktail targeting E. coli. This trial focused on understanding the pharmacokinetics, pharmacodynamics, and safety of the treatment when administered via the intraurethral route. Currently, one of the ongoing trials is recruiting participants for phase II/III trials to assess the efficacy of different doses of the same phage cocktail for acute uncomplicated UTIs caused by multi-drug-resistant E. coli in female participants (Kim et al., 2021). These trials highlight the potential of phage therapy in treating genitourinary tract infections, emphasizing the importance of factors such as phage concentration at the infection site and pathogen load in designing effective treatment regimens.
Biofilm formation is a crucial virulence factor in the case of most MDR pathogens. BPs have been extensively evaluated for their roles against bacterial biofilms. Several studies reported that specific BPs can cause the degradation of biofilms of S. aureus, K. pneumoniae, E. coli, and Acinetobacter baumannii (Lin et al., 2017; Dakheel et al., 2019; Wintachai et al., 2020; Osman et al., 2023). This property has been credited to the generation of multiple hydrolases in the capsid that can successfully disturb and disperse biofilms of bacteria (Lin et al., 2017). Interestingly, some BPs possess tail fibers along with inherent depolymerase functions (Kvachadze et al., 2011). However, more studies are required regarding the mechanisms and roles of BPs in disturbing biofilms (Carascal et al., 2022).
Typically, the storage medium was mixed with therapeutic BPs to generate a formulation of BP. BP formulations ought to be prepared according to the recognized quality assurance standards and current good manufacturing practices like any other therapies. Moreover, BP formulations should be free from impurities and contaminants including host cell proteins and endotoxins (Jennes et al., 2017; Furfaro et al., 2018; Lehman et al., 2019). As the delivery routes largely determine the effectiveness of BP treatment, several studies have evaluated distinct routes of administering BPs for therapeutic purposes. Topical application for burn and skin infections is a common method of administering BP therapy (Morozova et al., 2018). Oral administration is another mode of administering BPs. Since BPs will be taken internally, BPs need to be stable at human physiological pH (4–9) and body temperature (35–39°C). Various other factors such as bile salts and low gastric pH also need to be considered while formulating BPs. Thus, BP formulations can be enclosed in a gel or include bicarbonate water (Kakasis and Panitsa, 2019). In this regard, in chickens, chitosan nanoparticles loaded with BP ΦKAZ14 were used as an alternate approach to oral delivery. Researchers also suggested that the in vivo stability of chitosan-BP nanoparticles was higher than standalone BP (Kaikabo et al., 2016). In chicken, this nanoparticle also regulated the bacterial colonization of avian pathogenic E. coli and reduced the colibacillosis symptoms (Kaikabo et al., 2017). Various other routes of BP administration include the systemic route via intramuscular or intraperitoneal injection (Criscuolo et al., 2017; Jennes et al., 2017), the respiratory route via intranasal spray (Casey et al., 2018), and rectal route via fecal microbiota transplant. However, these routes are yet to be demonstrated clinically or in vivo (Carascal et al., 2022).
Bacteriophage (BP) resistance is another important factor that needs to be carefully considered in BP therapy. It is assumed that the evolutionary pressure exhibited by the BP markedly surpasses any resistance mechanisms gained by the target pathogen (El-Shibiny et al., 2017; Harper, 2018). Moreover, the fast evolution of BPs helps in ameliorating their virulence toward the target pathogen, which makes adaptive bacterial resistance ineffective to BPs. Nonetheless, the existence of BP-resistant bacteria is unavoidable and ought to be considered while developing BP-based therapies (Casey et al., 2018). In Singapore, a small colony variant of P. aeruginosa was found to be resistant to PB1 (a BP) has been described in Singapore (Lim et al., 2016); however, its susceptibility to other BPs is yet to be explored. Researchers should consider the bacterial mechanisms against BPs following their administration in order to prevent BP resistance. Such mechanisms might involve abortive infection, superinfection exclusion, and CRISPR-Cas system. BP cocktails also could be considered to avert the rising BP resistance (Carascal et al., 2022).
As with antibiotics, it is essential to determine the pharmacodynamic properties of BPs to substantiate an efficient dosage regimen for the effective control of a range of severe infections. An appropriate BP dosage is required to confirm that an adequate level of virus is present in the infection site to encounter the target pathogen (Zhang et al., 2018). A higher level (108–1010) of BPs is administered to the patients (typically in a single-dose regimen) in an inundated therapy to destroy numerous target pathogens (Danis-Wlodarczyk et al., 2021). However, some studies have revealed that a higher titer of BPs involves a risk of causing BP-mediated lysis of non-targeted bacterial cells. This can lead to disturbances in the delicate balance of the commensal gut microbiota, which plays a crucial role in maintaining health. However, in a study, it was significantly less when compared to the broad-spectrum antibiotic activity on the microbial flora. Indeed, further studies are required to establish this finding (Barbu et al., 2016; Cieplak et al., 2018).
Not all BPs can be used for therapeutic purposes. In addition, therapeutic BPs ought to be lytic to confirm the destruction of the target bacteria (Lehman et al., 2019). The BP also ought to have a short generation time, species-specific action, and an increased rate of adsorption to the target bacteria (Khawaja et al., 2016). As with antibiotics, a BP equivalent of an antibiogram or profiling of the BPs in a phagogram is also required (Barbu et al., 2016). In a phagogram, the BP efficacy is continuously tested against a well-defined collection of pathogens known as a diversity panel or a pathogen library (Casey et al., 2018). The phagogram profiling can make sure that the therapeutic BPs are specific to their target pathogen (Abdulamir et al., 2015; Jennes et al., 2017; Furfaro et al., 2018; Phothichaisri et al., 2018; Dakheel et al., 2019; Tan, 2020; Rahimi-Midani et al., 2021; Chung et al., 2023).
Bacteriophage therapy to treat bacterial infections encounters several obstacles and opportunities in terms of applicability and future development. As mentioned earlier, these include bacteriophage formulation, understanding its pharmacodynamic properties, the development of phage resistance in bacteria, and finally ethical and legal considerations (Zalewska-Piątek, 2023).
