- 1Laboratory of Internal Medicine, Cooperative Division of Veterinary Sciences, Tokyo University of Agriculture and Technology, Fuchu, Japan
- 2Department of Internal Medicine, Faculty of Veterinary Medicine, Damanhour University, Damanhour, Egypt
- 3Department of Biochemistry, Faculty of Veterinary Medicine, Damanhour University, Damanhour, Egypt
- 4Department of Biology, College of Science, Princess Nourah Bint Abdulrahman University, Riyadh, Saudi Arabia
- 5State Key Laboratory of Animal Biotech Breeding, Institute of Animal Science, Chinese Academy of Agricultural Science, Beijing, China
- 6PhD Program in Sciences Mentioning Applied Molecular and Cell Biology, La Frontera University, Temuco, Chile
- 7Laboratory of Engineering, Biotechnology and Applied Biochemistry – LIBBA, Department of Chemical Engineering, Faculty of Engineering and Science, La Frontera University, Temuco, Chile
- 8Department of Biological and Chemical Sciences, Faculty of Natural Resources, Catholic University of Temuco, Temuco, Chile
- 9Department of Surgery, Anesthesiology, and Radiology, Faculty of Veterinary Medicine, Zagazig University, Zagazig, Egypt
- 10Department of Animal and Poultry Production, Faculty of Agriculture, Damanhour University, Damanhour, Egypt
- 11Nucleus of Environmental Sciences, Faculty of Natural Resources, Catholic University of Temuco, Temuco, Chile
- 12Department of Pharmacology, Faculty of Veterinary Medicine, Benha University, Moshtohor, Toukh, Qaliobiya, Egypt
- 13Department of Clinical Microbiology and Immunology, Faculty of Medicine, King Abdulaziz University, Jeddah, Saudi Arabia
- 14Department of Medical Microbiology and Immunology, Faculty of Medicine, Suez Canal University, Ismailia, Egypt
Pseudomonas aeruginosa, a Gram-negative bacterium, is recognized for its adaptability and opportunistic nature. It poses a substantial challenge in clinical settings due to its complicated antibiotic resistance mechanisms, biofilm formation, and capacity for persistent infections in both animal and human hosts. Recent studies revealed a potential zoonotic transmission of P. aeruginosa between animals, the environment, and human populations which highlights awareness of this microbe. Implementation of the One Health approach, which underscores the connection between human, animal, and environmental health, we aim to offer a comprehensive perspective on the current landscape of P. aeruginosa management. This review presents innovative strategies designed to counteract P. aeruginosa infections. Traditional antibiotics, while effective in many cases, are increasingly compromised by the development of multidrug-resistant strains. Non-antibiotic avenues, such as quorum sensing inhibition, phage therapy, and nanoparticle-based treatments, are emerging as promising alternatives. However, their clinical application encounters obstacles like cost, side effects, and safety concerns. Effectively addressing P. aeruginosa infections necessitates persistent research efforts, advancements in clinical development, and a comprehension of host-pathogen interactions to deal with this resilient pathogen.
1 Introduction
Pseudomonas aeruginosa, a Gram-negative bacterium of paramount significance, resides predominantly within healthcare settings, where it assumes a pivotal role as a causative agent of nosocomial infections (Moradali et al., 2017; Reynolds and Kollef, 2021). Simultaneously, it exerts its pathogenicity through opportunistic infections in diverse hosts, including humans, animals, and plants. The challenge of treatment of P. aeruginosa is always due to its ability to survive under a spectrum of environmental conditions and its resistance to available antimicrobial agents (Laborda et al., 2021). A distinctive hallmark of P. aeruginosa is its propensity for biofilm formation, an intricate process wherein bacterial cells aggregate within a self-produced extracellular matrix. This biofilm formation, encompassing a complex architectural arrangement, renders the bacterium impervious to conventional antibiotic regimens and contributes significantly to its clinical impact (Sharma et al., 2023). In a paradoxical twist, these biofilm structures have been observed to enhance the bacterium’s vulnerability when exposed to certain stressors such as antimicrobial agents, ultraviolet (UV) light, and variations in salinity (Roy et al., 2018; Sharma et al., 2019).
The prevalent presence of P. aeruginosa in nosocomial settings is not limited to its role as a colonizer of biotic and abiotic surfaces but extends to its capacity to form biofilms on medical devices, implants, and instrumentation, thereby increasing the risk of infections in hospitalized patients (Muhammad et al., 2020). Moreover, its ability to develop multidrug resistance (MDR) is a compelling facet of concern, as it withstands a broad spectrum of antibiotic classes including aminoglycosides (amikacin, gentamicin, and tobramycin), fluoroquinolones (ciprofloxacin, ofloxacin, and norfloxacin), carbapenems, and tetracyclines (Avakh et al., 2023).
Beyond its clinical impact, P. aeruginosa extends its dominion into the realm of environmental microbiology. A ubiquitous environmental bacterium, it traverses various ecological niches with remarkable adaptability, aided by a genome characterized by plasticity and high nutritional versatility (Grosso-Becerra et al., 2014). Within this genetic diversity lies an extensive range of intrinsic and acquired resistance mechanisms that collectively contribute to the bacterium’s impressive ability to circumvent antimicrobial agents. Understanding the complexity of resistance mechanisms and their interplay within the P. aeruginosa population is paramount for the development of targeted therapeutic interventions, particularly in the context of MDR strains. Our exploration into the world of P. aeruginosa will illuminate the adaptive strategies that cause its clinical and environmental persistence (Langendonk et al., 2021). In this pursuit of knowledge, our mission is to organize a comprehensive summary of the current epidemiological situation related to Pseudomonas aeruginosa, investigate the widespread issue of antimicrobial resistance and its underlying mechanisms, and establish a pathway for the discovery of alternative antimicrobial agents targeting this bacterium.
2 Clinical infections of Pseudomonas aeruginosa
P. aeruginosa, initially identified in 1882 through Gessard’s work involving green pus, exhibits a versatile lifestyle that enables it to contribute to frequent infections in humans (Lyczak et al., 2000). While it can be part of the normal intestinal flora, it does not readily adhere to healthy intact epithelium and typically does not cause infections in individuals in good health. However, it becomes an opportunistic pathogen in immunocompromised patients. Due to its adaptability and robust survivability, it can persist on dry, inanimate surfaces within hospital environments for a long period (Muggeo et al., 2023). It often contaminates healthcare equipment and various surfaces such as monitors, ventilator controls, bed rails, and respiratory devices. P. aeruginosa is implicated in a spectrum of hospital-acquired infections, spanning from ventilator-associated pneumonia (VAP) to bloodstream infections (Figure 1). It stands as the fourth most frequently isolated nosocomial pathogen, contributing to 10% of all hospital-acquired infections (Caffrey et al., 2022). A recent analysis of 8,247 inpatients with nosocomial infections across various tertiary care hospitals in Greece identified 746 patients with active healthcare-associated infections. P. aeruginosa emerged as the second most frequently isolated bacterium, accounting for 16% of cases, and was responsible for 49.4% of resistance against the carbapenem group of antibiotics (Kritsotakis et al., 2017). Notably, P. aeruginosa has been linked to higher mortality rates in bloodstream infections compared to infections caused by other Gram-positive and Gram-negative organisms.
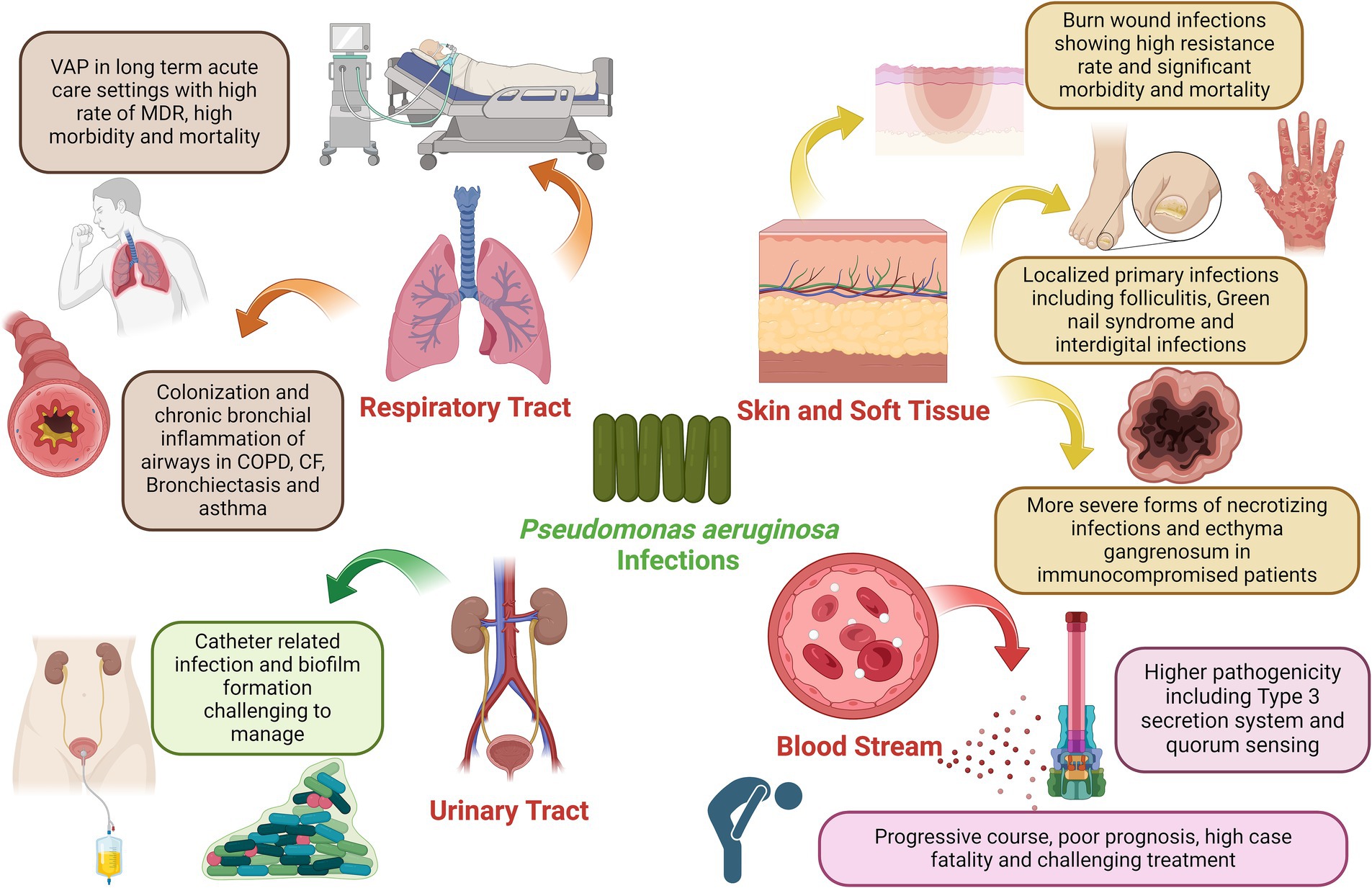
Figure 1. Clinical impact of P. aeruginosa infection in various organs of the human body, the figure was created with biorender.
2.1 Pseudomonas aeruginosa and respiratory infections
Pseudomonas aeruginosa is a prominent pathogen in healthcare settings, particularly among immunocompromised patients. It poses a significant threat as it can be transmitted through medical equipment and cross-contamination among patients (Wood et al., 2023). Ventilator-associated pneumonia (VAP) is a serious complication in patients kept on mechanical ventilation for more than 48 h in which P. aeruginosa is responsible for a high proportion of these infections in hospitalized patients. In the context of VAP, P. aeruginosa’s involvement is associated with higher case fatality rates than other bacterial causes. Tracheobronchial colonization is a critical factor in VAP, with Staphylococcus aureus, P. aeruginosa, and Enterobacteriaceae being the primary pathogens. High quantities of P. aeruginosa in ventilated patients’ tracheas correlate with an increased risk of death. The bacterium’s ability to resist conventional antibiotics, often due to complex drug-resistance genes present in plasmids or integrated into the bacterial genome, poses a significant challenge (Pachori et al., 2019). Studies have revealed that Pseudomonas spp. account for about 3–5% of nosocomial pneumonia cases, with P. aeruginosa being a prominent strain in ventilator-associated cases (Leroy et al., 2005). Nosocomial pneumonia secondary to P. aeruginosa has worse clinical outcomes when compared to other typical organisms. The prevalence of MDR P. aeruginosa VAP is reported to be generally high (33%) (Li et al., 2024). The crude mortality rate for P. aeruginosa VAP has been estimated at 42.1–87% even in patients receiving appropriate antimicrobial therapy (Foucrier et al., 2023). In addition to its impact on VAP, P. aeruginosa is among the top microorganisms responsible for healthcare respiratory infections. Infections caused by MDR-P. aeruginosa strains significantly increase the risk of mortality. Risk factors for VAP with P. aeruginosa include prior antibiotic exposure, airway colonization, and mechanical ventilation exceeding five days. On the other hand, P. aeruginosa is capable of chronic colonization, persistence, and infection of lower respiratory tracts in patients with chronic inflammatory airway diseases including asthma, chronic obstructive pulmonary disease (COPD), cystic fibrosis (CF), and bronchiectasis (Garcia-Clemente et al., 2020). Patients with chronic diseases like obstructive pulmonary disease and other respiratory conditions are also susceptible to the selection of hypermutator P. aeruginosa strains in chronic respiratory tract infections by P. aeruginosa facilitated by the frequent use of antimicrobials in these cases. Additionally, what makes P. aeruginosa particularly challenging is its ability to resist treatment, especially in the context of cystic fibrosis (CF) (Abdulwahab et al., 2017). In this hereditary disease, P. aeruginosa becomes a predominant pathogen in the lungs of affected individuals. Despite efforts to eradicate it, P. aeruginosa often persists, driving disease progression. It’s critical to understand how P. aeruginosa adapts and survives within the hyperinflammatory environment of the CF lung to develop effective treatments. Chronic bronchial infections in persons with CF, bronchiectasis, and COPD have been linked to more severe disease and poor prognosis. Bacterial colonization by P. aeruginosa has also been documented in people with chronic severe long-standing asthma with frequent exacerbations contributing to unfavorable clinical outcomes (Zhang et al., 2012).
P. aeruginosa presents multiple virulent factors and adapts through genotypic and phenotypic alterations to evade antibiotics and the immune system. The chronic infection environment within the CF lung subjects P. aeruginosa to significant stressors, including competition for resources, anaerobic conditions, high antibiotic concentrations, and immune responses like neutrophil attacks (Jurado-Martín et al., 2021). In response, P. aeruginosa undergoes microevolution, acquiring spontaneous mutations that lead to the selection of variants better suited for long-term colonization. These variants exhibit reduced virulence but greater resistance to antibiotics and host immunity. This adaptation includes the downregulation of virulence factors, increased biofilm formation, and upregulation of exopolysaccharides (Sousa and Pereira, 2014). These changes help P. aeruginosa avoid immune recognition, phagocytosis, and proinflammatory responses, promoting its persistence in the host. Moreover, P. aeruginosa forms biofilms within the CF lung, which significantly enhances its resistance to antibiotics and immune effectors. Biofilm communities appear as microcolonies or aggregates closely associated with the airway epithelium. They can evade the complement system, immobilize neutrophils, and resist the actions of neutrophil extracellular traps, further enabling P. aeruginosa to thrive within the CF lung (Maurice et al., 2018).
2.2 Pseudomonas aeruginosa and skin infections
Pseudomonas aeruginosa is a formidable pathogen with increasing clinical significance, particularly concerning skin and soft tissue infections. Its impact becomes far more pronounced in hospitalized patients due to its high resistance to a wide array of commonly available anti-pseudomonal agents (Wang et al., 2019). P. aeruginosa plays a critical role in burn wound infections, accounting for approximately one-third of all burn-related infections. These infections are notorious for their resistance to treatment, despite the availability of newer antibiotics with broad-spectrum activity (Gonzalez et al., 2016). Burned patients are particularly vulnerable, and infections caused by P. aeruginosa can lead to severe morbidity and, in some cases, mortality. The resistant nature of these infections not only prolongs hospital stays but also escalates treatment costs, representing a significant burden on healthcare systems. In skin infections, P. aeruginosa can cause a range of infections, from mild to severe, with unique clinical presentations. These infections typically affect otherwise healthy individuals and may sometimes resolve spontaneously without specific antibacterial therapy. Skin manifestations associated with P. aeruginosa infections may range from less severe localized forms of primary skin infections including Green Nail Syndrome, Interdigital Infections, and hot tub Folliculitis to more severe forms occurring in immunocompromised patients such as Ecthyma Gangrenosum and subcutaneous nodules usually occurring with bloodstream infections and also necrotizing skin and soft tissue infections occurring in diabetic, alcoholic and immunocompromised patients (Spernovasilis et al., 2021). P. aeruginosa plays an important role as a pathogen that extends beyond human infections; it also poses a significant threat to animals, including pets and livestock, with skin infections being a notable concern (Singh et al., 2005). In animals, P. aeruginosa can cause a range of dermatological conditions, including wound infections, hot spots, and otitis infections (de Sousa et al., 2023; Elfadadny et al., 2023b). These infections can lead to discomfort, pain, and, in severe cases, systemic illness. Veterinarians often encounter challenges in treating P. aeruginosa infections due to their ability to develop resistance to antibiotics, mirroring the issues seen in human medicine (Nielsen et al., 2022). The increasing trends of antibiotic resistance observed in this bacterium severely limit the choice of effective antimicrobial agents. Studies have demonstrated rising resistance rates of P. aeruginosa to numerous antibiotics, including those commonly used to combat skin and soft tissue infections.
2.3 Pseudomonas aeruginosa and ear infections
Pseudomonas aeruginosa can also invade the ears of both humans and animals. In humans, P. aeruginosa ear infections can lead to a painful condition known as swimmer’s ear or otitis externa. This infection often occurs after exposure to contaminated water, such as in swimming pools or hot tubs (Mittal et al., 2016). P. aeruginosa live in these moist environments, causing inflammation, itching, discharge, and sometimes even hearing difficulties or complicated to otitis media and malignant external otitis if left untreated. Treatment typically involves antibiotic ear drops, but the bacterium’s ability to develop resistance can complicate the therapeutic process (Sun et al., 2021). Malignant otitis externa is a serious invasive life-threatening condition that affects the external ear and skull base and can be complicated by mastoiditis and cranial nerve palsy if left untreated. It classically occurs in elderly, immunocompromised, and diabetic patients (Wu et al., 2011). Similarly, in animals, particularly dogs, cats, and other domestic pets, P. aeruginosa ear infections are a concerning issue. These infections can stem from various factors, including allergies, foreign objects in the ear canal, or underlying health conditions. P. aeruginosa’s adaptability and antibiotic resistance underscores the importance of prompt diagnosis and appropriate treatment to prevent further complications in both human and animal ear infections.
