- Federal Institute for Geosciences and Natural Resources (BGR), Hannover, Germany
The extraction of nickel, cobalt, and other metals from laterite ores via bioleaching with sulfur-oxidizing and ferric iron-reducing, autotrophic, acidophilic bacteria (e.g. Acidithiobacillus species) has been demonstrated under anaerobic as well as aerobic conditions in experiments in different laboratories. This study demonstrated the bioleaching of laterites from Brazil with the addition of elemental sulfur in 2-L stirred-tank bioreactors with pure and mixed cultures of Acidithiobacillus and Sulfobacillus species under aerobic conditions. In particular, a potential disturbance of mineral dissolution under aerobic conditions by ferrous iron-oxidizing acidophiles likely introduced as contaminants in an applied bioleaching process was investigated with Leptospirillum ferrooxidans at 30°C and Leptospirillum ferriphilum at 40°C, at maintained pH 1.5 or without maintained pH leading to an increase in acidity (with pH values <1.0) due to the biological production of sulfuric acid. Despite the proportion of ferrous iron to the total amount of extracted iron in the solution being drastically reduced in the presence of Leptospirillum species, there was a negligible effect on the extraction efficiency of nickel and cobalt, which is positive news for laterite bioleaching under aerobic conditions.
1 Introduction
The increasing demand for nickel and cobalt for battery production and other applications has led to increased mining of laterite ores. Pyrometallurgy is applied for saprolitic laterites but does not apply to limonitic laterites; here, hydrometallurgical processing options such as high-pressure acid leaching (HPAL) are chosen, which means high-energy consumption and processing costs (Stanković et al., 2020). Biohydrometallurgical processing of laterite ores promises lower energy consumption, lower acid consumption, and less expensive equipment due to relatively moderate acidic conditions over established laterite ore processing technologies.
Processing of limonitic laterites via bioleaching has been studied for about a decade (Roberto and Schippers, 2022; Santos and Schippers, 2023). Most studies focused on acidophiles that couple the oxidation of added elemental sulfur to sulfuric acid with the reduction of ferric iron in laterite mineral phases leading to a dissolution of particular mineral phases and solubilization of nickel and cobalt. The biogenic ferrous iron acts as a chemically reducing agent here (Stanković et al., 2022). First reports described a Ferredoxin process concept in which Acidithiobacillus (At.) ferrooxidans reduces ferric iron under anaerobic conditions from goethite as a main mineral phase, and the term “reductive bioleaching” was introduced (du Plessis et al., 2011; Hallberg et al., 2011; Johnson and du Plessis, 2015). A later study showed that other Ni- and Co-bearing mineral phases in laterites such as Mn-rich mineral phases and magnesium silicates are dissolved, rather than goethite (Stanković et al., 2022). This and other previous studies (Marrero et al., 2015, 2017) showed that Ni and Co recovery from limonitic laterites via bioleaching under aerobic conditions with the aerobic sulfur-oxidizers At. thiooxidans and At. caldus was at least as efficient as bioleaching under anaerobic conditions with At. ferrooxidans, with the advantage of lower acid consumption and no need for a costly gassing with dinitrogen to maintain anaerobic conditions. Aerobic bioleaching of ferric iron-rich laterites was efficient at low pH < 1. Overall, the reaction mechanism for this aerobic process is still unknown. However, it has been hypothesized that ferric iron is reduced only chemically, by reduced sulfur compounds released into solution by the sulfur-oxidizing Acidithiobacillus (Marrero et al., 2020; Johnson et al., 2021). Supporting this hypothesis, hydrogen sulfide and thiosulfate were recently detected as such sulfur intermediates in ferric iron reduction experiments with acidophiles (Breuker and Schippers, 2024).
A potential problem with aerobic bioleaching of limonitic laterites is that acidophilic ferrous iron-oxidizers such as Leptospirillum (L.) ferrooxidans would grow as contaminants in industrial bioleaching operations, which cannot run under sterile conditions (Smith and Johnson, 2018). In this case, ferrous iron would be oxidized to ferric iron and the ferrous iron concentration in solution might be too low for an efficient reductive dissolution of laterite mineral phases with the reductant ferrous iron (Moro et al., 2023). To address this concern, this study presents a couple of aerobic, stirred-tank bioleaching experiments in which iron-oxidizing acidophiles (Leptospirillum) were added to sulfur-oxidizing acidophiles (Acidithiobacillus) in co-culture, and the bioleaching efficiency was evaluated.
2 Materials and methods
2.1 Laterite sample
A laterite ore sample was obtained from a stockpile of the Anglo-American-owned Barro Alto mine in the state of Goiás, Brazil. The physical properties, geochemistry, and mineralogy of the sample BaSt are comprehensively described elsewhere (Stanković et al., 2022). Briefly, the sample was ocher-colored, fine-grained clayey-silty material, completely decomposed and disaggregated. It could be categorized as a mixed limonitic-saprolitic laterite with different nickel- and cobalt-bearing mineral phases. The chemical composition included approximately 42% Fe2O3, 26% SiO2, 9.4% MgO, 4.8% Al2O3, 0.8% MnO, 15.8 g/kg Cr, 13.6 g/kg Ni, and 1.3 g/kg Co.
2.2 Laterite bioleaching experiments
Bioleaching was carried out on a laboratory scale in 2-L stirred-tank bioreactors, containing 1.5 L of a basalt salts medium with trace elements (Ňancucheo et al., 2016) with an initial pH of 1.5, 1% (w/v) elemental sulfur, and 36 mM ferrous iron. Bioreactors were constantly stirred and supplied with compressed air to ensure oxygen and CO2 supply. Pre-grown type strain pure cultures of sulfur-oxidizing At. thiooxidans DSM 14887T and At. caldus DSM 8584T were used to evaluate the bioleaching efficiency in the absence of iron-oxidizers. To evaluate bioleaching efficiency in the presence of iron-oxidizers, type strains of L. ferrooxidans DSM 2705T and L. ferriphilum DSM 14647T were mixed with either At. thiooxidans or At. caldus, respectively (1:1, mixed cultures). Temperature was kept constant at 30°C for At. thiooxidans ± L. ferrooxidans and at 40°C for At. caldus ± L. ferriphilum. In addition, bioleaching efficiency was tested with artificial consortia consisting of different sulfur- and iron-oxidizers at 30°C and 40°C (Table 1) to get closer to non-sterile industrial conditions, mimicking possible contamination with multiple microorganisms. All bioreactors were inoculated with 10% (v/v) pre-grown pure or mixed cultures and run for 4 days for further growth. Afterward, 10% (w/v) laterite was added to start bioleaching. During 15 days of bioleaching, the pH was adjusted with 1 M H2SO4 or 1 M NaOH to be maintained at 1.5, or there was no pH maintenance. Samples for chemical and microbiological analyses were regularly taken. All experiments were performed in two replicate bioreactors if not stated otherwise.
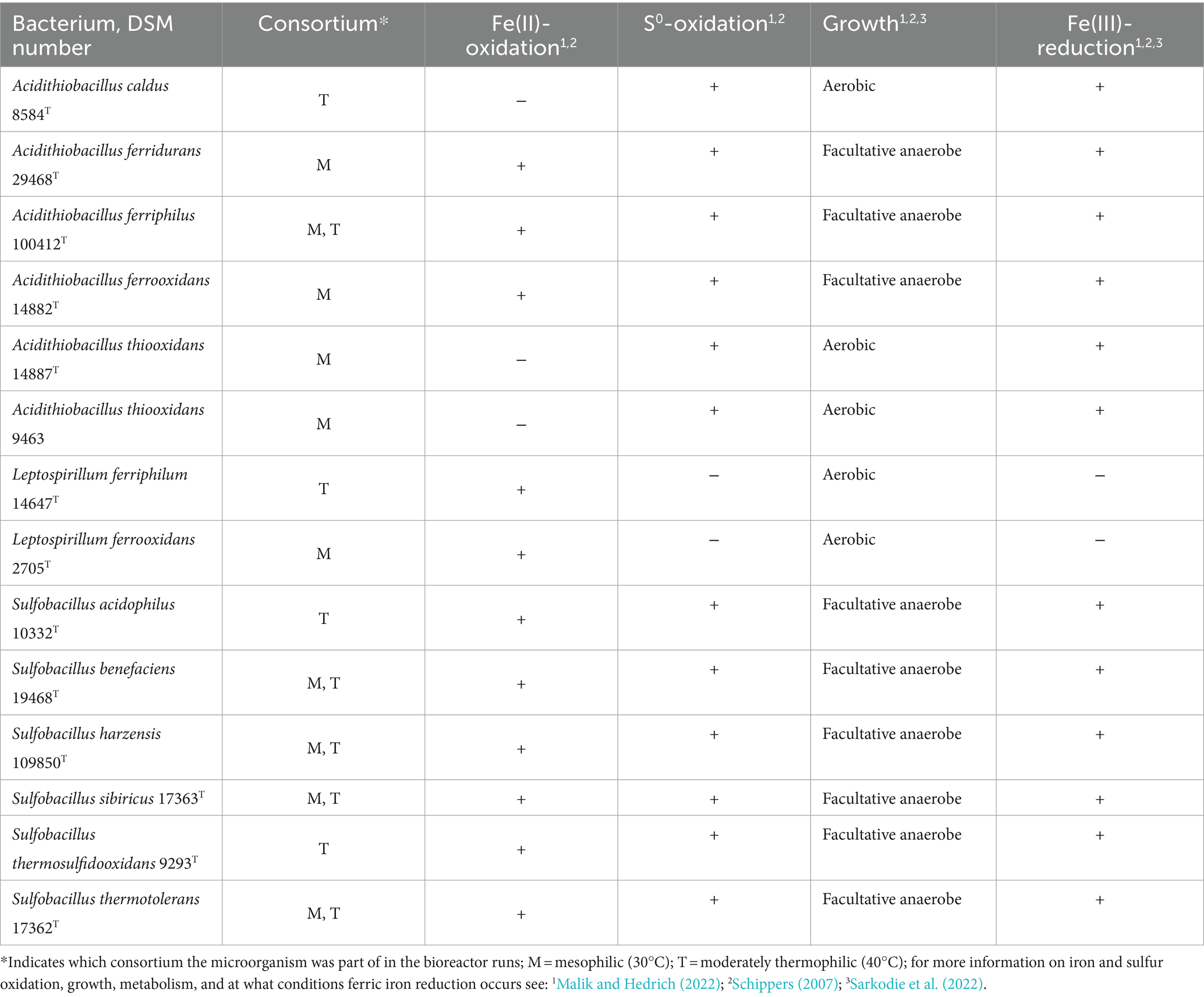
Table 1. Mesophilic and moderately thermophilic, acidophilic bacteria used for bioleaching experiments in the two consortia at 30°C and 40°C, respectively.
