- 1Department of Biological Sciences, Florida International University, Miami, FL, United States
- 2Biomolecular Sciences Institute, Florida International University, Miami, FL, United States
- 3Department of Chemistry and Biochemistry, Florida International University, Miami, FL, United States
Introduction: Endosymbiotic Wolbachia bacteria are widespread in nature, present in half of all insect species. The success of Wolbachia is supported by a commensal lifestyle. Unlike bacterial pathogens that overreplicate and harm host cells, Wolbachia infections have a relatively innocuous intracellular lifestyle. This raises important questions about how Wolbachia infection is regulated. Little is known about how Wolbachia abundance is controlled at an organismal scale.
Methods: This study demonstrates methodology for rigorous identification of cellular processes that affect whole-body Wolbachia abundance, as indicated by absolute counts of the Wolbachia surface protein (wsp) gene.
Results: Candidate pathways, associated with well-described infection scenarios, were identified. Wolbachia-infected fruit flies were exposed to small molecule inhibitors known for targeting those same pathways. Sequential tests in D. melanogaster and D. simulans yielded a subset of chemical inhibitors that significantly affected whole-body Wolbachia abundance, including the Wnt pathway disruptor, IWR-1 and the mTOR pathway inhibitor, Rapamycin. The implicated pathways were genetically retested for effects in D. melanogaster, using inducible RNAi expression driven by constitutive as well as chemically-induced somatic GAL4 expression. Genetic disruptions of armadillo, tor, and ATG6 significantly affected whole-body Wolbachia abundance.
Discussion: As such, the data corroborate reagent targeting and pathway relevance to whole-body Wolbachia infection. The results also implicate Wnt and mTOR regulation of autophagy as important for regulation of Wolbachia titer.
Introduction
Resident intracellular microbes, referred to as endosymbionts, are widespread in nature. Endosymbiotic microbes are commonly thought of as mutualists, in which the interaction between host and microbe benefits both partners of the symbiosis. However, endosymbionts can also exhibit relatively inert (commensal) or detrimental (parasitic) interactions with a host organism. Evidence suggests that some commensal and/or mutualistic microbes are descendants of formerly parasitic ancestors (Sachs et al., 2014). Other endosymbionts have been found to exhibit context-dependent plasticity in their symbiotic interactions, as seen in Salmonella, which is carried innocuously by chickens, but causes severe infection in humans (Foley et al., 2013). To account for this diversity, intracellular bacteria are now described in terms of a symbiotic spectrum, ranging from mutualistic to parasitic (Lewis, 1985).
For any endosymbiont, high infection prevalence in host populations is the mark of success. Members of the Wolbachia genus are naturally widespread bacterial endosymbionts, carried in certain lineages of mites, crustaceans, nematodes and in about 50% of all insect species (Sazama et al., 2019). Wolbachia are often described as reproductive parasites because some strains induce parthenogenesis, male-killing, feminization or cytoplasmic incompatibility, which ultimately favor the success of infected females (Werren et al., 2008). In other instances, Wolbachia have been found to serve as mutualists, by sustaining host viability and reproduction (Taylor et al., 2005; Pannebakker et al., 2007; Landmann et al., 2011), as well as by repelling harmful viral infections in the host (Hedges et al., 2008; Teixeira et al., 2008; Cogni et al., 2021). Due to absence of evident benefits or detriments, Wolbachia infections can often be described as commensal. The Wolbachia-host symbiosis thus provides a new and valuable perspective for investigating, defining, and understanding the cellular basis of commensalism.
To date, Wolbachia studies have shared an interest in understanding how endosymbiont amount (titer) is specified within tissue culture cells, dissected host tissues, and whole organisms. Wolbachia titer has been assessed as a function of various host/strain combinations as well as in response to host age, crowding, temperature, diet, genetic background, microbiota, and chemical exposure (Hoffmann et al., 1998; Veneti et al., 2004; Unckless et al., 2009; Wiwatanaratanabutr and Kittayapong, 2009; Voronin et al., 2012; Ali et al., 2019; López-Madrigal and Duarte, 2019). A patchwork of cytological and qPCR-based methods have been used across assessments of Wolbachia abundance in vivo, with shared recognition that cellular processes interacting with Wolbachia may also affect Wolbachia abundance within the host (López-Madrigal and Duarte, 2019). The field is now in a position to investigate more broadly how Wolbachia-host interactions inform mechanisms of infection.
Fundamental questions remain regarding the involvement of host cellular processes in endosymbiotic infection. It is not clear whether signaling pathways relevant to Wolbachia infection have been fully identified, nor which relays of those relays affect in vivo Wolbachia titer most strongly. It also remains unclear what mechanistic attributes distinguish commensal infections from detrimental scenarios. To this end, this study asked whether Wolbachia titer is affected by the same host cellular pathways as commonly studied bacterial infections. 14 candidate host pathways and processes were tested, using complementary chemical and genetic tools. Whole-body Wolbachia abundance was assessed by real-time qPCR, to determine absolute counts of the Wolbachia surface protein (wsp) gene. This work yielded a subset of host functions for further pursuit, with implications for the basis of commensalism.
Materials and methods
Drosophila stocks and maintenance
Two fly strains were used in this study. Preliminary screening was performed using Drosophila melanogaster of the genotype w; Sp/Cyo; Sb/TM6B carrying the endogenous wMel Wolbachia strain (Serbus and Sullivan, 2007; Christensen et al., 2016). Drosophila simulans (D. sim) infected with the endogenous Wolbachia riverside (wRi) strain were used for further analyses (Hoffmann et al., 1990; Serbus and Sullivan, 2007). GAL4 lines sourced from the Bloomington Drosophila Stock Center (BDSC) were also used to drive RNAi expression. Constitutive somatic expression was driven by the Actin5C-GAL4 driver w; P{Act5C-GAL4-w}E1/Cyo (BDSC# 25374) and the daughterless-GAL4 driver w; P{w+, GMR12B08-GAL4}attP2 (BDSC# 48489). Mifepristone-induced gene expression was driven by the GeneSwitch-GAL4 driver yw {hs-FLP}; {w+, UAS-GFP}; {w+, Act5C-GS-GAL4}/TM6B, Tb (BDSC #9431).
Fruit flies were maintained in plastic bottles/vials containing standard fly food media. The recipe was derived from Bloomington Drosophila Stock Center as described previously (Christensen et al., 2016). The flies were raised in an Invictus Drosophila incubator at 25°C, under standard 12:12 h light–dark cycle. For the experiments, “0-day old” flies were collected and kept on standard fly food medium for 2 days. The flies were then used for drug treatments in vials or within a plate assay format as described previously (Christensen et al., 2019). Only female flies were used for the plate-based screening experiments, to reduce possible variation in population behavior per well.
Chemical food preparation
Two or more chemicals were used to alter the functionality of each of the candidate host processes pursued in this study. Where possible, compounds with opposite effects on the process of interest were included, such as the microtubule-depolymerizing drug, colchicine, and the microtubule-stabilizing drug, taxol, as well as the phospholipase C (PLC) inhibitor, U73122, and the PLC activator, 3-M3MFBS. The final list included a total of 37 candidate compounds (Supplementary Table S1). All the drugs were dissolved in DMSO. Most stock solutions, including rifampicin, were prepared in advance as 10 mM solutions, aliquoted, and stored at −20°C. Rapamycin was ordered as a 5 mM solution in DMSO, also stored at −20°C. Light-sensitive drugs were stored in the dark.
Immediately before use, chemical stocks were thawed and diluted 100X into fly food that had been re-melted, then cooled. Control vials, prepared in parallel with the chemical treatment vials, were treated with equivalent amounts of DMSO alone. In all cases, the final concentration of DMSO in food was capped at 1%. For the chemical screen, a minimum of 10 mL drug food was prepared per condition, to be further dispensed in approximately 1 mL amounts per treatment well. For drug lethality tests and GS-GAL4 induction experiments that were carried out in vials, food containing control DMSO and DMSO-solubilized compounds was prepared in larger volumes, to be dispensed into vials as 5 mL final amounts. After pouring, plates and vials were cooled and solidified in the fume hood, with foil wrappings used to protect light-sensitive compounds. Treatment vials were stored in Ziplock bags at 4°C as needed.
For the chemical screen,10 female flies were transferred to each treatment well. A DMSO-solubilized rifampicin control was also run on every qPCR plate to confirm the ongoing capacity of Wolbachia to respond to compound treatments. After 3 days of chemical feeding, pools of 5 female flies were removed from each treatment well and processed as a group for wsp quantification using real-time qPCR. For the vial-based experiments that assessed drug lethality, flies were incubated in groups of 12, 6 females and 6 males per vial, with viability scored every 3 days. Flies were transferred to new treatment vials at day 6, using vials from the 4°C fridge that had been re-warmed. For vial-based experiments using DMSO and mifepristone, flies were incubated on treatment food in groups of 15 females and 5 males. Flies were transferred to new treatment vials every 3 days, using DMSO and mifepristone vials from the 4°C fridge that had been re-warmed. After 14 days of feeding were completed (Haselton et al., 2010; Serbus et al., 2015), pools of 5 female flies were removed from each vial and processed as a group for wsp quantification using real-time qPCR.
Genetic manipulations
To incorporate Wolbachia into GAL4 driver lines, the driver males were crossed to virgin females of the genotype w; Sp/Cyo; Sb/TM6B, carrying the wMel Wolbachia strain (Christensen et al., 2016), which in this study is referred to as DB wMel. F1 progeny were backcrossed to the parental lines to establish Wolbachia-infected driver stocks, with the same genotypes as the originally uninfected lines.
Genetic disruptions were achieved using VALIUM20 transgenic RNAi lines which depend on short hairpin RNA, also known as artificial microRNAs, to trigger gene silencing in both somatic and germline cells (Ni et al., 2011). Each host pathway was tested by two different UAS-shRNA responder lines (Supplementary Table S2), selected in accord with pathways targeted by “hit” compounds from the chemical screen. To generate RNAi-expressing flies, wMel-infected virgin females were selected from freshly emerging bottles of each GAL4 driver stock. These females were crossed to males that carried responder UAS (upstream activating sequence) elements adjacent to a promoter that drives shRNA production (Ni et al., 2011). The parent flies were removed from the vials after 3–4 days of mating. Emerging F1 flies were collected in daily cohorts and aged for 5 days. The F1s that carried the GAL4 driver and the UAS responder were identified by phenotypic markers and separated within each cohort. Control siblings that contained either the GAL4 or UAS responder, but not both, were collected when available. In some cases, a separate control set was also generated in parallel by outcrossing Wolbachia-infected driver females to Oregon R (OreR) males. In all cases, control and treatment groups were generated and processed in parallel for wsp quantification.