Optimizing the administration of bacteriophage preparations is crucial to maximizing their therapeutic potential. Understanding the pharmacokinetics of phages, including their stability, distribution, and clearance in the body, is essential for determining the most effective dosage regimens and administration routes (Dąbrowska, 2019). Encapsulation technologies, such as microencapsulation or liposome encapsulation, can protect phages from degradation in the gastrointestinal tract and enhance their bioavailability. Finally, advancing clinical experience and monitoring adverse reactions are paramount for refining phage therapy protocols and ensuring patient safety (Loh et al., 2021). Real-time observation of treatment outcomes, coupled with rigorous data collection and analysis, can provide valuable insights into the efficacy and safety of phage therapy in diverse clinical settings. Phage therapy necessitates the use of lytic phages, ensuring high purification, as lysogenic BPs are unable to lyse host bacteria and also hinder the lytic action of other phages upon integration. Moreover, they pose a significant concern as they can transfer toxins and antibiotic-resistance genes to bacteria (Carascal et al., 2022).
Regarding bacterial resistance to BPs, some bacteria develop defense techniques against phages, such as inhibiting surface receptors, altering mechanisms, and secreting extracellular polymeric capsules. These defenses can impair the capacity of bacteriophages to efficiently infect and destroy bacteria (Wang and Leptihn, 2024). Furthermore, BPs have a narrow cleavage spectrum which limits their effectiveness against different bacterial strains. This suggests that some bacteriophages may only be effective against a restricted group or species of bacteria, rendering them ineffective against a wide range of pathogens (Chung et al., 2023). Hence, the emergence of bacterial resistance mechanisms to bacteriophages emphasizes the need for novel techniques. Using a bacterial cocktail, which includes several phage types that target different bacterial strains, can improve treatment efficiency while decreasing the possibility of resistance development (Rahimi-Midani et al., 2021). Genetic engineering of BPs would increase their host range or boost their lytic activity against certain bacteria (Sun et al., 2023).
Additionally, ethical and legal issues encompass various aspects such as patient safety, informed consent, regulatory approval, and research ethics. The strategy is to establish a comprehensive phage bank and streamlined screening protocols to isolate phages that target antibiotic-resistant bacteria effectively (Anomaly, 2020; Zalewska-Piątek, 2023).
It facilitates the swift matching of BPs to bacterial isolates from infected patients, allowing for personalized treatment. Globally, only a few centers, such as those in Georgia, Belgium, Poland, the United States, and Australia, persist in offering phage-based treatments. These centers offer valuable data on therapy efficacy and conduct clinical trials to further enhance treatment outcomes (Zalewska-Piątek, 2023).
The objective is to eliminate bacterial infections in patients via cell lysis while minimizing adverse effects and preserving the natural microflora.
The growing global crisis of AR has inspired the development of BPs as an alternative to antibiotics. Indeed, BPs have great potential as effective, promising, and novel therapeutic agents to fight against AR. Despite the aspirations and novelty of the usage of BPs as an alternative to antibiotics, more studies are required to explore the clinical applications of these therapeutic BPs. As antimicrobial resistance is a global issue now, it is crucial to explore the potentiality of BPs as an effective alternative to antibiotics. However, more preclinical and clinical studies are required on the site-specificity, dosage regimens, half-life, and safety profile of the BPs to demonstrate their safety, efficacy, and potential as alternatives to antibiotics.
AS: Conceptualization, Methodology, Project administration, Formal Analysis, Writing – review & editing, Writing – original draft, Funding acquisition.
The author(s) declare financial support was received for the research, authorship, and/or publication of this article. The authors extend their appreciation to the Deanship of Research and Graduate Studies at King Khalid University for funding this work through small group research under grant number RGP1/131/45.
The author declares that the research was conducted in the absence of any commercial or financial relationships that could be construed as a potential conflict of interest.
All claims expressed in this article are solely those of the authors and do not necessarily represent those of their affiliated organizations, or those of the publisher, the editors and the reviewers. Any product that may be evaluated in this article, or claim that may be made by its manufacturer, is not guaranteed or endorsed by the publisher.
Abdulamir, A. S., Jassim, S. A. A., Hafidh, R. R., and Bakar, F. A. (2015). The potential of bacteriophage cocktail in eliminating methicillin-resistant Staphylococcus aureus biofilms in terms of different extracellular matrices expressed by PIA, cia A-D and FnBPA genes. Ann. Clin. Microbiol. Antimicrob. 14:49. doi: 10.1186/s12941-015-0106-0
Abedon, S. T. (2017). Information phage therapy research should report. Pharmaceuticals 10:43. doi: 10.3390/ph10020043
Aghaee, B. L., Khan Mirzaei, M., Alikhani, M. Y., Mojtahedi, A., and Maurice, C. F. (2021). Improving the inhibitory effect of phages against Pseudomonas aeruginosa isolated from a burn patient using a combination of phages and antibiotics. Viruses 13:334. doi: 10.3390/v13020334
Al-Anany, A. M., Hooey, P. B., Cook, J. D., Burrows, L. L., Martyniuk, J., Hynes, A. P., et al. (2023). Phage therapy in the management of urinary tract infections: a comprehensive systematic review. Phage 4, 112–127. doi: 10.1089/phage.2023.0024
Alaoui Mdarhri, H., Benmessaoud, R., Yacoubi, H., Seffar, L., Guennouni Assimi, H., Hamam, M., et al. (2022). Alternatives therapeutic approaches to conventional antibiotics: advantages, limitations and potential application in medicine. Antibiotics (Basel) 11:1826. doi: 10.3390/antibiotics11121826
Alemayehu, D., Casey, P. G., McAuliffe, O., Guinane, C. M., Martin, J. G., Shanahan, F., et al. (2012). Bacteriophages ϕMR299-2 and ϕNH-4 can eliminate Pseudomonas aeruginosa in the murine lung and on cystic fibrosis lung airway cells. mBio 3, e00029–e00012. doi: 10.1128/mBio.00029-12
Altamirano, F. L. G., Kostoulias, X., Subedi, D., Korneev, D., Peleg, A. Y., Barr, J. J., et al. (2022). Phage-antibiotic combination is a superior treatment against Acinetobacter baumannii in a preclinical study. EBioMedicine 80.
