- 1College of Water Conservancy and Hydropower Engineering, Gansu Agricultural University, Lanzhou, China
- 2College of Forestry, Gansu Agricultural University, Lanzhou, China
In grape cultivation, incorrect water regulation will lead to significant water wastage, which in turn will change soil structure and disrupt soil nutrient cycling processes. This study aimed to investigate the effects of different water regulation treatments [by setting moderate water stress (W1), mild water stress (W2), and adequate water availability (CK)] on soil physical–chemical properties and enzyme activity in greenhouse grape during the growing season. The result showed that the W2 treatment had a negative impact on the build-up of dissolved organic carbon (DOC), nitrate nitrogen (NO3-N), and available phosphorus (AP). Throughout the reproductive period, the W1 and W2 treatments decreased the soil’s microbial biomass carbon (MBC) and microbial biomass nitrogen (MBN) contents, and MBC was more vulnerable to water stress. During the growth period, the trends of urease, catalase, and sucrase activities in different soil depth were ranked as 10–20 cm > 0–10 cm > 20–40 cm. The urease activity in 0–10 cm soil was suppressed by both W1 and W2 treatments, while the invertase activity in various soil layers under W1 treatment differed substantially. The W1 treatment also reduced the catalase activity in the 20–40 cm soil layer in the grape growth season. These findings suggested that W2 treatment can conserve water and enhance microbial ecology of greenhouse grape soils. Therefore, W2 treatment was the most effective water regulation measure for local greenhouse grape cultivation.
1 Introduction
Water scarcity is the most main environmental stress for crop growth, and water stress-induced agriculture failure will cause serious ecological and food security issues (Yang et al., 2021; Zhang C. et al., 2023). Water stress not only affected crop growth and microbial structure, limits soil nutrient transport but also leaded to a significant decrease in soil microbial biomass (Manzoni et al., 2012). The rhizosphere is the site of plant–soil-microorganism interaction, and the soil area where plants are highly sensitive to external environmental stress (Zhang et al., 2019). Studies have shown that water stress can impact crop chlorophyll levels (Ru et al., 2020), metabolic processes (Kapoor et al., 2020), root growth (Wang et al., 2019), and soil microbial communities (Bogati and Walczak, 2022). Secretions released during crop growth period also changed soil enzyme activity (Xiao et al., 2023). Therefore, complex interactions between soil water and crops combine to influence changes of rhizosphere soil microbials.
Physical–chemical property and microbial biomass are important regulators of soil element cycling and crop nutrient supply, and are the most sensitive and potential soil biological indicators (Marschner et al., 2005; Zhang et al., 2016; Yang et al., 2019). Soil organic carbon (SOC), total nitrogen (TN), total phosphorus (TP), nitrate nitrogen (NO3-N), ammonia nitrogen (NH4-N), and availability phosphorus (AP) are the main nutrients in soil and are crucial to all biological processes (Wu et al., 2020). Water stress severely disrupted the structure and function of soil, soil microbial biomass reaches 14.3%, and soil moisture is maintained above 10%, which in turn avoided damaging the soil system (Geng et al., 2015). Water stress not only decreases soil chemical fertility but also reduces microbial activity, including the activities of invertase and urease (Wu et al., 2012). The plant root system will firstly experience water stress, mainly as a result of inadequate or excessive water in the soil (Kim et al., 2020). Soil moisture affects the transfer and transportation of soil nutrients, which in turn affects the growth and reproduction of microorganisms (Xue et al., 2017). Therefore, the investigation of the impact of water stress on rhizosphere soil moisture, nutrients, and microorganisms in plants were critical.
Soil enzymes are specialized proteins with biocatalytic activity, known as “active reservoirs of plant nutrients.” Enzyme activity is higher in rhizosphere soil than in bulk soil (Marschner et al., 2005). In addition, catalase, urease, and sucrose were the main environmental variables affecting the composition of the soil microbial community in the grape rhizosphere (Song et al., 2024). Soil enzyme activity increased with increasing soil moisture, but decreased with excess soil water, as shown by Chrost (2014). Gramss et al. (1999) also found that adequate water stress (80% field water holding capacity) could stimulate plant roots to produce more enzymes, and this was due to oxygen limiting microbial activity. Soil enzyme activities were related to soil temperature, physical–chemical properties, and pH, respectively, and varied in different planting types and growth stages (Barta et al., 2014; Menichetti et al., 2015; Liu and Zhang, 2019; Jing et al., 2020; Zhao et al., 2020). In addition, some studies have shown that water stress can increase soil peroxidase, phosphatase, dehydrogenase, saccharase, and phosphatase activities (Song et al., 2012; Padhy et al., 2018; Kátai et al., 2020). However, the response of soil enzyme activities to water stress remains highly uncertain. Therefore, the aim of this study was to analyze the changes in rhizosphere soil enzyme activities of greenhouse grapes by different water stresses, which could further characterize the changes in enzyme activities.
In this study, the rhizosphere soil of greenhouse grapes in arid area was the object of study, and different water stress treatments were established. We analyzed the physical–chemical properties, microbial biomass, and enzyme activities of the soil to provide a theoretical basis for the scientific cultivation of greenhouse grapes in the arid region. The objectives of the study were: (1) to analyze the effects of different water stress conditions on physical–chemical properties, microbial biomass, and enzyme activities in rhizosphere soil at different growth stages; and (2) to explore the correlation between soil physical–chemical properties, microbial biomass and enzyme activity.
2 Materials and methods
2.1 Study site
The field experiment was conducted in 2019 at the Yongdeng Irrigation Experiment Station (36°43′34″N; 103°16′24″E; altitude: 2100 m) in Gansu Province, China. The study area is located in a semi-arid region, with a typical continental monsoon climate, and the average annual rainfall, evaporation, and temperature were 290 mm, 1,000 mm, and 5.9°C, respectively. The soil type of the experimental field was mainly loam, the water capacity was 29.2%, the density was 1.42 g cm−3, and the soil pH was 8.15.
2.2 Experimental design and management
Red globe, a 5-year-old Eurasian grape variety, was used as the test material. Grapes were planted in a plastic greenhouse of 8 m × 80 m with an earth wall straw curtain and the cultivation method of a single-arm Y-shaped low single-hedge frame was adopted. The row spacing was 2.0 m, and the plant spacing was 0.8 m. Each row (each treatment) comprised eight grapes, and the row direction was perpendicular to the greenhouse direction (Zhang et al., 2019). The experimental plots were designed in a randomized block group design and there were three replications for each treatment, containing a total of nine plots with a plot size of 8 m × 2 m.
The growth period of grapes was divided into five stages based on local protected-cultivation grape water consumption and irrigation experience (Table 1). The experiment included three treatments: (1) W1 treatment was moderate water stress (lower limit of soil moisture content was 55%); (2) W2 treatment was mild water stress (lower limit of soil moisture content was 65%); (3) CK treatment was the control treatment (sufficient water supply). The field trial of greenhouse grapes was irrigated by drip irrigation with the control mode of “one pipe and one row,” and the flow rate of sprinkler head was 3 L H−1. The irrigation was performed when the soil moisture in the field trial reached the lower limit of the experimental design, and the irrigation rate was 270 m3 hm−2. The valves and the water meters installed in each plot were used to control the amount of irrigation. The irrigation amount and irrigation time were determined by the soil moisture content and measured using a water meter. The soil moisture ratio was 0.5, and the planned depth of the wetting layer was 80 cm.
