- 1Laboratory of Environmental Microbiology, Institute of Microbiology of the Czech Academy of Sciences, Prague, Czechia
- 2Institute of Geology of the Czech Academy of Sciences, Prague, Czechia
- 3Nuclear Physiscs Institute of the Czech Academy of Sciences, Husinec-Řež, Czechia
- 4Mycology and Microbiology Center, University of Tartu, Tartu, Estonia
- 5Natural Resources Institute Finland, Helsinki, Finland
- 6Department of Soil and Water Conservation Centre for Applied Soil Science and Biology of the Segura of the Spanish National Research Council, Campus Universitario de Espinardo, Murcia, Spain
Fungi are an integral part of the nitrogen and phosphorus cycling in trophic networks, as they participate in biomass decomposition and facilitate plant nutrition through root symbioses. Nutrient content varies considerably between the main fungal habitats, such as soil, plant litter or decomposing dead wood, but there are also large differences within habitats. While some soils are heavily loaded with N, others are limited by N or P. One way in which nutrient availability can be reflected in fungi is their content in biomass. In this study, we determined the C, N, and P content (in dry mass) of fruiting bodies of 214 fungal species to inspect how phylogeny and membership in ecological guilds (soil saprotrophs, wood saprotrophs, and ectomycorrhizal fungi) affect the nutrient content of fungal biomass. The C content of fruiting bodies (415 ± 25 mg g–1) showed little variation (324–494 mg g–1), while the range of N (46 ± 20 mg g–1) and P (5.5 ± 3.0 mg g–1) contents was within one order of magnitude (8–103 mg g–1 and 1.0–18.9 mg g–1, respectively). Importantly, the N and P contents were significantly higher in the biomass of soil saprotrophic fungi compared to wood saprotrophic and ectomycorrhizal fungi. While the average C/N ratio in fungal biomass was 11.2, values exceeding 40 were recorded for some fungi living on dead wood, typically characterized by low N content. The N and P content of fungal mycelium also showed a significant phylogenetic signal, with differences in nutrient content being relatively low within species and genera of fungi. A strong correlation was found between N and P content in fungal biomass, while the correlation of N content and the N-containing fungal cell wall biopolymer—chitin showed only weak significance. The content of macronutrients in fungal biomass is influenced by the fungal life style and nutrient availability and is also limited by phylogeny.
1 Introduction
Fungi are important decomposers of organic matter in terrestrial trophic networks (Lindahl et al., 2002; Bai et al., 2021). They inhabit environments where sufficient carbon (C) is usually available, but this is not the case for the main macronutrients, nitrogen (N) and phosphorus (P), which are often limiting. While in agricultural soils N and P deficiency is often overcome by fertilization, in most natural ecosystems available N or P sources are limited (Du et al., 2020). Both C and N are mainly present in soil organic matter, with N also coming from wet or dry atmospheric deposition (van Breemen and van Dijk, 1988), often enhanced by human activities such as combustion processes; P is also mobilized in soil by mineral weathering (Larsen, 1967). The access of fungi to N and P in the environment varies depending on their lifestyle (Root, 1967; Põlme et al., 2020), reflecting the C resources used by fungi. The most important ecological guilds of fungi (Põlme et al., 2020) in forest trophic networks are (i) wood-decomposing saprotrophs/parasites that accumulate C from decaying wood, (ii) soil and litter saprotrophs that acquire C through litter decomposition, and (iii) mycorrhizal fungi that acquire C from their plant hosts.
Of the substrates used by fungi, wood from living or dead trees contains the least N and P (Watkinson et al., 2006), whereas soil, and especially topsoil, is richer in these nutrients (Mooshammer et al., 2014). In a typical forest, N or P content may limit fungal activity in dead wood even when content of nutrients in soil is high (Piché-Choquette et al., 2023). Thus, wood saprotrophs (WS) must cope with nutrient limitation in their habitat. Soil saprotrophic (SS) and ectomycorrhizal (EcM) fungi utilize soil N and P, ectomycorrhizal fungi utilize C provided by their host trees in exchange for N (Rayner and Boddy, 1989; Lindahl et al., 2007; Smith and Read, 2008), and therefore their C acquisition costs are reduced. In principle, the benefits of EcM symbiosis appear to be highest in N-limited soils (Johnson, 2010), because the provision of N by fungi to plants leads to higher primary production, thereby increasing C availability to fungi. The ratio between N and P also appears to be very important for mycorrhizal fungi, as higher N supply promotes photosynthetic activity of host plants, which in turn have a higher capacity to exchange C for P (Johnson, 2010).
Increased N availability associated with human activities has been shown to increase the ratio of bacterial and fungal biomass in soil and inhibit microbial respiration and abundance (Ramirez et al., 2012; Zhou et al., 2017). Soil fungal communities are also affected by increased N availability due to anthropogenic deposition (Baldrian et al., 2023), including changes in abundance and balance between nutrient guilds and species within guilds (van der Linde et al., 2018; Baldrian et al., 2022).
Due to both natural variation and anthropogenic deposition, the total amounts of C, N, and P, as well as their ratios, vary substantially among soils (Cleveland and Liptzin, 2007). The amounts and ratios of these elements in fungal biomass also appear to vary among species, both under laboratory conditions and in nature (Brabcová et al., 2018; Camenzind et al., 2021). The analysis of nutrient content in fungal biomass under real conditions is methodically challenging, as it is virtually impossible to separate the hyphae of individual fungal species from their habitat matrix—plant litter, soil, or wood. However, assuming that nutrient content may be reflected in the biomass of fungi growing under laboratory conditions (Camenzind et al., 2021), it is also evident in the biomass that forms the fungal fruiting bodies (Vogt et al., 1981).
For ectomycorrhizal fungi, it has been suggested that the C:N ratio in their biomass remains relatively constant (Mooshammer et al., 2014), as a result of providing a “surplus” of N to their tree hosts (Kranabetter et al., 2019). In saprotrophic fungi, the storage of P and C is expected in their biomass (Quinché, 1997; Kalač, 2019), while any excess nitrogen, if present, is likely excreted (Mooshammer et al., 2014). Although nutrient content can vary even between different parts of the same individual (Trocha et al., 2016), it can be assumed that differences between ecological guilds should be much more pronounced.
Occasionally, higher concentrations of N and therefore lower C:N ratios in fruiting bodies have been observed in saprotrophic fungi compared to EcM fungi (Vogt et al., 1981; Zhang and Elser, 2017). This may indicate the lower costs of C in EcM fungi. However, it is well known that fungal species exhibit a preference for environments with specific (high or low) N availability (van der Linde et al., 2018). It should be noted that the nutrient content in fungal biomass is not only a response variable to species traits and environmental conditions; it also influences the fate of microbial biomass in the ecosystem. The rate of decomposition of fungal mycelia in forest soils has been reported to increase with the N content (Brabcová et al., 2018), and it is evident that N is a primary target for guilds of microorganisms responsible for decomposing fungal biomass (López-Mondéjar et al., 2020; Starke et al., 2020). While N is present in many biomolecules, including proteins and nucleic acids (Marzluf, 1996), it also constitutes a significant component of the fungal cell wall polysaccharide chitin (Lenardon et al., 2010), whose content in fungal cell walls varies (Blumenthal and Saul, 1957) and under starvation conditions this compound may undergo enzymatic self-degradation (White et al., 2002; Gruber and Seidl-Seiboth, 2012). However, the chitin content in biomass may to some extent determine the N requirement of each fungal species and potentially correlate with the N content in their biomass.
