- 1Faculty of Science and Technology, University of Canberra, Canberra, ACT, Australia
- 2School of Medicine, Science, Medicine and Health, University of Wollongong, Wollongong, NSW, Australia
Antimicrobial-resistant bacteria are frequently isolated from retail meat and may infect humans. To determine the diversity of antimicrobial-resistant bacteria in Australian retail meat, bacteria were cultured on selective media from raw chicken (n = 244) and pork (n = 160) meat samples obtained from all four major supermarket chains in the ACT/NSW, Australia, between March and June 2021. Antimicrobial susceptibility testing (AST) was performed for 13 critically and 4 highly important antibiotics as categorised by the World Health Organization (WHO) for a wide range of species detected in the meat samples. A total of 288 isolates underwent whole-genome sequencing (WGS) to identify the presence of antimicrobial resistance (AMR) genes, virulence genes, and plasmids. AST testing revealed that 35/288 (12%) of the isolates were found to be multidrug-resistant (MDR). Using WGS data, 232/288 (81%) of the isolates were found to harbour resistance genes for critically or highly important antibiotics. This study reveals a greater diversity of AMR genes in bacteria isolated from retail meat in Australia than previous studies have shown, emphasising the importance of monitoring AMR in not only foodborne pathogenic bacteria, but other species that are capable of transferring AMR genes to pathogenic bacteria.
Introduction
The prevalence of antimicrobial-resistant (AMR) bacteria and resistance to traditional antibiotics is increasing globally and is therefore a significant global health issue (Collignon, 2015). Antimicrobials are used to prevent and control bacterial infections in food and animal production systems; however, their overuse in the agri-food industry has expedited the spread of AMR bacteria worldwide. The use of antimicrobials in food animal production selects for AMR bacteria, which may be transmitted to humans via zoonotic bacteria in the food chain (Barlow et al., 2015). The continued prophylactic use of antimicrobials in the Australian meat industry no doubt contributes to the acquisition and maintenance of AMR (Landers et al., 2012; Kirchhelle, 2018).
European Union legislation imposed in 2022 prohibits the routine use and prophylactic use of antimicrobial medicinal products in farming, including the use of medicated feeds.1 The United States has followed a similar path; in 2019, approximately 60% of broilers were raised in no antibiotics ever (NAE) conditions.2 Australian government regulations do not go as far, as the prophylactic use of antimicrobials is still allowed. In 2015, Australia was reported to have relatively low rates of antibiotic resistance to third-generation cephalosporins, fluoroquinolones, aminoglycosides, and carbapenems (Collignon, 2015). However, a recent study showed that AMR rates are variable across Australia, with some areas showing high rates of AMR in hospital-acquired pathogens. It was estimated that 1,031 human deaths were attributed to five hospital-associated AMR pathogens in 2020 (Wozniak et al., 2022). This estimate is four times higher than an estimate provided by the OECD in 2018 (Dunachie et al., 2020).
Carbapenems are useful antibiotics because of their broad spectrum of activity and effectiveness against both Gram-positive and Gram-negative bacteria (Papp-Wallace et al., 2011). Colistin, a last resort antimicrobial, is used to treat carbapenem-resistant Enterobacteriaceae (CRE) infections in many countries; however, colistin resistance has emerged in CRE, producing conditions for which no effective antibiotic treatment is now available (antimicrobial resistance, El-Sayed Ahmed et al., 2020; WHO, 2020). Colistin is also used to treat infections caused by other MDR bacteria, including Pseudomonas aeruginosa and Acinetobacter baumannii; however, colistin resistance has emerged in these species as well. Some bacteria, such as Serratia spp., Proteus spp., and Burkholderia spp., are naturally resistant to colistin (Aghapour et al., 2019); however, they may still acquire plasmids with colistin resistance genes and therefore still participate in the spread of colistin resistance via horizontal gene transfer (Zhong et al., 2022). Very few studies have assessed the presence of colistin resistance genes in non-pathogenic species of bacteria; however, such species may act as reservoirs for colistin resistance.
Bacterial species (spp.), such as Campylobacter spp. (Habib et al., 2020), Escherichia coli (Vangchhia et al., 2018; Touchon et al., 2020; Abraham et al., 2020), Enterococcus spp. (Lee et al., 2021), and Salmonella spp. (Abraham et al., 2020), are known to be pathogenic. These species are frequently used as AMR “indicators” in surveillance studies of production animals because they are important in human disease, are relatively easy to culture and identify, and have known AMR minimum inhibitory concentrations (MIC) (Cameron and McAllister, 2016). While pathogenic bacteria typically contain AMR genes, other species of bacteria and bacteriophages are capable of transferring MGEs to pathogenic bacteria, but are often overlooked in surveillance studies because they are not pathogenic.
Many AMR studies have revealed Campylobacter spp., Escherichia spp., Salmonella spp., and Enterococcus spp. to be widespread in meat samples. E. coli is a common member of the enteric community of poultry and other birds (Blyton et al., 2015). The poultry sector has been identified as a likely source of extended-spectrum β-lactamase (ESBL)-producing Gram-negative bacteria that can infect people who consume or handle contaminated meat (Leverstein-van Hall et al., 2011). According to Overdevest et al. (2011), 80% of ESBL genes found in chickens are mostly identical to ESBL genes found in human rectal swabs, and E. coli typing confirmed the similarity between chicken and human strains, albeit using low-resolution typing methods (Kluytmans et al., 2013).
AMR bacteria are of serious concern because they pose a direct threat to humans. Screening for the presence of AMR bacteria in meat produced for human consumption, beyond the most common foodborne pathogens, may provide important information about the diversity of AMR genes and the bacteria that carry them in food-producing animals. Additionally, it is important to know the extent to which AMR genes are encoded on MGEs, as they may be transferred to pathogenic bacteria from bacteria not commonly screened in surveillance studies. The potential virulence of strains isolated from retail meat is also not commonly assessed. Therefore, the goals of this study were to isolate and identify bacterial species beyond the commonly surveyed food pathogens in Australian retail chicken and pork meat using selective media and whole-genome sequencing; to assess the extent of phenotypic AMR; and to identify MGEs and virulence genes present in the bacteria to understand their ability to disseminate AMR genes and cause disease.
Materials and methods
Sample acquisition and processing
A total of 404 meat samples (244 chicken and 160 pork) were purchased by a third-party contractor from Aldi (39 chicken, 39 pork), Coles (85 chicken, 41 pork), IGA (41 chicken, 33 pork), and Woolworths (79 chicken, 47 pork) supermarkets across 39.5/50 ACT/NSW electorates in Australia between March and June 2021. All chicken and pork meat samples available at each supermarket were purchased, provided they met the inclusion criteria: raw, unprocessed, unmarinated, unseasoned, and not labelled either “free range” or “organic.” Once purchased, all meat samples were transported, stored at 4°C, and processed within 24 h, before their expiration date. All sample packaging was disinfected with 80% ethanol before being processed aseptically in a Class II Biosafety Cabinet. Approximately 10 g of meat was taken from four locations of each sample and added to both 25 mL pre-warmed peptone buffered water and 25 mL Bolton broth (for Campylobacter isolation) and homogenised using a stomacher. Approximately 20 mL of homogenate for chicken samples obtained from a single supermarket were combined in a single tube. The same was done to combine pork samples from a single supermarket. This resulted in a total of 302 pooled samples (152 chicken, 150 pork). Of the pooled samples, 211 (70%) samples comprised a single brand product, 82 (27%) comprised two, seven (2%) comprised three, and two comprised four (1%). These pooled samples were grown in selective media.