Local farming management practices were referred to for the use of fertilization, and insecticide and herbicide management. On 24th February, basal fertilizer (chicken manure; 5,000 kg hm−2) was applied along with 2 kg diammonium phosphate and 4 kg ammonium bicarbonate. Each treatment received 1 kg diammonium phosphate, 0.8 kg calcium ammonium nitrate for agriculture, 0.8 kg of organic fertilizer, and 0.5 kg of potassium magnesium sulfate at the 16th June, respectively. On 16th August, 0.8 kg diammonium phosphate, 0.8 kg calcium ammonium nitrate for agriculture, 0.8 kg organic fertilizer, and 0.6 kg potassium and magnesium sulfate were applied.
2.3 Collection of rhizosphere soil sample
Soil samples (0–40 cm) were collected on May 15, June 15, July 20, August 15, and October 15, 2019 at different growth periods. Three soil cores (3 cm diameter) of grape rhizosphere soil in each plot were taken at a depth of 0–10, 10–20, and 20–40 cm, respectively. Then, the samples in each plot were pooled by depth and transported to the laboratory. In the lab, the samples were sieved through a 2 mm mesh. The soil layer of 10–20 cm was divided into three parts; one part was air-dried at room temperature for determining some basic physical–chemical indices of the soil, and the other part was stored in a refrigerator at 4°C for fresh sample analysis (partial basic physical–chemical indices of soil and enzyme activity). Sterile gloves were worn during the sampling process. The ziplock bag, spatula, and other tools used for sampling were sterilized at high temperature to eliminate test errors. The measurement of each index was completed within 1 month after the completion of the sampling.
2.4 Soil physical–chemical properties
Total nitrogen (TN), nitrate nitrogen (NO3-N), and ammonia nitrogen (NH4-N) contents were determined by the Kjeldahl method (Boell and Shen, 1954) (Table 2). The soil total organic carbon (TOC) content was measured using a carbon and nitrogen combined analyzer (Multi N/C 2100 s, Jena, Germany) after removing inorganic carbon with 0.5 mol/L dilute hydrochloric acid. The dissolved organic carbon (DOC) content was determined with a carbon and nitrogen combined analyzer (Multi C/N 2100 s) after leaching with ultrapure water (water: soil = 5: 1). The microbial biomass carbon (MBC) and microbial biomass nitrogen (MBN) content were determined using a carbon and nitrogen combined analyzer after 0.5 mol·L−1 K2SO4 extraction (Multi C/N 2100 s) (Xu et al., 2016).
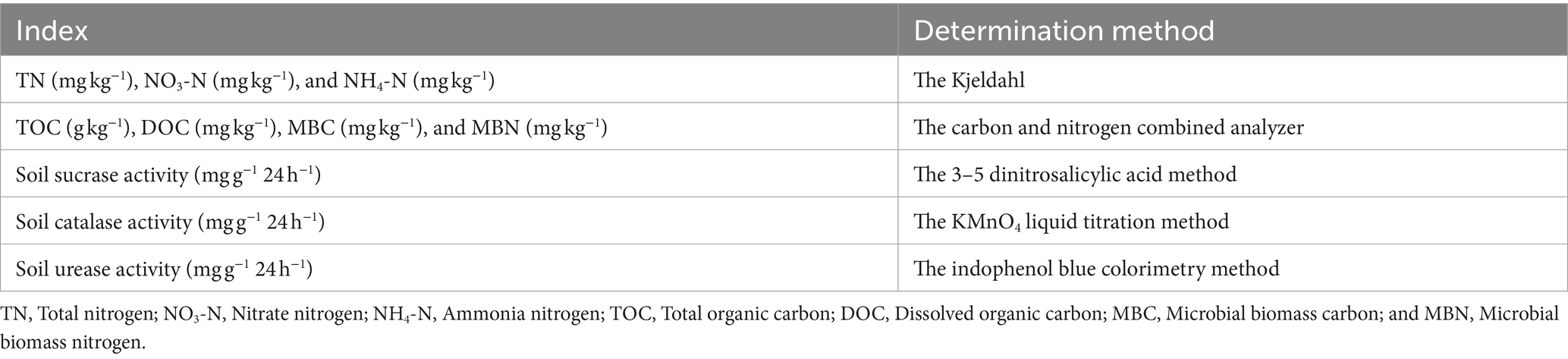
Table 2. Parametric determination of physical–chemical properties of greenhouse grape rhizosphere soils.
To determine the sucrase activity, 5 g of air-dried soils were incubated for 24 h at 37°C with 15 mL of 8% sucrose, 5 mL of phosphate buffer at pH 5.5, and 0.1 mL of toluene. The glucose released by sucrase reacted with 3-5-dinitrosalicylic acid and then was measured based on the absorbance at 508 nm (UV-2450, Shimadzu Corporation, Kyoto, Japan) (Wu et al., 2020). The results were expressed as mg glucose g−1⋅h−1. Soil urease activity was determined by indophenol blue colorimetry and expressed as mg of NH3-N in 5 g air-dried soil after incubating for 24 h at 37°C with 20 mL of citrate buffer at pH 6.7, 10 mL of 10% urea, and 0.1 mL of toluene (Xie et al., 2017). Soil catalase activity was determined by the KMnO4 liquid titration method and expressed as the volume of 0.02 mol·L−1 KMnO4 consumed of 2 g air-dried soil within 20 min (Li et al., 2014).
2.5 Statistical analysis
The relationships between soil microbial biomass, enzyme activities and soil physical–chemical properties were analyzed using Spearman’s correlation method (SPSS 27.0). Correlations among soil physical–chemical properties, enzyme activities, and microbial biomass were assessed using Person correlation analysis. One-way ANOVA was used to investigate the data for the different water stress treatments (p < 0.05).
3 Results
3.1 Effect of water stress on basic physical–chemical properties of greenhouse grape rhizosphere soil
Compared with CK treatment, the NO3-N content of W1 and W2 treatments decreased by 17.99, 16.07, 16.85, and 8.94% at the new shoot elongation and fruit enlargement stage, respectively (Table 3). The soil AP content showed an increasing and then stabilizing trend. In addition, the soil AP content was significantly (p < 0.05) higher in the CK treatment than in the W1 treatment during both shoot elongation and fruit enlargement stage. Thus, the AP content in the soil had specific adaptability to water stress. Moreover, there was no significant difference of TOC content between W1 and W2 treatment (Figure 1A). The soil DOC content showed an increasing trend and then decreasing during the growth period under different treatments, and peaked at the fruit enlargement stage (Aug-15) (Figure 1B). At the same time, compared with CK treatment, the DOC content of W1 treatment in maize growth stage was 19.84% (p < 0.05), 12.60% (p < 0.05) and 8.34% (p < 0.05) lower than that of CK treatment, respectively. Compared with CK treatment, the soil MBC content of W1 and W2 treatments was significantly lower by 5.61% (p < 0.05) and 15.63% (p < 0.05) at the coloring maturity stage (Oct-15), respectively (Figure 1C). With the increase duration of water stress, MBN was significantly lower in the W1 and W2 treatments than in the CK treatment (p < 0.05) when the coloring maturity stage was reached (Figure 1D).