2.4 Pseudomonas aeruginosa and urinary tract infections
Pseudomonas aeruginosa has been increasingly implicated in UTIs. These infections represent a significant clinical concern, particularly in healthcare environments where indwelling urinary catheters are prevalent (Newman et al., 2022). Catheter-associated UTIs by P. aeruginosa are of substantial clinical consequence due to their association with pyelonephritis, prolonged morbidity, and mortality. P. aeruginosa contributes to approximately 10% of catheter-associated UTIs (CAUTI) and up to 16% of UTIs in ICU patients, with an alarming increase in nosocomial-acquired infections (Rosenthal et al., 2016; Farzin et al., 2023). The bacterium’s ability to exploit catheters as a tool for host entry underscores its clinical significance in UTIs. The insertion of urinary catheters can disturb mucosal epithelial layers, thereby promoting bacterial colonization and subsequent infection. Consequently, P. aeruginosa emerges as a dominant uropathogen in this clinical context. P. aeruginosa encompasses cell-associated factors such as alginate, lipopolysaccharides (LPS), flagella, and pili, alongside secretory virulence factors like proteases, elastases, toxins, and hemolysins (Mekonnen et al., 2022). Biofilm formation represents a hallmark of P. aeruginosa’s strategy to establish and persist in UTIs. The bacterium’s innate tendency to adhere to catheter surfaces leads to robust biofilm development. These biofilms present exceptional resistance against antimicrobial agents and host immune defenses. The ability of biofilms to promote persistent and recurrent infections is a pressing clinical concern in UTIs which renders P. aeruginosa infections challenging to manage (Mekonnen et al., 2022). Additionally, the ability of P. aeruginosa to invade the bladder epithelium has been recently demonstrated. Extensive evidence exists about the ability of P. aeruginosa to preferentially bind to, invade, and injure wounded epithelium. This ability to invade vulnerable epithelial cells has been recently proven to correlate well with the high prevalence of P. aeruginosa UTI in hospitalized elderly populations (Newman et al., 2022).
2.5 Pseudomonas aeruginosa and blood infections
Bloodstream infections (BSIs) caused by P. aeruginosa are emerging as a significant concern in clinical settings. P. aeruginosa is responsible for a significant number of bacteremia, ranking third, after Escherichia coli and Klebsiella species, among Gram-negative bacteria isolated during nosocomial bloodstream infections, and seventh among all pathogens (Holmes et al., 2021). Its significance arises from its place in case of fatality among all pathogens. Bacteremia caused by P. aeruginosa carries a poor prognosis, rapidly progressive course, and high mortality rate (Murray et al., 2022). Overall mortality associated with P. aeruginosa BSIs was recently reported at 26.8% (Shi et al., 2019). The incidence of P. aeruginosa-induced BSI is on the rise, contributing to elevated rates of morbidity and mortality among affected individuals. Understanding the sources and risk factors associated with these infections is crucial for effective management and prevention. Various studies have attempted to identify the origins of BSI associated with P. aeruginosa. Among these reports, respiratory tract infections and central venous catheters stand out as the most frequent sources. Several risk factors have been linked to P. aeruginosa BSIs. Patients who are immunocompromised, particularly those in intensive care units (ICUs), face an increased susceptibility to such infections (Bhardwaj et al., 2021). Furthermore, individuals with underlying conditions like lung cancer are at an increased risk. Previous antimicrobial therapy has also been identified as a risk factor, emphasizing the need for judicious and appropriate use of antibiotics. The severity of infection and pathogenesis of bloodstream infections have been linked to producing type III secretion-dependent exo-products and quorum-sensing dependent virulence (van Delden, 2007). During the COVID-19 pandemic, the overall incidence of BSIs including those caused by P. aeruginosa increased. P. aeruginosa BSIs overall increased among COVID-19 patients due to multiple factors including prolonged hospitalization, high-dose systemic corticosteroids treatment for acute respiratory distress in the ICU, and the administration of immune-modulator treatment such as tocilizumab or baricitinib (Bongiovanni and Barda, 2023).
3 Pathogenicity and antimicrobial resistance of Pseudomonas aeruginosa
Pseudomonas aeruginosa stands at the forefront of antibiotic resistance, notably earning a place on the WHO list of “priority pathogens” (Balakrishnan, 2022). These pathogens have posed a critical therapeutic challenge by demonstrating resistance to multiple antimicrobials, including imipenem, meropenem, and 3rd generation cephalosporins, which are currently the most effective drugs for combatting MDR bacteria. P. aeruginosa is part of the notorious group of “ESKAPE” pathogens, named for Enterococcus faecium, Staphylococcus aureus, Klebsiella pneumoniae, Acinetobacter baumannii, Pseudomonas aeruginosa, and Enterobacter spp., which are deemed of the highest concern due to their urgent need for new antibiotics (Mulani et al., 2019) (Figure 2).
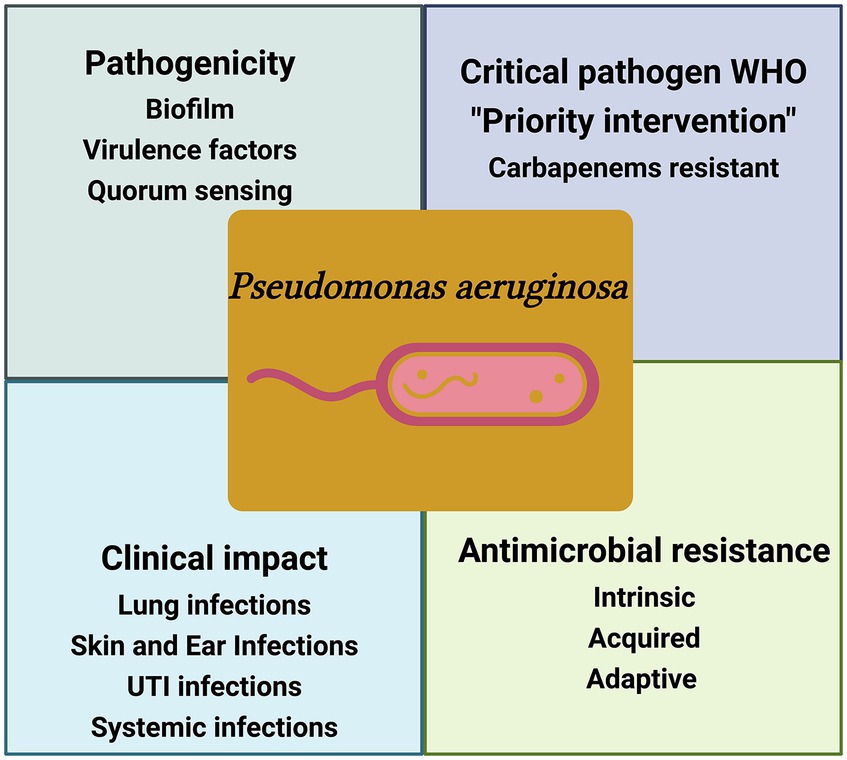
Figure 2. Schematic illustration of the key factors of P. aeruginosa pathogencity. WHO-designated critical pathogen, P. aeruginosa, exhibits intricate antimicrobial resistance, posing a global health threat and causing diverse clinical infections, the figure was created with biorender.
The clinical impact of P. aeruginosa infections is profound, particularly among vulnerable populations such as critically ill patients, immunocompromised individuals, and the very young and elderly. It is considered the 4th most frequently isolated nosocomial pathogen and one of the life-threatening bacteria showing a high mortality rate in immunocompromised patients (Hafiz et al., 2023). The impact of P. aeruginosa and its significance has been recognized early. Data from the National Nosocomial Infections Surveillance System (NNISS) between 1986 and 2003 revealed alarming statistics. P. aeruginosa ranked as the second most common cause of pneumonia (18.1%), the third most common cause of UTIs (16.3%), and the 8th most frequently isolated pathogen in BSIs (3.4%) (Gaynes and Edwards, 2005). However, what adds to the gravity of the situation is the disturbing surge in the proportion of resistant P. aeruginosa isolates over the subsequent years. Studies from China have reported a 28.7% prevalence of P. aeruginosa-related infections in tertiary care settings ranking second to Acinetobacter baumannii in its frequency of isolation (Oliveira et al., 2015). Other observational point-prevalence found that P. aeruginosa represented 16.2% of infections in ICU patients and was the cause of 23% of all ICU-acquired infections, with a respiratory source being the most common site of P. aeruginosa infection (Vincent et al., 2020). Resistance to key antibiotics like carbapenems, quinolones, and 3rd generation cephalosporins surged by significant margins. A particular strain known as the “pan drug-resistant” (PDR) P. aeruginosa was isolated in 2006, rendering it resistant to virtually all available antimicrobial agents, including cefepime, ceftazidime, imipenem, meropenem, piperacillin-tazobactam, ciprofloxacin, and levofloxacin (Wang et al., 2006). This emergence of “intractable” infectious agents heralds the drastic consequences of the antibiotic resistance era, as emphasized by numerous public health organizations.
Multiple studies conducted in subsequent years continued to demonstrate P. aeruginosa resistance profile. Resistance rates to various antimicrobial agents, including cefepime, piperacillin, ciprofloxacin, levofloxacin, gentamicin, and amikacin, displayed worrying trends. Eastern and southern parts of Europe, particularly Germany, Hungary, and Slovakia, reported high resistance percentages in P. aeruginosa (European Centre for Disease Control and Prevention, 2015). By 2015, resistance to third and 4th generation cephalosporins like ceftazidime and cefepime had also escalated. A study in 2016 noted P. aeruginosa involvement in nosocomial infections, with UTIs being predominant among patients. The global scope of the P. aeruginosa resistance problem is evident from studies conducted in India, where drug resistance rates to various antibiotics were reported, reflecting an alarming upward trend (Choudhuri et al., 2017). The sensitivity of P. aeruginosa strains to colistin, one of the last-resort antibiotics, remained relatively high, while susceptibility to other commonly used anti-pseudomonal drugs diminished. The prevalence of MDR strains further complicated treatment options, and high resistance levels against antibiotics like quinolones and aminoglycosides were noted (Horcajada et al., 2019). According to the European Centre for Disease Prevention and Control’s annual report, 18.7% of all P. aeruginosa isolates were carbapenem-resistant and 13.4% were resistant to three or more antimicrobial classes (ECDC, 2021). Additionally, reports from veterinary medicine and agriculture pointed to a challenging scenario, with resistance observed even in carbapenems, considered among the most potent antibiotics (Elfadadny et al., 2023b). Given the continuing advance of antimicrobial resistance within P. aeruginosa strains, there is an escalating urgency for the development of innovative therapeutic options. The appearance and widespread dissemination of PDR strains emphasize the severity of this issue. The collaborative endeavors of prominent global health organizations, including the Centers for Disease Control and Prevention (CDC), Infectious Disease Society of America (IDSA), World Economic Forum, and the WHO, emphasize the profound magnitude of the antimicrobial resistance challenge and the imperative for a worldwide response.
Pseudomonas aeruginosa is indebted its remarkable virulence to a formidable array of factors that enable it to initiate and sustain infections, particularly in immunocompromised individuals (Qin et al., 2022). These virulence factors organize a coherence of pathogenicity, enhancing the bacterium’s capacity to colonize, invade, and persist within host tissues. A closer examination of these factors reveals the intricate mechanisms by which P. aeruginosa inflicts damage. Alginate and biofilm formation: in patients with chronic respiratory infections, P. aeruginosa produces a remarkable exopolysaccharide known as alginate. This polysaccharide serves as an adhesive, allowing the bacterium to adhere to surfaces, and provides a protective shield against inhospitable environmental conditions. P. aeruginosa also secretes alginate lyase, an enzyme capable of cleaving alginate into shorter oligosaccharide units (Boyd and Chakrabarty, 1995). This dynamic interplay between alginate production and degradation plays a pivotal role in the pathogenicity of P. aeruginosa. Adhesion and twitching motility: P. aeruginosa initiates infection by binding to gangliosides on host epithelial surfaces. This interaction is facilitated by LPS and bacterial adhesins, including type-IV pili and flagella. Type-IV pili serve to enable adhesion and facilitate “twitching motility.” This unique form of movement allows the bacterium to traverse host cell surfaces, significantly enhancing biofilm development—a critical aspect of P. aeruginosa’s pathogenicity (Kühn et al., 2021). Type III secretion system (T3SS): upon attachment to host cells, P. aeruginosa activates its Type III secretion system creating a channel through which cytotoxic effector proteins are injected into the host cell’s cytosol. Four types of toxins are produced, including ExoS, ExoT, ExoU, and ExoY (Hardy et al., 2021). These toxins wield diverse capabilities, such as GTPase-activating proteinase (GAP) activity, ADP-ribosyl transferase activity (ADPRT), adenylate cyclase activity, and even membrane phospholipid cleavage, an effect seen in ExoU potent phospholipase A2 (PLA2) activity. These toxins collectively initiate inflammation and subvert host cell functions. Exotoxin A: P. aeruginosa secretes Exotoxin A, an ADPRT that disrupts protein synthesis by inhibiting the host elongation factor 2 (EF2). This disruption results in cell death, further exacerbating pathogenic effects (Michalska and Wolf, 2015). Lipases and phospholipases: lipases and phospholipases produced by P. aeruginosa contribute to pathogenicity by dissolving surfactant lipids and host cell membrane phospholipids. This destructive action compromises host cell integrity, aiding bacterial invasion (Rosenau et al., 2010). Pyocyanin and pyoverdine: the blue-green pigment pyocyanin plays a pivotal role in pathogenicity by inducing oxidative stress within host cells. It disrupts host catalase and the electron transport system (ETS), suppressing phagocytosis (Hall et al., 2016). Pyoverdine also interferes with host electron transport pathways and the redox cycling system, impairing immune system function (Durán et al., 2022). Type VI secretion system (T6SS): comprising H1, H2, and H3 variants, the T6SS enhances P. aeruginosa’s survival and virulence. H1-T6SS plays a role in antimicrobial activity, while H2-and H3-T6SS enable interactions with both prokaryotic and eukaryotic cells (Lesic et al., 2009). Elastases: two types of elastases, LasA and LasB, contribute to the pathogenesis of P. aeruginosa. LasA hydrolyzes the penta-glycine bridge crucial for peptidoglycan stabilization in the cell wall. In contrast, LasB is responsible for the opsonization of lung surfactant proteins A and D (Cigana et al., 2021).
Understanding the genetic similarity and dissemination of P. aeruginosa clones is paramount for both localized infection control and global public health surveillance (Dos Santos et al., 2023). Various methods with varying costs, labor intensity, and discriminatory power have been employed to assess genetic relatedness among P. aeruginosa strains. These methods serve as essential tools for not only managing local outbreaks but also identifying successful international clones. Traditional methods such as serotyping and phage typing rely on the differential sensitivity of isolates to standardized bacteriophages (Waters et al., 2012). These techniques, while less discriminatory, are valuable for managing local outbreaks. Pyocin typing involves the characterization of strains based on their susceptibility to pyocins, which are antibacterial proteins produced by P. aeruginosa (Michel-Briand and Baysse, 2002). This method offers moderate discriminatory power. Molecular techniques like Pulse-Field Gel Electrophoresis (PFGE), Field-Inversion Gel Electrophoresis (FIGE), and Random Amplified Polymorphic DNA Polymerase Chain Reaction (RAPD-PCR) (Hematzadeh and Haghkhah, 2021), provide higher discriminatory power and are often used in local outbreak investigations. Oligonucleotide microarrays allow for simultaneous analysis of multiple genes, offering more comprehensive strain characterization (Wagner et al., 2003). Additionally, Multi-Locus Sequence Typing (MLST) involves sequencing specific housekeeping genes to identify genetic variation among isolates (Curran et al., 2004). It provides a standardized nomenclature for strain comparison. Whole-genome sequencing (WGS) offers the highest discriminatory power and has become a gold standard in identifying international clones. It enables the comprehensive analysis of an organism’s entire genetic makeup (Madaha et al., 2020).
Currently, three major international MDR clones have emerged as global threats: ST111, ST175, and ST235 (del Barrio-Tofiño et al., 2020). ST111 (serotype O12) and ST235 (serotype O11) have demonstrated widespread distribution across continents, while ST175 (serotype O4) has primarily been confined to European countries, especially in Spain and France (Pea et al., 2015; del Barrio-Tofiño et al., 2020). ST235 clones are particularly noteworthy due to their high virulence, driven by the presence of ExoU, a potent cytotoxin. These isolates pose significant therapeutic challenges as they exhibit multidrug resistance. Interestingly, the fitness burden associated with maintaining MDR in ST235 clones appears to be comparatively lower, possibly contributing to their successful dissemination. WGS has further allowed for the categorization of P. aeruginosa isolates into three distinct resistotypes: PAO1, PA14, and PA7 (Reboud et al., 2017). PAO1 and PA14 are characterized by possessing the T3SS and the corresponding effector toxins, ExoS (PAO1) and ExoU (PA14) (Reboud et al., 2017). In contrast, PA7 lacks T3SS and employs the Type VI secretion system to secrete ExlA exolysin, damaging surrounding tissue cells. Interestingly, the carriage of the exoU gene in P. aeruginosa isolates has been associated with resistance to aminoglycosides and fluoroquinolones, suggesting a possible link between virulence and antibiotic resistance.
4 Types of resistance in Pseudomonas aeruginosa
Pseudomonas aeruginosa deploys a spectrum of resistance mechanisms challenging traditional antibiotic therapies. Intrinsic resistance, a foundational trait encoded in its genome, involves factors like low outer membrane permeability, Mex-type efflux pumps, and AmpC β-lactamase. These elements collectively establish a basal resistance level present across all strains. Acquired resistance includes mutations influencing antibiotic targets and the acquisition of modifying enzymes or resistance plasmids. This adaptability enables the bacterium to evolve swiftly, undermining the efficacy of conventional antibiotics. Intriguingly, P. aeruginosa exhibits adaptive resistance mechanisms, responding dynamically to environmental stimuli. This feature allows the bacterium to adjust its resistance profile based on host factors and signal molecules, further complicating treatment strategies. The interplay of these resistance mechanisms contributes to the development of MDR strains, posing a serious threat to public health (Figure 3). Clarification of the complexities of these mechanisms is imperative for the development of innovative therapies that can effectively combat the rising challenges posed by P. aeruginosa infections.
