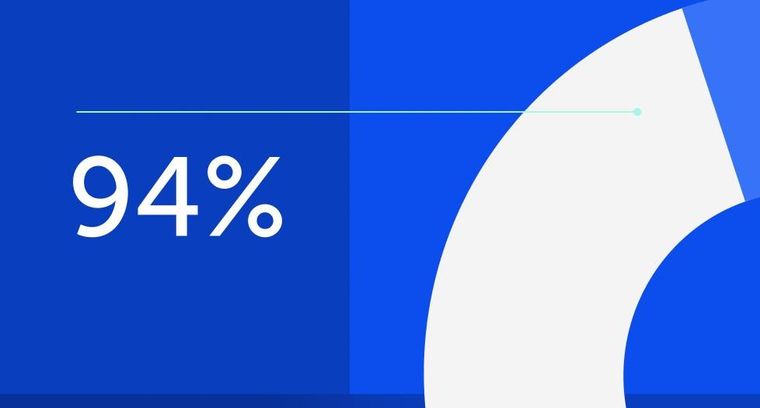
94% of researchers rate our articles as excellent or good
Learn more about the work of our research integrity team to safeguard the quality of each article we publish.
Find out more
ORIGINAL RESEARCH article
Front. Microbiol., 13 March 2024
Sec. Microbial Physiology and Metabolism
Volume 15 - 2024 | https://doi.org/10.3389/fmicb.2024.1369018
This article is part of the Research TopictRNA and Protein Synthesis in MicroorganismsView all 10 articles
Transfer RNA (tRNA) modifications play a crucial role in maintaining translational fidelity and efficiency, and they may function as regulatory elements in stress response and virulence. Despite their pivotal roles, a comprehensive mapping of tRNA modifications and their associated synthesis genes is still limited, with a predominant focus on free-living bacteria. In this study, we employed a multidisciplinary approach, incorporating comparative genomics, mass spectrometry, and next-generation sequencing, to predict the set of tRNA modification genes responsible for tRNA maturation in two intracellular pathogens—Bartonella henselae Houston I and Bartonella quintana Toulouse, which are causative agents of cat-scratch disease and trench fever, respectively. This analysis presented challenges, particularly because of host RNA contamination, which served as a potential source of error. However, our approach predicted 26 genes responsible for synthesizing 23 distinct tRNA modifications in B. henselae and 22 genes associated with 23 modifications in B. quintana. Notably, akin to other intracellular and symbiotic bacteria, both Bartonella species have undergone substantial reductions in tRNA modification genes, mostly by simplifying the hypermodifications present at positions 34 and 37. Bartonella quintana exhibited the additional loss of four modifications and these were linked to examples of gene decay, providing snapshots of reductive evolution.
The conversion of genetic information from messenger RNA (mRNA) into functional proteins represents a fundamental and intricately regulated process in all living organisms (Zaher and Green, 2009). At the heart of this complex mechanism, transfer RNA (tRNA) molecules function as indispensable adaptors, ensuring the precise and faithful translation of mRNA codons into amino acids (Berg and Brandl, 2021). Post-transcriptional modifications of tRNA molecules play pivotal roles in preserving the stability and integrity of tRNA molecules while enhancing the accuracy and efficiency of the decoding process (El Yacoubi et al., 2012). As tRNA modification levels can be affected by stress or the metabolic status of cells, and these variations can affect the translation of specific mRNAs, specific modifications can be recruited to fulfill regulatory roles (Chan et al., 2010; Helm and Alfonzo, 2014; de Crécy-Lagard and Jaroch, 2021; Wang and Lin, 2023). Even though such regulatory roles were postulated over 40 years ago (Persson, 1993), it is only recently that the full regulatory or homeostatic loops have been dissected in different model organisms, including several pathogenic bacteria where they have been found to have roles in resistance to stress and virulence (Koh and Sarin, 2018; Antoine et al., 2021; de Crécy-Lagard and Jaroch, 2021; Fruchard et al., 2022).
Exploration of the intricate regulatory mechanisms driven by tRNA modifications necessitates a comprehensive understanding of the entire set of modifications and the corresponding genes encoding the enzymes responsible for their installation. However, this knowledge remains largely incomplete, with significant gaps existing beyond a few well-studied model organisms, including the bacterial pathogen Mycoplasma capricolum (de Crécy-Lagard and Jaroch, 2021). Advancing our understanding requires the integration of analytical tools such as mass spectrometry (MS) or next-generation sequencing with comparative genomic analyses across diverse organisms spanning the tree of life (de Crécy-Lagard and Jaroch, 2021). Recent years have seen integrated studies that successfully cataloged tRNA modifications and associated genes in a few pathogenic bacteria, such as Vibrio cholerae (Kimura et al., 2020) and Mycobacterium tuberculosis (Tomasi et al., 2023), as well as in the antibiotic-producing Streptomyces albidoflavus (Koshla et al., 2023). Notably absent from these investigations are members of obligate intracellular bacterial families like Chlamydiaceae, Rickettsiaceae, or Ehrlichiaceae which harbor significant pathogenic species for humans (McClure et al., 2017; Loterio et al., 2021). Detecting tRNA modifications in intracellular pathogens presents unique challenges, including the low yield of tRNA during preparation and the potential for contamination by host cell tRNA. Yet, beyond unraveling the potential roles of tRNA modifications in the virulence of intracellular bacteria, mapping these modifications can contribute to our understanding of the genetic code’s evolution in organisms that undergo extensive genome reductions as part of their adaptations to specialized niches (Andersson and Kurland, 1998; Albalat and Cañestro, 2016).
Bartonella henselae Houston I, a member of the Hyphomicrobiales order, is a facultative intracellular Gram-negative pathogen that induces a spectrum of diseases, varying in severity. Bartonella henselae is linked to ailments such as cat-scratch disease, which primarily affects children, and bacillary angiomatosis (BA), more commonly observed in HIV/AIDS patients (Rovid Spickler, 2005; Raoult, 2007). Additionally, Bartonella quintana Toulouse, another facultative intracellular pathogen transmitted by human body lice, leads to trench fever and related illnesses (Alsmark et al., 2004; Foucault et al., 2006). These pathogens have complex life cycles as their reservoirs are mammalian vascular cells, but they are transmitted by arthropod vectors. Bartonella henselae has evolved from an insect symbiont ancestor undergoing a first cycle of genome reduction (Segers et al., 2017) with B. quintana undergoing a subsequent massive secondary genome reduction (Engel and Dehio, 2009). In addition, as these organisms can be readily cultured in vitro with vascular cells (erythrocytes, endothelial cells; Battisti and Minnick, 2008), they can be used as valuable models for employing the MS/NGS/comparative genomic approach to identify all genes related to tRNA modifications in intracellular bacteria.
Prior studies, which concentrated on rescuing the wobble base modification Queuosine (Q) in B. henselae and B. quintana, revealed that while the latter bacterium lost all genes associated with the pathway, B. henselae retained only two out of eight Q synthesis/salvage genes. These two genes constitute a basic salvage pathway, comprising a QPTR/YhhQ family transporter and a homolog of tRNA-guanosine (34) preQ1 transglycosylase (Tgt; Quaiyum et al., 2023). Interestingly, this minimal pathway not only successfully salvages the Q precursor PreQ1 but also enables the rescue of the q base when present in high concentrations, a capability not shared by the E. coli orthologs. It is not clear which conditions would allow the salvage of these two precursors in natural environments, but analysis of tRNA extracted from B. henselae cells showed that preQ1 could be detected at the wobble position, a unique situation, to date. The current study focuses on predicting the remaining tRNA modifications to generate a better picture of decoding in these organisms.