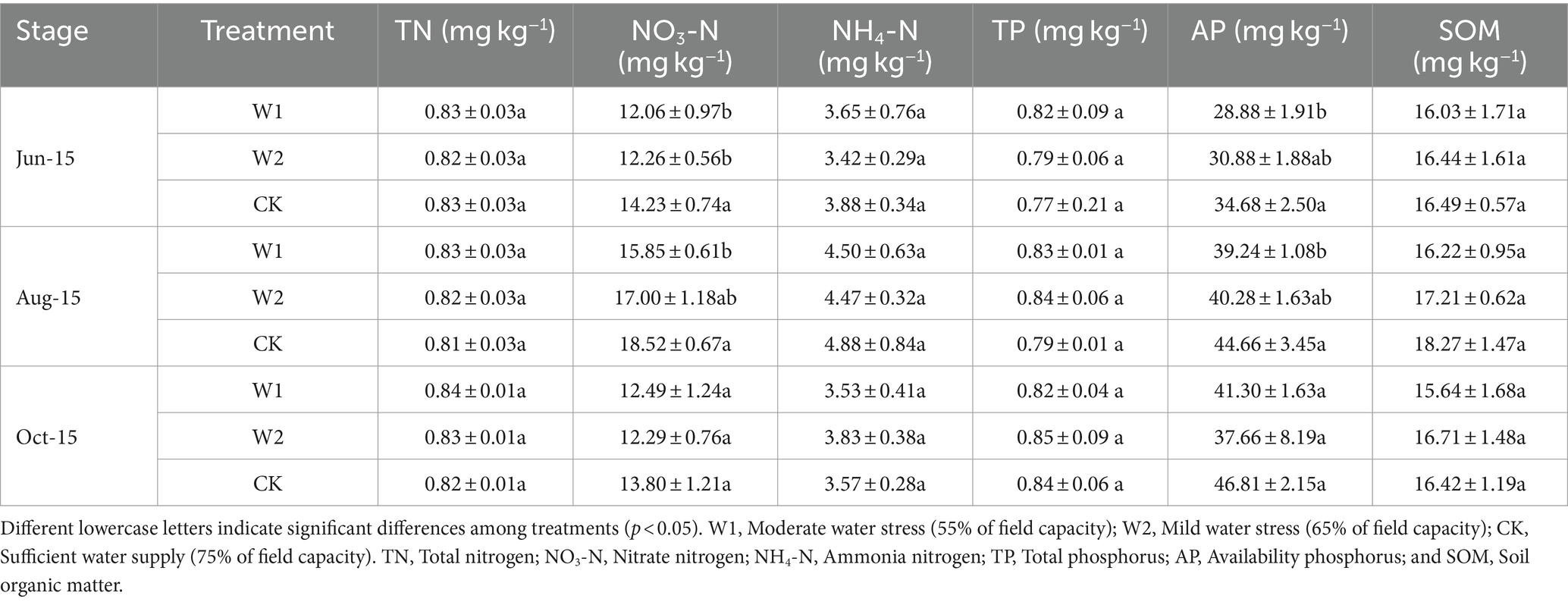
Table 3. Changes in basic physical–chemical properties (mean ± standard deviation, n = 3) of soil under different water stresses.
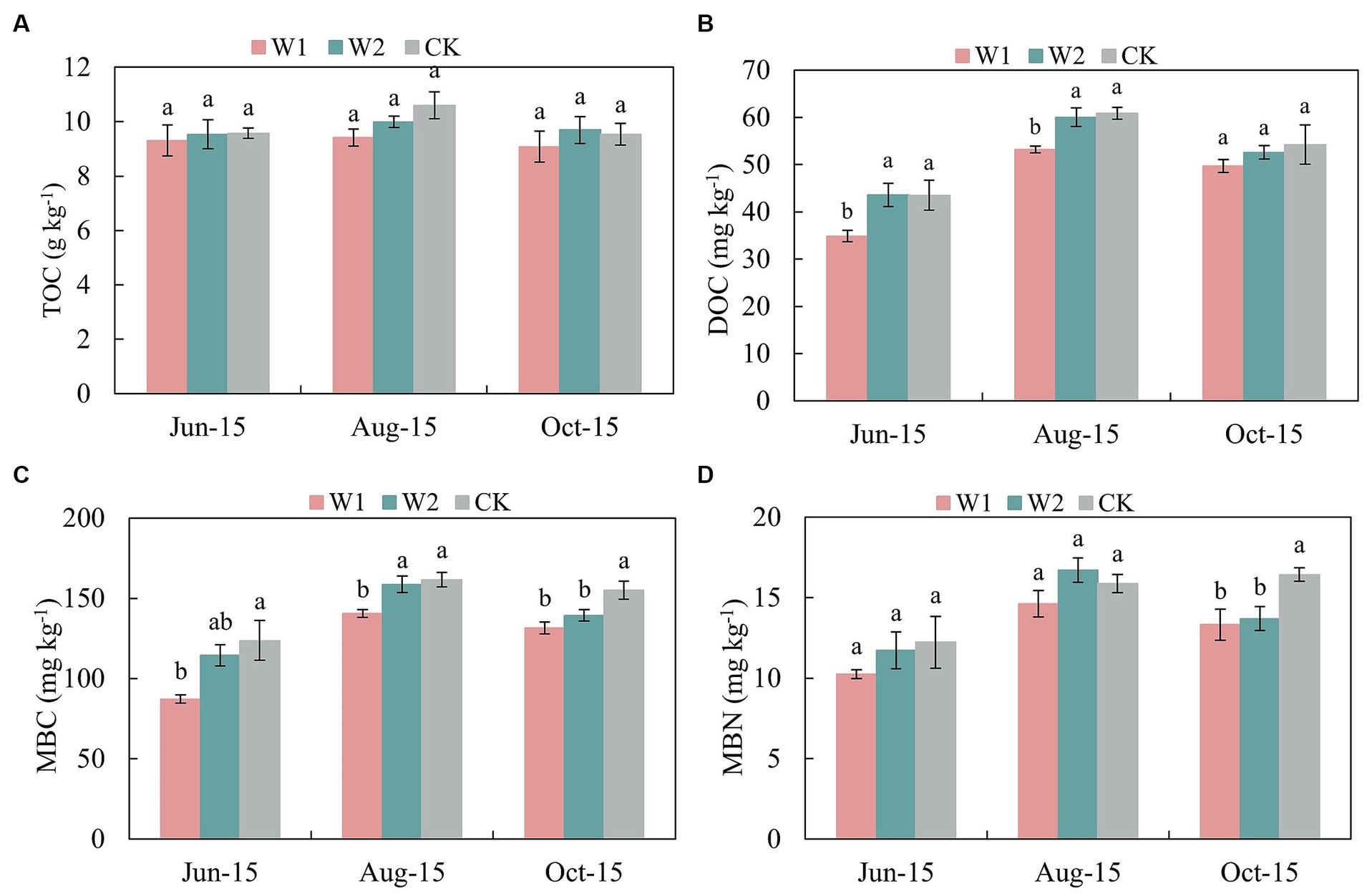
Figure 1. Effects of water stress on the TOC, DOC, MBC, and MBN content of greenhouse grape rhizosphere soil. Error bars indicate standard errors of the mean (n = 3). Different lowercase letters indicate significant differences among treatments (p < 0.05). W1, Moderate water stress (55% of field capacity); W2, Mild water stress (65% of field capacity); CK, Sufficient water supply (75% of field capacity). TOC, Total organic carbon; DOC, Dissolved organic carbon; MBC, Microbial biomass carbon; and MBN, Microbial biomass nitrogen. (A) is TOC content; (B) is DOC content; (C) is MBC content; (D) is MBN content.