DNA extraction and qPCR for whole-body Wolbachia quantification
Real-time qPCR was used to assess whole-body Wolbachia abundance, using the candidate gene wsp as a proxy for Wolbachia genomes per sample. Because Wolbachia reportedly carry one genome per bacterial cell (McGarry et al., 2004), resulting genome counts are expected to represent Wolbachia abundance per sample. DNA was extracted from pools of 5 female flies as per established methods (Christensen et al., 2019). Absolute measurements of the wsp gene from the extracted DNA samples were compared against reference plasmid standards, specifically a PGEM-T vector carrying a 160 bp PCR-amplified fragment of the wsp gene (Christensen et al., 2016). Real-time qPCR was carried out on a Bio-Rad CFX96 Connect Optics Module Real-Time System. Absolute wsp copy numbers were obtained by comparing cycle threshold (Ct) values to the standard curve generated from the plasmid standard. The wsp amplification primers were: Fwd 5’ CATTGGTGTTGGTGTTGGTG 3′ and Rev. 5’ ACCGAAATAACGAGCTCCAG 3′, used at 5 μM (Christensen et al., 2016).
Data display and analyses
Graphical displays showing “normalized” wsp counts as a scatter plot were created for display purposes only. To generate such graphs, median wsp counts for the DMSO controls per replicate were identified, then compared to the median wsp count of the entire dataset. A scaling factor was then identified and applied to each replicate, to normalize the median wsp value for the DMSO control and all associated experimental data. The raw absolute count data are available for review as needed (Supplementary material S1). Statistical analyses were conducted on raw (non-normalized) data within each experimental replicate for all experiments. Statistics appropriate to data normality and homogeneity were identified and applied as previously (Christensen et al., 2019). Power analysis was performed with an alpha set at 0.05 using a MATLAB-based data sub-sampling program, designed by Dr. Philip K. Stoddard. This program has the advantage that analyses can be customized to the statistical test appropriate to each dataset (Christensen et al., 2019). All statistical analysis worksheets for each experiment performed are also available (Supplementary material S1).
Results
Identifying and targeting candidate host processes relevant to bacterial infection
A literature search was first conducted to assess how intracellular bacterial abundance is regulated in commonly studied bacterial infections. After assessing 52 species from 17 genera, 26 bacterial species were identified, for which host gene/pathway effects on density regulation had been discussed (Supplementary Table S3). Of these, the literature highlighted 14 host mechanisms that altered the intracellular abundance of multiple bacterial classes (Supplementary Table S4; Supplementary material S2). Because these mechanisms were identified as more commonly involved in host–microbe interactions, they were prioritized for testing in the Wolbachia-Drosophila endosymbiosis model. Candidate compounds known to target each process were selected, with two or more compounds identified for testing each of the 14 classes of host targets. This culminated in the selection of 37 total candidate compounds to test for effects on whole-body Wolbachia titer (Table 1).
Host-directed small molecules alter wsp abundance in adult Drosophila hosts
The impact of the candidate compounds on whole-body Wolbachia titer was screened by absolute quantification of wsp by real-time qPCR (Kim et al., 2008; Serbus et al., 2012; Markstein et al., 2014; Christensen et al., 2019). D. melanogaster flies, carrying the endogenous wMel Wolbachia strain, termed DB wMel, were exposed for 3 days to food supplemented with DMSO-solubilized drugs or DMSO alone as a control. Treatments were initially tested for impact on whole-body wsp across two independent plate replicates. Those treatments which significantly changed wsp abundance in both plates were identified as preliminary hits. Of 37 chemicals tested, the primary screen identified 16 compounds were identified as meeting this criterion. These preliminary hit compounds were re-tested for reproducibility in a third plate replicate. 11 compounds were reconfirmed as hits, implicating a total of 9 host pathways and processes (Figure 1A). Most “hit” compounds elicited an increase in whole-body wsp abundance, with median values ranging 6–57% higher than the control (p < 0.001–0.036, n = 6 per plate replicate). The only exception was bortezomib, which reduced wsp to 48–71% of the DMSO control (p < 0.001, n = 6 amplifications per plate replicate) (Figure 1A; Table 2).
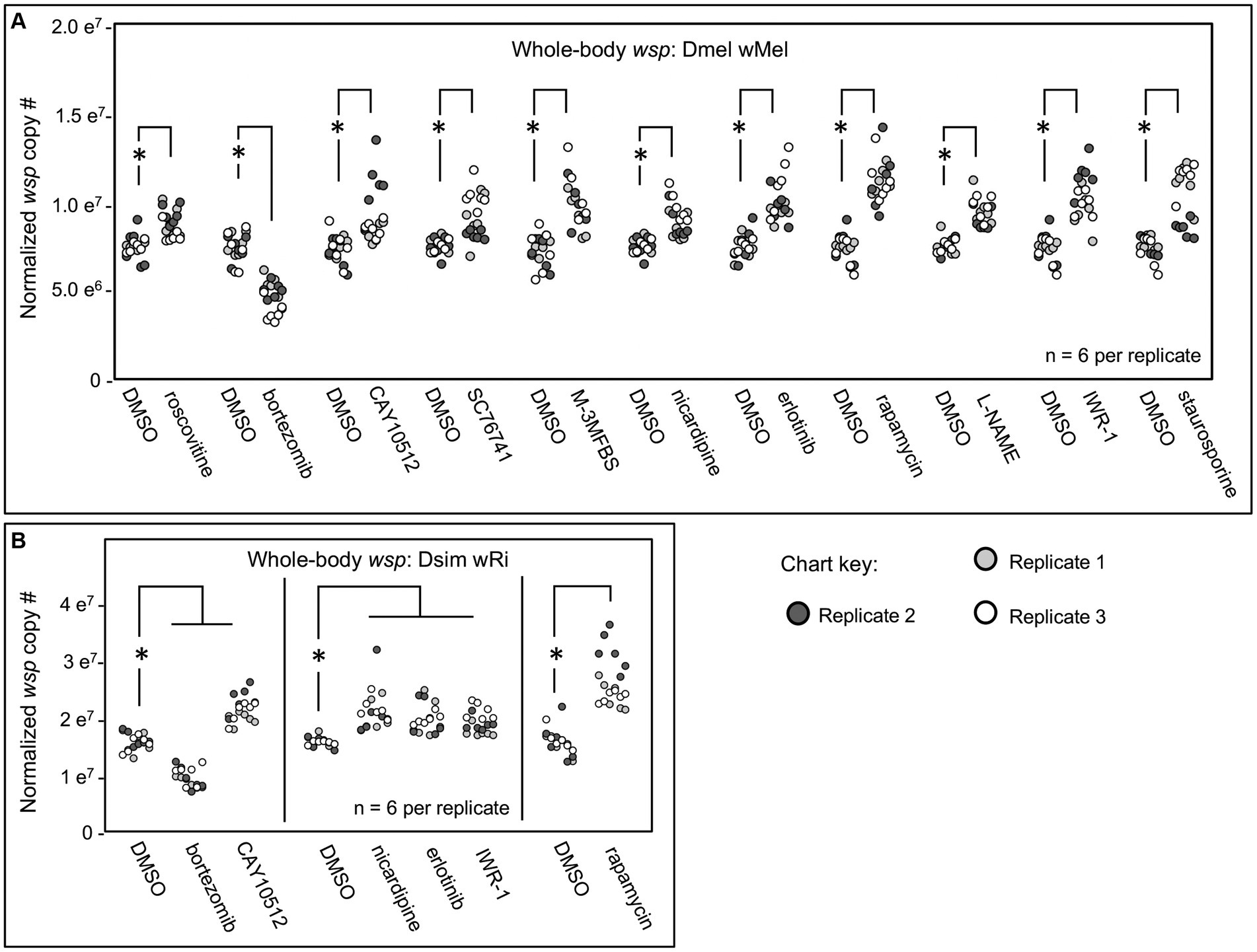
Figure 1. Whole body wsp abundance in response to chemical treatments. Display shows DMSO controls normalized across replicates, and the corresponding drug treatment data scaled accordingly. (A) Chemical treatment effects on whole-body wsp abundance in Dmel wMel. (B) Chemical treatment effects on whole-body wsp abundance in Dsim wRi. Significance was set at * p < 0.05, and is displayed only for conditions where all replicates met this standard.
To investigate a role for candidate processes across systems, the hits from DB wMel were retested against the D. simulans (Dsim) model, which naturally carries the wRi Wolbachia strain. The Dsim wRi re-screen identified a subset of 6 compounds that significantly affected whole-body wsp counts across 3 plate replicates (Figure 1B). 5 compounds increased whole-body wsp abundance to 15–52% higher than the DMSO control (p-value range: <0.001–0.041, n = 6 per plate replicate). These hits were associated with host Imd signaling, Calcium signaling, Ras/mTOR signaling, and Wnt signaling functions. By contrast, the proteasome inhibitor bortezomib continued to reduce wsp counts to 56–69% of the DMSO control (p < 0.001, n = 6 amplifications per plate replicate) (Figure 1B; Table 2).
To further investigate why some chemical treatments increase wsp counts, but others suppress wsp, a lethality assay was conducted. Flies were exposed to each of the “hit” compounds for a 12-day period. Bortezomib induced high lethality by the 6-day exposure timepoint for both DB wMel and Dsim wRi (Figures 2A,C). Thus, it is possible that wsp reductions by bortezomib reflect a Wolbachia response to toxic host conditions. However, flies exposed to all other “hit” compounds exhibited viability profiles comparable to DMSO controls, as per the example of IWR-1 (Figures 2B,D) (Supplementary Figure S1). Because the non-lethal “hit” compounds were all shown to elevate wsp counts, these results suggest that functions of multiple host pathways normally reduce whole-body Wolbachia loads.
