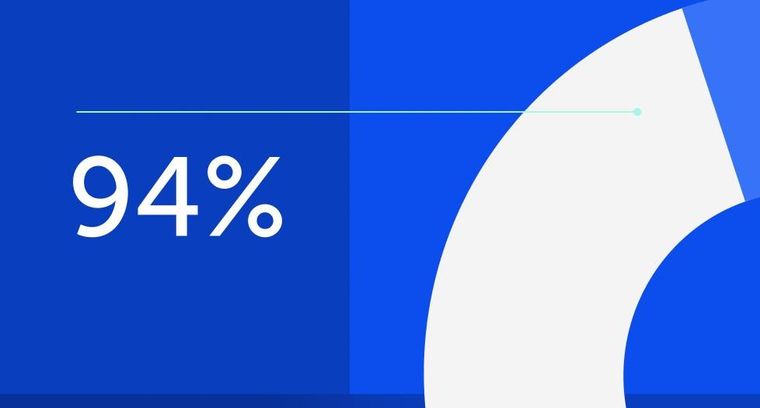
94% of researchers rate our articles as excellent or good
Learn more about the work of our research integrity team to safeguard the quality of each article we publish.
Find out more
REVIEW article
Front. Microbiol., 29 January 2024
Sec. Antimicrobials, Resistance and Chemotherapy
Volume 15 - 2024 | https://doi.org/10.3389/fmicb.2024.1347490
This article is part of the Research TopicConference Research Topic: 9th Symposium on Antimicrobial Resistance in Animals and the Environment (ARAE 2023)View all 21 articles
Antimicrobial resistance is a major threat to human health and must be approached from a One Health perspective. Use of antimicrobials in animal husbandry can lead to dissemination and persistence of resistance in human pathogens. Polyether ionophores (PIs) have antimicrobial activities and are among the most extensively used feed additives for major production animals. Recent discoveries of genetically encoded PI resistance mechanisms and co-localization of resistance mechanisms against PIs and antimicrobials used in human medicine on transferrable plasmids, have raised concerns that use of PIs as feed additives bear potential risks for human health. This review summarizes the current knowledge on PI resistance and discusses the potential consequences of PI-usage as feed additives in a One Health perspective.
Antimicrobial resistance (AMR) poses a serious threat to human health. It has been estimated that 1.27 million deaths were caused by bacterial AMR in 2019 (Murray et al., 2022) and the negative effect on human welfare is predicted to escalate in the next decades. Although the main focus on AMR has been on health care settings, it is recognized that veterinary medicine, plant- and animal production, and environmental sectors play an important role in the origin, persistence, and spread of AMR.
Polyether ionophores (PIs) have been used as feed additives for production animals since the early 1970 (Chapman et al., 2010). PIs possess both antibacterial and antiprotozoal activity and are currently used in poultry production worldwide to control severe diseases such as coccidiosis caused by Eimeria spp. and necrotic enteritis caused by Clostridium perfringens (Martins et al., 2022). The antibacterial activity of PIs has also proven useful to improve feed conversion in ruminants (Callaway et al., 2003; Kim et al., 2014; Scharen et al., 2017). PIs are not used in human medicine due to their cytotoxicity. However, PIs and PI-derivatives with low toxicity are considered for therapeutic treatment of cancer (Huczyński, 2012; Kaushik et al., 2018; Wang et al., 2021) and infectious diseases caused by bacteria (Wollesen et al., 2023), fungi, protozoa, and even virus (Huczyński, 2012; Lin et al., 2021).
Sales and use of PIs are not systematically reported in most countries, making it difficult to estimate the global consumption level (Hansen et al., 2009; Mulchandani et al., 2023). However, based on available data from countries in Europe, North America, and Australia, PIs are among the most extensively used antimicrobial feed additives for production animals across the world (DANMAP 2015, 2016; PHAC, 2016; SWEDRES/SVARM 2016, 2017; FDA, 2023a).
Polyether ionophores are produced and secreted by bacteria of the class Actinomycetia. They are highly lipophilic compounds that form lipid soluble complexes with cations and facilitate their diffusion through biological membranes. This disrupts chemical gradients across membranes and interferes with essential biological processes. The PIs display different ion selectivity for cations abundant in biological systems depending on their structure. Most commonly used PIs in animal husbandry can bind both K+ and Na+ under artificial conditions, and with the exception of monensin, these PIs prefer K+ over Na+. Lasalocid, however, has been shown to form complexes with both mono- and divalent cations (Table 1).
Table 1. Polyether ionophores commonly used in animal husbandry and their ion selectivity in artificial systems.
The antimicrobial mechanism(s) of these PIs are not fully understood. Independent of the cation preference in artificial systems, it appears that the effect of most PIs used in animal husbandry is disruption of the Na+/K+ homeostasis and a concomitant change in cytosolic pH (Russell and Strobel, 1989). This could theoretically be a direct effect of the PIs ability to bind both Na+ and K+ and thereby facilitate transport of Na+ into the cell and K+ out of the cell, and that the change in pH results from induction of endogenous membrane transport systems to restore the chemical gradients of Na+ and K+. However, the general opinion is that PIs transport a cation (Na+/K+) in one direction and H+ in the opposite direction. The primary effect would therefore be an intracellular change in Na+ or K+ gradient and a simultaneous change in pH. It has been hypothesized that the resulting disruption of membrane cation gradients induces compensatory mechanisms to restore cation-homeostasis, including Na+/K+ ATPase and F-ATPase. The activity of these compensatory mechanisms results in a secondary effect of disrupted Na+, K+, and H+ homeostasis, increased demand for ATP and subsequent tertiary effects on essential cellular processes (Figures 1A,B; Smith and Galloway, 1983; Russell and Strobel, 1989).
Figure 1. Proposed effects of monensin-mediated ion gradient disruption in Eimeria tenella (Smith and Galloway, 1983) parasites (A) and in gram-positive Streptococcus bovis (Russell, 1987) bacteria (B). Encircled numbers represent suggested sequence of ion transport events. Red arrows indicate direction of change in cystosolic ion concentrations. Cation gradients across a bilayer is displayed by triangles.
It is likely that the PI-mediated disruption of cation gradients directly or indirectly inhibits both primary and secondary membrane transport proteins resulting in disrupted import and export of nutrients, metabolites and xenobiotics. In addition, the disturbances in the intracellular cation concentration may inhibit enzymes involved in essential cellular processes. Although it has been suggested that bacterial growth inhibition could be caused either by energy depletion due to increased demand for ATP or by cell acidification as a result of influx of H+ (Russell, 1987), other mechanisms may also be involved.
