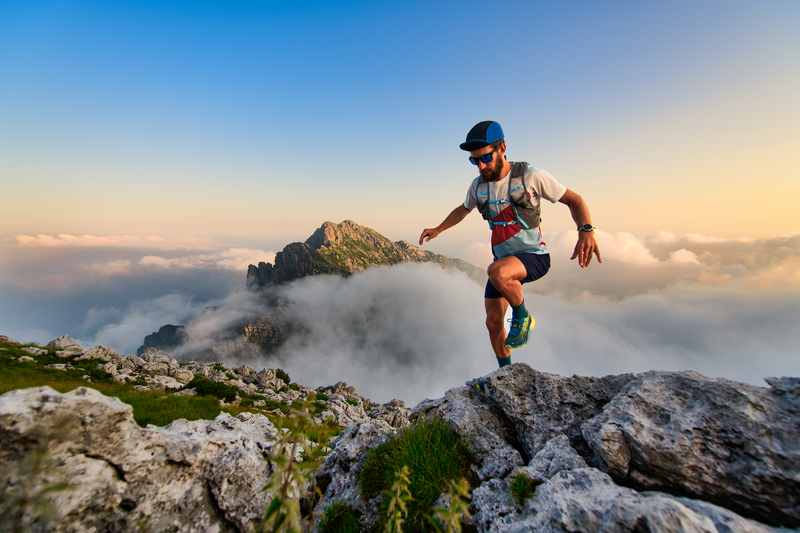
95% of researchers rate our articles as excellent or good
Learn more about the work of our research integrity team to safeguard the quality of each article we publish.
Find out more
REVIEW article
Front. Microbiol. , 06 February 2024
Sec. Infectious Agents and Disease
Volume 15 - 2024 | https://doi.org/10.3389/fmicb.2024.1355234
Major health events caused by pathogenic microorganisms are increasing, seriously jeopardizing human lives. Currently PCR and ITA are widely used for rapid testing in food, medicine, industry and agriculture. However, due to the non-specificity of the amplification process, researchers have proposed the combination of nucleic acid amplification technology with the novel technology CRISPR for detection, which improves the specificity and credibility of results. This paper summarizes the research progress of nucleic acid amplification technology in conjunction with CRISPR/Cas technology for the detection of pathogens, which provides a reference and theoretical basis for the subsequent application of nucleic acid amplification technology in the field of pathogen detection.
A pathogen is defined as an infectious micro-organism or agent, of which viruses and bacteria are the most encountered clinically (Casadevall and Pirofski, 2002). These pathogens are highly evolvable, pathogenic and rapidly spreading, posing a serious threat to human health. Microbial control programmes are increasingly used throughout society to reduce the risk of consumer infection. The bacterial culture method is widely recognised as the “gold standard” for pathogen detection due to its robustness in common laboratory experiments. However, it has disadvantages such as time-consuming, laborious, and inefficient detection, which have significantly hindered its widespread use in the clinic. An alternative method is immunological detection, which is based on the recognition and binding of antigens by specific antibodies (Kohl and Ascoli, 2017). Although it is advantageous in terms of speed, simplicity and specificity in the detection of pathogenic microorganisms, it requires a long period of time for antibody preparation and has a rather low detection sensitivity. Nucleic acid detection technologies, unlike the above-mentioned methods, can simultaneously meet the requirements of accuracy, rapidity and sensitivity for pathogen detection, thus showing superiority in ensuring human safety.
Nucleic acid amplification tests (NAATs) are the most widely used nucleic acid detection technology and have become indispensable tools throughout the life sciences since polymerase chain reaction (PCR) became widely used in biomedicine and other fields in 1980 (Seemayer, 1990). This paper mainly introduces the application of nucleic acid amplification technology combined with the Clustered Regularly Interspaced Short Prepeats (CRISPR)-Cas system in the field of pathogen detection, which provides a reference for the subsequent application of nucleic acid amplification technology in the field of rapid detection.
PCR is the most widely used technical tool for pathogen detection based on nucleic acid amplification. Simulating the process of DNA replication in the central dogma, the PCR process works in vitro by replicating the daughter strand of DNA that is complementary to the parent strand of template DNA after a cycle of denaturation-annealing-extension (Ramesh et al., 1992; Markoulatos et al., 2002). Specific primers are designed to detect different targets, and the target DNA amplification products accumulate exponentially within 25–30 PCR cycles. Due in part to its “amplification” nature, PCR has become a multifunctional tool with improved specificity and sensitivity compared to immunological assays, and can be used to detect a variety of pathogenic bacteria (Malorny et al., 2003; Hadi et al., 2023). To improve the detection performance of PCR, multiplex PCR (mPCR) and quantitative real-time PCR (qPCR) have also been established with the advancement of molecular biology and PCR technology. Alnakip et al. (2023) characterized Staphylococcus aureus and Escherichia coli in 150 cheese samples by multiplex PCR to evaluate their ability to produce virulence factors. Anejo-Okopi et al. (2016) designed specific primers to detect Salmonella virulence gene invA from selected poultry farms using PCR with 91% success rate. Amplification of virulence genes of suspected Salmonella in poultry using PCR is effective and can be used as an alternative tool for rapid detection of Salmonella. However, because the display of the detection results of the above methods is typically based on nucleic acid electrophoresis in either ultraviolet or fluorescence mode, these PCR-only detection methods have drawbacks such as long detection time and inconvenience of on-site testing. How to read the PCR results quickly and easily has become a practical problem that needs to be solved.
As part of the acquired immune system in prokaryotes, the CRISPR/Cas system defends the host organism against the invasion of exogenous genetic elements by destroying foreign plasmid or phage DNA, and this process is centered on CRISPR sequences and Cas proteins (Koonin and Wolf, 2015; Hryhorowicz et al., 2017; Paul and Montoya, 2020). Specifically, the CRISPR sequence is first transcribed and processed into a short crRNA that directs the recognition of the target DNA. The Cas proteins, known as nucleic acid endonucleases, then bind the crRNA to form an RNA-protein complex. After further binding to the target DNA under the guidance of the crRNA, the Cas proteins then cut the target DNA, creating a gap in the gene. Gene repair by homologous recombination or non-homologous end joining enables gene editing (Figure 1; Savić and Schwank, 2016; Paul and Montoya, 2020; Liu et al., 2022).
In a study conducted by Wang et al. (2023) they developed a method that can detect the presence of terminators in transgenic plants using a combination of PCR and CRISPR/Cas12a techniques. The method can be used to identify the target gene levels in about 50 minutes. In addition, accurate detection of all 11 samples confirmed the applicability. Yang et al. (2022) integrated the CRISPR/Cas system to amplify the amplification refractory mutation system qPCR (ARMS-qPCR) to detect single nucleotide polymorphism (SNP) in Salmonella enterica. The results of the experiment revealed that the detection rate was 0.5%, which is lower than the limit of detection that gel electrophoresis can provide. This method can be utilized to analyze the various genes that are involved in the resistance to drugs (Figure 2A). Cas13a, a remarkable Cas protein, exhibits non-canonical RNAase activity that can be activated by crRNA-mediated target RNA recognition. This feature allows the combinatorial use of Cas13a activity and isothermal amplification. Due to the programmability of the crRNA sequence and the spin-off effect of the Cas13a protein. Zhou et al. (2020) developed a CRISPR/Cas13a-based Bacterial Detection (CCB) assay to directly detect Staphylococcus aureus (S. aureus) after PCR amplification with high sensitivity and selectivity. The results showed that the CCB assay was able to successfully detect target genomic DNA (gDNA) down to 100 Am with LOD of 1 cfu/mL, and the dynamic detection range of S. aureus was 100 ~ 107 cfu/mL (Figure 2B).
Figure 2. (A) Schematic illustration of the Carms assay for distinguishing Salmonella enterica species with single-nucleotide resolution and the investigation of the resistance of drug-resistant S. enterica to salt stress (Yang et al., 2022); (B) proposed CCB-Detection for Staphylococcus aureus sensing (Zhou et al., 2020).
PCR, as the gold standard of molecular detection, is reproducible, sensitive and widely applicable, but requires electrophoresis, color development and other steps, which are instrument-dependent, complicated and not convenient for on-site detection. Despite the widespread application of PCR, amplifying complex or long DNA segments is challenging. The discovery of the CRISPR/Cas technology provides a feasible alternative to on-site detection of PCR technology. Meanwhile, the application of CRISPR/Cas system also provides new ideas and methods for the optimization and improvement of PCR technology. In order to overcome the shortcomings of PCR, researchers have explored new molecular detection techniques by combining PCR technology with CRISPR/Cas technology, and through further improvement and optimization, the technology has been widely used in many fields and has had a great impact (Rakshit et al., 2022; Zhang et al., 2022; Cao et al., 2023; Meng et al., 2023; Tian et al., 2023).