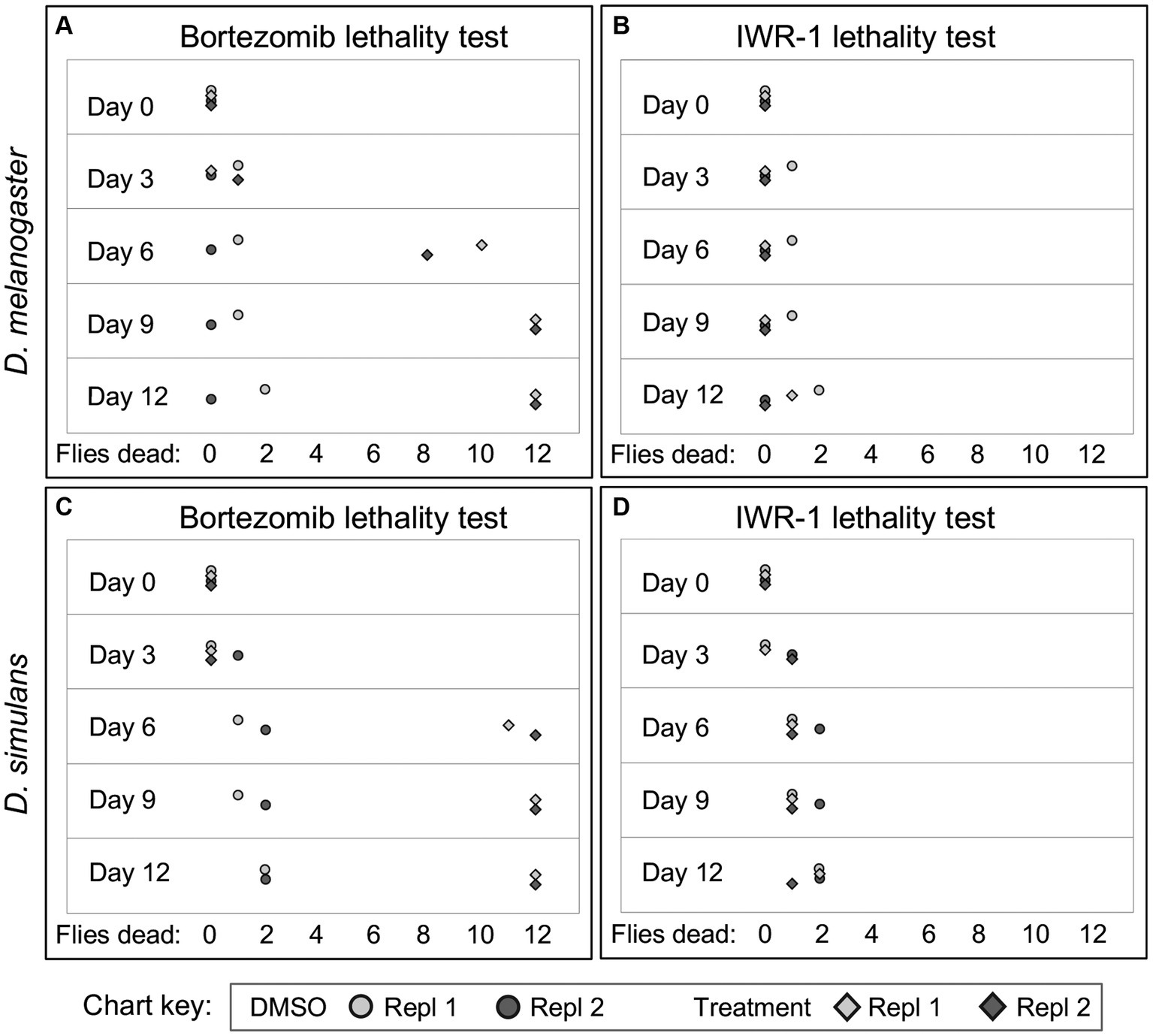
Figure 2. Example figure showing lethality data for bortezomib and IWR-1 compounds in D. melanogaster and D. simulans. (A–D) Depict the lethality effects two representative compounds, bortezomib (A,C) and IWR-1 (B,D), on D. melanogaster and D. simulans. Each panel shows the number of dead flies at different time points (days) following exposure across two independent replicates. Circles: Control groups treated with 1% DMSO vehicle only. Diamonds: Experimental groups treated with bortezomib (A,C) or IWR-1 (B,D).
Constitutive RNAi disruptions corroborate a subset of host pathway effects on wsp
To confirm the basis for host cellular effects on whole-body Wolbachia titer, genetic disruption experiments were performed, focusing on the pathways dually implicated by chemical screening of DB wMel and Dsim wRi. We used the GAL4::UAS system in D. melanogaster, which enables directed manipulation of gene expression (Brand and Perrimon, 1993; Duffy, 2002). In this case, the GAL4::UAS system was set to drive expression of short hairpin RNAi suppress the corresponding gene product (Perrimon et al., 2010; Ni et al., 2011; Perkins et al., 2015). The wMel strain was crossed into well-established GAL4 driver lines that drive constitutive whole-body expression, including the reputedly “strong” Actin5C-GAL4 driver (Act-5C), and the “milder” daughterless-GAL4 driver (da-GAL4) (Supplementary Table S5). Few to no F1 progeny were recovered that carried Act5C-GAL4 as well as UAS-shRNA chromosomes, indicating lethality for such genetic combinations. However, crossing the UAS-shRNA lines to da-GAL4 (Serbus et al., 2015) yielded ample RNAi-expressing F1 progeny for analysis.
No changes in wsp abundance were detected in response to constitutive shRNA disruption of Calcium signaling by knockdown of L-type calcium channels encoded by Ca-alpha1D and Cac. Inconsistent effects on wsp abundance were associated with disruptions to the Imd pathway by knockdown of NF-kappa-B/Rel, and the Rel activator, Tak1. Similar inconsistencies were observed for knockdown of the Wnt pathway gene, shaggy (sgg) gene (Supplementary material S1).
Constitutive shRNA disruption of the Wnt pathway gene armadillo (arm) yielded positive effects, increasing median whole-body wsp counts to 9–15% above the OreR-outcrossed control (p-value range: <0.001–0.034, n = 6 per plate replicate) (Figure 3). A significant wsp increase was also detected for the Ras/mTOR pathway, with tor disruption flies exhibiting higher whole-body wsp counts at 23–31% above both sibling controls and OreR-outcrossed controls (p < 0.001, n = 6 per plate replicate) (Figure 3). Ras/mTOR signaling was also retested by knockdown of the Epidermal Growth Factor Receptor (EGFR). Compared to OreR-outcrossed controls, EGFR knockdowns did not consistently affect wsp abundance measurements. However, in comparison to sibling controls, EGFR disruption yielded a 35–44% increase in median wsp counts (p-value range: <0.001–0.002, n = 6 per plate replicate) (Figure 3). Thus, detection of wsp responses to EGFR disruption may be context-dependent.
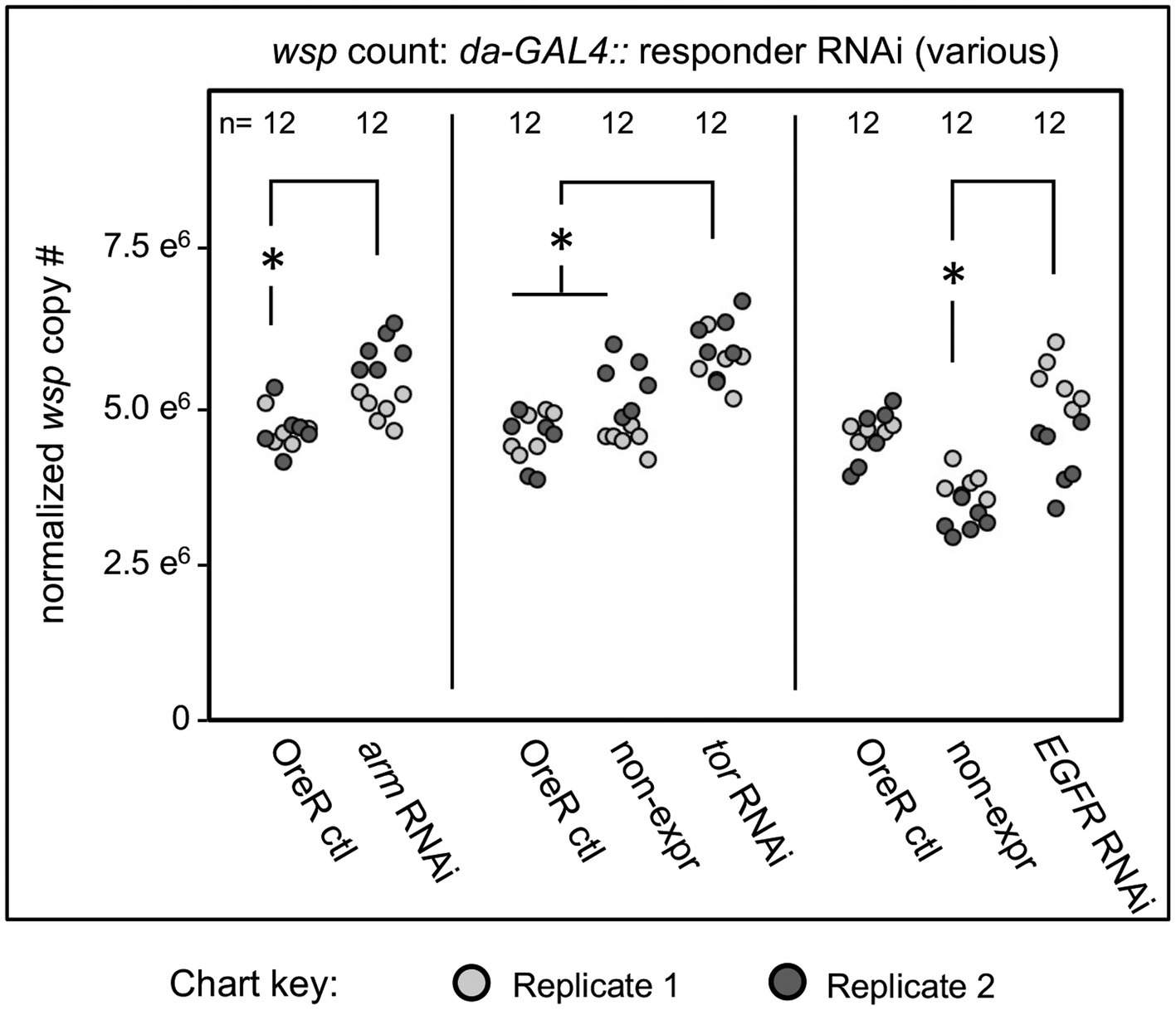
Figure 3. Whole body wsp abundance in control vs. da-GALA:UAS-RNAi knockdown flies. Oregon-R outcross controls are included throughout, and non-expressing sibling controls are shown where available. Display shows OreR controls normalized across replicates, and the corresponding conditions scaled accordingly. Panel shows data from 2 independent biological replicates. RNAi-expressing conditions shown from left to right are: armadillo, tor, and EGFR Significance was set at * p < 0.05, and is displayed only for conditions where all replicates met this standard.