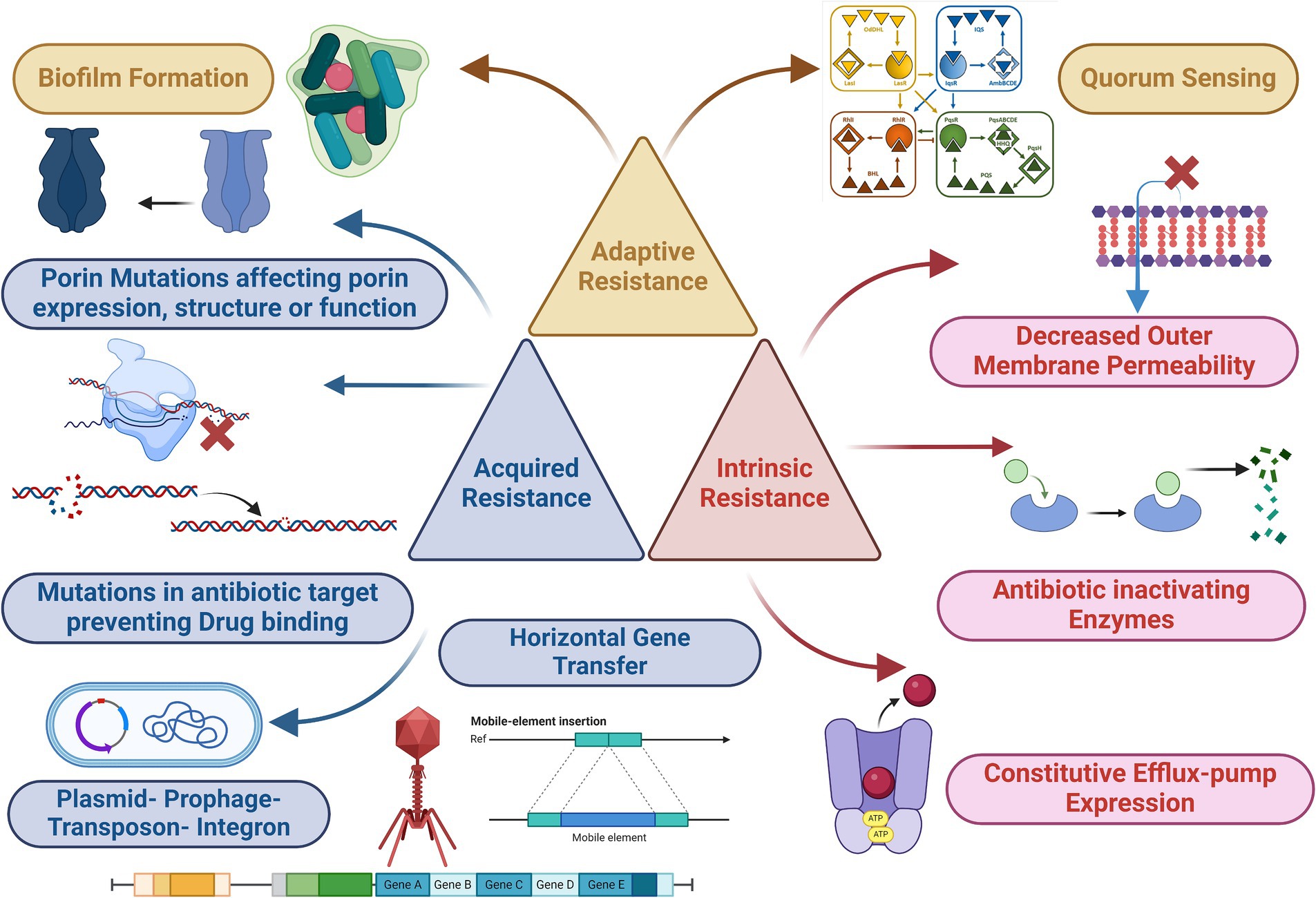
Figure 3. Mechanism of antimicrobial resistance (acquired, intrinsic, and adaptive) of P. aeruginosa. Parts of the figure was edited from (Schütz and Empting, 2018), the figure was created with biorender.
4.1 Acquired resistance
4.1.1 Mutation
Mutation-driven resistance in P. aeruginosa is a complex phenomenon involving various mechanisms that enable the bacterium to withstand the effects of antimicrobial drugs. These mutations affect critical aspects of bacterial biology, resulting in several resistance mechanisms (Serwecińska, 2020). Enhanced β-lactamase production: mutation-induced disruptions in the DNA oxidative repair system can increase mutation rates in P. aeruginosa. This, in turn, leads to enhanced production of β-lactamase enzymes, which can break down β-lactam antibiotics. Additionally, mutations can cause the overexpression of efflux pumps, such as MexCD-OprJ, which actively remove antibiotics from bacterial cells, reducing the drugs’ effectiveness. The primary mechanism driving β-lactam resistance, often caused by mutations, frequently involves the overproduction of the chromosomal cephalosporinase known as ampC (Lorusso et al., 2022). This process engages a complex network of genes associated with intricate regulatory pathways responsible for recycling cell wall components. Among these genes, mutational inactivation of dacB, which encodes the non-essential penicillin-binding protein (PBP) PBP4, and ampD, responsible for encoding N-acetyl-muramyl-l-alanine amidase, are the most common causes leading to ampC activation and consequent β-lactam resistance (Zamorano et al., 2010). Interestingly, the inactivation of PBP4 has been shown to activate the BlrAB/CreBC regulatory system, further intensifying the levels of resistance (Moya et al., 2009). Moreover, specific point mutations that induce a conformational change in the transcriptional regulator AmpR, thereby upregulating ampC and promoting β-lactam resistance, have been observed in clinical strains. Examples of such mutations include D135N and the R154H mutation, which is associated with the widespread MDR/XDR ST175 high-risk clone (Juan et al., 2017). Furthermore, numerous other genes, including those coding for various amidases (AmpDh2 and AmpDh3), different PBPs (such as PBP5 and PBP7), lytic transglycosylases (such as SltB1 and mltB), MPL (UDP-N-acetylmuramate: L-alanyl-γ-D-glutamyl-meso-diaminopimelate ligase), and NuoN (NADH dehydrogenase I chain N), have been shown to enhance ampC expression (Ropy et al., 2015). In addition to ampC overexpression, recent studies have unveiled alternative pathways to β-lactam resistance development. This includes the emergence of novel combinations of β-lactam-β-lactamase inhibitors such as ceftolozane/tazobactam and ceftazidime/avibactam due to mutations that induce structural modifications in AmpC (Haidar et al., 2017). To date, more than 500 Pseudomonas Derived Cephalosporinase (PDC) variants, https://arpbigidisba.com/pseudomonas-aeruginosa-derived-cephalosporinase-pdc-database/, have been characterized, including those linked to enhanced resistance against ceftolozane/tazobactam and ceftazidime/avibactam. Porin mutations and reduced membrane permeability: P. aeruginosa relies on porin proteins, like OprD the second major porin protein and one of the most well-characterized in P. aeruginosa, to create channels within its membrane for the entry of hydrophilic antibiotics (Welte et al., 1995). Mutations affecting porin expression or function can reduce bacterial membrane permeability, resulting in increased antibiotic resistance. OprD deficiency, for instance, confers high-level resistance to carbapenem antibiotics, particularly imipenem (Fang et al., 2014). A study conducted in southern China investigated 61 clinical isolates of P. aeruginosa that exhibited resistance to imipenem. Their findings indicated that among these isolates, 50 had mutations leading to the disruption of OprD (Fang et al., 2014). These mutations included frameshift mutations or the presence of premature stop codons. Additionally, 5 isolates showed reduced OprD expression, while in 6 isolates, OprD was undetectable through PCR analysis. Furthermore, the study conducted a functional analysis, revealing that loops 2 and 3 within the OprD protein served as entrance and binding sites for imipenem. Efflux pump overexpression and regulatory mutations: efflux pumps play a crucial role in antibiotic resistance by pumping antibiotics out of bacterial cells. Mutations in regulatory genes like mexR, nalB, nalC, or nalD can disrupt the normal control of efflux pump expression. Consequently, this leads to the overexpression of efflux pumps such as MexAB-OprM, reducing susceptibility to various antibiotics, including β-lactams and fluoroquinolones (Pang et al., 2019). Mutations in the mexZ gene can also induce overexpression of MexXY-OprM, increasing resistance to aminoglycosides, β-lactams, and fluoroquinolones. Modifications in antibiotic targets: P. aeruginosa can modify antibiotic targets through mutations. For example, mutations in genes encoding DNA gyrase (gyrA and gyrB) and topoisomerase IV (parC and parE) can alter the binding affinity of these proteins to quinolone antibiotics, reducing susceptibility to quinolones (Hooper and Jacoby, 2016). Additionally, mutations can affect ribosomal proteins (30S ribosomal subunit), leading to high resistance to aminoglycosides that target protein translation. Modifications in PBPs can increase resistance to β-lactam antibiotics. Resistance to polymyxin in P. aeruginosa has been demonstrated to correlate with alterations in the partner molecule for polymyxin binding-LPS (Gutu et al., 2013). This involves the addition of 4-amino-L-arabinose (L-Ara4N) to the phosphate groups located within the lipid A portion of LPS (Pang et al., 2019). Structural modifications of antibiotic targets: resistance to novel combinations of β-lactam-β-lactamase inhibitors can result from mutations leading to structural modifications of AmpC. Moreover, diverse AmpC variants have been associated with high-level cephalosporin resistance. Additionally, mutations in genes encoding proteins like PBP3 (encoded by ftsI) contribute to β-lactam resistance. Specific mutations in PBP3 have been associated with β-lactam resistance in clinical strains (Sethuvel et al., 2023).
4.1.2 Horizontal gene transfer
Horizontal gene transfer plays a pivotal role in the acquisition of antibiotic resistance genes by P. aeruginosa, enabling it to adapt to various environmental challenges. Antibiotic resistance genes can be harbored on diverse genetic elements such as plasmids, transposons, integrons, and prophages (Michaelis and Grohmann, 2023). These genes can be acquired by P. aeruginosa through horizontal gene transfer, either from closely related bacterial species or distant ones. Integrons, which are genetic elements specialized in inserting mobile gene cassettes through site-specific recombination, have emerged as critical players in the dissemination of antibiotic resistance within P. aeruginosa populations. These integrons serve as repositories for a wide array of antibiotic resistance determinants (Bhat et al., 2023). Horizontal gene transfer mechanisms include transformation, transduction, and conjugation. P. aeruginosa has demonstrated the ability to acquire genes conferring resistance to aminoglycosides and β-lactam antibiotics. Notably, various metallo-beta-lactamase (MBL) genes, such as IMP, VIM, SPM, GIM, NDM, and FIM, which encode enzymes capable of hydrolyzing a broad spectrum of β-lactam antibiotics, have been identified in P. aeruginosa (Potron et al., 2015). These MBL genes are often associated with genetic elements like integrons and plasmids. Furthermore, integrons have been found to harbor multiple antibiotic-resistance genes simultaneously. For example, aminoglycoside resistance genes aacA29a and aacA29b have been identified within class I integrons in clinical P. aeruginosa isolates, situated at the 5′ and 3′ ends of the carbapenem-hydrolyzing β-lactamase VIM-2 gene cassette, respectively. in addition, it is important to note that many resistance genes in P. aeruginosa are located within resistance islands rather than plasmids (Magnet et al., 2003). Unlike some plasmids that are readily transferable to E. coli, many Pseudomonas spp. plasmids have a narrow host range. These plasmids have been classified using a separate incompatibility typing system known as IncP-1 to IncP-13 (Xiong et al., 2013). IncP-2 plasmids, historically common in P. aeruginosa, were typically large and carried tellurite resistance in addition to antibiotic resistance. More recently, various plasmids from P. aeruginosa, including those from carbapenem-resistant strains, have been sequenced, revealing their role in the dissemination of resistance genes, particularly carbapenemase genes including blaIMP-9, its variant blaIMP-45, or blaVIM-2. These plasmids often carry class 1 integrons within Tn21 subfamily transposons (Xiong et al., 2013).
4.2 Intrinsic resistance
4.2.1 Efflux system resistance
Bacterial efflux systems are categorized into five major families: Resistance Nodulation Division (RND), ATP Binding Cassette (ABC), Major Facilitator Superfamily (MFS), Small Multidrug Resistance (SMR), and Multidrug & Toxic Compound Extrusion (MATE) (Huang et al., 2022). Among these, the RND family, characterized by its cytoplasmic membrane transporters, periplasmic linker proteins, and outer membrane porin channel proteins, holds a pivotal role in antibiotic resistance, particularly in P. aeruginosa. These efflux systems function as a defense mechanism by expelling harmful substances from bacterial cells (Poole, 2011). The RND family of efflux systems consists of two essential components: multidrug efflux (Mex) and outer membrane porin (Opr) (Qin et al., 2022). These components work in synergy to facilitate the efflux of various toxic compounds, including antibiotics. Within the RND family, the MexXY efflux system is a key player. It encodes two crucial proteins, MexY and MexX, which work together to expel toxic substances from the bacterial cell (Dreier and Ruggerone, 2015). This efflux system has a broad substrate range, allowing it to efficiently pump out a variety of antibiotics and other harmful compounds. MexAB-OprM is renowned for its resistance against multiple antibiotics, including ticarcillin, broad-spectrum cephalosporins, and β-lactams. In clinical isolates, it’s frequently associated with β-lactam resistance. A combination of efflux systems, including MexAB-OprM, MexCD-OprJ, and MexXY-OprM, contributes to carbapenem resistance (Riera et al., 2011). Although efflux plays a role, it often acts alongside other resistance mechanisms. Besides P. aeruginosa, other bacteria like Burkholderia pseudomallei and E. coli employ three-component RND-type aminoglycoside efflux systems in their resistance strategies. Notably, the expression of MexXY can be upregulated in mutants when exposed to antibiotics, enhancing their resistance compared to wild-type strains. Wild-type strains of P. aeruginosa inherently possess resistance to specific antibiotic classes, such as tetracyclines, aminoglycosides, glycylcyclines, and erythromycin. However, the MexXY efflux system extends this resistance to a wide array of antibiotics, including lincomycin, macrolides, fluoroquinolones, chloramphenicol, β-lactams, and novobiocin. This broad substrate range contributes significantly to the remarkable antibiotic resistance observed in Pseudomonas. The expression of the MexXY gene within the RND family is tightly regulated by the mexZ repressor, which belongs to the tetracycline repressor protein (TetR) and AcrR repressor protein family (Fujiwara et al., 2022). This ensures that the efflux system responds appropriately to the presence of antibiotics. Overexpression of these pumps has been documented in various clinical strains of P. aeruginosa, substantially amplifying bacterial antibiotic resistance.
4.2.2 Outer membrane impermeability resistance
In the context of antibiotic resistance, overcoming the protective fortress of the bacterial cell membrane is a challenge faced by antibiotics. These resilient pathogens, like P. aeruginosa, deploy an array of defense mechanisms that antibiotics must navigate. Distinct antibiotic classes employ specific strategies to combat P. aeruginosa. Aminoglycoside antibiotics, such as tobramycin, gentamicin, and amikacin, disrupt bacterial protein synthesis by binding to ribosomal 30S subunits (Krause et al., 2016). Meanwhile, quinolone antibiotics, including ciprofloxacin and levofloxacin, obstruct DNA replication through inhibition of DNA gyrase and topoisomerase IV (Hooper and Jacoby, 2016). The β-lactam antibiotics, encompassing penicillin, cephalosporin, carbapenem, and monobactam, hinder bacterial cell wall biosynthesis by targeting PBPs, pivotal enzymes in peptidoglycan synthesis (Cho et al., 2014). Polymyxins, exemplified by polymyxin B and polymyxin E (colistin), are polypeptide antibiotics that bind to the LPS on the outer membrane of Gram-negative bacteria (Slingerland et al., 2022). This binding enhances cell membrane permeability, facilitating greater antibiotic uptake. Remarkably, polymyxins execute their bactericidal effects by inducing a hydroxyl radical-mediated cell death pathway. For β-lactams and quinolones, penetration through porin channels within the bacterial cell membrane is the pathway of choice. In contrast, aminoglycosides and polymyxins employ a different strategy, interacting with bacterial LPS on the outer membrane of Gram-negative bacteria to enhance their uptake (Prajapati et al., 2021). The outer membrane of Gram-negative bacteria, including P. aeruginosa, acts as a selective barrier, complicating antibiotic penetration. This outer membrane is an asymmetric bilayer containing phospholipids and LPS. Porins, forming β-barrel protein channels, are scattered within this structure. LPS itself comprises lipid A, an oligosaccharide core, and O antigen. Notably, organic compounds like citric and lactic acid act as chelating agents, disrupting the oligosaccharide core by binding to Mg2+ cations in the LPS molecule. This interaction alters the bacterial cell wall’s permeability, adding an extra layer of resistance. Within P. aeruginosa, a diverse family of porins contributes to regulating permeability. OprF serves as the major non-specific porin, while OprB, OprD, OprE, OprO, and OprP are specific porins. OprC and OprH are categorized as gated porins, and the efflux porins include OprM, OprN, and OprJ (Qin et al., 2022). Understanding these intricate mechanisms offers insights into the multifaceted strategies employed by P. aeruginosa to resist antibiotic intrusion.