The genomic sequences of Bartonella henselae str. Houston I (NCBI Taxon ID 38323; NC_005956.1) and Bartonella quintana str. Toulouse (NCBI Taxon ID 283165; NC_005955) were used for all analyses. tRNA isoforms information was obtained from GtRNA database (Chan and Lowe, 2016).1,2 The BV-BRC platform (Olson et al., 2023) was used for protein family identifications, physical clustering analyses, and species tree construction using FastTree (version 47). Species trees were also generated using PhyloT3 (database version 2023.2) and Protein families were also identified with KO numbers in the KEGG database (Kanehisa et al., 2021). UniProt was used for ID mapping and advanced search tools (Bateman et al., 2023). NCBI was used for BlastP analyses (Altschul et al., 1997) and literature searches (NCBI Resource Coordinators, 2016). GizmoGene4 was used to make the physical clustering figures and Modomics (Boccaletto et al., 2018) for analyses of tRNA sequences. iToL5 (version 6.8.1; Letunic and Bork, 2021) and MORPHEUS6 were used to visualize the presence/absence data of protein families along the species trees.
Bartonella henselae Houston I was obtained from the American Type Culture Collection (ATCC 49882). Bartonella quintana Toulouse was a generous gift from Volkhard Kempf (Goethe-Universität Frankfurt). Both species were cultivated as previously described (Battisti and Minnick, 2008) on HIBB agar plates [i.e., Bacto heart infusion agar (Becton, Dickinson, Sparks, MD) supplemented with 4% defibrinated sheep blood and 2% sheep serum (Quad Five, Ryegate, MT) by volume] for 4 days (B. henselae) or 10 days (B. quintana) at 37°C, 5% CO2 and 100% relative humidity. Bacteria in free form were harvested into ice-cold heart infusion broth from the surface of HIBB plates using a sterile razor blade as previously described (Battisti and Minnick, 2008). This method left sheep cells embedded in the agar matrix and minimized the chance of cross-contamination. The bacterial suspension was centrifuged for 2 min at 16,000 × g at 4°C, and the resulting pellet was suspended in 1 mL of Trizol (Thermo Fisher Scientific, Waltham, MA, United States). Small RNAs were extracted with a PureLinkTm miRNA Isolation kit following manufacturer’s instructions (Thermo Fisher Scientific). Purified RNAs were eluted using 50 μL of RNase-free water and quantified using a Nanodrop 1000 spectrophotometer (Quaiyum et al., 2023).
tRNA modifications were analyzed by LC-MS using two similar methods. For samples S1–S3 in Supplementary Tables S1–S3, 3 μg of small RNA was hydrolyzed in a 50 μL (0.06 μg/μL) digestion cocktail containing 12.5 U of U benzonase (0.25 U/μL), 5 U CIAP (calf intestinal alkaline phosphatase; 0.1 U/μL), 0.15 U of PDE I (phosphodiesterase I; 0.003 U/μL), 0.1 mM deferoxamine, 0.1 mM BHT (butylated hydroxytoluene), 5 ng coformycin (0.1 ng/μL), 50 nM 15N-dA (internal standard [15N]5-deoxyadenosine), 2.5 mM MgCl2 and 5 mM Tris–HCL buffer pH 8.0. For samples S1–S5 in Supplementary Tables S1, S4, S5, 1.8 μg of small RNA was hydrolyzed in 30 μL (0.06 μg /μL) digestion cocktail containing 2.49 U benzonase (0.083 U/μL), 3 U CIAP (0.1 U/μL), 0.07 U PDE I (0.002 U/μL), 0.1 mM deferoxamine, 0.1 mM BHT, 3 ng coformycin (0.1 ng/μL), 25 nM 15N-dA, 2.5 mM MgCl2 and 5 mM Tris–HCL buffer pH 8.0. The digestion mixture was incubated at 37°C for 6 h. After digestion, all samples were analyzed by chromatography-coupled triple-quadrupole mass spectrometry (LC-MS/MS). For each sample, hydrolysate containing 600 ng of RNA was injected for each of the technical replicates (Supplementary Tables S3, S5). Using synthetic standards, HPLC retention times of RNA modifications were confirmed on a Waters Acuity BEH C18 column (50 × 2.1 mm inner diameter, 1.7 μm particle size) coupled to an Agilent 1,290 HPLC system and an Agilent 6,495 triple-quadrupole mass spectrometer (Supplementary Table S1). All references for the synthesis of the standards have been included to Table 1. The Agilent sample vial insert was used. The HPLC system was operated at 25°C and a flow rate of 0.35 mL/min or 0.3 mL/min in a gradient [Supplementary Tables S2, S4 with Buffer A (0.02% formic acid in water)] and Buffer B (0.02% formic acid in 70% acetonitrile). The HPLC column was coupled to the mass spectrometer with an electrospray ionization source in positive mode with the following parameters: dry gas temperature, 200°C; gas flow, 11 L/min; nebulizer, 20 psi; sheath gas temperature, 300°C; sheath gas flow, 12 L/min; capillary voltage, 3,000 V; nozzle voltage, 0 V. Multiple reaction monitoring (MRM) mode was used for detection of product ions derived from the precursor ions for all the RNA modifications with instrument parameters including the optimized collision energy (CE) optimized for maximal sensitivity for the modification. Signal intensities for each ribonucleoside were normalized by dividing by the sum of the UV signal intensities of the four canonical ribonucleosides recorded with an in-line UV spectrophotometer at 260 nm. The MS data was deposited in the PRIDE database7 with the accession number PXD048805.
Analysis of tRNA modifications present in B. henselae tRNA fractions was performed by a combination of three previously published original protocols, namely RiboMethSeq, AlkAnilineSeq and HydraPsiSeq. Additional analysis of eventual m5C residues was done by standard RNA bisulfite sequencing. All sequencing data was deposited in the Short Read Archive8 with the accession number PRJEB72223.
RiboMethSeq protocol allowed us to map 2′-O-methylations by assessing their protection against alkaline cleavage (Marchand et al., 2016, 2017). To further enhance our analysis, we also extracted reverse transcriptase (RT) misincorporation signatures at RT-arresting nucleotides (Motorin and Marchand, 2018; Werner et al., 2020). As part of the RiboMethSeq tRNA analysis, total RNA from B. henselae was fragmented under alkaline conditions, followed by library preparation and sequencing (Marchand et al., 2017). The RT misincorporation signatures were derived from the Samtools mpileup format and were manually verified by examining the aligned reads in the *.bam file using the Integrated Genome Viewer (IGV; Thorvaldsdóttir et al., 2012).
Analysis of m7G and D modifications in B. henselae tRNAs was done by AlkAnilineSeq protocol (Marchand et al., 2018, 2021). This method also detects m3C and ho5C, but these residues have not been reported in bacterial tRNAs. Eventual cleavage signals may be also observed for s2C and ho5U intermediates at position 34 of tRNAs. This method exploits sensitivity of certain RNA modified bases to ring-opening at high temperature and alkaline conditions, the resulting damaged base or RNA a basic site is further cleaved by aniline and adapter is ligated to the released 5′-P termini.
For the mapping of pseudouridine (Psi) modifications, we employed a chemical-based protocol using hydrazine cleavage, termed HydraPsiSeq (Motorin et al., 2007; Behm-Ansmant et al., 2011; de Brouwer et al., 2018). This protocol measures resistance of pseudouridines and m5U (rT) residues to the hydrazine cleavage. Subsequent aniline treatments convert damaged U residues to cleavages of the polynucleotide chain. Ligation of the sequencing adapter is done as in the AlkAnilineSeq protocol (see above). Of note, k2C (lysidine) present in bacterial tRNAIleCAU at the wobble position shows extensive cleavage upon hydrazine treatment and thus can be detected as positive cleavage signal.