Selection of isolates
The selective media used to grow bacteria from the meat samples included Brilliance™ ESBL agar, used for the detection of ESBL-producing bacteria; Brilliance™ CRE agar, used for the detection of carbapenem-resistant Enterobacteriaceae (CRE); Brilliance™ VRE agar, used for the detection of vancomycin-resistant enterococci (VRE); Campylobacter selective agar (CAMPY), used for the selection of Campylobacter spp.; MacConkey (MAC) agar, used for the identification and differentiation of Enterobacteriaceae spp., including E. coli; and xylose lysine deoxycholate (XLD) agar, used for the identification of Salmonella spp. A 1 mL aliquot of the PBW homogenate sample was added to selenite broth at 41°C for 18 h with shaking to select for Salmonella. Plating on XLD agar at 37°C overnight followed. A representative of each different colony, based on colony morphology and colour, was selected for each media type, regardless of whether they appeared to be a target organism for the selective agar or not. A freezer stock containing 30% glycerol was made for each isolate. Whole-genome sequencing (WGS) was performed for 288 isolates, with all isolates that grew on Brilliance™ ESBL, Brilliance™ CRE, Brilliance™ VRE, and CAMPY agar being prioritised, and the remainder being made up of isolates that grew on MAC or XLD agar. A single isolate of E. coli was randomly chosen from each electorate, despite having identified multiple different isolates of E. coli for each electorate. Due to the small number of isolates grown on XLD, MAC and XLD results are presented together.
Antimicrobial susceptibility testing
Antimicrobial sensitivity testing was performed for the 288 isolates using an automated MIC broth microdilution method and commercially prepared Gram-negative (CMV3AGNF™) and Campylobacter spp. (EUCAMP2™) Sensititre™ antibiotic plates (Thermo Scientific™). All bacterial isolates, apart from Campylobacter spp., were grown from glycerol freezer stocks on their respective agar (Brilliance™ ESBL/Brilliance™ CRE/Brilliance™ VRE, MAC, and XLD) and incubated overnight at 37°C. The Campylobacter isolates were grown on CAMPY agar and incubated at 41°C for 48 h in anaerobic jars with CampyGen sachets (Oxoid™).
After incubation, a few colonies from each agar plate were transferred to 5 mL Sensititre™ demineralised sterile water (Thermo Scientific™) to achieve a density equivalent to the 0.5 McFarland standard. A 10 μL aliquot of each 0.5 density dilution was transferred to a 5 mL Sensititre™ Mueller Hinton Broth and mixed well. A Sensititre™ 96-well plate was then inoculated with 50 μL volume per well of the suspension using the Sensititre™ AIM™ (Automated Inoculation Delivery) system. The Gram-negative CMV3AGNF™ plates were sealed and incubated at 37°C in a non-CO2 incubator for 24 h, and at 41°C for 48 h for the EUCAMP2™ plates. Following incubation, plates were placed inside a Sensititre™ Vizion™ Digital MIC viewing system, and results were recorded and interpreted using Sensititre™ SWIN™ software, based on the Clinical & Laboratory Standards Institute (CLSI) breakpoints for MIC determination.
To determine whether or not an isolate was MDR, we used the definitions as set out by Magiorakos et al. (2012). If a species was not included in this definition, then we used the same definition as a species from the same genus; if no species or genus encountered was included in their definition, then we searched the literature to determine if the genus/species was intrinsically resistant to the antibiotics tested. As with the Magiorakos et al. (2012) definition, intrinsic resistance was not taken into account.
Whole-genome sequencing and analysis
DNA from the 288 prioritised isolates was extracted from a 1 mL aliquot of an overnight broth culture using Bioline® ISOLATE II Genomic DNA Kits according to the manufacturer’s protocol. Quantification of DNA was performed using a TapeStation system (Agilent Technologies, Inc.). plexWell™ 96 Kits (seqWell™) were used for library preparation, and sequencing was performed on an Illumina® NovaSeq™ platform (Illumina®, Inc.) in a 150 bp paired-end format.
The raw paired-read data of each isolate were assembled using the St. Petersburg genome assembler (SPAdes) (Bankevich et al., 2012) tool from the Bacterial and Viral Bioinformatics Resource Center (BV-BRC) (Olson et al., 2023). The assembled sequences were annotated using the Rapid Annotations utilising Subsystems Technology (RASTtk) (Brettin et al., 2015) tool kit based on genus/species identification. Each assembled sequence was given a taxonomy-based annotation (genus or species) using the NCBI’s BLAST tool. The acquired antibiotic resistance genes, plasmids, and virulence genes were identified using the Mobile Genetic Element (MGE) finder tool from the Center for Genomic Epidemiology (CGE). The MGE tool identifies mobile genetic elements and their relation to AMR genes and virulence factors (Johansson et al., 2021). The PathogenFinder 1.1 tool, also from the CGE, was used to predict the likelihood of isolates being pathogenic to humans (Cosentino et al., 2013). Multilocus sequence typing was performed using the MLST tool from CGE, which can identify the sequence types (ST) of 66 bacterial species (Larsen et al., 2012).
Results
The breakdown of bacterial genera detected according to the selective media used for and the supermarket chain from which the meat samples were purchased for pooled chicken and pork samples is presented in Figure 1. For the pooled chicken samples, Serratia spp. were most commonly isolated (67/206, 32%), followed by E. coli (47/206, 23%), Pseudomonas spp. (29/206, 14%), and Acinetobacter spp. (13/206, 6%). For the pooled pork samples, Serratia spp. were most commonly isolated (35/82, 43%), followed by Hafnia spp. (14/82, 17%), Acinetobacter spp. (8/82, 9%), and E. coli (6/82, 7%). Overall, the 288 isolates represented 17 different genera (Table 1). A total of 41 isolates produced colonies on Brilliance™ CRE agar (30 chicken, 11 pork), 17 on Brilliance™ VRE agar (13 chicken, 4 pork), 132 on Brilliance™ ESBL agar (91 chicken, 41 pork), 7 on CAMPY agar (7 chicken, 0 pork), and 91 on MAC/XLD agar (65 chicken, 26 pork). None of the isolates that produced colonies on Brilliance™ VRE agar and were presumed to be Enterococcus, according to WGS identification, were indeed Enterococcus. All isolates from Brilliance™ VRE were Gram-negative bacteria, which vancomycin is not active against. None of the isolates that grew in selenite broth, and later on XLD, were Salmonella. All isolates from XLD belonged to the closely related genus Hafnia.
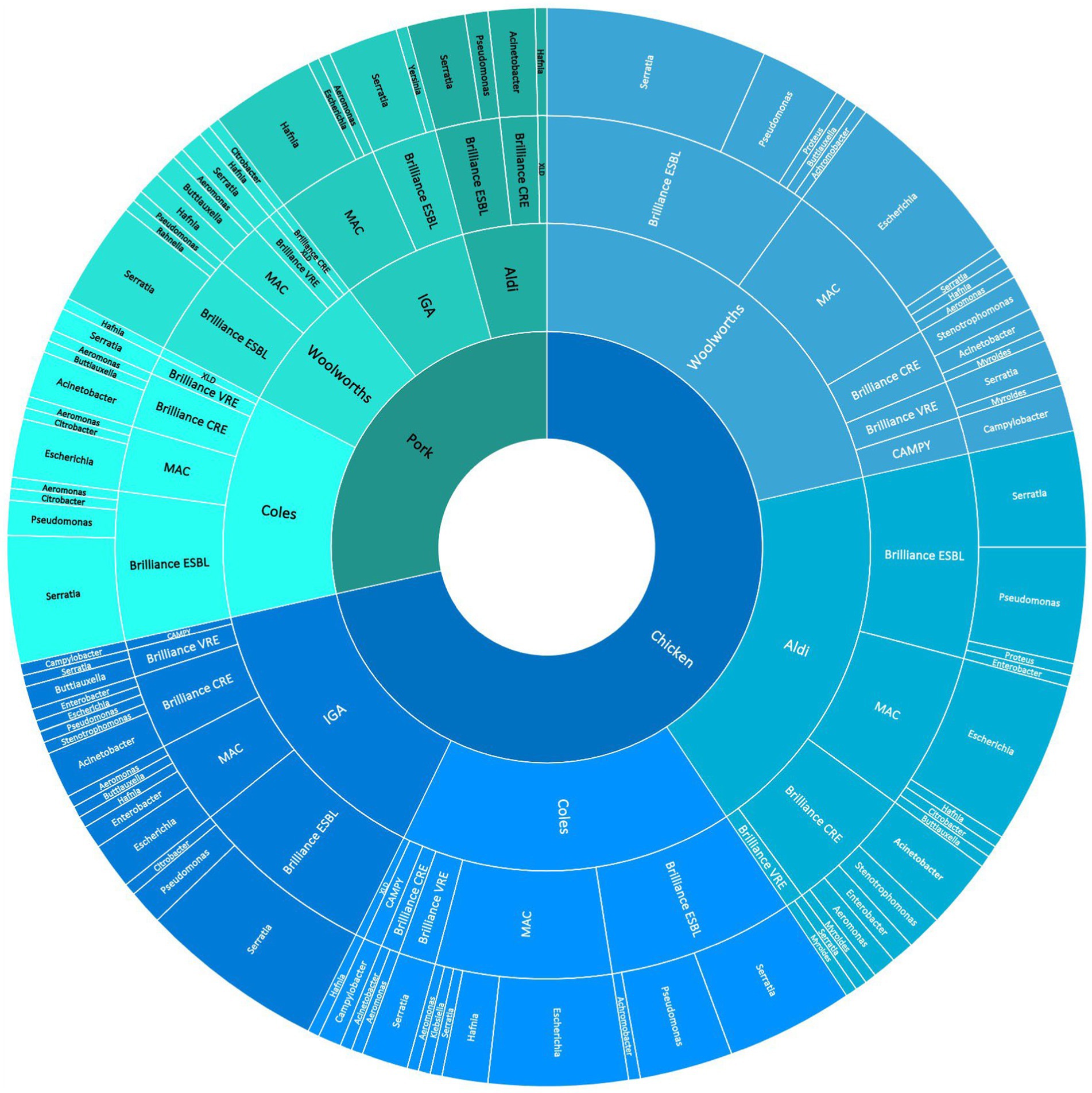
Figure 1. Sunburst diagram shows the breakdown of meat samples (innermost ring) across supermarket chains from 39.5 electorates in the ACT/NSW regions of Australia (second innermost ring), the abundance of isolates grown on various selective media (third innermost ring), and the abundance of bacterial genera grown on the media (outermost ring).