Polyether ionophores affect bacterial metabolism of carbohydrates, amino acids, and fatty acids. The specific effect of disrupting the chemical gradients across the cytoplasmic membrane varies depending on the cation-dependent processes and the metabolic requirements of the organism. Chow et al. showed that monensin and lasalocid inhibited growth of the gram-negative bacterium Fibrobacter succinogenes. They showed that the ATP synthesis was decreased, most likely as an effect of an inability to take up glucose via Na+/glucose symporters (Chow and Russell, 1992). PIs have also been shown to reduce amino acid transport in ruminal bacteria (Chen and Russell, 1989; Russell and Strobel, 1989; Van Kessel and Russell, 1992). In contrast, while monensin exposure caused cessation of growth of Streptococcus bovis in vitro (Russell, 1987), glucose transport was not inhibited and glucose fermentation continued resulting in continuous ATP production (Russell, 1987). The hypothesized explanation to this observation was that S. bovis can use the phosphotransferase system as well as facilitated diffusion for glucose uptake (Russell et al., 1990).
Similar biological effects have been observed in the protozoan parasite Eimeria tenella where monensin caused an increase in intracellular concentrations of both Na+ and K+ (Smith and Galloway, 1983). As specific inhibition of the Na+/K+-pump increased the K+ level, it was proposed that monensin caused an initial uptake of Na+ followed by an exchange of intracellular Na+ for extracellular K+ (Figure 1B). Several anticoccidial modes of PIs have been suggested, such as energy depletion, mitochondrial stress, and inhibition of invasion of enterocytes. However, parasite swelling, and eventual bursting, have been suggested as the most likely mode of action (Smith et al., 1981; Chapman et al., 2010). Whether the osmotic stress survival of bacteria is influenced by PIs has to our knowledge not been shown experimentally.
The specific activity of PIs is influenced by the extracellular conditions. Extracellular cation concentrations promoting the natural electrochemical gradients across the cytoplasm (high [Na+], [H+], [Ca2+]) enhances the activity of ionophores, whereas extracellular cation concentrations equilibrating the intracellular levels (high [K+]) decrease the ionophore-activities (Dawson and Boling, 1987; Russell et al., 1988; Chow and Russell, 1992; Van Kessel and Russell, 1992; Wollesen et al., 2023). Considering that the cation concentrations and pH varies along the length of the gastrointestinal tract of animals, it is likely that the antimicrobial effect of PIs differs in different parts of the gastrointestinal tract, and thereby exert different selection pressures on the local microbiota.
Antimicrobial resistance is the ability of bacteria to survive and grow in the presence of antimicrobials (Balaban et al., 2019). Since polyether ionophores have not been used in human medicine, clinical cut-off values have not been established. When we discuss resistance to polyether ionophores in this review, we refer to survival and growth of: (1) a particular strain of bacteria at PI concentrations to which it was previously susceptible, (2) a species at concentrations above the epidemiological cut-off values for that species, or (3) a species at the highest concentration of PI tested in susceptibility assays in vitro (intrinsic resistance).
Resistance mechanisms in bacteria are attributed to either intrinsic resistance, where all individuals of a certain type of bacteria can survive and grow in the presence of a specific antimicrobial, or acquired resistance that can arise in a previously susceptible population due to mutations or horizontal transfer of resistance genes. In addition, bacteria can survive high concentrations of antimicrobials due to the formation of persister cells or biofilms. Persister cells are subpopulations of a species with a different physiology (metabolically quiescent) compared to the general bacterial population. Biofilms are bacterial communities embedded in an extracellular matrix consisting of physiologically diverging subpopulations of bacteria (Olivares et al., 2013).
Although bacterial resistance to PIs was described 30 years ago, the mechanism(s) of resistance are poorly understood. Table 2 summarizes the currently known putative and confirmed bacterial resistance mechanisms.
Gram-negative bacteria generally display intrinsic resistance to PIs due to the nature of their cell envelope. The outer membrane of gram-negative bacteria is impenetrable to many macromolecules and allows passage of solutes through porins. Porins are hydrophilic channels embedded in the outer membrane with a size exclusion limit of approximately 600 daltons. Ionophores are highly lipophilic and in general larger than 600 daltons, making them unable to pass through the porins and the negatively charged LPS of the outer membrane (Nagaraja, 1995).
Intrinsic resistance to PIs is not universal to all gram-negative bacteria. Certain strains of Bacteroides, Fibrobacter, and Prevotella belonging to the ruminal microbiota were sensitive to monensin when grown in vitro (Chen and Wolin, 1979; Newbold et al., 1993; Callaway and Russell, 1999, 2000). Some sensitive strains developed resistance after exposure to sub-lethal monensin concentrations through unknown mechanisms, while others remained sensitive (Callaway and Russell, 1999, 2000). Prevotella ruminicola grown in the presence of increasing concentrations of tetronasin developed resistance to tetronasin, lasalocid, and monensin and to a lesser extent to the glycopeptide avoparcin. The resistant mutants did not lose the resistance phenotype after subculturing in the absence of ionophores and bound less radioactively labeled ionophore. Reduced metabolism of tetraphenylalanine (Mr = 607), but unaffected metabolism of triphenylalanine (Mr = 460), indicated reduced penetration through the outer membrane, and reduced porin exclusion limit was suggested as the mechanism of resistance (Newbold and Wallace, 1989).
In contrast to gram-negative bacteria, gram-positive bacteria do not possess a protective outer membrane. Although the outer peptidoglycan layer of gram-positive bacteria can be relatively thick, it is porous and permits diffusion of small molecules, and this allows for the lipophilic ionophores to readily dissolve into the cell membrane of gram-positive bacteria (Rutkowski and Brzezinski, 2013).
Although the biological role of PI production is not yet established (Bakker, 1979; Kevin et al., 2009), it is likely that the antibacterial activity of ionophores improves the competitiveness of PI secreting bacteria in their habitats. The secretion of ionophores into the environment suggests that PI producing bacteria concomitantly express protective mechanisms of self-resistance. A self-resistance mechanism against the PI tetronasin was identified in 1994 by screening of a genomic library from the tetronasin-resistant Strepmomyces longisporoflavus in the tetronasin-susceptible species Streptomyces lividans (Linton et al., 1994). Tetronasin-resistance was associated with a DNA region containing the tnrB2/B3 operon encoding an ATP-binding cassette (ABC) transporter consisting of ATPase (TnrB2) and permease (TnrB3) subunits. Later, the presence of plasmid-encoded homologs of tnrB2/B3 were identified in Enterococcus faecium isolates from Swedish and Norwegian poultry, and the presence of these genes on large mobile plasmids correlated with resistance to narasin (Nilsson et al., 2016). Naemi et al. (2020) cloned the putative narasin resistance genes, coined narAB, into a cloning vector under control of its natural promoter and unequivocally showed that this operon was sufficient to confer resistance to the PIs narasin, salinomycin and maduramicin, but not to monensin. Interestingly, Naemi et al. also showed that the narAB operon was transcriptionally upregulated by exposure to narasin, which potentially reduces the fitness cost associated with carrying the operon in PI-free conditions.