Analysis of eventual m5C modifications in B. henselae tRNAs was performed with the well-established bisulfite conversion protocol developed by Schaefer et al. (2009). The EZ RNA Methylation Kit (Zymo Research #R5001) was used for RNA bisulfite treatment followed by desulphonation. Bisulfite-converted RNAs were then end-repaired and subjected to NEBNExt Small RNA Library Prep Set for Illumina (NEB, E7330L), according to manufacturer’s recommendations. Sequencing was performed in SR50 mode, with sequencing reads aligned to C → T converted reference sequence for B. henselae tRNAs. 3.1.
The B. henselae genome encodes a total of 43 predicted tRNA genes, specifying 38 distinct iso-acceptors with only two tRNAs with multiple copies (Figure 1). In comparison, the genome of B. quintana comprises 42 tRNA genes with an equivalent number of iso-acceptors, albeit with the loss of one of the triplicate copies of initiator-tRNAMetCAU (Figure 1). This reduction in iso-acceptors is modest when compared to E. coli, with only four losses: tRNAArgCCT, tRNAGlyCCC, tRNAProCGG, and tRNASelCysUCA. This contrasts sharply with organisms with reduced genomes, such as mollicutes, which can pare down the number of tRNAs to 28 (Grosjean et al., 2014). Notably, while the total number of tRNA genes in E. coli is 86, often present in five copies (Chan and Lowe, 2016), the two Bartonella species have significantly reduced the duplicate gene copies of any given iso-acceptor (Figure 1).
Figure 1. tRNA isoacceptors genes distribution for Bartonella henselae (Bh) and Bartonella quintana (Bq). A circular representation of the genetic code, highlighting modified nucleosides, is presented, following the format described by Grosjean and Westhof (2016). In this representation, each amino acid codon sequence is read from the inside out (1-2-3). The dark dots represent the tRNA isoforms encoded by the genome. Additionally, the elongator or initiator tRNAMetCAU are denoted by “e” and “i,” respectively. The predicted modifications of the ASL of any given isoacceptor e and the predicted expanded decoding capacity through wobble or expanded wobble of each isoacceptor are noted by extended dark lines and white circles. All isoacceptors are present in one copy with two exceptions noted in boxes with the numbers of copies in parentheses.
In the absence of post-transcriptional modifications, these sets of tRNAs would be incapable of decoding the 64 codons (Grosjean et al., 2010). To illustrate, tRNAIleCAU requires the modification of cytosine to lysidine to decode AUA codons. However, the modification status of tRNA molecules from Bartonellaceae was unknown, as none had been previously sequenced (Boccaletto et al., 2018). Consequently, we undertook an LC–MS/MS analysis to search for known tRNA modifications, examining the ribonucleosides obtained from the enzymatic digestion of bulk tRNA extracted from both Bartonella species cultivated on HIBB plates, as detailed in the methods section. Here we used two different LC-MS/MS methods (Supplementary Tables S2, S4) to discover modified ribonucleoside in different biological replicate tRNA samples from B. henselae and from the more challenging-to-culture B. quintana (Supplementary Tables S3, S5). In all, 31 modified ribonucleosides were detected in these samples, 30 with standards and 1 (ms2t6A) without but with genetic evidence as discussed in the section below (Table 1; Supplementary Tables S3, S5). However, not all modified ribonucleosides in this list are derived from Bartonella tRNAs. Indeed, rRNA modifications are routinely detected in analyses of tRNA samples as we previously discussed when analyzing tRNA modification profiles in Bacillus subtilis (de Crécy-Lagard et al., 2020) and in the current analysis we can also detect modifications both from rRNA or tRNA from the sheep cells present in the culture media. To predict the tRNA modification profiles of the Bartonella species specifically, we combined the results of LC–MS with bioinformatic analyses and NGS-based modification detection methods as described in the next sections.
The compilation of tRNA modification genes, initially established for model organisms E. coli and B. subtilis (de Crécy-Lagard et al., 2020), underwent an update by incorporating two recently identified genes in B. subtilis (Jaroch et al., 2023) into the dataset (Supplementary Tables S6, S7). This updated compilation served as a foundation for identifying tRNA modification genes encoded by B. henselae strain Houston 1, utilizing both BlastP (Altschul et al., 1997) and advanced search tools provided by BV-BRC (Olson et al., 2023) and UniProt (Bateman et al., 2023). The predictions generated were then cross-referenced with the modifications detected through LC-MS, and with the sequencing-based (RiboMethSeq, AlkAnilineSeq, and HydraPsiSeq) detection analyses results (Figure 2), leading to the creation of the predicted tRNA modification map depicted in Figure 3. In total, we predicted 26 genes participating in the insertion of 23 modifications (Figure 3; Supplementary Table S8).
Figure 2. Overview of tRNA modifications mapping by deep sequencing-based protocols: AlkAnilineSeq, HydraPsiSeq and RiboMethSeq. Positions and identities of modified residues found in Bartonella henselae tRNA species (shown by amino acid specificity and anticodon) are shown on a canonical cloverleaf tRNA structure. Long tRNA variable loops are not shown.
Figure 3. Prediction of Bartonella henselae and Bartonella quintana tRNA modifications maps and corresponding synthesis proteins. (A) Bartonella henselae, underlined protein names denote high confidence based on orthology; in red are modifications detected by LC-MS/MS in all samples. A “*” denotes that the modification at this position was confirmed by NGS. Locus tags are given in parentheses, and when italicized, denote a low-confidence prediction. (B) Bartonella quintana, locus tags are given in parenthesis, and when italicized it denotes a low confidence.
For 18 of these modifications, the combination of gene information and LC–MS/MS data yielded predictions with high confidence, as highlighted in Figure 3 and Table 1. The position of a few of those such as m7G46, ho5U34, and s2C32 were confirmed by sequencing (Figure 2; Supplementary Figure S1). Additional mapping information was required for the remaining 5 modifications. Certain modification enzymes, like methyltransferases, pseudouridine synthases (Hamma and Ferre-D’Amare, 2006) or dihydrouridine synthases (Bou-Nader et al., 2018), have undergone multiple duplications and shifts in substrate specificity or may exhibit multisite specificity (Barraud and Tisné, 2019). Consequently, predicting their specificities based solely on orthologous relationships can be challenging. Many bacteria possess multiple D modifications, introduced by different enzymes such as DusA/B/C in E. coli (Bishop et al., 2002) or a single enzyme like DusB in M. capricolum (Faivre et al., 2021). In the B. henselae genome, the sole encoded member of the Dus family is a DusA homolog. While E. coli’s DusA modifies positions 20/20a (Bishop et al., 2002), we could not exclude the possibility that in B. henselae, the enzyme had evolved a relatively broader specificity. However, AlkAnilineSeq analysis conclusively confirmed that the only positions modified by D in Bartonella tRNAs were 20/20a (and possibly 20b; Figure 2; Supplementary Figure S1).