Substantial differences in the accessibility of C, N, and P by various ecological guilds of fungi raise the question of whether their amounts and ratios are conserved in fungal groups that obtain these nutrients from soil, plant litter, deadwood, or the roots of their host plants. In these environments, the relative content of C, N, and P can vary over more than an order of magnitude (Piché-Choquette et al., 2023). The composition of fungal biomass may also be conserved phylogenetically, at least over short phylogenetic distances (Mouginot et al., 2014), reflecting traits such as the proportion of protein or chitin content in their cell walls. Alternatively, the N and P content in fungal biomass may vary within each species, simply reflecting the accessibility of N and P in each individual site where the fungus is found.
We anticipate that the ecological traits of fungi, particularly those related to the mode and source of C and nutrient acquisition, are the primary drivers of the nutrient content in their biomass. This is because the differences in nutrient limitation between habitats are too significant to be overcome. Some level of phylogenetic conservation is also likely due to the evolutionary preservation of biochemical traits and the similarity in the ecology of closely related taxa. We analyzed the fruiting bodies of fungi from temperate forests to determine their C, N, and P contents, aiming to assess the contributions of ecology and phylogeny to biomass composition. We assume that N and P contents are higher in soil saprotrophic fungi than in wood-decomposing and ectomycorrhizal fungi. To je způsobeno nízkou koncentrací živin ve dřevě v případě dřevních saprotrofů a výměnou živin za uhlík s hostitelskou rostlinou v případě mykorhizních hub. Additionally, we expect a positive correlation between chitin content and N content in fungal biomass. Furthermore, our study was intended to provide data on the stoichiometric ratios of main macronutrients in the biomass of macrofungi, offering reliable information on this biogeochemically important feature.
2 Materials and methods
2.1 Samples
Fresh fungal fruiting bodies were collected in the Czech Republic, Germany, and Estonia between the years 2014 and 2020. Where possible, fruiting bodies were transferred to liquid nitrogen after collection and kept frozen or immediately transferred to the laboratory and stored at −18 to −20°C. Subsequently, whole fruiting bodies were lyophilized and homogenized using a mortar and pestle or a rotary mill to obtain a fine powder, followed by freeze-drying and storage at −18°C until further processing. In total, 282 samples of fungal fruiting bodies were included in the analysis (Supplementary Table 1).
2.2 Identification of fungal samples and phylogenetic analysis
Fungi were preliminarily identified during collection based on morphology of fungal fruiting bodies. This preliminary identification was later confirmed or corrected based on the analysis of DNA from the collected specimens. DNA extraction from 250 mg of freeze-dried powdered fruiting body biomass was performed using the DNeasy Qiagen PlantMini kit (Qiagen) in duplicate per specimen. For species identification, the primer combination ITS0F/ITS4 was used to amplify the regions of ITS1, 5.8S, and ITS2 of the rDNA; for phylogeny construction, the primer combination LR0R/LR7, amplifying a part of 28S rDNA gene encoding ribosomal RNA (28S rDNA), was used (Vilgalys and Hester, 1990; White et al., 1990; Hibbett and Vilgalys, 1993; Schoch et al., 2012; Schneider et al., 2015). The PCR mix contained 200 μM dNTPs (Bioline), 0.5 μM of both LR0R and LR7 primers, or 0.4 μM of both ITS0F and ITS4 primers, 0.02U/μl of Q5 High-Fidelity DNA polymerase (New England Biolabs), 5 ng/μl of DNA in 1 × Q5 Reaction Buffer enriched by 1 × Q5 HighGC Enhancer, and 9.03 μM BSA (GeneOn). The reaction parameters were identical for both DNA regions except for the annealing temperatures. The cycling conditions for ITS were as follows: 98°C for 30 s; 30 cycles of 98° for 10 s, annealing for 50°C for 30 s, 72°C for 30 s; then 72°C for 2 min, while for 28S rDNA these conditions were: 95°C for 5 min.; 35 cycles of 95°C for 1 min, 52°C for 1 min 72°C for 1 min; then 72°C for 10 min. The PCR product was sequenced using Sanger sequencing in an external facility (SeqMe, Czech Republic).
All DNA sequences were checked for accuracy and manually edited if necessary. They were deposited in GenBank under the accession numbers OR602209-OR602444, OR625684-OR625708, PP102200 and PP102454-PP102678 (Supplementary Table 1). For precise species determination, the sequences of the ITS rDNA regions were compared with the NCBI database using the BLASTn algorithm. For the construction of a phylogenetic tree, the 28S sequences were aligned using the Clustal-W algorithm in BioEdit 7.2.5 (Hall, 1999). Phylogenetic trees for the entire dataset and for partial alignments grouping taxonomic or functional groups were constructed using MrBayes 3.2.7a (Ronquist et al., 2012) with the standard setup of the GTR evolutionary model. The DNA data models were chosen based on AIC using jModeltest2 (Darriba et al., 2012). The number of MCMC generations was set individually for particular datasets between 5 × 105 and 5.5 × 106 to reach an effective number of independent draws from their posterior distribution higher than 100, and the Potential Scale Reduction Factor (comparing the estimated between-MCMC chain variance with the within-chain variance) close to 1.0.
Fungal species were assigned to taxa at different taxonomic levels and categorized into ecological guilds based on their primary lifestyles, as described in Põlme et al. (2020). We distinguished wood saprotrophs (WS, including plant pathogens of living trees), soil saprotrophs (SS, encompassing both soil and litter saprotrophs), and ectomycorrhizal species (EcM).
2.3 Analysis of chemical composition
The N and C content in freeze-dried powdered fruiting bodies were determined at the Institute of Botany of the Czech Academy of Sciences using the automatic element composition analyzer Flash 2000 (Thermo Scientific, USA). Samples (particle size < 0.1 mm, heaped 10–30 mg) were introduced into the burning tube and combusted at 1,000°C in a stream of pure O2. Moisture was removed on a separating column, and the content of separated oxides was determined by the conductivity detector. The signal was analyzed using the Eager Xperience software (Thermo Scientific) (Nelson and Sommers, 1996).
The determination of P content in fruiting bodies was conducted by burning 50 mg of the sample in a porcelain crucible for 6 h at 550°C. Subsequently, 1 mL of HNO3 was added and heated to a boil. The samples were then transferred to a volumetric flask and replenished with distilled H2O to a total volume of 50 mL. The concentration of P was measured colorimetrically (Ohno and Zibilske, 1991). Due to the limited fungal biomass in certain samples resulting from the small size of fruiting bodies, this analysis was conducted on only a subset of the total sample set.
For selected samples representing various ecological groups of fungi, chitin content was determined using a method based on acid hydrolysis of chitin, producing glucosamine. The concentration of glucosamine was measured after derivatization with FMOC-Cl (9-fluorenylmethyl-chloroformate). The measurement was conducted via HPLC (Arc HPLC, Waters, USA) with Hewlett Packard ODS Hypersil (5 μm, 250 × 4.6 mm) column and detected with a fluorescence detector (Adamczyk et al., 2020).
2.4 Statistical analysis
The statistical analyses were conducted in the R environment (R Core Team, 2022). Descriptive statistics (mean, standard deviation) for the content of C, N, and P were calculated using the R package “psych” (Revelle, 2023), coefficient of variation was calculated as the ratio of standard deviation to mean expressed as a percentage. Comparisons were made considering taxonomic placement and ecological guild membership. The results were visually presented using boxplots and line charts created with the R package “ggplot2” (Wickham, 2016). The significance of differences in the C, N, P, and chitin content between taxa at various taxonomic levels and between ecological guilds was evaluated using the Kruskal–Wallis test, followed by the Dunn test, implemented with the “FSA” R-package (Ogle et al., 2022). The strength of correlations between variables was assessed using Pearson’s r with the “ggpubr” R-package (Kassambara, 2020).