Despite the flourishing development of PCR techniques and its widespread use in combination with the CRSPR/Cas technique for molecular detection, the need for a thermal cycling step during PCR experiments has significantly hindered its use for on-site real-time detection. Isothermal nucleic acid amplification, a constant-temperature nucleic acid amplification technique, has circumvented the need for the demanding thermal cycling step in a canonical PCR experiment, and therefore can usually complete the amplification process in a shorter time while maintaining high efficiency, sensitivity, and accuracy. Representative isothermal amplification technologies mainly include Loop-Mediated Isothermal Amplification (LAMP) (Notomi et al., 2000), Recombinase Polymerase Amplification (RPA) (Piepenburg et al., 2006), Strand Displacement Amplification (SDA) (Walker et al., 1992), Helicase Dependent Amplification (HDA) (Vincent et al., 2004), Rolling Circle Amplification (RCA) (Nilsson et al., 1994), Hybridization Chain Reaction (HCR) (Dirks and Pierce, 2004), etc. It is noteworthy that a canonical isothermal amplification process typically requires an indicator readout (e.g., fluorescent probes) to detect and report the amplification products, while the specificity of the detection process can often be affected by the sequence of the target itself and the specificity of the amplification process. Given the inherent limitations of isothermal amplification, the CRSPR/Cas detection system can serve as both a highly specific method for nucleic acid sequence identification and an idle readout of the amplification process, and the combined use of isothermal amplification and CRSPR/Cas techniques can significantly improve the accuracy of test results.
LAMP technology is a new thermostatic nucleic acid amplification technology suitable for genetic diagnosis reported by Notomi et al. (2000), which has been widely used in the rapid detection of a variety of pathogens. This technique relies on (1) the catalysis of Bst DNA polymerase with strand-substitution ability, and (2) the design of a pair of external primers (i.e., F3 and B3) and a pair of internal primers (i.e., FIP and BIP). The primers can bind to the target sequence under the condition of 60–65°C, and the product is the structure of stem-loop DNA, which can realize 109–1010 times amplification within 15–60 min (Nagamine et al., 2001; Parida et al., 2008; Notomi et al., 2015; Becherer et al., 2020; Park, 2022). The amplification products can then be detected by gel electrophoresis or turbidimetry. Pyrophosphate byproducts are produced during the reaction due to the large amount of DNA being synthesized, and the compound binds to divalent metal ions to form insoluble compounds, which can be used to further observe the amplification reaction. This technology is not only able to use DNA as a template but also to detect target RNA by reverse transcription-LAMP (RT-LAMP) reaction. By using an AMV reverse transcriptase, it is also possible to complete the reaction in one step in a short period of time and under constant temperature conditions (Tomita et al., 2008).
Bao et al. (2020) reported a contamination-free LAMP assisted by CRISPR/Cas9 cleavage, which is superior to CRISPR/Cas9 in terms of reliability and durability. The core of the methodology is to design forward or reverse internal primers in target-independent regions so that the LAMP product contains an in-situ neighboring motif (PAM) site for CRISPR/Cas9 to recognize and cleave. A LAMP-CRISPR/Cas12a-based lateral flow biosensor was developed and used by Lee and Oh (2023) to detect Salmonella with a LOD of 1.22 × 100 CFU/mL. Wang et al. (2021) combined RT-LAMP with Cas12a to detect SARS-CoV-2, and a single-molecule sample could be detected in 45 min, which was consistent with the results of RT-PCR, and this method has the characteristics of short detection time, high sensitivity, and visualization of results. The paper equipment based on RT-LAMP and Cas12a by Cao et al. (2022) developed an RT-LAMP with Cas12a based paper device for SARS-CoV-2 detection in wastewater, where enriched wastewater samples were lysed and introduced into a paper device for detection of specific gene fragments, and the results could be read at 480 nm or in a paper device. Li et al. (2022) investigated the establishment of Immunocapture Magnetic Bead (ICB) enhanced LAMP-based CRISPR/Cas12a method (ICB-LAMP-CRISPR/Cas12a) for the rapid visualization and detection of Campylobacter jejuni. It was captured by the ICB, heated to release the bacterial genomic DNA and used in the LAMP reaction, and the LAMP product was cleaved by CRISPR/Cas12a for detection. Campylobacter jejuni can be detected down to 8 CFU/mL. Furthermore, in addition to ICB capture, the method is performed in a closed pipeline to avoid aerosol contamination. The RT-LAMP-CRISPR-Cas13a method developed by Ortiz-Cartagena et al. (2022) does not require RNA extraction and can be used to detect SARS-CoV-2 viruses in nasopharyngeal samples with 100% specificity and 83% sensitivity (Figure 3).
Figure 3. Workflow of the novel developed and optimized protocol for infectious disease diagnosis based on CRISPR-Cas13a technology (Ortiz-Cartagena et al., 2022).
RPA is a new nucleic acid thermostatic amplification technique developed by Piepenburg et al. in 2006 using protein recombination and repair machineries involved in cell DNA synthesis (Piepenburg et al., 2006). Since its establishment more than 10 years ago, RPA has been widely used in bacteria, fungi, parasites, viruses, drug resistance genes and other fields. Under ATP and PEG conditions, recombinase binds to primers to form a complex, and the DNA template of the complex searches for homologous sequences to start a strand-substitution reaction to form new DNA (Jaroenram and Owens, 2014; Daher et al., 2016; Jia et al., 2020; Tan et al., 2022). Compared with traditional PCR technology, this technology detection range is 37°C-42°C, the required sample concentration is low, can amplify as low as 1–10 DNA copies in 10 min, amplification of a variety of different targets, including RNA, miRNA, ssDNA, and dsDNA, RPA reactions can be detected by real-time fluorescence, gel electrophoresis, chemiluminescence and other methods (Munawar, 2022).
Xiong et al. (2021) established a three-line lateral flow assay mediated by RT-RPA in combination with CRISPR/Cas9 for SARS-CoV-2 diagnosis. The analysis of 64 clinical samples showed that the negative predictive concordance was 100% and the positive predictive concordance was 97.14%. Liu et al. (2021) developed a food safety detection technology (RPA-Cas12a-FS) combining RPA and CRISPR-Cas12a for rapid detection of foodborne pathogens. Zhang et al. (2022) established CRISPR/Cas12a combined with RPA to detect Aspergillus besseyi with a LOD of 1 copy/μL, which was combined with a lateral flow strip assay to visualize the results. Yu et al. (2023) coarsely extracted DNA from vaginal or cervical swabs of pregnant women by heat lysis, then selected the highly conserved region of the cfb gene encoding the Christie-Atkins-Munch-Petersen (CAMP) factor as a target for detection, and enriched the target sequences by amplification using RPA, and finally utilised the CRISPR/Cas12a system for target-specific identification and signal release(Figure 4A). Our team Tian et al. (2021) combined the RPA-Cas12a technique with lateral flow immunoassay, thus realizing the rapid detection of L. monocytogenes. In addition, they developed a method combining digital droplet microfluidic chip technology to realize the simultaneous detection of four pathogenic bacteria with high sensitivity and specificity, which can reach 10 CFU/mL. The future development of this technology has great potential and may be applied to the rapid detection of foodborne pathogens, which is of great significance to ensure food safety. A multiplexed microfluidic platform developed by Zhou et al. (2023) combines RPA and CRISPR technology with the assistance of a heated membrane to enable rapid and cost-effective detection of multiple HPV subtypes while providing convenient temperature control for RPA and CRISPR analysis. The technology can detect HPV16 and HPV18 in less than 30 minutes with high specificity and sensitivity (Figure 4B).
Figure 4. (A) Schematic diagram of the CRISPR-GBS assay (Yu et al., 2023); (B) detection of HPV in patient samples on CRISPR (Zhou et al., 2023).
SDA, or thermostatic amplification of DNA enzymes, is an in vitro DNA amplification technique based on the principle of DNA cleavage by restriction endonucleases at specific cleavage sites, as well as the extension of DNA polymerases at the cleavage sites, and the displacement of downstream DNA fragments in the thermostatic amplification technique (Walker et al., 1992). It also has good compatibility with various signal probes. These attributes render SDA an innovative signal amplification methodology with substantial potential for numerous types of biosensors, encompassing colorimetry, fluorescence, and chemiluminescence (Wang et al., 2022).