To confirm an effect of Wnt and Ras/mTOR pathways on Wolbachia, the most consistent outcomes from the da-GAL4::UAS-shRNA experiments were retested. Arm RNAi elicited a 16–50% increase in median wsp abundance over the OreR-outcrossed control (p < 0.001, n = 18) (Figure 4A). Power analysis indicated the arm RNAi outcome to be robust (β < 0.003 at n 12; total n = 18) (Figure 4B). tor RNAi triggered a 38–39% increase in wsp abundance as compared to OreR-outcrossed controls (p < 0.001, n = 18) (Figure 4C), a finding also well-supported by power analysis (β < 0.002 at n 4; total n = 18) (Figure 4D). Taken together, these data indicate that constitutive RNAi disruption of Wnt and Ras/mTOR signaling increases whole-body Wolbachia titer.
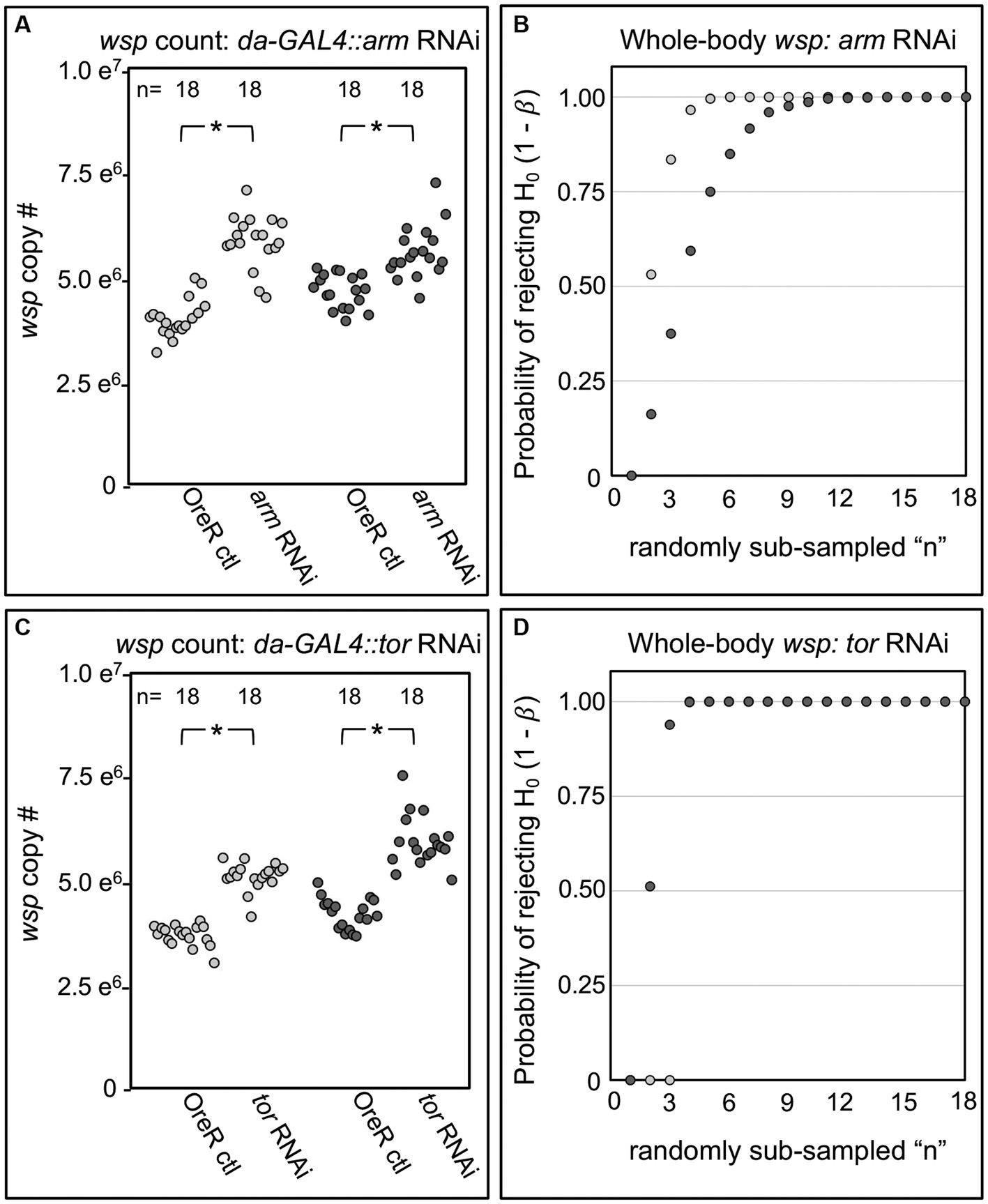
Figure 4. Whole body wsp abundance in control vs. da-GAL4::UAS-RNAi knockdown fies. Panels show data from 2 independent biological replicates. (A) Whole-body wsp abundance in arm-RNAi conditions. (B) Power analysis, testing the likelihood of significance as a function of sample size in (A). (C) Whole-body wsp abundance in tor-RNAi conditions. (D) Power analysis, testing the likelihood of significance in (C). Significance was set at * p < 0.05.
Adult-induced RNAi disruptions provide further context for host effects on wsp
An intrinsic limitation of certain genetic disruption approaches, like constitutive RNAi induction, is that cumulative disruption effects could occur. To confirm ongoing Wnt and mTOR pathway effects on whole-body wsp abundance, “Gene-switch” GAL4 driver flies were used to induce GAL4 activity in adult flies. The Gene-switch version of GAL4 carries an inhibitory domain that blocks GAL4 function, until a de-repressor compound, mifepristone, is added (Roman et al., 2001). Because trial experiments on mifepristone identified low-power but potentially significant effects on wsp (Supplementary Figure S2), all GS-GAL4 experiments were carried out with multiple controls. Flies carrying GS-GAL4::UAS-shRNA genotypes were always compared to non-expressing siblings, with half of the flies DMSO-treated, and the other half exposed to DMSO-solubilized mifepristone.
In tests of arm and tor RNAi knockdowns, no significant wsp abundance differences were observed between the DMSO-treated flies and mifepristone-treated flies that were incapable of RNAi expression (Figures 5A,B). However, mifepristone-fed flies that were capable of shRNA expression did show significant differences in their wsp counts. In the case of GS-GAL4::arm-shRNA, the mifepristone-treated flies exhibited reduced wsp counts, down to 45–71% of all other conditions tested in parallel (p-value range: <0.001–0.033, n = 9) (Figure 5A). By contrast, mifepristone-treated GS-GAL4::tor-shRNA flies carried 81–184% more wsp than all other conditions run in parallel (p-value range: <0.001–0.031, n = 9) (Figure 5B). These results confirm ongoing Wolbachia sensitivity to Wnt and mTOR disruption in adult hosts. Unlike the da-GAL4 data, the GS-GAL4 results notably show that Wnt and mTOR exert opposing effects on Wolbachia titer. This highlights a functional difference between constitutive and adult-specific disruptions of the Wnt pathway with respect to regulation of Wolbachia titer in adult insects.