The phylogenetic trees, along with the data on nutrient levels for all samples, were utilized to calculate Pagel’s lambda (λP), expressing the value of phylogenetic signal (0 = closely related samples are not more similar than distant ones, 1 = total determination of the trait by phylogenetic relations between samples, Molina-Venegas and Rodríguez, 2017) for the content of N and the C:N ratio. The calculation of λP was carried out using the “phytools” R-package (Revell, 2012) with the lambda method. To rule out the possibility of the influence of the number of samples (n) in respective categories on the λP value, a correlation test between λP and n was performed using Pearson’s r, implemented with the “ggpubr” R-package (Kassambara, 2020).
3 Results
The fungal fruiting bodies samples belonged to 13 orders, predominantly covering Basidiomycota, with some species of Ascomycota. Among the 282 samples, there were 212 species from 82 fungal genera and 53 families. These were classified according to Põlme et al. (2020) into EcM (154 samples), SS (73 samples), and WS (55 samples; Supplementary Table 1 and Figure 1).
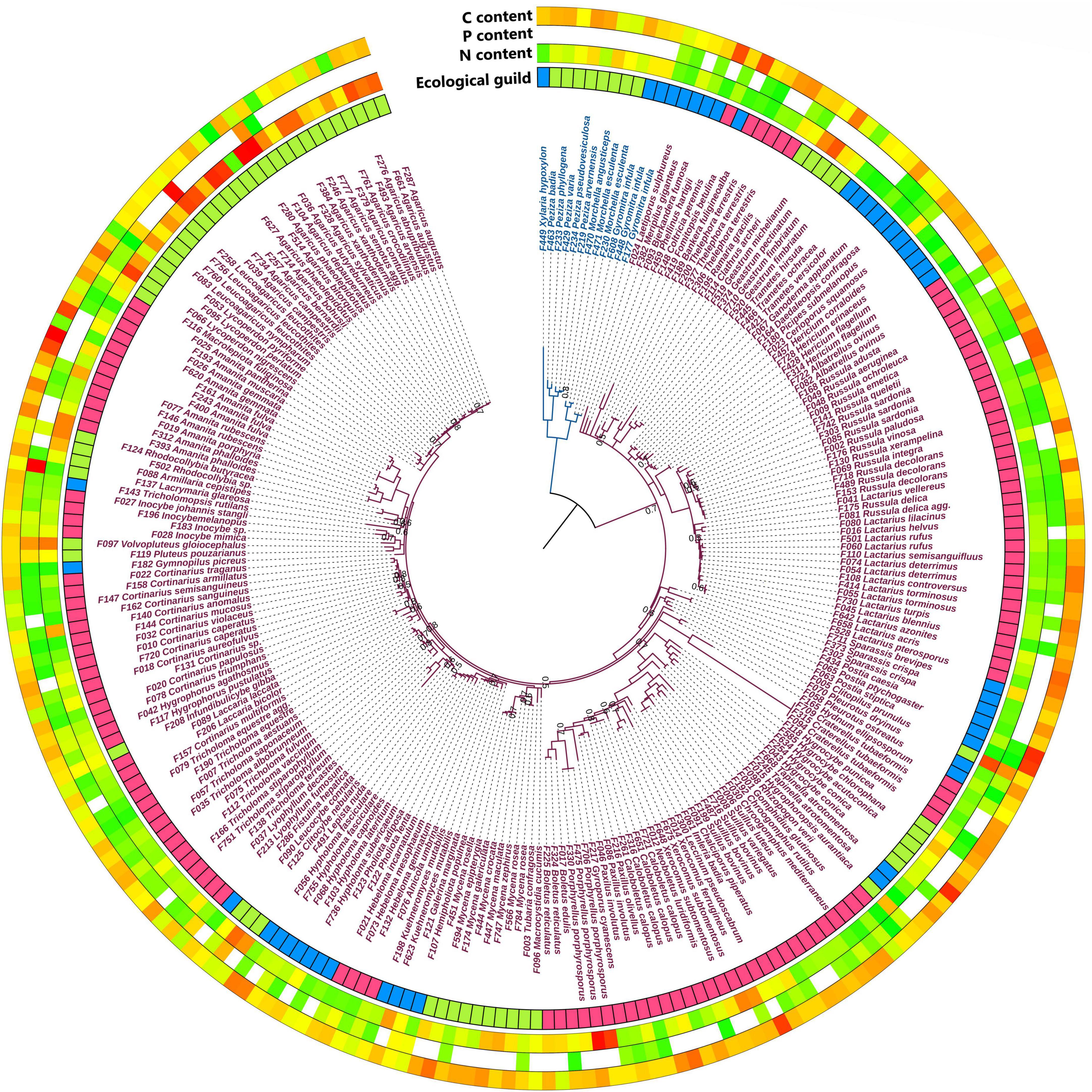
Figure 1. Phylogenetic tree of 282 fungal samples based on the 28S rDNA region. Ecological guild: blue—wood saprotrophs, red—ectomycorrhizal fungi, green—soil saprotrophs. Colors of the outer rings indicate content from low (green) to high (red). Species names of the Basidiomycota are purple, and the Ascomycetes are blue. At tree nodes, only values of posterior probabilities less than or equal to 0.8 are given.
3.1 C, N, P, and chitin content in fungal biomass
The C content in fungal fruiting bodies ranged from 29.8 to 49.4% and showed relatively little variation (41.5 ± 2.6%, n = 271). The N content was more variable, spanning more than one order of magnitude from 0.83 to 10.3% (4.6 ± 2.0%, n = 271). The highest N content, exceeding 10%, was observed in the soil saprotrophic fungi Agaricus depauperatus and Agaricus phaeolepidotus, as well as in the ectomycorrhizal species Porphyrellus porphyrosporus. The P content ranged from 1.0 mg g–1 to 18.9 mg g–1 (5.5 ± 3.0 mg g–1, n = 155), with the highest content above 1.8% observed in the soil saprotrophic species Agaricus campestris and Lacrymaria glareosa. The average ratio of C:N:P across the whole dataset was 99:9:1, but the variation of stoichiometric ratios among samples was wide: C/N ranged from 3.6 to 50.0 (11.2 ± 6.4, n = 271), and C/P ranged from 20.8 to 349 (99 ± 61, n = 145; Table 1).
There was a significant negative correlation between the contents of C and P (r = −0.226, p = 0.0063, Figure 2) within whole dataset, while the content of N and P showed a stronger positive correlation (r = 0.478, p < 0.001, Figure 2). However, when considering ecological guilds, this correlation was found to be significant only within the EcM fungi group, which had the highest number of observations (r = 0.285, p = 0.0048). There was a correlation (r = 0.298, p = 0.0239, Figure 2) between chitin and N, as well as between chitin and the C:N ratio (r = −0.290, p = 0.0282) when all samples are considered together.