Combining real-time fluorescence detection with SDA provides ultrasensitive detection of ochratoxin A with a LOD of 0.01 ng/mL (Guo et al., 2022). Zhou et al. (2018) published a novel method named CRISPR-Cas9-triggered nucleic acid endonuclease-mediated signal-amplified DNA assay (hereinafter referred to as CRISDA), which employs the CRISPR-Cas9 system to amplify and detect double-stranded DNA (dsDNA) efficiently and sensitively. The proposed method employs the high sensitivity and accuracy of CRISPR for target DNA recognition, which is integrated with the powerful peptide nucleic acid (PNA) invasion-mediated endpoint detection to achieve sub-atomic level sensitivity and single-base specificity in even the most complex sample backgrounds (Figure 5). The researchers Wang et al. (2022) developed a colorimetric sensor platform that combines SDA with CRISPR/Cas12a for the detection of serum prostate-specific antigen (PSA). The platform is able to differentiate between blood samples were collected from prostate cancer patients, other types of cancer patients, and healthy individuals. PSA allows SDA to produce amplicons that are recognised by the CRISPR-Cas12a system and will mediate the cleavage of the trans ssDNA of the adjacent ligated DNA, thereby activating the gold nanoparticles (AuNPs), which signal the probe and provide a colourimetric readout with a LOD of 0.03 ng/mL, which reduces the background signal and improves the specificity of the assay. Gong et al. (2021) reported an SDA-assisted CRISPR-Cas12a method for the colorimetric analysis of viral nucleic acids. The Hepatitis B Virus DNA was used as a triggering target, activating the trans-cleavage activity of CRISPR-Cas12a and producing ssDNA. This ssDNA was then hybridized with template DNA and non-specifically cleaved on GOx-modified magnetic beads. The released GOx catalyzed the discoloration of the substrate solution and resulted in visualization of the results. Chi et al. (2022) combined SDA with Cas14 for the detection of circulating miRNAs, a biomarker of cholangiocarcinoma. miRNA was directly amplified by SDA without the need for reverse transcription, which reduced the risk of non-specific amplification, differentiated between miRNAs with similar sequences, and improved the sensitivity of the assay by detecting miRNAs as low as 680 fM within 1 h. Cas14a was efficiently activated by the single-stranded SDA amplicon with a 2.86-fold increase in sensitivity compared to the use of Cas12a (Figure 5).
Figure 5. Schematic reaction mechanism of CRISDA (Zhou et al., 2018).
HDA technology simulates in vivo DNA replication by using deconjugating enzymes to unravel the DNA double-stranded structure, primers to bind to the single-stranded target sequence, synthesize the new double-stranded DNA under the action of DNA polymerase, and repeat the amplification steps. The system requires only two primers (Vincent et al., 2004; Jeong et al., 2009; Barreda-García et al., 2018).
Thermophilic deconvolution enzyme-dependent amplification (tHDA) in combination with CRISPR/Cas12a specifically detects the virulence factor stx2 of E. coli O157:H7, eliminating false-positive results generated by primer dimers due to the binding of crRNA and Cas12a to the target, and detecting E. coli O157:H7 in salad mixtures as low as 103 CFU/g (Kim et al., 2023; Figure 6).
Figure 6. Schematic diagram of the detection of E. coli O157:H7 based on HDA-CRISPR (Kim et al., 2023).
RCA is a nucleic acid assay established to mimic the natural process of microbial circular DNA roll-over replication, acting on the circular DNA molecule (Nilsson et al., 1994), and small cyclic oligonucleotides act as templates for DNA or RNA polymerases to generate long and repetitive product strands (He et al., 2019). The two ends of the loop template are annealed with the ligating template and connected to make the template into a loop, after which the primers and the loop template are annealed and extended by DNA polymerase, and ultimately a long DNA single strand containing multiple repetitions of the template sequence can be obtained, with an amplification efficiency of up to 109-fold (Deng and Gao, 2015).
Qiu et al. (2018) investigated a novel RCA-CRISPR-split-HRP (horseradish peroxidase) method constructed from synthetic biological components. This technology enables the cost-effective and convenient detection of miRNAs with sensitivity as low as fM and single-base specificity. The substrate color changed from light yellow to blue after isothermal amplification by RCA for in vitro inactivation of Cas9 binding and splitting HRP activity assessment, indicating the presence of specific miRNAs. The whole process does not take more than 4 h, is easy to operate and does not require large equipment (Figure 7). Chen et al. (2023) combined branched-chain rolling ring amplification (BRCA) and CRISPR-Cas12a (BRCA-Cas) to establish a detection platform for colorectal cancer-related circulating non-coding RNA, which realized the detection of different circulating RNA in serum. Abnous et al. (2021) combined CRISPR-Cas12a, RCA and AuNPs to introduce an aptamer sensor for highly sensitive detection of aflatoxin and the color of the sample changed from yellow to colorless. The addition of T4 DNA ligase and phi29 DNA polymerase in the presence of the target results in the inactivation of the CRISPR-Cas12a gene editing system and the formation of larger single-stranded DNA structures on the surface of AuNPs. Qing et al. (2021) introduced an immobilization-free electrochemical biosensing platform into the CRISPR/Cas system, which can be used to accurately detect nucleic acids and small molecules associated with diseases, where RCA converts and amplifies the target recognition sequences and is used to activate CRISPR/Cas12a activity. Universal blocker probe (BP) can be cleaved by activated Cas12a, which is not the case for unactivated Cas12a. This electrochemical biosensor combines an RCA-CRISPR/Cas12a platform with a rGO/GCE electrode to detect dsDNA, yielding a cost-effective and versatile tool for clinical diagnostics.
Figure 7. Schematic representation for the blueprint and detection workflow of the RCH method for detecting miRNAs (Qiu et al., 2018).
Dirks and Pierce (2004) introduced the HCR, a type of isothermal and enzyme-free nucleic acid amplification technology, in 2004. HCR is gaining popularity in biotech, forensics, and infectious disease detection. A single-stranded DNA initiator triggers an alternating hybridisation event between two hairpins forming a double helix polymer (Figure 8A; Bi et al., 2017; Zhang et al., 2020; Li et al., 2022). HCR with its unique properties of isothermal, enzyme-free, and high amplification efficiency is widely used in biosensing and biomedical sectors due to its excellent analytical abilities and wide application potential (Zhou et al., 2021).
Figure 8. (A) Fundamental working principle of HCR (Zhang et al., 2020); (B) detection of HPV in patient samples on CRISPR (Qiao et al., 2023).
CRISPR/Cas systems have ignited increasing attention in accurate and sensitive nucleic acids detection. Ke et al. (2022) proposed a new CRISPR/Cas12a-based chemiluminescence enhancement biosensor for nucleic acid detection. With a remarkable sensitivity, it is capable of detecting synthetic DNA targets at a detection limit of 3 pM, and can also single-copy detect plasmids. This study demonstrated the high accuracy of HPV testing, achieving a sensitivity of 88.89% and specificity of 100% in practical application, respectively, showing its superior accuracy. Xing et al. (2020) based on the dual amplification of HCR and CRISPR-Cas12a, we developed the apta-HCR-CRISPR assay for the direct, highly sensitive detection of tumor-derived extracellular vesicle (TEV) protein. HCR amplified TEV protein-targeted aptamer with long-repeated sequences and multiple barcodes, enhancing detection sensitivity and specificity. CRISPR-Cas12a boosts fluorescence signals for highly sensitive quantification of TEV proteins in clinical samples. Qiao et al. (2023) established an amplification free detection method for Salmonella by magnetic separation of dual-function HCR scaffold polyvalent aptamers with CRISPR/Cas12a activity coupling. In the detection system, the dual-function HCR scaffold polyvalent aptamers with high binding affinity and specificity were first prepared by assembling several Salmonella-specific aptamers on the long HCR products. In addition to the enhanced affinity, HCR-Multiapt also contains many repetitive CRISPR-targeting DNA units in its HCR scaffold, which may trigger the trans-cutting activity of Cas12a. In the presence of the target bacteria, HCR-scaffold polyvalents can efficiently attach to the bacterial surface and amplify the bacterial signal into a CRISPR/Cas12a based fluorescent readout. The detection system can perform ultra-sensitive detection of Salmonella in the linear range of 100 ~ 107 cfu/mL with a detection limit of 2 cfu/mL. This method, which possesses high sensitivity, can be effectively utilized for clinical diagnosis (Figure 8B). With the use of CRISPR-Cas13a and enzyme-free nucleic acid amplification. Yang et al. (2021) reported the development and validation of a HCR coupled CRISPR-Cas13a-based assay (Cas-HCR) for detection of SARS-CoV-2. The detection performance of Cas-HCR assay was demonstrated on a home-made optical-fiber evanescent wave fluorescence biosensor capable of collecting the fluorescence excited within an exponentially decaying evanescent field around the optical fiber.