Anomaly, J. (2020). The future of phage: ethical challenges of using phage therapy to treat bacterial infections. Public Health Ethics 13, 82–88. doi: 10.1093/phe/phaa003
Armata Pharmaceuticals, Inc. (2024a). Phase 1b/2a, randomized, double-blind, placebo-controlled, multiple ascending dose study of safety, tolerability, and efficacy of intravenous AP-SA02 as an adjunct to best available antibiotic therapy for the treatment of adults with bacteremia due to Staphylococcus Aureus. clinicaltrials.gov
Armata Pharmaceuticals, Inc. (2024b). A phase 1b/2a, multi-center, double-blind, randomized, placebo-controlled, single and multiple ascending dose study to evaluate the safety and tolerability of AP-PA02 multi-phage therapeutic candidate for inhalation in subjects with cystic fibrosis and chronic pulmonary Pseudomonas Aeruginosa (Pa) infection. clinicaltrials.gov
Aslam, B., Arshad, M. I., Aslam, M. A., Muzammil, S., Siddique, A. B., Yasmeen, N., et al. (2021). Bacteriophage proteome: insights and potentials of an alternate to antibiotics. Infect. Dis. Ther. 10, 1171–1193. doi: 10.1007/s40121-021-00446-2
Aslam, B., Wang, W., Arshad, M. I., Khurshid, M., Muzammil, S., Rasool, M. H., et al. (2018). Antibiotic-resistance: a rundown of a global crisis. Infect. Drug Resist. 11, 1645–1658. doi: 10.2147/IDR.S173867
Baloch, Z., Aslam, B., Muzammil, S., Khurshid, M., Rasool, M. H., and Ma, K. (2018). Selection inversion: a probable tool against antibiotic resistance. Infect. Drug Resist. 11, 1903–1905. doi: 10.2147/IDR.S176759
Barbu, E. M., Cady, K. C., and Hubby, B. (2016). Phage therapy in the era of synthetic biology. Cold Spring Harb. Perspect. Biol. 8:a023879. doi: 10.1101/cshperspect.a023879
Burmeister, A. R., Fortier, A., Roush, C., Lessing, A. J., Bender, R. G., Barahman, R., et al. (2020). Pleiotropy complicates a trade-off between phage resistance and antibiotic resistance. Proc. Natl. Acad. Sci. USA 117, 11207–11216. doi: 10.1073/pnas.1919888117
Carascal, M. B., dela Cruz-Papa, D. M., Remenyi, R., MCB, C., and Destura, R. V. (2022). Phage revolution against multidrug-resistant clinical pathogens in Southeast Asia. Front. Microbiol. 13:13. doi: 10.3389/fmicb.2022.820572
Casey, E., Van Sinderen, D., and Mahony, J. (2018). In vitro characteristics of phages to guide ‘real life’ phage therapy suitability. Viruses 10:163. doi: 10.3390/v10040163
Chanishvili, N. (2012). “Chapter 1 – Phage therapy—History from Twort and d’Herelle through soviet experience to current approaches” in Advances in virus research, vol. 83. eds. M. Łobocka and W. Szybalski (Academic Press), 3–40.
Chaudhry, W. N., Concepción-Acevedo, J., Park, T., Andleeb, S., Bull, J. J., and Levin, B. R. (2017). Synergy and order effects of antibiotics and phages in killing Pseudomonas aeruginosa biofilms. PLoS One 12:e0168615. doi: 10.1371/journal.pone.0168615
Chegini, Z., Khoshbayan, A., Vesal, S., Moradabadi, A., Hashemi, A., and Shariati, A. (2021). Bacteriophage therapy for inhibition of multi drug-resistant uropathogenic bacteria: a narrative review. Ann. Clin. Microbiol. Antimicrob. 20:30. doi: 10.1186/s12941-021-00433-y
Chung, K. M., Liau, X. L., and Tang, S. S. (2023). Bacteriophages and their host range in multidrug-resistant bacterial disease treatment. Pharmaceuticals (Basel) 16:1467. doi: 10.3390/ph16101467
Cieplak, T., Soffer, N., Sulakvelidze, A., and Nielsen, D. S. (2018). A bacteriophage cocktail targeting Escherichia coli reduces E. coli in simulated gut conditions, while preserving a non-targeted representative commensal normal microbiota. Gut Microbes 9, 391–399. doi: 10.1080/19490976.2018.1447291
Cohan, F. M., Zandi, M., and Turner, P. E. (2020). Broadscale phage therapy is unlikely to select for widespread evolution of bacterial resistance to virus infection. Virus Evol. 6:veaa060. doi: 10.1093/ve/veaa060
Colom, J., Cano-Sarabia, M., Otero, J., Aríñez-Soriano, J., Cortés, P., Maspoch, D., et al. (2017). Microencapsulation with alginate/CaCO3: a strategy for improved phage therapy. Sci. Rep. 7:41441. doi: 10.1038/srep41441
Criscuolo, E., Spadini, S., Lamanna, J., Ferro, M., and Burioni, R. (2017). Bacteriophages and their immunological applications against infectious threats. J Immunol Res 2017, 3780697–3780613. doi: 10.1155/2017/3780697
Cui, H., Yuan, L., and Lin, L. (2017). Novel chitosan film embedded with liposome-encapsulated phage for biocontrol of Escherichia coli O157: H7 in beef. Carbohydr. Polym. 177, 156–164. doi: 10.1016/j.carbpol.2017.08.137
Dąbrowska, K. (2019). Phage therapy: what factors shape phage pharmacokinetics and bioavailability? Systematic and critical review. Med. Res. Rev. 39, 2000–2025. doi: 10.1002/med.21572
Dakheel, K. H., Rahim, R. A., Neela, V. K., Al-Obaidi, J. R., Hun, T. G., Isa, M. N. M., et al. (2019). Genomic analyses of two novel biofilm-degrading methicillin-resistant Staphylococcus aureus phages. BMC Microbiol. 19:114. doi: 10.1186/s12866-019-1484-9
Danis-Wlodarczyk, K., Dąbrowska, K., and Abedon, S. T. (2021). Phage therapy: the pharmacology of antibacterial viruses. Curr. Issues Mol. Biol. 40, 81–164. doi: 10.21775/cimb.040.081