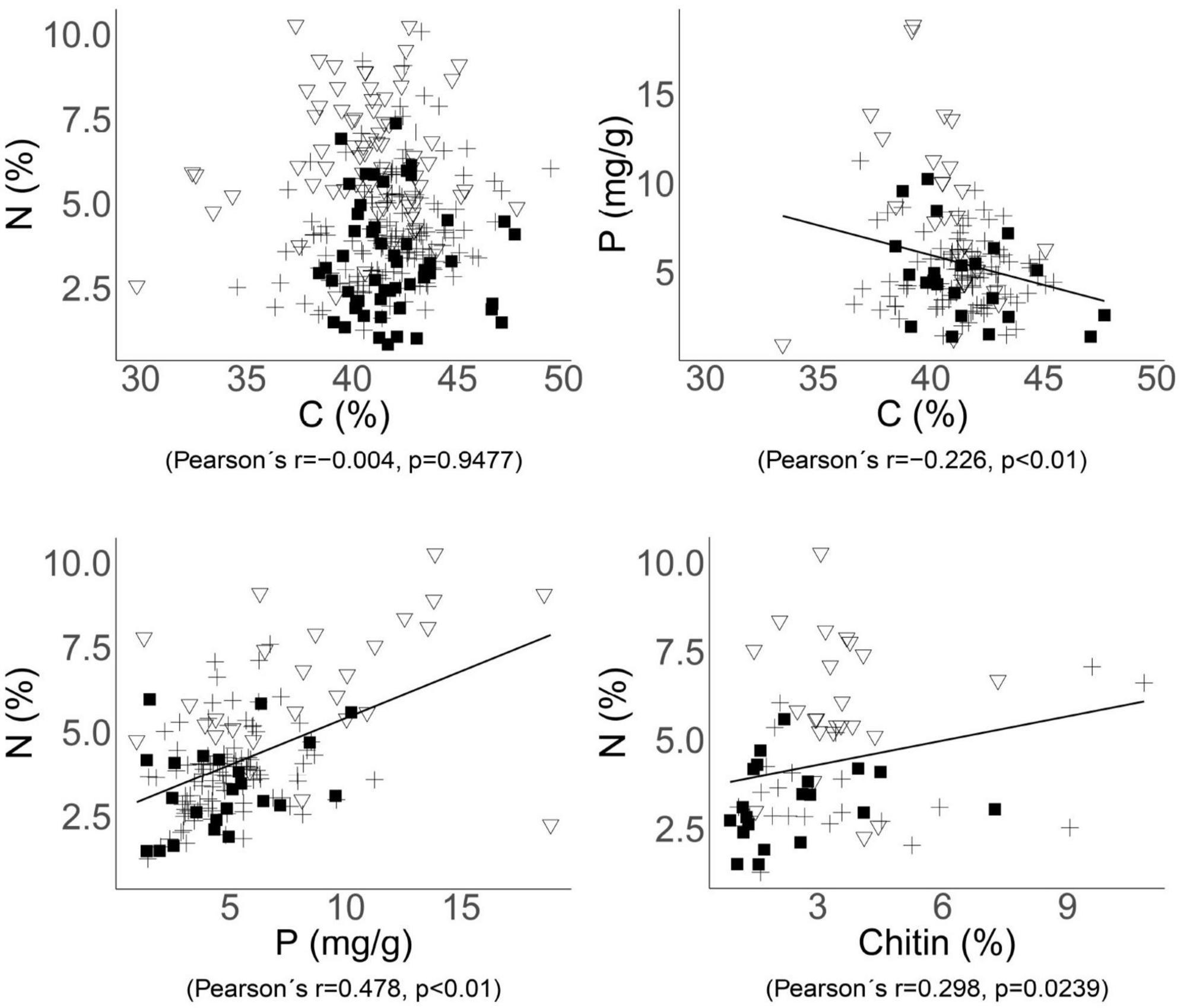
Figure 2. Relationships between the C, N, P, and chitin contents in fungal biomass. Significant trends across the whole dataset are indicated by correlations with Pearson’s correlation coefficients and p-values. Each point represents one species of ectomycorrhizal (cross), soil saprotrophic (triangle), or wood saprotrophic (square) fungi.
The chitin content in fungal fruiting bodies varied between 0.9 and 10.8% (3.1 ± 2.0, n = 70). The content of chitin was smallest in wood saprotrophs (2.4% ± 1.6% of dry mass), significantly less than in soil saprotrophs (3.4% ± 1.2%). In ectomycorrhizal fungi, chitin content varied more widely (3.6% ± 2.8%; Supplementary Table 2), and this group also harbored species with the highest chitin content around 10%–Amanita citrina and Cortinarius anomalus. The chitin content showed a significant positive correlation with N content in fungal biomass (r = 0.298, p = 0.0239; Figure 2), despite the fact that N contained in chitin represented just a small proportion of the total N in fungal biomass–between 4.56 and 7.36%.
3.2 Nutrient contents in fungi of contrasting ecology
Interestingly, the nutrient content in fungal biomass differed between ecological groups of fungi (Table 1 and Figure 3). Although no significant differences were found for C content between fungal groups (Figure 3), the mean content of N and P in the biomass of soil saprotrophs was significantly higher, by approximately 80%, than in wood saprotrophs (p < 0.05, Kruskal–Wallis test). The content of N in ectomycorrhizal fungi was significantly lower than in soil saprotrophs yet higher than in wood saprotrophs (p < 0.05). Regarding P content, the mean values in ectomycorrhizal and wood saprotrophs fungi were similar, but significantly different in soil saprotrophs. When comparing the ratios between elements, we observed that the C:N ratio exhibited an inverse trend to the N content. Specifically, wood saprotrophs displayed the highest C:N ratio values, whereas soil saprotrophs exhibited the lowest values. Despite the values for C:P and N:P ratios being highly variable between the three ecological guilds, our results showed that the C:P ratio was significantly different between wood saprotrophs and soil saprotrophs.
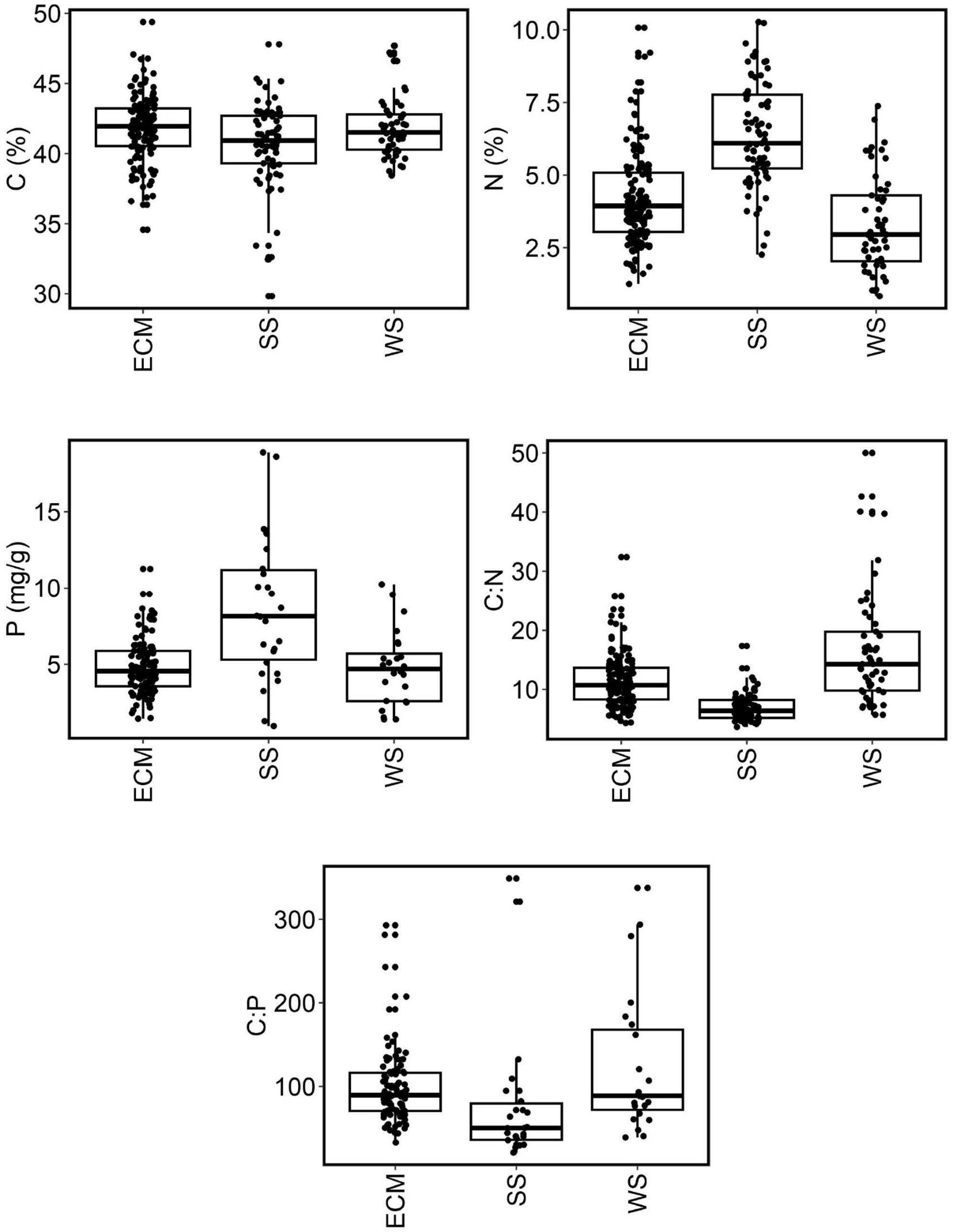
Figure 3. Nutrient content and nutrient stoichiometry in ectomycorrhizal fungi, soil saprotrophic fungi, and wood saprotrophic fungi. Boxplots indicate averages, lower and upper quartiles, and ranges (outliers excluded). ECM, ectomycorrhizal fungi; SS, soil saprotrophs; WS, wood saprotrophs.