3.2 Effects of water stress on soil enzyme activities in greenhouse grape rhizosphere soil
Throughout the grape growing period, urease, catalase, and sucrase activities in different soil layers were ranked as: 10–20 cm > 0–10 cm > 20–40 cm (Table 4). Compared with the CK treatment, the urease activity in the 0–10 cm soil layer of W2 and W2 treatments was significantly lower by 27.40% (p < 0.05), 26.03% (p < 0.05), 20.69% (p < 0.05), and 44.87% (p < 0.05) at the new shoot elongation stage (Jun-15) and flowering stage (Jul-20), respectively. The urease activity in the 10–20 and 20–40 cm soil layers were significantly higher in the CK treatment than in the W1 treatment at the coloring maturity stage (Oct-15) (p < 0.05). The peroxidase activity of all soil layers of grapes decreased significantly during the period from budburst stage (May-15) to fruit enlargement (Aug-15). Compared with CK treatment, the peroxidase activity of W1 treatment in different soil layers (0–10, 10–20, and 20–40 cm) was significantly decreased by 3.65% (p < 0.05), 1.09% (p < 0.05), and 8.89% (p < 0.05) at budburst stage (May-15), respectively. With the increase of stress duration, the surface soil invertase activity of W1 treatment was significantly lower than that of CK treatment by 32.07% (p < 0.05) and 42.73% (p < 0.05) at the fruit enlargement stage (Aug-15) and coloring maturity stage (Oct-15), respectively. Furthermore, the conversion enzyme activity in the 20–40 cm soil layer under the W2 treatment was slightly higher than that in the W1 treatment during the period from the new shoot elongation stage (Jun-15) to coloring maturity stage (Oct-15). This result indicated that both W2 and W1 treatments inhibited soil urease, catalase, and sucrase activity convertase during the greenhouse grape growth.
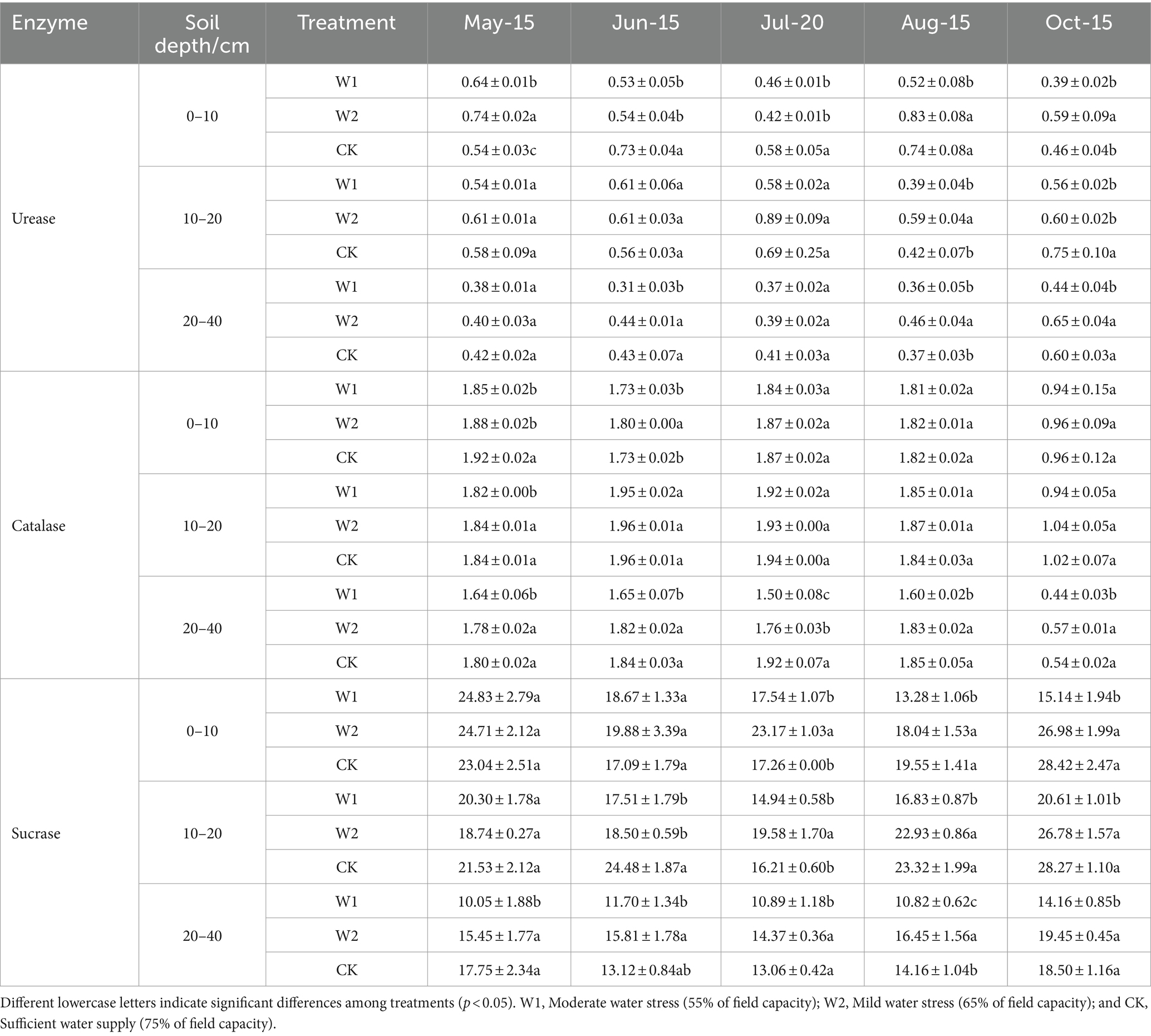
Table 4. Effect of water stress on urease, catalase, and sucrase enzyme activity (mean ± standard deviation, n = 3) in the rhizosphere soil of delayed-cultivation greenhouse grapes (mg·g−1·day−1).
3.3 Correlation analysis between soil physical–chemical and soil enzymes
The correlations between soil microbial biomass and basic soil physical–chemical indicators differed considerably between different growth periods of greenhouse grape rhizospheres (Figure 2). Among them, MBC and MBN showed significantly negative correlations (r = −0.67 and-0.78) with soil TP at the new growth stage (Jun-15) (p < 0.05), but significant positive correlations with soil AP and DOC (p < 0.05). In contrast, there was a highly significant positive correlation between MBC and DOC in the fruit enlargement stage (Aug-15) and coloring maturity stage (Oct-15). The correlation analysis between soil physical–chemical indicators and soil enzyme activities (Figure 3) showed that there were significant positive correlations between soil urease, catalase, and sucrase to varying degrees during the new growth stage (Jun-15), fruit enlargement stage (Aug-15), and coloring maturity stage (Oct-15). There were significant positive correlations between soil urease (Jun-15) and catalase (Aug-15) and nitrate nitrogen (r = 0.81, 0.70) (p < 0.05). Meanwhile, catalase showed a significant positive correlation between organic matter and TOC during the fruit enlargement stage (Aug-15). Soil enzyme activity was positively correlated with DOC, MBC, and MBN at different stages.