In E. faecium isolated from broilers in Norway (Sletvold et al., 2007, 2008, 2010), Sweden (Nilsson et al., 2016), Denmark (Leinweber et al., 2018), and the Netherlands (Pikkemaat et al., 2022), the narAB operon was located on plasmids belonging to the broad-host-range inc18-group (Gilmore et al., 2014). Inc18 plasmids are widespread in isolates from the environment, the clinic, and domestic animals (Kohler et al., 2018). They are naturally occurring in streptococci and enterococci, often carry resistance genes, and have been shown to be transferable from enterococci to staphylococci (Kohler et al., 2018). The assembled NarAB-encoding plasmids published to date, vary in size and carry mobile elements such as transposons and insertion sequences. These plasmids share only limited gene synteny. However, the narAB operon is often associated with a full or truncated ω-ε-ζ toxin-antitoxin system and flanked by insertion sequences, such as IS1216 (Sletvold et al., 2007, 2008, 2010; Leinweber et al., 2018). Filter mating experiments demonstrated that NarAB encoding plasmids were transferable between E. faecium strains despite the lack of apparent plasmid encoded transfer systems (Dahl et al., 2007; Nilsson et al., 2012; Leinweber et al., 2018; Naemi et al., 2020). Leinweber et al. (2018) observed that the NarAB encoding plasmid was transferred by conjugation along with a larger co-residing conjugative plasmid suggesting that the larger plasmid acted as a helper plasmid. In Dutch E. faecalis isolates, narAB was most often localized on large plasmids of the RepA_N family (Pikkemaat et al., 2022). RepA_N plasmids display a relatively broad distribution but appear to be adapted to their host and display restricted transferability to other species (Weaver et al., 2009).
The resistance mechanism(s) of NarAB and TnrB2/B3 have not yet been characterized. The ABC transporter superfamily is an ancient family of membrane transporters that utilizes the energy released from hydrolysis of ATP to drive transport of substrates against a concentration gradient. ABC-transporters transport a wide range of substrates including ions, nutrients, xenobiotics, and secondary metabolites, including antibiotics. It is obvious to assume that NarAB and other TnrB2/B3 homologs function as drug efflux proteins, but this has not yet been proven experimentally.
The origin of narAB is not known. The occurrence of narAB in certain subpopulations of enterococci and the general localization of this operon on mobilizable plasmids suggests acquisition by horizontal gene transfer. The similar function of NarAB and TnrB2/B3 suggests that NarAB may have originated as a self-resistance mechanism in PI-producing bacteria. However, the permease subunit NarB (Accession: QHA94815.1) displays 33% identity to TnrB3 (Accession: CAA52013.1) of Strepmomyces longisporoflavus and 30% identity to a putative self-resistance gene (Accession: WP_030553101.1) of the narasin producing Kitasatospora aureofaciens (our unpublished data). This suggests that if the gene originates from horizontal gene transfer from PI-producing bacteria it would have been an early event predating the use of ionophores in animal husbandry. Alternatively, narAB originates from a so far unidentified bacterium, and it cannot be excluded that it has divergently evolved from an operon that is intrinsic to a species of enterococci.
In a screen for natural compounds displaying in vitro anti-mycobacterial activity, Huang et al. (2017) identified the PIs nigericin, calcimycin (A23187), and salinomycin as hits. Spontaneous mutants of Mycobacterium spp. resistant to nigericin and calcimycin were isolated on selective plates. Deleterious mutations in a tetR family regulator resulted in resistance to both PIs, while a non-synonymous mutation in nhaA resulted in resistance to nigericin. Mutation of the tetR regulator led to increased transcription of an RND family efflux pump hypothesized to export the PIs, while mutation in nhaA encoding a homolog of Na+/H+-antiporter likely compensates for a disrupted sodium gradient.
Wollesen et al. (2023) grew Staphylococcus aureus in the presence of the PI nanchangmycin with the intention to isolate mutants resistant to PIs and to identify potential resistance mechanisms. Nanchangmycin-resistant mutants carried mutations in genes encoding a potassium importer TrkH, a transcriptional activator involved in regulating autolysis SarV, and a membrane stabilizing protein MspA. Interestingly, the authors did not detect cross-resistance in these mutants to salinomycin, lasalocid, or calcimycin. To gain further insight into the resistance mechanisms, a methicillin-resistant S. aureus transposon mutant library was screened for increased sensitivity to lasalocid, salinomycin, calcimycin, and nanchangmycin. Mutations in the electron transport chain (ETC) genes qoxABC, nhd2, and cyoE, conferred a modest increase in sensitivity toward lasalocid, salinomycin, and nanchangmycin (Wollesen et al., 2023). These findings indicate a role of the ETC in PI-resistance in S. aureus, potentially to meet the high demand for energy to uphold cation homeostasis across the cell membrane.
Recently, spontaneous mutants with reduced susceptibility to monensin were isolated after growth of clinical S. aureus isolates under laboratory conditions. Mutations were found in different genes, such as apt encoding an adenine phosphoribosyltransferase, purR, encoding a repressor of nucleotide biosynthesis, and non-synonymous mutations in different genes of the mnh operon encoding a Na+/H+ antiporter (Dan I. Andersson, personal communication, March 9, 2023; published with permission). While apt and purR theoretically are involved in compensating for increased need for ATP to counteract a PI-induced cation imbalance, the mnh mutations are likely directly compensating for a disrupted transmembrane chemical gradient.
Persister cells and biofilm formation are mechanism considered to play key-roles in persistence of bacteria in different environments including the human host (chronic infections) and causing antimicrobial treatment failures (Olivares et al., 2013). Wollesen et al. (2023) analyzed the antimicrobial effect of PIs on a laboratory-induced persister phenotype and preformed biofilms of S. aureus. They observed that in general both persister cells and biofilms were more resistant to PIs compared to exponentially growing bacteria. Interestingly, persister cells were susceptible to lasalocid, and biofilms were susceptible to lasalocid, calcimycin, and nanchangmycin (Wollesen et al., 2023).
In the gram-positive bacteria Clostridium aminophilum F, Clostridium perfringens, E. faecalis, and E. faecium and in the gram-negative species Prevotella ruminants, adaptation to growth in monensin was associated with thickening of the cell wall (Callaway and Russell, 1999; Rychlik and Russell, 2002; Simjee et al., 2012). The observed increase in cell wall thickness was reversed after passage in monensin-free medium and the authors concluded that monensin resistance was due to physiological changes rather than mutations (Simjee et al., 2012). However, it should be noted that this was not confirmed by whole genome sequencing.