3.3 Phylogenetic conservation of nutrient content
The variation in nutrient content, as well as stoichiometric ratios of nutrients, increased from low taxonomic ranks to higher taxonomic ranks. While the coefficient of variation for C was 1.4 times higher at the order level compared to the species level, the corresponding values for N and P were approximately double and triple, respectively (Table 2).
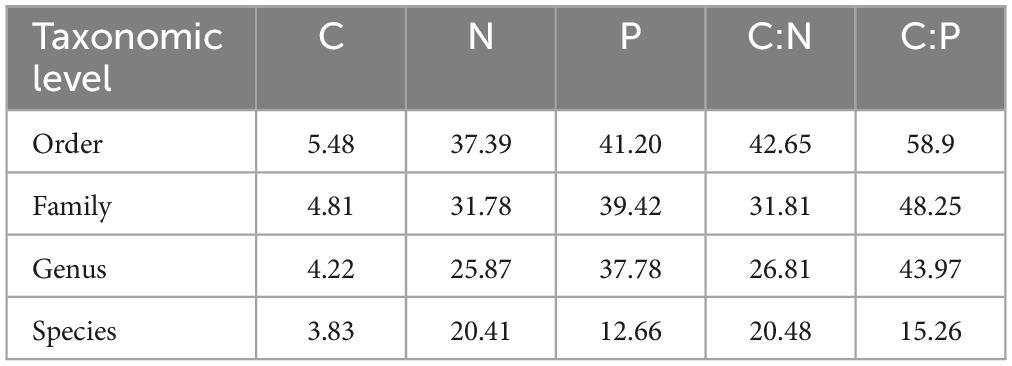
Table 2. Variation of nutrient content and nutrient stoichiometry within taxonomic ranks of fungi, expressed as the mean coefficient of variation.
When analyzing the phylogenetic conservation of nutrient content traits in all samples, we found a significant phylogenetic signal for N and P content, as well as for the C:N and C:P ratios; no phylogenetic signal was found for C content (Supplementary Table 3). When testing lower taxonomic levels (order, genus), λP values differed between various taxa and generally decreased at lower taxonomy levels (although λP values were slightly affected by sampling depth, p = 0.08). The fact that phylogenetic conservation was observed for selected genera indicates that nutrient contents are phylogenetically conserved at the species level. This corroborates the observations of the limited variation of N and P content within fungal species and genera (Table 2).
The three most abundantly recorded orders—Agaricales, Boletales, and Russulales (138, 42, and 50 samples, respectively)—showed differences in the level of phylogenetic conservation of traits. While no phylogenetic signal was recorded in the Russulales, high levels of phylogenetic conservation for all three nutrients were observed in the Boletales.
4 Discussion
Our results support the hypothesis that N and P content in fungal biomass differs among ecological guilds (Root, 1967; Põlme et al., 2020)—mycorrhizal, soil, and wood saprotrophic fungi. Thus, the nutrient content is, to some extent, determined by the environments to which these fungal groups have been adapted and the source of C that they use. In comparison to both saprotrophic groups, mycorrhizal fungi have an advantage of a stable supply of C as a result of their mutualistic relations with host plants (Bödeker et al., 2016). As a consequence of the trade with the host plants for C, N, and P, these fungi should pass the excess of N and P acquired from the soil to their host, retaining limited amounts of these nutrients in their mycelia (Trocha et al., 2016; Zhang and Elser, 2017; Kranabetter et al., 2019). This is reflected by lower contents of N and P in this guild, as well as lower variation in the content of these two nutrients. Conversely, the high average values discovered among soil saprotrophic species, where such nutrient trade does not take place, could be explained as an ability of soil saprotrophic fungi to store N and P in case of their excess (Quinché, 1997; Mooshammer et al., 2014; Trocha et al., 2016; Kalač, 2019; Kranabetter et al., 2019). The low content of N and P in wood saprotrophs compared to soil saprotrophs reflects their adaptation to the low nutrient contents in deadwood (Watkinson et al., 2006; Piché-Choquette et al., 2023).
Our results show—in addition to the effect of fungal lifestyle—the phylogenetic conservation of nutrient content. Lilleskov et al. (2011) showed that the response of fungi to elevated N content in soil is consistent only at the level of genera, and other ecological traits of fungi are also only rarely conserved at higher taxonomic levels (Zanne et al., 2020). This was also confirmed by the results of our study, where the conservation of nutrient content in biomass was highest at low taxonomic levels, while its variability increased with taxonomic rank. This may have been due to the fact that fungi have repeatedly evolved different lifestyles during evolution (Martin et al., 2016; Zanne et al., 2020), and therefore phylogenetic relationships at higher taxonomic levels may not correspond to the ecological features of fungal species. Although the differences in nutrient content between taxonomic groups are not as pronounced as differences between ecological guilds, phylogenetic relations seem to play some role in the determination of nutrient content in biomass.
Neither the phylogenetic relationship nor the membership in ecological guilds strongly determines the nutrient content in biomass, as indicated by the share of unexplained variation. This unexplained variation is likely associated with local, highly variable environmental conditions, where nutrient levels in fungi somewhat mirror those in the surroundings. This variability may also suggest an adaptive response to fluctuating environmental conditions (Vogt et al., 1981; Camenzind et al., 2020).
Due to dissimilar sources of N and P, their soil content could be highly variable. Such variability is associated with the type of geological bedrock, soil type, altitude, soil depth, and other factors; there can be many-fold differences in N content between various forest soils (Huntington et al., 1988; Batjes, 1996). Even higher is the variability of soil P content, exceeding differences of two orders of magnitude between various sites (Deng et al., 2017). Substantially low is nutrient content in wood, but the differences among tree species are considerable (Cowling and Merrill, 1966). Despite these dissimilarities and high, mutually independent variabilities of N and P causing considerable variability of their ratios within fungal habitats, the content of N and P in fungal biomass shows a significant positive correlation (r = 0.478, p < 0.01, Figure 2). No significant differences were found between all three ecological guilds in the N:P ratio, which could indicate that fungi in general maintain an approximately similar ratio of these nutrients, although the variability of this ratio is high, especially within both groups of saprotrophs. The N:P ratio is important particularly for mycorrhizal fungi, because it is tightly associated with their successful C, N, and P trade-off with the host plant (Johnson, 2010). This could explain the lower variability of the N:P ratio in the EcM group compared to the other two fungal groups (Table 1). Conversely, saprotrophic fungi may store both N and P independently of each other, according to their obtainable quantity, which could explain their higher variability in this ratio.