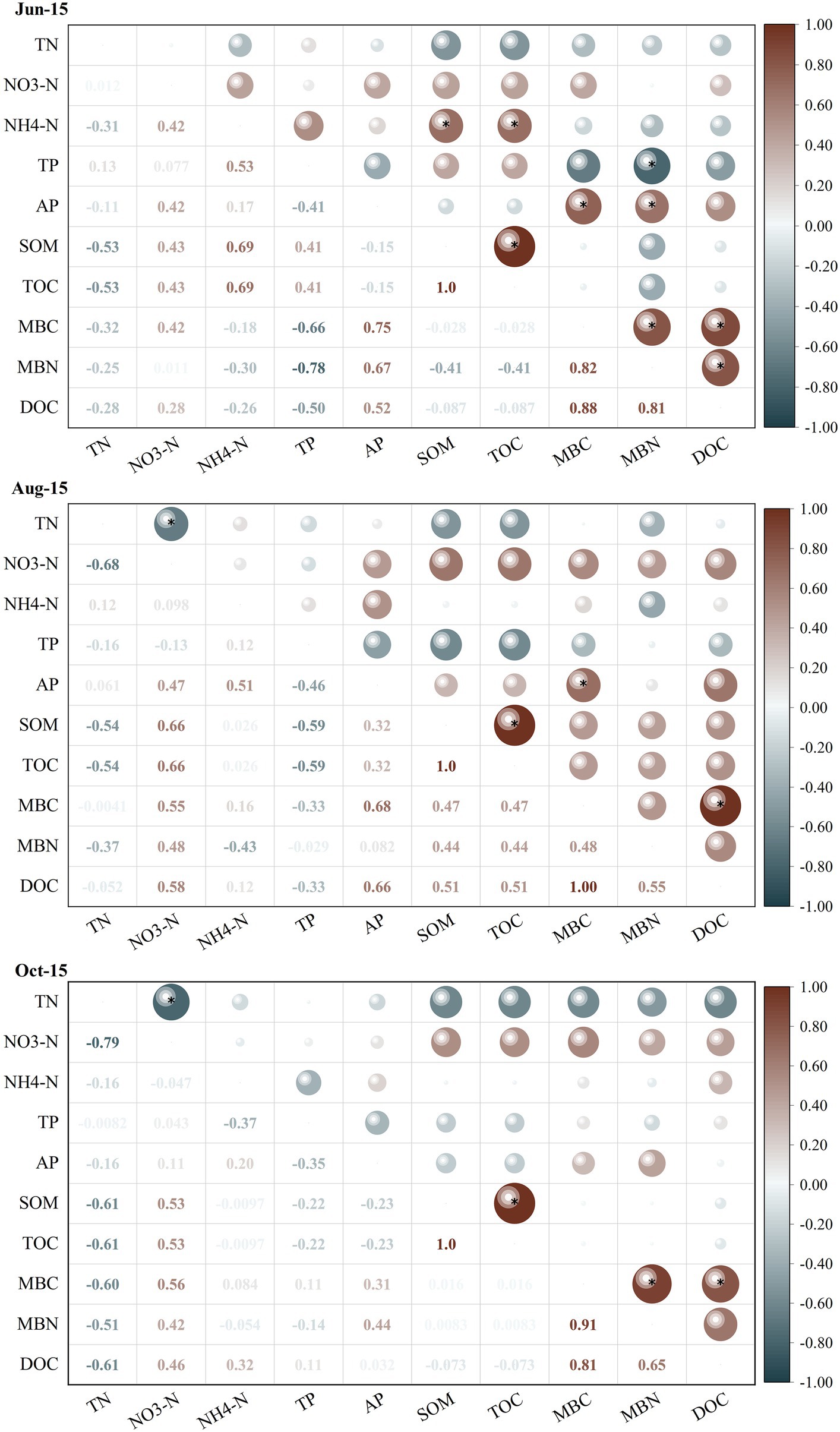
Figure 2. Correlation between soil physical–chemical indicators and microbial biomass indicators. *Significantly correlated at the 0.05 level (two sides). TN, Total nitrogen; NO3-N, Nitrate nitrogen; NH4-N, Ammonia nitrogen; TP, Total phosphorus; AP, Availability phosphorus; SOM Soil organic matter; TOC, Total organic carbon; MBC, Microbial biomass carbon; MBC, Microbial biomass nitrogen; and DOC, Dissolved organic carbon.
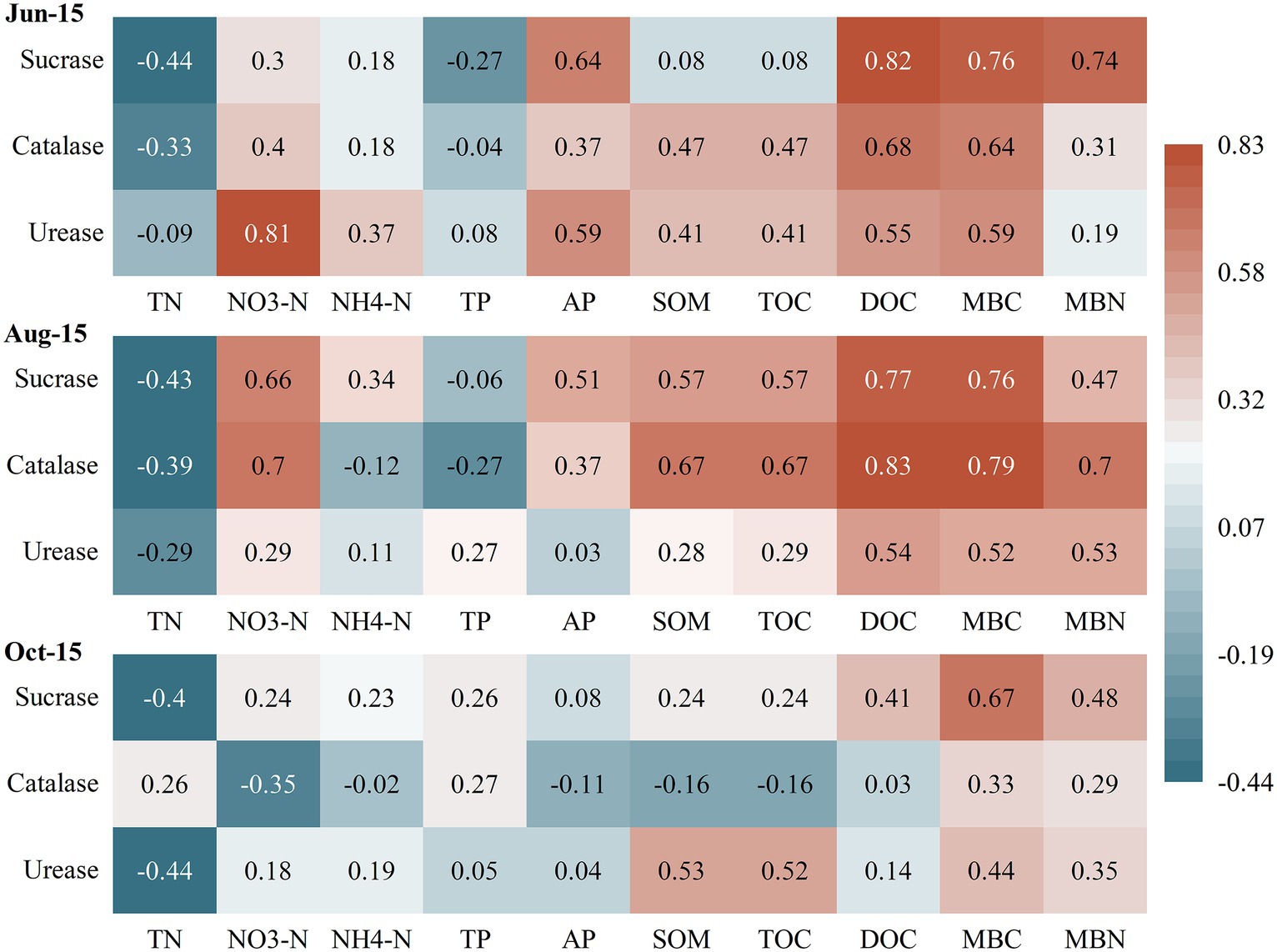
Figure 3. Correlation between soil physical–chemical indicators and soil enzymes. *Significantly correlated at the 0.05 level (two sides). TN, Total nitrogen; NO3-N, Nitrate nitrogen; NH4-N, Ammonia nitrogen; TP, Total phosphorus; AP, Availability phosphorus; SOM, Soil organic matter; TOC, Total organic carbon; MBC, Microbial biomass carbon; MBC, Microbial biomass nitrogen; and DOC, Dissolved organic carbon.