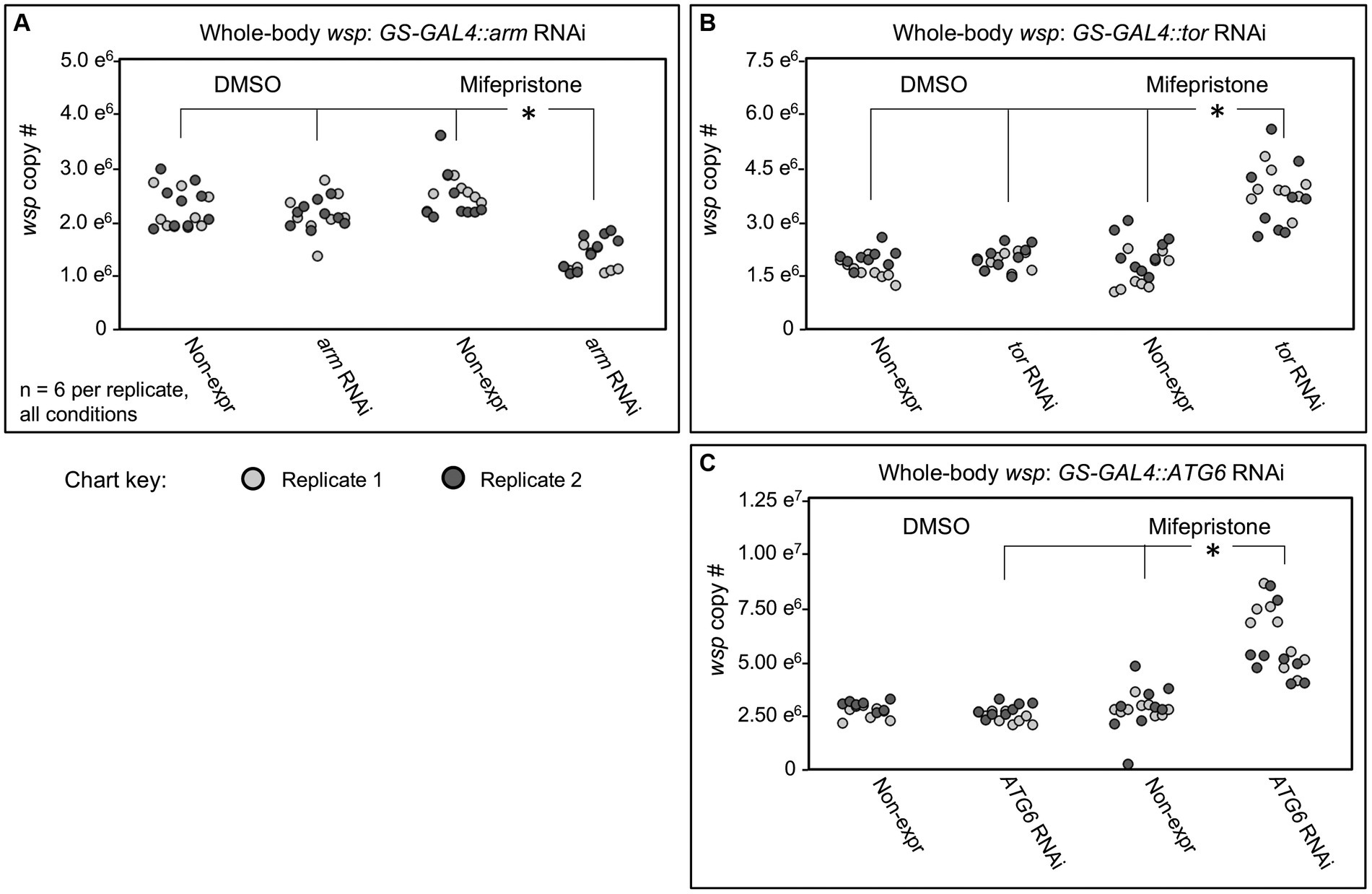
Figure 5. Whole body wsp abundance in control vs. GS-GAL4::UAS-RNAi knockdown flies. Flies capable of dsRNA expression were compared against non- expressing siblings, in the presence of DMSO or Mifepristone dissolved into DMSO. Genetic disruptions tested: (A) arm RNAi, (B) tor RNAi, (C) ATG6 RNAi. Data out of range for (C): 3 outliers for the Non-expressing DMSO condition at 2.01×107, 2.54×107, 2.064×107, and 1 outlier for the ATG6 RNAi DMSO condition at 2.28×107. Significance was set at * p < 0.05, and is displayed only for conditions where all replicates met this standard.
One way to reconcile effects of arm and tor disruptions on wsp abundance is to consider the possibility that both may affect a consensus target relevant to Wolbachia. Literature indicates that Wnt signaling can suppress autophagy onset via multiple routes (Pérez-Plasencia et al., 2020), including through down-regulation of Beclin-1, also known as ATG6 (Tao et al., 2017). mTORC1 is also known to inhibit ATG6 by suppressing the ATG6 activator, ULK1 (Hill et al., 2019). To test the effect of ATG6 on whole-body Wolbachia titer, the GS-GAL4::ATG6-shRNA flies were generated. The mifepristone-fed, RNAi-expressing condition exhibit 67–173% higher wsp levels than non-expressing mifepristone-fed siblings and DMSO-fed flies of equivalent genotype (p-value range: <0.001–0.045, n = 9) (Figure 5C). These data suggest that ATG6 normally suppresses whole-body wsp abundance, consistent with autophagy as a general suppressor of Wolbachia titer (Voronin et al., 2012; Strunov et al., 2022). Implications for Wnt and mTORC1 pathway interaction with autophagy are discussed below.
Discussion
This study explored the basis for endosymbiosis by inipvestigating the effect of candidate host processes on Wolbachia titer in two different host-strain combinations. To identify consensus cellular effects on whole-body wsp counts, candidate compounds were screened against DB wMel and Dsim wRi systems. This was followed by constitutive as well as inducible genetic disruptions in DB wMel to further verify effects of the drug-implicated pathways on wsp abundance. The amenability of Drosophila to mechanistic cross-validation in this rigorous capacity has opened a series of questions and opportunities, while also informing on the mechanisms of endosymbiont titer control.
After identifying infection-related pathways of potential interest from the literature, a candidate drug screen was performed to test these pathways. Wolbachia titer responses were assessed via absolute quantification of the wsp gene from whole insect samples. This is a targeted approach, relative to prior comprehensive screens of Wolbachia-host interactions in Drosophila tissue culture cells (White et al., 2017; Grobler et al., 2018). The organism-centered approach provides a unique advantage in detecting system-level, endogenous responses, with measurements inclusive of bacterial relocation events within the organism (Landmann et al., 2012; White et al., 2017). Detection of an organismal titer change is also a stringent requirement because Wolbachia infection is carried in a variety of tissues (Heddi et al., 1999; Bian et al., 2013; Ali et al., 2018; Schneider et al., 2018; Kaur et al., 2020), and it cannot be assumed that host manipulation will elicit uniform titer change across all tissues. Treatments yielding mild or contradictory outcomes at the tissue level will not be detected as hits by this method. Starting with a chemical screen provides an additional advantage in helping to narrow down the range of pathways for follow-up genetic testing, which as shown here, requires calibration at the level of tool selection, sample size, experiment duration, and controls required. Absolute counts by real-time qPCR are indispensible for the success of such analyses, to mitigate artifacts attributable to variable host ploidy, which may not always be foreseeable across tissues, systems, organism age, and nutritional conditions (Christensen et al., 2019; Ren et al., 2020).
This study emphasized pursuit of host pathways that are associated with commonly studied bacterial infections, as a springboard to delve deeper into processes which may also be involved in commensal Wolbachia infection. The cellular microbiology literature yielded a range of interesting host-side effects on bacterial genera such as Coxiella, Legionella, Brucella, Rickettsia, Ehrlichia, Chlamydia, and Ehrlichia (Rikihisa et al., 1995; Kessler et al., 2012; Czyż et al., 2014; Luo et al., 2016), for which host Calcium and Wnt signaling promotes bacterial proliferation. Another example is the Epidermal Growth Factor Receptor (EGFR) signaling pathway, which has been implicated in promoting host cell invasion by pathogens like Salmonella and Neisseria (Galán et al., 1992; Slanina et al., 2014). Other processes, such as the mTOR/autophagy pathway, have been shown to exert differential density effects depending on the bacterial strain. For example, mTOR signaling disruptions reduce intracellular loads for Ehrlichia (Luo et al., 2017), Chlamydia, Listeria (Derré et al., 2007), and Salmonella (Birmingham et al., 2006), but increase titers for Anaplasma and Rickettsia (Niu et al., 2008; Bechelli et al., 2018). Recurrent titer-related effects for host cellular processes on unrelated bacterial taxa invoke the possibility of generalized infection roles for host cellular pathways, and thus of potential interest in endosymbiosis as well (Supplementary Table S4) (Porter and Sullivan, 2023).
The candidate chemical screen yielded 11 compounds that consistently altered whole-body wsp levels in DB wMel, 6 of which repeated the effect in Dsim wRi. The “hit” compounds that were identified reflect roles for the Imd pathway, Calcium signaling, the Ras/mTOR pathway, and the Wnt pathway (Table 2), prioritizing these pathways for genetic follow-up experiments. The basis for a reduction in compound “hits” between DB wMel to Dsim wRi is inconclusive at this time. Some of the compounds may also have differential bioavailability, bioactivity and perdurance across systems, among other possibilities.
A notable aspect of the consensus, non-lethal hit compounds in both systems is that they all significantly increased wsp counts. Elevated Wolbachia titer has previously been observed in response to ribosome disruption, for example (Grobler et al., 2018). Perhaps Wolbachia suppression by a suite of host cellular processes is relieved by disruption to host functional networks, allowing a favorable shift in Wolbachia life cycle dynamics. It is reasonable to consider commensalism as an artifact of endosymbiont genome reduction, with virulence factors eliminated over time (Metcalf et al., 2014; Sachs et al., 2014; Latorre and Manzano-Marín, 2017). The findings of this study suggest that for Wolbachia, commensalism is further supported by ongoing containment of endosymbiont population by the host, consistent with a view that commensalism is not necessarily free of conflict (Keeling and McCutcheon, 2017).
This study used complementary genetic approaches to cross-validate pathways implicated as Wolbachia-related by the chemical screen. Genetic corroboration of the host pathway functions is not always possible, as internal redundancies may render certain knockdowns ineffective. Developmental tolerance limits may also preclude analysis of the strongest knockdown effects, as when Actin5C-GAL4 was used in this study. Regardless of this, RNAi disruptions to host arm and tor genes affected whole-body wsp levels consistently and significantly. Dual effects of Wnt and mTOR disruptions on Wolbachia titer are consistent with literature connecting these signaling inputs to regulation of autophagy (Figure 6). There is a robust literature on antimicrobial functions of autophagy (Moy and Cherry, 2013), with reports of insects succumbing to pathogen infection when genes like ATG6 have been disrupted (Edosa et al., 2020).