The content of chitin in fungal biomass was significantly lower in wood saprotrophic fungi than in the other two groups, although the variability in all three groups was high. As we hypothesized, the chitin content showed a positive correlation with the total N in mycelium (r = 0.298, p = 0.0239; Figure 2), indicating that fungi with sufficient N supply may synthesize more chitin. Chitin, as a chemical compound, contains approximately 6.9% of N (No and Meyers, 1995; Tracey, 1955), and N in chitin constitutes only 4.6–7.4% of the total N in the biomass (Supplementary Table 2). Therefore, the contribution of N bound in chitin to the differences in N content among fungal ecological guilds is limited. These differences are more likely caused by N in proteins and intracellular content rather than by N localized in chitin. This is further supported by the observation that soil saprotrophic fungi, which have the highest levels of total nitrogen, do not necessarily contain the highest levels of chitin.
Fungal mycelium is an important component of food chains, serving as a source of nutrients after its decomposition. Differences in N content in biomass between fungi of all three ecological guilds are significant, indicating variable attractiveness of dead mycelium for subsequent decomposition. Not surprisingly, N content in fungal biomass was the best predictor of its decomposition rate (Fernandez et al., 2016; Brabcová et al., 2018). In general, the biomass of soil saprotrophs is anticipated to decompose more rapidly than that of the ECM and wood saprotrophic fungi owing to the higher nitrogen content; however, the rate of decomposition is contingent upon the particular combination of environmental conditions and the unique properties of each fungus. The increasing deposition of N, which was frequently documented (Morrison et al., 2016; Hůnová et al., 2017; Baldrian et al., 2023), raises the question of its influence on fungi of various ecological guilds. While the wood, as the habitat of wood saprotrophic fungi, should be relatively little affected by N deposition, the impact on soil saprotrophic and mycorrhizal fungi could be more pronounced. Since the provision of N to plants is one of the essential functions of mycorrhizal symbioses (Kranabetter et al., 2019), increased N availability as a result of N deposition might affect the importance of mycorrhiza for plants. In such a case, at least the shift of the limitation to P could be expected. For the saprotrophic fungi, the direct impact of higher N availability should not be of cardinal significance since these fungi seem to be able to cope with various levels of nutrients and their ratios. But some indirect influence, such as increasing competition of other groups of microorganisms, could be assumed instead.
Our results indicate that both ecology and evolution determine nutrient contents in fungal biomass. In the light of ongoing global climatic change and continuing increased N deposition, it is especially important to assess how the differences in nutrient content among fungal guilds and the share of C that they receive within the soil affect the further fate of fungal biomass and whether it can impact organic matter accumulation or C storage in soils.
Data availability statement
The datasets presented in this study can be found in online repositories. The names of the repository/repositories and accession number(s) can be found in this article/Supplementary material.
Author contributions
MP: Conceptualization, Formal analysis, Investigation, Methodology, Writing – original draft. TV: Formal analysis, Resources, Writing – review & editing. TM: Formal analysis, Resources, Writing – review & editing. JB: Formal analysis, Resources, Writing – review & editing. LT: Resources, Writing – review & editing. BA: Formal analysis, Writing – review & editing. PB: Conceptualization, Methodology, Supervision, Writing – review & editing. RL-M: Conceptualization, Funding acquisition, Methodology, Writing – review & editing.
Funding
The authors declare that financial support was received for the research, authorship, and/or publication of this article. This work was supported by the Czech Science Foundation (22-30769S) and by the Ministry of Education, Youth and Sports of the Czech Republic (CZ.02.01.01/00/22_008/0004635–AdAgriF–Advanced methods of greenhouse gas emission reduction and sequestration in agriculture and forest landscape for climate change mitigation). The work of JB was supported by the Long-term Development Projects RVO67985831 and RVO61389005.
Conflict of interest
The authors declare that the research was conducted in the absence of any commercial or financial relationships that could be construed as a potential conflict of interest.
Publisher’s note
All claims expressed in this article are solely those of the authors and do not necessarily represent those of their affiliated organizations, or those of the publisher, the editors and the reviewers. Any product that may be evaluated in this article, or claim that may be made by its manufacturer, is not guaranteed or endorsed by the publisher.
Supplementary material
The Supplementary Material for this article can be found online at: https://www.frontiersin.org/articles/10.3389/fmicb.2024.1379825/full#supplementary-material
References
Adamczyk, S., Larmola, T., Peltoniemi, K., Laiho, R., Näsholm, T., and Adamczyk, B. (2020). An optimized method for studying fungal biomass and necromass in peatlands via chitin concentration. Soil Biol. Biochem. 149:107932. doi: 10.1016/j.soilbio.2020.107932
Bai, Z., Ye, J., Wei, Y. L., Yan, S. K., and Yuan, H. S. (2021). Soil depth-dependent C/N stoichiometry and fungal and bacterial communities along a temperate forest succession gradient. Catena 207:105613. doi: 10.1016/j.catena.2021.105613
Baldrian, P., Bell-Dereske, L., Lepinay, C., Větrovský, T., and Kohout, P. (2022). Fungal communities in soils under global change. Stud. Mycol. 103, 1–24. doi: 10.3114/sim.2022.103.01
Baldrian, P., López-Mondéjar, R., and Kohout, P. (2023). Forest microbiome and global change. Nat. Rev. Microbiol. 21, 487–501. doi: 10.1038/s41579-023-00876-4
Batjes, N. H. (1996). Total carbon and nitrogen in the soils of the world. Eur. J. Soil Sci. 47, 151–163. doi: 10.1111/j.1365-2389.1996.tb01386.x
Blumenthal, H. J., and Saul, R. (1957). Quantitative estimation of chitin in fungi. J. Bacteriol. 74, 222–224. doi: 10.1128/jb.74.2.222-224.1957
Bödeker, I., Lindahl, B., Olson, A., and Clemmensen, K. (2016). Mycorrhizal and saprotrophic fungal guilds compete for the same organic substrates but affect decomposition differently. Funct. Ecol. 30, 1967–1978. doi: 10.1111/1365-2435.12677
Brabcová, V., Štursová, M., and Baldrian, P. (2018). Nutrient content affects the turnover of fungal biomass in forest topsoil and the composition of associated microbial communities. Soil Biol. Biochem. 118, 187–198. doi: 10.1016/j.soilbio.2017.12.012
Camenzind, T., Lehmann, A., Ahland, J., Rumpel, S., and Rillig, M. C. (2020). Trait-based approaches reveal fungal adaptations to nutrient-limiting conditions. Environ. Microbiol. 22, 3548–3560. doi: 10.1111/1462-2920.15132
Camenzind, T., Philipp Grenz, K., Lehmann, J., and Rillig, M. C. (2021). Soil fungal mycelia have unexpectedly flexible stoichiometric C:N and C:P ratios. Ecol. Lett. 24, 208–218. doi: 10.1111/ele.13632
Cleveland, C. C., and Liptzin, D. (2007). C:N:P stoichiometry in soil: Is there a “Redfield ratio” for the microbial biomass? Biogeochemistry 85, 235–252. doi: 10.1007/s10533-007-9132-0
Cowling, E. B., and Merrill, W. (1966). Nitrogen in wood and its role in wood deterioration. Can. J. Bot. 44, 1539–1554. doi: 10.1139/b66-167
Darriba, D., Taboada, G. L., Doallo, R., and Posada, D. (2012). jModelTest 2: More models, new heuristics and parallel computing. Nat. Methods 9, 772–772.
