- 1School of Life Science, Ludong University, Yantai, China
- 2Key Laboratory of Marine Biotechnology in Universities of Shandong, Ludong University, Yantai, China
The aim was to determine the response of a bloom-forming Microcystis aeruginosa to climatic changes. Cultures of M. aeruginosa FACHB 905 were grown at two temperatures (25°C, 30°C) and exposed to high photosynthetically active radiation (PAR: 400–700 nm) alone or combined with UVR (PAR + UVR: 295–700 nm) for specified times. It was found that increased temperature enhanced M. aeruginosa sensitivity to both PAR and PAR + UVR as shown by reduced PSII quantum yields (Fv/Fm) in comparison with that at growth temperature (25°C), the presence of UVR significantly exacerbated the photoinhibition. M. aeruginosa cells grown at high temperature exhibited lower PSII repair rate (Krec) and sustained nonphotochemical quenching (NPQs) induction during the radiation exposure, particularly for PAR + UVR. Although high temperature alone or worked with UVR induced higher SOD and CAT activity and promoted the removal rate of PsbA, it seemed not enough to prevent the damage effect from them showing by the increased value of photoinactivation rate constant (Kpi). In addition, the energetic cost of microcystin synthesis at high temperature probably led to reduced materials and energy available for PsbA turnover, thus may partly account for the lower Krec and the declination of photosynthetic activity in cells following PAR and PAR + UVR exposure. Our findings suggest that increased temperature modulates the sensitivity of M. aeruginosa to UVR by affecting the PSII repair and defense capacity, thus influencing competitiveness and abundance in the future water environment.
1 Introduction
With the process of modern industrialization, nitrogen and other elements such as phosphorus and potassium are increasingly enriched in oceans, lakes and rivers. This enrichment leads to large-scale reproduction of certain species of cyanobacteria in water bodies, resulting in blooms that threaten the quality and sustainability of aquatic ecosystems (He et al., 2016; Zhou et al., 2023). Meanwhile, as a result of the increase in CO2 concentration and the depletion of the ozone layer, there has been a gradual rise in both air temperature and ground-level UV radiation, especially UV-B (280–315 nm) (Häder and Barnes, 2019; Williamson et al., 2019). It shows that the global surface temperature increased by 1.1°C in 2011–2020 compared to 1850–1900 with the increasing emissions of greenhouse gas (IPCC, 2023). Increasing temperature may stimulate the development of cyanobacterial blooms by prolonging the growing season, increasing stability of water column and enhancing the growth rates (Visser et al., 2016; Wang et al., 2020; Ma and Wang, 2021). UV radiation usually has negative effects on photosynthesis and growth of cyanobacteria (Rastogi et al., 2014; El-Sheekh et al., 2021). Freshwater ecosystems, particularly in low and mid latitudes, are more likely to be influenced by alterations in UV radiation, as global warming and release of trace gases may reduce the barrier properties of the ozone layer (Williamson et al., 2019). Thus, the combined influence of UV radiation and temperature on algae, particularly cyanobacteria that form blooms on water surfaces, is of significant interest.
Microcystis aeruginosa is a bloom-forming cyanobacterium found throughout the world (Huo et al., 2021; Zhang et al., 2023), with several strains producing toxins known as microcystins (MCs) that can lead to poisoning of both humans and livestock. M. aeruginosa grows optimally at temperatures between 20 and 25°C (Tan, 2011; Yang et al., 2020; Ji et al., 2021; Guo et al., 2023) and changes in temperature can affect growth and photosynthesis either positively or negatively (Ma et al., 2015; Islam and Beardall, 2020; Zheng et al., 2020; Guo et al., 2023). For example, Zheng et al. found that high temperature at 30 and 35°C in contrast to at 25°C reduced the photosynthetic pigment content and PSII efficiency by increasing reactive oxygen species (ROS) level, thus resulted in growth inhibition in M. aeruginosa FACHB 912 (Zheng et al., 2020), similar low growth rate at 30°C was also shown in M. aeruginosa CS558 (Islam and Beardall, 2020), in contrast, rapid cell density accumulation from 26°C to 35°C was reported in M. aeruginosa FACHB 1343 (Guo et al., 2023), and temperature ≥ 25°C promoted the growth of both strains of M. aeruginosa FACHB905 and 419 (Ma et al., 2015). As for UV radiation, its negative influences on M. aeruginosa has been demonstrated by numerous studies. UV radiation inhibits the photosynthetic activity (Jiang and Qiu, 2011; Yang et al., 2015), regulates the microcystin synthesis (Ding et al., 2013) and decreases the pigments content, growth rate and abundance of M. aeruginosa (Yang et al., 2014; Hernando et al., 2018; De la Rosa et al., 2021).
There have been several recent studies on interactions between these factors. For example, it is reported that UV-B radiation and high temperature led to lower growth rate and photosynthetic pigments content in M. aeruginosa, while the activities of antioxidative enzymes were enhanced and production of photoprotective mycosporine-like amino acids (MAAs) was induced (Babele et al., 2017; Cordeiro-Araύjo et al., 2022); in addition, polyunsaturated fatty acids (PUFAs) (especially ω6) are involved in adaptations to increased temperature and UVR stress (De la Rosa et al., 2021). In contrast, raised temperatures have also been reported to counteract the negative influence of UV radiation on growth and photosynthesis in M. aeruginosa (Islam and Beardall, 2020; Noyma et al., 2021). Although these results are varied depending on the strain selection or the UV radiation treatments design, the mechanisms responsible for these changes in sensitivity to UV radiation with temperature elevation are not known.
Here, the photosynthetic performance of M. aeruginosa under varying temperature and photosynthetically active radiation (PAR) and PAR + UVR conditions were investigated, together with the turnover of PsbA subunits, antioxidant functions, dissipation of excess energy dissipation, and MCs production. The objective was to determine the effects of temperature increases on the UVR sensitivity of M. aeruginosa, with specific reference to PSII repair and the defense response.
2 Materials and methods
2.1 Culture conditions
The toxic FACHB 905 strain of the unicellular cyanobacterium M. aeruginosa was obtained from the Institute of Hydrobiology, Chinese Academy of Sciences, and grown in BG-11 medium at 25°C with a 40 μmol m−2 s−1 (8.2 Wm−2) PAR in a photoperiod 12 L:12D in a temperature- and light-controlled incubator (GXZ-280B, Ningbo Jiangnan Instrument, China). According to the summer high temperature at Lake Taihu, China surface water (more than 30°C) (Jiang et al., 2009; Zheng et al., 2020), the culture temperature was set at 25°C for control temperature and 30°C for higher temperature group. The organisms were, respectively, pre-adapted for 7 days at two temperature conditions prior to the radiation treatments. Cultures in the exponential growth phase were used for all experiments.