In the mapping of Ψ residues, the identification of homologs of TruA and TruB, and the absence of TruC and TruD, suggested modifications at positions 38–40 (TruA) and 55 (TruB) but excluding positions 13 and 65 formed by TruD and TruC, respectively. However, a conclusive determination for position 32, known to be modified in E. coli by a dual-specific RluA enzyme that modifies both rRNAs and tRNAs, was not as straightforward. Three predicted rRNA pseudouridine synthases are encoded in the B. henselae genome (BH02610, RluC/BH10200, or RluD/BH03820), and any of these might have shifted specificity to modify tRNAs, akin to the observed phenomenon with RluA and RluF in E. coli (Addepalli and Limbach, 2016). HydraPsiSeq analysis confirmed the absence of Ψ residues at positions 13 and 65 and the presence of Ψ at position 55 in many tRNAs and at positions 38/39/40 in a few tRNAs (Figure 2; Supplementary Figure S2) confirming the predictions based on the presence/absence of the corresponding synthesis genes. Ψ was also detected at position 32 in several tRNAs (Figure 2; Supplementary Figure S2). We propose that BH02610, the lone orphan pseudouridine synthase, catalyzes the formation of Ψ32 in B. henselae, but further experimental validation will be required. HydraPsiSeq protocol also detects 5′-modified U (namely m5U), which also shows protection against hydrazine cleavage, and k2C (lysidine) which is efficiently cleaved under conditions used in HydraPsiSeq (Supplementary Figure S2) The results of this mapping indicated that U54 in many tRNAs is only partially protected, indicating sub-stoichiometric modification by TrmFO. As anticipated, lysidine-related signal in HydraPsiSeq was found in tRNAIleCAU at wobble position C34 (Supplementary Figure S2). The presence of homologs of TrmJ and TrmL strongly predicts the presence of ribose −2′-O-methylations at positions 32 and 34, respectively, but the exact nature of the modified nucleosides could vary. RiboMethSeq analyses were able to map Am/Cm/Um at positions 32, but only Cm at position 34 (Figure 2; Supplementary Figure S3). Finally, the presence of a homolog of Tgt, the signature enzyme for the queuosine pathway in B. henselae, suggested the presence of Q in this organism. However, we previously showed that the Q observed in the LC-MS/MS analysis could be a contamination from mammalian host and that the preQ1 precursor could be the biological form of the deazapurine found in this organism (Quaiyum et al., 2023).
A significant number of modifications identified by LC–MS/MS could not be attributed to specific genes (Table 1), including those present in substantial quantities like m5C (Supplementary Tables S3, S5). For m5C it was confirmed by bisulfite sequencing that indeed it is not present in the B. henselae tRNAs (data not shown). The levels of many of these “orphan” modifications exhibited considerable variation among the analyzed samples. For instance, m22G was present in certain samples and absent in others (Supplementary Tables S3, S5). One plausible source for these modified nucleosides is rRNA from the bacteria or tRNA/rRNA from the host. Indeed, m3U, m5C, and m62A are well-known rRNA modifications (Boccaletto et al., 2018), while contamination by mammalian host tRNAs, might contribute to the observed pools of m1A, Gm, m3C, m22G and m2G (Boccaletto et al., 2018).
Bartonella henselae exhibits a streamlined tRNA modification machinery, evident both in the reduced number of modifications and the simplicity of the existing ones. In comparison to E. coli, B. henselae encodes less than half the modification genes (26 genes compared to E. coli’s 59). Studies on insect symbionts have indicated that modifications of the tRNA body are the first to diminish and can even be entirely lost by reductive evolution, as observed in the genome of louse symbionts (de Crécy-Lagard et al., 2012). Notably, common tRNA body modifications such as D16/17, Ψ13/65, and s4U8 are absent in B. henselae tRNAs based on the absence of the genes and confirmed by the lack of the NGS signals (data not shown). DusB family is predicted to be the ancestral Dus enzyme (Bou-Nader et al., 2018), and depending on the organism it modifies position 16 or is multisite-specific (Faivre et al., 2021). It seems to have been lost near the root of the Bartonellaceae clade (Supplementary Figure S4B). The only remaining Dus enzyme in B. henselae is DusA which is found in all Hyphomicrobiales (Supplementary Figure S4A). TruC and TruD and ThiI are totally absent in Alphaprotebacteria, hence the observed losses in the Bartonellaceae are not recent events (Supplementary Figure S5).
Losing modifications in the Anticodon Stem Loop (ASL) should significantly impact decoding capacity, efficiency, and accuracy. Only two ASL modifications found in E. coli are absent in B. henselae: ac4C34 and Ψ35. The first is found in the elongator tRNAMetCAU and prevents misreading of the near cognate AUA (Ile) codon (Kawai et al., 1989). The second affects the decoding efficiency of Tyr codon stretches (Addepalli and Limbach, 2016). However, as the synthesis enzymes for both these modifications (TmcA and RluF) seem to be absent in nearly all Alphaproteobacteria (Supplementary Figure S5), organisms must compensate for the absence of these modifications.
Interestingly, several complex modifications present in E. coli exist in B. henselae but in a simpler form that requires a truncated synthesis pathway with fewer genes. The simplification of the Q pathway to salvaging and inserting preQ1 and possibly queuine (q) bases was reported previously (Quaiyum et al., 2023), but it looks like all complex pathways have been simplified in this organism. For instance, the cmnm5s2U precursor, synthesized by MmmGEA enzymes, is present, but its derivative mnm5s2U, requiring two additional enzymatic steps, is not. Similarly, the ho5U precursor is present, but not its derivative, cmo5U. Likewise, the t6A modification is present but it is not further modified to m6t6A. These simplifications of complex ASL modifications to minimal forms have facilitated the reduction of the number of tRNA modification genes without affecting the capacity of the 43 B. henselae tRNAs to decode the full set of codons (Figure 1). There is only one example of a modification that is more complex in B. henselae than in E. coli: ms2t6A. The enzyme involved in its synthesis, MtaB, is widespread in Alphaprotebacteria, so its presence in Bartonellaceae reflects the evolutionary history of the species (Supplementary Figure S5).
A parallel analysis was executed to predict the tRNA modification gene sets in B. quintana, as depicted in Figure 2B and detailed in Supplementary Table S9. This analysis identified 22 genes linked to 23 modifications. While most genes were found in both Bartonella species, instances of gene decay were observed, resulting in the loss of corresponding modifications in the extracted tRNA digests (Supplementary Tables S3, S5; Figure 4). Prior findings had noted the decay of the tgt gene (Quaiyum et al., 2023), and three more instances were identified: ttcA, trmFO, and trmL. In each case, the complete gene is present in B. henselae, but only fragments are discernible at the corresponding loci in the sequenced B. quintana Toulouse genome (Figure 5; Supplementary Table S10). Notably, the decay of tgt, ttcA, and trmFO genes appears consistent across all sequenced B. quintana strains, while the truncation of trmL seems specific to the B. quintana Toulouse strain. Results from LC-MS/MS analyses of bulk tRNAs extracted from B. quintana seem to align with these losses. For instance, the disappearance of the ttcA gene correlates with the absence of s2C in the nucleoside analysis profile from B. quintana, contrasting with its presence in B. henselae (Figure 4; Supplementary Tables S3, S5). Levels of Cm are not dramatically affected in B. quintana (Figure 4; Supplementary Tables S3, S5). Given that Cm can also be derived from contaminating rRNAs or host tRNAs and that RiboMethSeq for B. quintana tRNAs was not performed, additional targeted experiments will be essential to validate the absence of this modification at position 34. Members of the TtcA family are present in most Bartonella species (Supplementary Figure S4B). s2C32 has been shown to have a role in preventing frameshift (Jager et al., 2004) but is also important to restrict the ability of tRNAArgICG to decode the rare arginine codon CGA (Vangaveti et al., 2020). Losing the Cm methylation in tRNALeuCAA reduces the efficiency of codon-wobble base interaction, as demonstrated in an amber suppressor system (Benítez-Páez et al., 2010). It is therefore expected that a loss of translation accuracy should occur with the loss of both Cm and s2C modifications in B. quintana, a phenomenon often observed in host-restricted organisms (Melnikov et al., 2018).