In addition to the above widely used isothermal amplification technologies, there are many isothermal technologies that are being deeply explored and improved. Enzymatic Recombinase Amplification (ERA) is a domestic independent research and development amplification technology, the reaction relies on recombinase derived from low-temperature bacteriophages, can replace amino acids at specific sites, has good amplification speed and specificity, combines ERA with Cas12a to achieve highly sensitive detection of porcine circovirus (Zhang et al., 2021). Real-time fluorescence nucleic acid isothermal amplification detection (SAT) is a combination of nucleic acid isothermal amplification and real-time fluorescence detection, which can design specific primers for the target nucleic acid of pathogens, and can rapidly amplify and detect Mycoplasma pneumoniae with high sensitivity and specificity (Barreda-García et al., 2018; Li et al., 2019). NASBA technology amplifies target RNA under thermostatic conditions by using single-stranded RNA as a template to mimic the replication mechanism of retroviruses in vivo by reverse transcriptase, RNase H and T7 RNA polymerase, and forward and reverse primers (Compton, 1991), and T7RNA polymerase recognizes the DNA promoter sequence and transcribes it into single-stranded RNA for the next cycle (Hønsvall and Robertson, 2017; Ju et al., 2021). Wang et al. (2021) used NASBA technology to diagnose clinical samples from 25 patients with cryptococcal disease with LOD of 10 CFU/mL. Pardee et al. (2016) designed a paper-based sensor for rapid detection of Zika virus and binding to CRISPR/Cas9 to identify virus strains, a platform that can directly detect 2.8 fM Zika virus RNA from infected rhesus monkey serum. This system displays the results through color changes, and can be judged by the naked eye, reducing the cost of use and improving the range of use. The novel exonuclease assisted isothermal nucleic acid amplification (Exo-NAT) technology developed by Ye et al. (2018) was analyzed by using full-length Bst DNA polymerase combined with melting curve, which had ultra-high specificity and good detection limits in both singleplex and multiplex detection, and showed Rotavirus A and Rotavirus A in 42 clinical samples. Astrovirus and adenovirus have been validated with up to 100% specificity and sensitivity. The Hairpins Mediated Amplification (HMA) technique developed by Hongbo et al. (2022) combines LFD to detect specific amplification products and reduce the signal of non-specific oligonucleotide hybridization, thereby making it more specific. HMA was validated by detecting IS6110 fragments of Mtb, combined with LFD to visualize the results. Isothermal Exponential Amplification Reaction (EXPAR) technology relies on the synergy of nickease and DNA polymerase with strand displacement function to achieve exponential amplification of targets in buffer systems containing dNTP, primers (in most cases, targets to be measured), and amplification templates (Mok et al., 2016; Reid et al., 2018). Huang et al. (2018) created a groundbreaking CRISPR/Cas9-based isothermal exponential amplification reaction strategy for rapid and site-specific nucleic acid detection. CRISPR/Cas9 with exponential amplification generates multiple DNA replicas that are detected by real-time fluorescence monitoring. The method is characterized by specificity and rapid amplification kinetics.
Among these, isothermal NAATs have garnered interest due to their operation at a constant temperature (thus requiring minimal laboratory setup) and cost-effectiveness (Dueñas et al., 2022), the combination of isothermal NAATs and CRISPR/Cas technology has been successfully applied in the rapid detection of various pathogens, which has the advantages of low detection cost, high precision, fast quantification, and on-site rapid detection, and has a high potential for popularization and application. Compared to PCR, isothermal NAATs have reduced temperature requirements and assay costs, and different isothermal NAATs combined with CRISPR/Cas systems have different results. The programmable features of CRISPR-Cas12a, accurate target identification and indiscriminate cutting characteristics offer great promise for building fluorescence and colorimetric sensing platforms, especially since the CRISPR-Cas12a system has good compatibility with SDA and other warm signal amplification technologies (Wang et al., 2022). Isothermal amplification, as a novel technology, has many advantages but also faces many challenges, such as false positive signals generated by LAMP non-specific amplification and the complexity of primer design are still widely used technical challenges (Zhang et al., 2021). Compared to other isothermal techniques, RPA is limited by the concentration of DNA, too high a concentration will inhibit the reaction, the amplification process is easily contaminated and the purification process of RPA products is difficult. NASBA amplification has many steps, the reaction system is more complex, not easy to develop, the reaction system needs to be added to the enzyme reaction solution, and the cost is high (Debayan et al., 2023). The HDA technique requires 2 to 3 reaction temperatures, and the unlooped lock-in probe and template DNA or RNA that is not bound to the lock-in probe during the RCA process can also generate signals, which may reduce the sensitivity of the assay, and continuous improvement is still needed. Correctly and efficiently amplifying target sequences at appropriate temperatures and being able to accurately detect sequences and distinguish signals without interference is one of the major problems facing isothermal amplification technology today. Despite the simplicity, low cost, and high accuracy of advanced CRISPR-Cas-based biosensors, the synergistic use of CRISPR-Cas-based dual signal amplification systems for rapid diagnosis of pathogens is still rare (Tables 1, 2).
Nucleic acids, being essential components of all living organisms, provide a wealth of information regarding biological and pathological aspects of life (Zhang et al., 2022). The impact of sample pretreatment on nucleic acid amplification technology is very important, and it can directly affect the efficiency and accuracy of the amplification reaction. DNA or RNA extraction from the sample is the first step in nucleic acid amplification, and the quality and purity of the extraction is critical to the amplification reaction. Low-quality DNA or RNA may result in inefficient amplification, spurious products, or failure. Therefore, selecting the appropriate extraction method and kit and following best practices for the extraction steps can ensure a high quality nucleic acid sample (He et al., 2017; Mullegama et al., 2019). Some inhibitory substances may be present in the sample (Lantz et al., 1996), such as hemoglobin, anticoagulants, and polyketides in blood. These inhibitory substances can affect the efficiency and accuracy of the amplification reaction. Therefore, it is necessary to use appropriate methods to remove these inhibitory substances during sample pretreatment, such as using special extraction kits, purification columns, or other removal methods. The impact of portable devices on nucleic acid amplification technology has been significant, allowing for the amplification of nucleic acids outside of the laboratory, providing easier, faster and more flexible applications (Wang et al., 2020; Chen et al., 2023).
Pathogens can cause serious diseases in humans and animals, and detection technology based on nucleic acid amplification plays an important role in pathogen detection. PCR is still the most mature technology in detection, and isothermal nucleic acid amplification technology is becoming more mature in microbial detection, which can compensate for PCR’s dependence on application scenarios and instrumentation, and has the characteristics of rapid, sensitive and convenient detection, and is especially suitable for rapid on-site detection in remote areas (Gill and Ghaemi, 2008). It is the main direction of future development in the field of pathogen nucleic acid detection (Deng and Gao, 2015; Jiang et al., 2023). However, compared with traditional PCR, primer design in isothermal nucleic acid amplification is complicated and difficult, lacks appropriate design software, requires screening and optimization of reasonable primers (Mayboroda et al., 2018), and is more prone to false-positive results due to aerosol contamination or non-specific amplification, and there is still a certain gap between detection sensitivity and qPCR. Methods that enable rapid and on-site detection of pathogenic bacteria are a prerequisite for public health assurance, medical diagnosis, ensuring food safety and security, and research. Many current bacterial detection technologies are inconvenient and time-consuming, making them unsuitable for on-site detection. New technologies based on the CRISPR/Cas system have the potential to fill the existing detection gap (Chakraborty et al., 2022). CRISPR-based sensors for the detection of pathogens, proteins, miRNAs, etc., have a wide range of applications (Wang et al., 2022; Zhang et al., 2023). There are risks associated with CRISPR technology: it may lead to off-target effects (Cheng et al., 2020), i.e., the editing process may accidentally alter DNA sequences in non-target regions of the genome, which may lead to unintended consequences, such as genetic mutations or cytotoxicity. The immune effect triggered by the Cas protein itself is also one of the shortcomings (Sun et al., 2015). Unselected ssDNA cleavage activity of cas12a impairs targeted host cells (Paul and Montoya, 2020). Although there are still some problems with CRISPR/Cas9 gene editing technology, researchers have been committed to finding solutions, and through a series of measures such as modification of Cas protein, use of Cas direct homologous enzyme, and chemical assistance, CRISPR/Cas9 gene editing technology has improved its ability to accurately repair broken DNA, reduced its off-target rate, and weakened the target site restriction caused by PAM. target site restriction, controlling the occurrence of mosaicism to a certain extent. Furthermore, we are aware of the limitations of our CRISPR-Cas13a based assay and recognize the enhancements that would be required prior to its potential utilization in resource-limited settings as a clinical POC test. While researchers are looking for ways to make up for the shortcomings, they are also expanding the application areas of this technology (Knott and Doudna, 2018). The combination of isothermal nucleic acid amplification and CRISPR/Cas system can realize the secondary amplification of the detection signal and effectively improve the detection sensitivity, which has been applied in some applications in rapid detection, but it is mainly for a single pathogen, and needs to be combined with the multiplex nucleic acid amplification technology to improve the detection throughput and realize the multi-targeted joint detection. CRISPR/Cas technology has a very promising future in nucleic acid amplification technology. It can provide higher specificity, target selection ability, multiple amplification ability, while combining with gene editing and modification to realize real-time monitoring and detection, etc. With the continuous development and improvement of the technology, CRISPR/Cas technology is expected to bring more innovations and applications in nucleic acid amplification technology.