Díaz, A., Del Valle, L. J., Rodrigo, N., Casas, M. T., Chumburidze, G., Katsarava, R., et al. (2018). Antimicrobial activity of poly (ester urea) electrospun fibers loaded with bacteriophages. Fibers 6:33. doi: 10.3390/fib6020033
Dufour, N., Clermont, O., Combe, B. L., Messika, J., Dion, S., Khanna, V., et al. (2016). Bacteriophage LM33_P1, a fast-acting weapon against the pandemic ST131-O25b: H4 Escherichia coli clonal complex. J. Antimicrob. Chemother. 71, 3072–3080. doi: 10.1093/jac/dkw253
El-Shibiny, A., El-Sahhar, S., and Adel, M. (2017). Phage applications for improving food safety and infection control in Egypt. J. Appl. Microbiol. 123, 556–567. doi: 10.1111/jam.13500
Fish, R., Kutter, E., Wheat, G., Blasdel, B., Kutateladze, M., and Kuhl, S. (2016). Bacteriophage treatment of intransigent diabetic toe ulcers: a case series. J. Wound Care 25, S27–S33. doi: 10.12968/jowc.2016.25.Sup7.S27
Fish, R., Kutter, E., Wheat, G., Blasdel, B., Kutateladze, M., and Kuhl, S. (2018). Compassionate use of bacteriophage therapy for foot ulcer treatment as an effective step for moving toward clinical trials. Methods Mol. Biol. 1693, 159–170. doi: 10.1007/978-1-4939-7395-8_14
Fukuta, T., Asai, T., Kiyokawa, Y., Nakada, T., Bessyo-Hirashima, K., Fukaya, N., et al. (2017). Targeted delivery of anticancer drugs to tumor vessels by use of liposomes modified with a peptide identified by phage biopanning with human endothelial progenitor cells. Int. J. Pharm. 524, 364–372. doi: 10.1016/j.ijpharm.2017.03.059
Furfaro, L. L., Payne, M. S., and Chang, B. J. (2018). Bacteriophage therapy: clinical trials and regulatory hurdles. Front. Cell. Infect. Microbiol. 8:376. doi: 10.3389/fcimb.2018.00376
Galtier, M., de Sordi, L., Sivignon, A., de Vallée, A., Neut, C., Rahmouni, O., et al. (2017). Bacteriophages targeting adherent invasive Escherichia coli strains as a promising new treatment for Crohn’s disease. J. Crohn's Colitis 11, 840–847. doi: 10.1093/ecco-jcc/jjw224
Gordillo Altamirano, F. L., and Barr, J. J. (2019). Phage therapy in the postantibiotic era. Clin. Microbiol. Rev. 32. doi: 10.1128/cmr.00066-18
Gu Liu, C., Green, S. I., Min, L., Clark, J. R., Salazar, K. C., Terwilliger, A. L., et al. (2020). Phage-antibiotic synergy is driven by a unique combination of antibacterial mechanism of action and stoichiometry. MBio 11:10.1128/mbio.01462-20. doi: 10.1128/mbio.01462-20
Harper, D. R. (2018). Criteria for selecting suitable infectious diseases for phage therapy. Viruses 10:177.
Hitchcock, N. M., Devequi Gomes Nunes, D., Shiach, J., Valeria Saraiva Hodel, K., Dantas Viana Barbosa, J., Alencar Pereira Rodrigues, L., et al. (2023). Current clinical landscape and global potential of bacteriophage therapy. Viruses 15:1020. doi: 10.3390/v15041020
Hoyle, N., Zhvaniya, P., Balarjishvili, N., Bolkvadze, D., Nadareishvili, L., Nizharadze, D., et al. (2018). Phage therapy against Achromobacter xylosoxidans lung infection in a patient with cystic fibrosis: a case report. Res. Microbiol. 169, 540–542. doi: 10.1016/j.resmic.2018.05.001
Jault, P., Leclerc, T., Jennes, S., Pirnay, J. P., Que, Y.-A., Resch, G., et al. (2019). Efficacy and tolerability of a cocktail of bacteriophages to treat burn wounds infected by Pseudomonas aeruginosa (Phago burn): a randomised, controlled, double-blind phase 1/2 trial. Lancet Infect. Dis. 19, 35–45. doi: 10.1016/S1473-3099(18)30482-1
Jennes, S., Merabishvili, M., Soentjens, P., Pang, K. W., Rose, T., Keersebilck, E., et al. (2017). Use of bacteriophages in the treatment of colistin-only-sensitive Pseudomonas aeruginosa septicaemia in a patient with acute kidney injury—a case report. Crit. Care 21:129. doi: 10.1186/s13054-017-1709-y
Jo, A., Ding, T., and Ahn, J. (2016a). Synergistic antimicrobial activity of bacteriophages and antibiotics against Staphylococcus aureus. Food Sci. Biotechnol. 25, 935–940. doi: 10.1007/s10068-016-0153-0
Jo, A., Kim, J., Ding, T., and Ahn, J. (2016b). Role of phage-antibiotic combination in reducing antibiotic resistance in Staphylococcus aureus. Food Sci. Biotechnol. 25, 1211–1215. doi: 10.1007/s10068-016-0192-6
Jun, J. W., Shin, T. H., Kim, J. H., Shin, S. P., Han, J. E., Heo, G. J., et al. (2014). Bacteriophage therapy of a Vibrio parahaemolyticus infection caused by a multiple-antibiotic–resistant O3: K6 pandemic clinical strain. J. Infect. Dis. 210, 72–78. doi: 10.1093/infdis/jiu059
Kaikabo, A. A., Abdul Karim, S. M., and Abas, F. (2017). Evaluation of the efficacy of chitosan nanoparticles loaded ΦKAZ14 bacteriophage in the biological control of colibacillosis in chickens. Poult. Sci. 96, 295–302. doi: 10.3382/ps/pew255
Kaikabo, A. A., Sabo Mohammed, A., and Abas, F. (2016). Chitosan nanoparticles as carriers for the delivery of ΦKAZ14 bacteriophage for oral biological control of colibacillosis in chickens. Molecules 21:256. doi: 10.3390/molecules21030256