Figure 4. Quantification of Cm, m5U, s2C, and t6A modifications in Bartonella henselae and Bartonella quintana. LC-MS/MS analysis was performed on 600 ng of small RNA, with signal intensities normalized to the sum of UV absorbances of the canonical ribonucleosides, using an inline UV detector, to correct for differences in amounts of injected RNA. Data represent means ± SD for three technical replicates of one biological replicate for B. henselae (S1) and two biological replicates (shown individually) for B. quintana (S2, S3). N.D., Not detected.
Figure 5. Decay of ttcA, trmL, and trmFO genes in Bartonella quintana species. Gene neighborhoods surrounding the three modification genes in Bartonella species. Bartonella quintana str. Toulouse and the Bartonella henselae Houston-I are boxed in red and purple, respectively. The BV-BRC gene ids (or fig ids) of the specific anchoring genes [ttcA in (A), trmFO in (B) and trmL in (C)] are given for every cluster.
Finally, m5U levels are reduced by approximately 80% in B. quintana compared to B. henselae (Figure 4; Supplementary Tables S3, S5). The residual m5U could be derived from contaminating rRNA and/or host tRNAs (Boccaletto et al., 2018). Two non-orthologous families of methylases can catalyze the formation of m5U54 in tRNA: the SAM-dependent TrmA and the FAD-dependent TrmFO. The two enzymes are mutually exclusive, and genomes encode one or the other (Myllykallio et al., 2018). Members of the alphaproteobacterial clade use TrmFO (Supplementary Figure S5), hence its presence in Bartonellaceae is to be expected. The loss of m5U54 in B. quintana is like what is observed in other organisms with minimal genomes (de Crécy-Lagard et al., 2012; Grosjean et al., 2014).
This study shows that the tRNA modifications profiles and most corresponding genes can be predicted for the facultative intracellular pathogens B. henselae and B. quintana, but one must be wary of contamination by host tRNAs and rRNA and combine different types of evidence. Also, it is possible that certain modifications may have been missed in the study, e.g., /if novel pathways are present to insert modifications that could not be chemically identified because of a lack of standards. Nevertheless, even with these potential omissions, it is possible to conclude that while B. henselae has not greatly reduced the number of tRNA isoacceptors, they are all in single copy. In addition, the number of modified bases in tRNAs has been reduced from 43 in E. coli to 28 in B. henselae (a~35% relative reduction). Finally, the number of tRNA modification genes has undergone an even greater reduction with 59 genes in E. coli to 26 in B. henselae (a~56% relative reduction) because of the simplification of the most complex pathways. Further simplifications of the tRNA modifications apparatus are observed in B. quintana with an additional loss of four modifications (preQ1, Cm. s2C and m5U) compared to B. henselae, which correlates with the extensive genome reduction following its divergence from B. henselae and specialization for the human host and louse vector (Alsmark et al., 2004). It is also possible that an increase error rate caused by the loss of tRNA modifications in these organisms could lead to a better evasion of the immune system by providing greater variability to surface epitopes, as postulated for other pathogens (Melnikov et al., 2018).
The data presented in the study are deposited in the Short Read Archive with the accession number PRJEB72223 and in the PRIDE database with the accession number PXD048805.
SQ: Investigation, Writing – original draft. JS: Data curation, Investigation, Writing – original draft. VM: Investigation, Methodology, Writing – review & editing. GS: Data curation, Investigation, Methodology, Visualization, Writing – original draft, Writing – review & editing. CR: Data curation, Investigation, Visualization, Writing – review & editing. YM: Funding acquisition, Methodology, Supervision, Visualization, Writing – original draft, Writing – review & editing. PD: Funding acquisition, Project administration, Supervision, Writing – review & editing. MM: Conceptualization, Investigation, Methodology, Writing – review & editing. VC-L: Conceptualization, Data curation, Funding acquisition, Investigation, Project administration, Resources, Supervision, Visualization, Writing – original draft, Writing – review & editing.
The author(s) declare that financial support was received for the research, authorship, and/or publication of this article. This work was funded by the National Institutes of Health (project numbers GM070641, ES026856, and ES024615) and the National Research Foundation of Singapore through the Singapore-MIT Alliance for Research and Technology Antimicrobial Resistance Interdisciplinary Research Group.
The authors thank Agnieszka Dziergowska (Lodz University of Technology) for synthesizing standards for LC-MS.
The authors declare that the research was conducted in the absence of any commercial or financial relationships that could be construed as a potential conflict of interest.
All claims expressed in this article are solely those of the authors and do not necessarily represent those of their affiliated organizations, or those of the publisher, the editors and the reviewers. Any product that may be evaluated in this article, or claim that may be made by its manufacturer, is not guaranteed or endorsed by the publisher.
The Supplementary material for this article can be found online at: https://www.frontiersin.org/articles/10.3389/fmicb.2024.1369018/full#supplementary-material
1. ^http://gtrnadb.ucsc.edu/GtRNAdb2/genomes/bacteria/Bart_hens_Houston_1/
2. ^http://gtrnadb.ucsc.edu/GtRNAdb2/genomes/bacteria/Bart_quin_Toulouse/
6. ^https://software.broadinstitute.org/morpheus
Abdel Rahman, A. A.-H., Wada, T., and Saigo, K. (2001). Facile methods for the synthesis of 5-formylcytidine. Tetrahedron Lett. 42, 1061–1063. doi: 10.1016/S0040-4039(00)02191-2
Addepalli, B., and Limbach, P. A. (2016). Pseudouridine in the anticodon of Escherichia coli tRNATyr(QΨA) is catalyzed by the dual specificity enzyme RluF. J. Biol. Chem. 291, 22327–22337. doi: 10.1074/jbc.M116.747865
Albalat, R., and Cañestro, C. (2016). Evolution by gene loss. Nat. Rev. Genet. 17, 379–391. doi: 10.1038/nrg.2016.39