In conclusion, the rapid emergence of CRISPR-Cas technology for genome manipulation has been revolutionary for the life sciences. Despite the broad spectrum of applications of this technology, CRISPR molecular tools available at present face a range of limitations and challenges: e.g., reliance on DNA repair mechanisms, safety and ethical issues all need to be addressed with attention. In the future research, nucleic acid amplification technology still needs to be integrated, high-throughput, multiplexed and convenient as the research objectives, from the various technical difficulties, optimize the pre-treatment method, primer design method, product detection method and so on, in order to improve the efficiency of the detection; nucleic acid amplification technology has an important application prospect in the field of nucleic acid analysis. With the continuous development and innovation of the technology, it is believed that nucleic acid amplification technology will play a greater role in biomedical research, disease diagnosis and environmental monitoring, and bring more new breakthroughs and progress in the field of life sciences. With the continuous development and innovation of the technology, it is believed that nucleic acid amplification technology will play a greater role in biomedical research, disease diagnosis and environmental monitoring, and bring more new breakthroughs and progress in the field of life sciences.
DZ: Writing – original draft, Writing – review & editing. JJ: Writing – review & editing. TM: Writing – review & editing.
The author(s) declare financial support was received for the research, authorship, and/or publication of this article. This work is supported in part by grants from National Natural Science Foundation of China (32370092), from the National Key Research and Development Program (2023YFF1103603).
The authors declare that the research was conducted in the absence of any commercial or financial relationships that could be construed as a potential conflict of interest.
All claims expressed in this article are solely those of the authors and do not necessarily represent those of their affiliated organizations, or those of the publisher, the editors and the reviewers. Any product that may be evaluated in this article, or claim that may be made by its manufacturer, is not guaranteed or endorsed by the publisher.
Abnous, K., Danesh, N. M., Ramezani, M., Alibolandi, M., Nameghi, M. A., Zavvar, T. S., et al. (2021). A novel colorimetric aptasensor for ultrasensitive detection of aflatoxin M(1) based on the combination of Crispr-Cas12a, rolling circle amplification and catalytic activity of gold nanoparticles. Anal. Chim. Acta 1165:338549. doi: 10.1016/j.aca.2021.338549
Alnakip, M. E., Youssef, M. Z., Abd-Elaal, S. F., and Bayoumi, M. A. (2023). Screening of food-borne Staphylococcus aureus and E. coli pathogens in artisanal white soft cheese in Delta region, Egypt. J. Adv. Vet. Res. 13, 1203–1209,
Anejo-Okopi, J. A., Isa, S. E., Audu, O., Fagbamila, I. O., Iornenge, J. C., and Smith, I. S. (2016). Isolation and polymerase chain reaction detection of virulence invA gene in Salmonella spp. from poultry farms in Jos, Nigeria. J. Med. Trop. 18:98. doi: 10.4103/2276-7096.192237
Bao, Y., Jiang, Y., Xiong, E., Tian, T., Zhang, Z., Lv, J., et al. (2020). Cut-Lamp: contamination-free loop-mediated isothermal amplification based on the Crispr/Cas9 cleavage. ACS Sensors 5, 1082–1091. doi: 10.1021/acssensors.0c00034
Barreda-García, S., Miranda-Castro, R., de-los-Santos-Álvarez, N., Miranda-Ordieres, A. J., and Lobo-Castañón, M. J. (2018). Helicase-dependent isothermal amplification: a novel tool in the development of molecular-based analytical systems for rapid pathogen detection. Anal. Bioanal. Chem. 410, 679–693. doi: 10.1007/s00216-017-0620-3
Becherer, L., Borst, N., Bakheit, M., Frischmann, S., Zengerle, R., and von Stetten, F. (2020). Loop-mediated isothermal amplification (Lamp)–review and classification of methods for sequence-specific detection. Anal. Methods 12, 717–746. doi: 10.1039/C9AY02246E
Bi, S., Yue, S., and Zhang, S. (2017). Hybridization chain reaction: a versatile molecular tool for biosensing, bioimaging, and biomedicine. Chem. Soc. Rev. 46, 4281–4298. doi: 10.1039/C7CS00055C
Cao, H., Mao, K., Ran, F., Xu, P., Zhao, Y., Zhang, X., et al. (2022). Paper device combining Crispr/Cas12a and reverse-transcription loop-mediated isothermal amplification for Sars-CoV-2 detection in wastewater. Environ. Sci. Technol. 56, 13245–13253. doi: 10.1021/acs.est.2c04727
Cao, Y., Tian, Y., Huang, J., Xu, L., Fan, Z., Pan, Z., et al. (2023). Crispr/Cas13-assisted carbapenem-resistant Klebsiella pneumoniae detection. J. Microbiol. Immunol. Infect. doi: 10.1016/j.jmii.2023.10.010
Casadevall, A., and Pirofski, L. A. (2002). What is a pathogen? Ann. Med. 34, 2–4. doi: 10.1080/078538902317338580
Chakraborty, J., Chaudhary, A. A., Khan, S. U., Rudayni, H. A., Rahaman, S. M., and Sarkar, H. (2022). Crispr/Cas-based biosensor as a new age detection method for pathogenic Bacteria. ACS Omega 7, 39562–39573. doi: 10.1021/acsomega.2c04513
Chen, H., Zhou, X., Wang, M., and Ren, L. (2023). Towards point of care Crispr-based diagnostics: from method to device. J. Funct. Biomater. 14:97. doi: 10.3390/jfb14020097
Chen, H., Zhuang, Z., Chen, Y., Qiu, C., Qin, Y., Tan, C., et al. (2023). A universal platform for one-pot detection of circulating non-coding RNA combining Crispr-Cas12a and branched rolling circle amplification. Anal. Chim. Acta 1246:340896. doi: 10.1016/j.aca.2023.340896
Cheng, X., Fan, S., Wen, C., and Du, X. (2020). Crispr/Cas9 for cancer treatment: technology, clinical applications and challenges. Brief. Funct. Genomics 19, 209–214. doi: 10.1093/bfgp/elaa001
Chi, Z., Wu, Y., Chen, L., Yang, H., Khan, M. R., Busquets, R., et al. (2022). Crispr-Cas14a-integrated strand displacement amplification for rapid and isothermal detection of cholangiocarcinoma associated circulating micrornas. Anal. Chim. Acta 1205:339763. doi: 10.1016/j.aca.2022.339763
Compton, J. (1991). Nucleic acid sequence-based amplification. Nature 350, 91–92. doi: 10.1038/350091a0
Daher, R. K., Stewart, G., Boissinot, M., and Bergeron, M. G. (2016). Recombinase polymerase amplification for diagnostic applications. Clin. Chem. 62, 947–958. doi: 10.1373/clinchem.2015.245829
Debayan, D., Manaswini, M., and Aashish, P. (2023). Paper-based loop mediated isothermal amplification (Lamp) platforms: integrating the versatility of paper microfluidics with accuracy of nucleic acid amplification tests. Chemosensors 11:163. doi: 10.3390/chemosensors11030163
Deng, H., and Gao, Z. (2015). Bioanalytical applications of isothermal nucleic acid amplification techniques. Anal. Chim. Acta 853, 30–45. doi: 10.1016/j.aca.2014.09.037
Dirks, R. M., and Pierce, N. A. (2004). Triggered amplification by hybridization chain reaction. Proc. Natl. Acad. Sci. U. S. A. 101, 15275–15278. doi: 10.1073/pnas.0407024101
Dueñas, E., Nakamoto, J. A., Cabrera-Sosa, L., Huaihua, P., Cruz, M., Arévalo, J., et al. (2022). Novel Crispr-based detection of Leishmania species. Front. Microbiol. 13:958693. doi: 10.3389/fmicb.2022.958693
Feng, S., Chen, H., Hu, Z., Wu, T., and Liu, Z. (2023). Ultrasensitive detection of mirna via Crispr/Cas12a coupled with Strand displacement amplification reaction. ACS Appl. Mater. Interfaces 15, 28933–28940. doi: 10.1021/acsami.3c03399