Kakasis, A., and Panitsa, G. (2019). Bacteriophage therapy as an alternative treatment for human infections. A comprehensive review. Int. J. Antimicrob. Agents 53, 16–21. doi: 10.1016/j.ijantimicag.2018.09.004
Kamal, F., and Dennis, J. J. (2015). Burkholderia cepacia complex phage-antibiotic synergy (PAS): antibiotics stimulate lytic phage activity. Appl. Environ. Microbiol. 81, 1132–1138. doi: 10.1128/AEM.02850-14
Kebriaei, R., Lev, K., Morrisette, T., Stamper, K. C., Abdul-Mutakabbir, J. C., Lehman, S. M., et al. (2020). Bacteriophage-antibiotic combination strategy: an alternative against methicillin-resistant phenotypes of Staphylococcus aureus. Antimicrob. Agents Chemother. 64, e00461–e00420. doi: 10.1128/AAC.00461-20
Khan, A., Rao, T. S., and Joshi, H. M. (2022). Phage therapy in the Covid-19 era: advantages over antibiotics. Curr. Res. Microb. Sci. 3:100115. doi: 10.1016/j.crmicr.2022.100115
Khawaja, K. A., Rauf, M., Abbas, Z., and Rehman, S. U. (2016). A virulent phage JHP against Pseudomonas aeruginosa showed infectivity against multiple genera. J. Basic Microbiol. 56, 1090–1097. doi: 10.1002/jobm.201500764
Khurshid, M., Rasool, M. H., Ashfaq, U. A., Aslam, B., and Waseem, M. (2017). Emergence of ISAba1 harboring carbapenem-resistant Acinetobacter baumannii isolates in Pakistan. Future Microbiol. 12, 1261–1269. doi: 10.2217/fmb-2017-0080
Khurshid, M., Rasool, M. H., Ashfaq, U. A., Aslam, B., Waseem, M., Ali, M. A., et al. (2020). Acinetobacter baumannii sequence types harboring genes encoding aminoglycoside modifying enzymes and 16SrRNA Methylase; a multicenter study from Pakistan. Infect. Drug Resist. 13, 2855–2862. doi: 10.2147/IDR.S260643
Kim, P., Sanchez, A., and Kime, J. (2021). Ousterout D. 1083. Phase 1b results of pharmacokinetics, pharmacodynamics, and safety for LBP-EC01, a CRISPR-Cas 3 enhanced bacteriophage cocktail targeting Escherichia coli that cause urinary tract infections. Open Forum Infect. Dis. 8:S633. doi: 10.1093/ofid/ofab466.1277
Knezevic, P., Curcin, S., Aleksic, V., Petrusic, M., and Vlaski, L. (2013). Phage-antibiotic synergism: a possible approach to combatting Pseudomonas aeruginosa. Res. Microbiol. 164, 55–60. doi: 10.1016/j.resmic.2012.08.008
Koderi Valappil, S., Shetty, P., Deim, Z., Terhes, G., Urbán, E., Váczi, S., et al. (2021). Survival comes at a cost: a coevolution of phage and its host leads to phage resistance and antibiotic sensitivity of Pseudomonas aeruginosa multidrug resistant strains. Front. Microbiol. 12. doi: 10.3389/fmicb.2021.783722
Koff, J. CYstic fibrosis bacterio PHage study at Yale (CYPHY): A single-site, randomized, double-blind, placebo-controlled study of bacteriophage therapy YPT-01 for Pseudomonas Aeruginosa infections in adults with cystic fibrosis. clinicaltrials.gov; (2023).
Korehei, R., and Kadla, J. F. (2014). Encapsulation of T4 bacteriophage in electrospun poly (ethylene oxide)/cellulose diacetate fibers. Carbohydr. Polym. 100, 150–157. doi: 10.1016/j.carbpol.2013.03.079
Kortright, K. E., Chan, B. K., Koff, J. L., and Turner, P. E. (2019). Phage therapy: a renewed approach to combat antibiotic-resistant Bacteria. Cell Host Microbe 25, 219–232. doi: 10.1016/j.chom.2019.01.014
Kuerban, K., Gao, X., Zhang, H., Liu, J., Dong, M., Wu, L., et al. (2020). Doxorubicin-loaded bacterial outer-membrane vesicles exert enhanced anti-tumor efficacy in non-small-cell lung cancer. Acta Pharm. Sin. B 10, 1534–1548. doi: 10.1016/j.apsb.2020.02.002
Kutateladze, M., and Adamia, R. (2008). Phage therapy experience at the Eliava institute. Med. Mal. Infect. 38, 426–430. doi: 10.1016/j.medmal.2008.06.023
Kvachadze, L., Balarjishvili, N., Meskhi, T., Tevdoradze, E., Skhirtladze, N., Pataridze, T., et al. (2011). Evaluation of lytic activity of staphylococcal bacteriophage Sb-1 against freshly isolated clinical pathogens. Microb. Biotechnol. 4, 643–650. doi: 10.1111/j.1751-7915.2011.00259.x
Lehman, S. M., Mearns, G., Rankin, D., Cole, R. A., Smrekar, F., Branston, S. D., et al. (2019). Design and preclinical development of a phage product for the treatment of antibiotic-resistant Staphylococcus aureus infections. Viruses 11:88. doi: 10.3390/v11010088
Leitner, L., Sybesma, W., Chanishvili, N., Goderdzishvili, M., Chkhotua, A., Ujmajuridze, A., et al. (2017). Bacteriophages for treating urinary tract infections in patients undergoing transurethral resection of the prostate: a randomized, placebo-controlled, double-blind clinical trial. BMC Urol. 17:90. doi: 10.1186/s12894-017-0283-6
Leitner, L., Ujmajuridze, A., Chanishvili, N., Goderdzishvili, M., Chkonia, I., Rigvava, S., et al. (2021). Intravesical bacteriophages for treating urinary tract infections in patients undergoing transurethral resection of the prostate: a randomised, placebo-controlled, double-blind clinical trial. Lancet Infect. Dis. 21, 427–436. doi: 10.1016/S1473-3099(20)30330-3
Li, H. X., Yang, X. J., Zhu, X. Y., Gao, L., Rao, S. Q., Yuan, L., et al. (2021). Isolation and characterization of broad host-range of bacteriophages infecting Cronobacter sakazakii and its biocontrol potential in dairy products. Qual. Assur. Saf. Crop. Foods 13, 21–44.