Antimicrobial resistance phenotyping
The 288 isolates that underwent WGS were tested for antibiotic sensitivity using an automated minimum inhibitory concentration (MIC) broth microdilution method and commercially available Gram-negative (CMV3AGNF) and Campylobacter (EUCAMP2) Sensititre™ antibiotic plates (Thermo Scientific™). According to World Health Organization (2022), each antibiotic on the list is either a critically important antibiotic (CIA) or a highly important antibiotic (HIA) for human health. Based on the chosen antibiotics, each isolate was evaluated to determine whether it was MDR, XDR, or PDR.
The AMR phenotype and MDR results for all 288 isolates that underwent WGS are provided in Table 2, for all pooled chicken and pork samples across all selective media used in the study. Of the 288 isolates, 35 (12%) were MDR, and of these, 17 were Serratia spp. that grew on Brilliance™ ESBL (15 chicken, 2 pork). The MDR criteria did not include antibiotics for which Serratia spp. are intrinsically resistant. The remaining 18 MDR isolates belonged to a variety of bacterial genera, including Proteus spp. (2/18, 11%), Rahnella spp. (1/18, 6%), Yersinia spp. (1/18, 6%), Buttiauxella spp. (2/18, 11%), Citrobacter spp. (2/18, 11%), Aeromonas spp. (3/18, 17%), Acinetobacter spp. (1/18, 6%), Enterobacter spp. (4/18, 22%), Pseudomonas spp. (1/18, 6%), and Escherichia spp. (1/18, 6%). All of the MDR bacteria were isolated from Brilliance™ ESBL (25/35, 71%), Brilliance™ CRE (9/35, 26%), or Brilliance™ VRE agar (1/35, 3%). No MDR isolate was cultured from either MAC or XLD. The frequency of MDR varied across bacterial isolates from chicken and pork samples and across supermarkets, with 28% (11/39), 1% (1/85), 15% (6/41), and 13% (10/79) of chicken isolates; and 3% (1/39), 10% (4/41), 0% (0/33), and 4% (2/47) of pork isolates being MDR from Aldi, Coles, IGA, and Woolworths, respectively.
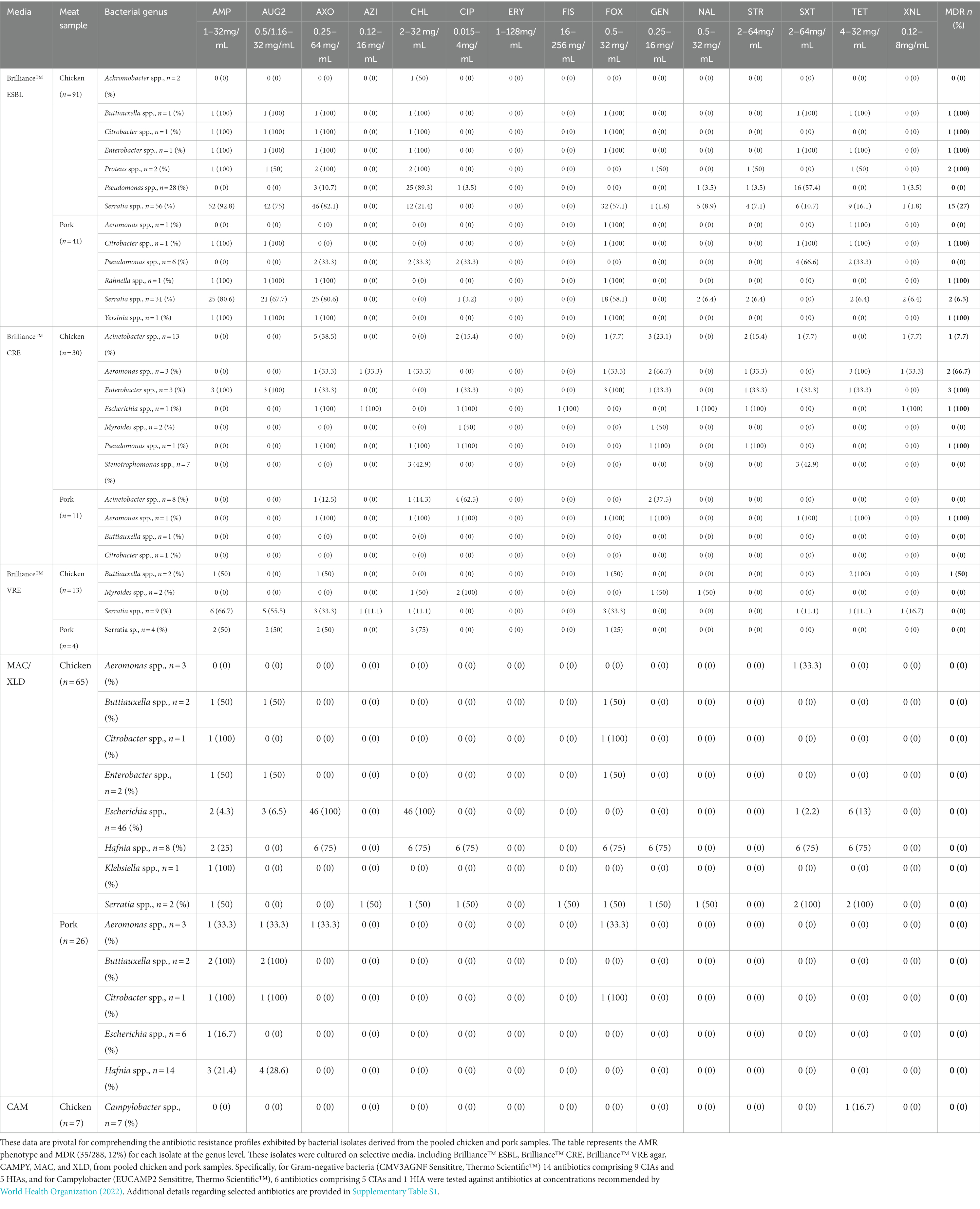
Table 2. Antibiotic resistance phenotype and multiple drug resistance results for bacterial genera isolated from various media for all pooled chicken and pork samples.