Alsmark, C. M., Frank, A. C., Karlberg, E. O., Legault, B. A., Ardell, D. H., Canbäck, B., et al. (2004). The louse-borne human pathogen Bartonella quintana is a genomic derivative of the zoonotic agent Bartonella henselae. Proc. Natl. Acad. Sci. U. S. A. 101, 9716–9721. doi: 10.1073/pnas.0305659101
Altschul, S. F., Madden, T. L., Schaffer, A. A., Zhang, J., Zhang, Z., Miller, W., et al. (1997). Gapped BLAST and PSI-BLAST: a new generation of protein database search programs. Nucleic Acids Res. 25, 3389–3402. doi: 10.1093/nar/25.17.3389
Andersson, S. G. E., and Kurland, C. G. (1998). Reductive evolution of resident genomes. Trends Microbiol. 6, 263–268. doi: 10.1016/S0966-842X(98)01312-2
Antoine, L., Bahena-Ceron, R., Bunwaree, H. D., Gobry, M., Loegler, V., Romby, P., et al. (2021). Rna modifications in pathogenic bacteria: impact on host adaptation and virulence. Genes 12:1125. doi: 10.3390/genes12081125
Barraud, P., and Tisné, C. (2019). To be or not to be modified: miscellaneous aspects influencing nucleotide modifications in tRNAs. IUBMB Life 71, 1126–1140. doi: 10.1002/iub.2041
Bartosik, K., and Leszczynska, G. (2015). Synthesis of various substituted 5-methyluridines (xm5U) and 2-thiouridines (xm5s2U) via nucleophilic substitution of 5-pivaloyloxymethyluridine/2-thiouridine. Tetrahedron Lett. 56, 6593–6597. doi: 10.1016/j.tetlet.2015.10.023
Bateman, A., Martin, M.-J., Orchard, S., Magrane, M., Ahmad, S., Alpi, E., et al. (2023). UniProt: the universal protein knowledgebase in 2023. Nucleic Acids Res. 51, D523–D531. doi: 10.1093/nar/gkac1052
Battisti, J. M., and Minnick, M. F. (2008). Laboratory maintenance of Bartonella quintana. Curr. Protoc. Microbiol. 3:mc03c01s10. doi: 10.1002/9780471729259.mc03c01s10
Behm-Ansmant, I., Helm, M., and Motorin, Y. (2011). Use of specific chemical reagents for detection of modified nucleotides in RNA. J Nucleic Acids 2011:408053. doi: 10.4061/2011/408053
Benítez-Páez, A., Villarroya, M., Douthwaite, S., Gabaldón, T., and Armengod, M. E. (2010). YibK is the 2’-O-methyltransferase TrmL that modifies the wobble nucleotide in Escherichia coli tRNALeu isoacceptors. RNA 16, 2131–2143. doi: 10.1261/rna.2245910
Berg, M. D., and Brandl, C. J. (2021). Transfer RNAs: diversity in form and function. RNA Biol. 18, 316–339. doi: 10.1080/15476286.2020.1809197
Bishop, A. C., Xu, J., Johnson, R. C., Schimmel, P., and de Crécy-Lagard, V. (2002). Identification of the tRNA-dihydrouridine synthase family. J. Biol. Chem. 277, 25090–25095. doi: 10.1074/jbc.M203208200
Boccaletto, P., MacHnicka, M. A., Purta, E., Pitkowski, P., Baginski, B., Wirecki, T. K., et al. (2018). MODOMICS: a database of RNA modification pathways. 2017 update. Nucleic Acids Res. 46, D303–D307. doi: 10.1093/nar/gkx1030
Bou-Nader, C., Montémont, H., Guérineau, V., Jean-Jean, O., Brégeon, D., and Hamdane, D. (2018). Unveiling structural and functional divergences of bacterial tRNA dihydrouridine synthases: perspectives on the evolution scenario. Nucleic Acids Res. 46, 1386–1394. doi: 10.1093/nar/gkx1294
Broom, A. D., Townsend, L. B., Jones, J. W., and Robins, R. K. (1964). Purine nucleosides. VI. Further methylation studies of naturally occurring purine nucleosides. Biochemistry 3, 494–500. doi: 10.1021/bi00892a005
Chan, C. T. Y., Dyavaiah, M., DeMott, M. S., Taghizadeh, K., Dedon, P. C., and Begley, T. J. (2010). A quantitative systems approach reveals dynamic control of tRNA modifications during cellular stress. PLoS Genet. 6, e1001247–e1001249. doi: 10.1371/journal.pgen.1001247
Chan, P. P., and Lowe, T. M. (2016). GtRNAdb 2.0: an expanded database of transfer RNA genes identified in complete and draft genomes. Nucleic Acids Res. 44, D184–D189. doi: 10.1093/nar/gkv1309
Chow, S., Wen, K., Sanghvi, Y. S., and Theodorakis, E. A. (2003). Novel synthesis of 2′-O-methylguanosine. Bioorg. Med. Chem. Lett. 13, 1631–1634. doi: 10.1016/S0960-894X(03)00291-9
Corder, A. L., Subedi, B. P., Zhang, S., Dark, A. M., Foss, F. W., and Pierce, B. S. (2013). Peroxide-shunt substrate-specificity for the Salmonella typhimurium O 2 -dependent tRNA modifying monooxygenase (MiaE). Biochemistry 52, 6182–6196. doi: 10.1021/bi4000832
de Brouwer, A. P. M., Abou Jamra, R., Körtel, N., Soyris, C., Polla, D. L., Safra, M., et al. (2018). Variants in PUS7 cause intellectual disability with speech delay, microcephaly, short stature, and aggressive behavior. Am. J. Hum. Genet. 103, 1045–1052. doi: 10.1016/j.ajhg.2018.10.026
de Crécy-Lagard, V., and Jaroch, M. (2021). Functions of bacterial tRNA modifications: from ubiquity to diversity. Trends Microbiol. 29, 41–53. doi: 10.1016/j.tim.2020.06.010
de Crécy-Lagard, V., Marck, C., and Grosjean, H. (2012). Decoding in Candidatus Riesia pediculicola, close to a minimal tRNA modification set? Trends Cell Mol. Biol. 7, 11–34.
de Crécy-Lagard, V., Ross, R. L., Jaroch, M., Marchand, V., Eisenhart, C., Brégeon, D., et al. (2020). Survey and validation of tRNA modifications and their corresponding genes in Bacillus subtilis sp subtilis strain 168. Biomol. Ther. 10, 1–23. doi: 10.3390/biom10070977
Debiec, K., and Sochacka, E. (2021). Efficient access to 3′-O-phosphoramidite derivatives of tRNA related N6-threonylcarbamoyladenosine (t6A) and 2-methylthio-N6-threonylcarbamoyladenosine (ms2t6A). RSC Adv. 11, 1992–1999. doi: 10.1039/D0RA09803E
El Yacoubi, B., Bailly, M., and de Crécy-Lagard, V. (2012). Biosynthesis and function of posttranscriptional modifications of transfer RNAs. Annu. Rev. Genet. 46, 69–95. doi: 10.1146/annurev-genet-110711-155641
Engel, P., and Dehio, C. (2009). Genomics of host-restricted pathogens of the genus Bartonella. Genome Dyn. 6, 158–169. doi: 10.1159/000235769
Faivre, B., Lombard, M., Fakroun, S., Vo, C. D. T., Goyenvalle, C., Guérineau, V., et al. (2021). Dihydrouridine synthesis in tRNAs is under reductive evolution in Mollicutes. RNA Biol. 18, 2278–2289. doi: 10.1080/15476286.2021.1899653
Foucault, C., Brouqui, P., and Raoult, D. (2006). Bartonella quintana characteristics and clinical management. Emerg. Infect. Dis. 12, 217–223. doi: 10.3201/eid1202.050874
Fruchard, L., Babosan, A., Carvalho, A., Lang, M., Li, B., Duchateau, M., et al. (2022). Queuosine modification of tRNA-tyrosine elicits translational reprogramming and enhances growth of Vibrio cholerae with aminoglycosides. bioRxiv [Preprint], bioRxiv:2022.09.26.509455.