Deng, Q., McMahon, D. E., Xiang, Y., Yu, C.-L., Jackson, R. B., and Hui, D. (2017). A global meta-analysis of soil phosphorus dynamics after afforestation. New Phytol. 213, 181–192. doi: 10.1111/nph.14119
Du, E., Terrer, C., Pellegrini, A. F. A., Ahlström, A., van Lissa, C. J., Zhao, X., et al. (2020). Global patterns of terrestrial nitrogen and phosphorus limitation. Nat. Geosci. 13, 221–226. doi: 10.1038/s41561-019-0530-4
Fernandez, C. W., Langley, J. A., Chapman, S., McCormack, M. L., and Koide, R. T. (2016). The decomposition of ectomycorrhizal fungal necromass. Soil Biol. Biochem. 93, 38–49. doi: 10.1016/j.soilbio.2015.10.017
Gruber, S., and Seidl-Seiboth, V. (2012). Self versus non-self: Fungal cell wall degradation in Trichoderma. Microbiology 158, 26–34. doi: 10.1099/mic.0.052613-0
Hall, T. (1999). BioEdit: A user-friendly biological sequence alignment editor and analysis program for windows 95/98/NT. Nucleic Acids Symp. Ser. 41, 95–98. doi: 10.1021/bk-1999-0734.ch008
Hibbett, D. S., and Vilgalys, R. (1993). Phylogenetic relationships of Lentinus (Basidiomycotina) inferred from molecular and morphological characters. Syst. Bot. 18, 409–433. doi: 10.2307/2419417
Hůnová, I., Kurfürst, P., Stráník, V., and Modlík, M. (2017). Nitrogen deposition to forest ecosystems with focus on its different forms. Sci. Total Environ. 575, 791–798. doi: 10.1016/j.scitotenv.2016.09.140
Huntington, T. G., Ryan, D. F., and Hamburg, S. P. (1988). Estimating soil nitrogen and carbon pools in a northern hardwood forest ecosystem. Soil Sci. Soc. Am. J. 52, 1162–1167. doi: 10.2136/sssaj1988.03615995005200040049x
Johnson, N. C. (2010). Resource stoichiometry elucidates the structure and function of arbuscular mycorrhizas across scales. New Phytol. 185, 631–647. doi: 10.1111/j.1469-8137.2009.03110.x
Kalač, P. (2019). “Major essential elements,” in Mineral composition and radioactivity of edible mushrooms, ed. P. Kalač (Cambridge, MA: Academic Press), 25–74. doi: 10.1016/B978-0-12-817565-1.00003-0
Kassambara, A. (2020). ggpubr: “ggplot2” based publication ready plots. Available online at: https://CRAN.R-project.org/package=ggpubr (accessed May 5, 2024).
Kranabetter, J. M., Harman-Denhoed, R., and Hawkins, B. J. (2019). Saprotrophic and ectomycorrhizal fungal sporocarp stoichiometry (C: N: P) across temperate rainforests as evidence of shared nutrient constraints among symbionts. New Phytol. 221, 482–492. doi: 10.1111/nph.15380
Larsen, S. (1967). “Soil phosphorus,” in Advances in agronomy, ed. A. G. Norman (Cambridge, MA: Academic Press), 151–210.
Lenardon, M. D., Munro, C. A., and Gow, N. A. R. (2010). Chitin synthesis and fungal pathogenesis. Curr. Opin. Microbiol. 13, 416–423. doi: 10.1016/j.mib.2010.05.002
Lilleskov, E. A., Hobbie, E. A., and Horton, T. R. (2011). Conservation of ectomycorrhizal fungi: Exploring the linkages between functional and taxonomic responses to anthropogenic N deposition. Fungal Ecol. 4, 174–183. doi: 10.1016/j.funeco.2010.09.008
Lindahl, B. D., Ihrmark, K., Boberg, J., Trumbore, S. E., Högberg, P., Stenlid, J., et al. (2007). Spatial separation of litter decomposition and mycorrhizal nitrogen uptake in a boreal forest. New Phytol. 173, 611–620. doi: 10.1111/j.1469-8137.2006.01936.x
Lindahl, B. O., Taylor, A. F. S., and Finlay, R. D. (2002). Defining nutritional constraints on carbon cycling in boreal forests – towards a less “phytocentric” perspective. Plant Soil 242, 123–135. doi: 10.1023/A:1019650226585
López-Mondéjar, R., Tláskal, V., Větrovský, T., Štursová, M., Toscan, R., and Nunes, et al. (2020). Metagenomics and stable isotope probing reveal the complementary contribution of fungal and bacterial communities in the recycling of dead biomass in forest soil. Soil Biol. Biochem. 148:107875. doi: 10.1016/j.soilbio.2020.107875
Martin, F., Kohler, A., Murat, C., Veneault-Fourrey, C., and Hibbett, D. S. (2016). Unearthing the roots of ectomycorrhizal symbioses. Nat. Rev. Microbiol. 14, 760–773. doi: 10.1038/nrmicro.2016.149
Marzluf, G. A. (1996). “Regulation of nitrogen metabolism in mycelial fungi,” in Biochemistry and molecular biology, eds R. Brambl and G. A. Marzluf (Berlin: Springer), 357–368. doi: 10.1007/978-3-662-10367-8_16
Molina-Venegas, R., and Rodríguez, M. A. (2017). Revisiting phylogenetic signal; strong or negligible impacts of polytomies and branch length information? BMC Evol. Biol. 17:53. doi: 10.1186/s12862-017-0898-y
Mooshammer, M., Wanek, W., Zechmeister-Boltenstern, S., and Richter, A. (2014). Stoichiometric imbalances between terrestrial decomposer communities and their resources: Mechanisms and implications of microbial adaptations to their resources. Front. Microbiol. 5:22. doi: 10.3389/fmicb.2014.00022
Morrison, E. W., Frey, S. D., Sadowsky, J. J., van Diepen, L. T. A., Thomas, W. K., and Pringle, A. (2016). Chronic nitrogen additions fundamentally restructure the soil fungal community in a temperate forest. Fungal Ecol. 23, 48–57. doi: 10.1016/j.funeco.2016.05.011
Mouginot, C., Kawamura, R., Matulich, K. L., Berlemont, R., Allison, S. D., Amend, A. S., et al. (2014). Elemental stoichiometry of fungi and bacteria strains from grassland leaf litter. Soil Biol. Biochem. 76, 278–285. doi: 10.1016/j.soilbio.2014.05.011
Nelson, D. W., and Sommers, L. E. (1996). “Total carbon, organic carbon, and organic matter,” in Methods of soil analysis, eds D. L. Sparks, A. L. Page, P. A. Helmke, R. H. Loeppert, P. N. Soltanpour, M. A. Tabatabai, et al. (New York, NY: Oxford University Press), 961–1010. doi: 10.2136/sssabookser5.3.c34
No, H. K., and Meyers, S. P. (1995). Preparation and characterization of chitin and chitosan– review. J. Aquat. Food Prod. Technol. 4, 27–52. doi: 10.1300/J030v04n02_03
Ogle, D. H., Doll, J. C., Wheeler, P., and Dinno, A. (2022). Simple fisheries stock assessment methods. R package version 0.9.5. Available online at: https://CRAN.R-project.org/package=FSA (accessed May 5, 2024).