2.2 Radiation treatments
Exponentially growing M. aeruginosa cultures were placed in UV-transparent quartz tubes (inside diameter, 6.4 cm; length, 20 cm) with a concentration of about 5× 106 cells mL−1 and growth in a temperature-controlled (25 ± 1°C or 30 ± 1°C) flow-through water baths. To assess PSII damage, an inhibitor of chloroplast protein synthesis, lincomycin, was introduced into one of the tubes, with a final concentration of 500 μg mL−1. The cultures were grown under aeration (0.3 L min−1) with simultaneous exposure to two irradiation treatments, namely, (1) PAR (400–700 nm), with covering of the quartz tubes by Ultraphan film 395 (UV Opak, Digefra, Munich, Germany) and (2) PAR + UVR (295–700 nm), with covering of the tubes by Ultraphan film 295. Radiation was generated by a solar simulator (Sol 1,200, Dr. Hönle GmbH, Germany) with a 1,000-W xenon arc lamp, and irradiance levels were monitored using a radiometer (PMA 2100, Solar light, USA), using 40.8 Wm−2 PAR (200 μmol m−2 s−1) and 5.0 Wm−2 UVR, as described (Xu et al., 2019). Cultures were exposed to PAR or PAR + UVR for 90 min and then shifted to growth light of 40 μmol m−2 s−1 of PAR for 1 h recovery. The cultures were sampled at intervals of 30 min to analyze antioxidant functions, chlorophyll fluorescence, and protein levels using western blotting.
2.3 Measurement of chlorophyll fluorescence
A pulse amplitude-modulated fluorometer (WATER-ED PAM, Walz, Germany) was used for measuring chlorophyll fluorescence. The PSII photochemical efficiency (Fv/Fm) was determined using the minimal (F0) and maximal (Fm) fluorescence yields of cells that had been adapted to the dark for 10 min, using the formula Fv = (Fm–F0). For determination of F0 and Fm, low irradiance with 0.5-s pulses of saturating light at <0.1 μmol m−2 s−1 and 4,000 μmol m−2 s−1, respectively, were used. A sustained NPQ phase, indicating the inducible increased relaxation time as a fraction of NPQ, persisted following the 10-min period in the dark (Wu et al., 2012). This was defined as NPQs = (Fmt0–Fm)/Fm, where Fmt0 indicates the Fm value of samples at time zero prior to radiation exposure, with Fm determined at each specific time point.
Rate constants for photoinactivation (Kpi, s−1) and PSII repair (Krec, s−1) were obtained by fitting a single-phase exponential decay curve to the Fv/Fm versus cumulative photon plot generated during radiation exposure processing of lincomycin-treated samples. Krec was determined from non-lincomycin treated samples using the Kok equation (Kok, 1956; Campbell and Tyystjärvi, 2012).
2.4 Immunoblotting for PsbA protein
Samples (20 mL) were vacuum-filtered onto glass-fiber filters (GF/F, diameter, 25 mm; Whatman), frozen in liquid nitrogen, and kept at −80°C before analysis. The total protein was quantified by the Lowry method using a kit (Bio-Rad) before probing for PsbA. Samples of total protein (1 μg) were separated on 6–12% SDS-PAGE gels (60 min, 200 V) before transfer to PVDF membranes which were probed with a primary antibody recognizing the C-terminal region of PsbA (Agrisera, 1:50,000) (Xu et al., 2019) and an HRP-coupled anti-rabbit secondary antibody. Tanno Gis Analyzer software (Tanno 5,200, China) was used for scanning and analysis of the blots. The removal rate of PsbA (KPsbA, s−1) was determined as described (Gao et al., 2018a,b).
2.5 Determination of catalase (CAT) and superoxide dismutase (SOD) activities
Twenty milliliters of sample were collected onto a polycarbonate membrane (0.22 μm, Whatman) and rinsed with phosphate buffer (pH 7.6). Proteins were extracted in 600 μL of the same buffer containing 1 mM EDTA, 50 mM KH2PO4, and 1% (w/v) polyvinylpolypyrrolidone followed by centrifugation (12,000 × g, 10 min, 4°C). CAT activity was determined using a kit (Nanjing Jiancheng Biological Engineering Company, China) by examining the initial disappearance rate of hydrogen peroxide (H2O2) at 240 nm (Li et al., 2015). SOD was also measured using a kit (Nanjing Jiancheng Biological Engineering Company, China) with the enzyme concentration causing 50% dismutation of the superoxide radical corresponding to one unit (De la Rosa et al., 2021).
2.6 Microcystins (MCs) measurement
The samples were collected and centrifuged (10,000 × g, 10 min, 4°C). The pellets were resuspended in PBS and subjected to freezing and thawing (liquid nitrogen and 4°C, respectively) to induce cell breakage. After re-centrifugation (10,000 × g, 10 min, 4°C), the supernatants were diluted with Milli-Q water and the MCs contents measured by ELISA with a QuantiPlate™ Kit for Microcystin (EnviroLogix, USA), following the provided directions, reading absorbances at 450 nm with a multimode reader (BioTek, USA). The validation of ELISA has been demonstrated to exhibit a high correlation of over 99% with high-performance liquid chromatography, as evidenced by Sheng et al. (2007).
2.7 Statistical analysis
SPSS v. 22.0 was used for data analysis. The effects of light exposure times on Fv/Fm, NPQs, PsbA and CAT and SOD activities were evaluated by ANOVAs (RM-ANOVAs), while the effects of temperature, UVR, and lincomycin were examined using multivariate analysis of variance (MANOVA), followed by Tukey’s HSD tests. Two-way ANOVAs were used for assessing the influence of temperature and UVR on KPsbA, Kpi and Krec, while one-way ANOVAs were used to assessing MCs differences. p < 0.05 was considered statistically significant.
3 Results
When M. aeruginosa cells cultured at different temperature were treated with PAR and PAR + UVR, the PSII photochemical yield (Fv/Fm) decreased in correspondence with the duration of exposure (p < 0.05) (Figure 1). After 90 min, the Fv/Fm of the cells growing at 25°C decreased to about 90.5 and 73.6% of the initial values for the PAR and PAR + UVR treatments, respectively (Figure 1A), while at 30°C, the reduction in the Fv/Fm value was larger, being 80.0% for PAR and 52.0% for PAR + UVR (Figure 1B). Cells exposed to lincomycin showed more marked decreases in Fv/Fm over the 90-min period at both temperatures (p < 0.05), especially in the case of UVR treatment where Fv/Fm was reduced to 26.3% at 25°C and 16.0% at 30°C of the initial values. Following transfer to low-light conditions for recovery, the Fv/Fm returned to 88.2–94.9% of the time-0 value at both temperatures when lincomycin was absent (p < 0.05). After UVR treatment, cells recovered more slowly, with values of 86.3% at 25°C and 71.7% at 30°C. The cells treated with lincomycin, however, did not recover (Figure 1).