4.2.3 Enzyme modification
P. aeruginosa wields a formidable weapon, antibiotic-inactivating enzymes. These enzymes possess the ability to dismantle or modify antibiotics, rendering them powerless. This intrinsic defense mechanism presents a significant hurdle for clinicians combating P. aeruginosa infections. One of the most critical classes of antibiotic-inactivating enzymes in P. aeruginosa is β-lactamases. β-lactam antibiotics, including penicillin and cephalosporins, target bacterial cell walls by inhibiting PBP. P. aeruginosa has honed its β-lactamase production to counteract these antibiotics by hydrolyzing the b-lactam ring that is present in b-lactam antibiotics. β-lactamases are categorized into four classes: A, B, C, and D, depending on their amino acid sequences (Bush and Bradford, 2016). Classes A, C, and D utilize an active site serine to hydrolyze β-lactams, whereas class B β-lactamases are metalloenzymes requiring zinc ions for this process. P. aeruginosa produces class C β-lactamases, which have been demonstrated to hinder antipseudomonal cephalosporins, a subgroup of β-lactam antibiotics. Some P. aeruginosa strains go a step further by generating extended-spectrum-β-lactamases (ESBLs), primarily falling into class A. Interestingly, OXA-type ESBLs, recognized for their ability to hydrolyze oxacillin, are classified under enzyme class D and were initially identified in P. aeruginosa isolates (Castanheira et al., 2021). These ESBLs confer a high level of resistance to a wide array of β-lactam antibiotics, encompassing penicillins, cephalosporins, and aztreonam. Additionally, AmpC β-lactamase, an Ambler class C enzyme, encoded by the chromosomal blaAmpC gene, is a central player in P. aeruginosa’s resistance strategy. AmpC is constitutively produced and can be induced further by aminopenicillins and cephalosporins. It hydrolyzes the β-lactam ring, effectively inactivating β-lactam antibiotics. Metallo β-lactamases (MBLs) are categorized as Ambler class B enzymes, distinct from the serine-based hydrolytic system. Notably, MBLs feature zinc in their active sites. In P. aeruginosa, the most clinically significant MBLs are the Verona integron-encoded MBLs (VIM) and the active-on-imipenem type MBLs (IMP) (Tooke et al., 2019). These enzymes are frequently situated on integrons, often alongside other resistance genes, and confer high-level resistance to carbapenem antibiotics.
Aminoglycoside antibiotics, frequently employed in the treatment of P. aeruginosa infections, face a formidable challenge in the form of enzymatic modification-based resistance mechanisms employed by this bacterium. P. aeruginosa employs an array of enzymes with specific functions in this process. Aminoglycoside Phosphotransferases (APHs) play a crucial role by modifying aminoglycosides like kanamycin, neomycin, and streptomycin. They achieve this by transferring a phosphoryl group, effectively rendering these antibiotics inactive. In parallel, Aminoglycoside Acetyltransferases (AACs) enter the scene, transferring an acetyl group to amino groups at positions 3′ and 6′ of aminoglycosides. This acetylation process leads to the inactivation of a range of aminoglycosides, including gentamicin, tobramycin, netilmicin, kanamycin, and amikacin (Ramirez and Tolmasky, 2010). Furthermore, Aminoglycoside Nucleotidyltransferases (ANTs) add another layer of defense by transferring an adenylyl group to either the amino or hydroxyl group of aminoglycosides, effectively conferring resistance against antibiotics such as gentamicin, amikacin, and tobramycin (Ramirez and Tolmasky, 2010).
4.3 Adaptive resistance
4.3.1 Biofilm formation and quorum sensing mediated resistance
Biofilm formation is a remarkable survival strategy employed by P. aeruginosa. In this complex process, P. aeruginosa communities adhere to surfaces and are encapsulated within a matrix of extracellular polymeric substances (EPSs), which includes exopolysaccharides, proteins, metabolites, and extracellular DNA (eDNA). P. aeruginosa biofilms are characterized by a sophisticated matrix composed of various EPS components, including complex polysaccharides, proteins, lipids, and eDNA (Thi et al., 2020). This matrix provides structural integrity to the biofilm and offers several critical advantages to the bacteria within it. Immune System Evasion: Biofilms provide physical protection from the host immune system. The matrix acts as a barrier, preventing immune cells from reaching and eliminating the bacteria (Vestby et al., 2020). Antimicrobial Resistance: Bacteria within biofilms exhibit a significantly higher resistance to antimicrobial agents, including antibiotics. This resistance arises from reduced antibiotic penetration and decreased metabolic activity within biofilm-associated cells (Singh et al., 2017). Water Retention and Desiccation Tolerance: Biofilms retain water, which helps the bacteria survive in arid or hostile environments. This property contributes to the resilience of biofilm communities (Secor et al., 2015). Nutrient Storage and High Enzymatic Activity: Biofilms can efficiently sorb and store nutrients, supporting bacterial growth. They also exhibit heightened extracellular enzymatic activity, aiding in resource utilization. Adhesion and Virulence: The biofilm matrix facilitates adhesion to infection sites, promoting persistent colonization (Limoli et al., 2015).
Biofilm-associated infections pose significant clinical challenges. P. aeruginosa’s biofilm formation results in a profound increase in antibiotic tolerance, making treatment difficult. Furthermore, the diffusion barrier created by the biofilm matrix limits antibiotic exposure to only the superficial layers of the biofilm, rendering deep-seated infections highly resistant to treatment. P. aeruginosa is renowned for its biofilm-forming capabilities. These biofilms can develop in various environments, including the anaerobic conditions found in the lungs of cystic fibrosis (CF) patients (Valentin et al., 2022). Biofilm formation by P. aeruginosa is a finely tuned process regulated by various factors: Quorum Sensing Systems: P. aeruginosa employs multiple quorum sensing systems, including LasI-LasR, RhlI-RhlR, and PQS-MvfR, which contribute to the formation of mature and differentiated biofilms (Ambreetha and Singh, 2023). Two-Component Regulatory Systems: The GacS/GacA and RetS/LadS two-component systems play crucial roles in regulating biofilm formation. GacA promotes biofilm formation, while RetS inhibits it (Mikkelsen et al., 2011). Exopolysaccharides: Alginate, Pel, and Psl are exopolysaccharides produced by P. aeruginosa, stabilizing the biofilm structure (Colvin et al., 2012). eDNA: eDNA serves as a vital component of the biofilm matrix. It contributes to initial cell–cell adhesion, protects the biofilm from detergents, and influences aminoglycoside resistance (Wei and Ma, 2013). Cyclic di-GMP (c-di-GMP): Intracellular levels of c-di-GMP play a crucial role in biofilm formation. High c-di-GMP levels are associated with biofilm formation, whereas low levels correspond to planktonic growth (Park and Sauer, 2022).
During biofilm formation, P. aeruginosa undergoes various physiological changes. In CF chronic infections, P. aeruginosa strains switch to a mucoid phenotype, characterized by increased alginate production. Additionally, P. aeruginosa initially relies on its flagellum for motility but downregulates flagellum expression after surface attachment to evade immune detection. Recent investigations have unveiled critical insights into the formidable antibiotic resistance mechanisms at play within P. aeruginosa biofilms. In a study by Sadovskaya and colleagues in 2010 (Sadovskaya et al., 2010), a family of cyclic glycerophosphorylated β-(1, 3)-glucans secreted by P. aeruginosa was identified within the extracellular matrix of biofilms. These glucans were found to interact with and sequester kanamycin, a frequently used antibiotic, rendering it less effective. Additionally, research conducted shed light on novel genes present in the P. aeruginosa clinical isolate PA14. While these genes did not directly influence biofilm formation, they played a crucial role in enhancing biofilm-specific antibiotic resistance. The ndvB gene, for instance, encoded a vital glucosyltransferase responsible for synthesizing periplasmic cyclic-β-(1, 3)-glucans, which physically captured tobramycin, preventing it from reaching its site of action. Another gene cluster participated in the creation of a novel efflux pump within P. aeruginosa. Its deletion resulted in reduced resistance of the bacterium to antibiotics like gentamicin and ciprofloxacin within the biofilm. Lastly, the tssC1 gene, associated with the type VI secretion system in P. aeruginosa, exhibited heightened expression in biofilms. When tssC1 was deleted, it led to diminished resistance to antibiotics commonly employed in the treatment of P. aeruginosa infections, including tobramycin, gentamicin, and ciprofloxacin (Zhang et al., 2011).
Quorum sensing (QS) is an intriguing communication system used by P. aeruginosa to coordinate its behavior. In this system, P. aeruginosa relies on the release of specific signaling molecules known as autoinducers to communicate with other bacteria. Notably, P. aeruginosa employs four distinct QS pathways to achieve this communication. Two of these pathways involve LasR and LasI, as well as RhlR and RhlI. LasI, part of the LasR/I system, produces a molecule called 3O-C12-HSL. This molecule plays a vital role in the QS process (Kanak et al., 2023). It binds to LasR, which triggers the expression of LasI and leads to the production of virulence factors. Additionally, it contributes to the formation of biofilms. On the other hand, the Rhl system involves RhlI, which synthesizes another signaling molecule called C4-HSL. When C4-HSL binds to RhlR, it activates RhlI, initiating the expression of genes responsible for virulence factors and biofilm components. These two systems, LasR/I and RhlR/I, are interconnected, and their activities are influenced by a molecule called the Pseudomonas quinolone signaling system (PQS), which acts as a bridge between them. Las and Rhl systems regulate the synthesis of PQS, and in turn, regulate the activity of RhlR and RhlI (Venturi, 2006). Understanding this intricate web of quorum sensing in P. aeruginosa provides valuable insights into how this bacterium causes disease and becomes resistant to antibiotics. Researchers are actively exploring innovative approaches to combat P. aeruginosa infections, focusing on anti-virulence strategies that target QS and the inhibition of biofilm formation.
5 Innovative therapies for resistance in Pseudomonas aeruginosa
To combat P. aeruginosa infections, healthcare practitioners commonly rely on eight categories of antimicrobial agents. These encompass aminoglycosides (including gentamicin, amikacin, netilmicin, and tobramycin), carbapenems (such as imipenem, meropenem, and doripenem), cephalosporins (notably ceftazidime and cefepime), fluoroquinolones (like ciprofloxacin and levofloxacin), penicillins combined with β-lactamase inhibitors (including ticarcillin-clavulanic acid and piperacillin-tazobactam), monobactams (represented by aztreonam), phosphonic acids (specifically Fosfomycin), and polymyxins (comprising colistin and polymyxin B). Notably, Ceftazidime-avibactam and ceftolozane-tazobactam have received FDA approval and are accessible for clinical use (Bassetti et al., 2019). Additionally, cefiderocol and imipenem-cilastatin/relebactam are presently in the developmental stages (Bassetti and Garau, 2021). Magiorakos et al. established criteria for categorizing P. aeruginosa strains based on their resistance profiles. According to these criteria, strains are considered MDR if they exhibit non-susceptibility to at least one agent in three or more antimicrobial categories. Strains are categorized as extensively drug-resistant (XDR) when they display non-susceptibility to at least one agent in all categories except for two or fewer (Magiorakos et al., 2012). Lastly, strains are classified as pandrug-resistant (PDR) if they demonstrate non-susceptibility to all the listed antimicrobial agents (Magiorakos et al., 2012). The excessive and improper use of antibiotics is causing growing concerns in the realm of public health. This misuse not only leads to unnecessary side effects but also contributes to the development of drug-resistant bacteria. Adding to the challenge is the slow and limited progress in developing new antibiotics. As a result, there has been a noteworthy shift in focus over the past decade toward innovative approaches for tackling P. aeruginosa infections (Figure 4). These fresh strategies can function independently or in conjunction with traditional treatments, offering a diverse toolbox for combating these infections.
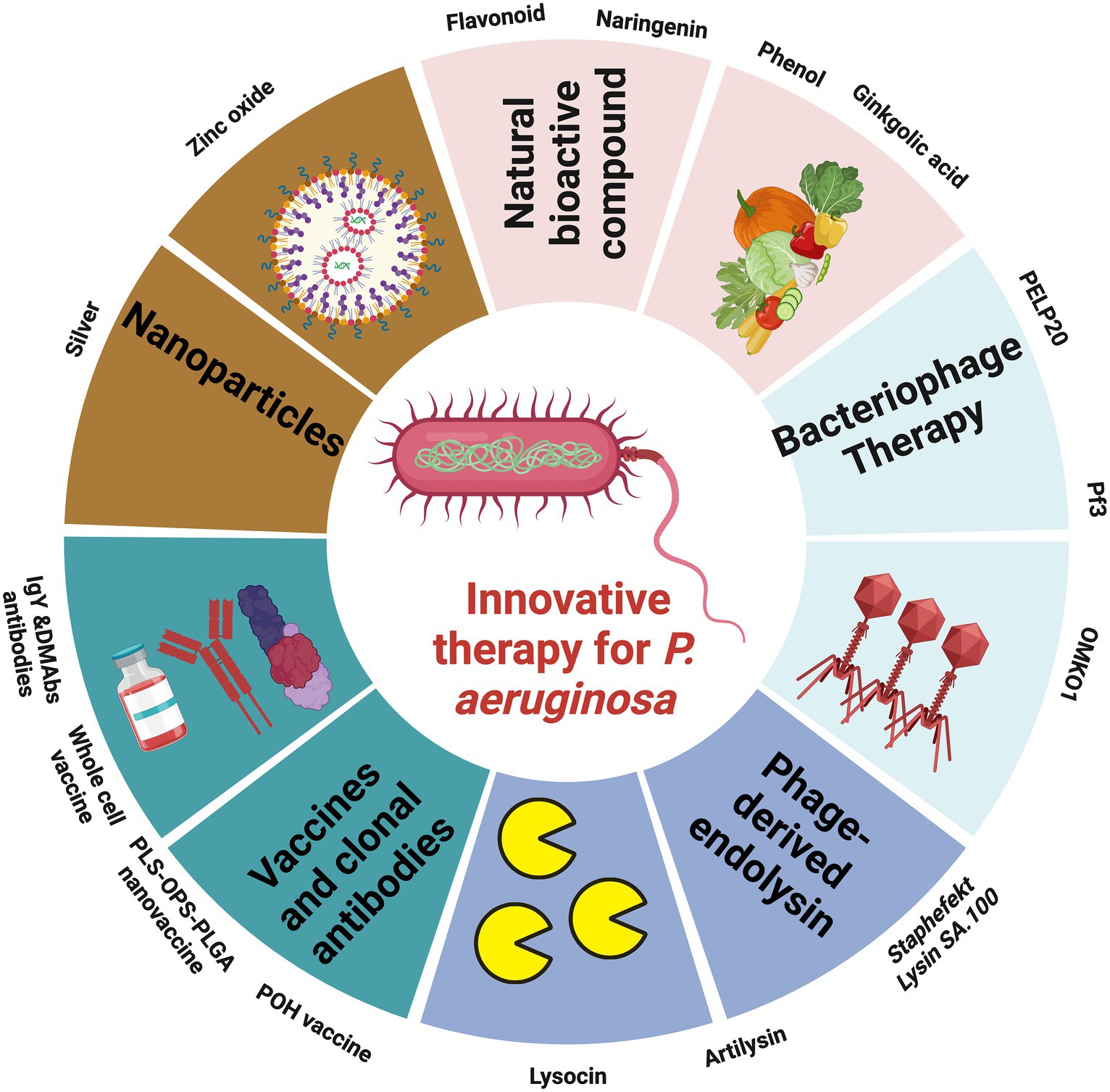
Figure 4. Illustration showing the promising therapies to combat the outbreak of MDR P. aeruginosa, the figure was created with biorender.
5.1 Natural bioactive compounds
Natural Bioactive Compounds (NBCs) are metabolites originating from plants that possess antioxidant, antitumor, anti-inflammatory, and antimicrobial functions (Youns and Abdel Halim Hegazy, 2017; Pai et al., 2022; Elfadadny et al., 2023a). Researchers worldwide are actively investigating novel antimicrobial agents to combat P. aeruginosa infections. These studies employ advanced molecular techniques and thorough drug screening to explore the potential of NBCs in countering P. aeruginosa. These NBCs have shown promise in inhibiting P. aeruginosa’s quorum sensing system, thereby reducing its virulence factors and preventing biofilm formation, a key protective mechanism of P. aeruginosa.
Flavonoids, a group of compounds found in plants, play a dual role in this context. They act as antioxidants for the host system, contributing to overall health without toxicity. However, they also act as pro-oxidants when encountered by pathogenic microbes, inducing the formation of reactive oxygen species (ROS) that cause oxidative stress within the bacteria. Researchers have developed an inhibitor called meta-bromo-thiolactone (mBTL) targeting LasR and RhlR, key components of the QS system in P. aeruginosa (Eltayb et al., 2022). This inhibitor has demonstrated efficacy both in laboratory settings and in vivo. Furthermore, flavonoids have been explored for their inhibitory effects on P. aeruginosa biofilm formation, hinting at their potential to disrupt the QS system (Paczkowski et al., 2017). Certain flavonoids with specific structural features, such as dihydroxyl groups in the A ring of flavones, have been identified as inhibitors of LasR and RhlR. Examples include baicalein, naringenin, and quercetin. These flavonoids act allosterically, reducing the binding affinity of LasR and RhlR to regulatory promoters.
Naringenin, a unique QS inhibitor derived from a natural source, is found in plant flavonoids. Its mechanism of action involves reducing the production of QS-regulated virulence factors in P. aeruginosa by directly binding to LasR, thereby competing with N-(3-oxo-dodecanoyl)-l-homoserine lactone (HSL), which activates LasR (Hernando-Amado et al., 2020). Consequently, this QS inhibitor can effectively disrupt QS responses only when administered during the early exponential growth phase, allowing naringenin to compete with unbound HSL for binding to LasR. It’s worth mentioning that naringenin is most suitable for targeting P. aeruginosa populations at low cellular densities. This scenario may not always align with the clinical infection situation, where bacterial densities tend to be higher. To fully harness the potential of QS inhibition, there is a need for the development of inhibitors capable of targeting P. aeruginosa QS signaling irrespective of bacterial density or QS status (Hernando-Amado et al., 2020).
Phenols, specifically 2,4-Di-tert-butylphenol (2,4-DBP), sourced from Daldinia eschscholtzii, an endophytic fungus, exhibit significant potential in combating P. aeruginosa infections. This compound plays a crucial role in reducing the secretion of pyocyanin and chitinase. Additionally, 2,4-DBP effectively suppresses the expression of genes associated with QS in P. aeruginosa, such as lasI, lasR, rhII, and rhIR (Mishra et al., 2020).