Since PIs are currently not utilized to combat bacterial infections in humans due to cytotoxicity, the prevalence of PI-resistance in bacterial isolates from animals has not been considered a threat to public health. However, use of PIs in animal husbandry and PI-resistance can contribute to resistance to medically important antimicrobials if PI-resistance confers cross-resistance or co-resistance to antimicrobials used to treat infections in humans. While cross-resistance occurs in a microbe carrying a resistance mechanism conferring resistance to structurally different antibiotics, co-resistance arises if genes encoding resistance mechanisms for different antimicrobials are genetically linked.
The ruminal bacterium C. aminophilum that had been adapted to grow in monensin or lasalocid, was only resistant to the cell-wall acting bacitracin out of 16 tested medically relevant antibiotics (Houlihan and Russell, 2003). Interestingly, these results are in agreement with a strong association between narasin resistance and bacitracin resistance as discovered in Enterococcus spp. isolates from broilers in Norway (NORM/NORM-VET 2004, 2005). However, it was shown that NarAB does not confer cross-resistance to bacitracin, suggesting two separate AMR mechanisms for these antimicrobials in enterococci (Naemi et al., 2020). As described above, the gram-negative bacterium P. ruminicola, adapted to grow in the presence of tetronasin, displayed a moderately reduced (65%) susceptibility to the glycopeptide avoparcin (Newbold et al., 1992). The cross-resistance between tetronasin and avoparcin was likely due to changes in the porins of the outer membrane. Some of the mutants of S. aureus that are resistant to monensin due to mutations in apt, purR, or mnh displayed minor changes in resistance to antimicrobials used to treat infections in humans (Dan I. Andersson, personal communication, March 9, 2023; published with permission). These resistance mechanisms have not been characterized in detail and a mechanism of cross-resistance to medically relevant antimicrobials have not been confirmed.
Naemi et al. (2020) tested the resistance profile of E. faecium carrying the narAB operon on a plasmid under control of the natural promoter and compared it to the resistance profile of the isogenic strain carrying the vector control. They detected no difference between the two strains in minimum inhibitory concentration (MIC) values of any of the tested medically important antimicrobials, suggesting that NarAB does not confer resistance to antimicrobials used in human medicine (Naemi et al., 2020).
Vancomycin-resistant E. faecium has regularly been isolated at low levels from broilers in Norway and Sweden using a selective isolation method (NORM/NORM-VET 2004, 2005; SWEDRES/SVARM 2013, 2014). All of these vancomycin-resistant enterococci (VRE), which carry vanA, were also resistant to narasin and carried the narAB genes (Nilsson et al., 2012; Simm et al., 2019). Filter mating experiments showed that vancomycin and narasin resistance were frequently co-transferred to a recipient (Nilsson et al., 2016; Naemi et al., 2020). Comparative genomics revealed that narAB and vanA can be physically linked on transferrable plasmids (Johnsen et al., 2005; Nilsson et al., 2016).
A study from the Netherlands on 35 E. faecium and 61 Enterococcus faecalis isolates from poultry products found statistically significant correlations between phenotypic resistance to salinomycin, tetracycline, and erythromycin in both species (Pikkemaat et al., 2022). Sequencing of a selection of 20 isolates revealed that narAB was present in all of the salinomycin-resistant isolates of both E. faecium and E. faecalis. In the E. faecalis isolates, narAB was physically linked with ermB (conferring macrolide resistance) and tet(L) and tet(M) or tet(O) (tet-genes confer resistance to tetracycline) on plasmids (Pikkemaat et al., 2022), confirming the co-occurrence of resistance genes. The correlation between salinomycin and tetracycline resistance aligns with a metagenomics study in which narasin-fed chickens were enriched for bacteria encoding tetracycline resistance genes, suggesting that narasin and tetracycline resistance are co-selected (Plata et al., 2022). Taken together these data provide strong evidence for transferrable co-resistance of PIs and medically important antimicrobials.
The worldwide prevalence of PI-resistance is challenging to assess because resistance to PIs is not reported systematically, and large-scale surveys of resistance has not been performed. However, a few countries in Europe have regularly reported on the prevalence of PI-resistance in indicator bacteria such as the opportunistic pathogens E. faecium and E. faecalis isolated from animals or animal products.
In a Dutch report from 2022, the authors analyzed the antimicrobial susceptibility of E. faecium and E. faecalis from an in-house collection of isolates gathered in the years 2013, 2016, 2018, and 2020. They reported that 31% of E. faecium and 23% of E. faecalis isolates collected from broilers and broiler products of conventionally reared poultry displayed a MIC of salinomycin >2 mg/L, while 48.6% of E. faecium and 47.5% of E. faecalis, displayed a MIC value >1 mg/L. The authors performed whole genome sequencing of a subset of the isolates and discovered that all isolates with a MIC >1 mg/L carried plasmids encoding the PI-resistance genes narAB (Pikkemaat et al., 2022). Based on this, it was suggested that the cut-off value for salinomycin defining a non-wild-type should be adjusted to >1 mg/L. The Dutch AMR monitoring program (MARAN) historically used a MIC of 4 mg/L as cut-off for resistance and reported the number of resistant isolates for each year. The proportion of resistant E. faecium and E. faecalis isolates from Dutch broilers varied between 41.3–81.7% and 3.9–34.5%, for E. faecium and E. faecalis, respectively, in the years 2004–2014 (Figure 2; MARAN, 2023). Applying a cut-off of >1 mg/L in place of >4 mg/L to the MIC results from the last MARAN report to document salinomycin resistance, increased resistance rates from 5.6 to 61.9% and 38.5 to 84.6% for E. faecalis and E. faecium, respectively (MARAN, 2014). The Danish surveillance program (DANMAP) reported during the same period that their proportion of isolates from broilers with an MIC of salinomycin >4 mg/L varied between 50 and 74.8% for E. faecium and 0–10.5% for E. faecalis (Figure 2; DANMAP, 2023). Considering the results of Pikkemaat et al. that cut-off values as low as 1 mg/L correlated with occurrence of the narAB resistance operon, the occurrence of PI-resistant enterococci in both Denmark and the Netherlands must be regarded as significantly higher than previously reported.
Figure 2. Comparison of the proportion of polyether ionophore resistant isolates from conventionally reared broilers of Enterococcus faecium and Enterococcus faecalis in four European countries sorted by year. The data were retrieved from the reports of the surveillance programs of each country (MARAN, The Netherlands; DANMAP, Denmark; SVARM, Sweden; NORM-VET, Norway). Cut-off values used were > 4 mg/L for salinomycin, and > 2 mg/L for narasin. Note that these cut-off values are higher than the values proposed to correlate with the narAB resistance genes by Pikkemaat et al. (2022) and Nilsson et al. (2016) and the results likely underestimate the true prevalence of PI resistant isolates in broiler populations.