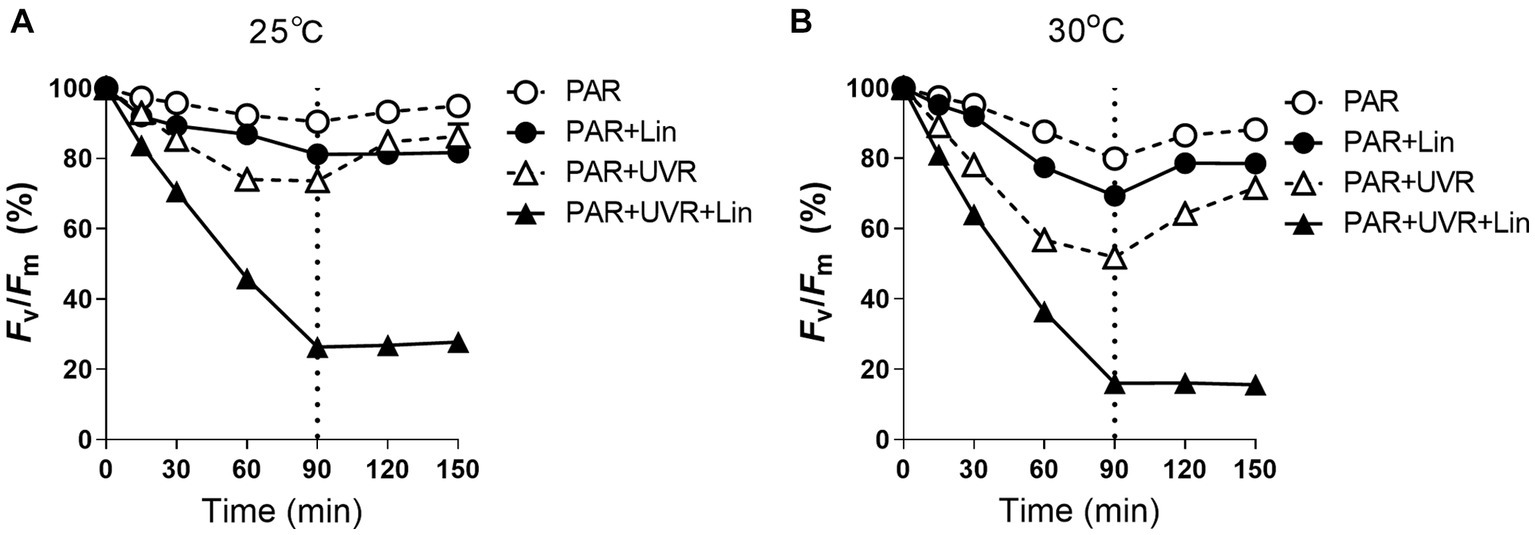
Figure 1. The maximum photochemical yield (Fv/Fm) changes in Microcystis aeruginosa FACHB 905 treated without or with lincomycin (+Lin). Cultures grown at 25°C (A) or 30°C (B) were exposed to PAR (40.8 Wm−2) and PAR + UVR (40.8 Wm−2 + 5.0 Wm−2) for 90 min, and then shifted to PAR (8.2 Wm−2) for 60 min. The dotted line indicates the division between light exposure and recovery period. Data are the means ± SE (n = 4), most error bars within symbols.
The rate constants for PSII repair (Krec, s−1) and photoinactivation (Kpi, s−1) are shown in Table 1. The rise of temperature provoked higher Kpi, while exposure to UVR resulted in a marked increase in Kpi to approximately five times than that of PAR (p < 0.05). Interactions between temperature and UVR were more obvious in the case of Krec (p < 0.05), where higher values resulted from UVR treatment relative to those of PAR at 25°C, while only temperature or temperature combined with UVR significantly lowed the Krec at 30°C compared with that at 25°C (p < 0.05).

Table 1. The rate constant for PSII repair (Krec, s−1) and photoinactivation (Kpi, s−1) and the ratio of Krec to Kpi for various treatments.
Growth of cultures with PAR and PAR + UVR led to marked reductions in the PsbA levels as the treatment duration increased (p < 0.05) (Figure 2). After 90 min of radiation treatment, both the UVR (p < 0.05) and the temperature (p < 0.05) markedly altered the PsbA content, but there was no interaction between them (p = 0.666) (Figure 2). PsbA content decreased to 71.9 and 65.9% of the initial values after 90 min of PAR or PAR+ UVR treatment at 25°C respectively, while 57.4 and 55.4% at 30°C (p < 0.05). After culture with lincomycin, cells contained markedly lower concentrations of PsbA irrespective of temperature or UVR changes (p < 0.05) (Figure 2). However, after the restoration under growth light, the PsbA content rose to about 85% at 25°C and 76% at 30°C compared with the initial for both radiation treatments, while cells treated with lincomycin did not recover.
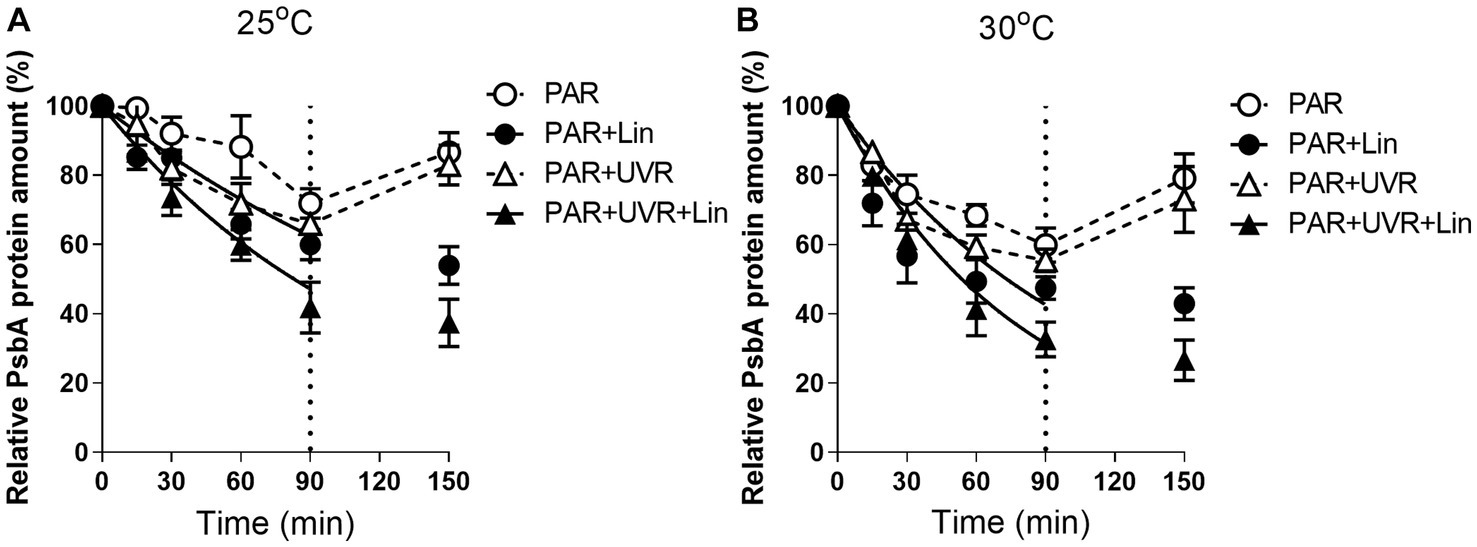
Figure 2. PsbA content changes in M. aeruginosa FACHB 905 treated without or with lincomycin (+Lin). Cultures grown at 25°C (A) or 30°C (B) were exposed to PAR (40.8 Wm−2) and PAR + UVR (40.8 Wm−2 + 5.0 Wm−2) for 90 min, and then shifted to PAR (8.2 Wm−2) for 60 min. The dotted line indicates the division between light exposure and recovery period. Data are the means ± SE (n = 4).