Gavriliu, D., Fossey, C., Fontaine, G., Benzaria, S., Ciurea, A., Delbederi, Z., et al. (2000). Synthesis and antiviral activity of C-5 substituted analogues of D4T bearing methylamino- or methyldiamino-linker arms. Nucleosides Nucleotides Nucleic Acids 19, 1017–1031. doi: 10.1080/15257770008033040
Grosjean, H., Breton, M., Sirand-Pugnet, P., Tardy, F., Thiaucourt, F., Citti, C., et al. (2014). Predicting the minimal translation apparatus: lessons from the reductive evolution of Mollicutes. PLoS Genet. 10:e1004363. doi: 10.1371/journal.pgen.1004363
Grosjean, H., de Crécy-Lagard, V., and Marck, C. (2010). Deciphering synonymous codons in the three domains of life: co-evolution with specific tRNA modification enzymes. FEBS Lett. 584, 252–264. doi: 10.1016/j.febslet.2009.11.052
Grosjean, H., and Westhof, E. (2016). An integrated, structure- and energy-based view of the genetic code. Nucleic Acids Res. 44, 8020–8040. doi: 10.1093/nar/gkw608
Hamma, T., and Ferre-D’Amare, A. R. (2006). Pseudouridine synthases. Chem. Biol. 13, 1125–1135. doi: 10.1016/j.chembiol.2006.09.009
Helm, M., and Alfonzo, J. D. (2014). Posttranscriptional RNA modifications: playing metabolic games in a cell’s chemical legoland. Chem. Biol. 21, 174–185. doi: 10.1016/j.chembiol.2013.10.015
Höbartner, C., Kreutz, C., Flecker, E., Ottenschläger, E., Pils, W., Grubmayr, K., et al. (2003). The synthesis of 2′-O-[(Triisopropylsilyl)oxy] methyl (TOM) phosphoramidites of pethylated Ribonucleosides (m1G, m2G, m22G, m1I, m3U, m4C, m6A, m62A) for use in automated RNA solid-phase synthesis. Monatshefte für Chemie 134, 851–873. doi: 10.1007/s00706-003-0592-1
Hyde, R. M., Broom, A. D., and Buckheit, R. W. (2003). Antiviral amphipathic oligo- and polyribonucleotides: analogue development and biological studies. J. Med. Chem. 46, 1878–1885. doi: 10.1021/jm0203276
Jager, G., Leipuviene, R., Pollard, M. G., Qian, Q., and Björk, G. R. (2004). The conserved Cys-X1-X2-Cys motif present in the TtcA protein is required for the thiolation of cytidine in position 32 of tRNA from Salmonella enterica serovar typhimurium. J. Bacteriol. 186, 750–757. doi: 10.1128/JB.186.3.750-757.2004
Jaroch, M., Sun, G., Tsui, H.-C. T., Reed, C., Sun, J., Jörg, M., et al. (2023). Alternate routes to mnm5s2U synthesis in gram-positive bacteria. bioRxiv [Preprint]. bioRxiv: 2023.12.21.572861.
Kanehisa, M., Furumichi, M., Sato, Y., Ishiguro-Watanabe, M., and Tanabe, M. (2021). KEGG: integrating viruses and cellular organisms. Nucleic Acids Res. 49, D545–D551. doi: 10.1093/nar/gkaa970
Kawai, G., Hashizume, T., Miyazawa, T., McCloskey, J. A., and Yokoyama, S. (1989). Conformational characteristics of 4-acetylcytidine found in tRNA. Nucleic Acids Symp. Ser. 21, 61–62.
Kimura, S., Dedon, P. C., and Waldor, M. K. (2020). Comparative tRNA sequencing and RNA mass spectrometry for surveying tRNA modifications. Nat. Chem. Biol. 16, 964–972.
Knoblauch, B. (1999). 5-substituted UTP derivatives as P2Y2 receptor agonists. Eur. J. Med. Chem. 34, 809–824. doi: 10.1016/S0223-5234(99)00211-1
Koh, C. S., and Sarin, L. P. (2018). Transfer RNA modification and infection – implications for pathogenicity and host responses. Biochimica et Biophysica Acta Gene Regul Mech 1861, 419–432. doi: 10.1016/j.bbagrm.2018.01.015
Kondo, T., Okamoto, K., Ohgi, T., and Goto, T. (1986). Syntheses of hypermodified nucleoside q, and its biosynthetic precursors preQ0 and preQ1. Tetrahedron 42, 207–213. doi: 10.1016/S0040-4020(01)87419-6
Koshla, O., Vogt, L. M., Rydkin, O., Sehin, Y., Ostash, I., Helm, M., et al. (2023). Landscape of post-transcriptional tRNA modifications in Streptomyces albidoflavus J1074 as portrayed by mass spectrometry and genomic data mining. J. Bacteriol. 205:e0029422. doi: 10.1128/jb.00294-22
Letunic, I., and Bork, P. (2021). Interactive tree of life (iTOL) v5: an online tool for phylogenetic tree display and annotation. Nucleic Acids Res. 49, W293–W296. doi: 10.1093/nar/gkab301
Loterio, R. K., Zamboni, D. S., and Newton, H. J. (2021). Keeping the host alive—lessons from obligate intracellular bacterial pathogens. Pathog Dis 79:ftab052. doi: 10.1093/femspd/ftab052
Luvino, D., Smietana, M., and Vasseur, J.-J. (2006). Selective fluorescence-based detection of dihydrouridine with boronic acids. Tetrahedron Lett. 47, 9253–9256. doi: 10.1016/j.tetlet.2006.10.150
Marchand, V., Ayadi, L., Ernst, F. G. M., Hertler, J., Bourguignon-Igel, V., Galvanin, A., et al. (2018). AlkAniline-Seq: profiling of m7G and m3C RNA modifications at single nucleotide resolution. Angewandte Chemie 57, 16785–16790. doi: 10.1002/anie.201810946
Marchand, V., Blanloeil-Oillo, F., Helm, M., and Motorin, Y. (2016). Illumina-based RiboMethSeq approach for mapping of 2’-O-me residues in RNA. Nucleic Acids Res. 44:e135. doi: 10.1093/nar/gkw547
Marchand, V., Bourguignon-Igel, V., Helm, M., and Motorin, Y. (2021). Mapping of 7-methylguanosine (m7G), 3-methylcytidine (m3C), dihydrouridine (D) and 5-hydroxycytidine (ho5C) RNA modifications by AlkAniline-Seq. Methods Enzymol., 25–47. doi: 10.1016/bs.mie.2021.06.001
Marchand, V., Pichot, F., Thüring, K., Ayadi, L., Freund, I., Dalpke, A., et al. (2017). Next-generation sequencing-based ribomethseq protocol for analysis of tRNA 2’-O-methylation. Biomol. Ther. 7:13. doi: 10.3390/biom7010013
McClure, E. E., Chávez, A. S. O., Shaw, D. K., Carlyon, J. A., Ganta, R. R., Noh, S. M., et al. (2017). Engineering of obligate intracellular bacteria: Progress, challenges and paradigms. Nat. Rev. Microbiol. 15, 544–558. doi: 10.1038/nrmicro.2017.59
Melnikov, S. V., van den Elzen, A., Stevens, D. L., Thoreen, C. C., and Söll, D. (2018). Loss of protein synthesis quality control in host-restricted organisms. Proc. Natl. Acad. Sci. U. S. A. 115, E11505–E11512. doi: 10.1073/pnas.1815992115
Miles, R. W., Samano, V., and Robins, M. J. (1995). Nucleic acid related compounds. 86. Nucleophilic functionalization of adenine, adenosine, tubercidin, and formycin derivatives via elaboration of the heterocyclic amino group into a readily displaced 1,2,4-triazol-4-yl substituent. J. Am. Chem. Soc. 117, 5951–5957. doi: 10.1021/ja00127a007
Motorin, Y., and Marchand, V. (2018). Detection and analysis of RNA ribose 2’-O-methylations: challenges and solutions. Genes 9:642. doi: 10.3390/genes9120642
Motorin, Y., Muller, S., Behm-Ansmant, I., and Branlant, C. (2007). Identification of modified residues in RNAs by reverse transcription-based methods. Methods Enzymol. 425, 21–53. doi: 10.1016/S0076-6879(07)25002-5
Myllykallio, H., Sournia, P., Heliou, A., and Liebl, U. (2018). Unique features and anti-microbial targeting of folate- and flavin-dependent methyltransferases required for accurate maintenance of genetic information. Front. Microbiol. 9:918. doi: 10.3389/fmicb.2018.00918
NCBI Resource Coordinators (2016). Database resources of the National Center for biotechnology information. Nucleic Acids Res. 44, D7–D19. doi: 10.1093/nar/gkv1290
Nyilas, A., Chattopadhyaya, J., Drake, A., Öberg, B., and Chattopadhyaya, J. (1986). Synthesis of O2′-methyluridine, O2’-methylcytidine, N4,O2’-dimethylcytidine and N4,N4,O2’-trimethylcytidine from a common intermediate. Acta Chem. Scand. 40b, 826–830. doi: 10.3891/acta.chem.scand.40b-0826
Olson, R. D., Assaf, R., Brettin, T., Conrad, N., Cucinell, C., Davis, J. J., et al. (2023). Introducing the bacterial and viral bioinformatics resource center (BV-BRC): a resource combining PATRIC, IRD and ViPR. Nucleic Acids Res. 51, D678–D689. doi: 10.1093/nar/gkac1003
Pagano, A. R., Lajewski, W. M., and Jones, R. A. (1995). Syntheses of [6,7-15N]-adenosine, [6,7-15N]-2’-deoxyadenosine, and [7-15N]-hypoxanthine. J. Am. Chem. Soc. 117, 11669–11672. doi: 10.1021/ja00152a006
Persson, B. C. (1993). Modification of tRNA as a regulatory device. Mol. Microbiol. 8, 1011–1016. doi: 10.1111/j.1365-2958.1993.tb01645.x
Quaiyum, S., Yuan, Y., Giuanxin, S., Ratnayakec, R. M. M., Hutinet, G., Dedon, P. C., et al. (2023). Queuosine salvage in Bartonella henselae Houston 1: a unique evolutionary path. bioRxiv [Preprint], bioRxiv:2023.12.05.570228.