In Norway and Sweden, narasin was used in the test-panels for surveillance of PI resistance in indicator enterococci. In Sweden, 77% of E. faecium and 27% of E. faecalis isolated from broilers in 2014 were considered narasin-resistant with MIC over the EUCAST epidemiological cut-off (ECOFF) value (>2 mg/L) (SWEDRES/SVARM 2013, 2014). The prevalence of resistant isolates varied in the years 2000–2014 with 77–93.3% for E. faecium and 22.7–44.9% for E. faecalis (Figure 2) (SWEDRES-SVARM, 2023). This is similar to the situation in Norway, where 61–91% of the E. faecium isolates from broilers were resistant to narasin in the years 2002–2014 (Figure 2) (NORM/NORM-VET, 2023). Since PIs are not currently used in human medicine, most countries have removed PIs from the AMR test panels in monitoring of indicator enterococci. However, due to a decision by the poultry industry in Norway to remove PIs as feed additives in conventional rearing of broilers in 2015, occurrence of narasin resistance in E. faecium and E. faecalis was monitored in 2018 and 2020 to follow the development after discontinuation. The occurrence of narasin-resistant E. faecium isolates was reduced from >90% in 2014 to 24.7% in 2018 and 15.6% in 2020 (NORM/NORM-VET 2018, 2019; Simm et al., 2019; NORM/NORM-VET 2020, 2021). These data strongly suggests that in-feed PIs select for PI-resistant enterococci in broilers, a conclusion that is supported by a controlled study comparing occurrence of PI-resistant enterococci in broilers fed diets with and without PIs (Simm et al., 2019).
DANMAP reported that 0 of 1,349 E. faecalis isolates and 1 of 1,217 E. faecium isolates collected from pigs between 2004 and 2013 had a MIC of salinomycin >4 mg/L (DANMAP, 2023). The Norwegian monitoring program for AMR in the veterinary sector (NORM-VET) reported that 0 and 3.2% of E. faecalis and E. faecium pig isolates were resistant to narasin (MIC >2 mg/L) in 2004, 2008, and 2009 (NORM/NORM-VET 2004, 2005; NORM/NORM-VET 2008, 2009; NORM/NORM-VET 2009, 2010). Similarly, narasin resistance was detected in only 2% of E. faecium isolates from layers in Norway in 2013 (NORM/NORM-VET 2013, 2014). Enterococcus faecium and E. faecalis collected from turkeys in Norway in 2007, 2013, and 2020 displayed 76.2 and 7.3% narasin-resistant isolates, respectively (NORM/NORM-VET 2007, 2008; NORM/NORM-VET 2013, 2014; NORM/NORM-VET 2020, 2021). PIs have not been used in rearing of pigs or layers in the sampling period, but turkeys were fed a diet containing monensin. In the Netherlands, the prevalence of salinomycin resistant enterococci has steadily declined since 2006, when use of salinomcyin was banned in rearing of pigs. These data further support that in-feed PIs select for PI-resistant bacteria.
In Norway, the glycopeptide avoparcin was used as a feed additive in broiler production between 1986 and 1995 (Borgen et al., 2000b). Vancomycin resistance provides cross resistance against avoparcin and use of avoparcin as a feed additive avidly selected for VRE in Norwegian broilers (Kruse et al., 1999). In 2000, it was still possible to isolate VRE from 99% of sampled broiler farms in Norway by plating samples directly onto agar supplemented with vancomycin (Borgen et al., 2000a). This selective method has also been used in the Swedish and Norwegian surveillance programs. Data from Norway showed decreasing detectable occurrence of VRE in broilers between 2002 and 2014 and non-detectable levels since 2018. Avoparcin has not been used in Sweden since the ban of antimicrobial growth promoters in 1986. The occurrence of VRE in Swedish broiler flocks first increased in the early 2000, reached a peak at >40% in 2005 (Nilsson et al., 2019) and has decreased since then. VRE were detected in 11% of samples from Swedish broilers in 2015 (Nilsson et al., 2019) and 6% of the samples in 2020 (SWEDRES/SVARM 2020, 2021). Corresponding data does not exist for other European countries since the national surveillance programs have not used the selective method of isolation.
Considering that all VRE in Norway and Sweden are co-resistant to narasin, the fact that VRE has not been detected in Norwegian broilers after the discontinuation of prophylactic use of in-feed narasin, and the continued detection of VRE in Swedish broilers fed narasin in the same time period, is strong evidence that narasin selects for VRE in these broiler production systems. It should be noted that there has been a continuous decline in occurrence of VRE in both Norway (between 2002 and 2014) and Sweden (between 2005 and 2020) despite use of narasin as a feed additive. This does not contradict the selection pressure of narasin for VRE co-resistant to PIs. VRE have only been detected occasionally and at very low levels by non-selective methods (0.14 and 0.75% in Sweden and Norway, respectively from 2000 to 2020). This means that VRE represent a very small proportion of the narasin resistant population of enterococci. Therefore, it is fair to assume that the apparent occurrence of VRE decreases over time even under narasin selection pressure as long as VRE are not re-introduced into the broiler production system.
One in 50 E. faecium isolates from healthy human volunteers sampled in Denmark in 2005 displayed an MIC of salinomycin >4 mg/L (DANMAP 2005, 2006). Interestingly, one isolate from the same material was also vancomycin resistant. Considering that all VRE isolated from broilers in Norway are also PI-resistant, it is tempting to speculate that the VRE isolate from healthy humans in Denmark was the same isolate that was salinomycin resistant. Furthermore, in depth analysis of WGS data from three large collections of enterococci reveals that narAB is also found in human isolates, including clinical isolates, though at low prevalence (Gouliouris et al., 2018; Arredondo-Alonso et al., 2020; Pöntinen et al., 2021). This clearly shows that even though PIs have never been used in human medicine, PI resistant isolates can colonize humans and cause invasive infections.