2.3 Analytical methods and statistics
Liquid samples were analyzed for dissolved metals via inductively coupled plasma optical emission spectroscopy (ICP-OES). Ferrous iron and total iron concentrations were measured by colorimetric assays (Stookey, 1970). The pH and redox potentials (platinum-silver/silver chloride electrodes with results converted corresponding to the standard hydrogen electrode) were measured with electrodes (Blue Line, Xylem Analytics Germany Sales GmbH & Co. KG, Achalaich, Germany) and the Calimatic 766 Laboratory Meter (Knick Elektronische Messgeräte GmbH & Co. KG, Berlin, Germany). SYBR Green staining was used to determine cell numbers in liquid samples via fluorescence microscopy (Hedrich et al., 2016). Statistical analyses were performed in SigmaPlot version 12.3 (Systat Software, Inc., San Jose, CA, USA). Prior to statistical tests, basic data analyses were performed, including visual inspection of all measured variables coupled with the Shapiro–Wilk normality test. Analysis of variance (ANOVA) was used to test for the treatment effect, that is, differences between temperature and pH.
3 Results
3.1 Physiological data of laterite bioleaching experiments (pH, redox potential, and iron concentration)
3.1.1 Laterite bioleaching at 30°C with Acidithiobacillus thiooxidans in the presence or absence of Leptospirillum ferrooxidans
Mean solution pH values during the pH-maintained bioleaching experiments with the At. thiooxidans pure culture and the At. thiooxidans–L. ferrooxidans mixed cultures were 1.52 ± 0.1 and 1.49 ± 0.1, respectively (Figure 1). In bioreactors containing the At. thiooxidans–L. ferrooxidans mixed culture without maintained pH, the pH was similar, with a mean pH of 1.52 ± 0.1. For the pure culture of At. thiooxidans, the pH dropped during incubation and reached 1.12 ± 0.1 after 15 days and was significantly different from the mixed culture experiments (p = 0.001). The redox potential in pure culture bioreactors with and without maintained pH behaved similarly (621 ± 32 mV and 622 ± 25 mV, respectively), whereas the redox potential of mixed cultures was considerably higher, reaching 857 ± 5 mV at adjusted maintained pH and 779 ± 11 mV when pH was not maintained (Figure 1). Disregarding whether the pH in the bioreactors was maintained or not, the differences in redox potential between pure and mixed cultures were significant with p = 0.005 and p < 0.001, respectively. Total iron concentration in the solution reached 29.8 ± 2.2 mM and 54.5 ± 13.4 mM in pure and mixed cultures, respectively, when pH was maintained (Figure 2). When pH was not maintained total iron in solution reached 37.8 ± 4.7 mM and 12.4 ± 0.4 mM in pure and mixed cultures, respectively. Ferrous iron as a percentage of total iron in solution behaved very differently in pure and mixed cultures, independent of pH. In all At. thiooxidans cultures, most of the total iron was present as ferrous iron, 98.7 ± 4.3% and 100 ± 1.8% when pH was maintained or not, respectively (Figure 2). In mixed cultures of At. thiooxidans and L. ferrooxidans, the ferrous iron proportion dropped quickly and at the end of experiments only represented 1.8 ± 0.6% and 2.3 ± 0.2% of total iron when pH was maintained or not, respectively. Differences in the amount of ferrous iron as a percentage of total iron were significantly different for pH 1.5 (p < 0.001) and not maintained pH (p < 0.001) between pure and mixed cultures.
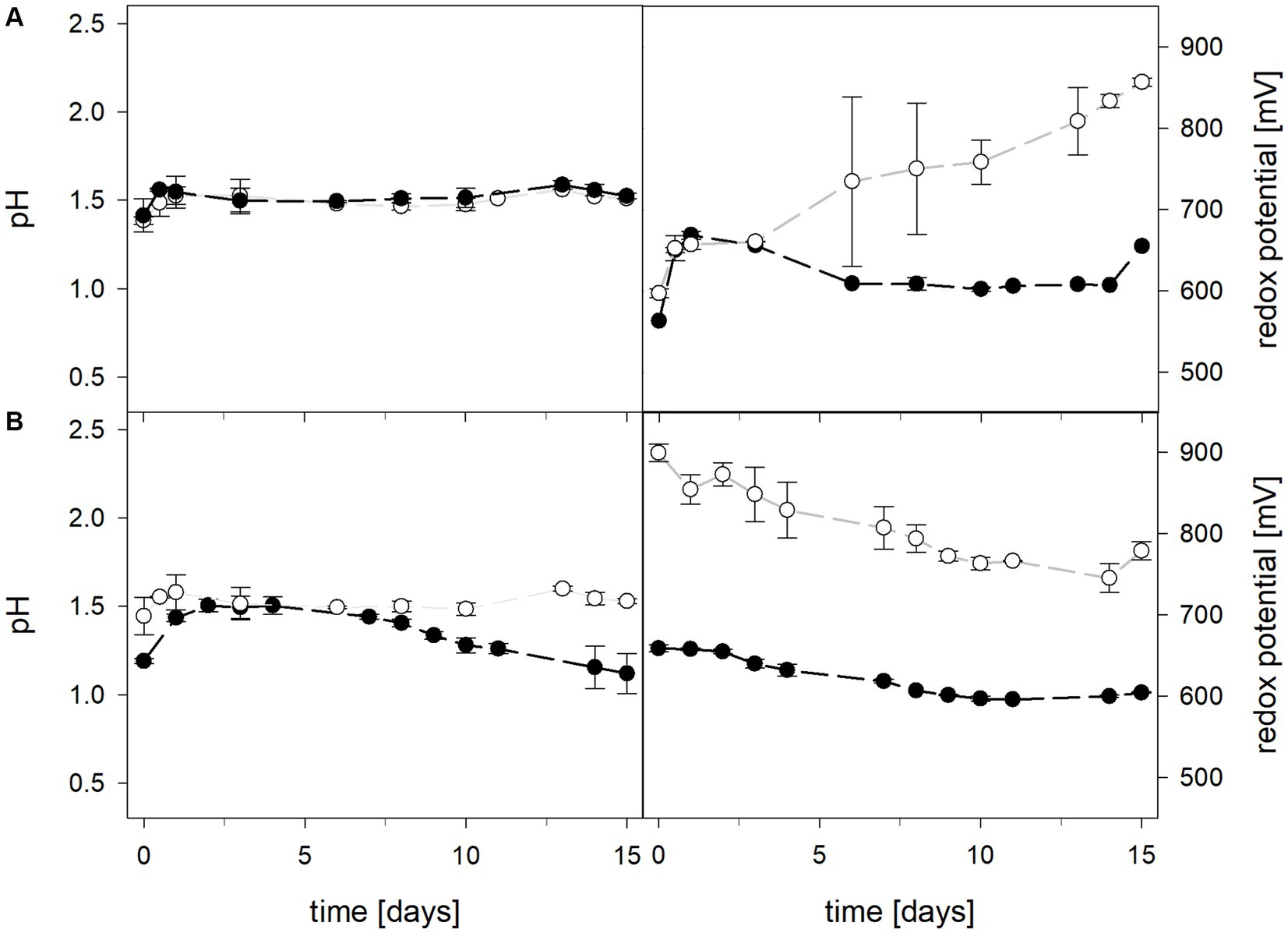
Figure 1. pH (left) and redox potential (right) during laterite bioleaching with a pure culture of At. thiooxidans () and a mixed culture of At. thiooxidans and L. ferrooxidans (
) over 15 days under oxic conditions in 2-L stirred-tank reactors at maintained pH 1.5 (A) or not maintained pH (B). Error bars show standard deviations from the mean values for two parallel bioreactor runs, except for At. thiooxidans and L. ferrooxidans at maintained pH 1.5 (n = 3).