4 Discussion
The results of this study indicated that TN, NH4-N, and TP content in grape rhizosphere soil had the similar response to water stress, without the significant difference among three treatments (moderate and mild water stress and adequate water supply). The results were consistent with previous finding that soil TN and TP contents were not sensitive under different water stress (Wu et al., 2012; Bu et al., 2018). In addition, the study also found that the effect of water stress on soil NO3-N and AP contents were closely related to the degree and time of stress because the soil NO3-N and AP contents were significantly lower under moderate (W2) and mild (W1) water stress than with sufficient water (CK) in the new shoot elongation stage (Jun-15) and fruit enlargement (Aug-15) stages. Water stress reduced the vitality of the plant’s root system, which in turn decreased the secretion of all kinds of organic and inorganic substances by the plant’s root system (Draye et al., 2010). On the contrary, in the coloring maturity stage (Oct-15), there was no significant difference in the NO3-N and AP contents of grape rhizosphere soil under continuous water stress (Li et al., 2021). The cause was due to the decrease of nutrient uptake by the crop’s root system as the grapes mature. However, the result showed stronger selective absorption of nitrate nitrogen compared with NH4-N, as well as the promotion of the absorption of AP content in the soil under water stress.
Another important finding was that there was no significant effect on soil TOC content in the rhizosphere of grapes under water stress during the growth period. A possible explanation may be that the accumulation in soil organic carbon pool storage was a relatively long process, and the change in the amount of grape root exudates caused by water stress was not enough to cause an obvious change in the soil organic carbon content. Additionally, the grape rhizosphere exudates were first supplied to rhizosphere soil microorganisms for utilization and reproduction (Song et al., 2012). Therefore, water stress had no significant effect on the soil TOC content; during the growth period had small fluctuations. Meanwhile the soil DOC content significantly reduced. The soil DOC content under moderate water stress was significantly lower than that under mild water stress and for control treatment with the promoted degree of water stress (W2 and W1 treatments) and the prolonged stress time. This finding was contrary to previous results that suggested DOC could increase with the decline of soil moisture (Wang et al., 2017). The results of the present study showed that moderate water stress reduced the DOC content of grape rhizosphere soil, but mild water stress had no significant effect. This finding was also reported by Li et al. (2016). In summary, soil carbon content may be related to a number of factors such as geographical location, field management, and irrigation practices (Zhang R. et al., 2023). However, water stress reduced the soil MBC and MBN contents of the grape rhizosphere soil, which was contrary to previous findings that drought stress increased the MBC content (Mganga et al., 2019). Previous studies also suggested that excessive water stress (Hueso et al., 2012) and long-term water stress also reduced the soil microbial biomass content (Schimel et al., 1999; Wang et al., 2017). This could be explained by the fact that water stress reduced the vitality of plant roots, which in turn caused the reduction of various organic and inorganic substances secreted by plant roots. This finding was contrary to previous results suggesting that water-soluble compounds and mucilage secreted by plant roots under drought stress promoted the production of microbial biomass (Sanaullah et al., 2011).
Soil moisture was an important factor affecting soil enzyme activity in plant rhizosphere (Geisseler et al., 2011; A'Bear et al., 2014). This experimental study showed that the soil urease activity increased during the initial period of water stress and decreased significantly in the mid-stress stage in soil layers of 0–10 and 10–20 cm, which was probably varied in different plant growth period (Song et al., 2012; Zhang et al., 2018). With the increase of stress duration, moderate water stress reduced the soil urease activity in the soil layer of 0–10 cm, and the moderate and mild water stress reduced the soil urease activity in the soil layer of 40 cm during the coloring mature stage. Adequate water supply and mild water stress helped increase the urease activity in the soil layer of 20–40 cm. Another finding was that in terms of spatial distribution, the activity of urease, catalase, and sucrase in greenhouse grape rhizosphere soil in different soil layers followed the order: 10–20 cm > 0–10 cm > 20–40 cm. This result might be explained by the fact that fertilizers (organic fertilizer, farmyard manure, and chemical fertilizer) were sprayed onto the soil trough 20 cm away from the grape roots and then covered with topsoil (Liu et al., 2024). The urease and sucrase activities in rhizosphere soil were basically stable during the whole growth period of grapes. Hence, further studies should be conducted to investigate the growth, yield, and quality of grapes under different water stresses, and to provide a better description of the influence of water regulation on the water-rhizosphere soil–plant system. In addition, mild water stress helped increase the catalase activity in grape rhizosphere soil in the soil layer of 0–10 cm on Jun-15 (new shoot growth stage) and Aug-15 (fruit expansion stage). On May-15 (budburst stage), the soil catalase activity under moderate water stress was significantly lower than that with an adequate water supply and under mild water stress. Soil catalase activity was related to various factors such as soil fertility, texture, pH, aeration, and climatic conditions (Bao et al., 2022). This experimental study found that W2 treatment helped increase the soil sucrase activity in the soil layer of 0–10 cm on July 20 (flowering stage), but it reduced the soil invertase activity from May-15 (budburst stage) to Jun-15 (new shoot growth stage). This was because the soil conditions between the roots may become anaerobic microdomains due to reduced water content, and could lead to the inhibition of sucrase activities because of limited substrate diffusion and oxygen content (Zhang, 2005).
5 Conclusion
This study detected significant differences in rhizosphere soil enzyme activities and microbial biomass of greenhouse grape under water stress (p < 0.05). Moreover, water stress had less effect on soil physical–chemical properties. Compared with the adequate water supply conditions, the water stress (mild W2 and moderate W1) effectively reduced the accumulation of soil MBC content throughout the grape growing season and reduced soil MBN content in later growth. Both W2 (mild water stress) and W1 (moderate water stress) treatments inhibited the activities of urease, catalase, and sucrase activities transforming enzymes in the soil during greenhouse grape growth. These results illustrated that water stress altered both soil microbial structure and function in rhizosphere soil enzyme activities. Overall, this study provides a theoretical basis for water-saving greenhouse grape cultivation and soil environment regulation. Considering the effects of successive years of water stress on soil microbial and rhizosphere enzyme activities in greenhouse grapes needs to be further deepened and broadened. We suggest that future studies should focus more on changes in greenhouse grape rhizosphere soil enzyme activities and microorganisms as a result of multi-year water stress, and should incorporate changes in microbial communities, which play a crucial role in the regulation of soil quality and plant acclimatization.
Data availability statement
The original contributions presented in the study are included in the article/supplementary material, further inquiries can be directed to the corresponding author.
Author contributions
RZ: Funding acquisition, Writing – review & editing. HZ: Data curation, Writing – original draft. CY: Data curation, Writing – review & editing. HL: Investigation, Writing – review & editing. JW: Formal Analysis, Methodology, Writing – review & editing.
Funding
The author(s) declare financial support was received for the research, authorship, and/or publication of this article. This study was supported by the National Natural Science Foundation of China (52169007), the Key R&D Plan of Gansu Province (22YF7GA107), and the Fuxi youth talent training program of Gansu Agricultural University (Gaufx-03Y10) for funding.
Conflict of interest
The authors declare that the research was conducted in the absence of any commercial or financial relationships that could be construed as a potential conflict of interest.
Publisher’s note
All claims expressed in this article are solely those of the authors and do not necessarily represent those of their affiliated organizations, or those of the publisher, the editors and the reviewers. Any product that may be evaluated in this article, or claim that may be made by its manufacturer, is not guaranteed or endorsed by the publisher.