Lim, W. S., Phang, K. K. S., Tan, A. H.-M., Li, S. F.-Y., and Ow, D. S.-W. (2016). Small Colony variants and single nucleotide variations in Pf1 region of PB1 phage-resistant Pseudomonas aeruginosa. Front. Microbiol. 7:282. doi: 10.3389/fmicb.2016.00282
Lin, D. M., Koskella, B., and Lin, H. C. (2017). Phage therapy: an alternative to antibiotics in the age of multi-drug resistance. World J. Gastrointest. Pharmacol. Ther. 8, 162–173. doi: 10.4292/wjgpt.v8.i3.162
Ling, H., Lou, X., Luo, Q., He, Z., Sun, M., and Sun, J. (2022). Recent advances in bacteriophage-based therapeutics: insight into the post-antibiotic era. Acta Pharm. Sin. B 12, 4348–4364. doi: 10.1016/j.apsb.2022.05.007
Loh, B., Gondil, V. S., Manohar, P., Khan, F. M., Yang, H., and Leptihn, S. (2021). Encapsulation and delivery of therapeutic phages. Appl. Environ. Microbiol. 87, e01979–e01920. doi: 10.1128/AEM.01979-20
Łusiak-Szelachowska, M., Międzybrodzki, R., Drulis-Kawa, Z., Cater, K., Knežević, P., Winogradow, C., et al. (2022). Bacteriophages and antibiotic interactions in clinical practice: what we have learned so far. J. Biomed. Sci. 29:23. doi: 10.1186/s12929-022-00806-1
Ma, Y.-H., Islam, G. S., Wu, Y., Sabour, P. M., Chambers, J. R., Wang, Q., et al. (2016). Temporal distribution of encapsulated bacteriophages during passage through the chick gastrointestinal tract. Poult. Sci. 95, 2911–2920. doi: 10.3382/ps/pew260
Maciejewska, B., Olszak, T., and Drulis-Kawa, Z. (2018). Applications of bacteriophages versus phage enzymes to combat and cure bacterial infections: an ambitious and also a realistic application? Appl. Microbiol. Biotechnol. 102, 2563–2581. doi: 10.1007/s00253-018-8811-1
Malik, S., Nehra, K., and Rana, J. S. (2021). Bacteriophage cocktail and phage antibiotic synergism as promising alternatives to conventional antibiotics for the control of multi-drug-resistant uropathogenic Escherichia coli. Virus Res. 302:198496. doi: 10.1016/j.virusres.2021.198496
Manohar, P., Madurantakam Royam, M., Loh, B., Bozdogan, B., Nachimuthu, R., and Leptihn, S. (2022). Synergistic effects of phage–antibiotic combinations against Citrobacter amalonaticus. ACS Infect. Dis. 8, 59–65. doi: 10.1021/acsinfecdis.1c00117
Manyi-Loh, C., Mamphweli, S., Meyer, E., and Okoh, A. (2018). Antibiotic use in agriculture and its consequential resistance in environmental sources: potential public health implications. Molecules 23:795. doi: 10.3390/molecules23040795
Mardiana, M., Teh, S.-H., Lin, L.-C., and Lin, N.-T. (2022). Isolation and characterization of a novel Siphoviridae phage, vB_AbaS_TCUP2199, infecting multidrug-resistant Acinetobacter baumannii. Viruses 14:1240. doi: 10.3390/v14061240
Monteiro, R., Pires, D. P., Costa, A. R., and Azeredo, J. (2019). Phage therapy: going temperate? Trends Microbiol. 27, 368–378. doi: 10.1016/j.tim.2018.10.008
Morozova, V. V., Vlassov, V. V., and Tikunova, N. V. (2018). Applications of bacteriophages in the treatment of localized infections in humans. Front. Microbiol. 9:1696. doi: 10.3389/fmicb.2018.01696
Morrisette, T., Lev, K. L., Canfield, G. S., Duerkop, B. A., Kebriaei, R., Stamper, K. C., et al. (2022). Evaluation of bacteriophage cocktails alone and in combination with Daptomycin against Daptomycin-nonsusceptible Enterococcus faecium. Antimicrob. Agents Chemother. 66:e01623-21. doi: 10.1128/AAC.01623-21
Munita, J. M., and Arias, C. A. (2016). Mechanisms of antibiotic resistance. Virulence mechanisms of bacterial pathogens : John Wiley & Sons, Ltd, 481–511.
Nale, J. Y., Chutia, M., Carr, P., Hickenbotham, P. T., and Clokie, M. R. J. (2016). ‘Get in early’; biofilm and wax moth (galleria mellonella) models reveal new insights into the therapeutic potential of Clostridium difficile bacteriophages. Front. Microbiol. 7:1383. doi: 10.3389/fmicb.2016.01383
Nasr Azadani, D., Zhang, D., Hatherill, J. R., Silva, D., and Turner, J. W. (2020). Isolation, characterization, and comparative genomic analysis of a phage infecting high-level aminoglycoside-resistant (HLAR) Enterococcus faecalis. Peer J 8:e9171. doi: 10.7717/peerj.9171
Nieth, A., Verseux, C., Barnert, S., Süss, R., and Römer, W. (2015). A first step toward liposome-mediated intracellular bacteriophage therapy. Expert Opin. Drug Deliv. 12, 1411–1424. doi: 10.1517/17425247.2015.1043125
Oechslin, F., Piccardi, P., Mancini, S., Gabard, J., Moreillon, P., Entenza, J. M., et al. (2017). Synergistic interaction between phage therapy and antibiotics clears Pseudomonas Aeruginosa infection in endocarditis and reduces virulence. J. Infect. Dis. 215, 703–712. doi: 10.1093/infdis/jiw632
Opperman, C. J., Wojno, J. M., and Brink, A. J. (2022). Treating bacterial infections with bacteriophages in the 21st century. S. Afr. J. Infect. Dis. 37:346. doi: 10.4102/sajid.v37i1.346
Osman, A.-H., Kotey, F. C. N., Odoom, A., Darkwah, S., Yeboah, R. K., Dayie, N. T. K. D., et al. (2023). The potential of bacteriophage-antibiotic combination therapy in treating infections with multidrug-resistant bacteria. Antibiotics 12:1329. doi: 10.3390/antibiotics12081329
Otero, J., García-Rodríguez, A., Cano-Sarabia, M., Maspoch, D., Marcos, R., Cortés, P., et al. (2019). Biodistribution of liposome-encapsulated bacteriophages and their transcytosis during oral phage therapy. Front. Microbiol. 10:10. doi: 10.3389/fmicb.2019.00689
Patangia, D. V., Anthony Ryan, C., Dempsey, E., Paul Ross, R., and Stanton, C. (2022). Impact of antibiotics on the human microbiome and consequences for host health. Microbiol. Open 11:e1260. doi: 10.1002/mbo3.1260
Perron, G. G., Whyte, L., Turnbaugh, P. J., Goordial, J., Hanage, W. P., Dantas, G., et al. (2015). Functional characterization of bacteria isolated from ancient Arctic soil exposes diverse resistance mechanisms to modern antibiotics. PLoS One 10:e0069533. doi: 10.1371/journal.pone.0069533
Phothichaisri, W., Ounjai, P., Phetruen, T., Janvilisri, T., Khunrae, P., Singhakaew, S., et al. (2018). Characterization of bacteriophages infecting clinical isolates of Clostridium difficile. Front. Microbiol. 9:1701. doi: 10.3389/fmicb.2018.01701
Price, L. B., Newland, J., Bole, A., Bortolaia, V., Larsen, J., Loneragan, G. H., et al. (2017). Combating antibiotic resistance – a policy roadmap to reduce use of medically important antibiotics in livestock. Washington, D.C: George Washington University.