UVR significantly increased the rate of PsbA removal (KPsbA, s−1) in M. aeruginosa FACHB 905 (p < 0.05) at both temperatures (Figure 3), the rise of temperature alone or in combination with UVR further promoted the removal rate (p < 0.05).
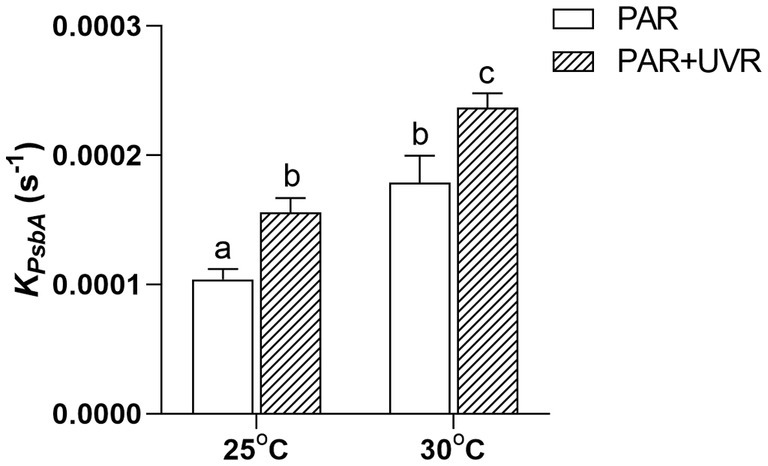
Figure 3. PsbA protein removal rate constant (KPsbA, s−1) in M. aeruginosa FACHB 905 grown at 25°C or 30°C. Data are the means ± SE (n = 4). Different letters above error bars indicate significant differences (Tukey HSD) (p < 0.05) among treatments.
NPQs was induced sharply during the radiation exposure with PAR + UVR at 25°C (p < 0.05), while only a slight increase showed under PAR exposure (Figure 4A) The rise of temperature to 30°C decreased the induction of NPQs under both PAR and PAR + UVR exposure with no significant difference between them (Figure 4B p > 0.05). After recovery, the NPQs relaxed irrespective of the radiation treatments.
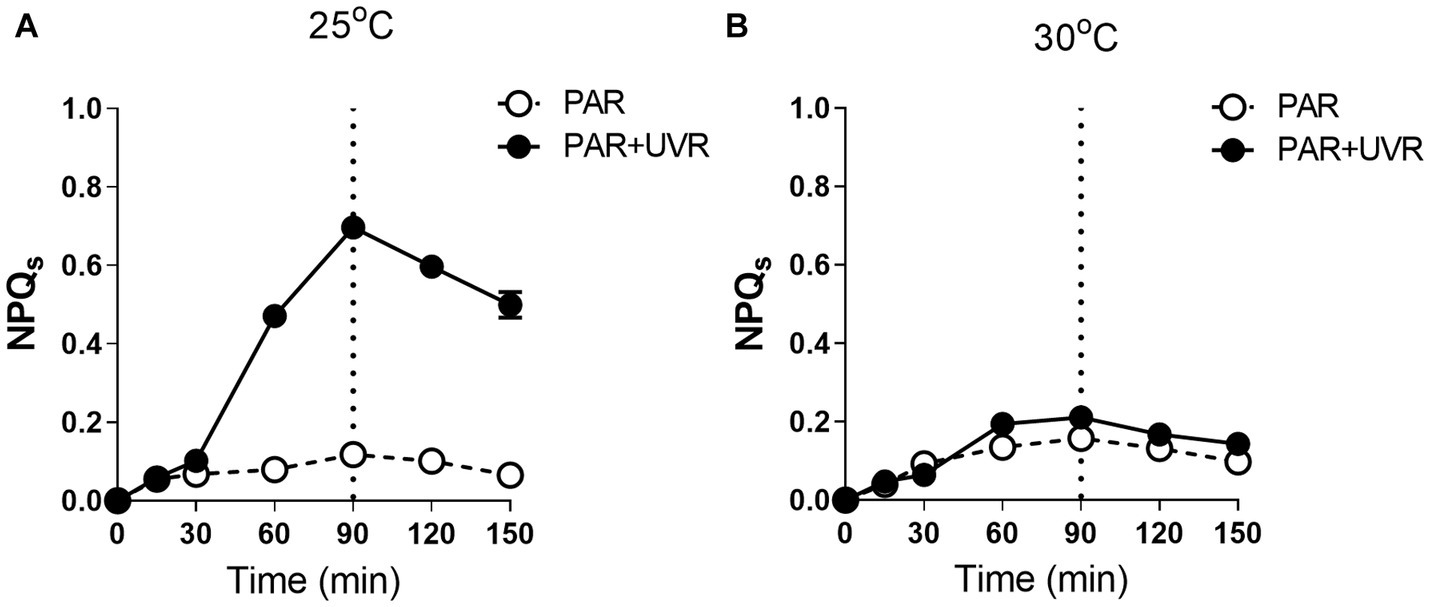
Figure 4. The sustained NPQ (NPQs) changes in M. aeruginosa FACHB 905 grown at 25°C (A) or 30°C (B). Cultures were exposed to PAR (40.8 Wm−2) and PAR + UVR (40.8 Wm−2 + 5.0 Wm−2) for 90 min, and then shifted to PAR (8.2 Wm−2) for 60 min. The dotted line indicates the division between light exposure and recovery period. Data are the means ± SE (n = 4).
Cells grown at 30°C had higher activities of both CAT and SOD before the radiation treatment than those at 25°C (Figure 5, p < 0.05). Both activities remained stable during exposure to PAR except the CAT activity in cells exposed to PAR at 30°C, which decreased to 73% of the initial value at time 0 (p < 0.05). In contrast, PAR + UVR exposure markedly elevated both SOD (p < 0.05) and CAT (p < 0.05) activities at both temperatures.