Gill, P., and Ghaemi, A. (2008). Nucleic acid isothermal amplification technologies: a review. Nucleosides Nucleotides Nucleic Acids 27, 224–243. doi: 10.1080/15257770701845204
Gong, S., Zhang, S., Wang, X., Li, J., Pan, W., Li, N., et al. (2021). Strand displacement amplification assisted Crispr-Cas12a strategy for colorimetric analysis of viral nucleic acid. Anal. Chem. 93, 15216–15223. doi: 10.1021/acs.analchem.1c04133
Guo, W., Yang, H., Zhang, Y., Wu, H., Lu, X., Tan, J., et al. (2022). A novel fluorescent Aptasensor based on real-time fluorescence and Strand displacement amplification for the detection of ochratoxin A. Foods 11:2443. doi: 10.3390/foods11162443
Hadi, J., Rapp, D., Dhawan, S., Gupta, S. K., Gupta, T. B., and Brightwell, G. (2023). Molecular detection and characterization of foodborne bacteria: recent progresses and remaining challenges. Compr. Rev. Food Sci. Food Saf. 22, 2433–2464. doi: 10.1111/1541-4337.13153
He, H., Li, R., Chen, Y., Pan, P., Tong, W., Dong, X., et al. (2017). Integrated Dna and Rna extraction using magnetic beads from viral pathogens causing acute respiratory infections. Sci. Rep. 7:45199. doi: 10.1038/srep45199
He, Z., Luo, T., Fan, F., Zhang, P., and Chen, Z. (2019). Universal identification of lethal amanitas by using Hyperbranched rolling circle amplification based on α-amanitin gene sequences. Food Chem. 298:125031. doi: 10.1016/j.foodchem.2019.125031
Hønsvall, B. K., and Robertson, L. J. (2017). From research lab to standard environmental analysis tool: will Nasba make the leap? Water Res. 109, 389–397. doi: 10.1016/j.watres.2016.11.052
Hryhorowicz, M., Lipiński, D., Zeyland, J., and Słomski, R. (2017). Crispr/Cas9 immune system as a tool for genome engineering. Arch. Immunol. Ther. Exp. 65, 233–240. doi: 10.1007/s00005-016-0427-5
Huang, M., Zhou, X., Wang, H., and Xing, D. (2018). Clustered regularly interspaced short palindromic repeats/Cas9 triggered isothermal amplification for site-specific nucleic acid detection. Anal. Chem. 90, 2193–2200. doi: 10.1021/acs.analchem.7b04542
Jaroenram, W., and Owens, L. (2014). Recombinase polymerase amplification combined with a lateral flow dipstick for discriminating between infectious Penaeus stylirostris densovirus and virus-related sequences in shrimp genome. J. Virol. Methods 208, 144–151. doi: 10.1016/j.jviromet.2014.08.006
Jeong, Y. J., Park, K., and Kim, D. E. (2009). Isothermal DNA amplification in vitro: the helicase-dependent amplification system. Cell. Mol. Life Sci. 66, 3325–3336. doi: 10.1007/s00018-009-0094-3
Jia, L., Joanne, M., and Felix, V. S. (2020). Correction: review: a comprehensive summary of a decade development of the recombinase polymerase amplification. Analyst 145, 1950–1960. doi: 10.1039/C9AN90127B
Jiang, H., Li, Y., Lv, X., Deng, Y., and Li, X. (2023). Recent advances in cascade isothermal amplification techniques for ultra-sensitive nucleic acid detection. Talanta 260:124645. doi: 10.1016/j.talanta.2023.124645
Ju, Y., Kim, H. Y., Ahn, J. K., and Park, H. G. (2021). Ultrasensitive version of nucleic acid sequence-based amplification (Nasba) utilizing a nicking and extension chain reaction system. Nanoscale 13, 10785–10791. doi: 10.1039/D1NR00564B
Ke, X., Ou, Y., Lin, Y., and Hu, T. (2022). Enhanced chemiluminescence imaging sensor for ultrasensitive detection of nucleic acids based on HCR-Crispr/Cas12a. Biosens. Bioelectron. 212:114428. doi: 10.1016/j.bios.2022.114428
Kim, U., Lee, S. Y., and Oh, S. W. (2023). Thermophilic helicase-dependent amplification-based Crispr/Cas12a system: detection of stx2 in Escherichia coli O157:H7 by controlling primer dimers. Anal. Chim. Acta 1239:340679. doi: 10.1016/j.aca.2022.340679
Knott, G. J., and Doudna, J. A. (2018). Crispr-Cas guides the future of genetic engineering. Science (New York, N.Y.) 361, 866–869. doi: 10.1126/science.aat5011
Kohl, T. O., and Ascoli, C. A. (2017). Immunometric antibody Sandwich enzyme-linked immunosorbent assay. Cold Spring Harb. Protoc. 2017:pdb.prot093716. doi: 10.1101/pdb.prot093716
Koonin, E. V., and Wolf, Y. I. (2015). Evolution of the Crispr-Cas adaptive immunity systems in prokaryotes: models and observations on virus–host coevolution. Mol. BioSyst. 11, 20–27. doi: 10.1039/C4MB00438H
Lantz, P. G., Tjerneld, F., Hahn-Hägerdal, B., and Rådström, P. (1996). Use of aqueous two-phase systems in sample preparation for polymerase chain reaction-based detection of microorganisms. J. Chromatogr. B Biomed. Appl. 680, 165–170. doi: 10.1016/0378-4347(95)00496-3
Lee, S.-Y., and Oh, S.-W. (2023). Lateral flow biosensor based on Lamp-Crispr/Cas12a for sensitive and visualized detection of Salmonella spp. Food Control 145:109494. doi: 10.1016/j.foodcont.2022.109494
Li, C., Chen, X., Wen, R., Ma, P., Gu, K., Li, C., et al. (2022). Immunocapture magnetic beads enhanced the Lamp-Crispr/Cas12a method for the sensitive, specific, and visual detection of Campylobacter jejuni. Biosensors 12:154:154. doi: 10.3390/bios12030154
Li, M., Luo, N., Liao, X., and Zou, L. (2023). Proximity hybridization-regulated Crispr/Cas12a-based dual signal amplification strategy for sensitive detection of circulating tumor Dna. Talanta 257:124395. doi: 10.1016/j.talanta.2023.124395
Li, J., Sun, L., Wu, X., Guo, Y., Jiao, W., Xiao, J., et al. (2019). Early diagnosis of Mycoplasma pneumoniae in children: simultaneous amplification and testing (sat) is the key. Front. Pediatr. 7:7. doi: 10.3389/fped.2019.00441
Li, H., Wang, X., Wei, S., Zhao, C., Song, X., Xu, K., et al. (2022). Applications of hybridization chain reaction optical detection incorporating nanomaterials: a review. Anal. Chim. Acta 1190:338930. doi: 10.1016/j.aca.2021.338930
Liu, Y., Chen, Y., Zhang, Y., Zhong, Q., Zhu, X., and Wu, Q. (2022). A functionalized magnetic nanoparticle regulated Crispr-Cas12a sensor for the ultrasensitive detection of alpha-fetoprotein. Analyst 147, 3186–3192. doi: 10.1039/d2an00697a
Liu, G., Lin, Q., Jin, S., and Gao, C. (2022). The Crispr-Cas toolbox and gene editing technologies. Mol. Cell 82, 333–347. doi: 10.1016/j.molcel.2021.12.002
Liu, H., Wang, J., Zeng, H., Liu, X., Jiang, W., Wang, Y., et al. (2021). Rpa-Cas12a-fs: a frontline nucleic acid rapid detection system for food safety based on Crispr-Cas12a combined with recombinase polymerase amplification. Food Chem. 334:127608. doi: 10.1016/j.foodchem.2020.127608
Liu, S., Xie, T., Pei, X., Li, S., He, Y., Tong, Y., et al. (2023). Crispr-Cas12a coupled with universal gold nanoparticle strand-displacement probe for rapid and sensitive visual Sars-CoV-2 detection. Sensors Actuators B Chem. 377:133009. doi: 10.1016/j.snb.2022.133009
Ma, X., Suo, T., Zhao, F., Shang, Z., Chen, Y., Wang, P., et al. (2023). Integrating Crispr/Cas12a with strand displacement amplification for the ultrasensitive aptasensing of cadmium(ii). Anal. Bioanal. Chem. 415, 2281–2289. doi: 10.1007/s00216-023-04650-6
Malorny, B., Tassios, P. T., Rådström, P., Cook, N., Wagner, M., and Hoorfar, J. (2003). Standardization of diagnostic Pcr for the detection of foodborne pathogens. Int. J. Food Microbiol. 83, 39–48. doi: 10.1016/S0168-1605(02)00322-7
Markoulatos, P., Siafakas, N., and Moncany, M. (2002). Multiplex polymerase chain reaction: a practical approach. J. Clin. Lab. Anal. 16, 47–51. doi: 10.1002/jcla.2058
Mayboroda, O., Katakis, I., and O'sullivan, C. K. (2018). Multiplexed isothermal nucleic acid amplification. Anal. Biochem. 545, 20–30. doi: 10.1016/j.ab.2018.01.005