Raoult, D. (2007). From cat scratch disease to Bartonella henselae infection. Clin. Infect. Dis. 45, 1541–1542. doi: 10.1086/523716
Robin, A., Julienne, K., Meslin, J., and Deniaud, D. (2006). Straightforward pyrimidine ring construction: a versatile tool for the synthesis of nucleobase and nucleoside analogues. European J Org Chem 2006, 634–643. doi: 10.1002/ejoc.200500556
Rovid Spickler, A. (2005). Cat Scratch Disease. Available at: www.cfsph.iastate.edu
Sato, T., Hayakawa, Y., and Noyori, R. (1984). A stereocontrolled total synthesis of C -nucleosides. Bull. Chem. Soc. Jpn. 57, 2515–2525. doi: 10.1246/bcsj.57.2515
Schaefer, M., Pollex, T., Hanna, K., and Lyko, F. (2009). RNA cytosine methylation analysis by bisulfite sequencing. Nucleic Acids Res. 37:e12. doi: 10.1093/nar/gkn954
Segers, F. H., Kešnerová, L., Kosoy, M., and Engel, P. (2017). Genomic changes associated with the evolutionary transition of an insect gut symbiont into a blood-borne pathogen. ISME J. 11, 1232–1244. doi: 10.1038/ismej.2016.201
Sun, L., and Singer, B. (1974). Reaction of cytidine with ethylating agents. Biochemistry 13, 1905–1913. doi: 10.1021/bi00706a020
Thorvaldsdóttir, H., Robinson, J. T., and Mesirov, J. P. (2012). Integrative genomics viewer (IGV): high-performance genomics data visualization and exploration. Brief. Bioinform. 14, 178–192. doi: 10.1093/bib/bbs017
Tomasi, F. G., Kimura, S., Rubin, E. J., and Waldor, M. K. (2023). A tRNA modification in Mycobacterium tuberculosis facilitates optimal intracellular growth. eLife 12:RP87146. doi: 10.7554/eLife.87146
Van Aerschot, A. A., Mamos, P., Weyns, N. J., Ikeda, S., De Clercq, E., and Herdewijn, P. A. (1993). Antiviral activity of C-alkylated purine nucleosides obtained by cross-coupling with tetraalkyltin reagents. J. Med. Chem. 36, 2938–2942. doi: 10.1021/jm00072a013
van Montagu, M., Molemans, F., and Stockx, J. (1968). Preparation of cytidine, cytidylic acids and ribonucleic acid specifically acetylated in the exocyclic amino group of cytosine. Bull Soc Chim Belges 77, 171–179. doi: 10.1002/bscb.19680770307
Vangaveti, S., Cantara, W. A., Spears, J. L., DeMirci, H., Murphy, F. V., Ranganathan, S. V., et al. (2020). A structural basis for restricted codon recognition mediated by 2-thiocytidine in tRNA containing a wobble position inosine. J. Mol. Biol. 432, 913–929. doi: 10.1016/j.jmb.2019.12.016
Wang, R., Haruehanroengra, P., and Sheng, J. (2017). Synthesis of geranyl-2-thiouridine-modified RNA. Curr. Protoc. Nucleic Acid Chem. 68:4.72.1-4.72.13. doi: 10.1002/cpnc.22
Wang, L., and Lin, S. (2023). Emerging functions of tRNA modifications in mRNA translation and diseases. J. Genet. Genomics 50, 223–232. doi: 10.1016/j.jgg.2022.10.002
Werner, S., Schmidt, L., Marchand, V., Kemmer, T., Falschlunger, C., Sednev, M. V., et al. (2020). Machine learning of reverse transcription signatures of variegated polymerases allows mapping and discrimination of methylated purines in limited transcriptomes. Nucleic Acids Res. 48, 3734–3746. doi: 10.1093/nar/gkaa113
Xu, J., Green, N. J., Russell, D. A., Liu, Z., and Sutherland, J. D. (2021). Prebiotic photochemical coproduction of purine ribo- and deoxyribonucleosides. J. Am. Chem. Soc. 143, 14482–14486. doi: 10.1021/jacs.1c07403
Keywords: intracellular pathogens, tRNA modification, mass spectrometry, next-generation sequencing, Bartonella
Citation: Quaiyum S, Sun J, Marchand V, Sun G, Reed CJ, Motorin Y, Dedon PC, Minnick MF and de Crécy-Lagard V (2024) Mapping the tRNA modification landscape of Bartonella henselae Houston I and Bartonella quintana Toulouse. Front. Microbiol. 15:1369018. doi: 10.3389/fmicb.2024.1369018
Received: 11 January 2024; Accepted: 26 February 2024;
Published: 13 March 2024.
Edited by:
Jiqiang Ling, University of Maryland, United StatesReviewed by:
Assaf Katz, University of Chile, ChileCopyright © 2024 Quaiyum, Sun, Marchand, Sun, Reed, Motorin, Dedon, Minnick and de Crécy-Lagard. This is an open-access article distributed under the terms of the Creative Commons Attribution License (CC BY). The use, distribution or reproduction in other forums is permitted, provided the original author(s) and the copyright owner(s) are credited and that the original publication in this journal is cited, in accordance with accepted academic practice. No use, distribution or reproduction is permitted which does not comply with these terms.
*Correspondence:Valérie de Crécy-Lagard, dmNyZWN5QHVmbC5lZHU=
†These authors have contributed equally to this work
Disclaimer: All claims expressed in this article are solely those of the authors and do not necessarily represent those of their affiliated organizations, or those of the publisher, the editors and the reviewers. Any product that may be evaluated in this article or claim that may be made by its manufacturer is not guaranteed or endorsed by the publisher.
Research integrity at Frontiers
Learn more about the work of our research integrity team to safeguard the quality of each article we publish.