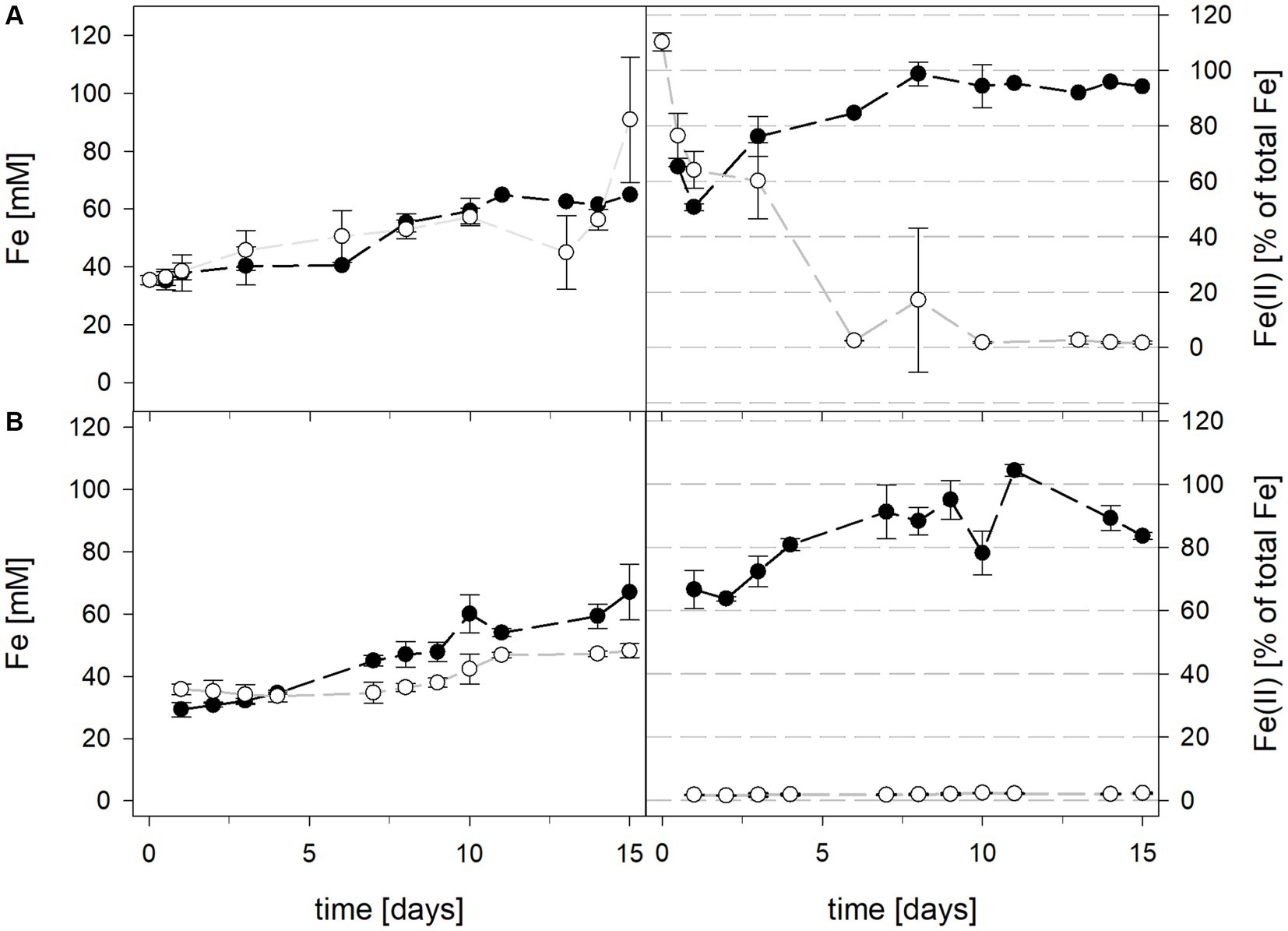
Figure 2. Total iron content (left) and ferrous iron as a percentage of total iron (right) during laterite bioleaching with a pure culture of At. thiooxidans () and a mixed culture of At. thiooxidans and L. ferrooxidans (
) over 15 days under oxic conditions in 2-L stirred-tank reactors at maintained pH 1.5 (A) or not maintained pH (B). Error bars show standard deviations from the mean values for two parallel bioreactor runs, except for At. thiooxidans and L. ferrooxidans at maintained pH 1.5 (n = 3).
3.1.2 Laterite bioleaching at 40°C with Acidithiobacillus caldus in the presence or absence of Leptospirillum ferriphilum
Mean solution pH values of At. caldus and At. caldus–L. ferriphilum during bioleaching at maintained pH were 1.52 ± 0.1 and 1.50 ± 0.1, respectively (Figure 3). When the pH was not maintained in the pure culture of At. caldus and mixed culture of At. caldus and L. ferriphilum, the pH behaved similarly and dropped below 1, reaching a minimum of 0.78 ± 0.0 in pure culture and 0.77 ± 0.1 in mixed culture, despite being significantly different throughout incubation (p = 0.012). The mean redox potential in pure culture at maintained pH was 649 ± 19 mV, and 668 ± 13 mV when the pH was not maintained (Figure 3). Again, the redox potential of the mixed culture was higher and climbed to 882 ± 8 mV at pH 1.5 and 773 ± 42 mV when pH was not maintained. For both maintained pH 1.5 and not maintained pH, the differences in redox potential between pure and mixed cultures were significant with p = 0.000 and p < 0.001, respectively. Total iron in solution was almost identical at maintained pH in pure and mixed cultures after 15 days reaching 19.5 ± 1.2 mM and 19.2 ± 3.1 mM, respectively. However, ferrous iron represented 94.1% of total iron in pure culture and only 1.8 ± 0.6% in mixed culture (Figure 4), and the difference between the two treatments was significant (p = 0.013). In bioreactors without maintained pH, total iron in solution reached 92.4 ± 5.0 mM in pure culture and 42.2 ± 9.7 mM in mixed culture, of which 59.1 ± 13.6% and 11.2 ± 2.0% were present as ferrous iron, respectively (Figure 4), presenting a statistically significant difference with p < 0.001.
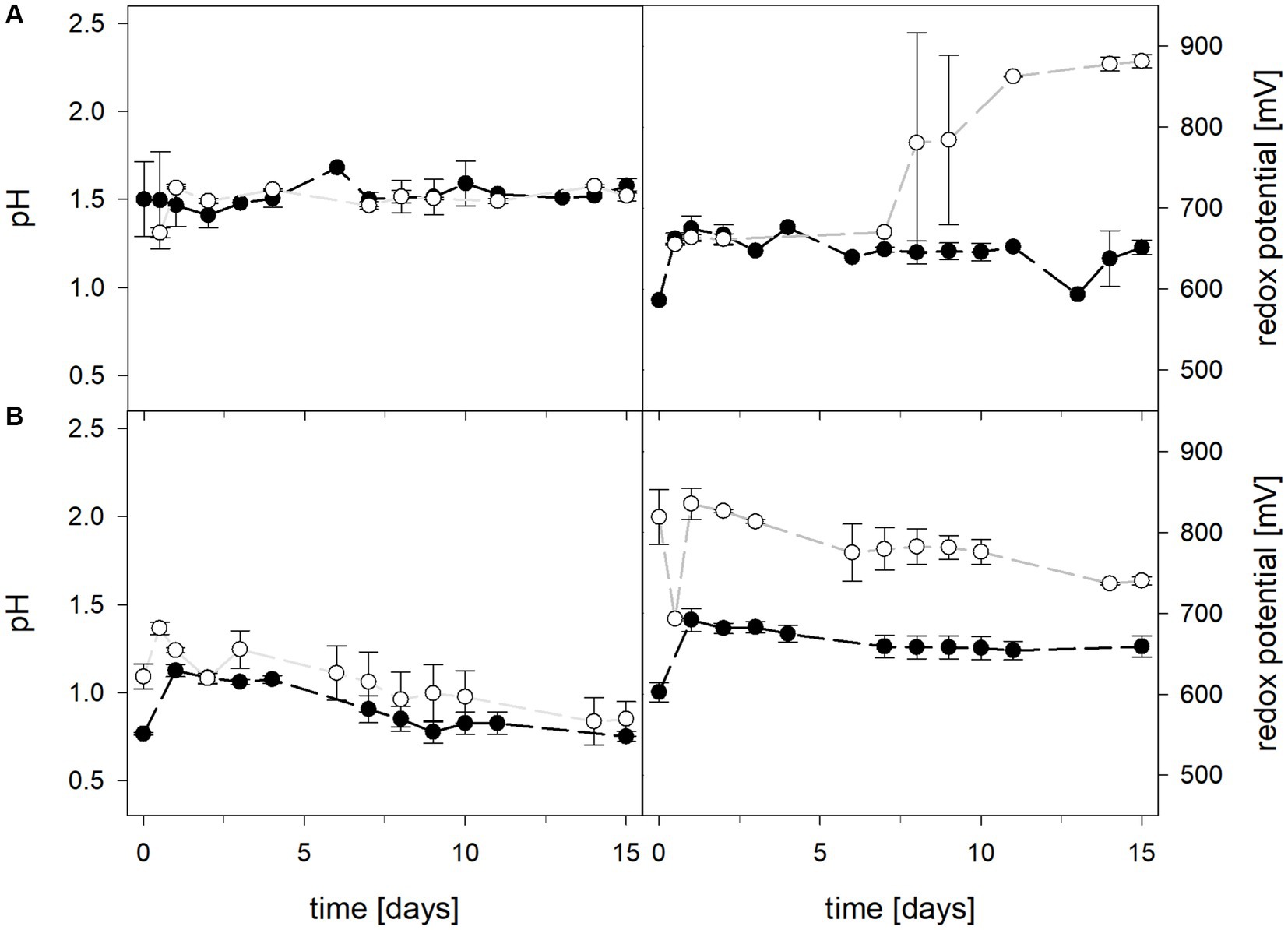
Figure 3. pH (left) and redox potential (right) during laterite bioleaching with a pure culture of At. caldus () and a mixed culture of At. caldus and L. ferriphilum (
) over 15 days under oxic conditions in 2-L stirred-tank reactors at maintained pH 1.5 (A) or not maintained pH (B). Error bars show standard deviations from the mean values for two parallel bioreactor runs.