References
A'Bear, A. D., Jones, T. H., Kandeler, E., and Boddy, L. (2014). Interactive effects of temperature and soil moisture on fungal-mediated wood decomposition and extracellular enzyme activity. Soil Biol. Biochem. 70, 151–158. doi: 10.1016/j.soilbio.2013.12.017
Bao, L., Sun, B., Wei, Y., Xu, N., Zhang, S., Gu, L., et al. (2022). Grape cultivar features differentiate the grape rhizosphere microbiota. Plan. Theory 11:1111. doi: 10.3390/plants11091111
Barta, J., Slajsova, P., Tahovska, K., Picek, T., and Santruckova, H. (2014). Different temperature sensitivity and kinetics of soil enzymes indicate seasonal shifts in C, N and P nutrient stoichiometry in acid forest soil. Biogeochemistry 117, 525–537. doi: 10.1007/s10533-013-9898-1
Boell, E. J., and Shen, S. C. (1954). An improved ultramicro Kjeldahl technique. Exp. Cell Res. 7, 147–152. doi: 10.1016/0014-4827(54)90049-X
Bogati, K., and Walczak, M. (2022). The impact of drought stress on soil microbial community, enzyme activities and plants. Agronomy 12:189. doi: 10.3390/agronomy12010189
Bu, X., Gu, X., Zhou, X., Zhang, M., Guo, Z., Zhang, J., et al. (2018). Extreme drought slightly decreased soil labile organic C and N contents and altered microbial community structure in a subtropical evergreen forest. Forest Ecol. Manag. 429, 18–27. doi: 10.1016/j.foreco.2018.06.036
Chrost, R. J. (2014). Microbial enzymes in aquatic environments. Freshwater Sci. 13:978. doi: 10.1007/978-1-4612-3090-8
Draye, X., Kim, Y., Lobet, G., and Javaux, M. (2010). Model-assisted integration of physiological and environmental constraints affecting the dynamic and spatial patterns of root water uptake from soils. J. Exp. Bot. 61, 2145–2155. doi: 10.1093/jxb/erq077
Geisseler, D., Horwath, W. R., and Scow, K. M. (2011). Soil moisture and plant residue addition interact in their effect on extracellular enzyme activity. Pedobiologia 54, 71–78. doi: 10.1016/j.pedobi.2010.10.001
Geng, S. M., Yan, D. H., Zhang, T. X., Weng, B. S., Zhang, Z. B., and Qin, T. L. (2015). Effects of drought stress on agriculture soil. Nat. Hazards 75, 1997–2011. doi: 10.1007/s11069-014-1409-8
Gramss, G., Voigt, K. D., and Kirsche, B. (1999). Oxidoreductase enzymes liberated by plant roots and their effects on soil humic material. Chemosphere 38, 1481–1494. doi: 10.1016/S0045-6535(98)00369-5
Hueso, S., Garcia, C., and Hernandez, T. (2012). Severe drought conditions modify the microbial community structure, size and activity in amended and unamended soils. Soil Biol. Biochem. 50, 167–173. doi: 10.1016/j.soilbio.2012.03.026
Jing, Y., Zhang, Y., Han, I., Wang, P., Mei, Q., and Huang, Y. (2020). Effects of different straw biochars on soil organic carbon, nitrogen, available phosphorus, and enzyme activity in paddy soil. Sci. Rep. 10:8837. doi: 10.1038/s41598-020-65796-2
Kapoor, D., Bhardwaj, S., Landi, M., Sharma, A., Ramakrishnan, M., and Sharma, A. (2020). The impact of drought in plant metabolism: how to exploit tolerance mechanisms to increase crop production. Appl. Sci. 10:5692. doi: 10.3390/app10165692
Kátai, J., Zsuposné, Á. O., Tállai, M., and Alshaal, T. (2020). Would fertilization history render the soil microbial communities and their activities more resistant to rainfall fluctuations? Ecotoxicol. Environ. Saf. 201:110803. doi: 10.1016/j.ecoenv.2020.110803
Kim, Y., Chung, Y. S., Lee, E., Tripathi, P., Heo, S., and Kim, K. (2020). Root response to drought stress in Rice (Oryza sativa L.). Int. J. Mol. Sci. 21:1513. doi: 10.3390/ijms21041513
Li, Q., Liang, J. H., He, Y. Y., Hu, Q. J., and Yu, S. (2014). Effect of land use on soil enzyme activities at karst area in Nanchuan, Chongqing, Southwest China. Plant Soil Environ. 60, 15–20. doi: 10.17221/599/2013-PSE
Li, S., Tan, D., Wu, X., Degré, A., Long, H., Zhang, S., et al. (2021). Negative pressure irrigation increases vegetable water productivity and nitrogen use efficiency by improving soil water and NO3–N distributions. Agric. Water Manag. 251:106853. doi: 10.1016/j.agwat.2021.106853
Li, Z., Zhao, B., and Zhang, J. (2016). Effects of maize residue quality and soil water content on soil labile organic carbon fractions and microbial properties. Pedosphere 26, 829–838. doi: 10.1016/S1002-0160(15)60088-1
Liu, X., and Zhang, S. (2019). Nitrogen addition shapes soil enzyme activity patterns by changing pH rather than the composition of the plant and microbial communities in an alpine meadow soil. Plant Soil 440, 11–24. doi: 10.1007/s11104-019-04054-5
Liu, S., Zhang, P., Wang, X., Hakeem, A., Niu, M., Song, S., et al. (2024). Comparative analysis of different bio-organic fertilizers on growth and rhizosphere environment of grapevine seedlings. Sci. Hortic. 324:112587. doi: 10.1016/j.scienta.2023.112587
Manzoni, S., Schimel, J. P., and Porporato, A. (2012). Responses of soil microbial communities to water stress: results from a meta-analysis. Ecology 93, 930–938. doi: 10.1890/11-0026.1
Marschner, P., Grierson, P. F., and Rengel, Z. (2005). Microbial community composition and functioning in the rhizosphere of three Banksia species in native woodland in Western Australia. Appl. Soil Ecol. 28, 191–201. doi: 10.1016/j.apsoil.2004.09.001
Menichetti, L., Reyes Ortigoza, A. L., García, N., Giagnoni, L., Nannipieri, P., and Renella, G. (2015). Thermal sensitivity of enzyme activity in tropical soils assessed by the Q10 and equilibrium model. Biol. Fertil. Soils 51, 299–310. doi: 10.1007/s00374-014-0976-x
Mganga, K. Z., Razavi, B. S., Sanaullah, M., and Kuzyakov, Y. (2019). Phenological stage, plant biomass, and drought stress affect microbial biomass and enzyme activities in the rhizosphere of Enteropogon macrostachyus. Pedosphere 29, 259–265. doi: 10.1016/S1002-0160(18)60799-X
Padhy, S. R., Nayak, S., Dash, P. K., Das, M., Roy, K. S., Nayak, A. K., et al. (2018). Elevated carbon dioxide and temperature imparted intrinsic drought tolerance in aerobic rice system through enhanced exopolysaccharide production and rhizospheric activation. Agric. Ecosyst. Environ. 268, 52–60. doi: 10.1016/j.agee.2018.08.009
Ru, C., Hu, X., Wang, W., Ran, H., Song, T., and Guo, Y. (2020). Evaluation of the crop water stress index as an indicator for the diagnosis of grapevine water deficiency in greenhouses. Horticulturae 6:86. doi: 10.3390/horticulturae6040086
Schimel, J. P., Gulledge, J. M., Clein-Curley, J. S., Lindstrom, J. E., and Braddock, J. F. (1999). Moisture effects on microbial activity and community structure in decomposing birch litter in the Alaskan taiga. Soil Biol. Biochem. 31, 831–838. doi: 10.1016/S0038-0717(98)00182-5