Meng, F., Yin, Z., Lu, F., Wang, W., and Zhang, H. (2023). Disruption of LPA-LPAR1 pathway results in lung tumor growth inhibition by downregulating B7-H3 expression in fibroblasts. Thorac. Cancer. 1–11. doi: 10.1111/1759-7714.15193
Mok, E., Wee, E., Wang, Y., and Trau, M. (2016). Comprehensive evaluation of molecular enhancers of the isothermal exponential amplification reaction. Sci. Rep. 6:37837. doi: 10.1038/srep37837
Mullegama, S. V., Alberti, M. O., Au, C., Li, Y., Toy, T., Tomasian, V., et al. (2019). Nucleic acid extraction from human biological samples. Methods Mol. Biol. 1897, 359–383. doi: 10.1007/978-1-4939-8935-5_30
Munawar, M. A. (2022). Critical insight into recombinase polymerase amplification technology. Expert. Rev. Mol. Diagn. 22, 725–737. doi: 10.1080/14737159.2022.2109964
Nagamine, K., Watanabe, K., Ohtsuka, K., Hase, T., and Notomi, T. (2001). Loop-mediated isothermal amplification reaction using a nondenatured template. Clin. Chem. 47, 1742–1743. doi: 10.1093/clinchem/47.9.1742
Nilsson, M., Malmgren, H., Samiotaki, M., Kwiatkowski, M., Chowdhary, B. P., and Landegren, U. (1994). Padlock probes: circularizing oligonucleotides for localized DNA detection. Science (New York, N.Y.) 265, 2085–2088. doi: 10.1126/science.7522346
Notomi, T., Mori, Y., Tomita, N., and Kanda, H. (2015). Loop-mediated isothermal amplification (Lamp): principle, features, and future prospects. J. Microbiol. 53, 1–5. doi: 10.1007/s12275-015-4656-9
Notomi, T., Okayama, H., Masubuchi, H., Yonekawa, T., Watanabe, K., Amino, N., et al. (2000). Loop-mediated isothermal amplification of DNA. Nucleic Acids Res. 28, 63e–663e. doi: 10.1093/nar/28.12.e63
Ortiz-Cartagena, C., Fernández-García, L., Blasco, L., Pacios, O., Bleriot, I., López, M., et al. (2022). Reverse transcription-loop-mediated isothermal amplification-Crispr-Cas13a technology as a promising diagnostic tool for Sars-CoV-2. Microbiol. Spect. 10:e0239822. doi: 10.1128/spectrum.02398-22
Pardee, K., Green, A. A., Takahashi, M. K., Braff, D., Lambert, G., Lee, J. W., et al. (2016). Rapid, low-cost detection of Zika virus using programmable biomolecular components. Cell 165, 1255–1266. doi: 10.1016/j.cell.2016.04.059
Parida, M., Sannarangaiah, S., Dash, P. K., Rao, P. V. L., and Morita, K. (2008). Loop mediated isothermal amplification (Lamp): a new generation of innovative gene amplification technique; perspectives in clinical diagnosis of infectious diseases. Rev. Med. Virol. 18, 407–421. doi: 10.1002/rmv.593
Park, J. W. (2022). Principles and applications of loop-mediated isothermal amplification to point-of-care tests. Biosensors 12:857. doi: 10.3390/bios12100857
Paul, B., and Montoya, G. (2020). Crispr-Cas12a: functional overview and applications. Biom. J. 43, 8–17. doi: 10.1016/j.bj.2019.10.005
Piepenburg, O., Williams, C. H., Stemple, D. L., and Armes, N. A. (2006). DNA detection using recombination proteins. PLoS Biol. 4:e204. doi: 10.1371/journal.pbio.0040204
Qiao, Z., Xue, L., Sun, M., Zhang, M., Chen, M., Xu, X., et al. (2023). Highly sensitive detection of Salmonella based on dual-functional HCR-mediated multivalent aptamer and amplification-free Crispr/Cas12a system. Anal. Chim. Acta 1284:341998. doi: 10.1016/j.aca.2023.341998
Qing, M., Chen, S. L., Sun, Z., Fan, Y., Luo, H. Q., and Li, N. B. (2021). Universal and programmable rolling circle amplification-Crispr/Cas12a-mediated immobilization-free electrochemical biosensor. Anal. Chem. 93, 7499–7507. doi: 10.1021/acs.analchem.1c00805
Qiu, X. Y., Zhu, L. Y., Zhu, C. S., Ma, J. X., Hou, T., Wu, X. M., et al. (2018). Highly effective and low-cost Microrna detection with Crispr-Cas9. ACS Synth. Biol. 7, 807–813. doi: 10.1021/acssynbio.7b00446
Rakshit, S., Sunny, J. S., George, M., Hanna, L. E., Leela, K. V., and Sarkar, K. (2022). T helper cell-mediated epitranscriptomic regulation via m6A RNA methylation bridges link between coronary artery disease and invasive ductal carcinoma. J. Cancer Res. Clin. Oncol. 148, 3421–3436. doi: 10.1007/s00432-022-04130-x
Ramesh, R., Munshi, A., and Panda, S. K. (1992). Polymerase chain reaction. Natl. Med. J. India 5, 115–119
Reid, M. S., Le, X. C., and Zhang, H. (2018). Exponential isothermal amplification of nucleic acids and assays for proteins, cells, small molecules, and enzyme activities: an Expar example. Angew. Chem. Int. Ed. Engl. 57, 11856–11866. doi: 10.1002/anie.201712217
Savić, N., and Schwank, G. (2016). Advances in therapeutic Crispr/Cas9 genome editing. Transl. Res. 168, 15–21. doi: 10.1016/j.trsl.2015.09.008
Seemayer, T. A. (1990). Polymerase chain reaction. Pediatr. Pathol. 10, 311–317. doi: 10.3109/15513819009067120
Shan, H., Wang, Y., Wu, T., Ying, B., and Xu, G. (2022). Development of asymmetric hairpins-mediated nucleic acid isothermal amplification-based lateral flow detection of Mycobacterium tuberculosis. Sensors Actuators B Chem. 350:130836. doi: 10.1016/j.snb.2021.130836
Shi, K., Yi, Z., Han, Y., Chen, J., Hu, Y., Cheng, Y., et al. (2023). Pam-free cascaded strand displacement coupled with Crispr-Cas12a for amplified electrochemical detection of Sars-CoV-2 Rna. Anal. Biochem. 664:115046. doi: 10.1016/j.ab.2023.115046
Sun, W., Ji, W., Hall, J. M., Hu, Q., Wang, C., Beisel, C. L., et al. (2015). Self-assembled DNA nanoclews for the efficient delivery of Crispr-Cas9 for genome editing. Angew. Chem. Int. Ed. Engl. 54, 12029–12033. doi: 10.1002/anie.201506030
Sun, X., Wang, Y., Zhang, L., Liu, S., Zhang, M., Wang, J., et al. (2020). Crispr-Cas9 triggered two-step isothermal amplification method for E. coli O157:H7 detection based on a metal-organic framework platform. Anal. Chem. 92, 3032–3041. doi: 10.1021/acs.analchem.9b04162
Tan, M., Liao, C., Liang, L., Yi, X., Zhou, Z., and Wei, G. (2022). Recent advances in recombinase polymerase amplification: principle, advantages, disadvantages and applications. Front. Cell. Infect. Microbiol. 12:1019071. doi: 10.3389/fcimb.2022.1019071
Tian, Y., Fan, Z., Zhang, X., Xu, L., Cao, Y., Pan, Z., et al. (2023). Crispr/Cas13a-assisted accurate and portable hepatitis D virus RNA detection. Emerg. Microbes Infect. 12:2276337. doi: 10.1080/22221751.2023.2276337
Tian, Y., Liu, T., Liu, C., Xu, Q., Fang, S., Wu, Y., et al. (2021). An ultrasensitive and contamination-free on-site nucleic acid detection platform for Listeria monocytogenes based on the Crispr-Cas12a system combined with recombinase polymerase amplification. LWT 152:112166. doi: 10.1016/j.lwt.2021.112166
Tomita, N., Mori, Y., Kanda, H., and Notomi, T. (2008). Loop-mediated isothermal amplification (Lamp) of gene sequences and simple visual detection of products. Nat. Protoc. 3, 877–882. doi: 10.1038/nprot.2008.57
van der Veer, H., van Aalen, E., Michielsen, C. M. S., Hanckmann, E. T. L., Deckers, J., van Borren, M., et al. (2023). Glow-in-the-dark infectious disease diagnostics using Crispr-Cas9-based Split luciferase complementation. ACS Cent. Sci. 9, 657–667. doi: 10.1021/acscentsci.2c01467
Vincent, M., Xu, Y., and Kong, H. (2004). Helicase-dependent isothermal DNA amplification. EMBO Rep. 5, 795–800. doi: 10.1038/sj.embor.7400200
Walker, G. T., Fraiser, M. S., Schram, J. L., Little, M. C., Nadeau, J. G., and Malinowski, D. P. (1992). Strand displacement amplification--an isothermal, in vitro DNA amplification technique. Nucleic Acids Res. 20, 1691–1696. doi: 10.1093/nar/20.7.1691