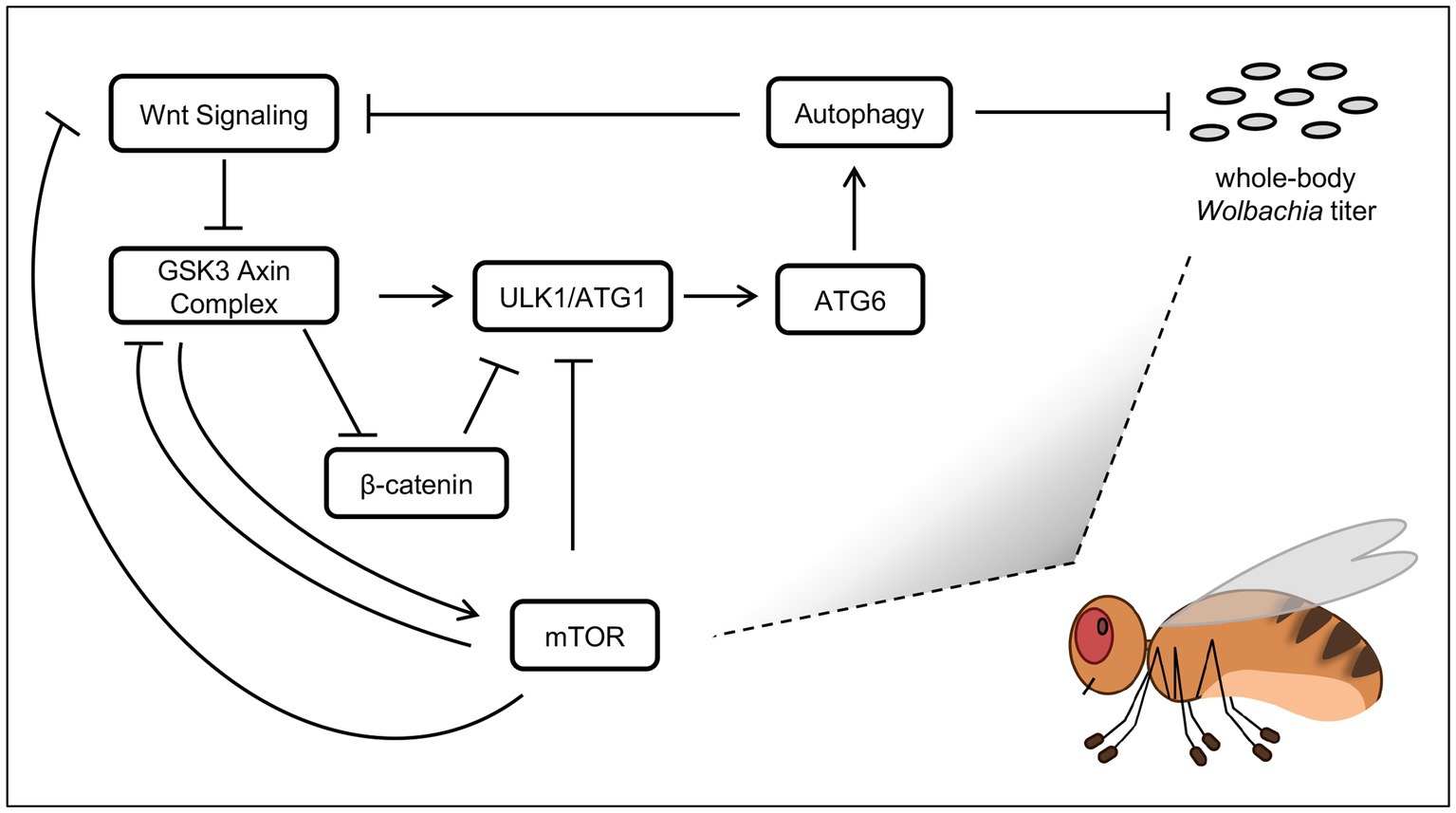
Figure 6. Models of host effects on whole-body Wolbachia titer. Pathway functions are displayed as per field literature. Boxes: host functions. Grey ovals: Wolbachia bacteria.
Autophagy has been discussed previously as a regulator of endosymbiont titer, with functional effects dependent on the system used (Supplementary Table S6). Of the factors analyzed in this study, the one most immediate to the process of autophagy is ATG6 (Su et al., 2020). Thus, ATG6 suppression by RNAi would be expected to down-regulate autophagy. RNAi knockdowns of ATG6 yielded Wolbachia titer elevation, suggesting a model in which autophagy normally suppresses Wolbachia titer (Figure 6). This outcome is consistent with past results from others reporting that somatic autophagy antagonizes somatic Wolbachia titer (Voronin et al., 2012; Strunov et al., 2022).
The literature has also shown that the Wnt pathway affects autophagy regulation. In the absence of Wnt ligand, GSK-3 promotes autophagy activity by activating ULK1 (Ryu et al., 2021) as well as by suppressing Arm (Aros et al., 2021), which is a negative regulator of autophagy (Petherick et al., 2013; Feng et al., 2023) (Figure 6). Notably, GSK-3 works in a complex together with AXIN to suppress Arm (Ikeda et al., 1998), therefore AXIN disruption by IWR-1 should disrupt that function, with an indirect consequence of down-regulating autophagy, and allowing Wolbachia titer to increase. Tests of IWR-1 in this study yielded consistent Wolbachia titer elevation in both D. melanogaster and D. simulans. This finding is in accord with autophagy as a suppressor of whole-body Wolbachia abundance.
There is at least some complexity in Wnt pathway effects on whole-body Wolbachia titer. Because the GSK-3/AXIN complex antagonizes Arm in the Wnt pathway (Aros et al., 2021), Arm disruption would be expected to show the opposite results from an AXIN disruption. Meaning, since IWR-1 caused a titer increase, genetic disruptions in arm would be expected to prompt a titer decrease. From a mechanistic standpoint, this scenario would also make sense. It has been shown that Arm can suppress autophagy (Petherick et al., 2013; Feng et al., 2023) (Figure 6), so autophagy functions should increase when Arm RNAi is expressed, resulting in Wolbachia titer reduction. In this study, Wolbachia titer decreased in the induced GS-GAL4:: Arm-shRNA conditions. However, the constitutive da-GAL4:: Arm-shRNA treatment yielded a titer increase, not decrease. This disparity may be due to constitutive da-GAL4 disruption eliciting a cumulative, lifelong effect, with the possibility of involvement by other compensatory pathways. By contrast, the induction of GS-GAL4 in adults is uncoupled from earlier developmental events and may provide a more focused view of host pathway effects on Wolbachia titer in adult insects.
The mTOR pathway is well-known for regulation of autophagy. The mTORC1 complex has been shown to down-regulate autophagy through suppression of ULK1 (Yamamoto et al., 2023), as well as through suppression of GSK3 (Papadopoli et al., 2021) which would otherwise promote ULK1 function (Ryu et al., 2021) (Figure 6). In an alternate scenario, mTORC1 has the opposite regulatory effect, by inhibiting Wnt signaling at the level of the receptor, shutting down its function (Zeng et al., 2018). In that case, GSK3 would remain free to perform the complementary functions of activating ULK1 (Ryu et al., 2021) while also preventing Arm from suppressing ULK1 function (Petherick et al., 2013; Feng et al., 2023) (Figure 6). With this range of possibility, mTOR effects on autophagy function could go either way.
All mTORC1 disruptions in this study yielded a whole-body Wolbachia titer increase, regardless of the experimental system or type of manipulation tool used. This robust set of results is also compatible with the possibility of autophagy suppression of Wolbachia. If true, the implication would be that under normal conditions, mTORC1 function emphasizes suppression of Wnt receptor activity relative to other autophagy-related targets, to promoting autophagy and indirectly, Wolbachia titer suppression. This model comes with a grain of salt, as signaling processes can be complex. The reported finding that autophagy can down-regulate Wnt signaling (Pérez-Plasencia et al., 2020) is just one example of the nuance that may be involved (Figure 6). Future studies will be needed to elucidate how signaling and autophagy initiation affect Wolbachia and other microbial endosymbionts.
Data availability statement
The original contributions presented in the study are included in the article/Supplementary material, further inquiries can be directed to the corresponding author.
Ethics statement
The animal study was approved by FIU Institutional Biosafety Committee (IBC). The study was conducted in accordance with the local legislation and institutional requirements.
Author contributions
ZS: Conceptualization, Data curation, Methodology, Supervision, Validation, Visualization, Writing – original draft, Writing – review & editing, Formal analysis, Investigation. HS: Conceptualization, Data curation, Formal analysis, Investigation, Methodology, Supervision, Validation, Visualization, Writing – review & editing. RB: Conceptualization, Data curation, Formal analysis, Investigation, Methodology, Validation, Visualization, Writing – review & editing. LO: Conceptualization, Data curation, Formal analysis, Investigation, Methodology, Validation, Visualization, Writing – review & editing. LS: Conceptualization, Data curation, Methodology, Validation, Visualization, Writing – review & editing, Funding acquisition, Project administration, Resources, Software, Supervision, Writing – original draft.
Funding
The author(s) declare financial support was received for the research, authorship, and/or publication of this article. Support for this project was provided by startup funds from Florida International University (Academic Affairs) and a research grant awarded by the National Science Foundation (IOS-1656811).
Acknowledgments
Funding for this project came from FIU Academic Affairs and the NSF Division of Integrated Organismal Systems (#1656811). We thank Steen Christensen, Moises Camacho, Anthony Bellantuono, AJM Zehadee Momtaz, Erasmo Perera and FIU Biological Sciences for helpful discussions and logistical support. We also thank the University Graduate School, the College of Arts, Sciences and Education, the Biological Sciences Graduate program, the Transdisciplinary Biomolecular and Biomedical Sciences program, the Honors College and the Biology Honors Program at FIU for supporting our students.
Conflict of interest
The authors declare that the research was conducted in the absence of any commercial or financial relationships that could be construed as a potential conflict of interest.
Publisher’s note
All claims expressed in this article are solely those of the authors and do not necessarily represent those of their affiliated organizations, or those of the publisher, the editors and the reviewers. Any product that may be evaluated in this article, or claim that may be made by its manufacturer, is not guaranteed or endorsed by the publisher.