Ohno, T., and Zibilske, L. M. (1991). Determination of low concentrations of phosphorus in soil extracts using malachite green. Soil Sci. Soc. Am. J. 55, 892–895. doi: 10.2136/sssaj1991.03615995005500030046x
Piché-Choquette, S., Tláskal, V., Vrška, T., Jiráska, L., Větrovský, T., and Baldrian, P. (2023). Continuous microhabitats as crossroads of fungal communities in a primeval temperate forest. Soil Biol. Biochem. 187:109187. doi: 10.1016/j.soilbio.2023.109187
Põlme, S., Abarenkov, K., Henrik Nilsson, R., Lindahl, B. D., Clemmensen, K. E., Kauserud, H., et al. (2020). FungalTraits: A user-friendly traits database of fungi and fungus-like stramenopiles. Fungal Divers. 105, 1–16. doi: 10.1007/s13225-020-00466-2
Quinché, J. P. (1997). Phosphorus and heavy metals in some species of fungi. Rev. Suisse Agric. 29, 151–156.
Ramirez, K. S., Craine, J. M., and Fierer, N. (2012). Consistent effects of nitrogen amendments on soil microbial communities and processes across biomes. Glob. Change Biol. 18, 1918–1927. doi: 10.1111/j.1365-2486.2012.02639.x
Rayner, A., and Boddy, L. (1989). Fungal decomposition of wood. Its biology and ecology. Chichester: John Wiley & Sons Ltd.
Revell, L. J. (2012). phytools: An R package for phylogenetic comparative biology (and other things). Methods Ecol. Evol. 3, 217–223. doi: 10.1111/j.2041-210X.2011.00169.x
Revelle, W. (2023). psych: Procedures for psychological, psychometric, and personality research. Available online at: https://CRAN.R-project.org/package=psych (accessed May 5, 2024).
Ronquist, F., Teslenko, M., van der Mark, P., Ayres, D. L., Darling, A., Höhna, S., et al. (2012). MrBayes 3.2: Efficient Bayesian phylogenetic inference and model choice across a large model space. Syst. Biol. 61, 539–542. doi: 10.1093/sysbio/sys029
Root, R. B. (1967). The niche exploitation pattern of the blue-gray gnatcatcher. Ecol. Monogr. 37, 317–350. doi: 10.2307/1942327
Schneider, K., Resl, P., Westberg, M., and Spribille, T. (2015). A new, highly effective primer pair to exclude algae when amplifying nuclear large ribosomal subunit (LSU) DNA from lichens. Lichenologist 47, 269–275. doi: 10.1017/S002428291500016X
Schoch, C. L., Seifert, K. A., Huhndorf, S., Robert, V., Spouge, J. L., Levesque, C., et al. (2012). From the cover: Nuclear ribosomal internal transcribed spacer (ITS) region as a universal DNA barcode marker for Fungi. Proc. Natl. Acad. Sci. U.S.A. 109, 6241–6246. doi: 10.1073/pnas.1117018109
Starke, R., Morais, D., Větrovský, T., López Mondéjar, R., Baldrian, P., and Brabcová, V. (2020). Feeding on fungi: Genomic and proteomic analysis of the enzymatic machinery of bacteria decomposing fungal biomass. Environ. Microbiol. 22, 4604–4619. doi: 10.1111/1462-2920.15183
Tracey, M. V. (1955). “Chitin,” in Modern methods of plant analysis / Moderne methoden der pflanzenanalyse, eds K. Paech and M. V. Tracey (Berlin: Springer), 2. doi: 10.1007/978-3-642-64955-4_9
Trocha, L. K., Rudy, E., Chen, W., Dabert, M., and Eissenstat, D. M. (2016). Linking the respiration of fungal sporocarps with their nitrogen concentration: Variation among species, tissues and guilds. Funct. Ecol. 30, 1756–1768. doi: 10.1111/1365-2435.12688
van Breemen, N., and van Dijk, H. F. G. (1988). Ecosystem effects of atmospheric deposition of nitrogen in The Netherlands. Environ. Pollut. 54, 249–274. doi: 10.1016/0269-7491(88)90115-7
van der Linde, S., Suz, L. M., Orme, C. D. L., Cox, F., Andreae, H., Asi, E., et al. (2018). Environment and host as large-scale controls of ectomycorrhizal fungi. Nature 558, 243–248. doi: 10.1038/s41586-018-0189-9
Vilgalys, R., and Hester, M. (1990). Rapid genetic identification and mapping of enzymatically amplified ribosomal DNA from several Cryptococcus species. J. Bacteriol. 172, 4238–4246. doi: 10.1128/jb.172.8.4238-4246.1990
Vogt, K. A., Edmonds, R. L., and Grier, C. C. (1981). Biomass and nutrient concentrations of sporocarps produced by mycorrhizal and decomposer fungi in Abies amabilis stands. Oecologia 50, 170–175. doi: 10.1007/BF00348033
Watkinson, S., Bebber, D., Darrah, P., Fricker, M., Tlalka, M., and Boddy, L. (2006). “The role of wood decay fungi in the carbon and nitrogen dynamics of the forest floor,” in Fungi in biogeochemical cycles, ed. G. M. Gadd (Cambridge: Cambridge University Press), 151–181. doi: 10.1017/CBO9780511550522.008
White, S., McIntyre, M., Berry, D. R., and McNeil, B. (2002). The autolysis of industrial filamentous fungi. Crit. Rev. Biotechnol. 22, 1–14. doi: 10.1080/07388550290789432
White, T. J., Bruns, T., Lee, S., and Taylor, J. (1990). “Amplification and direct sequencing of fungal ribosomal RNA genes for phylogenetics,” in PCR protocols: A guide to methods and applications, eds M. A. Innis, D. H. Gelfand, J. J. Sninsky, and T. J. White (New York, NY: Academic Press), 315–322.
Zanne, A. E., Abarenkov, K., Afkhami, M. E., Aguilar-Trigueros, C. A., Bates, S., and Bhatnagar, et al. (2020). Fungal functional ecology: Bringing a trait-based approach to plant-associated fungi. Biol. Rev. 95, 409–433. doi: 10.1111/brv.12570
Zhang, J., and Elser, J. J. (2017). Carbon:nitrogen:phosphorus stoichiometry in fungi: A meta-analysis. Front. Microbiol. 8:1281. doi: 10.3389/fmicb.2017.01281
Keywords: fungal biomass composition, nutrient stoichiometry, nutrient content variation, phylogenetic signal, ecological traits
Citation: Pánek M, Vlková T, Michalová T, Borovička J, Tedersoo L, Adamczyk B, Baldrian P and Lopéz-Mondéjar R (2024) Variation of carbon, nitrogen and phosphorus content in fungi reflects their ecology and phylogeny. Front. Microbiol. 15:1379825. doi: 10.3389/fmicb.2024.1379825
Received: 31 January 2024; Accepted: 06 May 2024;
Published: 21 May 2024.
Edited by:
Fenliang Fan, Chinese Academy of Agricultural Sciences (CAAS), ChinaReviewed by:
Hai-Sheng Yuan, Chinese Academy of Sciences (CAS), ChinaYanan Wang, Chinese Academy of Agricultural Sciences (CAAS), China
Copyright © 2024 Pánek, Vlková, Michalová, Borovička, Tedersoo, Adamczyk, Baldrian and Lopéz-Mondéjar. This is an open-access article distributed under the terms of the Creative Commons Attribution License (CC BY). The use, distribution or reproduction in other forums is permitted, provided the original author(s) and the copyright owner(s) are credited and that the original publication in this journal is cited, in accordance with accepted academic practice. No use, distribution or reproduction is permitted which does not comply with these terms.
*Correspondence: Matěj Pánek, bWF0ZWoucGFuZWtAYmlvbWVkLmNhcy5jeg==