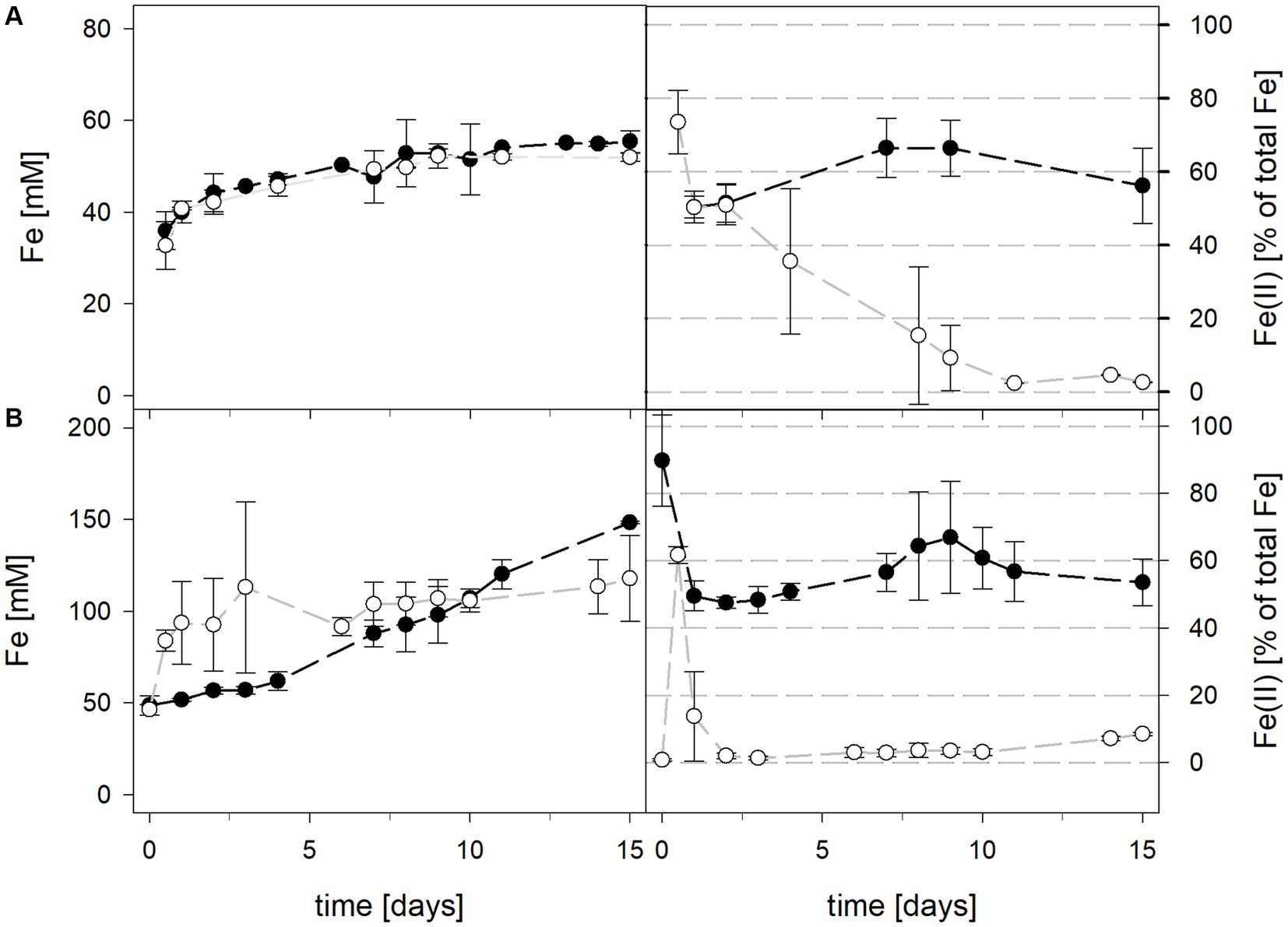
Figure 4. Total iron content (left) and ferrous iron as a percentage of total iron (right) during laterite bioleaching with a pure culture of At. caldus () and a mixed culture of At. caldus and L. ferriphilum (
) over 15 days under oxic conditions in 2-L stirred-tank reactors at maintained pH 1.5 (A) or not maintained pH (B). Error bars show standard deviations from the mean values for two parallel bioreactor runs.
3.1.3 Laterite bioleaching with mesophilic or moderately thermophilic consortia of acidophiles in the presence or absence of Leptospirillum species
During bioleaching at maintained pH, the mean pH values of the 30°C and 40°C consortia were 1.50 ± 0.1 and 1.49 ± 0.1, respectively (Figure 5). When pH was not maintained in consortia bioreactors at 30°C and 40°C, the pH behaved similarly and dropped down during incubation, reaching 1.06 ± 0.2 at 30°C and 1.30 ± 0.1 at 40°C. Differences in pH between maintained and not maintained treatments with consortia were statistically significant at 40°C (p = 0.046), but not at 30°C. The mean redox potential at 30°C and maintained pH was 818 ± 39 mV, and 828 ± 31 mV when the pH was not maintained (Figure 5). The redox potential at 40°C was higher, though also in a similar range with mean values of 824 ± 23 mV at pH 1.5 and 838 ± 18 mV when pH was not maintained. Total iron concentration in solution was comparable at 30°C and 40°C after 15 days when pH was maintained (48.3 ± 6.3 mM and 51.6 ± 6.8 mM, respectively) and not maintained (57.7 ± 10.8 mM and 61.4 ± 15.8 mM, respectively) (Figure 6). The ferrous iron concentration was low during incubation and the mean values ranged between 2.4 and 3.3% of total iron in all reactors (Figure 6).
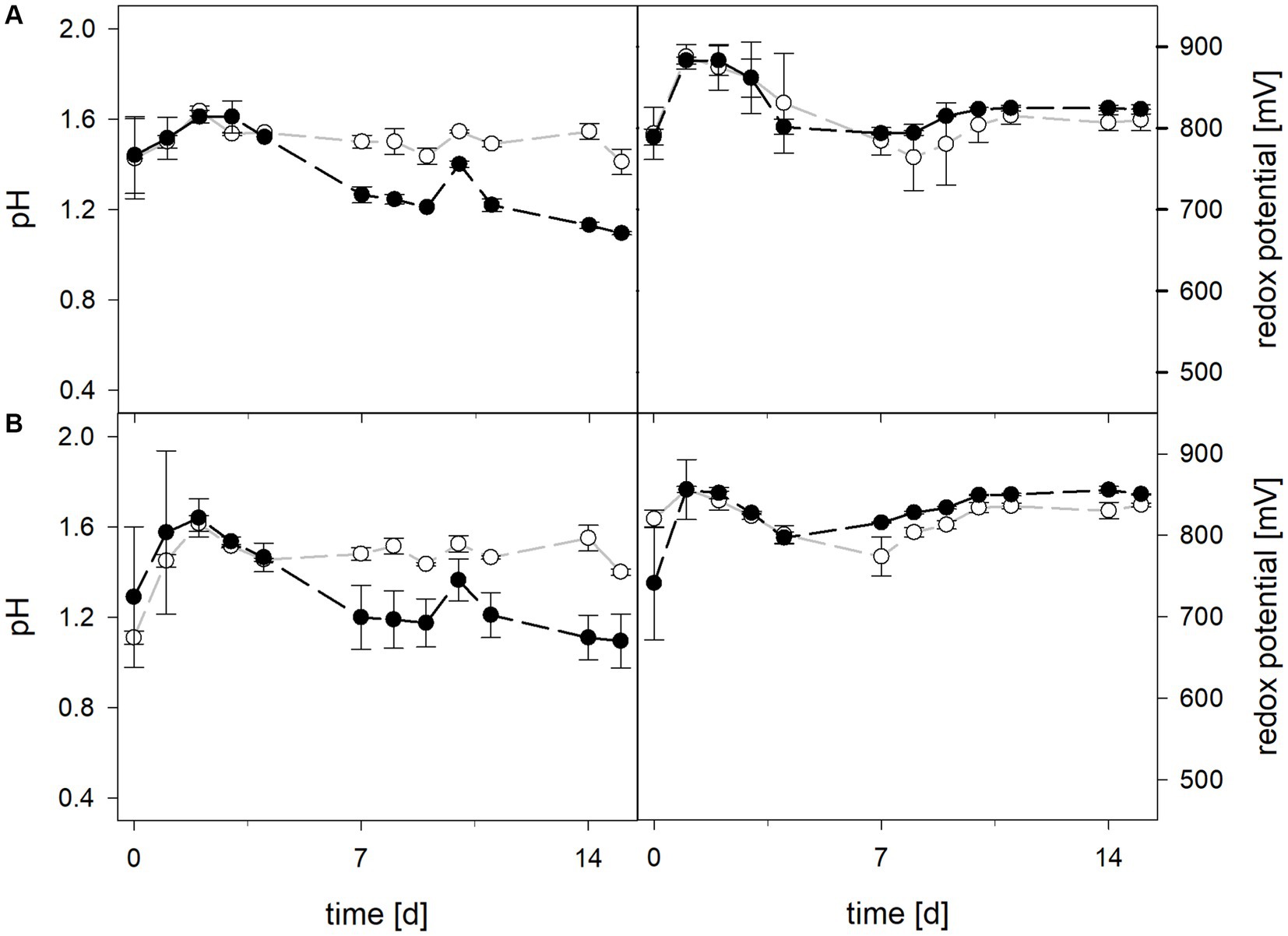
Figure 5. pH (left) and redox potential (right) during laterite bioleaching with consortia consisting of acidophilic sulfur- and iron-oxidizers at maintained pH 1.5 () and not maintained pH (
) over 15 days under oxic conditions in 2-L stirred-tank reactors at 30°C (A) or 40°C (B). Error bars show standard deviations from the mean values for three and two parallel bioreactor runs at maintained pH 1.5 and not maintained pH, respectively.