Supplementary material
The Supplementary material for this article can be found online at: https://www.frontiersin.org/articles/10.3389/fmicb.2024.1364009/full#supplementary-material
References
Ali, H., Muhammad, A., Islam, S. U., Islam, W., and Hou, Y. (2018). A novel bacterial symbiont association in the hispid beetle, Octodonta nipae (Coleoptera: Chrysomelidae), their dynamics and phylogeny. Microb. Pathog. 118, 378–386. doi: 10.1016/j.micpath.2018.03.046
Ali, H., Muhammad, A., Sanda, N. B., Huang, Y., and Hou, Y. (2019). Pyrosequencing uncovers a shift in bacterial communities across life stages of Octodonta nipae (Coleoptera: Chrysomelidae). Front. Microbiol. 10:466. doi: 10.3389/fmicb.2019.00466
Aros, C. J., Pantoja, C. J., and Gomperts, B. N. (2021). Wnt signaling in lung development, regeneration, and disease progression. Commun. Biol. 4:601. doi: 10.1038/s42003-021-02118-w
Bechelli, J., Vergara, L., Smalley, C., Buzhdygan, T. P., Bender, S., Zhang, W., et al. (2018). Atg5 supports Rickettsia australis infection in macrophages in vitro and in vivo. Infect. Immun. 87:e00651-18. doi: 10.1128/IAI.00651-18
Bian, G., Joshi, D., Dong, Y., Lu, P., Zhou, G., Pan, X., et al. (2013). Wolbachia invades Anopheles stephensi populations and induces refractoriness to plasmodium infection. Science (New York, N.Y.) 340, 748–751. doi: 10.1126/science.1236192
Birmingham, C. L., Smith, A. C., Bakowski, M. A., Yoshimori, T., and Brumell, J. H. (2006). Autophagy controls Salmonella infection in response to damage to the Salmonella-containing vacuole. J. Biol. Chem. 281, 11374–11383. doi: 10.1074/jbc.M509157200
Brand, A. H., and Perrimon, N. (1993). Targeted gene expression as a means of altering cell fates and generating dominant phenotypes. Development 118, 401–415. doi: 10.1242/dev.118.2.401
Christensen, S., Camacho, M., Sharmin, Z., Momtaz, A. J. M. Z., Perez, L., Navarro, G., et al. (2019). Quantitative methods for assessing local and bodywide contributions to Wolbachia titer in maternal germline cells of Drosophila. BMC Microbiol. 19:206. doi: 10.1186/s12866-019-1579-3
Christensen, S., Pérez Dulzaides, R., Hedrick, V. E., Momtaz, A. J. M. Z., Nakayasu, E. S., Paul, L. N., et al. (2016). Wolbachia endosymbionts modify Drosophila ovary protein levels in a context-dependent manner. Appl. Environ. Microbiol. 82, 5354–5363. doi: 10.1128/AEM.01255-16
Cogni, R., Ding, S. D., Pimentel, A. C., Day, J. P., and Jiggins, F. M. (2021). Wolbachia reduces virus infection in a natural population of Drosophila. Commun. Biol. 4:1327. doi: 10.1038/s42003-021-02838-z
Czyż, D. M., Potluri, L.-P., Jain-Gupta, N., Riley, S. P., Martinez, J. J., Steck, T. L., et al. (2014). Host-Directed Antimicrobial Drugs with Broad- 51 Spectrum Efficacy against Intracellular Bacterial Pathogens. MBio, 5. doi: 10.1128/mBio.01534-14
Derré, I., Pypaert, M., Dautry-Varsat, A., and Agaisse, H. (2007). RNAi screen in Drosophila cells reveals the involvement of the tom complex in Chlamydia infection. PLoS Pathog. 3, 1446–1458. doi: 10.1371/journal.ppat.0030155
Duffy, J. B. (2002). GAL4 system in Drosophila: a fly geneticist's Swiss army knife. Genesis 34, 1–15. doi: 10.1002/gene.10150
Edosa, T. T., Jo, Y. H., Keshavarz, M., Park, K. B., Cho, J. H., Bae, Y. M., et al. (2020). TmAtg6 plays an important role in anti-microbial defense against Listeria monocytogenes in the mealworm, Tenebrio molitor. Int. J. Mol. Sci. 21:1232. doi: 10.3390/ijms21041232
Feng, X., Sun, D., Li, Y., Zhang, J., Liu, S., Zhang, D., et al. (2023). Local membrane source gathering by p62 body drives autophagosome formation. Nature Communications 14, 7338. doi: 10.1038/s41467-023-42829-8
Foley, S. L., Johnson, T. J., Ricke, S. C., Nayak, R., and Danzeisen, J. (2013). Salmonella pathogenicity and host adaptation in chicken-associated Serovars. Microbiol. Mol. Biol. Rev. 77, 582–607. doi: 10.1128/MMBR.00015-13
Galán, J. E., Pace, J., and Hayman, M. J. (1992). Involvement of the epidermal growth factor receptor in the invasion of cultured mammalian cells by Salmonella typhimurium. Nature 357, 588–589. doi: 10.1038/357588a0
Grobler, Y., Yun, C. Y., Kahler, D. J., Bergman, C. M., Lee, H., Oliver, B., et al. (2018). Whole genome screen reveals a novel relationship between Wolbachia levels and Drosophila host translation. PLoS Pathog. 14:e1007445. doi: 10.1371/journal.ppat.1007445
Haselton, A., Sharmin, E., Schrader, J., Sah, M., Poon, P., and Fridell, Y.-W. C. (2010). Partial ablation of adult Drosophila insulin-producing neurons modulates glucose homeostasis and extends life span without insulin resistance. Cell Cycle 9, 3063–3071. doi: 10.4161/cc.9.15.12458
Heddi, A., Grenier, A. M., Khatchadourian, C., Charles, H., and Nardon, P. (1999). Four intracellular genomes direct weevil biology: nuclear, mitochondrial, principal endosymbiont, and Wolbachia. Proc. Natl. Acad. Sci. USA 96, 6814–6819. doi: 10.1073/pnas.96.12.6814
Hedges, L. M., Brownlie, J. C., O’Neill, S. L., and Johnson, K. N. (2008). Wolbachia and virus protection in insects. Science (New York, N.Y.) 322:702. doi: 10.1126/science.1162418
Hill, S. M., Wrobel, L., and Rubinsztein, D. C. (2019). Post-translational modifications of Beclin 1 provide multiple strategies for autophagy regulation. Cell Death Differ. 26, 617–629. doi: 10.1038/s41418-018-0254-9
Hoffmann, A. A., Hercus, M., and Dagher, H. (1998). Population dynamics of the Wolbachia infection causing cytoplasmic incompatibility in Drosophila melanogaster. Genetics 148, 221–231. doi: 10.1093/genetics/148.1.221
Hoffmann, A. A., Turelli, M., and Harshman, L. G. (1990). Factors affecting the distribution of cytoplasmic incompatibility in Drosophila simulans. Genetics 126, 933–948. doi: 10.1093/genetics/126.4.933
Ikeda, S., Kishida, S., Yamamoto, H., Murai, H., Koyama, S., and Kikuchi, A. (1998). Axin, a negative regulator of the Wnt signaling pathway, forms a complex with GSK-3beta and beta-catenin and promotes GSK-3beta-dependent phosphorylation of beta-catenin. EMBO J. 17, 1371–1384. doi: 10.1093/emboj/17.5.1371
Kaur, R., Martinez, J., Rota-Stabelli, O., Jiggins, F. M., and Miller, W. J. (2020). Age, tissue, genotype and virus infection regulate Wolbachia levels in Drosophila. Mol. Ecol. 29, 2063–2079. doi: 10.1111/mec.15462
Keeling, P. J., and McCutcheon, J. P. (2017). Endosymbiosis: the feeling is not mutual. J. Theor. Biol. 434, 75–79. doi: 10.1016/j.jtbi.2017.06.008
Kessler, M., Zielecki, J., Thieck, O., Mollenkopf, H.-J., Fotopoulou, C., and Meyer, T. F. (2012). Chlamydia trachomatis disturbs epithelial tissue homeostasis in fallopian tubes via paracrine Wnt signaling. The American Journal of Pathology, 180, 186–198. doi: 10.1016/j.ajpath.2011.09.015
Kim, B. H., Yin, C.-H., Guo, Q., Bach, E. A., Lee, H., Sandoval, C., et al. (2008). A small molecule compound identified through a cell-based screening inhibits JAK/STAT pathway signaling in human cancer cells. Molecular Cancer Therapeutics, 7, 2672–2680. doi: 10.1158/1535-7163.MCT-08-0309
Landmann, F., Bain, O., Martin, C., Uni, S., Taylor, M. J., and Sullivan, W. (2012). Both asymmetric mitotic segregation and cell-to-cell invasion are required for stable germline transmission of Wolbachia in filarial nematodes. Biol. Open 1, 536–547. doi: 10.1242/bio.2012737
Landmann, F., Voronin, D., Sullivan, W., and Taylor, M. J. (2011). Anti-filarial activity of antibiotic therapy is due to extensive apoptosis after Wolbachia depletion from filarial nematodes. PLoS Pathog. 7:e1002351. doi: 10.1371/journal.ppat.1002351
Latorre, A., and Manzano-Marín, A. (2017). Dissecting genome reduction and trait loss in insect endosymbionts. Ann. N. Y. Acad. Sci. 1389, 52–75. doi: 10.1111/nyas.13222
Lewis, D. (1985). “Symbiosis and mutualism: crisp concepts and soggy semantics” in The biology of mutualism: ecology and evolution. ed. D. H. Boucher (London: Oxford University Press).