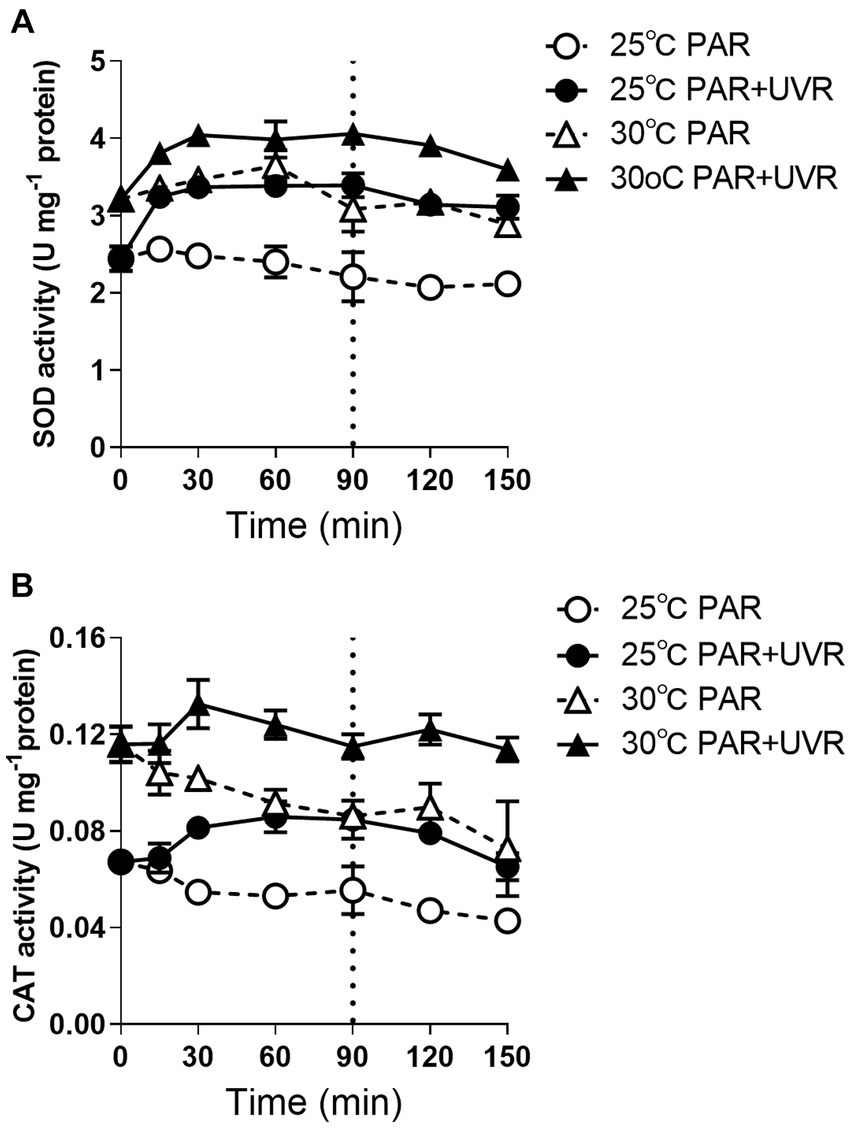
Figure 5. SOD (A) and CAT (B) activity changes in M. aeruginosa FACHB 905 grown at 25°C or 30°C. Cultures were exposed to PAR (40.8 Wm−2) and PAR + UVR (40.8 Wm−2 + 5.0 Wm−2) for 90 min, and then shifted to PAR (8.2 Wm−2) for 60 min. The dotted line indicates the division between light exposure and recovery period. Data are the means ± SE (n = 4).
MCs content increased obviously after the exposure of PAR and PAR + UVR at 25°C (Figure 6); the difference between the two was non-significant (p > 0.05). Cells grown at 30°C showed higher MCs content than those at 25°C regardless of the radiation treatments. It showed that increased temperature exhibited the main effect on MCs production (p < 0.05).
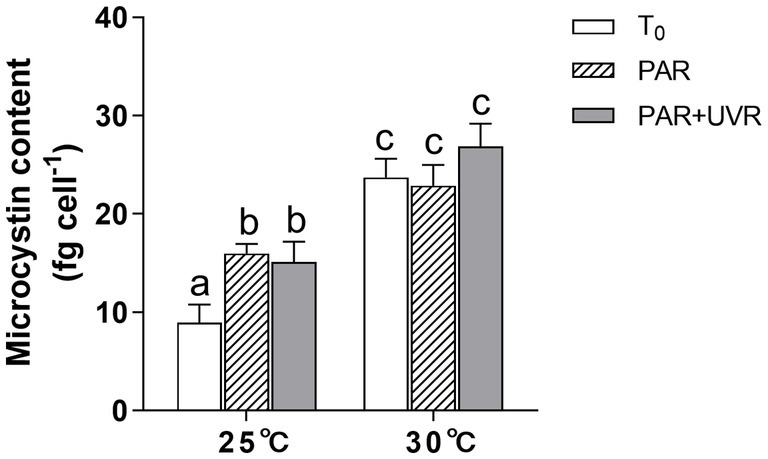
Figure 6. Changes of the microcystin content in M. aeruginosa FACHB 905 grown at 25°C or 30°C before (T0) and after 90 min-exposure with PAR (40.8 Wm−2) and PAR + UVR (40.8 Wm−2 + 5.0 Wm−2). Data are the means ± SE (n = 4).
4 Discussion
This study showed that M. aeruginosa FACHB 905 was more resistant to both PAR and PAR + UVR exposure at growth temperature (25°C) as indicated by the less inhibition on PSII quantum yield (Fv/Fm) in comparison with that at increased temperature of 30°C, as expected, the presence of UVR significantly exacerbated the photoinhibition, and the increased temperature worked synergistically with UVR leading to a further inhibition on Fv/Fm. To explore the underlying mechanism, we evaluated the alterations in PsbA content to evaluate the turnover of subunits and recovery of PSII function. The function of PSII often drops more rapidly than the content of PsbA, suggesting that the photoinactivated but intact PbsA may accumulate (Wu et al., 2011). However, here, it was found that the reductions in PSII activity coincided approximately with decreases in the PsbA levels for the samples exposed to PAR + UVR at high temperature, and in other treatments PSII function dropped slower than PsbA content. The different correspondences between PsbA levels and PSII function in M. aeruginosa FACHB 905 suggest the possibility of a regulatory adaptation (Edelman and Mattoo, 2008; Wu et al., 2011), in which radiation treatment triggers increased PsbA clearance from accumulated intact but photoinactivated PSII pools in the presence of low growth light (Gao et al., 2018a,b). This removal of PsbA from photoinactivated PSII centers was increased after exposure to UVR and elevated temperature (Figure 3), suggesting a possible protective mechanism against these forms of stress (Xu et al., 2023), as rapid clearance of defective PsbA may lead to the incorporation of fresh PsbA proteins, as has been reported in several diatoms (Zang et al., 2022; Xu et al., 2023). However, different from diatoms, cyanobacteria possess a small gene family coding for the various, functionally distinct PsbA isoforms (Mulo et al., 2012). For example, in Synechococcus PCC7942, under environmental stresses, including reduced temperature, increased light or UV-B, the expression of psbA shifts to the replacement of the PsbA:1isoform (encoded by psbA1) with PsbA:2 (encoded by psbA2 and psbA3) (Kós et al., 2008). When PSII centers contains PsbA:2, cells showed increased resistance against UV radiation (Campbell et al., 1998). This could partly contribute to the relatively higher Krec values in the presence of UVR, particularly for cells grown at 25°C. In contrast, the PsbA protein isoform exchange might not occur at high temperature in M. aeruginosa, as found in the cyanobacterium Thermosynechococcus elongates BP-1 and Synechococcus sp. PCC 7942 (Campbell et al., 1996; Kós et al., 2008), thus we assumed that the antagonistic effect between high temperature and radiation treatments on the regulation of PsbA protein isoform exchange might somehow account for the lower Krec value at 30°C. However, the turnover of PsbA isoforms in this study regardless of the treatment conditions was insufficient to compensate for the progressively declination of PSII function as shown in Figure 1.