Principi, N., Silvestri, E., and Esposito, S. (2019). Advantages and limitations of bacteriophages for the treatment of bacterial infections. Front. Pharmacol. 10:457104. doi: 10.3389/fphar.2019.00513
Rahimi-Midani, A., Lee, S.-W., and Choi, T.-J. (2021). Potential solutions using bacteriophages against antimicrobial resistant Bacteria. Antibiotics (Basel) 10:1496. doi: 10.3390/antibiotics10121496
Rai, S., and Kumar, A. (2022). Bacteriophage therapeutics to confront multidrug-resistant Acinetobacter baumannii – a global health menace. Environ. Microbiol. Rep. 14, 347–364. doi: 10.1111/1758-2229.12988
Roach, D. R., and Donovan, D. M. (2015). Antimicrobial bacteriophage-derived proteins and therapeutic applications. Bacteriophage 5:e1062590. doi: 10.1080/21597081.2015.1062590
Roach, D. R., Leung, C. Y., Henry, M., Morello, E., Singh, D., Di Santo, J. P., et al. (2017). Synergy between the host immune system and bacteriophage is essential for successful phage therapy against an acute respiratory pathogen. Cell Host Microbe 22, 38–47.e4. doi: 10.1016/j.chom.2017.06.018
Ryan, E. M., Alkawareek, M. Y., Donnelly, R. F., and Gilmore, B. F. (2012). Synergistic phage-antibiotic combinations for the control of Escherichia coli biofilms in vitro. FEMS Immunol. Med. Microbiol. 65, 395–398. doi: 10.1111/j.1574-695X.2012.00977.x
Sarker, S. A., Berger, B., Deng, Y., Kieser, S., Foata, F., Moine, D., et al. (2017). Oral application of Escherichia coli bacteriophage: safety tests in healthy and diarrheal children from Bangladesh. Environ. Microbiol. 19, 237–250. doi: 10.1111/1462-2920.13574
Sarker, S. A., Sultana, S., Reuteler, G., Moine, D., Descombes, P., Charton, F., et al. (2016). Oral phage therapy of acute bacterial diarrhea with two Coliphage preparations: a randomized trial in children from Bangladesh. EBioMedicine 4, 124–137. doi: 10.1016/j.ebiom.2015.12.023
Schmelcher, M., Donovan, D. M., and Loessner, M. J. (2012). Bacteriophage endolysins as novel antimicrobials. Future Microbiol. 7, 1147–1171. doi: 10.2217/fmb.12.97
Schmelcher, M., and Loessner, M. J. (2021). Bacteriophage endolysins — extending their application to tissues and the bloodstream. Curr. Opin. Biotechnol. 68, 51–59. doi: 10.1016/j.copbio.2020.09.012
Semler, D. D., Goudie, A. D., Finlay, W. H., and Dennis, J. J. (2014). Aerosol phage therapy efficacy in Burkholderia cepacia complex respiratory infections. Antimicrob. Agents Chemother. 58, 4005–4013. doi: 10.1128/AAC.02388-13
Sharma, U., Vipra, A., and Channabasappa, S. (2018). Phage-derived lysins as potential agents for eradicating biofilms and persisters. Drug Discov. Today 23, 848–856. doi: 10.1016/j.drudis.2018.01.026
Silva Batalha, L., Pardini Gontijo, M. T., Novaes, V., de Carvalho Teixeira, A., Meireles Gouvêa Boggione, D., Soto Lopez, M. E., et al. (2021). Santos Mendonça RC encapsulation in alginate-polymers improves stability and allows controlled release of the UFV-AREG1 bacteriophage. Food Res. Int. 139, 109947–101016. doi: 10.1016/j.foodres.2020.109947
Simon, K., Pier, W., Krüttgen, A., and Horz, H.-P. (2021). Synergy between phage Sb-1 and Oxacillin against methicillin-resistant Staphylococcus aureus. Antibiotics 10:849. doi: 10.3390/antibiotics10070849
Singla, S., Harjai, K., Raza, K., Wadhwa, S., Katare, O. P., and Chhibber, S. (2016). Phospholipid vesicles encapsulated bacteriophage: a novel approach to enhance phage biodistribution. J. Virol. Methods 236, 68–76. doi: 10.1016/j.jviromet.2016.07.002
Skurnik, M. (2022). Can bacteriophages replace antibiotics? Antibiotics 11:575. doi: 10.3390/antibiotics11050575
SNIPR Biome aps. A phase 1, randomized, double-blind, first-in-human, dose escalation study investigating the safety, recovery, and pharmacodynamics of multiple Oral administrations of SNIPR001 in healthy subjects. clinicaltrials.gov; (2023).
Sun, Q., Shen, L., Zhang, B.-L., Yu, J., Wei, F., Sun, Y., et al. (2023). Advance on engineering of bacteriophages by synthetic biology. Infect. Drug Resist. 16, 1941–1953. doi: 10.2147/IDR.S402962
Taati Moghadam, M., Amirmozafari, N., Shariati, A., Hallajzadeh, M., Mirkalantari, S., Khoshbayan, A., et al. (2020). How phages overcome the challenges of drug resistant bacteria in clinical infections. Infect. Drug Resist. 13, 45–61. doi: 10.2147/IDR.S234353
Tamma, P. D., and Suh, G. A. (2021). Phage are all the rage: bacteriophage in clinical practice. J. Pediatric Infect. Dis. Soc. 10, 749–753. doi: 10.1093/jpids/piab012
Tan, C. S. (2020). Could bacteriophages isolated from the sewage be the solution to methicillin-resistant Staphylococcus aureus? Med. J. Malaysia 75, 110–116.