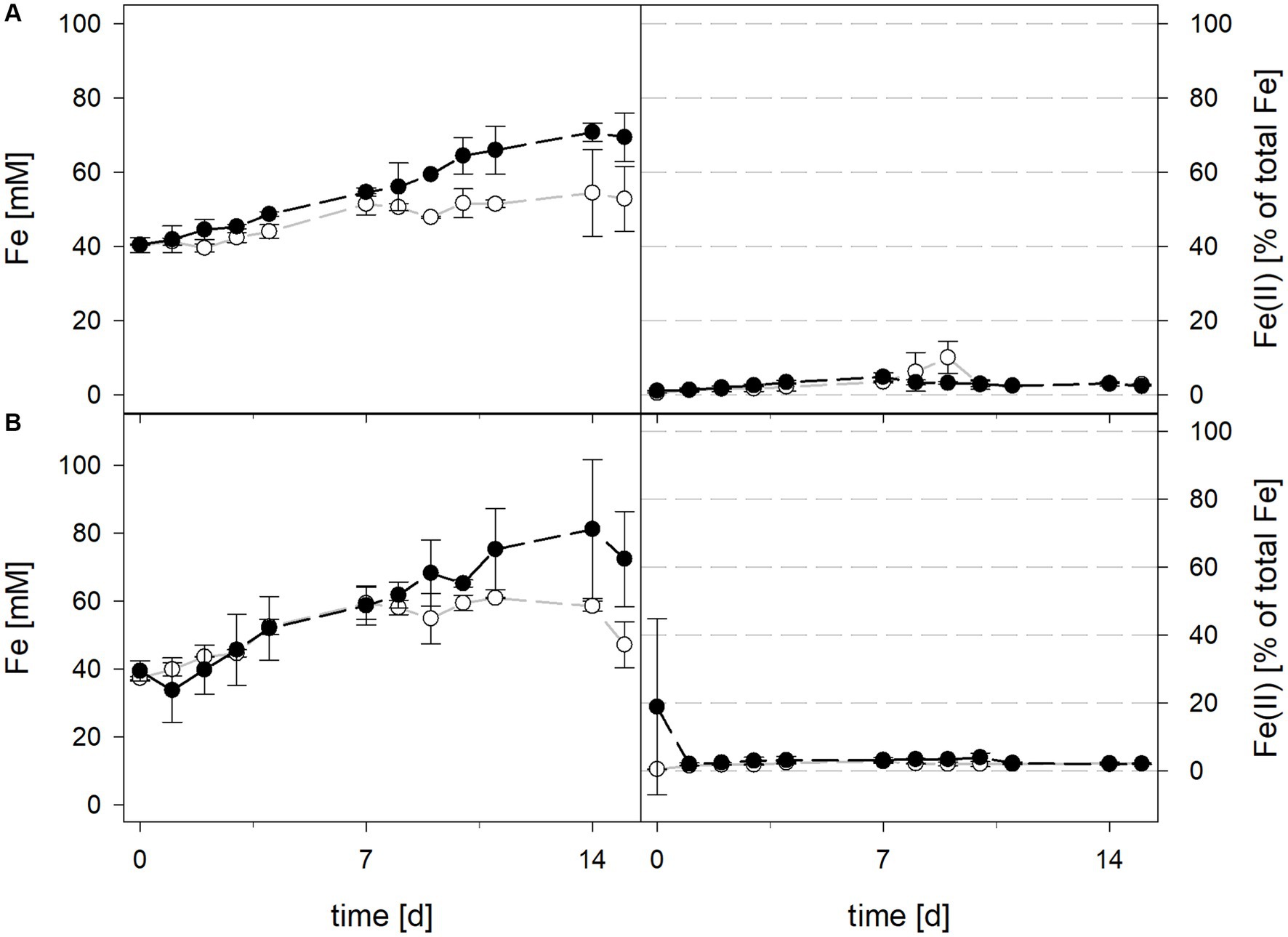
Figure 6. Total iron content (left) and ferrous iron as a percentage of total iron (right) during laterite bioleaching with consortia consisting of acidophilic sulfur- and iron-oxidizers at maintained pH 1.5 () and not maintained pH (
) over 15 days under oxic conditions in 2-L stirred-tank reactors at 30°C (A) or 40°C (B). Error bars show standard deviations from the mean values for three and two parallel bioreactor runs at maintained pH 1.5 and not maintained pH, respectively.
3.2 Cell numbers during laterite bioleaching
Planktonic cell numbers were similar in 30°C bioreactors with the pure culture of At. thiooxidans and the mixed culture of At. thiooxidans–L. ferrooxidans when pH was not maintained (Figure 7). Bioreactors were inoculated with 2.51 × 105 ± 2.96 × 104 cells mL−1 of At. thiooxidans and 4.33 × 106 ± 4.74 × 106 cells mL−1 of the At. thiooxidans–L. ferrooxidans mixed culture. After 4 days of growth and before the addition of the laterite sample, bioreactors with pure culture contained 2.25 × 106 ± 9.14 × 105 cells mL−1, whereas bioreactors with mixed culture contained 8.64 × 106 ± 7.47 × 106 cells mL−1. Cell numbers increased in all bioreactors (with the initial difference in cell densities being reduced), reaching 3.35 × 107 ± 7.68 × 106 cells mL−1 in pure culture and 4.95 × 107 ± 1.46 × 106 cells mL−1 in mixed culture. Cell numbers for bioreactors with the mixed culture of At. caldus and L. ferriphilum started at 2.78 × 105 ± 3.87 × 105 cells mL−1 and reached 9.16 × 106 ± 4.98 × 106 cells mL−1 after 4 days, just before the addition of laterite (Figure 7). After 14 days of bioleaching by mixed cultures, the mean cell count reached 2.65 × 107 ± 4.97 × 106 cells mL−1. Unfortunately, cell numbers for At. caldus pure culture for inoculation of the bioreactors are not available due to technical reasons, but cell numbers had reached 1.95 × 107 ± 1.71 × 106 cells mL−1 before laterite was added (Figure 7). The cell counts showed some fluctuation during bioleaching and reached 3.97 × 107 ± 8.65 × 106 cells mL−1 after 5 days. The mean cell number of pure At. caldus culture and that of At. caldus–L. ferriphilum mixed culture was statistically significantly different (p = 0.024).
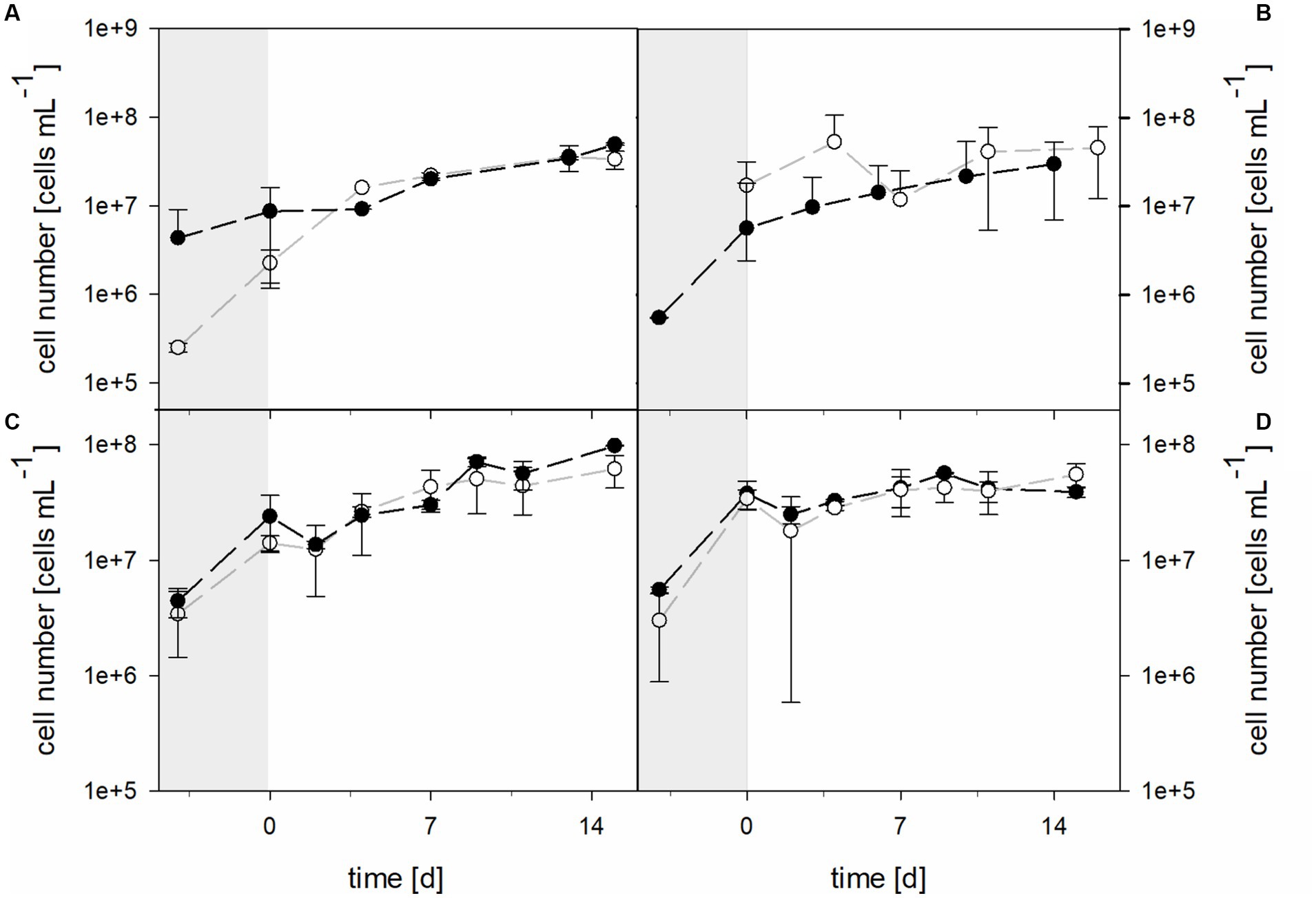
Figure 7. Cell numbers during pre-growth (shaded areas) and laterite bioleaching (days 0 to 14/15) with (A) At. thiooxidans () and At. thiooxidans–L. ferrooxidans (
) at not maintained pH and 30°C, (B) At. caldus (
) and At. caldus–L. ferriphilum (
) at not maintained pH and 40°C, and consortia consisting of acidophilic sulfur- and iron-oxidizers at maintained pH 1.5 (
) and not maintained pH (
) under oxic conditions in 2-L stirred-tank reactors at 30°C (C) or 40°C (D). Error bars show standard deviations from the mean values for two parallel bioreactor runs.