Both temperature and UVR stress can lead to increased production of ROS, potentially generating oxidative stress. The antioxidant enzyme SOD responds rapidly by catalyzing the conversion of superoxide radicals into H2O2 that is then converted to H2O by CAT (Rastogi et al., 2014; Cho et al., 2021). In this study, cells grown at elevated temperature showed higher SOD and CAT activity than that at 25°C, and the subsequent exposure with UVR further stimulated the activity of both antioxidases, indicating their protective role in mitigating harmful effects of oxidative stress caused by UVR. Both UVR and increases in temperature are known to induce increased production of these enzymes in Anabaena sp. and M. aeruginosa CAAT 2005–3 (Singh et al., 2013; De la Rosa et al., 2021). However, the response in M. aeruginosa did not appear to be sufficient to counteract ROS entirely as there was still photosynthetic activity along with reduced PsbA. On the other hand, elevated temperature and UVR may increase the membrane peroxidation by triggering the up-regulation of ROS and destabilization of membranes (Mittler et al., 2012), thus probably affecting the formation of NPQ, since cyanobacteria possess a medium NPQ quenching component, i.e., state transition process (qT), associated with thylakoid membrane fluidity (Wang et al., 2012). It has been found that M. aeruginosa strains may have a fast NPQ component, resulting from high-light conditions, derived from conformational alterations in photosynthetic pigment proteins in the thylakoid membrane (PPPTM) (Wang et al., 2012). Thus, it is possible that UVR may alter the conformations of PPPTMs, inducing NPQ at normal growth temperatures (Xu et al., 2019), while less NPQ was formed due to the elevated temperature and together with the outweighted UVR damage effects.
MCs have been reported to act as protein-modulating metabolites and protectants, thus increasing the fitness of MCs producers under stress conditions (Zilliges et al., 2011). In this study, both PAR and PAR + UVR treatment markedly increased MCs synthesis at growth temperature of 25°C, indicating their protective role against oxidative stress. In addition, elevated temperature provoked further production of MCs, this result aligns with the previous research that the rise of culture temperature from 20°C to 30°C induced a marked elevation in the expression of one of the MCs genes, mcyB (Scherer et al., 2017). However, MCs production may be energetically costly (Kardinaal et al., 2007a,b; Lei et al., 2015), reducing the availability of resources for other functions, for example, PsbA synthesis (Xu et al., 2019), thus probably causing the lower Krec at high temperature.
5 Conclusion
In this study, changes in physiological parameters features and PSII repair in M. aeruginosa grown at higher temperatures with UV radiation were investigated. Increased temperature was observed to enhance the sensitivity of M. aeruginosa to UV radiation mainly through reducing the PSII repair rate and depressing the NPQs induction. Although elevated temperature itself or in combination with UV radiation induced higher SOD and CAT activity and promoted the removal rate of PsbA, these did not appear sufficient to counter their negative effects. In addition, high temperature may favor the preferential synthesis of MCs, or this energetically costly process might lead to the less energy and materials allocated to the synthesis of PsbA, which partly accounting for the lower Krec and the declination of photosynthetic activity of cells exposed to PAR and PAR + UVR. These findings suggest that high temperature reduced the adaptability of M. aeruginosa to UVR stress, which would affect its competitiveness and abundance in water environments of the future.
Data availability statement
The original contributions presented in the study are included in the article/supplementary material, further inquiries can be directed to the corresponding author.
Author contributions
FY: Conceptualization, Writing – original draft. ML: Conceptualization, Methodology, Writing – review & editing. SZ: Data curation, Formal analysis, Writing – review & editing. ZX: Formal analysis, Funding acquisition, Investigation, Writing – review & editing. MB: Formal analysis, Funding acquisition, Writing – review & editing. HW: Conceptualization, Funding acquisition, Project administration, Writing – review & editing.
Funding
The author(s) declare financial support was received for the research, authorship, and/or publication of this article. This work was supported by the National Natural Science Foundation of China (42376107, 42276119), the Provincial Natural Science Foundation of Shandong, China (ZR2023QD091).
Conflict of interest
The authors declare that the research was conducted in the absence of any commercial or financial relationships that could be construed as a potential conflict of interest.
Publisher’s note
All claims expressed in this article are solely those of the authors and do not necessarily represent those of their affiliated organizations, or those of the publisher, the editors and the reviewers. Any product that may be evaluated in this article, or claim that may be made by its manufacturer, is not guaranteed or endorsed by the publisher.
References
Babele, P. K., Singh, G., Singh, A., Kumar, A., Tyagi, M. B., and Sinha, R. P. (2017). UV-B radiation and temperature stress induced alterations in metabolic events and defense mechanisms in a bloom-forming cyanobacterium Microcystis aeruginosa. Acta Physiol. Plant. 39, 248–259. doi: 10.1007/s11738-017-2540-4
Campbell, D., Clarke, A. K., Gustafsson, P., and Öquist, G. (1996). D1 exchange and the photosystem II repair cycle in the cyanobacterium Synechococcus. Plant Sci. 115, 183–190. doi: 10.1016/0168-9452(96)04344-0
Campbell, D., Eriksson, M. J., Öquist, G., Gustafsson, P., and Clarke, A. K. (1998). The cyanobacterium Synechococcus resists UV-B by exchanging photosystem II reaction-center D1 proteins. Plant Biol. 95, 364–369. doi: 10.1073/pnas.95.1.364
Campbell, D. A., and Tyystjärvi, E. (2012). Parameterization of photosystem II photoinactivation and repair. BBA-Bioenergetics 1817, 258–265. doi: 10.1016/j.bbabio.2011.04.010
Cho, S. H., Jeong, Y., Hong, S. J., Lee, H., Choi, H. K., Kim, D. M., et al. (2021). Different regulatory modes of Synechocystis sp. PCC 6803 in response to photosynthesis inhibitory conditions. mSystems 6:e0094321. doi: 10.1128/mSystems.00943-21