López-Madrigal, S., and Duarte, E. H. (2019). Titer regulation in arthropod-Wolbachia symbioses. FEMS Microbiol. Lett. 366:fnz232. doi: 10.1093/femsle/fnz232
Luo, T., Dunphy, P. S., Lina, T. T., and McBride, J. W. (2016). Ehrlichia chaffeensis exploits canonical and noncanonical host Wnt signaling pathways to stimulate phagocytosis and Promote Intracellular Survival. Infection and Immunity, 84, 686–700. doi: 10.1128/IAI.01289-15
Luo, T., Dunphy, P. S., and McBride, J. W. (2017). Ehrlichia chaffeensis tandem repeat effector targets differentially influence infection. Front. Cell. Infect. Microbiol. 7:178. doi: 10.3389/fcimb.2017.00178
Markstein, M., Dettorre, S., Cho, J., Neumuller, R. A., Craig-Muller, S., and Perrimon, N. (2014). Systematic screen of chemotherapeutics in Drosophila stem cell tumors. Proceedings of the National Academy of Sciences, 111, 4530–4535. doi: 10.1073/pnas.1401160111
McGarry, H. F., Egerton, G. L., and Taylor, M. J. (2004). Population dynamics of Wolbachia bacterial endosymbionts in Brugia malayi. Mol. Biochem. Parasitol. 135, 57–67. doi: 10.1016/j.molbiopara.2004.01.006
Metcalf, J. A., Jo, M., Bordenstein, S. R., Jaenike, J., and Bordenstein, S. R. (2014). Recent genome reduction of Wolbachia in Drosophila recens targets phage WO and narrows candidates for reproductive parasitism. PeerJ 2:e529. doi: 10.7717/peerj.529
Moy, R. H., and Cherry, S. (2013). Antimicrobial autophagy: a conserved innate immune response in Drosophila. J. Innate Immun. 5, 444–455. doi: 10.1159/000350326
Ni, J.-Q., Zhou, R., Czech, B., Liu, L.-P., Holderbaum, L., Yang-Zhou, D., et al. (2011). A genome-scale shRNA resource for transgenic RNAi in Drosophila. Nat. Methods 8, 405–407. doi: 10.1038/nmeth.1592
Niu, H., Yamaguchi, M., and Rikihisa, Y. (2008). Subversion of cellular autophagy by Anaplasma phagocytophilum. Cell. Microbiol. 10, 593–605. doi: 10.1111/j.1462-5822.2007.01068.x
Pannebakker, B. A., Loppin, B., Elemans, C. P. H., Humblot, L., and Vavre, F. (2007). Parasitic inhibition of cell death facilitates symbiosis. Proc. Natl. Acad. Sci. USA 104, 213–215. doi: 10.1073/pnas.0607845104
Papadopoli, D., Pollak, M., and Topisirovic, I. (2021). The role of GSK3 in metabolic pathway perturbations in cancer. Biochim. Biophys. Acta, Mol. Cell Res. 1868:119059. doi: 10.1016/j.bbamcr.2021.119059
Pérez-Plasencia, C., López-Urrutia, E., García-Castillo, V., Trujano-Camacho, S., López-Camarillo, C., and Campos-Parra, A. D. (2020). Interplay between autophagy and Wnt/β-catenin signaling in Cancer: therapeutic potential through drug repositioning. Front. Oncol. 10:1037. doi: 10.3389/fonc.2020.01037
Perkins, L. A., Holderbaum, L., Tao, R., Hu, Y., Sopko, R., McCall, K., et al. (2015). The transgenic RNAi project at Harvard Medical School: resources and validation. Genetics 201, 843–852. doi: 10.1534/genetics.115.180208
Perrimon, N., Ni, J.-Q., and Perkins, L. (2010). In vivo RNAi: today and tomorrow. Cold Spring Harb. Perspect. Biol. 2:a003640. doi: 10.1101/cshperspect.a003640
Petherick, K. J., Williams, A. C., Lane, J. D., Ordóñez-Morán, P., Huelsken, J., Collard, T. J., et al. (2013). Autolysosomal β-catenin degradation regulates Wnt-autophagy-p62 crosstalk. EMBO J. 32, 1903–1916. doi: 10.1038/emboj.2013.123
Porter, J., and Sullivan, W. (2023). The cellular lives of Wolbachia. Nat. Rev. Microbiol. 21, 750–766. doi: 10.1038/s41579-023-00918-x
Ren, D., Song, J., Ni, M., Kang, L., and Guo, W. (2020). Regulatory mechanisms of cell polyploidy in insects. Front. Cell Dev. Biol. 8:361. doi: 10.3389/fcell.2020.00361
Rikihisa, Y., Zhang, Y., and Park, J. (1995). Role of Ca2 and calmodulin in ehrlichial infection in macrophages. Infection and Immunity, 63, 2310–2316.
Roman, G., Endo, K., Zong, L., and Davis, R. L. (2001). P{switch}, a system for spatial and temporal control of gene expression in Drosophila melanogaster. Proc. Natl. Acad. Sci. 98, 12602–12607. doi: 10.1073/pnas.221303998
Ryu, H. Y., Kim, L. E., Jeong, H., Yeo, B. K., Lee, J. W., Nam, H., et al. (2021). GSK3B induces autophagy by phosphorylating ULK1. Exp. Mol. Med. 53, 369–383. doi: 10.1038/s12276-021-00570-6
Sachs, J. L., Skophammer, R. G., Bansal, N., and Stajich, J. E. (2014). Evolutionary origins and diversification of proteobacterial mutualists. Proc. R. Soc. B Biol. Sci. 281:20132146. doi: 10.1098/rspb.2013.2146
Sazama, E. J., Ouellette, S. P., and Wesner, J. S. (2019). Bacterial endosymbionts are common among, but not necessarily within, Insect Species. Environ. Entomol. 48, 127–133. doi: 10.1093/ee/nvy188
Serbus, L. R., Landmann, F., Bray, W. M., White, P. M., Ruybal, J., Lokey, R. S., et al. (2012). A cell-based screen reveals that the albendazole metabolite, albendazole sulfone, targets Wolbachia. PLoS Pathogens, 8, e1002922. doi: 10.1371/journal.ppat.1002922255
Schneider, D. I., Parker, A. G., Abd-Alla, A. M., and Miller, W. J. (2018). High-sensitivity detection of cryptic Wolbachia in the African tsetse fly (Glossina spp.). BMC Microbiol. 18:140. doi: 10.1186/s12866-018-1291-8
Serbus, L. R., and Sullivan, W. (2007). A cellular basis for Wolbachia recruitment to the host germline. PLoS Pathog. 3:e190. doi: 10.1371/journal.ppat.0030190
Serbus, L. R., White, P. M., Silva, J. P., Rabe, A., Teixeira, L., Albertson, R., et al. (2015). The impact of host diet on Wolbachia titer in Drosophila. PLoS Pathog. 11:e1004777. doi: 10.1371/journal.ppat.1004777
Slanina, H., Mündlein, S., Hebling, S., and Schubert-Unkmeir, A. (2014). Role of epidermal growth factor receptor signaling in the interaction of Neisseria meningitidis with endothelial cells. Infect. Immun. 82, 1243–1255. doi: 10.1128/IAI.01346-13
Strunov, A., Schmidt, K., Kapun, M., and Miller, W. J. (2022). Restriction of Wolbachia Bacteria in early embryogenesis of Neotropical Drosophila species via endoplasmic reticulum-mediated autophagy. MBio 13:e0386321. doi: 10.1128/mbio.03863-21
Su, T., Li, X., Yang, M., Shao, Q., Zhao, Y., Ma, C., et al. (2020). Autophagy: An intracellular degradation pathway regulating plant survival and stress response. Frontiers Plant Science, 11, 164. doi: 10.3389/fpls.2020.00164
Tao, H., Chen, F., Liu, H., Hu, Y., Wang, Y., and Li, H. (2017). Wnt/β-catenin signaling pathway activation reverses gemcitabine resistance by attenuating Beclin1-mediated autophagy in the MG63 human osteosarcoma cell line. Mol. Med. Rep. 16, 1701–1706. doi: 10.3892/mmr.2017.6828
Taylor, M. J., Bandi, C., and Hoerauf, A. (2005). Wolbachia bacterial endosymbionts of filarial nematodes. Adv. Parasitol. 60, 245–284. doi: 10.1016/S0065-308X(05)60004-8
Teixeira, L., Ferreira, A., and Ashburner, M. (2008). The bacterial symbiont Wolbachia induces resistance to RNA viral infections in Drosophila melanogaster. PLoS Biol. 6:e2. doi: 10.1371/journal.pbio.1000002
Unckless, R. L., Boelio, L. M., Herren, J. K., and Jaenike, J. (2009). Wolbachia as populations within individual insects: causes and consequences of density variation in natural populations. Proc. Biol. Sci. 276, 2805–2811. doi: 10.1098/rspb.2009.0287
Veneti, Z., Clark, M. E., Karr, T. L., Savakis, C., and Bourtzis, K. (2004). Heads or tails: host-parasite interactions in the Drosophila-Wolbachia system. Appl. Environ. Microbiol. 70, 5366–5372. doi: 10.1128/AEM.70.9.5366-5372.2004
Voronin, D., Cook, D. A. N., Steven, A., and Taylor, M. J. (2012). Autophagy regulates Wolbachia populations across diverse symbiotic associations. Proc. Natl. Acad. Sci. 109, E1638–E1646. doi: 10.1073/pnas.1203519109
Werren, J. H., Baldo, L., and Clark, M. E. (2008). Wolbachia: master manipulators of invertebrate biology. Nat. Rev. Microbiol. 6, 741–751. doi: 10.1038/nrmicro1969
White, P. M., Pietri, J. E., Debec, A., Russell, S., Patel, B., and Sullivan, W. (2017). Mechanisms of horizontal cell-to-cell transfer of Wolbachia spp. in Drosophila melanogaster. Appl. Environ. Microbiol. 83:e03425-16. doi: 10.1128/AEM.03425-16
White, P. M., Serbus, L. R., Debec, A., Codina, A., Bray, W., Guichet, A., et al. (2017). Reliance of Wolbachia on high rates of host proteolysis revealed by a genome-wide RNAi screen of Drosophila cells. Genetics 205, 1473–1488. doi: 10.1534/genetics.116.198903
Wiwatanaratanabutr, I., and Kittayapong, P. (2009). Effects of crowding and temperature on Wolbachia infection density among life cycle stages of Aedes albopictus. J. Invertebr. Pathol. 102, 220–224. doi: 10.1016/j.jip.2009.08.009
Yamamoto, H., Zhang, S., and Mizushima, N. (2023). Autophagy genes in biology and disease. Nat. Rev. Genet. 24, 382–400. doi: 10.1038/s41576-022-00562-w
Keywords: Wolbachia, Drosophila, titer, Wnt, mTOR, autophagy, endosymbiosis, commensalism
Citation: Sharmin Z, Samarah H, Aldaya Bourricaudy R, Ochoa L and Serbus LR (2024) Cross-validation of chemical and genetic disruption approaches to inform host cellular effects on Wolbachia abundance in Drosophila. Front. Microbiol. 15:1364009. doi: 10.3389/fmicb.2024.1364009
Edited by:
Takema Fukatsu, National Institute of Advanced Industrial Science and Technology (AIST), JapanReviewed by:
Xiao-Li Bing, Nanjing Agricultural University, ChinaHabib Ali, Khwaja Fareed University of Engineering and Information Technology (KFUEIT), Pakistan
Copyright © 2024 Sharmin, Samarah, Aldaya Bourricaudy, Ochoa and Serbus. This is an open-access article distributed under the terms of the Creative Commons Attribution License (CC BY). The use, distribution or reproduction in other forums is permitted, provided the original author(s) and the copyright owner(s) are credited and that the original publication in this journal is cited, in accordance with accepted academic practice. No use, distribution or reproduction is permitted which does not comply with these terms.
*Correspondence: Laura Renee Serbus, THNlcmJ1c0BmaXUuZWR1
†These authors have contributed equally to this work