Of the MDR isolates cultured on Brillance™ ESBL agar, Serratia spp. from chicken meat had the highest rate of MDR (15/56, 27%), which was significantly higher than Serratia spp. isolated from pork samples (2/30, 6.67%), although pooled chicken samples were more likely to be comprised of more than one product. Ciprofloxacin resistance was found in one of the MDR pork strains. One Buttiauxella spp. isolate from the chicken was resistant to four CIAs (AMP, AUG2, AXO, and FOX) and one HIA (CHL, SXT, and TET). One Citrobacter spp. isolate from the chicken was resistant to four CIAs (AMP, AUG2, AXO, and FOX) and one HIA (CHL), while the Citrobacter spp. isolate from pork was resistant to three CIAs (AMP, AUG2, and FOX) and two HIAs (SXT and TET). One chicken meat-derived Enterobacter spp. isolate was resistant to four CIAs (AMP, AUG2, AXO, and FOX) and three HIAs (CHL, SXT, and TET). One of the two MDR Proteus isolates from the chicken was resistant to two CIAs (AMP and AXO) and two HIAs (CHL, TET), while the other was resistant to four CIAs (AUG2, AXO, GEN, and STR) and one HIA (CHL). One Rahnella spp. and one Yersinia spp. isolate, both from pork meat, were resistant to three CIAs (AUG2, AMP, and AXO). One Pseudomonas spp. isolate from chicken meat and two strains from pork meat were resistant to multiple antibiotics; however, when the Magiorakos et al. (2012) definition of MDR was applied to P. aeruginosa, none of them were classified as MDR.
A total of nine isolates that grew on Brilliance™ CRE were MDR; eight were from chicken samples and one from a pork sample. Strains isolated from chicken samples belonged to the following genera: Acinetobacter (1/13, 7.7%), Aeromonas (2/3, 66.7%), Enterobacter (3/3, 100%), E. coli (1/1, 100%), and Pseudomonas (1/1, 100%). The MDR pork isolate was from Aeromonas spp. Several MDR isolates from Brilliance™ CRE were resistant to ciprofloxacin. One Enterobacter spp. isolate was highly MDR, as it was resistant to six CIAs (AUG2, AMP, FOX, AXO, CIP, and GEN) and one HIA (SXT), including ciprofloxacin. A strain of E. coli was resistant to five CIAs (AZI, AXO, CIP, NAL, and STR) and two HIAs (XNL and FIS), including ciprofloxacin. In addition, two Acinetobacter spp. isolates and one Pseudomonas spp. isolate were ciprofloxacin-resistant and MDR. Of the three Enterobacter spp. isolates from chicken meat that were MDR, one was resistant to ciprofloxacin. One Aeromonas spp. isolate from a pork sample was ciprofloxacin-resistant and MDR.
Of the 17 isolates that grew on Brilliance™ VRE agar, one Buttiauxella spp. isolate from a chicken sample displayed MDR. No isolates grown on MAC or XLD were MDR. Of the seven Campylobacter spp. isolates that grew on CAMPY agar, one displayed tetracycline resistance, but none were deemed MDR. No Campylobacter spp. were isolated from pork samples.
Distribution of antimicrobial resistance genes
AMR genes were detected in the genomes of the 288 isolates using MobileElementFinder,3 a database for the identification of horizontally acquired AMR genes, virulence genes, and mobile genetic elements. Using a detection threshold of 95%, we found that 232/288 (81%) of the isolates carried at least one resistance gene (Table 3). AMR genes detected in these 232 isolates confer resistance to aminoglycosides, amphenicols, β-lactams, colistin, fosfomycin, hydrogen peroxide, olaquindox, quinolones, sulphonamides, tetracyclines, and trimethoprim. A full outline of the AMR genes for each strain is provided in Supplementary Table S1.
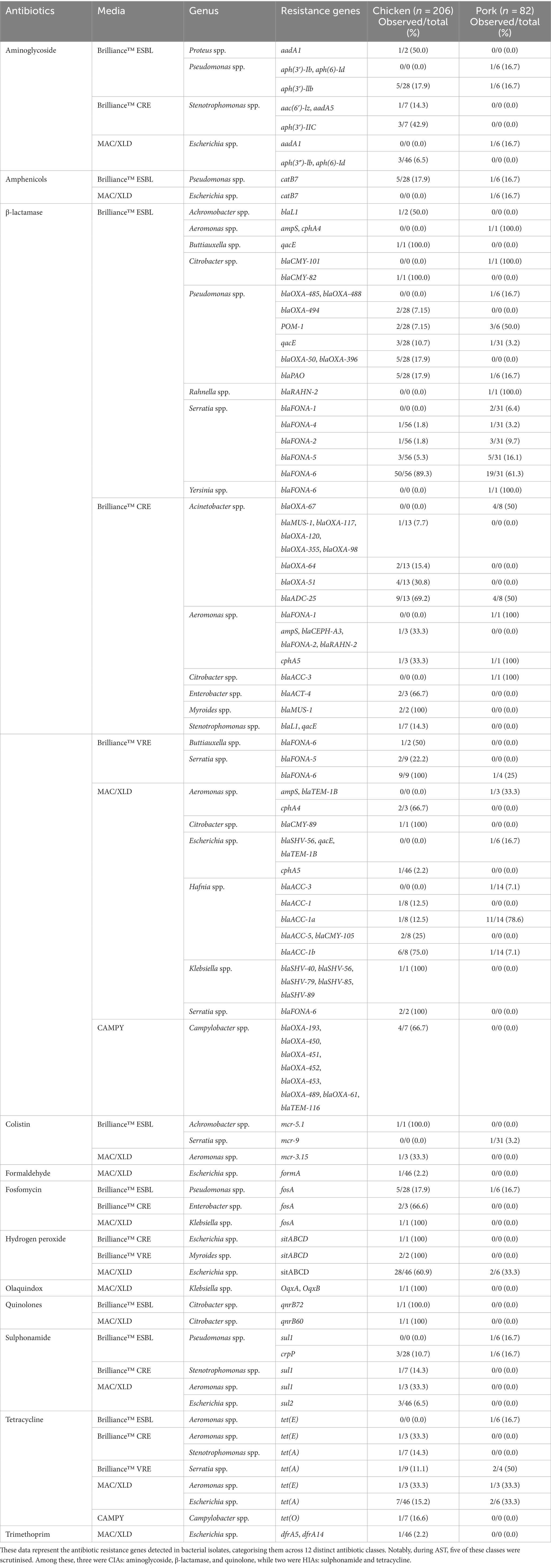
Table 3. Distribution of antimicrobial resistance genes found in bacterial isolates from pooled chicken and pork samples.
Aminoglycoside resistance genes
A total of 6/91 (7%) isolates from Brilliance™ ESBL agar from chicken meat and 1/41 (2%) from pork were found to contain aminoglycoside resistance genes. Among the chicken isolates, one Proteus spp. isolate (1/2, 50%) carried the aadA1 gene, and 5/28 (18%) of Pseudomonas spp. carried the aph(3′)-IIb gene. One (17%) pork Pseudomonas spp. isolate carried the aph(3′)-Ib gene. A total of four (13%) chicken isolates from Brilliance™ CRE agar harboured aminoglycoside resistance genes: two Stenotrophomonas spp. isolates carried aph(3′)-IIC, one carried aph(3′)-IIC and aac(6′)-Iz, and another carried aph(3′)-IIC and aadA5. None of the pork isolates from Brilliance™ CRE agar nor any isolates from Brilliance™ VRE agar (both chicken and pork) carried aminoglycoside resistance genes. Of the isolates that grew on MAC, 3/46 (7%) Escherichia spp. isolates from chicken carried both aph(3″)-Ib and aph(6)-Id, and one isolate from pork carried the aadA1 gene.
β-lactamase resistance genes
Of the isolates obtained from Brilliance™ ESBL agar, 59/91 (65%) from chicken and 26/41 (63%) from pork harboured genes conferring resistance to β-lactam antibiotics. Of the chicken isolates, one Achromobacter spp. carried blaL1, one Buttiauxella spp. carried qacE, and one Citrobacter spp. carried blaCMY-82. A total of 11/28 (39%) Pseudomonas spp. carried β-lactamase resistance genes; two carried multiple genes (blaOXA-494, blaOXA-50, blaOXA-396, and blaPAO); another two carried only POM-1; three carried blaOXA-50 and blaPAO; and four isolates carried only qacE. Of the Serratia spp. isolates, 55/56 (98%) carried a blaFONA gene variant, with variant blaFONA-6 being the most common (n = 50). Among the pork isolates, one Aeromonas spp. carried ampS and cphA4, one Citrobacter spp. carried blaCMY-101, three Pseudomonas spp. carried POM-1 only, and another Pseudomonas spp. isolate carried blaPAO, blaOXA-485, and blaOXA-488. One Rahnella spp. carried blaRAHN-2, and 30/31 (97%) Serratia spp. carried a blaFONA variant.