Cordeiro-Araύjo, M., Lorenzi, A. S., Chia, M. A., Mota, E. C., and Bittencourt-Oliveira, M. C. (2022). Insights into the impact of increasing temperature, light intensity, and UV-B exposure on the circadian rhythm of microcystin production and release, and the expression of mcy genes in the cyanobacterium Microcystis aeruginosa. J. Appl. Phycol. 34, 231–242. doi: 10.1007/s10811-021-02635-5
De la Rosa, F., De Troch, M., Gabriela, M., and Marcelo, H. (2021). Physiological responses and specific fatty acids composition of Microcystis aeruginosa exposed to total solar radiation and increased temperature. Photochem. Photobiol. Sci. 20, 805–821. doi: 10.1007/s43630-021-00061-7
Ding, Y., Song, L., and Sedmak, B. (2013). UVB radiation as a potential selective factor favoring microcystin producing bloom forming cyanobacteria. PLoS One 8:e73919. doi: 10.1371/journal.pone.0073919
Edelman, M., and Mattoo, A. K. (2008). D1-protein dynamics in photosystem II: the lingering enigma. Photosynth. Res. 98, 609–620. doi: 10.1007/s11120-008-9342-x
El-Sheekh, M. M., Alwaleed, E. A., and Ibrahim, A. (2021). Detrimental effect of UV-B radiation on growth, photosynthetic pigments, metabolites and ultrastructure of some cyanobacteria and freshwater chlorophyta. Int. J. Radiat. Biol. 97, 265–275. doi: 10.1080/09553002.2021.1851060
Gao, G., Shi, Q., Xu, Z., Xu, J., Campbell, D. A., and Wu, H. Y. (2018a). Global warming interacts with ocean acidification to alter PSII function and protection in the diatom Thalassiosira weissflogii. Environ. Exp. Bot. 147, 95–103. doi: 10.1016/j.envexpbot.2017.11.014
Gao, G., Xu, Z., Shi, Q., and Wu, H. (2018b). Increased CO2 exacerbates the stress of ultraviolet radiation on photosystem II function in the diatom Thalassiosira weissflogii. Environ. Exp. Bot. 156, 96–105. doi: 10.1016/j.envexpbot.2018.08.031
Guo, Y., Meng, H., Zhao, S., Wang, Z., Zhu, L., Deng, D., et al. (2023). How does Microcystis aeruginosa respond to elevated temperature? Sci. Total Environ. 889:164277. doi: 10.1016/j.scitotenv.2023.164277
Häder, D. P., and Barnes, P. W. (2019). Comparing the impacts of climate change on the responses and linkages between terrestrial and aquatic ecosystems. Sci. Total Environ. 682, 239–246. doi: 10.1016/j.scitotenv.2019.05.024
He, X., Liu, Y. L., Conklin, A., Westrick, J., Weavers, L. K., Dionysiou, D. D., et al. (2016). Toxic cyanobacteria and drinking water: impacts, detection, and treatment. Harmful Algae 54, 174–193. doi: 10.1016/j.hal.2016.01.001
Hernando, M. P., Crettaz Minaglia, M. C., Malanga, G., Houghton, C., Andrinolo, D., Sedan, D., et al. (2018). Physiological responses and toxin production of Microcystis aeruginosa in short term exposure to solar UV radiation. Photochem. Photobiol. Sci. 17, 69–80. doi: 10.1039/c7pp00265c
Huo, D., Gan, N., Geng, R., Cao, Q., Song, L., Yu, G., et al. (2021). Cyanobacterial blooms in China: diversity, distribution, and cyanotoxins. Harmful Algae 109:102106. doi: 10.1016/j.hal.2021.102106
IPCC (2023) in Climate change 2023: Synthesis report. Contribution of working groups I, II, III to the three special reports: Global warming of 1.5°C, climate change and land, the ocean and cryosphere in a changing climate. eds. C. W. Team, R. K. Pachauri, and L. A. Meyer (Geneva, Switzerland: IPCC), 151.
Islam, M. A., and Beardall, J. (2020). Effects of temperature on the UV-B sensitivity of toxic cyanobacteria Microcystis aeruginosa CS558 and Anabaena circinalis CS537. Photochem. Photobiol. 96, 936–940. doi: 10.1111/php.13214
Ji, X., Wu, T., Xiao, J., Yang, K., Sun, Z., Yang, T., et al. (2021). Strong spring winds acceleratedthe recruitment and reinvasion of cyanobacteria. Environ. Sci. Pollut. Res. Int. 28, 16855–16866. doi: 10.1007/s11356-020-12197-7
Jiang, H., and Qiu, B. (2011). Inhibition of phtotosynthesis by UV-B exposure and its repair in the bloom-forming cyanobacterium Microcystis aeruginosa. J. Appl. Phycol. 23, 691–696. doi: 10.1007/s10811-010-9562-2
Jiang, S., Zhang, Y., Jiang, J., and Jin, Y. (2009). Research on relationship between cyanobacteria bloom and water temperature in Lake Taihu based on MODIS data. Environ. Sci. Technol. 22, 28–31 (in Chinese with English abstract).
Kardinaal, W. E. A., Janse, I., Agterveld, M. P. K., Meima, M., Snoek, J., Mur, L. R., et al. (2007a). Microcystis genotype succession in relation to microcystin concentrations in freshwater lakes. Aquat. Microb. Ecol. 48, 1–12. doi: 10.3354/ame048001
Kardinaal, W. E. A., Tonk, L., Janse, I., Hol, S., Slot, P., Huisman, J., et al. (2007b). Competition for light between toxic and nontoxic strains of the harmful cyanobacterium Microcystis. Appl. Environ. Microb. 73, 2939–2946. doi: 10.1128/AEM.02892-06
Kok, B. (1956). On the inhibition of photosynthesis by intense light. Biochim. Biophys. Acta 21, 234–244. doi: 10.1016/0006-3002(56)90003-8
Kós, P. B., Deák, Z., Cheregi, O., and Vass, I. (2008). Differential regulation of psb a and psbD gene expression, and the role of the different D1 protein copies in the cyanobacterium Thermosynechococcus elongatus BP-1. BBA-Bioenergetics 1777, 74–83. doi: 10.1016/j.bbabio.2007.10.015
Lei, L., Li, C., Peng, L., and Han, B. (2015). Competition between toxic and non-toxic Microcystis aeruginosa and its ecological implication. Ecotoxicology 24, 1411–1418. doi: 10.1007/s10646-015-1456-2
Li, W., Gao, K., and Beardall, J. (2015). Nitrate limitation and ocean acidification interact with UV-B to reduce photosynthetic performance in the diatom Phaeodactylum tricornutum. Biogeosciences 12, 2383–2393. doi: 10.5194/bg-12-2383-2015
Ma, Z., Fang, T., Thring, R. W., Li, Y., Yu, H., Zhou, Q., et al. (2015). Toxic and non-toxic strains of Microcystis aeruginosa induce temperature dependent allelopathy toward growth and photosynthesis of Chlorella vulgaris. Harmful Algae 48, 21–29. doi: 10.1016/j.hal.2015.07.002
Ma, J., and Wang, P. (2021). Effects of rising atmospheric CO2 levels on physiological response of cyanobacteria and cyanobacterial bloom development: a review. Sci. Total Environ. 754:141889. doi: 10.1016/j.scitotenv.2020.141889
Mittler, R., Finka, A., and Goloubinoff, P. (2012). How do plants feel the heat? Trends Biochem. Sci. 37, 118–125. doi: 10.1016/j.tibs.2011.11.007
Mulo, P., Sakurai, I., and Aro, E. M. (2012). Strategies for psbA gene expression in cyanobacteria, green algae and higher plants: from transcription to PSII repair. Biochim. Biophys. Acta 1817, 247–257. doi: 10.1016/j.bbabio.2011.04.011