Ginkgolic acid is an NBC found in medicinal plants, which are rich sources of various bioactive compounds known for their antimicrobial, antiviral, antitumor, and other beneficial properties. In a study conducted by Tahrioui et al. (2020), the antivirulent properties of ginkgolic acid were investigated concerning its impact on P. aeruginosa in infected C. elegans models and found that ginkgolic acid inhibits pyocyanin formation in P. aeruginosa. Moreover, ginkgolic acid also influences the mechanical properties of P. aeruginosa’s cell membrane by modulating SigX, an extracytoplasmic function σ-factor. SigX plays a role in responding to cell wall stress, contributing to the maintenance of the cell envelope’s integrity, and interacting with specific envelope-related compounds, including ginkgolic acid and hydroginkgolic acid. Exposure of P. aeruginosa to ginkgolic acid leads to a significant reduction in the expression of SigX and its associated genes, such as accA, accB, and fabY, which are involved in fatty acid biosynthesis (Tahrioui et al., 2020).
Various other NBCs, like itaconimides, operate on signaling pathways and exhibit the ability to suppress the expression of QS-related genes, thereby mitigating the pathogenicity of P. aeruginosa. Additionally, compounds like N-acyl cyclopentamine, the lactone SsoPox enzyme, and N-acyl homoserine lactonase have emerged as potent inhibitors of QS (Chan et al., 2015). Substances such as curcumin and coumarin possess dual capabilities, acting against virulence factors and biofilm formation, while naringenin, gliptin, and taxifolin demonstrate the potential to reduce the expression of QS-related genes (Khayat et al., 2022; Sadaqat et al., 2022). These findings highlight the rich potential of natural compounds in combatting P. aeruginosa infections through multifaceted mechanisms.
5.2 Bacteriophage therapy
Phages, which are viruses capable of causing bacterial lysis, were initially identified in 1915 by British bacteriologist Frederick Twort. Independently, two years later, Félix d’Herelle in Paris made a similar discovery and introduced the concept of phage therapy. Phage therapy boasts numerous advantages, including its ability to replicate at the infection site, highly specific targeting of bacteria without affecting beneficial flora, minimal side effects compared to other treatments, effectiveness against antibiotic-resistant strains, and ease of administration (Summers, 2012). As an alternative to antibiotics, the use of phages to combat P. aeruginosa infections has been extensively researched. Currently, there are 137 distinct phages characterized to target the Pseudomonas genus (Pires et al., 2015). Numerous in vitro and in vivo studies have explored the efficacy of phages against chronic P. aeruginosa infections. Phage therapy offers a notable advantage over antibiotic treatment due to its effectiveness against P. aeruginosa biofilms. A recent study demonstrated that phage PELP20 effectively eradicated P. aeruginosa strain LESB65, isolated from CF patients, within an artificial sputum medium biofilm model in vitro. It also significantly enhanced bacterial clearance in a mouse model of chronic lung infection caused by P. aeruginosa (Waters et al., 2017). Significantly, pretreating hydrogel-coated catheters with phage M4 substantially reduced the formation of P. aeruginosa biofilms. Another advantage of phage therapy lies in the potential to genetically engineer phages as delivery vehicles for antimicrobial agents, thereby enhancing treatment efficacy (Fu et al., 2010). Over the past decade, researchers have tested genetically engineered P. aeruginosa phages. For instance, the P. aeruginosa phage Pf3 was genetically modified into a nonlytic, nonreplicating phage, Pf3R, by replacing an export protein gene with a BglII restriction endonuclease gene. Pf3R efficiently killed P. aeruginosa PAO1 in vitro, similarly to the lytic phage Pf3, while significantly reducing endotoxin release. Moreover, Pf3R-treated mice displayed an increased survival rate compared to those treated with Pf3, likely due to reduced endotoxin levels and fewer host inflammatory responses (Hagens et al., 2004). An alternative strategy in phage therapy involves using phages to influence the evolution of antibiotic resistance, favoring the development of phage resistance while simultaneously restoring antibiotic susceptibility. As an example, the lytic Myoviridae bacteriophage, OMKO1, employs the bacterial multidrug efflux systems MexAB and MexXY, specifically OprM, as its receptor-binding site. Selecting for resistance to OMKO1 phage attack triggers an evolutionary trade-off in MDR P. aeruginosa. This trade-off results in a modification of the efflux pump mechanism, which in turn increases the sensitivity of the bacteria to various antibiotics, including ciprofloxacin, tetracycline, ceftazidime, and erythromycin—four drugs representing different antibiotic classes (Chan et al., 2016). Phage steering becomes achievable when the binding receptor for the bacteriophage is implicated in both antibiotic resistance and phage resistance. This approach offers the advantage of employing two distinct and opposing mechanisms to combat bacterial infections (Gurney et al., 2020).
Despite the proven effectiveness of phages against bacterial infections in vitro and animal models, the number of clinical trials for phage therapy remains limited. This is primarily attributed to concerns about phage clearance after treatment, impurities in phage preparations, poor stability of phage preparations, and a lack of detailed knowledge about phage mechanisms of action and the potential for bacterial resistance to phages (Jaglan et al., 2022). Clinical trials examining the use of phages against P. aeruginosa infections in patients with venous leg ulcers, burn wounds, and otitis have reported no adverse effects.
5.3 Phage-derived endolysin
Among the recent alternative antibacterial agents being studied now are phage lytic antimicrobial peptides (AMPs). These are bacterial peptidoglycan-degrading enzymes produced by bacteriophages and cause instantaneous cell death (Briers, 2019). Endolysins are lytic enzymes produced during the late phase of the lytic bacteriophage replication cycle to target the bacterial cell walls for progeny release. The use of endolysins as antimicrobial agents against P. aeruginosa presents a promising avenue for combatting this resilient bacterium (Furusawa et al., 2016). However, deploying endolysin therapy against P. aeruginosa is not straightforward, primarily due to the protective outer membrane that shields the bacterial cell from lysin attack. The outer membrane of Gram-negative bacteria, including P. aeruginosa, consists of phospholipids and lipopolysaccharides. LPS molecules are held together by phosphate bonds, making them challenging to breach. To enable endolysins to access the peptidoglycan cell wall and effectively target P. aeruginosa, researchers have employed creative strategies. One such method involves the use of ethylenediaminetetraacetic acid (EDTA) to permeabilize the Gram-negative cell membrane (Murray et al., 2021). EDTA is a chelating agent that removes divalent cations, essential for stabilizing the outer membrane, from their binding sites. This disrupts the membrane’s integrity, rendering it vulnerable to endolysin attack. Permeabilizing the outer membrane can also be achieved through mechanical means, such as high hydrostatic pressure (HPP). HPP has been employed to sensitize Gram-negative bacteria, including P. aeruginosa, to molecules like bacteriocins and antimicrobial peptides by optimizing pressure and time parameters (Prudêncio et al., 2015). Another approach involves fusing endolysins with components such as antimicrobial peptides that facilitate penetration of the peptidoglycan layer. This fusion strategy enhances the endolysin’s ability to reach its target within the bacterial cell. In the case of P. aeruginosa, a bacterium renowned for its antibiotic resistance, endolysin therapy has shown promise. Researchers isolated a phage-derived endolysin called LysPA26 in 2017, which exhibited potent lytic activity against MDR strains of P. aeruginosa. Remarkably, this endolysin achieved a reduction in bacterial numbers of up to four log units in just 30 min. Furthermore, LysPA26 demonstrated stability under varying pH and temperature conditions (Guo et al., 2017). Notably, this endolysin exhibited a broad spectrum of activity, effectively targeting other Gram-negative bacteria such as Klebsiella pneumoniae, Acinetobacter baumannii, and Escherichia coli. The success of LysPA26 underscores the potential of endolysin therapy in addressing MDR P. aeruginosa infections. Its selective targeting of Gram-negative bacteria without the use of antibiotics holds promise as a primary therapeutic option for the future. Currently, not enough data is available regarding the safety profile of endolysins. Also, there is a lack of information about the pharmacokinetics and pharmacodynamics of endolysins as possible medications. Cellular toxicity and cell-penetrating properties of phage-derived endolysins should be studied before being administered as human therapeutic medications (Nandi et al., 2022). In vivo studies are limited to topical models with some concerns about systemic administration related to their short half-life, immunogenicity, and release of proinflammatory components during bacteriolysis which should addressed before further development (Lai et al., 2020).
5.4 Vaccine development
Developing effective vaccines against P. aeruginosa infections is a crucial strategy to mitigate their consequences and reduce the excessive use of antibiotics, which can lead to antibiotic resistance. Various types of vaccines are under development to enhance the immune response against different elements involved in the infection process. These vaccines often target components of the bacterial surface, such as Opr, and various polysaccharides, including LPS, mucoid exopolysaccharide, and O-polysaccharides (Sharma et al., 2011). Additionally, they focus on structures critical for P. aeruginosa ‘s adhesion and movement, such as flagella and pili, as well as several virulence factors like TTSS, exotoxin A, or proteases. Developing effective vaccines for P. aeruginosa is challenging due to the substantial variability among Pseudomonas species, the complexity of the infection process, and the intricate interaction between the pathogen and the host immune response (Killough et al., 2022). During phases I, II, and III studies, certain vaccine candidates have proven insufficient in providing broad coverage against different P. aeruginosa strains or have exhibited low immunogenicity or safety concerns. Currently, research is ongoing to develop novel P. aeruginosa vaccines. For example, a novel P. aeruginosa vaccine called PcrV28-294-OprI25-83-Hcp11-162 (POH) has been evaluated for its protective efficacy. This vaccine contains components like PcrV, OprI, and a vital part of the type VI secretion system, Hcp1 (Yang et al., 2017). Studies showed that POH vaccination significantly triggered T-cell responses, proliferation, and protected mice against clinical P. aeruginosa strains. The development of multivalent vaccines is also being explored as a potential means of protecting against P. aeruginosa infections in the future. Furthermore, efforts have been directed toward vaccines that target notorious virulence factors responsible for evading the host immune response. Different formulations of LPS extracts have been experimented in clinical trials since 1970 including Pseudogen® and although showing some success in reducing mortality and sepsis in burn patients, it did not show clinical efficacy and did not show any reduction in colonization in CF patients (Langford and Hiller, 1984). Aerugen® was another successful candidate consisting of a component of LPS, the O-polysaccharide from eight P. aeruginosa serotypes and exotoxin A and showed favorable outcomes in CF patients (Lang et al., 2004). One promising approach involves using LPS and Oligopolysaccharides (OPS) antigens conjugated with Poly Lactic-co-Glycolic Acid (PLGA) nanoparticles as nano-vaccines. These nano-vaccines have shown the potential to stimulate both cellular and humoral immune responses against P. aeruginosa infections (Wusiman et al., 2022). Additionally, nano-vaccines containing antigens from bacterial lysates of P. aeruginosa and membrane antigens from double-layered membrane vesicles have demonstrated efficiency in preventing infections by drug-resistant P. aeruginosa strains. Alginate, a significant contributor to P. aeruginosa pathogenesis, is also being explored as a target for therapeutic vaccines. Mannuronic acid tetra saccharide, an antigen epitope, has been used to induce immunity against P. aeruginosa in mice. Hybrid proteins composed of the full-length V-antigen (PcrV) and the C-terminal domain of exoenzyme S (ExoS) from P. aeruginosa, along with adjuvants like alum and monophosphoryl Lipid A, have been considered as vaccine candidates to protect against urinary tract infections caused by P. aeruginosa (Asadi Karam et al., 2022). Whole-cell vaccines inactivated by X-ray irradiation, containing nucleic acids and 8-hydroxyguanosine, have demonstrated humoral and innate immune responses, leading to reduced infection levels in mouse models (Ma et al., 2021). Moreover, the concept of multi-target antigens has been explored. Triple-target antigens have been designed to stimulate protective and neutralizing antibodies against multiple pathogens, including P. aeruginosa (Inoue et al., 2023). These antigens combine epitopes from different bacterial species and have the potential to provide broad-spectrum protection. Vaccines represent a promising strategy for preventing P. aeruginosa infections and reducing their impact. They can induce protective responses through active or passive immunization and, in some cases, may even cross-react to protect against related pathogens. Although no effective vaccine is currently available for clinical use despite studies being performed over the last 50 years, the development of glycoconjugate vaccines, protein engineering techniques, and innovative carrier proteins has opened new avenues for creating effective vaccines against P. aeruginosa. Progress has been achieved in pre-clinical studies including antigen discovery, adjuvant use, and novel delivery systems, however, the wealth of virulence factors, its large genome size and great adaptability, and its changing phenotypes across acute and chronic infections all form challenges for vaccine development (Killough et al., 2022).
5.5 Monoclonal antibodies production
Monoclonal antibodies (mAbs) have emerged as a valuable strategy in combatting P. aeruginosa infections, particularly for high-risk individuals who may not benefit from vaccines or traditional antibiotics (Kang et al., 2023). These mAbs provide an alternative treatment avenue by directly targeting P. aeruginosa and offering immediate protection. They can be especially effective when used alongside prophylactic vaccines. One notable application of mAbs is their ability to tackle antimicrobial resistance within biofilms, where bacteria reside. These antibodies have been developed to disrupt protective biofilms, making P. aeruginosa and its co-pathogens vulnerable to subsequent antibiotic treatment. Additionally, mAbs targeting specific epitopes like DNABII proteins or type IV pilus have shown promise in impairing biofilms formed by P. aeruginosa and related pathogens (Sanya et al., 2023). Chicken egg yolk immunoglobulins, known as IgY antibodies, have garnered special attention for passive immunization due to their unique properties. IgY antibodies do not cross-react with mammalian IgG or activate the complement system. They offer high antigen-specific production yields without causing disease resistance and can be easily administered to humans. For example, IgY antibodies raised against the T3SS translocating protein PcrV from P. aeruginosa have demonstrated enhanced bacterial killing and reduced invasion in murine models of acute pneumonia and burn wounds (Ranjbar et al., 2019). Moreover, a synergistic effect has been observed when combining anti-P. aeruginosa IgY with beta-lactam antibiotics, suggesting a potential combination therapy for MDR P. aeruginosa infections. In severe cases of P. aeruginosa pneumonia, mAbs can be coupled with antibiotics to enhance therapeutic efficacy (Sanches et al., 2022). DNA-delivered monoclonal antibodies (DMAbs) produced in vivo have been effective in protecting mice from lethal pneumonia caused by aggressive P. aeruginosa strains (Patel et al., 2017). These antibodies not only reduced bacterial colonization but also acted synergistically with commonly used antibiotics, such as meropenem. Importantly, they exhibited stability and hold promise for treating high-risk patients, including those with chronic diseases and infections refractory to broad-spectrum antibiotics. However, it’s crucial to acknowledge that therapeutic monoclonal antibodies have their limitations. For instance, some mAbs, like MEDI3902, have not proven effective in mitigating P. aeruginosa nosocomial pneumonia in clinical trials (Chastre et al., 2022). Additionally, immunogenicity and protective efficacy can vary based on the antibody type and dosing. For example, IgY antibodies may protect certain infection models but not others, depending on the dose and specific target. Current research efforts are focused on using mAbs to prevent infections in high-risk patients and modulate virulence rather than solely aiming for bacterial clearance. Several mAbs are in various stages of development and clinical trials, targeting different aspects of P. aeruginosa infections, such as protein secretion systems and exopolysaccharides. These mAbs hold promise as adjunctive strategies in managing P. aeruginosa infections, particularly in critical care settings.
5.6 Nanoparticles
Nanoparticles have emerged as a promising frontier in the battle against P. aeruginosa. These minuscule materials, typically measuring less than 100 nanometers, have attracted significant attention for their potential in treating P. aeruginosa infections. Their diminutive size and high surface area-to-mass ratio make them ideal candidates for a wide range of chemical, biological, and biomedical applications (Al Saqr et al., 2021; Varma et al., 2023). One key advantage of nanoparticles is their ability to penetrate the protective barriers of bacterial cells. P. aeruginosa possesses an outer membrane rich in LPS, creating a formidable defense. However, researchers have developed methods to breach this barrier, allowing nanoparticles to access the bacterial cell wall. One technique involves using EDTA, a chelating agent that removes divalent cations stabilizing the outer membrane. This disruption leaves the membrane vulnerable to nanoparticle attack, allowing them to effectively target and combat P. aeruginosa. Another approach involves engineering nanoparticles to carry antimicrobial agents directly to their target. Porous silicon nanoparticles, for instance, have been designed to transport novel antimicrobial peptides fused with synthetic bacterial toxins. These engineered nanoparticles have demonstrated the ability to enhance survival rates and bacterial clearance in mouse models of P. aeruginosa lung infections (Patel and Hunt, 2023). Moreover, nanoparticles can serve as carriers for antibiotics, significantly augmenting their efficacy. Silver nanoparticles, when coupled with antibiotics like ampicillin, exhibit enhanced killing rates of ampicillin-resistant P. aeruginosa isolates in vitro. Silver nanoparticles, in particular, have shown remarkable promise as antimicrobial agents against P. aeruginosa. They release silver ions, which disrupt crucial bacterial enzymatic systems, including DNA synthesis, leading to bacterial cell death (Palau et al., 2023). Notably, these silver nanoparticles have demonstrated significant antimicrobial effects on clinical strains of P. aeruginosa, effectively eradicating the bacterium and inhibiting its growth in laboratory settings. Furthermore, they exhibit low cytotoxicity toward mammalian cells, a crucial consideration for potential clinical applications. Additionally, nanoparticles have been used as antigen delivery systems as a cost-effective approach to vaccine development. However, it’s important to recognize that the use of nanoparticles is not without challenges. Their reactivity, stemming from their high surface area, can lead to unintended reactions within the human body. Nanoparticles can also migrate to distant organs, potentially causing systemic toxicity. Inhalation of nanoparticles has been associated with pulmonary inflammation and potential cardiovascular effects, making their use in treating bacterial pulmonary infections a matter of careful consideration (Missaoui et al., 2018). To advance the field, future research should focus on material selection, nanoparticle size, and administered doses to optimize their therapeutic efficacy while minimizing potential risks in the clinical aspect.