Of the isolates that grew on Brilliance™ CRE agar, 15/30 (50%) of the chicken isolates and 6/11 (55%) of the pork isolates carried a β-lactamase resistance gene. Among the chicken isolates, 11/13 (85%) Acinetobacter spp. carried various genes (blaMUS-1, blaADC-25, and blaOXA variants). Three Aeromonas spp., two Enterobacter spp., two Myroides spp., and one Stenotrophomonas spp. also carried various genes (ampS, blaCEPH, balRAHN, cph, blaACT, blaMUS, blaL1, qacE, and blaFONA variants). Among the pork isolates, four Acinetobacter spp., one Aeromonas spp., and one Citrobacter spp. carried β-lactamase resistance genes (blaOXA, blaADC, cph, blaACC, and blaFONA-1). Of the isolates that grew on Brilliance™ VRE agar, 12/13 (92%) of chicken and 3/4 (75%) of pork isolates carried β-lactamase resistance genes, with blaFONA variants being most common, particularly in Serratia spp.
Notably, isolates from MAC and XLD agar did not grow on Brilliance™ ESBL agar but were found to harbour β-lactamase resistance genes. These genes were identified in various species, including Aeromonas spp., Citrobacter spp., Escherichia spp., Hafnia spp., Klebsiella spp., and Serratia spp., in both chicken and pork samples. Of the Campylobacter isolates grown on CAMPY agar from chicken samples, 6/7 (86%, one E. coli, five C. jejuni) carried β-lactamase resistance genes, mostly blaOXA variants. One C. jejuni isolate harboured blaTEM-116.
Quinolone resistance genes
A single Citrobacter spp. chicken isolate from Brilliance™ ESBL harboured qnrB72. Two Enterobacter spp. chicken isolates from Brilliance™ CRE carried qnrE1. No pork samples carried quinolone resistance genes. One Citrobacter spp. chicken isolate from MAC carried qnrB60. No pork isolates harboured a quinolone resistance gene.
Sulphonamide resistance genes
Three chicken and one pork isolate from Brilliance™ ESBL agar harboured a sulphonamide resistance gene. All of these isolates were Pseudomonas spp., with chicken isolates carrying the crpP gene and the pork isolate carrying both crpP and sul1. One Stenotrophomonas spp. chicken isolate from Brilliance™ CRE agar carried sul1. Of the MAC isolates, one Aeromonas spp. carried sul1, and three Escherichia spp. carried sul2.
Tetracycline resistance genes
A single Aeromonas spp. pork isolate from Brilliance™ ESBL carried tet(E). Of the isolates obtained from Brilliance™ CRE agar, one Aeromonas spp. chicken isolate carried tet(E) and one Stenotrophomonas spp. carried tet(A). Of the Brilliance™ VRE isolates, one Serratia spp. chicken isolate carried tet(A), and two Serratia spp. pork isolates also harboured tet(A). The majority of tetracycline resistance genes were detected in isolates from MAC/XLD agar. This included 7/46 (15%) Escherichia spp. from chicken samples and 2/6 (33%) Escherichia spp. from pork, which carried tet(A). One Aeromonas spp. isolate from chicken and one from pork carried the tet(E) gene. A single strain of C. jejuni from CAMPY agar carried the tet(O).
Polymixin (colistin) resistance genes
Three isolates in this study were found to harbour a mobile colistin resistance (mcr) gene. One was a Serratia strain that was isolated on Brilliance™ ESBL agar and harboured the mcr-9 variant. This strain also harboured the IncHI2 plasmid, which is known to be capable of carrying mcr genes, as well as a β-lactam resistance gene (blaFONA-6) and a gene conferring resistance to antiseptics (qacE). An Aeromonas strain, isolated from MacConkey agar, harboured the mcr-3.15 variant and was detected in the same section of DNA (contig) as transposon Tn4671 and insertion sequence ISAs17, suggesting that these genetic elements may have played a role in the acquisition of mcr-3.15. This strain also harboured the tetracycline resistance gene, tet(E). The third strain harbouring an mcr gene grew on Brilliance™ ESBL agar and was classified as Achromobacter. It harboured mcr-5.1, a contig containing the insertion sequence ISRme15, and blaL1.
Resistance genes associated with other antimicrobials
Resistance genes associated with various other antimicrobials were identified. A fosfomycin resistance gene, fosA, was detected in eight different isolates, including five Pseudomonas spp., two Enterobacter spp., and one Klebsiella spp. A single Escherichia spp. isolate from MAC/XLD agar was identified as carrying the formaldehyde resistance gene, formA, and the hydrogen peroxide resistance and metal transporter gene sitABCD was present in 28/46 (61%) of Escherichia spp. A small number of strains from MAC/XLD carried genes conferring resistance to amphenicols, olaquindox, and trimethoprim.
Distribution of plasmids
A large number of plasmids were detected across the 288 isolates (Supplementary Table S2). A list of plasmids (and resistance genes) associated with the MDR isolates is provided in Table 4.
Of the MDR isolates, 13/35 (37%) carried one or more plasmids. Nine of these isolates were Serratia spp., and all except one strain carried the ColE10 plasmid. Other plasmids in these strains included IncHI1A, IncHI1B, IncN2, Col4401, ColRAAI, and ColYe4449. One Citrobacter spp. carried the IncFIB(pB171) plasmid, and another Citrobacter spp. carried the Col(Ye4449) plasmid. One Escherichia spp. carried the IncFIB(AP001918) and IncFII plasmids.
Distribution of virulence factors
One or more virulence genes were present in 61/288 (21%) of the isolates (Table 5). The vast majority of virulence factors were detected in E. coli, likely owing to the large number of virulence factors associated with this species in the VirulenceFinder database. All of the E. coli isolates in the study, apart from one isolate from pork, were found to harbour multiple virulence genes, ranging from 3 to 31 genes. Of note was the MDR E. coli isolate from Brilliance™ CRE agar, which had 17 virulence genes, nine of which were encoded on a plasmid (etsC, iroN, cia, hlyF, cvaC, mchF, traT, ompT, and iss), and the remainder elsewhere in the genome (sitA, gad, terC, eilA, air, hlyE, and chuA). All of the E. coli isolates (53/53, 100%) carried terC, consistent with other studies (Byarugaba et al., 2023). The next most frequent virulence factor was traT (37/46, 80%). Other common virulence factors included chuA, cia, cvaC, etsC, fyuA, gad, hlyE, hlyF, hra, ipfA, ireA, iroN, irp2, iss, iucC, iutA, ompT, and sitA.