Technophage, SA. A double-blind and randomized study to determine the safety and tolerability of multiple doses of TP-102 in subjects with non-infected and infected diabetic foot ulcers. clinicaltrials.gov; (2022).
Terwilliger, A., Clark, J., Karris, M., Hernandez-Santos, H., Green, S., Aslam, S., et al. (2021). Phage therapy related microbial succession associated with successful clinical outcome for a recurrent urinary tract infection. Viruses 13:2049. doi: 10.3390/v13102049
Tian, F., Li, J., Nazir, A., and Tong, Y. (2021). Bacteriophage – a promising alternative measure for bacterial biofilm control. Infect. Drug Resist. 14, 205–217. doi: 10.2147/IDR.S290093
Torres-Barceló, C., and Hochberg, M. E. (2016). Evolutionary rationale for phages as complements of antibiotics. Trends Microbiol. 24, 249–256. doi: 10.1016/j.tim.2015.12.011
University Hospital, Montpellier. Bacteriophages effects on Pseudomonas Aeruginosa presents in sputum of cystic fibrosis (CF) patients. clinicaltrials.gov; (2013).
Van Belleghem, J. D., Dąbrowska, K., Vaneechoutte, M., Barr, J. J., and Bollyky, P. L. (2019). Interactions between bacteriophage, Bacteria, and the mammalian immune system. Viruses 11:10. doi: 10.3390/v11010010
Wang, J., Hu, B., Xu, M., Yan, Q., Liu, S., Zhu, X., et al. (2006). Therapeutic effectiveness of bacteriophages in the rescue of mice with extended spectrum β-lactamase-producing Escherichia coli bacteremia. Int. J. Mol. Med. 17, 347–355. doi: 10.3892/ijmm.17.2.347
Wang, X., and Leptihn, S. (2024). Defense and anti-defense mechanisms of bacteria and bacteriophages. J Zhejiang Univ Sci B 25, 181–196. doi: 10.1631/jzus.B2300101
Watanabe, R., Matsumoto, T., Sano, G., Ishii, Y., Tateda, K., Sumiyama, Y., et al. (2007). Efficacy of bacteriophage therapy against gut-derived Sepsis caused by Pseudomonas aeruginosa in mice. Antimicrob. Agents Chemother. 51, 446–452. doi: 10.1128/AAC.00635-06
Waters, E. M., Neill, D. R., Kaman, B., Sahota, J. S., Clokie, M. R. J., Winstanley, C., et al. (2017). Phage therapy is highly effective against chronic lung infections with Pseudomonas aeruginosa. Thorax 72, 666–667. doi: 10.1136/thoraxjnl-2016-209265
Wei, J., Peng, N., Liang, Y., Li, K., and Li, Y. (2020). Phage therapy: consider the past, embrace the future. Appl. Sci. 10:7654. doi: 10.3390/app10217654
Wills, Q. F., Kerrigan, C., and Soothill, J. S. (2005). Experimental bacteriophage protection against Staphylococcus aureus abscesses in a rabbit model. Antimicrob. Agents Chemother. 49, 1220–1221. doi: 10.1128/AAC.49.3.1220-1221.2005
Wintachai, P., Naknaen, A., Thammaphet, J., Pomwised, R., Phaonakrop, N., Roytrakul, S., et al. (2020). Characterization of extended-spectrum-β-lactamase producing Klebsiella pneumoniae phage KP1801 and evaluation of therapeutic efficacy in vitro and in vivo. Sci. Rep. 10:11803. doi: 10.1038/s41598-020-68702-y
Wolcott, R. A prospective, randomized double-blind controlled study of WPP-201 for the safety and efficacy of treatment of venous leg ulcers. clinicaltrials.gov; (2011).
Wright, A., Hawkins, C. H., Anggård, E. E., and Harper, D. R. (2009). A controlled clinical trial of a therapeutic bacteriophage preparation in chronic otitis due to antibiotic-resistant Pseudomonas aeruginosa; a preliminary report of efficacy. Clin. Otolaryngol. 34, 349–357. doi: 10.1111/j.1749-4486.2009.01973.x
Xu, C., Kong, L., Gao, H., Cheng, X., and Wang, X. (2022). A review of current bacterial resistance to antibiotics in food animals. Front. Microbiol. 13:822689. doi: 10.3389/fmicb.2022.822689
Yelin, I., and Kishony, R. (2018). Antibiotic Resistance. Cell 172, 1136–1136.e1. doi: 10.1016/j.cell.2018.02.018
Yen, M., Cairns, L. S., and Camilli, A. (2017). A cocktail of three virulent bacteriophages prevents Vibrio cholerae infection in animal models. Nat. Commun. 8:14187. doi: 10.1038/ncomms14187
Zalewska-Piątek, B. (2023). Phage therapy—challenges, opportunities and future prospects. Pharmaceuticals (Basel) 16:1638. doi: 10.3390/ph16121638
Keywords: antibiotic resistance, bacteriophage-based therapeutics, synergistic effect, preclinical and clinical studies, formulation strategies, advantages over antibiotics
Citation: Subramanian A (2024) Emerging roles of bacteriophage-based therapeutics in combating antibiotic resistance. Front. Microbiol. 15:1384164. doi: 10.3389/fmicb.2024.1384164
Received: 08 February 2024; Accepted: 06 June 2024;
Published: 05 July 2024.
Edited by:
Kenneth K. S. Ng, University of Windsor, CanadaReviewed by:
Birbal Singh, Indian Veterinary Research Institute (IVRI), IndiaCopyright © 2024 Subramanian. This is an open-access article distributed under the terms of the Creative Commons Attribution License (CC BY). The use, distribution or reproduction in other forums is permitted, provided the original author(s) and the copyright owner(s) are credited and that the original publication in this journal is cited, in accordance with accepted academic practice. No use, distribution or reproduction is permitted which does not comply with these terms.
*Correspondence: Anandhalakshmi Subramanian, YW5hbmRoYWxha3NodUBnbWFpbC5jb20=
†ORCID: Anandhalakshmi Subramanian, orcid.org/0000-0001-5543-5130
Disclaimer: All claims expressed in this article are solely those of the authors and do not necessarily represent those of their affiliated organizations, or those of the publisher, the editors and the reviewers. Any product that may be evaluated in this article or claim that may be made by its manufacturer is not guaranteed or endorsed by the publisher.
Research integrity at Frontiers
Learn more about the work of our research integrity team to safeguard the quality of each article we publish.