The cell numbers in consortia followed similar trends and showed no significant differences during laterite bioleaching at either 30°C or 40°C at maintained pH 1.5 or not maintained pH (Figure 7).
At 30°C the initial cell density was 3.42 × 106 ± 1.99 × 106 cells mL−1 in bioreactors with pH maintained at 1.5, while 4.44 × 106 ± 1.28 × 106 cells mL−1 were present in bioreactors without maintained pH. After 4 days of growth and before the addition of the laterite sample, bioreactors with pH 1.5 contained 1.41 × 107 ± 2.15 × 106 cells mL−1, whereas bioreactors without maintained pH contained 2.40 × 107 ± 1.24 × 107 cells mL−1. Cell numbers increased in all approaches, reaching 6.18 × 107 ± 1.95 × 107 cells mL−1 at pH 1.5 and 9.84 × 107 ± 9.71 × 105 cells mL−1 when pH was not maintained. Bioreactors running at 40°C with the moderately thermophilic consortium at maintained or not maintained pH had initial cell densities of 3.01 × 106 ± 2.12 × 106 and 5.56 × 106 ± 3.34 × 105 cells mL−1, respectively, and reached 3.42 × 107 ± 6.64 × 106 and 3.78 × 107 ± 10.4 × 107 cells mL−1 before the addition of the laterite sample (Figure 7). During bioleaching, the cell numbers did not change much and were at 5.55 × 107 ± 1.30 × 107 and 3.91 × 107 ± 4.17 × 106 cells mL−1 at the end of the runs at pH 1.5 and without maintained pH.
3.3 Extraction of nickel, cobalt, and iron in laterite bioleaching experiments
The leaching degree after 15 days with maintained pH for cobalt was 56.9% and for nickel 29.3% in At. thiooxidans pure culture and 57.8 ± 4.6% for cobalt and 26.5 ± 1.7% for nickel in mixed culture with L. ferrooxidans. When the pH was not maintained the leaching degrees for cobalt and nickel in pure culture were 69.3 ± 3.9% and 38.6 ± 3.6%, respectively, and 67.4 ± 7.8% and 36.7 ± 8.1% for cobalt and nickel, respectively, in mixed cultures (Figure 8). No statistically significant difference was detected between pure and mixed cultures or pH treatments.
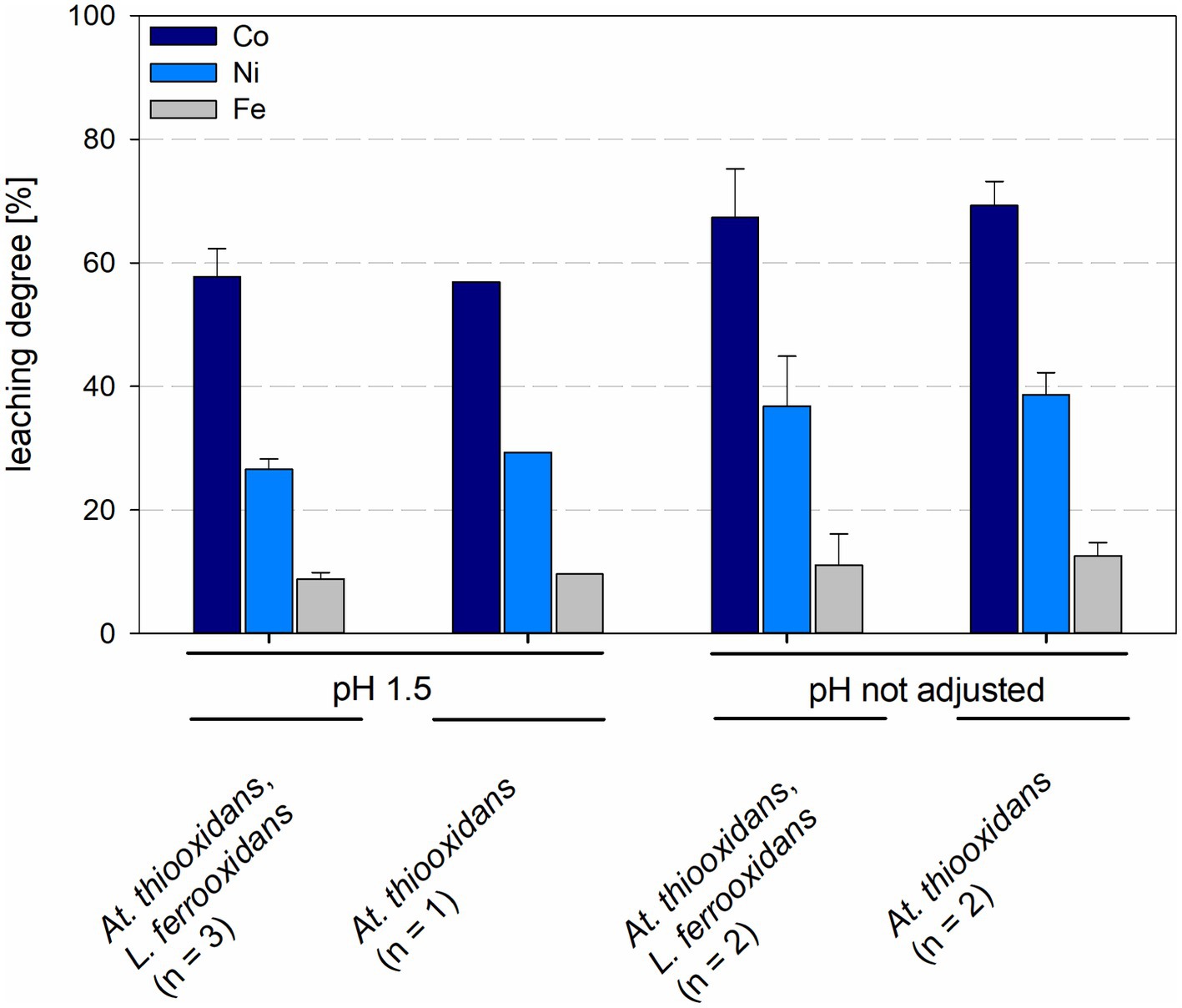
Figure 8. Leaching degree of Co, Ni, and Fe in laterite bioleaching experiments with a pure culture of At. thiooxidans and a mixed culture of At. thiooxidans and L. ferrooxidans after 15 days under oxic conditions in 2-L stirred-tank reactors at pH 1.5 or not maintained pH. Error bars show standard deviations from the mean values for n parallel bioreactor runs.
Leaching degrees for cobalt and nickel were 67.8 ± 3.5% and 33.8 ± 0.7%, respectively, for At. caldus at pH 1.5 and 70.8 ± 2.2% and 45.2 ± 1.6%, respectively, for At. caldus at not maintained pH. Mixed cultures of At. caldus and L. ferriphilum at pH 1.5 showed leaching degrees of 61.5 ± 9.4% for cobalt and 31.0 ± 5.9% for nickel, when the pH was not maintained the leaching degrees were 68.5 ± 0.2% for cobalt and 38.8 ± 2.2% for nickel (Figure 9). The leaching degree of Ni was statistically significantly different between At. caldus at pH 1.5 and not maintained pH (p = 0.012).
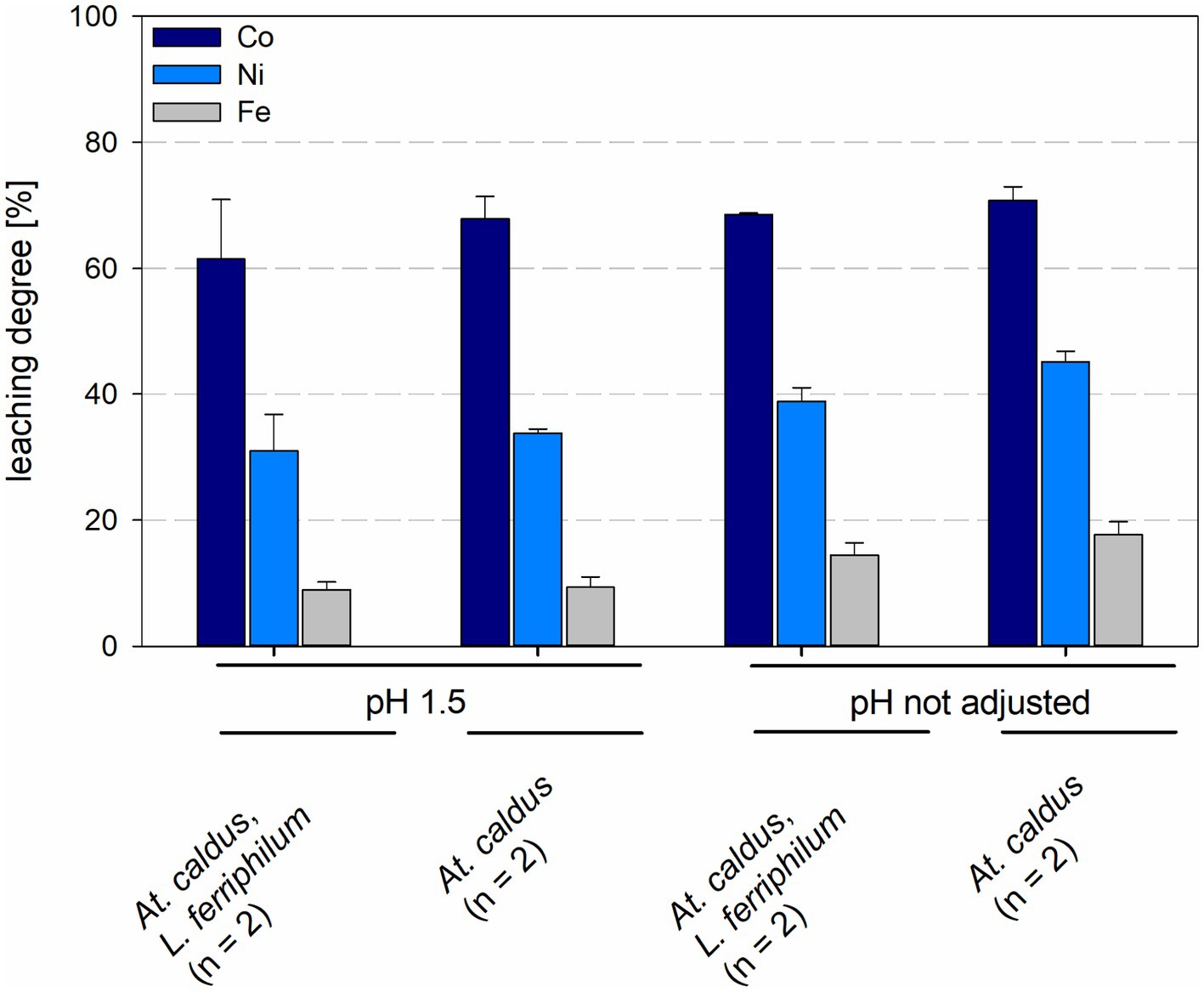
Figure 9. Leaching degree for Co, Ni, and Fe in laterite bioleaching experiments with a pure culture of At. caldus and a mixed culture of At. caldus and L. ferriphilum after 15 days under oxic conditions in 2-L stirred-tank reactors at pH 1.5 or not maintained pH. Error bars show standard deviations from the mean values for n parallel bioreactor runs.