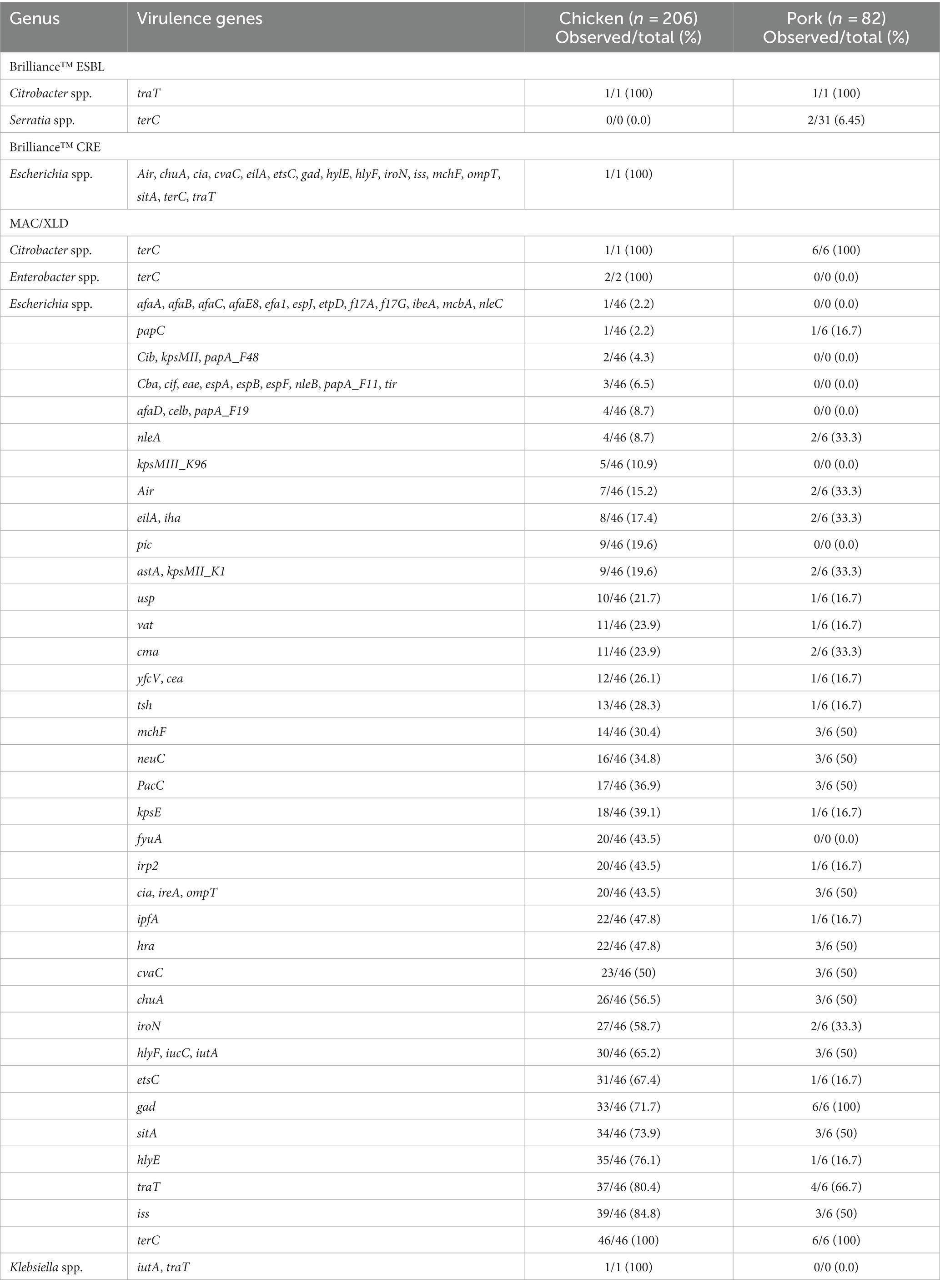
Table 5. Distribution of virulence genes found in bacterial isolates from pooled chicken and pork samples.
Pathogenicity of bacterial isolates
To determine the likelihood that an isolate is pathogenic to humans, the PathogenFinder tool from the CGE database was employed.4 Of the 288 bacterial isolates, 233 (81%) were determined to be pathogenic to humans, with probabilities ranging from 0.563 to 0.92. Campylobacter spp. and Escherichia coli exhibited notably high pathogenicity, with an average pathogenicity score of 0.91 and 0.87, respectively. In contrast, Hafnia spp. had the lowest pathogenicity, averaging 0.59. Acinetobacter spp. possessed the highest abundance of pathogenic protein families, totalling 402.2 on average. In contrast, Hafnia spp. exhibited the lowest number of pathogenic protein families, containing only 22.8 on average.
Discussion
In this study, we describe the phenotypic and sequence-based AMR profiles of bacteria isolated from chicken and pork meat. Since only one representative for each colony morphology and colour was chosen from a limited number of selective agars, the total number and diversity of bacterial isolates present in the chicken and pork meat samples are likely to have been underestimated. Preferential selection of 288 isolates resulted in the detection of 17 bacterial genera or 33 bacterial species. Of the 288 isolates, 12% were MDR. By assessing the AMR phenotypic and genotypic profiles of species other than those typically used in surveillance studies, we were able to show that the reservoir of antimicrobial resistance to critically and highly important antibiotics in bacteria isolated from retail meat in Australia is more diverse than previously demonstrated.
In this study, we detected three mobilised colistin resistance (mcr) genes, conferring resistance to a last-line antimicrobial (colistin) for multidrug-resistant Gram-negative infections. These genes are capable of transmitting to different genera and species of bacteria that colonise humans and animals or are present in the environment. While Australia is thought to have a low abundance of colistin resistance, a recent analysis of host-derived and environmental metagenomes revealed that the highest log-ratio abundances of mcr fragments could be found in metagenomes from Australia when compared to countries all over the world (Martiny et al., 2022). This study showed that the mcr-9 variant is most common in Australia and that mcr variants are not equally distributed among different countries or bacterial genus/species. Surveillance studies of common foodborne pathogens would not have detected the presence of mcr variants in this study, as they were carried by bacteria not normally included in such studies, such as Aeromonas, Achromobacter, and Serratia.
The detection of multiple variants of mcr (variants −3.15, −5.1, and − 9) across different species of bacteria, harbouring other AMR genes on MGEs known to transmit mcr genes suggests that the distribution of mcr genes in Australia is likely to increase. Co-selection of mcr genes with AMR genes conferring resistance to antibiotics that are permitted for use in the agri-food industry in Australia, such as tetracycline, may accelerate the spread of colistin resistance. Although Serratia are known to be intrinsically resistant to colistin (Bean et al., 2020), we showed that Serratia are capable of acquiring mcr genes on plasmids that can be transferred to other species of bacteria. A ban on the prophylactic use of antibiotics, similar to that adopted by EU countries, may provide protection against the spread of colistin resistance. It is known that removing an antibiotic for use in the agri-food industry in Australia can result in a dramatic decline in resistance to that antibiotic. For example, a steady and significant reduction in erythromycin resistance in Campylobacter and Enterococcus isolated from food-producing chickens has been observed since the reduction in the use of macrolides in the 1990s, owing to the introduction of Mycoplasma vaccines (Australian Chicken Meat Federation, 2022). Similarly, the frequency of resistance to quinupristin-dalfopristin in Enterococcus faecium also significantly declined from 54.5 to 6.1% following a ban on the use of virginamycin in chickens in Australia. Removal of all antibiotics for prophylactic use in Australia would provide the best strategy for halting the spread of resistance to CIAs that arises due to the co-selection of genes conferring antimicrobial resistance to antibiotics currently used for “prevention” and genes conferring resistance to CIAs on MGEs.
The majority of the Enterobacteriaceae identified in this study belonged to the genus Serratia. Members of this genus were found to have acquired resistance genes for aminoglycosides, β-lactamases, fosfomycin, quinolones, amphenicols, and polypeptides, in a study by Sandner-Miranda et al. (2018). Serratia spp. isolates in this study harboured acquired resistance genes for colistin, β-lactamases, fosfomycin, and quinolones. Serratia marcesensi, which was previously thought to be a non-pathogenic environmental species, is responsible for a number of hospital-acquired infections, and MDR is already making infections with this species difficult to treat (Moradigaravand et al., 2016). It is largely unknown if other species of Serratia are contributing to AMR in S. marcesens and if AMR in the wider species poses a threat to human health as opportunistic pathogens.