Wang, W., Liu, J., Wu, L. A., Ko, C. N., Wang, X., Lin, C., et al. (2022). Nicking enzyme-free strand displacement amplification-assisted Crispr-Cas-based colorimetric detection of prostate-specific antigen in serum samples. Anal. Chim. Acta 1195:339479. doi: 10.1016/j.aca.2022.339479
Wang, Y., Peng, C., Ding, L., Su, Z., Chen, X., Wang, X., et al. (2023). An accurate, rapid and cost-effective method for T-nos detection based on Crispr/Cas12a. Foods 12:615. doi: 10.3390/foods12030615
Wang, R., Qian, C., Pang, Y., Li, M., Yang, Y., Ma, H., et al. (2021). Opvcrispr: one-pot visual Rt-Lamp-Crispr platform for Sars-cov-2 detection. Biosens. Bioelectron. 172:112766. doi: 10.1016/j.bios.2020.112766
Wang, X., Qin, Y., Huang, Y., Hu, K., Zhao, S., and Tian, J. (2022). A sensitive and facile microrna detection based on Crispr-Cas12a coupled with strand displacement amplification. Spectrochim. Acta A Mol. Biomol. Spectrosc. 279:121476. doi: 10.1016/j.saa.2022.121476
Wang, K., Wang, Z., Zeng, H., Luo, X., and Yang, T. (2020). Advances in portable visual detection of pathogenic Bacteria. ACS Appl. Biomater. 3, 7291–7305. doi: 10.1021/acsabm.0c00984
Wang, J., Yang, X., Wang, X., and Wang, W. (2022). Recent advances in Crispr/Cas-based biosensors for protein detection. Bioengineering (Basel) 9:512. doi: 10.3390/bioengineering9100512
Wang, Y., Yang, M., Xia, Y., Yan, J., Zou, J., and Zhang, D. (2021). Application and evaluation of nucleic acid sequence-based amplification, PCR and cryptococcal antigen test for diagnosis of cryptococcosis. BMC Infect. Dis. 21:1020. doi: 10.1186/s12879-021-06678-4
Xing, S., Lu, Z., Huang, Q., Li, H., Wang, Y., Lai, Y., et al. (2020). An ultrasensitive hybridization chain reaction-amplified Crispr-Cas12a aptasensor for extracellular vesicle surface protein quantification. Theranostics 10, 10262–10273. doi: 10.7150/thno.49047
Xiong, E., Jiang, L., Tian, T., Hu, M., Yue, H., Huang, M., et al. (2021). Simultaneous dual-gene diagnosis of Sars-CoV-2 based on Crispr/Cas9-mediated lateral flow assay. Angew. Chem. Int. Ed. Engl. 60, 5307–5315. doi: 10.1002/anie.202014506
Yang, Y., Liu, J., and Zhou, X. (2021). A Crispr-based and post-amplification coupled Sars-CoV-2 detection with a portable evanescent wave biosensor. Biosens. Bioelectron. 190:113418. doi: 10.1016/j.bios.2021.113418
Yang, H., Yang, S., Xia, X., Deng, R., Gao, H., and Dong, Y. (2022). Sensitive detection of a single-nucleotide polymorphism in foodborne pathogens using Crispr/Cas12a-signaling Arms-Pcr. J. Agric. Food Chem. 70, 8451–8457. doi: 10.1021/acs.jafc.2c03304
Ye, X., Li, Y., Wang, L., Fang, X., and Kong, J. (2018). A novel exonuclease-assisted isothermal nucleic acid amplification with ultrahigh specificity mediated by full-length Bst Dna polymerase. Chem. Commun. (Camb.) 54, 10562–10565. doi: 10.1039/c8cc04577a
Yu, D., Liang, B., Xu, H., Chen, L., Ye, Z., Wu, Z., et al. (2023). Crispr/Cas12a-based assay for the rapid and high-sensitivity detection of Streptococcus agalactiae colonization in pregnant women with premature rupture of membrane. Ann. Clin. Microbiol. Antimicrob. 22:8. doi: 10.1186/s12941-023-00558-2
Zhang, C., Chen, J., Sun, R., Huang, Z., Luo, Z., Zhou, C., et al. (2020). The recent development of hybridization chain reaction strategies in biosensors. ACS Sensors 5, 2977–3000. doi: 10.1021/acssensors.0c01453
Zhang, A., Sun, B., Zhang, J., Cheng, C., Zhou, J., Niu, F., et al. (2022). Crispr/Cas12a coupled with recombinase polymerase amplification for sensitive and specific detection of Aphelenchoides besseyi. Front. Bioeng. Biotechnol. 10:912959. doi: 10.3389/fbioe.2022.912959
Zhang, X., Tian, Y., Xu, L., Fan, Z., Cao, Y., Ma, Y., et al. (2022). Crispr/Cas13-assisted hepatitis B virus covalently closed circular DNA detection. Hepatol. Int. 16, 306–315. doi: 10.1007/s12072-022-10311-0
Zhang, M., Wang, H., Wang, H., Wang, F., and Li, Z. (2021). Crispr/Cas12a-assisted ligation-initiated loop-mediated isothermal amplification (Cal-Lamp) for highly specific detection of micrornas. Anal. Chem. 93, 7942–7948. doi: 10.1021/acs.analchem.1c00686
Zhang, W., Xu, L., Liu, Q., Cao, Y., Yang, K., Song, X., et al. (2021). Enzymatic recombinase amplification coupled with Crispr-Cas12a for ultrasensitive, rapid, and specific porcine circovirus 3 detection. Mol. Cell. Probes 59:101763. doi: 10.1016/j.mcp.2021.101763
Zhang, Q., Zhang, X., Zou, X., Ma, F., and Zhang, C. Y. (2023). Crispr/Cas-based Microrna biosensors. Chemistry 29:e202203412. doi: 10.1002/chem.202203412
Zhang, Z., Zhao, S., Jiang, L., Wu, J., Zhao, W., Guo, X., et al. (2022). A sample-to-answer Dna detection microfluidic system integrating sample pretreatment and smartphone-readable gradient plasmonic photothermal continuous-flow Pcr. Analyst 147, 4876–4887. doi: 10.1039/D2AN00908K
Zhou, W., Hu, L., Ying, L., Zhao, Z., Chu, P. K., and Yu, X. F. (2018). A Crispr-Cas9-triggered strand displacement amplification method for ultrasensitive Dna detection. Nat. Commun. 9:5012. doi: 10.1038/s41467-018-07324-5
Zhou, M., Wang, H., Li, C., Yan, C., Qin, P., and Huang, L. (2022). Crispr/Cas9 mediated triple signal amplification platform for high selective and sensitive detection of single base mutations. Anal. Chim. Acta 1230:340421. doi: 10.1016/j.aca.2022.340421
Zhou, H., Xu, Z., He, L., Wang, Z., Zhang, T., Hu, T., et al. (2023). Coupling Crispr/Cas12a and recombinase polymerase amplification on a stand-alone microfluidics platform for fast and parallel nucleic acid detection. Anal. Chem. 95, 3379–3389. doi: 10.1021/acs.analchem.2c04713
Zhou, J., Yin, L., Dong, Y., Peng, L., Liu, G., Man, S., et al. (2020). Crispr-Cas13a based bacterial detection platform: sensing pathogen Staphylococcus aureus in food samples. Anal. Chim. Acta 1127, 225–233. doi: 10.1016/j.aca.2020.06.041
Zhou, R., Zeng, Z., Sun, R., Liu, W., Zhu, Q., Zhang, X., et al. (2021). Traditional and new applications of the Hcr in biosensing and biomedicine. Analyst 146, 7087–7103. doi: 10.1039/D1AN01371H
Keywords: nucleic acid amplification, CRISPR/Cas, rapid detection, pathogen detection, specific
Citation: Zeng D, Jiao J and Mo T (2024) Combination of nucleic acid amplification and CRISPR/Cas technology in pathogen detection. Front. Microbiol. 15:1355234. doi: 10.3389/fmicb.2024.1355234
Received: 14 December 2023; Accepted: 16 January 2024;
Published: 06 February 2024.
Edited by:
Jens Andre Hammerl, Bundesinstitut für Risikobewertung, GermanyReviewed by:
Sinem Oktem Okullu, Acibadem Mehmet Ali Aydinlar University, TürkiyeCopyright © 2024 Zeng, Jiao and Mo. This is an open-access article distributed under the terms of the Creative Commons Attribution License (CC BY). The use, distribution or reproduction in other forums is permitted, provided the original author(s) and the copyright owner(s) are credited and that the original publication in this journal is cited, in accordance with accepted academic practice. No use, distribution or reproduction is permitted which does not comply with these terms.
*Correspondence: Tianlu Mo, bW90aWFubHVAdXNzdC5lZHUuY24=
Disclaimer: All claims expressed in this article are solely those of the authors and do not necessarily represent those of their affiliated organizations, or those of the publisher, the editors and the reviewers. Any product that may be evaluated in this article or claim that may be made by its manufacturer is not guaranteed or endorsed by the publisher.
Research integrity at Frontiers
Learn more about the work of our research integrity team to safeguard the quality of each article we publish.