6 Conclusion and future directions
Mitigating the resistance of Pseudomonas aeruginosa is a formidable challenge, prompting the exploration of innovative therapeutic avenues. Future directions in research and treatment focus on advanced adjuvants and combination therapy. Although innovative therapies exhibit advantages over current antibiotics, some limitations still persist. Notably, we view endolysins or enzybiotics as superior, owing to their efficacy in combatting bacterial resistance. The challenge of penetrating P. aeruginosa’s outer membrane is addressed through proposed solutions. Fusion with antimicrobial peptides enhances endolysins’ effectiveness, targeting the bacterium’s cell wall and inner membrane. Administering endolysins with permeabilizer agents facilitates membrane passage, optimizing antimicrobial effects. Precision medicine emerges as a promising future direction, leveraging genomic profiling for tailored treatments. Real-time antibiograms aid clinicians in data-driven decisions based on strain-specific resistance profiles. This personalized approach optimizes treatment outcomes and restricts unnecessary antibiotic use. The potential of phage therapy, biotechnology, and synthetic antimicrobial peptides in combating P. aeruginosa infections is underscored. Bacteriophages offer targeted solutions, with ongoing research into identifying and engineering phages with broad-spectrum activity. Biotechnological advancements enable synthetic peptides to disrupt bacterial resistance effectively.
In conclusion, managing P. aeruginosa infections remains a complex challenge. Its adaptability and antibiotic resistance mechanisms, resulting in multidrug-resistant strains, hinder conventional treatments. Biofilm formation and persistent cells contribute to persistent infections, especially in conditions like cystic fibrosis. Advances in antibiotics and drug delivery methods face continual challenges from P. aeruginosa ability to develop novel resistance mechanisms. The global misuse of antibiotics intensifies the need for alternative therapies. Non-antibiotic strategies, including quorum sensing inhibition, phage therapy, and nanoparticles, show promise but face translation hurdles. The multifaceted battle requires combining innovative strategies with traditional antibiotics. Combination therapies, especially for immunocompromised patients, hold potential.
Author contributions
AE: Conceptualization, Supervision, Investigation, Resources, Visualization, Writing – original draft, Writing – review & editing. RR: Data curation, Resources, Writing – original draft, Writing – review & editing. MaA: Writing – review & editing, Supervision, Investigation, Resources, Funding acquisition. FB: Formal analysis, Investigation, Writing – original draft, Writing – review & editing. EI-A: Data curation, Investigation, Resources, Writing – original draft, Writing – review & editing. AF: Investigation, Resources, Writing – original draft, Writing – review & editing. AH: Data curation, Investigation, Writing – original draft, Writing – review & editing. PR-E: Investigation, Resources, Supervision, Writing – original draft, Writing – review & editing. MoA: Data curation, Investigation, Writing – original draft, Writing – review & editing. SAZ: Writing – review & editing, Supervision, Investigation, Resources, Funding acquisition. WN: Conceptualization, Investigation, Visualization, Resources, Writing – original draft, Writing – review & editing.
Funding
The author(s) declare financial support was received for the research, authorship, and/or publication of this article. No competing financial interests have been declared. Funding for this work was provided by the Project MECESUP UCT 0804.
Acknowledgments
The authors would like to thank Damanhour University and the Mission Sector, Ministry of Higher Education, Egypt for accomplishing the current work (Program 2018/2019). The authors also thank the Princess Nourah bint Abdulrahman University Researchers Supporting Project number (PNURSP2024R182), Princess Nourah bint Abdulrahman University, Riyadh, Saudi Arabia.
Conflict of interest
The authors declare that the research was conducted in the absence of any commercial or financial relationships that could be construed as a potential conflict of interest.
Publisher’s note
All claims expressed in this article are solely those of the authors and do not necessarily represent those of their affiliated organizations, or those of the publisher, the editors and the reviewers. Any product that may be evaluated in this article, or claim that may be made by its manufacturer, is not guaranteed or endorsed by the publisher.
References
Abdulwahab, A., Zahraldin, K., Sid Ahmed, M. A., Jarir, S. A., Muneer, M., Mohamed, S. F., et al. (2017). The emergence of multidrug-resistant Pseudomonas aeruginosa in cystic fibrosis patients on inhaled antibiotics. Lung India 34, 527–531. doi: 10.4103/lungindia.lungindia_39_17
Al Saqr, A., Khafagy, E.-S., Alalaiwe, A., Aldawsari, M. F., Alshahrani, S. M., Anwer, M. K., et al. (2021). Synthesis of gold nanoparticles by using green machinery: characterization and in vitro toxicity. Nano 11:808. doi: 10.3390/nano11030808
Ambreetha, S., and Singh, V. (2023). Genetic and environmental determinants of surface adaptations in Pseudomonas aeruginosa. Microbiology (Reading) 169:001335. doi: 10.1099/mic.0.001335
Asadi Karam, M. R., Badmasti, F., Ahmadi, K., and Habibi, M. (2022). Vaccination of mice with hybrid protein containing exotoxin S and PcrV with adjuvants alum and MPL protects Pseudomonas aeruginosa infections. Sci. Rep. 12:1325. doi: 10.1038/s41598-022-05157-3
Avakh, A., Grant, G. D., Cheesman, M. J., Kalkundri, T., and Hall, S. (2023). The art of war with Pseudomonas aeruginosa: targeting Mex efflux pumps directly to strategically enhance antipseudomonal drug efficacy. Antibiotics 12:1304. doi: 10.3390/antibiotics12081304
Balakrishnan, VS. (2022). WHO’s first global infection prevention and control report. Lancet Infect Dis. 22:1122. doi: 10.1016/S1473-3099(22)00459-5
Bassetti, M., Castaldo, N., Cattelan, A., Mussini, C., Righi, E., Tascini, C., et al. (2019). Ceftolozane/tazobactam for the treatment of serious Pseudomonas aeruginosa infections: a multicentre nationwide clinical experience. Int. J. Antimicrob. Agents 53, 408–415. doi: 10.1016/j.ijantimicag.2018.11.001
Bassetti, M., and Garau, J. (2021). Current and future perspectives in the treatment of multidrug-resistant gram-negative infections. J. Antimicrob. Chemother. 76, IV23–IV37. doi: 10.1093/jac/dkab352
Bhardwaj, S., Bhatia, S., Singh, S., and Franco, F. (2021). Growing emergence of drug-resistant Pseudomonas aeruginosa and attenuation of its virulence using quorum sensing inhibitors: a critical review. Iran. J. Basic Med. Sci. 24, 699–719. doi: 10.22038/IJBMS.2021.49151.11254
Bhat, B. A., Mir, R. A., Qadri, H., Dhiman, R., Almilaibary, A., Alkhanani, M., et al. (2023). Integrons in the development of antimicrobial resistance: critical review and perspectives. Front. Microbiol. 14:1231938. doi: 10.3389/fmicb.2023.1231938
Bongiovanni, M., and Barda, B. (2023). Pseudomonas aeruginosa bloodstream infections in SARS-CoV-2 infected patients: a systematic review. J. Clin. Med. 12:2252. doi: 10.3390/jcm12062252
Boyd, A., and Chakrabarty, A. M. (1995). Pseudomonas aeruginosa biofilms: role of the alginate exopolysaccharide. J. Ind. Microbiol. 15, 162–168. doi: 10.1007/BF01569821
Bush, K., and Bradford, P. A. (2016). β-Lactams and β-lactamase inhibitors: an overview. Cold Spring Harb. Perspect. Med. 6:a025247. doi: 10.1101/cshperspect.a025247
Caffrey, A. R., Appaneal, H. J., Liao, J. X., Piehl, E. C., Lopes, V., and Puzniak, L. A. (2022). Treatment heterogeneity in Pseudomonas aeruginosa pneumonia. Antibiotics 11:1033. doi: 10.3390/antibiotics11081033
Castanheira, M., Simner, P. J., and Bradford, P. A. (2021). Extended-spectrum β-lactamases: an update on their characteristics, epidemiology and detection. JAC-Antimicrobial Resist 3:dlab092. doi: 10.1093/jacamr/dlab092
Chan, K. G., Liu, Y. C., and Chang, C. Y. (2015). Inhibiting N-acyl-homoserine lactone synthesis and quenching Pseudomonas quinolone quorum sensing to attenuate virulence. Front. Microbiol. 6:1173. doi: 10.3389/fmicb.2015.01173
Chan, B. K., Sistrom, M., Wertz, J. E., Kortright, K. E., Narayan, D., and Turner, P. E. (2016). Phage selection restores antibiotic sensitivity in MDR Pseudomonas aeruginosa. Sci. Rep. 6:26717. doi: 10.1038/srep26717
Chastre, J., François, B., Bourgeois, M., Komnos, A., Ferrer, R., Rahav, G., et al. (2022). Safety, efficacy, and pharmacokinetics of gremubamab (MEDI3902), an anti-Pseudomonas aeruginosa bispecific human monoclonal antibody, in P. aeruginosa-colonised, mechanically ventilated intensive care unit patients: a randomised controlled trial. Crit. Care 26:355. doi: 10.1186/s13054-022-04204-9
Cho, H., Uehara, T., and Bernhardt, T. G. (2014). Beta-lactam antibiotics induce a lethal malfunctioning of the bacterial cell wall synthesis machinery. Cell 159, 1300–1311. doi: 10.1016/j.cell.2014.11.017
Choudhuri, A. H., Chakravarty, M., and Uppal, R. (2017). Epidemiology and characteristics of nosocomial infections in critically ill patients in a tertiary care intensive care unit of northern India. Saudi J Anaesth 11, 402–407. doi: 10.4103/sja.SJA_230_17
Cigana, C., Castandet, J., Sprynski, N., Melessike, M., Beyria, L., Ranucci, S., et al. (2021). Pseudomonas aeruginosa elastase contributes to the establishment of chronic lung colonization and modulates the immune response in a murine model. Front. Microbiol. 11:620819. doi: 10.3389/fmicb.2020.620819
Colvin, K. M., Irie, Y., Tart, C. S., Urbano, R., Whitney, J. C., Ryder, C., et al. (2012). The Pel and Psl polysaccharides provide Pseudomonas aeruginosa structural redundancy within the biofilm matrix. Environ. Microbiol. 14, 1913–1928. doi: 10.1111/j.1462-2920.2011.02657.x
Curran, B., Jonas, D., Grundmann, H., Pitt, T., and Dowson, C. G. (2004). Development of a multilocus sequence typing scheme for the opportunistic pathogen Pseudomonas aeruginosa. J. Clin. Microbiol. 42, 5644–5649. doi: 10.1128/JCM.42.12.5644-5649.2004
de Sousa, T., Garcês, A., Silva, A., Lopes, R., Alegria, N., Hébraud, M., et al. (2023). The impact of the virulence of Pseudomonas aeruginosa isolated from dogs. Vet. Sci. 10:343. doi: 10.3390/vetsci10050343
del Barrio-Tofiño, E., López-Causapé, C., and Oliver, A. (2020). Pseudomonas aeruginosa epidemic high-risk clones and their association with horizontally-acquired β-lactamases: 2020 update. Int. J. Antimicrob. Agents 56:106196. doi: 10.1016/j.ijantimicag.2020.106196
Dos Santos, P. A. S., Rodrigues, Y. C., Marcon, D. J., Lobato, A. R. F., Cazuza, T. B., Gouveia, M. I. M., et al. (2023). Endemic high-risk clone ST277 is related to the spread of SPM-1-producing Pseudomonas aeruginosa during the COVID-19 pandemic period in northern Brazil. Microorganisms 11:2069. doi: 10.3390/microorganisms11082069
Dreier, J., and Ruggerone, P. (2015). Interaction of antibacterial compounds with RND efflux pumps in Pseudomonas aeruginosa. Front. Microbiol. 6:660. doi: 10.3389/fmicb.2015.00660
Durán, O., Ramos, C., Chen, O., Castillo, J., de Mayorga, B., and de Chial, M. (2022). “Pyoverdine as an important virulence factor in Pseudomonas aeruginosa antibiotic resistance” in The global antimicrobial resistance epidemic—innovative approaches and cutting-edge solutions. ed. G. Tellez-Isaias (Rijeka: IntechOpen) Ch. 8.
ECDC (2021). Antimicrobial resistance in the EU/EEA (EARS-net)—Annual epidemiological report 2019. ECDC, Stock.
Elfadadny, A., Ragab, R. F., Hamada, R., Al Jaouni, S. K., Fu, J., Mousa, S. A., et al. (2023a). Natural bioactive compounds-doxorubicin combinations targeting topoisomerase II-alpha: anticancer efficacy and safety. Toxicol. Appl. Pharmacol. 461:116405. doi: 10.1016/j.taap.2023.116405
Elfadadny, A., Uchiyama, J., Goto, K., Imanishi, I., Ragab, R. F., Nageeb, W. M., et al. (2023b). Antimicrobial resistance and genotyping of Pseudomonas aeruginosa isolated from the ear canals of dogs in Japan. Front. Vet. Sci. 10:1074127. doi: 10.3389/fvets.2023.1074127
Eltayb, E. K., Aleanizy, F. S., Alqahtani, F. Y., Alkahtani, H. M., Ansari, S. A., and Alsarra, I. (2022). Preparation and characterization of Meta-bromo-thiolactone calcium alginate nanoparticles. Saudi Pharm. J. 30, 946–953. doi: 10.1016/j.jsps.2022.05.008
European Centre for Disease Control and Prevention (2015). Annual epidemiological report: Antimicrobial resistance and healthcare-associated infections 2014. Stockholm: ECDC, Stock.