Noyma, N. P., Mesquita, M. C. B., Roland, F., Marinho, M. M., Huszar, V. L. M., and Lürling, M. (2021). Increasing temperature counteracts the negative effect of UV radiation on growth and photosynthetic efficiency of Microcystis aeruginosa and Raphidiopsis raciborskii. Photochem. Photobiol. 97, 753–762. doi: 10.1111/php.13377
Rastogi, R. P., Sinha, R. P., Moh, S. H., Lee, T. K., Kottuparambil, S., Kim, Y. J., et al. (2014). Ultraviolet radiation and cyanobacteria. J. Photochem. Photobiol. B Biol. 141, 154–169. doi: 10.1016/j.jphotobiol.2014.09.020
Scherer, P. I., Raeder, U., Geist, J., and Zwirglmaier, K. (2017). Influence of temperature, mixing, and addition of microcystin-LR on microcystin gene expression in Microcystis aeruginosa. Microbiology 6:e00393. doi: 10.1002/mbo3.393
Sheng, J., He, M., Yu, S., Shi, H., and Qian, Y. (2007). Microcystin-LR detection based on indirect competitive enzyme-linked immunosorbent assay. Front. Envirom. Sci. Eng. China 1, 329–333. doi: 10.1007/s11783-007-0056-7
Singh, G., Babele, P. K., Sinha, R. P., Tyagi, M. B., and Kumar, A. (2013). Enzymatic and non-enzymatic defense mechanisms against ultraviolet-B radiation in two anabaena species. Process Biochem. 48, 796–802. doi: 10.1016/j.procbio.2013.04.022
Tan, X. (2011). Effects of temperature on recruitment and phytoplankton community composition. Afr. J. Microbiol. Res. 5, 5896–5901. doi: 10.5897/AJMR11.841
Visser, P. M., Verspagen, J. M. H., Sandrini, G., Stal, L. J., Matthijs, H. C. P., Davis, T. W., et al. (2016). How rising CO2 and global warming may stimulate harmful cyanobacterial blooms. Harmful Algae 54, 145–159. doi: 10.1016/j.hal.2015.12.006
Wang, Z., Dong, J., and Li, D. H. (2012). Conformational changes in photosynthetic pigment proteins on thylakoid membranes can lead to fast non-photochemical quenching in cyanobacteria. Sci. China Life Sci. 55, 726–734. doi: 10.1007/s11427-012-4360-5
Wang, P., Ma, J., Wang, X., and Tan, Q. (2020). Rising atmospheric CO2 levels result in an earlier cyanobacterial bloom-maintenance phase with higher algal biomass. Water Res. 185:116267. doi: 10.1016/j.watres.2020.116267
Williamson, C. E., Neale, P. J., Hylander, S., Rose, K. C., Figueroa, F. L., Robinson, S. A., et al. (2019). The interactive effects of stratospheric ozone depletion, UV radiation, and climate change on aquatic ecosystems. Photochem. Photobiol. Sci. 18, 717–746. doi: 10.1039/c8pp90062k
Wu, H., Cockshutt, A. M., McCarthy, A., and Campbell, D. A. (2011). Distinctive photosystem II photoinactivation and protein dynamics in marine diatoms. Plant Physiol. 156, 2184–2195. doi: 10.1104/pp.111.178772
Wu, H., Roy, S., Alami, M., Green, B. R., and Campbell, D. A. (2012). Photosystem II photoinactivation, repair, and protection in marine centric diatoms. Plant Physiol. 160, 464–476. doi: 10.1104/pp.112.203067
Xu, Z., Gao, G., Tu, B., Qiao, H., Ge, H., and Wu, H. (2019). Physiological response of the toxic and non-toxic strains of a bloom-forming cyanobacterium Microcystis aeruginosa to changing ultraviolet radiation regimes. Hydrobiologia 833, 143–156. doi: 10.1007/s10750-019-3896-9
Xu, Z., Yang, S., Li, M., Bao, M., and Wu, H. (2023). Warming modulates the photosynthetic performance of Thalassiosira pseudonana in response to UV radiation. Front. Microbiol. 14:1284792. doi: 10.3389/fmicb.2023.1284792
Yang, J., Deng, X., Xian, Q., Qian, X., and Li, A. (2014). Allelopathic effect of Microcystis aeruginosa on Microcystis wesenbergii: Microcystin-LR as a potential allelochemical. Hydrobiologia 727, 65–73. doi: 10.1007/s10750-013-1787-z
Yang, Z., Kong, F., Shi, X., Yu, Y., and Zhang, M. (2015). Effects of UV-B radiation on microcystin production of a toxic strain of Microcystis aeruginosa and its competitiveness against a non-toxic strain. J. Hazard. Mater. 283, 447–453. doi: 10.1016/j.jhazmat.2014.09.053
Yang, Z., Zhang, M., Yu, Y., and Shi, X. L. (2020). Temperature triggers the annual cycle of Microcystis, comparable results from the laboratory and a large shallow lake. Chemosphere 260:127543. doi: 10.1016/j.chemosphere.2020.127543
Zang, S. S., Xu, Z. G., Yan, F., and Wu, H. Y. (2022). Elevated CO2 modulates the physiological responses of Thalassiosira pseudonana to ultraviolet radiation. J. Photochem. Photobiol. B Biol. 236:112572. doi: 10.1016/j.jphotobiol.2022.112572
Zhang, H., Xie, Y., Zhang, R., Zhang, Z., Hu, X., Cheng, Y., et al. (2023). Discovery of a high-efficient Algicidal bacterium against Microcystis aeruginosa based on examinations toward culture strains and natural bloom samples. Toxins 15:220. doi: 10.3390/toxins15030220
Zheng, T., Zhou, M., Yang, L., Wang, Y., Wang, Y., Meng, Y., et al. (2020). Effects of high light and temperature on Microcystis aeruginosa cell growth and β-cyclocitral emission. Ecotoxicol. Environ. Saf. 192:110313. doi: 10.1016/j.ecoenv.2020.110313
Zhou, Y., Xu, J., Mac Isaac, H. J., McKay, R. M., Xu, R., Pei, Y., et al. (2023). Comparative metabolomic analysis of exudates of microcystin-producing and microcystin-free Microcystis aeruginosa strains. Front. Microbiol. 13:1075621. doi: 10.3389/fmicb.2022.1075621
Keywords: Microcystis aeruginosa, UV radiation, temperature, photosynthesis, cyanobacteria
Citation: Yan F, Li M, Zang S, Xu Z, Bao M and Wu H (2024) UV radiation and temperature increase alter the PSII function and defense mechanisms in a bloom-forming cyanobacterium Microcystis aeruginosa. Front. Microbiol. 15:1351796. doi: 10.3389/fmicb.2024.1351796
Edited by:
Feixue Fu, University of Southern California, United StatesReviewed by:
Gang Li, Chinese Academy of Sciences (CAS), ChinaYaping Wu, Jiangsu Ocean Universiity, China
Peng Jin, University of Guangzhou, China
Copyright © 2024 Yan, Li, Zang, Xu, Bao and Wu. This is an open-access article distributed under the terms of the Creative Commons Attribution License (CC BY). The use, distribution or reproduction in other forums is permitted, provided the original author(s) and the copyright owner(s) are credited and that the original publication in this journal is cited, in accordance with accepted academic practice. No use, distribution or reproduction is permitted which does not comply with these terms.
*Correspondence: Hongyan Wu, c2R3dWhvbmd5YW5AMTI2LmNvbQ==
†These authors have contributed equally to this work