Several reports have demonstrated the temporary colonization of human intestine with antimicrobial-resistant E. faecium and E. faecalis transmitted by direct or indirect animal contact or by meat consumption (Bortolaia and Guardabassi, 2015) including narasin-resistant VRE (Johnsen et al., 2005; Sørum et al., 2006). Such temporary colonization could allow for transfer of antimicrobial resistance genes from isolates of animal origin to bacteria in the human host, such as in the case of vanA-encoding E. faecium (Lester et al., 2006). Plasmids encoding narasin and vancomycin resistance have been shown to transfer readily from poultry derived VRE to human isolates of E. faecium in a mouse model (Dahl et al., 2007). Transfer was observed within a day after inoculation of the donor strain. Plasmids from enterococci can also spread to other human pathogens. Experimental transfer of vancomycin resistance from E. faecium to S. aureus has for instance been demonstrated on mouse skin (Noble et al., 1992). Clinical isolates of vancomycin-resistant S. aureus (VRSA) from humans are often accompanied by VRE (Cong et al., 2020) and genomic comparisons have demonstrated that the vanA locus can transfer from plasmids of VRE and be stably integrated into the chromosome of S. aureus to create VRSA (Haas et al., 2023). This demonstrates that temporary colonization of humans by resistant bacteria of animal origin can pose a threat to human health even if the animal derived strain does not stably colonize the human host or cause infection in humans.
High prevalence of PI-resistance has mostly been documented for the indicator bacteria and prevalent nosocomial opportunistic pathogens, E. faecium and E. faecalis, collected from farm animals in Scandinavia and the Netherlands. However, PI resistance is likely globally widespread in enterococci from animals fed diets supplemented with PIs, and potentially also present in other opportunistic pathogens, such as S. aureus. So, although the available evidence does not indicate a major transmission of PI resistant bacteria from poultry to humans, transmission does occur and may be more pronounced in countries with practices for animal husbandry that differ from the regulations set by the European Union.
There are still major knowledge gaps on the risks associated with PI resistance for human health: (1) The global consumption level of PIs and hence the potential selection pressure for PI-resistance in different parts of the world are unknown since data on use of PIs in animal husbandry is not reported in most countries; (2) The frequency of PI resistance among isolates from human infections is unknown since these isolates are rarely tested for PI susceptibility; (3) The carriage rate of PI-resistant bacteria in humans is unknown. The proportion of PI-resistant bacteria in a microbiota not exposed to a selection pressure may be low and selective identification methods are required to assess the prevalence of PI resistance; (4) The distribution of PI resistance and the potential for dissemination of the resistance mechanism(s) among human pathogens are unknown. PI-resistance has mainly been tested in gram-positive indicator bacteria (enterococci) from production animals. Systematic studies from different environments and different parts of the world are needed to properly assess the risks; (5) The PI resistance mechanism(s) must be identified and characterized in detail and the full complement of resistance mechanisms to medically important antimicrobials that exist on PI-resistance plasmids should be determined.
Bacteria can become resistant to PIs through horizontal gene transfer of resistance genes and by mutations of intrinsic genes. So far, none of the putative resistance mechanisms have been characterized in detail. However, accumulating evidence suggests that several mechanisms can confer resistance to PIs, including efflux of PIs from the cell, upregulation of cation transporters counteracting the action of the PI and reduced permeability of PIs into the cell. The narAB operon is localized on transferrable plasmids in the human opportunistic pathogens E. faecium and E. faecalis. These plasmids have been shown to carry various resistance mechanisms to antimicrobials used in human medicine. Use of PIs in rearing of production animals provides a selection pressure that promotes expansion of a PI-resistant population of bacteria and persistence of co-localized resistance mechanisms. PI-resistant bacteria can colonize humans and cause invasive infections and the PI resistance plasmids can spread in bacterial populations, both in vitro and in vivo. Therefore, there is a potential risk associated with the use of in-feed PIs, though more research is needed to explore this further to be able to conduct a thorough risk assessment with a One Health perspective.
RF: Conceptualization, Data curation, Formal analysis, Investigation, Methodology, Visualization, Writing – original draft, Writing – review & editing. JS: Writing – review & editing, Conceptualization. SG: Writing – review & editing, Conceptualization. KL: Supervision, Writing – review & editing, Conceptualization. MP: Conceptualization, Data curation, Funding acquisition, Investigation, Methodology, Project administration, Writing – review & editing. AU: Conceptualization, Data curation, Formal analysis, Funding acquisition, Investigation, Methodology, Project administration, Supervision, Visualization, Writing – original draft, Writing – review & editing. RS: Conceptualization, Data curation, Formal analysis, Funding acquisition, Investigation, Methodology, Project administration, Supervision, Visualization, Writing – original draft, Writing – review & editing.
The author(s) declare financial support was received for the research, authorship, and/or publication of this article. This project received funding from the Norwegian Research Council under grant number 333858 and the Netherlands Organization for Health Research and Development ZonMw under grant number 1070132110001 under the umbrella of the JPIAMR—Joint Programming Initiative on Antimicrobial Resistance.
The authors declare that the research was conducted in the absence of any commercial or financial relationships that could be construed as a potential conflict of interest.
All claims expressed in this article are solely those of the authors and do not necessarily represent those of their affiliated organizations, or those of the publisher, the editors and the reviewers. Any product that may be evaluated in this article, or claim that may be made by its manufacturer, is not guaranteed or endorsed by the publisher.
Antonenko, Y. N., and Yaguzhinsky, L. S. (1988). The ion selectivity of nonelectrogenic ionophores measured on a bilayer lipid membrane: nigericin, monensin, A23187 and lasalocid a. BBA Biomembr. 938, 125–130. doi: 10.1016/0005-2736(88)90151-4
Arredondo-Alonso, S., Top, J., McNally, A., Puranen, S., Pesonen, M., Pensar, J., et al. (2020). Plasmids shaped the recent emergence of the major nosocomial pathogen Enterococcus faecium. MBio 11, e03284–e03219. doi: 10.1128/mBio.03284-19
Bakker, E. P. (1979). “Ionophore antibiotics” in Mechanism of Action of Antibacterial Agents. ed. F. E. Hahn (Heidelberg: Springer-Verlag Berlin)
Balaban, N. Q., Helaine, S., Lewis, K., Ackermann, M., Aldridge, B., Andersson, D. I., et al. (2019). Definitions and guidelines for research on antibiotic persistence. Nat. Rev. Microbiol. 17, 441–448. doi: 10.1038/s41579-019-0196-3
Borgen, K., Simonsen, G. S., Sundsfjord, A., Wasteson, Y., Olsvik, O., and Kruse, H. (2000a). Continuing high prevalence of VanA-type vancomycin-resistant enterococci on Norwegian poultry farms three years after avoparcin was banned. J. Appl. Microbiol. 89, 478–485. doi: 10.1046/j.1365-2672.2000.01137.x
Borgen, K., Sørum, M., Kruse, H., and Wasteson, Y. (2000b). Persistence of vancomycin-resistant enterococci (VRE) on Norwegian broiler farms. FEMS Microbiol. Lett. 191, 255–258. doi: 10.1016/S0378-1097(00)00399-2
Bortolaia, V., and Guardabassi, L. (2015). “Zoonotic transmission of antimicrobial resistant enterococci: a threat to public health or an overemphasised risk?” in Zoonoses-infections affecting humans and animals: Focus on public health aspects.