The vast majority of virulence genes identified in this study were in E. coli isolates. However, genomic data for E. coli are more common than some of the lesser-known species identified, and therefore the virulence database is likely biased towards detecting virulence genes associated with E. coli. Several studies have shown that APEC and ExPEC virulence genes share similarities. Virulence genes such as iss, iuA, ompT, papGII, and sfa have been detected in zoonotic pathogens (Najafi et al., 2019). In this study, papA, papC, usp., kpsMII, and ibeA were frequently detected in E. coli, similar to APEC isolates harbouring pap, sfa, usp., cnf1, kpsMTII, hlyA, and ibeA virulence genes (Cunha et al., 2017). ExPEC-related virulence genes include astA, cvaC, hra, hlyF, fyuA, ibeA, ireA, iss, ompT, papA, papC, papE, papF, tsh, and traT, all of which were detected in this study and were previously found to be prevalent in E. coli isolated from chicken meat samples (Mitchell et al., 2015). One study performed a cluster analysis of E. coli isolated from UTIs, community-dwelling humans, meat, and meat production animals, which included data on the presence of eight ExPEC-related virulence genes (kpsM II, papA, papC, iutA, sfaS, focG, afa, hlyD) and AMR and found a strong association between the isolates from the various sources, suggesting that strains isolated from meat and meat production animals may be zoonotic pathogens (Jakobsen et al., 2010). The findings of the current study suggest that E. coli isolates from chicken meat may pose a zoonotic risk to humans given that most of the isolates (98%) were predicted to be pathogenic towards humans and that 47/53 (89%) of the E. coli isolates were isolated from chicken meat.
The level of MDR in this study was not particularly high relative to other countries (Collignon, 2015). However, given that we used selective media containing antibiotics, this figure does not accurately reflect the level of MDR in bacteria isolated from chicken and pork meat in Australia. This approach did allow us, however, to observe the diversity of MDR bacteria that grew on the various selective media. While few species identified in this study are commonly associated with infections in humans, most have been associated with infections in humans. For the most part, the AMR genes detected using WGS did not explain the resistance phenotypes observed on the selective agars or in the commercial antibiotic plates. This may be due to not all resistance genes being discovered, particularly for the less-well-studied species identified in this study, or the resistance mechanisms being chromosomally encoded, rather than acquired. Nonetheless, we identified a variety and diversity of bacteria that harboured horizontally acquired genes conferring resistance to either critically or highly important antibiotics. Some of these were MDR and are likely to be pathogenic to humans.
AMR is a worldwide issue, and its management calls for a “One Health” approach. The use of antibiotics in food-producing animals maintains antibiotic resistance mechanisms that make treatment of resistant bacterial infections in humans and animals difficult. Future surveillance studies should include analysis of a greater diversity of bacteria, to ensure the full diversity of AMR genes is revealed.
Data availability statement
The original contributions presented in the study are included in the article/Supplementary material, further inquiries can be directed to the corresponding author.
Ethics statement
The article presents research on animals that do not require ethical approval for their study.
Author contributions
OD: Data curation, Formal analysis, Methodology, Writing – original draft, Investigation. MB: Methodology, Writing – review & editing. AP: Methodology, Writing – review & editing, Investigation. CO’B: Methodology, Writing – review & editing, Conceptualization, Data curation, Formal analysis, Funding acquisition, Project administration, Resources, Supervision, Writing – original draft.
Funding
The author(s) declare financial support was received for the research, authorship, and/or publication of this article. This research study was funded by the Animals Australia.
Conflict of interest
The authors declare that the research was conducted in the absence of any commercial or financial relationships that could be construed as a potential conflict of interest.
Publisher’s note
All claims expressed in this article are solely those of the authors and do not necessarily represent those of their affiliated organizations, or those of the publisher, the editors and the reviewers. Any product that may be evaluated in this article, or claim that may be made by its manufacturer, is not guaranteed or endorsed by the publisher.
Supplementary material
The Supplementary material for this article can be found online at: https://www.frontiersin.org/articles/10.3389/fmicb.2024.1347597/full#supplementary-material
Footnotes
1. ^https://eur-lex.europa.eu/eli/reg/2019/6/oj
2. ^https://poultryhealthtoday.com/nearly-60-of-us-broilers-now-raised-without-antibiotics-but-that-number-may-have-peaked/
References
Abraham, S., O'Dea, M., Sahibzada, S., Hewson, K., Pavic, A., Veltman, T., et al. (2020). Correction: Escherichia coli and Salmonella spp. isolated from Australian meat chickens remain susceptible to critically important antimicrobial agents. PLoS One 15:e0227383. doi: 10.1371/journal.pone.0227383
Aghapour, Z., Gholizadeh, P., Ganbarov, K., Bialvaei, A. Z., Mahmood, S. S., Tanomand, A., et al. (2019). Molecular mechanisms related to colistin resistance in Enterobacteriaceae. Infect. Drug Resist. 12, 965–975. doi: 10.2147/IDR.S199844
Australian Chicken Meat Federation (2022). Surveillance for antimicrobial resistance in enteric commensals and pathogens in Australian meat chickens 2022. Available at: https://chicken.org.au/wp-content/uploads/2023/07/Chicken-Meat-AMR-survey-Final-report-1.pdf.
Bankevich, A., Nurk, S., Antipov, D., Gurevich, A. A., Dvorkin, M., Kulikov, A. S., et al. (2012). SPAdes: a new genome assembly algorithm and its applications to single-cell sequencing. J. Comput. Biol. 19, 455–477. doi: 10.1089/cmb.2012.0021
Barlow, R. S., McMillan, K. E., Duffy, L. L., Fegan, N., Jordan, D., and Mellor, G. E. (2015). Prevalence and antimicrobial resistance of Salmonella and Escherichia coli from Australian cattle populations at slaughter. J. Food Prot. 78, 912–920. doi: 10.4315/0362-028X.JFP-14-476
Bean, D. C., Wigmore, S. M., Momin, M. H. F. A., and Wareham, D. W. (2020). Polymyxin resistance bacteria in Australian poultry. Front. Sustain. Food Syst. 4:550318. doi: 10.3389/fsufs.2020.550318
Blyton, M. D., Pi, H., Vangchhia, B., Abraham, S., Trott, D. J., Johnson, J. R., et al. (2015). Genetic structure and antimicrobial resistance of Escherichia coli and cryptic clades in birds with diverse human associations. Appl. Environ. Microbiol. 81, 5123–5133. doi: 10.1128/AEM.00861-15
Brettin, T., Davis, J. J., Disz, T., Edwards, R. A., Gerdes, S., Olsen, G. J., et al. (2015). RASTtk: a modular and extensible implementation of the RAST algorithm for building custom annotation pipelines and annotating batches of genomes. Sci. Rep. 5:8365. doi: 10.1038/srep08365
Byarugaba, D. K., Erima, B., Wokorach, G., Alafi, S., Kibuuka, H., Mworozi, E., et al., (2023). Resistome and virulome of high-risk pandemic clones of multidrug-resistant extra-intestinal pathogenic Escherichia coli (ExPEC) isolated from tertiary healthcare settings in Uganda. PloS one, 18, e0294424. doi: 10.1371/journal.pone.0294424
Cameron, A., and McAllister, T. A. (2016). Antimicrobial usage and resistance in beef production. J. Anim. Sci. Biotechnol. 7:68. doi: 10.1186/s40104-016-0127-3
Collignon, P. (2015). Antibiotic resistance: are we all doomed? Intern. Med. J. 45, 1109–1115. doi: 10.1111/imj.12902
Cosentino, S., Voldby Larsen, M., Møller Aarestrup, F., and Lund, O. (2013). PathogenFinder--distinguishing friend from foe using bacterial whole genome sequence data. PLoS One 8:e77302. doi: 10.1371/journal.pone.0077302