In bioreactors with consortia consisting of different acidophilic sulfur- and iron-oxidizers leaching degrees of cobalt and nickel were similar in all reactors, regardless of temperature and pH. Leaching degrees for cobalt and nickel at 30°C were 68.7 ± 8.8% and 31.8 ± 1.2%, respectively, at pH 1.5 and 66.8 ± 0.7% and 37.4 ± 0.3%, respectively, at not maintained pH, with leaching degrees of Ni showing a statistically significant difference (p = 0.024) between maintained and not maintained pH for 30°C. Consortia at 40°C and maintained pH 1.5 showed leaching degrees of 67.9 ± 4.5% for cobalt and 35.4 ± 2.3% for nickel, when the pH was not maintained the leaching degrees were 65.2 ± 1.2% for cobalt and 39.6 ± 3.9% for nickel (Figure 10). No statistically significant difference was detected between maintained and not maintained pH for 40°C.
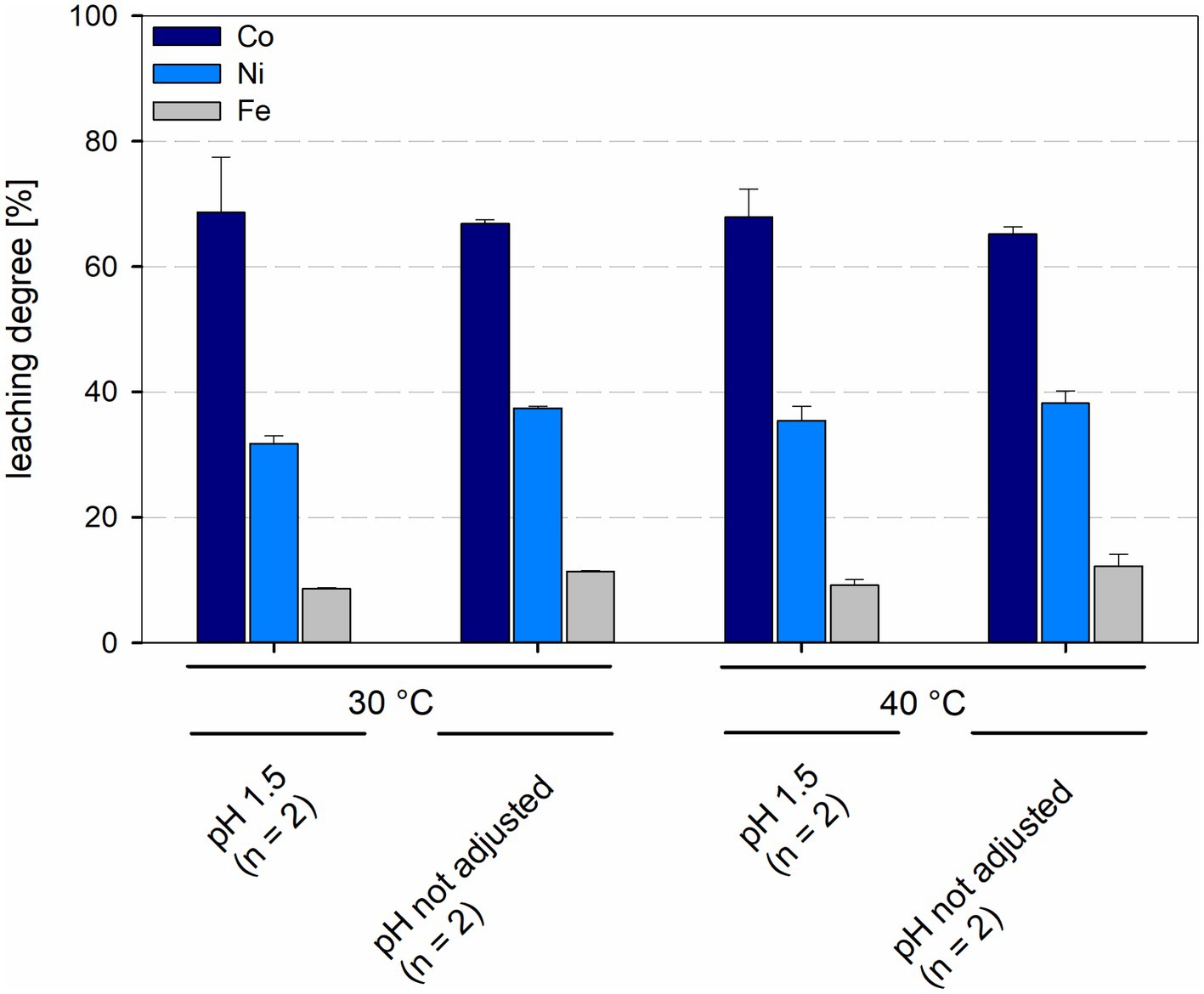
Figure 10. Leaching degree for Co, Ni, and Fe in laterite bioleaching experiments with a mesophilic (30°C) and a moderately thermophilic (40°C) consortium after 15 days under oxic conditions in 2-L stirred-tank reactors at pH 1.5 or not maintained pH. Error bars show standard deviations from the mean values for two parallel bioreactor runs.
4 Discussion
This study confirmed that bioleaching of laterite with acidophilic, sulfur-oxidizing bacteria under oxic conditions is a viable process option. Extraction of nickel and cobalt in stirred-tank reactor bioleaching experiments with the same laterite sample BaSt and pure cultures of acidophiles was 50 and 76%, respectively, for At. thiooxidans and 56 and 88%, respectively, for At. caldus in a previous study in our laboratory (Stanković et al., 2022); thus, similar values were obtained in this new study. The effect of potential contamination in industrial bioleaching operations with ferrous iron-oxidizing acidophiles active at low pH such as Leptospirillum species (Smith and Johnson, 2018) was particularly investigated in a couple of laterite bioleaching experiments with the addition of Leptospirillum species as artificial contaminants in stirred-tank reactors. As expected, for both mixed cultures, At. thiooxidans with L. ferrooxidans at 30°C as well as At. caldus with L. ferriphilum at 40°C, ferrous iron was efficiently oxidized to ferric iron leading to a small proportion of ferrous iron to total iron and a much higher redox potential than in the bioreactors with the respective pure cultures, At. thiooxidans and At. caldus. Surprisingly, despite acidophilic ferrous iron-oxidizers, that is, Leptospirillum spp., being present and therefore ferrous iron concentrations being low and redox potentials maintained high, leaching of cobalt and nickel was almost as efficient as in pure cultures of sulfur-oxidizing Acidithiobacillus spp. under identical conditions in bioreactors. Results imply an efficient reductive dissolution of the laterite mineral phase, despite the concentrations of ferrous iron being barely detectable in mixed cultures of sulfur- and iron-oxidizers (Smith and Johnson, 2018).
In their study using a co-culture of At. caldus with L. ferriphilum under oxic conditions, Smith and Johnson (2018) stated that ferric iron sensitivity and a high positive redox potential were responsible for lower rates of both sulfur oxidation and growth of At. caldus, which might explain the somehow lower but not statistically significant bioleaching efficiency in mixed culture in our experiment. In fact, At. thiooxidans might be less sensitive to high ferric iron concentrations, and therefore, the leaching degree was almost the same as in mixed culture with L. ferrooxidans.
Overall, the difference in cobalt and nickel extraction was negligible for the different experiments (despite different pH values); thus, the question remains why a dramatically lowered ferrous iron concentration in the presence of Leptospirillum spp. did not have a significant effect on the bioleaching of laterite. Ferrous iron is considered to be an efficient reductant for several oxidized cobalt and nickel-bearing mineral phases in laterite throughout bioleaching with acidophiles (Stanković et al., 2022; Moro et al., 2023; Santos and Schippers, 2023). However, during the oxidation of elemental sulfur by acidophiles under oxic conditions ferric iron is likely chemically reduced, probably by sulfur compounds formed during the process as suggested by Marrero et al. (2020). Recently, hydrogen sulfide and thiosulfate were detected as such sulfur intermediates in ferric iron reduction experiments with acidophilic sulfur-oxidizers (Breuker and Schippers, 2024). Such inorganic sulfur compounds would also directly (not only indirectly via iron cycling) reduce mineral phases such as manganese and iron(hydr)oxides (Schippers and Jørgensen, 2001) carrying nickel and cobalt; thus, in fact, these compounds might be even more relevant for reductive laterite bioleaching under oxic conditions than ferrous iron.