Callaway, T. R., Edrington, T. S., Rychlik, J. L., Genovese, K. J., Poole, T. L., Jung, Y. S., et al. (2003). Ionophores: their use as ruminant growth promotants and impact on food safety. Curr. Issues Intest. Microbiol. 4, 43–51.
Callaway, T. R., and Russell, J. B. (1999). Selection of a highly monensin-resistant Prevotella bryantii subpopulation with altered outer membrane characteristics. Appl. Environ. Microbiol. 65, 4753–4759. doi: 10.1128/AEM.65.11.4753-4759.1999
Callaway, T. R., and Russell, J. B. (2000). Variations in the ability of ruminal gram-negative Prevotella species to resist monensin. Curr. Microbiol. 40, 185–189. doi: 10.1007/s002849910037
Caughey, B., Painter, G. R., and Gibbson, W. A. (1986). Equilibrium cation binding selectivity of the carboxylic ionophore narasin a: a comparison with transport selectivities reported in two biological test systems. Biochem. Pharmacol. 35, 4103–4105. doi: 10.1016/0006-2952(86)90035-3
Chapman, H. D., Jeffers, T. K., and Williams, R. B. (2010). Forty years of monensin for the control of coccidiosis in poultry. Poult. Sci. 89, 1788–1801. doi: 10.3382/ps.2010-00931
Chen, G., and Russell, J. B. (1989). More monensin-sensitive, ammonia-producing bacteria from the rumen. Appl. Environ. Microbiol. 55, 1052–1057. doi: 10.1128/aem.55.5.1052-1057.1989
Chen, M., and Wolin, M. J. (1979). Effect of monensin and lasalocid-sodium on the growth of methanogenic and rumen saccharolytic bacteria. Appl. Environ. Microbiol. 38, 72–77. doi: 10.1128/aem.38.1.72-77.1979
Chow, J. M., and Russell, J. B. (1992). Effect of pH and Monensin on glucose transport by Fibrobacter succinogenes, a cellulolytic ruminal bacterium. Appl. Environ. Microbiol. 58, 1115–1120. doi: 10.1128/aem.58.4.1115-1120.1992
Cong, Y., Yang, S., and Rao, X. (2020). Vancomycin resistant Staphylococcus aureus infections: a review of case updating and clinical features. J. Adv. Res. 21, 169–176. doi: 10.1016/j.jare.2019.10.005
Dahl, K. H., Mater, D. D. G., Flores, M. J., Johnsen, P. J., Midtvedt, T., Corthier, G., et al. (2007). Transfer of plasmid and chromosomal glycopeptide resistance determinants occurs more readily in the digestive tract of mice than in vitro and exconjugants can persist stably in vivo in the absence of glycopeptide selection. J. Antimicrob. Chemother. 59, 478–486. doi: 10.1093/jac/dkl530
DANMAP (2023). Use of antimicrobial agents and occurrence of antimicrobial resistance in bacteria from food animals, food and humans in Denmark. Available at: https://www.danmap.org/reports (Accessed November 15, 2023).
DANMAP 2005 (2006). Use of antimicrobial agents and occurrence of antimicrobial resistance in bacteria from food animals, foods and humans in Denmark. ISSN 1600-2032.
DANMAP 2015 (2016). Use of antimicrobial agents and occurrence of antimicrobial resistance in bacteria from food animals, food and humans in Denmark.
Dawson, K. A., and Boling, J. A. (1987). Effects of potassium ion concentrations on the antimicrobial activities of ionophores against ruminal anaerobes. Appl. Environ. Microbiol. 53, 2363–2367. doi: 10.1128/aem.53.10.2363-2367.1987
EUR-lex (2023). Access to European Union law. Available at: https://eur-lex.europa.eu/homepage.html (Accessed January 2, 2023).
FDA (2023a). 2022 summary report on antimicrobials sold or distributed for use in food-producing animals. FDA. Available at: https://www.fda.gov/animal-veterinary/antimicrobial-resistance/2022-summary-report-antimicrobials-sold-or-distributed-use-food-producing-animals (Accessed January 6, 2024).
FDA (2023b). Animal Drugs @ FDA. Available at: https://animaldrugsatfda.fda.gov (Accessed January 2, 2023).
Gilmore, M. S., Clewell, D. B., Ike, Y., and Shankar, N. eds. (2014). Enterococci: From Commensals to Leading Causes of Drug Resistant Infection. Boston: Massachusetts Eye and Ear Infirmary.
Gouliouris, T., Raven, K. E., Ludden, C., Blane, B., Corander, J., Horner, C. S., et al. (2018). Genomic surveillance of Enterococcus faecium reveals limited sharing of strains and resistance genes between livestock and humans in the United Kingdom. MBio 9:e01780. doi: 10.1128/mBio.01780-18
Gräfe, U., Reinhardt, G., and Miosga, N. (1989). Monovalent cation specificity of passive transport mediated by laidlomycin and 26-deoxylaidlomycin. J. Basic Microbiol. 29, 391–394. doi: 10.1002/jobm.3620290620
Haas, W., Singh, N., Lainhart, W., Mingle, L., Nazarian, E., Mitchell, K., et al. (2023). Genomic analysis of vancomycin-resistant Staphylococcus aureus isolates from the 3rd case identified in the United States reveals chromosomal integration of the vanA locus. Microbiol. Spectr. 11:e0431722. doi: 10.1128/spectrum.04317-22
Hansen, M., Krogh, K. A., Björklund, E., Halling-Sørensen, B., and Brandt, A. (2009). Environmental risk assessment of ionophores. TrAC Trends Anal. Chem. 28, 534–542. doi: 10.1016/j.trac.2009.02.015
Houlihan, A. J., and Russell, J. B. (2003). The susceptibility of ionophore-resistant Clostridium aminophilum F to other antibiotics. J. Antimicrob. Chemother. 52, 623–628. doi: 10.1093/jac/dkg398
Huang, W., Briffotaux, J., Wang, X., Liu, L., Hao, P., Cimino, M., et al. (2017). Ionophore A23187 shows anti-tuberculosis activity and synergy with tebipenem. Tuberculosis 107, 111–118. doi: 10.1016/j.tube.2017.09.001
Huczyński, A. (2012). Polyether ionophores—promising bioactive molecules for cancer therapy. Bioorg. Med. Chem. Lett. 22, 7002–7010. doi: 10.1016/j.bmcl.2012.09.046