Cunha, M. P. V., Saidenberg, A. B., Moreno, A. M., Ferreira, A. J. P., Vieira, M. A. M., Gomes, T. A. T., et al. (2017). Pandemic extra-intestinal pathogenic Escherichia coli (ExPEC) clonal group O6-B2-ST73 as a cause of avian colibacillosis in Brazil. PLoS One 12:e0178970. doi: 10.1371/journal.pone.0178970
Dunachie, S. J., Day, N. P. J., and Dolecek, C. (2020). The challenges of estimating the human global burden of disease of antimicrobial resistant bacteria. Curr. Opin. Microbiol. 57, 95–101. doi: 10.1016/j.mib.2020.09.013
El-Sayed Ahmed, M. A. E., Zhong, L. L., Shen, C., Yang, Y., Doi, Y., and Tian, G. B. (2020). Colistin and its role in the era of antibiotic resistance: an extended review (2000–2019). Emerg. Microbes Infect. 9, 868–885. doi: 10.1080/22221751.2020.1754133
Habib, I., Coles, J., Fallows, M., and Goodchild, S. (2020). Human campylobacteriosis related to cross-contamination during handling of raw chicken meat: Application of quantitative risk assessment to guide intervention scenarios analysis in the Australian context. Int. J. Food Microbiol. 332:108775. doi: 10.1016/j.ijfoodmicro.2020.108775
Jakobsen, L., Spangholm, D. J., Pedersen, K., Jensen, L. B., Emborg, H. D., Agersø, Y., et al. (2010). Broiler chickens, broiler chicken meat, pigs and pork as sources of ExPEC related virulence genes and resistance in Escherichia coli isolates from community-dwelling humans and UTI patients. Int. J. Food Microbiol. 142, 264–272. doi: 10.1016/j.ijfoodmicro.2010.06.025
Johansson, M. H. K., Bortolaia, V., Tansirichaiya, S., Aarestrup, F. M., Roberts, A. P., and Petersen, T. N. (2021). Detection of mobile genetic elements associated with antibiotic resistance in Salmonella enterica using a newly developed web tool: MobileElementFinder. J. Antimicrob. Chemother. 76, 101–109. doi: 10.1093/jac/dkaa390
Kirchhelle, C. (2018). Pharming animals: a global history of s in food production (1935–2017). Palgrave Commun. 4:96. doi: 10.1057/s41599-018-0152-2
Kluytmans, J. A., Overdevest, I. T., Willemsen, I., Kluytmans-van den Bergh, M. F., van der Zwaluw, K., Heck, M., et al. (2013). Extended-spectrum β-lactamase-producing Escherichia coli from retail chicken meat and humans: comparison of strains, plasmids, resistance genes, and virulence factors. Clin. Infect. Dis. 56, 478–487. doi: 10.1093/cid/cis929
Landers, T. F., Cohen, B., Wittum, T. E., and Larson, E. L. (2012). A review of use in food animals: perspective, policy, and potential. Public Health Rep. 127, 4–22. doi: 10.1177/003335491212700103
Larsen, M. V., Cosentino, S., Rasmussen, S., Friis, C., Hasman, H., Marvig, R. L., et al. (2012). Multilocus sequence typing of total-genome-sequenced bacteria. J. Clin. Microbiol. 50, 1355–1361. doi: 10.1128/JCM.06094-11
Lee, T., Jordan, D., Sahibzada, S., Abraham, R., Pang, S., Coombs, G. W., et al. (2021). Antimicrobial resistance in porcine enterococci in Australia and the ramifications for human health. Appl. Environ. Microbiol. 87, e03037–e03020. doi: 10.1128/AEM.03037-20
Leverstein-van Hall, M. A., Dierikx, C. M., Cohen Stuart, J., Voets, G. M., van den Munckhof, M. P., van Essen-Zandbergen, A., et al. (2011). Dutch patients, retail chicken meat and poultry share the same ESBL genes, plasmids and strains. Clin. Microbiol. Infect. 17, 873–880. doi: 10.1111/j.1469-0691.2011.03497.x
Magiorakos, A. P., Srinivasan, A., Carey, R. B., Carmeli, Y., Falagas, M. E., Giske, C. G., et al. (2012). Multidrug-resistant, extensively drug-resistant and pandrug-resistant bacteria: an international expert proposal for interim standard definitions for acquired resistance. Clin. Microbiol. Infect. 18, 268–281. doi: 10.1111/j.1469-0691.2011.03570.x
Martiny, H. M., Munk, P., Brinch, C., Szarvas, J., Aarestrup, F. M., and Petersen, T. N. (2022). Global distribution of mcr gene variants in 214K metagenomic samples. mSystems 7:e0010522. doi: 10.1128/msystems.00105-22
Mitchell, N. M., Johnson, J. R., Johnston, B., Curtiss, R. 3rd, and Mellata, M. (2015). Zoonotic potential of Escherichia coli isolates from retail chicken meat products and eggs. Appl. Environ. Microbiol. 81, 1177–1187. doi: 10.1128/AEM.03524-14
Moradigaravand, D., Boinett, C. J., Martin, V., Peacock, S. J., and Parkhill, J. (2016). Recent independent emergence of multiple multidrug-resistant Serratia marcescens clones within the United Kingdom and Ireland. Genome Res. 26, 1101–1109. doi: 10.1101/gr.205245.116
Najafi, S., Rahimi, M., and Nikousefat, Z. (2019). Extra-intestinal pathogenic Escherichia coli from human and avian origin: Detection of the most common virulence-encoding genes. Vet. Res. Forum. 10, 43–49. doi: 10.30466/vrf.2019.34307
Olson, R. D., Assaf, R., Brettin, T., Conrad, N., Cucinell, C., Davis, J. J., et al. (2023). Introducing the bacterial and viral bioinformatics resource center (BV-BRC): a resource combining PATRIC, IRD and ViPR. Nucleic Acids Res. 51, D678–D689. doi: 10.1093/nar/gkac1003
Overdevest, I., Willemsen, I., Rijnsburger, M., Eustace, A., Xu, L., Hawkey, P., et al. (2011). Extended-spectrum β-lactamase genes of Escherichia coli in chicken meat and humans, the Netherlands. Emerg. Infect. Dis. 17, 1216–1222. doi: 10.3201/eid1707.110209
Papp-Wallace, K. M., Endimiani, A., Taracila, M. A., and Bonomo, R. A. (2011). Carbapenems: past, present, and future. Antimicrob. Agents Chemother. 55, 4943–4960. doi: 10.1128/AAC.00296-11
Sandner-Miranda, L., Vinuesa, P., Cravioto, A., and Morales-Espinosa, R. (2018). The genomic basis of intrinsic and acquired resistance in the genus Serratia. Front. Microbiol. 9:828. doi: 10.3389/fmicb.2018.00828
Touchon, M., Perrin, A., de Sousa, J. A. M., Vangchhia, B., Burn, S., O’Brien, C. L., et al., (2020). Phylogenetic background and habitat drive the genetic diversification of Escherichia coli. PLoS Genet. 16:e1008866. doi: 10.1371/journal.pgen.1008866
Vangchhia, B., Blyton, M., Collignon, P., Kennedy, K., and Gordon, D. M. (2018). Factors affecting the presence, genetic diversity and antimicrobial sensitivity of Escherichia coli in poultry meat samples collected from Canberra, Australia. Environ. Microbiol. 20, 1350–1361. doi: 10.1111/1462-2920.14030
World Health Organization (WHO). (2020). Antimicrobial resistance. Fact sheet. Available at: https://www.who.int/news-room/fact-sheets/detail/antimicrobial-resistance.
World Health Organization. (2022). Critically importants for human medicine: 6th revision. Available at: https://www.who.int/publications/i/item/9789241515528.
Wozniak, T. M., Dyda, A., Merlo, G., and Hall, L. (2022). Disease burden, associated mortality and economic impact of antimicrobial resistant infections in Australia. Lancet Reg. Health West. Pac. 27:100521. doi: 10.1016/j.lanwpc.2022.100521
Keywords: antibiotic, antibiotic resistance, antimicrobial resistance, antimicrobial susceptibility testing, multidrug-resistance, resistance genes, whole-genome sequencing
Citation: Dixit OVA, Behruznia M, Preuss AL and O’Brien CL (2024) Diversity of antimicrobial-resistant bacteria isolated from Australian chicken and pork meat. Front. Microbiol. 15:1347597. doi: 10.3389/fmicb.2024.1347597
Edited by:
Benoit Doublet, Institut National de recherche pour l’agriculture, l’alimentation et l’environnement (INRAE), FranceReviewed by:
Reham M. El-Tarabili, Suez Canal University, EgyptValerio Giaccone, Produzioni e Salute Università di Padova, Italy
Copyright © 2024 Dixit, Behruznia, Preuss and O’Brien. This is an open-access article distributed under the terms of the Creative Commons Attribution License (CC BY). The use, distribution or reproduction in other forums is permitted, provided the original author(s) and the copyright owner(s) are credited and that the original publication in this journal is cited, in accordance with accepted academic practice. No use, distribution or reproduction is permitted which does not comply with these terms.
*Correspondence: Claire L. O’Brien, Y2xhaXJlLm9icmllbkBjYW5iZXJyYS5lZHUuYXU=