Overall, the dissolution of oxide minerals such as goethite in limonitic laterites is based on three processes (Hallberg et al., 2011; Johnson et al., 2021; Vera et al., 2022), which might co-occur during “reductive” bioleaching of laterites with sulfur-oxidizing acidophiles:
1. Microbial acid generation via oxidation of elemental sulfur to sulfuric acid leads to acid leaching.
2. Microbial ferric iron reduction coupled with elemental sulfur oxidation shifts the equilibrium between goethite solid-phase and soluble ferric iron, thereby contributing to goethite dissolution.
3. Microbial release of inorganic sulfur compounds such as hydrogen sulfide serving as a chemical reductant of oxide minerals.
Conclusively, despite the presence of ferrous iron-oxidizing acidophiles (Leptospirillum spp.) significantly reducing the ferrous iron concentration, no significant decrease in the extraction rates of cobalt and nickel from a laterite ore occurred using bioleaching with sulfur-oxidizing acidophiles (Acidithiobacillus spp.) under oxic conditions. Therefore, bioleaching of laterite under oxic conditions remains a suitable process option.
Data availability statement
The raw data supporting the conclusions of this article will be made available by the authors, without undue reservation.
Author contributions
SH: Investigation, Methodology, Formal analysis, Validation, Writing – original draft. AS: Conceptualization, Funding aquisition, Project administration, Writing – review & editing.
Funding
The author(s) declare that financial support was received for the research, authorship, and/or publication of this article. This study was financially supported by the German Federal Ministry of Education and Research (BMBF), CLIENT II project BioProLat, FKZ 033R271A.
Acknowledgments
The authors thank the company Anglo American in Brazil for providing the sample from the Barro Alto mine and several colleagues in BGR: Matthias Hilsberg, Jens Stummeyer, and Dennis Kraemer for chemical ICP-OES analysis; Marcel Weber, Bela Krawinkel, and Isabell Kruckemeyer for technical assistance with bioreactor experiments.
Conflict of interest
The authors declare that the research was conducted in the absence of any commercial or financial relationships that could be construed as a potential conflict of interest.
The author(s) declared that they were an editorial board member of Frontiers, at the time of submission. This had no impact on the peer review process and the final decision.
Publisher’s note
All claims expressed in this article are solely those of the authors and do not necessarily represent those of their affiliated organizations, or those of the publisher, the editors and the reviewers. Any product that may be evaluated in this article, or claim that may be made by its manufacturer, is not guaranteed or endorsed by the publisher.
References
Breuker, A., and Schippers, A. (2024). Rates of iron(III) reduction coupled to elemental sulfur or tetrathionate oxidation by acidophilic microorganisms and detection of sulfur intermediates. Res. Microbiol. 175:104110. doi: 10.1016/j.resmic.2023.104110
du Plessis, C. A., Slabbert, W., Hallberg, K. B., and Johnson, D. B. (2011). Ferredox: a biohydrometallurgical processing concept for limonitic nickel laterites. Hydrometallurgy 109, 221–229. doi: 10.1016/j.hydromet.2011.07.005
Hallberg, K. B., Grail, B. M., du Plessis, C. A., and Johnson, D. B. (2011). Reductive dissolution of ferric iron minerals: a new approach for bio-processing nickel laterites. Miner. Eng. 24, 620–624. doi: 10.1016/j.mineng.2010.09.005
Hedrich, S., Guézennec, A.-G., Charron, M., Schippers, A., and Joulian, C. (2016). Quantitative monitoring of microbial species during bioleaching of a copper concentrate. Front. Microbiol. 7:2044. doi: 10.3389/fmicb.2016.02044
Johnson, D. B., and du Plessis, C. A. (2015). Biomining in reverse gear: Using bacteria to extract metals from oxidised ores. Min. Eng. 75, 2–5.
Johnson, D. B., Smith, S. L., and Santos, A. L. (2021). Bioleaching of transition metals from limonitic laterite deposits and reassessment of the multiple roles of sulfur-oxidizing acidophiles in the process. Front. Microbiol. 12:703177. doi: 10.3389/fmicb.2021.703177
Malik, L., and Hedrich, S. (2022). Ferric iron reduction in extreme acidophiles. Front. Microbiol. 12:818414. doi: 10.3389/fmicb.2021.818414
Marrero, J., Coto, O., Goldmann, S., Graupner, T., and Schippers, A. (2015). Recovery of nickel and cobalt from laterite tailings by reductive dissolution under aerobic conditions using Acidithiobacillus species. Environ. Sci. Technol. 49, 6674–6682. doi: 10.1021/acs.est.5b00944
Marrero, J., Coto, O., and Schippers, A. (2017). Anaerobic and aerobic reductive dissolutions of iron-rich nickel laterite overburden by Acidithiobacillus. Hydrometallurgy 168, 49–55. doi: 10.1016/j.hydromet.2016.08.012
Marrero, J., Coto, O., and Schippers, A. (2020). “11 metal bioleaching: fundamentals and geobiotechnical application of aerobic and anaerobic acidophiles” in Biotechnological applications of extremophilic microorganisms. ed. N. M. Lee (Berlin, Boston: De Gruyter), 261–288.
Moro, K., Haubrich, F., Martin, M., Grimmer, M., Hoth, N., and Schippers, A. (2023). Reductive leaching behaviour of manganese and cobalt phases in laterite and manganese ores. Hydrometallurgy 220:106101. doi: 10.1016/j.hydromet.2023.106101
Ňancucheo, I., Rowe, O. F., Hedrich, S., and Johnson, D. B. (2016). Solid and liquid media for isolating and cultivating acidophilic and acid-tolerant sulfate-reducing bacteria. FEMS Microbiol. Lett. 363:fnw083. doi: 10.1093/femsle/fnw083
Roberto, F. F., and Schippers, A. (2022). Progress in bioleaching: part B, applications of microbial processes by the minerals industries. Appl. Microbiol. Biotechnol. 106, 5913–5928. doi: 10.1007/s00253-022-12085-9
Santos, A. L., and Schippers, A. (2023). “Reductive mineral bioprocessing” in Biomining technologies: extracting and recovering metals from ores and wastes. eds. D. B. Johnson, C. G. Bryan, M. Schlömann, and F. Roberto (Cham: Springer International Publishing), 261–274.
Sarkodie, E. K., Jiang, L., Li, K., Yang, J., Guo, Z., Shi, J., et al. (2022). A review on the bioleaching of toxic metal(loid)s from contaminated soil: insight into the mechanism of action and the role of influencing factors. Front. Microbiol. 13:1049277. doi: 10.3389/fmicb.2022.1049277
Schippers, A. (2007). “Microorganisms involved in bioleaching and nucleic acid-based molecular methods for their identification and quantification” in Microbial processing of metal sulfides. eds. E. R. Donati and W. Sand (Dordrecht: Springer Netherlands), 3–33.
Schippers, A., and Jørgensen, B. B. (2001). Oxidation of pyrite and iron sulfide by manganese dioxide in marine sediments. Geochim. Cosmochim. Acta 65, 915–922. doi: 10.1016/S0016-7037(00)00589-5
Smith, S. L., and Johnson, D. B. (2018). Growth of Leptospirillum ferriphilum in sulfur medium in co-culture with Acidithiobacillus caldus. Extremophiles 22, 327–333. doi: 10.1007/s00792-018-1001-3
Stanković, S., Martin, M., Goldmann, S., Gäbler, H.-E., Ufer, K., Haubrich, F., et al. (2022). Effect of mineralogy on co and Ni extraction from Brazilian limonitic laterites via bioleaching and chemical leaching. Miner. Eng. 184:107604. doi: 10.1016/j.mineng.2022.107604
Stanković, S., Stopić, S., Sokić, M., Marković, B., and Friedrich, B. (2020). Review of the past, present, and future of the hydrometallurgical production of nickel and cobalt from lateritic ores. Metall. Mater. Eng. 26, 199–208. doi: 10.30544/513
Stookey, L. L. (1970). Ferrozine - a new spectrophotometric reagent for iron. Anal. Chem. 42, 779–781. doi: 10.1021/ac60289a016
Keywords: bioleaching, reductive dissolution, laterite, Acidithiobacillus , Leptospirillum , nickel, cobalt
Citation: Hetz SA and Schippers A (2024) Do ferrous iron-oxidizing acidophiles (Leptospirillum spp.) disturb aerobic bioleaching of laterite ores by sulfur-oxidizing acidophiles (Acidithiobacillus spp.)? Front. Microbiol. 15:1359019. doi: 10.3389/fmicb.2024.1359019
Edited by:
Mohammad Ali Amoozegar, University of Tehran, IranReviewed by:
Eva Pakostova, Laurentian University, CanadaWilliam D. Burgos, The Pennsylvania State University, United States
Copyright © 2024 Hetz and Schippers. This is an open-access article distributed under the terms of the Creative Commons Attribution License (CC BY). The use, distribution or reproduction in other forums is permitted, provided the original author(s) and the copyright owner(s) are credited and that the original publication in this journal is cited, in accordance with accepted academic practice. No use, distribution or reproduction is permitted which does not comply with these terms.
*Correspondence: Stefanie A. Hetz, c3RlZmFuaWUuaGV0ekBiZ3IuZGU=; Axel Schippers, YXhlbC5zY2hpcHBlcnNAYmdyLmRl