Sanaullah, M., Blagodatskaya, E., Chabbi, A., Rumpel, C., and Kuzyakov, Y. (2011). Drought effects on microbial biomass and enzyme activities in the rhizosphere of grasses depend on plant community composition. Applied Soil Ecology 48: 38–44. doi: 10.1016/j.apsoil.2011.02.004
Song, F., Han, X., Zhu, X., and Herbert, S. J. (2012). Response to water stress of soil enzymes and root exudates from drought and non-drought tolerant corn hybrids at different growth stages. Can. J. Soil Sci. 92, 501–507. doi: 10.4141/cjss2010-057
Song, R., Zhu, W. Z., and Li, H. (2024). Impact of wine-grape continuous cropping on soil enzyme activity and the composition and function of the soil microbial community in arid areas. Front. Microbiol. 15:1348259. doi: 10.3389/fmicb.2024.1348259
Wang, Y., Yan, D., Wang, J., Ding, Y., and Song, X. (2017). Effects of elevated CO2 and drought on plant physiology, soil carbon and soil enzyme activities. Pedosphere 27, 846–855. doi: 10.1016/S1002-0160(17)60458-2
Wang, Y., Zhang, X., Chen, J., Chen, A., Wang, L., Guo, X., et al. (2019). Reducing basal nitrogen rate to improve maize seedling growth, water and nitrogen use efficiencies under drought stress by optimizing root morphology and distribution. Agric. Water Manag. 212, 328–337. doi: 10.1016/j.agwat.2018.09.010
Wu, F., Bao, W., Zhou, Z., and Li, F. (2012). Appropriate nitrogen supply could improve soil microbial and chemical characteristics with Sophora davidii seedlings cultivated in water stress conditions. Acta Agric. Scand. 62, 49–58. doi: 10.1080/09064710.2011.568515
Wu, J., Wang, H., Li, G., Ma, W., Wu, J., Gong, Y., et al. (2020). Vegetation degradation impacts soil nutrients and enzyme activities in wet meadow on the Qinghai-Tibet plateau. Sci. Rep. 10:21271. doi: 10.1038/s41598-020-78182-9
Xiao, L., Min, X., Liu, G., Li, P., and Xue, S. (2023). Effect of plant–plant interactions and drought stress on the response of soil nutrient contents, enzyme activities and microbial metabolic limitations. Appl. Soil Ecol. 181:104666. doi: 10.1016/j.apsoil.2022.104666
Xie, X., Pu, L., Wang, Q., Zhu, M., Xu, Y., and Zhang, M. (2017). Response of soil physicochemical properties and enzyme activities to long-term reclamation of coastal saline soil, Eastern China. Sci. Total Environ. 607-608, 1419–1427. doi: 10.1016/j.scitotenv.2017.05.185
Xu, N., Tan, G., Wang, H., and Gai, X. (2016). Effect of biochar additions to soil on nitrogen leaching, microbial biomass and bacterial community structure. Eur. J. Soil Biol. 74, 1–8. doi: 10.1016/j.ejsobi.2016.02.004
Xue, R., Shen, Y. Y., and Marschner, P. (2017). Soil water content during and after plant growth influence nutrient availability and microbial biomass. J. Soil Sci. Plant Nutr. 17, 702–715. doi: 10.4067/s0718-95162017000300012
Yang, N., Nesme, J., Røder, H. L., Li, X., Zuo, Z., and Petersen, M. (2021). Emergent bacterial community properties induce enhanced drought tolerance in Arabidopsis. NPJ Biofilms Microbiomes 7:82. doi: 10.1038/s41522-021-00253-0
Yang, H., Wu, F., Fang, H., Hu, J., and Hou, Z. (2019). Mechanism of soil environmental regulation by aerated drip irrigation. Acta Phys. Sin. 68:019201. doi: 10.7498/aps.68.20181357
Zhang, K. (2005). Forest litter decomposition is affected by different sources of soil and plant growth. China Agricultural University, Beijing. Available at: https://kns.cnki.net/kcms2/article/abstract?v=sf24_f5fySZ3gys5wcV9Fejlhr5HYn2N_vIFKizFL3ADTh1MiUN8UL56URf57GMPz3YsiaaW9cY2ln92bEffXcixiLSPa5DVBviS7QvngpFpNPBxC745bRKkPwYGzHhbjIi9MNVPdCV7X4Qhftvbyw=&uniplatform=NZKPT&language=CHS
Zhang, R., Chen, L., Niu, Z., Song, S., and Zhao, Y. (2019). Water stress affects the frequency of Firmicutes, Clostridiales and Lysobacter in rhizosphere soils of greenhouse grape. Agric. Water Manag. 226:105776. doi: 10.1016/j.agwat.2019.105776
Zhang, C., Nie, S., Liang, J., Zeng, G., Wu, H., Hua, S., et al. (2016). Effects of heavy metals and soil physicochemical properties on wetland soil microbial biomass and bacterial community structure. Sci. Total Environ. 557-558, 785–790. doi: 10.1016/j.scitotenv.2016.01.170
Zhang, C., Wang, F., Wang, R., Wang, Z., Song, P., Guo, L., et al. (2023). Drought stress affects bacterial community structure in the rhizosphere of Paeonia ostii ‘Feng Dan’. J. Hortic. Sci. Biotechnol. 98, 109–120. doi: 10.1080/14620316.2022.2095311
Zhang, R., Wu, J., Yang, C., Li, H., Lin, B., Gao, Y., et al. (2023). Response of water stress and bacterial fertilizer addition to the structure of microbial flora in the rhizosphere soil of grapes under delayed cultivation. Commun. Soil Sci. Plant Anal. 54, 2609–2624. doi: 10.1080/00103624.2023.2234947
Zhang, W., Zhu, J., Zhou, X., and Li, F. (2018). Effects of shallow groundwater table and fertilization level on soil physico-chemical properties, enzyme activities, and winter wheat yield. Agric. Water Manag. 208, 307–317. doi: 10.1016/j.agwat.2018.06.039
Zhao, Y., Mao, W., Pang, L., Li, R., and Li, S. (2020). Influence of Phragmites communis and Zizania aquatica on rhizosphere soil enzyme activity and bacterial community structure in a surface flow constructed wetland treating secondary domestic effluent in China. Environ. Sci. Pollut. R. 27, 26141–26152. doi: 10.1007/s11356-020-08904-z
Keywords: water stress, physical–chemical properties, microbial biomass, enzyme activity, greenhouse grape
Citation: Zhang R, Zhang H, Yang C, Li H and Wu J (2024) Effects of water stress on nutrients and enzyme activity in rhizosphere soils of greenhouse grape. Front. Microbiol. 15:1376849. doi: 10.3389/fmicb.2024.1376849
Edited by:
Huai Li, Chinese Academy of Sciences (CAS), ChinaReviewed by:
Jiuling Li, The University of Queensland, AustraliaYafei Zhang, Jiangsu University, China
Copyright © 2024 Zhang, Zhang, Yang, Li and Wu. This is an open-access article distributed under the terms of the Creative Commons Attribution License (CC BY). The use, distribution or reproduction in other forums is permitted, provided the original author(s) and the copyright owner(s) are credited and that the original publication in this journal is cited, in accordance with accepted academic practice. No use, distribution or reproduction is permitted which does not comply with these terms.
*Correspondence: Jiangqi Wu, amlhbmdxaXc2MjM2QGZveG1haWwuY29t