Fang, Z., Zhang, L. Y., Huang, Y. M., Qing, Y., Cao, K. Y., Tian, G. B., et al. (2014). OprD mutations and inactivation in imipenem-resistant Pseudomonas aeruginosa isolates from China. Infect. Genet. Evol. 21, 124–128. doi: 10.1016/j.meegid.2013.10.027
Farzin, A., Mizanur Rahman, M., and Ara Mollika, F. (2023). “Pseudomonas aeruginosa: the alarming pathogen of hospital acquired infection” in Pseudomonas aeruginosa—new perspectives and applications [working title]. eds. A. P. O. M. Darwesh and D. I. Matter (Rijeka: IntechOpen) Ch. 7
Foucrier, A., Dessalle, T., Tuffet, S., Federici, L., Dahyot-Fizelier, C., Barbier, F., et al. (2023). Association between combination antibiotic therapy as opposed as monotherapy and outcomes of ICU patients with Pseudomonas aeruginosa ventilator-associated pneumonia: an ancillary study of the iDIAPASON trial. Crit. Care 27:211. doi: 10.1186/s13054-023-04457-y
Fu, W., Forster, T., Mayer, O., Curtin, J. J., Lehman, S. M., and Donlan, R. M. (2010). Bacteriophage cocktail for the prevention of biofilm formation by Pseudomonas aeruginosa on catheters in an in vitro model system. Antimicrob. Agents Chemother. 54, 397–404. doi: 10.1128/AAC.00669-09
Fujiwara, M., Yamasaki, S., Morita, Y., and Nishino, K. (2022). Evaluation of efflux pump inhibitors of MexAB-or MexXY-OprM in Pseudomonas aeruginosa using nucleic acid dyes. J. Infect. Chemother. 28, 595–601. doi: 10.1016/j.jiac.2022.01.003
Furusawa, T., Iwano, H., Higuchi, H., Yokota, H., Usui, M., Iwasaki, T., et al. (2016). Bacteriophage can lyse antibiotic-resistant Pseudomonas aeruginosa isolated from canine diseases. J. Vet. Med. Sci. 78, 1035–1038. doi: 10.1292/jvms.15-0310
Garcia-Clemente, M., de la Rosa, D., Máiz, L., Girón, R., Blanco, M., Olveira, C., et al. (2020). Impact of Pseudomonas aeruginosa infection on patients with chronic inflammatory airway diseases. J. Clin. Med. 9:3800. doi: 10.3390/jcm9123800
Gaynes, R., and Edwards, J. R. (2005). Overview of nosocomial infections caused by gram-negative bacilli. Clin. Infect. Dis. 41, 848–854. doi: 10.1086/432803
Gonzalez, M. R., Fleuchot, B., Lauciello, L., Jafari, P., Applegate, L. A., Raffoul, W., et al. (2016). Effect of Human burn wound exudate on Pseudomonas aeruginosa virulence. mSphere 1:e00111. doi: 10.1128/msphere.00111-15
Grosso-Becerra, M. V., Santos-Medellín, C., González-Valdez, A., Méndez, J. L., Delgado, G., Morales-Espinosa, R., et al. (2014). Pseudomonas aeruginosa clinical and environmental isolates constitute a single population with high phenotypic diversity. BMC Genomics 15:318. doi: 10.1186/1471-2164-15-318
Guo, M., Feng, C., Ren, J., Zhuang, X., Zhang, Y., Zhu, Y., et al. (2017). A novel antimicrobial endolysin, LysPA26, against Pseudomonas aeruginosa. Front. Microbiol. 8:293. doi: 10.3389/fmicb.2017.00293
Gurney, J., Pradier, L., Griffin, J. S., Gougat-Barbera, C., Chan, B. K., Turner, P. E., et al. (2020). Phage steering of antibiotic-resistance evolution in the bacterial pathogen, Pseudomonas aeruginosa. Evol. Med. Public Heal. 2020, 148–157. doi: 10.1093/EMPH/EOAA026
Gutu, A. D., Sgambati, N., Strasbourger, P., Brannon, M. K., Jacobs, M. A., Haugen, E., et al. (2013). Polymyxin resistance of Pseudomonas aeruginosa phoQ mutants is dependent on additional two-component regulatory systems. Antimicrob. Agents Chemother. 57, 2204–2215. doi: 10.1128/AAC.02353-12
Hafiz, T. A., Bin Essa, E. A., Alharbi, S. R., Alyami, A. S., Alkudmani, Z. S., Mubaraki, M. A., et al. (2023). Epidemiological, microbiological, and clinical characteristics of multi-resistant Pseudomonas aeruginosa isolates in king Fahad Medical City, Riyadh, Saudi Arabia. Trop. Med. Infect. Dis. 8:205. doi: 10.3390/tropicalmed8040205
Hagens, S., Habel, A., Von Ahsen, U., Von Gabain, A., and Bläsi, U. (2004). Therapy of experimental Pseudomonas infections with a nonreplicating genetically modified phage. Antimicrob. Agents Chemother. 48, 3817–3822. doi: 10.1128/AAC.48.10.3817-3822.2004
Haidar, G., Philips, N. J., Shields, R. K., Snyder, D., Cheng, S., Potoski, B. A., et al. (2017). Ceftolozane-Tazobactam for the treatment of multidrug-resistant Pseudomonas aeruginosa infections: clinical effectiveness and evolution of resistance. Clin. Infect. Dis. 65, 110–120. doi: 10.1093/cid/cix182
Hall, S., McDermott, C., Anoopkumar-Dukie, S., McFarland, A. J., Forbes, A., Perkins, A. V., et al. (2016). Cellular effects of pyocyanin, a secreted virulence factor of Pseudomonas aeruginosa. Toxins (Basel) 8:236. doi: 10.3390/toxins8080236
Hardy, K. S., Tessmer, M. H., Frank, D. W., and Audia, J. P. (2021). Perspectives on the pseudomonas aeruginosa type iii secretion system effector exou and its subversion of the host innate immune response to infection. Toxins (Basel) 13:880. doi: 10.3390/toxins13120880
Hematzadeh, A., and Haghkhah, M. (2021). Biotyping of isolates of Pseudomonas aeruginosa isolated from human infections by RAPD and ERIC-PCR. Heliyon 7:e07967. doi: 10.1016/j.heliyon.2021.e07967
Hernando-Amado, S., Alcalde-Rico, M., Gil-Gil, T., Valverde, J. R., and Martínez, J. L. (2020). Naringenin inhibition of the Pseudomonas aeruginosa quorum sensing response is based on its time-dependent competition with N-(3-Oxo-dodecanoyl)-L-homoserine lactone for LasR binding. Front. Mol. Biosci. 7:25. doi: 10.3389/fmolb.2020.00025
Holmes, C. L., Anderson, M. T., Mobley, H. L. T., and Bachman, M. A. (2021). Pathogenesis of gram-negative bacteremia. Clin. Microbiol. Rev. 34:e00234. doi: 10.1128/CMR.00234-20
Hooper, D. C., and Jacoby, G. A. (2016). Topoisomerase inhibitors: fluoroquinolone mechanisms of action and resistance. Cold Spring Harb. Perspect. Med. 6:a025320. doi: 10.1101/cshperspect.a025320
Horcajada, J. P., Montero, M., Oliver, A., Sorlí, L., Luque, S., Gómez-Zorrilla, S., et al. (2019). Epidemiology and treatment of multidrug-resistant and extensively drug-resistant Pseudomonas aeruginosa infections. Clin. Microbiol. Rev. 32:e00031. doi: 10.1128/CMR.00031-19
Huang, L., Wu, C., Gao, H., Xu, C., Dai, M., Huang, L., et al. (2022). Bacterial multidrug efflux pumps at the frontline of antimicrobial resistance: an overview. Antibiotics 11:520. doi: 10.3390/antibiotics11040520
Inoue, K., Kinoshita, M., Muranishi, K., Ohara, J., Sudo, K., Kawaguchi, K., et al. (2023). Effect of a novel trivalent vaccine formulation against acute lung injury caused by Pseudomonas aeruginosa. Vaccine 11:1088. doi: 10.3390/vaccines11061088
Jaglan, A. B., Anand, T., Verma, R., Vashisth, M., Virmani, N., Bera, B. C., et al. (2022). Tracking the phage trends: a comprehensive review of applications in therapy and food production. Front. Microbiol. 13:993990. doi: 10.3389/fmicb.2022.993990
Juan, C., Torrens, G., González-Nicolau, M., and Oliver, A. (2017). Diversity and regulation of intrinsic β-lactamases from non-fermenting and other gram-negative opportunistic pathogens. FEMS Microbiol. Rev. 41, 781–815. doi: 10.1093/femsre/fux043
Jurado-Martín, I., Sainz-Mejías, M., and McClean, S. (2021). Pseudomonas aeruginosa: an audacious pathogen with an adaptable arsenal of virulence factors. Int. J. Mol. Sci. 22, 1–37. doi: 10.3390/ijms22063128
Kanak, K. R., Dass, R. S., and Pan, A. (2023). Anti-quorum sensing potential of selenium nanoparticles against LasI/R, RhlI/R, and PQS/MvfR in Pseudomonas aeruginosa: a molecular docking approach. Front. Mol. Biosci. 10:1203672. doi: 10.3389/fmolb.2023.1203672
Kang, J., Mateu-Borrás, M., Monroe, H. L., Sen-Kilic, E., Miller, S. J., Dublin, S. R., et al. (2023). Monoclonal antibodies against lipopolysaccharide protect against Pseudomonas aeruginosa challenge in mice. Front. Cell. Infect. Microbiol. 13:1191806. doi: 10.3389/fcimb.2023.1191806
Khayat, M. T., Abbas, H. A., Ibrahim, T. S., Khayyat, A. N., Alharbi, M., Darwish, K. M., et al. (2022). Anti-quorum sensing activities of gliptins against Pseudomonas aeruginosa and Staphylococcus aureus. Biomedicines 10:1169. doi: 10.3390/biomedicines10051169
Killough, M., Rodgers, A. M., and Ingram, R. J. (2022). Pseudomonas aeruginosa: Recent Advances in Vaccine Development. Vaccine 10:1100. doi: 10.3390/vaccines10071100
Krause, K. M., Serio, A. W., Kane, T. R., and Connolly, L. E. (2016). Aminoglycosides: an overview. Cold Spring Harb. Perspect. Med. 6:a027029. doi: 10.1101/cshperspect.a027029
Kritsotakis, E. I., Kontopidou, F., Astrinaki, E., Roumbelaki, M., Ioannidou, E., and Gikas, A. (2017). Prevalence, incidence burden, and clinical impact of healthcare-associated infections and antimicrobial resistance: a national prevalent cohort study in acute care hospitals in Greece. Infect Drug Resist 10, 317–328. doi: 10.2147/IDR.S147459
Kühn, M. J., Talà, L., Inclan, Y. F., Patino, R., Pierrat, X., Vos, I., et al. (2021). Mechanotaxis directs Pseudomonas aeruginosa twitching motility. Proc. Natl. Acad. Sci. USA 118:e2101759118. doi: 10.1073/pnas.2101759118
Laborda, P., Sanz-García, F., Hernando-Amado, S., and Martínez, J. L. (2021). Pseudomonas aeruginosa: an antibiotic resilient pathogen with environmental origin. Curr. Opin. Microbiol. 64, 125–132. doi: 10.1016/j.mib.2021.09.010
Lai, W. C. B., Chen, X., Ho, M. K. Y., Xia, J., and Leung, S. S. Y. (2020). Bacteriophage-derived endolysins to target gram-negative bacteria. Int. J. Pharm. 589:119833. doi: 10.1016/j.ijpharm.2020.119833
Lang, A. B., Rüdeberg, A., Schöni, M. H., Que, J. U., Fürer, E., and Schaad, U. B. (2004). Vaccination of cystic fibrosis patients against Pseudomonas aeruginosa reduces the proportion of patients infected and delays time to infection. Pediatr. Infect. Dis. J. 23, 504–510. doi: 10.1097/01.inf.0000129688.50588.ac
Langendonk, R. F., Neill, D. R., and Fothergill, J. L. (2021). The building blocks of antimicrobial resistance in Pseudomonas aeruginosa: implications for current resistance-breaking therapies. Front. Cell. Infect. Microbiol. 11:665759. doi: 10.3389/fcimb.2021.665759
Langford, D. T., and Hiller, J. (1984). Prospective, controlled study of a polyvalent pseudomonas vaccine in cystic fibrosis--three year results. Arch. Dis. Child. 59, 1131–1134. doi: 10.1136/adc.59.12.1131
Leroy, O., D’Escrivan, T., Devos, P., Dubreuil, L., Kipnis, E., and Georges, H. (2005). Hospital-acquired pneumonia in critically III patients: factors associated with episodes due to imipenem-resistant organisms. Infection 33, 129–135. doi: 10.1007/s15010-005-4021-8
Lesic, B., Starkey, M., He, J., Hazan, R., and Rahme, L. G. (2009). Quorum sensing differentially regulates Pseudomonas aeruginosa type VI secretion locus I and homologous loci II and III, which are required for pathogenesis. Microbiology 155, 2845–2855. doi: 10.1099/mic.0.029082-0
Li, Y., Roberts, J. A., Walker, M. M., Aslan, A. T., Harris, P. N. A., and Sime, F. B. (2024). The global epidemiology of ventilator-associated pneumonia caused by multi-drug resistant Pseudomonas aeruginosa: a systematic review and meta-analysis. Int. J. Infect. Dis. 139, 78–85. doi: 10.1016/j.ijid.2023.11.023
Limoli, D. H., Jones, C. J., and Wozniak, D. J. (2015). Bacterial extracellular polysaccharides in biofilm formation and function. Microbiol. Spectr. 3:10.1128. doi: 10.1128/microbiolspec.mb-0011-2014
Lorusso, A. B., Carrara, J. A., Barroso, C. D. N., Tuon, F. F., and Faoro, H. (2022). Role of efflux pumps on antimicrobial resistance in Pseudomonas aeruginosa. Int. J. Mol. Sci. 23:15779. doi: 10.3390/ijms232415779
Lyczak, J. B., Cannon, C. L., and Pier, G. B. (2000). Establishment of Pseudomonas aeruginosa infection: lessons from a versatile opportunist. Microbes Infect. 2, 1051–1060. doi: 10.1016/S1286-4579(00)01259-4
Ma, C., Ma, X., Jiang, B., Pan, H., Liao, X., Zhang, L., et al. (2021). A novel inactivated whole-cell Pseudomonas aeruginosa vaccine that acts through the cGAS-STING pathway. Signal Transduct. Target. Ther. 6:353. doi: 10.1038/s41392-021-00752-8
Madaha, E. L., Mienie, C., Gonsu, H. K., Bughe, R. N., Fonkoua, M. C., Mbacham, W. F., et al. (2020). Whole-genome sequence of multi-drug resistant Pseudomonas aeruginosa strains UY1PSABAL and UY1PSABAL2 isolated from human broncho-alveolar lavage, Yaoundé, Cameroon. PLoS One 15:e0238390. doi: 10.1371/journal.pone.0238390
Magiorakos, A. P., Srinivasan, A., Carey, R. B., Carmeli, Y., Falagas, M. E., Giske, C. G., et al. (2012). Multidrug-resistant, extensively drug-resistant and pandrug-resistant bacteria: an international expert proposal for interim standard definitions for acquired resistance. Clin. Microbiol. Infect. 18, 268–281. doi: 10.1111/j.1469-0691.2011.03570.x
Magnet, S., Smith, T. A., Zheng, R., Nordmann, P., and Blanchard, J. S. (2003). Aminoglycoside resistance resulting from tight drug binding to an altered aminoglycoside acetyltransferase. Antimicrob. Agents Chemother. 47, 1577–1583. doi: 10.1128/AAC.47.5.1577-1583.2003
Maurice, N. M., Bedi, B., and Sadikot, R. T. (2018). Pseudomonas aeruginosa biofilms: host response and clinical implications in lung infections. Am. J. Respir. Cell Mol. Biol. 58, 428–439. doi: 10.1165/rcmb.2017-0321TR
Mekonnen, S. A., Husseini, N.El, Turdiev, A., Carter, J. A., Belew, A. T., El-Sayed, N. M., et al. (2022). Catheter-associated urinary tract infection by Pseudomonas aeruginosa progresses through acute and chronic phases of infection. Proc. Natl. Acad. Sci. USA 119,:e2209383119. doi: 10.1073/pnas.2209383119
Michaelis, C., and Grohmann, E. (2023). Horizontal gene transfer of antibiotic resistance genes in biofilms. Antibiotics 12:328. doi: 10.3390/antibiotics12020328
Michalska, M., and Wolf, P. (2015). Pseudomonas exotoxin a: optimized by evolution for effective killing. Front. Microbiol. 6:963. doi: 10.3389/fmicb.2015.00963
Michel-Briand, Y., and Baysse, C. (2002). The pyocins of Pseudomonas aeruginosa. Biochimie 84, 499–510. doi: 10.1016/S0300-9084(02)01422-0
Mikkelsen, H., Sivaneson, M., and Filloux, A. (2011). Key two-component regulatory systems that control biofilm formation in Pseudomonas aeruginosa. Environ. Microbiol. 13, 1666–1681. doi: 10.1111/j.1462-2920.2011.02495.x
Mishra, R., Kushveer, J. S., Khan, M. I. K., Pagal, S., Meena, C. K., Murali, A., et al. (2020). 2,4-Di-Tert-Butylphenol isolated from an endophytic fungus, Daldinia eschscholtzii, reduces virulence and quorum sensing in Pseudomonas aeruginosa. Front. Microbiol. 11:1668. doi: 10.3389/fmicb.2020.01668
Missaoui, W. N., Arnold, R. D., and Cummings, B. S. (2018). Toxicological status of nanoparticles: what we know and what we don’t know. Chem. Biol. Interact. 295, 1–12. doi: 10.1016/j.cbi.2018.07.015
Mittal, R., Grati, M., Yan, D., and Liu, X. Z. (2016). Pseudomonas aeruginosa activates PKC-alpha to invade middle ear epithelial cells. Front. Microbiol. 7:255. doi: 10.3389/fmicb.2016.00255
Moradali, M. F., Ghods, S., and Rehm, B. H. A. (2017). Pseudomonas aeruginosa lifestyle: a paradigm for adaptation, survival, and persistence. Front. Cell. Infect. Microbiol. 7:39. doi: 10.3389/fcimb.2017.00039
Moya, B., Dötsch, A., Juan, C., Blázquez, J., Zamorano, L., Haussler, S., et al. (2009). Β-lactam resistance response triggered by inactivation of a nonessential penicillin-binding protein. PLoS Pathog. 5:e1000353. doi: 10.1371/journal.ppat.1000353
Muggeo, A., Coraux, C., and Guillard, T. (2023). Current concepts on Pseudomonas aeruginosa interaction with human airway epithelium. PLoS Pathog. 19:e1011221. doi: 10.1371/JOURNAL.PPAT.1011221
Muhammad, M. H., Idris, A. L., Fan, X., Guo, Y., Yu, Y., Jin, X., et al. (2020). Beyond risk: bacterial biofilms and their regulating approaches. Front. Microbiol. 11:928. doi: 10.3389/fmicb.2020.00928
Mulani, M. S., Kamble, E. E., Kumkar, S. N., Tawre, M. S., and Pardesi, K. R. (2019). Emerging strategies to combat ESKAPE pathogens in the era of antimicrobial resistance: a review. Front. Microbiol. 10:539. doi: 10.3389/fmicb.2019.00539
Murray, E., Draper, L. A., Ross, R. P., and Hill, C. (2021). The advantages and challenges of using endolysins in a clinical setting. Viruses 13:680. doi: 10.3390/v13040680
Murray, C. J., Ikuta, K. S., Sharara, F., Swetschinski, L., Robles Aguilar, G., Gray, A., et al. (2022). Global burden of bacterial antimicrobial resistance in 2019: a systematic analysis. Lancet 399, 629–655. doi: 10.1016/S0140-6736(21)02724-0
Nandi, A., Yadav, R., and Singh, A. (2022). Phage derived lytic peptides, a secret weapon against Acinetobacter baumannii—an in silico approach. Front. Med. 9:1047752. doi: 10.3389/fmed.2022.1047752
Newman, J. N., Floyd, R. V., and Fothergill, J. L. (2022). Invasion and diversity in Pseudomonas aeruginosa urinary tract infections. J. Med. Microbiol. 71:001458. doi: 10.1099/jmm.0.001458
Nielsen, S. S., Bicout, D. J., Calistri, P., Canali, E., Drewe, J. A., Garin-Bastuji, B., et al. (2022). Assessment of listing and categorisation of animal diseases within the framework of the animal health law (regulation (EU) no 2016/429): antimicrobial-resistant Escherichia coli in dogs and cats, horses, swine, poultry, cattle, sheep and goats. EFSA J. 20:e07311. doi: 10.2903/j.efsa.2022.7311
Oliveira, V. D. C., Rubio, F. G., Almeida, M. T. G., Nogueira, M. C. L., Pignatari, A. C. C., Oliveira, V. D. C., et al. (2015). Trends of 9,416 multidrug-resistant gram-negative bacteria. Rev. Assoc. Med. Bras. 61, 244–249. doi: 10.1590/1806-9282.61.03.244
Pachori, P., Gothalwal, R., and Gandhi, P. (2019). Emergence of antibiotic resistance Pseudomonas aeruginosa in intensive care unit; a critical review. Genes Dis. 6, 109–119. doi: 10.1016/j.gendis.2019.04.001
Paczkowski, J. E., Mukherjee, S., McCready, A. R., Cong, J. P., Aquino, C. J., Kim, H., et al. (2017). Flavonoids suppress Pseudomonas aeruginosa virulence through allosteric inhibition of quorum-sensing receptors. J. Biol. Chem. 292, 4064–4076. doi: 10.1074/jbc.M116.770552
Pai, S., Hebbar, A., and Selvaraj, S. (2022). A critical look at challenges and future scopes of bioactive compounds and their incorporations in the food, energy, and pharmaceutical sector. Environ. Sci. Pollut. Res. 29, 35518–35541. doi: 10.1007/s11356-022-19423-4
Palau, M., Muñoz, E., Gusta, M. F., Larrosa, N., Gomis, X., Gilabert, J., et al. (2023). In vitro antibacterial activity of silver nanoparticles conjugated with amikacin and combined with hyperthermia against drug-resistant and biofilm-producing strains. Microbiol. Spectr. 11:e0028023. doi: 10.1128/spectrum.00280-23
Pang, Z., Raudonis, R., Glick, B. R., Lin, T.-J., and Cheng, Z. (2019). Antibiotic resistance in Pseudomonas aeruginosa: mechanisms and alternative therapeutic strategies. Biotechnol. Adv. 37, 177–192. doi: 10.1016/j.biotechadv.2018.11.013
Park, S., and Sauer, K. (2022). Controlling biofilm development through cyclic di-GMP signaling. Adv. Exp. Med. Biol. 1386, 69–94. doi: 10.1007/978-3-031-08491-1_3
Patel, A., Digiandomenico, A., Keller, A. E., Smith, T. R. F., Park, D. H., Ramos, S., et al. (2017). An engineered bispecific DNA-encoded IgG antibody protects against Pseudomonas aeruginosa in a pneumonia challenge model. Nat. Commun. 8:637. doi: 10.1038/s41467-017-00576-7
Patel, U., and Hunt, E. C. (2023). Recent advances in combating bacterial infections by using hybrid Nano-Systems. J. Nanotheranostics 4, 429–462. doi: 10.3390/jnt4030019
Pea, C., Cabot, G., Gómez-Zorrilla, S., Zamorano, L., Ocampo-Sosa, A., Murillas, J., et al. (2015). Influence of virulence genotype and resistance profile in the mortality of pseudomonas aeruginosa bloodstream infections. Clin. Infect. Dis. 60, 539–548. doi: 10.1093/cid/ciu866
Pires, D. P., Vilas Boas, D., Sillankorva, S., and Azeredo, J. (2015). Phage therapy: a step forward in the treatment of Pseudomonas aeruginosa infections. J. Virol. 89, 7449–7456. doi: 10.1128/jvi.00385-15
Poole, K. (2011). Pseudomonas aeruginosa: resistance to the max. Front. Microbiol. 2:65. doi: 10.3389/fmicb.2011.00065
Potron, A., Poirel, L., and Nordmann, P. (2015). Emerging broad-spectrum resistance in Pseudomonas aeruginosa and Acinetobacter baumannii: mechanisms and epidemiology. Int. J. Antimicrob. Agents 45, 568–585. doi: 10.1016/j.ijantimicag.2015.03.001
Prajapati, J. D., Kleinekathöfer, U., and Winterhalter, M. (2021). How to enter a bacterium: bacterial Porins and the permeation of antibiotics. Chem. Rev. 121, 5158–5192. doi: 10.1021/acs.chemrev.0c01213
Prudêncio, C. V., dos Santos, M. T., and Vanetti, M. C. D. (2015). Strategies for the use of bacteriocins in gram-negative bacteria: relevance in food microbiology. J. Food Sci. Technol. 52, 5408–5417. doi: 10.1007/s13197-014-1666-2
Qin, S., Xiao, W., Zhou, C., Pu, Q., Deng, X., Lan, L., et al. (2022). Pseudomonas aeruginosa: pathogenesis, virulence factors, antibiotic resistance, interaction with host, technology advances and emerging therapeutics. Signal Transduct. Target. Ther. 7:199. doi: 10.1038/s41392-022-01056-1
Ramirez, M. S., and Tolmasky, M. E. (2010). Aminoglycoside modifying enzymes. Drug Resist. Updat. 13, 151–171. doi: 10.1016/j.drup.2010.08.003
Ranjbar, M., Behrouz, B., Norouzi, F., and Mousavi Gargari, S. L. (2019). Anti-PcrV IgY antibodies protect against Pseudomonas aeruginosa infection in both acute pneumonia and burn wound models. Mol. Immunol. 116, 98–105. doi: 10.1016/j.molimm.2019.10.005
Reboud, E., Basso, P., Maillard, A. P., Huber, P., and Attrée, I. (2017). Exolysin shapes the virulence of pseudomonas aeruginosa clonal outliers. Toxins (Basel) 9:364. doi: 10.3390/toxins9110364
Reynolds, D., and Kollef, M. (2021). The epidemiology and pathogenesis and treatment of Pseudomonas aeruginosa infections: an update. Drugs 81, 2117–2131. doi: 10.1007/s40265-021-01635-6
Riera, E., Cabot, G., Mulet, X., García-Castillo, M., del Campo, R., Juan, C., et al. (2011). Pseudomonas aeruginosa carbapenem resistance mechanisms in Spain: impact on the activity of imipenem, meropenem and doripenem. J. Antimicrob. Chemother. 66, 2022–2027. doi: 10.1093/jac/dkr232
Ropy, A., Cabot, G., Sánchez-Diener, I., Aguilera, C., Moya, B., Ayala, J. A., et al. (2015). Role of Pseudomonas aeruginosa low-molecular-mass penicillin-binding proteins in AmpC expression, β-lactam resistance, and peptidoglycan structure. Antimicrob. Agents Chemother. 59, 3925–3934. doi: 10.1128/AAC.05150-14
Rosenau, F., Isenhardt, S., Gdynia, A., Tielker, D., Schmidt, E., Tielen, P., et al. (2010). Lipase LipC affects motility, biofilm formation and rhamnolipid production in Pseudomonas aeruginosa. FEMS Microbiol. Lett. 309, 25–34. doi: 10.1111/j.1574-6968.2010.02017.x
Rosenthal, V. D., Al-Abdely, H. M., El-Kholy, A. A., AlKhawaja, S. A. A., Leblebicioglu, H., Mehta, Y., et al. (2016). International nosocomial infection control consortium report, data summary of 50 countries for 2010-2015: device-associated module. Am. J. Infect. Control 44, 1495–1504. doi: 10.1016/j.ajic.2016.08.007
Roy, R., Tiwari, M., Donelli, G., and Tiwari, V. (2018). Strategies for combating bacterial biofilms: a focus on anti-biofilm agents and their mechanisms of action. Virulence 9, 522–554. doi: 10.1080/21505594.2017.1313372
Sadaqat, M. H., Mobarez, A. M., and Nikkhah, M. (2022). Curcumin carbon dots inhibit biofilm formation and expression of esp and gelE genes of Enterococcus faecium. Microb. Pathog. 173:105860. doi: 10.1016/j.micpath.2022.105860
Sadovskaya, I., Vinogradov, E., Li, J., Hachani, A., Kowalska, K., and Filloux, A. (2010). High-level antibiotic resistance in Pseudomonas aeruginosa biofilm: the ndvB gene is involved in the production of highly glycerol-phosphorylated β(1→3)-glucans, which bind aminoglycosides. Glycobiology 20, 895–904. doi: 10.1093/glycob/cwq047
Sanches, R. F., dos Santos Ferraro, A. C. N., Marroni, F. E. C., and Venancio, E. J. (2022). Synergistic activity between beta-lactams and igy antibodies against Pseudomonas aeruginosa in vitro. Mol. Immunol. 148, 1–5. doi: 10.1016/j.molimm.2022.05.010
Sanya, D. R. A., Onésime, D., Vizzarro, G., and Jacquier, N. (2023). Recent advances in therapeutic targets identification and development of treatment strategies towards Pseudomonas aeruginosa infections. BMC Microbiol. 23:86. doi: 10.1186/s12866-023-02832-x
Schütz, C., and Empting, M. (2018). Targeting the Pseudomonas quinolone signal quorum sensing system for the discovery of novel anti-infective pathoblockers. Beilstein J. Org. Chem. 14, 2627–2645. doi: 10.3762/bjoc.14.241
Secor, P. R., Sweere, J. M., Michaels, L. A., Malkovskiy, A. V., Lazzareschi, D., Katznelson, E., et al. (2015). Filamentous bacteriophage promote biofilm assembly and function. Cell Host Microbe 18, 549–559. doi: 10.1016/j.chom.2015.10.013
Serwecińska, L. (2020). Antimicrobials and antibiotic-resistant bacteria: a risk to the environment and to public health. Water (Switzerland) 12:3313. doi: 10.3390/w12123313
Sethuvel, D. P. M., Bakthavatchalam, Y. D., Karthik, M., Irulappan, M., Shrivastava, R., Periasamy, H., et al. (2023). β-Lactam resistance in ESKAPE pathogens mediated through modifications in penicillin-binding proteins: an overview. Infect. Dis. Ther. 12, 829–841. doi: 10.1007/s40121-023-00771-8
Sharma, A., Krause, A., and Worgall, S. (2011). Recent developments for Pseudomonas vaccines. Hum. Vaccin. 7, 999–1011. doi: 10.4161/hv.7.10.16369
Sharma, D., Misba, L., and Khan, A. U. (2019). Antibiotics versus biofilm: an emerging battleground in microbial communities. Antimicrob. Resist. Infect. Control 8:76. doi: 10.1186/s13756-019-0533-3
Sharma, S., Mohler, J., Mahajan, S. D., Schwartz, S. A., Bruggemann, L., and Aalinkeel, R. (2023). Microbial biofilm: a review on formation, infection, antibiotic resistance, control measures, and innovative treatment. Microorganisms 11:1614. doi: 10.3390/microorganisms11061614
Shi, Q., Huang, C., Xiao, T., Wu, Z., and Xiao, Y. (2019). A retrospective analysis of Pseudomonas aeruginosa bloodstream infections: prevalence, risk factors, and outcome in carbapenem-susceptible and-non-susceptible infections. Antimicrob. Resist. Infect. Control 8:68. doi: 10.1186/s13756-019-0520-8
Singh, T. N., Devi, K. M., and Devi, K. S. (2005). Ecthyma gangrenosum: a rare cutaneous manifestation caused by Pseudomonas aeruginosa without bacteraemia in a leukaemic patient-a case report. Indian J. Med. Microbiol. 23, 262–263. doi: 10.1016/s0255-0857(21)02534-2
Singh, S., Singh, S. K., Chowdhury, I., and Singh, R. (2017). Understanding the mechanism of bacterial biofilms resistance to antimicrobial agents. Open Microbiol. J. 11, 53–62. doi: 10.2174/1874285801711010053
Slingerland, C. J., Kotsogianni, I., Wesseling, C. M. J., and Martin, N. I. (2022). Polymyxin stereochemistry and its role in antibacterial activity and outer membrane disruption. ACS Infect. Dis. 8, 2396–2404. doi: 10.1021/acsinfecdis.2c00307
Sousa, A. M., and Pereira, M. O. (2014). Pseudomonas Aeruginosa diversification during infection development in cystic fibrosis lungs-a review. Pathogens 3, 680–703. doi: 10.3390/pathogens3030680
Spernovasilis, N., Psichogiou, M., and Poulakou, G. (2021). Skin manifestations of Pseudomonas aeruginosa infections. Curr. Opin. Infect. Dis. 34, 72–79. doi: 10.1097/QCO.0000000000000717
Summers, W. C. (2012). The strange history of phage therapy. Bacteriophage 2, 130–133. doi: 10.4161/bact.20757
Sun, P. P., Won, J., Choo-Kang, G., Li, S., Chen, W., Monroy, G. L., et al. (2021). Inactivation and sensitization of Pseudomonas aeruginosa by microplasma jet array for treating otitis media. NPJ Biofilms Microbiomes 7:48. doi: 10.1038/s41522-021-00219-2
Tahrioui, A., Ortiz, S., Azuama, O. C., Bouffartigues, E., Benalia, N., Tortuel, D., et al. (2020). Membrane-interactive compounds from Pistacia lentiscus L. Thwart Pseudomonas aeruginosa Virulence. Front. Microbiol. 11:1068. doi: 10.3389/fmicb.2020.01068
Thi, M. T. T., Wibowo, D., and Rehm, B. H. A. (2020). Pseudomonas aeruginosa biofilms. Int. J. Mol. Sci. 21, 1–25. doi: 10.3390/ijms21228671
Tooke, C. L., Hinchliffe, P., Bragginton, E. C., Colenso, C. K., Hirvonen, V. H. A., Takebayashi, Y., et al. (2019). β-Lactamases and β-lactamase inhibitors in the 21st century. J. Mol. Biol. 431, 3472–3500. doi: 10.1016/j.jmb.2019.04.002
Valentin, J. D. P., Straub, H., Pietsch, F., Lemare, M., Ahrens, C. H., Schreiber, F., et al. (2022). Role of the flagellar hook in the structural development and antibiotic tolerance of Pseudomonas aeruginosa biofilms. ISME J. 16, 1176–1186. doi: 10.1038/s41396-021-01157-9
van Delden, C. (2007). Pseudomonas aeruginosa bloodstream infections: how should we treat them? Int. J. Antimicrob. Agents 30, 71–75. doi: 10.1016/j.ijantimicag.2007.06.015
Varma, A., Warghane, A., Dhiman, N. K., Paserkar, N., Upadhye, V., Modi, A., et al. (2023). The role of nanocomposites against biofilm infections in humans. Front. Cell. Infect. Microbiol. 13:1104615. doi: 10.3389/fcimb.2023.1104615
Venturi, V. (2006). Regulation of quorum sensing in Pseudomonas. FEMS Microbiol. Rev. 30, 274–291. doi: 10.1111/j.1574-6976.2005.00012.x
Vestby, L. K., Grønseth, T., Simm, R., and Nesse, L. L. (2020). Bacterial biofilm and its role in the pathogenesis of disease. Antibiotics 9:59. doi: 10.3390/antibiotics9020059
Vincent, J.-L., Sakr, Y., Singer, M., Martin-Loeches, I., Machado, F. R., Marshall, J. C., et al. (2020). Prevalence and outcomes of infection among patients in intensive care units in 2017. JAMA 323, 1478–1487. doi: 10.1001/jama.2020.2717
Wagner, V. E., Bushnell, D., Passador, L., Brooks, A. I., and Iglewski, B. H. (2003). Microarray analysis of Pseudomonas aeruginosa quorum-sensing regulons: effects of growth phase and environment. J. Bacteriol. 185, 2080–2095. doi: 10.1128/JB.185.7.2080-2095.2003
Wang, T., Hou, Y., and Wang, R. (2019). A case report of community-acquired Pseudomonas aeruginosa pneumonia complicated with MODS in a previously healthy patient and related literature review. BMC Infect. Dis. 19:130. doi: 10.1186/s12879-019-3765-1
Wang, C. Y., Jerng, J. S., Cheng, K. Y., Lee, L. N., Yu, C. J., Hsueh, P. R., et al. (2006). Pandrug-resistant Pseudomonas aeruginosa among hospitalised patients: clinical features, risk-factors and outcomes. Clin. Microbiol. Infect. 12, 63–68. doi: 10.1111/j.1469-0691.2005.01305.x
Waters, E. M., Neill, D. R., Kaman, B., Sahota, J. S., Clokie, M. R. J., Winstanley, C., et al. (2017). Phage therapy is highly effective against chronic lung infections with Pseudomonas aeruginosa. Thorax 72, 666–667. doi: 10.1136/thoraxjnl-2016-209265
Waters, V., Zlosnik, J. E. A., Yau, Y. C. W., Speert, D. P., Aaron, S. D., and Guttman, D. S. (2012). Comparison of three typing methods for Pseudomonas aeruginosa isolates from patients with cystic fibrosis. Eur. J. Clin. Microbiol. Infect. Dis. 31, 3341–3350. doi: 10.1007/s10096-012-1701-z
Wei, Q., and Ma, L. Z. (2013). Biofilm matrix and its regulation in Pseudomonas aeruginosa. Int. J. Mol. Sci. 14, 20983–21005. doi: 10.3390/ijms141020983
Welte, W., Nestel, U., Wacker, T., and Diederichs, K. (1995). Structure and function of the porin channel. Kidney Int. 48, 930–940. doi: 10.1038/ki.1995.374
Wood, S. J., Kuzel, T. M., and Shafikhani, S. H. (2023). Pseudomonas aeruginosa: infections, animal modeling, and therapeutics. Cells 12:199. doi: 10.3390/cells12010199
Wu, D. C., Chan, W. W., Metelitsa, A. I., Fiorillo, L., and Lin, A. N. (2011). Pseudomonas skin infection. Am. J. Clin. Dermatol. 12, 157–169. doi: 10.2165/11539770-000000000-00000
Wusiman, A., Zhang, Y., Ayihengbieke, T., Cheng, X., Kudereti, T., Liu, D., et al. (2022). Poly (lactic-co-glycolic acid) nanoparticle-based vaccines delivery systems as a novel adjuvant for H9N2 antigen enhance immune responses. Poult. Sci. 101:101791. doi: 10.1016/j.psj.2022.101791
Xiong, J., Alexander, D. C., Jennifer, H. M., Dérasp, M., Low, D. E., Jamieson, F. B., et al. (2013). Complete sequence of pOZ176, a 500-kilobase incp-2 plasmid encoding imp-9-mediated carbapenem resistance, from outbreak isolate pseudomonas aeruginosa 96. Antimicrob. Agents Chemother. 57, 3775–3782. doi: 10.1128/AAC.00423-13
Yang, F., Gu, J., Yang, L., Gao, C., Jing, H., Wang, Y., et al. (2017). Protective efficacy of the trivalent Pseudomonas aeruginosa vaccine candidate PcrV-OprI-Hcp1 in murine pneumonia and burn models. Sci. Rep. 7:3957. doi: 10.1038/s41598-017-04029-5
Youns, M., and Abdel Halim Hegazy, W. (2017). The natural flavonoid Fisetin inhibits cellular proliferation of hepatic, colorectal, and pancreatic Cancer cells through modulation of multiple signaling pathways. PLoS One 12:e0169335. doi: 10.1371/journal.pone.0169335
Zamorano, L., Reeve, T. M., Deng, L., Juan, C., Moyá, B., Cabot, G., et al. (2010). NagZ inactivation prevents and reverts β-lactam resistance, driven by AmpD and PBP 4 mutations, in Pseudomonas aeruginosa. Antimicrob. Agents Chemother. 54, 3557–3563. doi: 10.1128/AAC.00385-10
Zhang, L., Hinz, A. J., Nadeau, J. P., and Mah, T. F. (2011). Pseudomonas aeruginosa tssC1 links type VI secretion and biofilm-specific antibiotic resistance. J. Bacteriol. 193, 5510–5513. doi: 10.1128/JB.00268-11
Keywords: Pseudomonas aeruginosa, resistance, pathogenicity, virulence, therapy
Citation: Elfadadny A, Ragab RF, AlHarbi M, Badshah F, Ibáñez-Arancibia E, Farag A, Hendawy AO, De los Ríos-Escalante PR, Aboubakr M, Zakai SA and Nageeb WM (2024) Antimicrobial resistance of Pseudomonas aeruginosa: navigating clinical impacts, current resistance trends, and innovations in breaking therapies. Front. Microbiol. 15:1374466. doi: 10.3389/fmicb.2024.1374466
Edited by:
Sílvia A. Sousa, Institute for Bioengineering and Biosciences, PortugalReviewed by:
Salome N. Seiffert, Zentrum für Labormedizin (ZLM), SwitzerlandTahir Hussain, Abdul Wali Khan University Mardan, Pakistan
Copyright © 2024 Elfadadny, Ragab, AlHarbi, Badshah, Ibáñez-Arancibia, Farag, Hendawy, De los Ríos-Escalante, Aboubakr, Zakai and Nageeb. This is an open-access article distributed under the terms of the Creative Commons Attribution License (CC BY). The use, distribution or reproduction in other forums is permitted, provided the original author(s) and the copyright owner(s) are credited and that the original publication in this journal is cited, in accordance with accepted academic practice. No use, distribution or reproduction is permitted which does not comply with these terms.
*Correspondence: Ahmed Elfadadny, czE5MjAyOXRAc3QuZ28udHVhdC5hYy5qcA==