Johnsen, P. J., Østérhus, J. I., Sletvold, H., Sørum, M., Kruse, H., Nielsen, K., et al. (2005). Persistence of animal and human glycopeptide-resistant enterococci on two Norwegian poultry farms formerly exposed to avoparcin is associated with a widespread plasmid-mediated vanA element within a polyclonal Enterococcus faecium population. Appl. Environ. Microbiol. 71, 159–168. doi: 10.1128/AEM.71.1.159-168.2005
Kaushik, V., Yakisich, J. S., Kumar, A., Azad, N., and Iyer, A. K. V. (2018). Ionophores: potential use as anticancer drugs and Chemosensitizers. Cancers 10:360. doi: 10.3390/cancers10100360
Kevin, D. A., Meujo, D. A. F., and Hamann, M. T. (2009). Polyether ionophores: broad-spectrum and promising biologically active molecules for the control of drug-resistant bacteria and parasites. Expert Opin. Drug Discovery 4, 109–146. doi: 10.1517/17460440802661443
Kim, M., Eastridge, M. L., and Yu, Z. (2014). Investigation of ruminal bacterial diversity in dairy cattle fed supplementary monensin alone and in combination with fat, using pyrosequencing analysis. Can. J. Microbiol. 60, 65–71. doi: 10.1139/cjm-2013-0746
Kohler, V., Vaishampayan, A., and Grohmann, E. (2018). Broad-host-range Inc18 plasmids: occurrence, spread and transfer mechanisms. Plasmid 99, 11–21. doi: 10.1016/j.plasmid.2018.06.001
Kruse, H., Johansen, B. K., Rørvik, L. M., and Schaller, G. (1999). The use of avoparcin as a growth promoter and the occurrence of vancomycin-resistant Enterococcus species in Norwegian poultry and swine production. Microb. Drug Resist. 5, 135–139. doi: 10.1089/mdr.1999.5.135
Leinweber, H., Alotaibi, S. M. I., Overballe-Petersen, S., Hansen, F., Hasman, H., Bortolaia, V., et al. (2018). Vancomycin resistance in Enterococcus faecium isolated from Danish chicken meat is located on a pVEF4-like plasmid persisting in poultry for 18 years. Int. J. Antimicrob. Agents 52, 283–286. doi: 10.1016/j.ijantimicag.2018.03.019
Lester, C. H., Frimodt-Møller, N., Sørensen, T. L., Monnet, D. L., and Hammerum, A. M. (2006). In vivo transfer of the vanA resistance gene from an Enterococcus faecium isolate of animal origin to an E. faecium isolate of human origin in the intestines of human volunteers. Antimicrob. Agents Chemother. 50, 596–599. doi: 10.1128/AAC.50.2.596-599.2006
Lin, S., Liu, H., Svenningsen, E. B., Wollesen, M., Jacobsen, K. M., Andersen, F. D., et al. (2021). Expanding the antibacterial selectivity of polyether ionophore antibiotics through diversity-focused semisynthesis. Nat. Chem. 13, 47–55. doi: 10.1038/s41557-020-00601-1
Linton, K. J., Cooper, H. N., Hunter, L. S., and Leadlay, P. F. (1994). An ABC-transporter from Streptomyces longisporoflavus confers resistance to the polyether-ionophore antibiotic tetronasin. Mol. Microbiol. 11, 777–785. doi: 10.1111/j.1365-2958.1994.tb00355.x
Liu, C. M., Hermann, T. E., Downey, A., Prosser, B. L., Schildknecht, E., Palleroni, N. J., et al. (1983). Novel polyether antibiotics X-14868A, B, C, and D produced by a Nocardia. Discovery, fermentation, biological as well as ionophore properties and taxonomy of the producing culture. J. Antibiot. 36, 343–350. doi: 10.7164/antibiotics.36.343
MARAN (2014). Monitoring of antimicrobial resistance and antibiotic usage in animals in the Netherlands in 2013. 1–168.
MARAN (2023). Monitoring of antimicrobial resistance and antibiotic usage in animals in the Netherlands. Available at: https://www.wur.nl/en/dossiers/file/antibiotic-resistance-1.htm/ (Accessed November 15, 2023).
Martins, R. R., Silva, L. J. G., Pereira, A. M. P. T., Esteves, A., Duarte, S. C., and Pena, A. (2022). Coccidiostats and poultry: a comprehensive review and current legislation. Foods 11:2738. doi: 10.3390/foods11182738
Mulchandani, R., Wang, Y., Gilbert, M., and Van Boeckel, T. P. (2023). Global trends in antimicrobial use in food-producing animals: 2020 to 2030. PLOS Glob. Public Health 3:e0001305. doi: 10.1371/journal.pgph.0001305
Murray, C. J. L., Ikuta, K. S., Sharara, F., Swetschinski, L., Robles Aguilar, G., Gray, A., et al. (2022). Global burden of bacterial antimicrobial resistance in 2019: a systematic analysis. Lancet 399, 629–655. doi: 10.1016/S0140-6736(21)02724-0
Naemi, A. O., Dey, H., Kiran, N., Sandvik, S. T., Slettemeås, J. S., Nesse, L. L., et al. (2020). NarAB is an ABC-type transporter that confers resistance to the polyether Ionophores Narasin, Salinomycin, and Maduramicin, but not Monensin. Front. Microbiol. 11:104. doi: 10.3389/fmicb.2020.00104
Nagaraja, T. G. (1995). “Ionophores and antibiotics in ruminants” in Biotechnology in Animal Feeds and Animal Feeding. Eds. R. John Wallace and A. Chesson (Hoboken, NJ, USA: John Wiley & Sons, Ltd.) 173–204.
Newbold, C. J., and Wallace, R. J. (1989). Changes in the rumen bacterium, Bacteroides ruminicola, grown in the presence of the Ionophore, Tetronasin. Asian Australas. J. Anim. Sci. 2, 452–453. doi: 10.5713/ajas.1989.452
Newbold, C. J., Wallace, R. J., and Walker, N. D. (1993). The effect of tetronasin and monensin on fermentation, microbial numbers and the development of ionophore-resistant bacteria in the rumen. J. Appl. Bacteriol. 75, 129–134. doi: 10.1111/j.1365-2672.1993.tb02757.x
Newbold, C. J., Wallace, R. J., and Watt, N. D. (1992). Properties of ionophore-resistant Bacteroides rurninicola enriched by cultivation in the presence of tetronasin. J. Appl. Bacteriol. 72, 65–70. doi: 10.1111/j.1365-2672.1992.tb04883.x
Nilsson, O., Alm, E., Greko, C., and Bengtsson, B. (2019). The rise and fall of a vancomycin-resistant clone of Enterococcus faecium among broilers in Sweden. J. Glob. Antimicrob. Resist. 17, 233–235. doi: 10.1016/j.jgar.2018.12.013
Nilsson, O., Greko, C., Bengtsson, B., and Englund, S. (2012). Genetic diversity among VRE isolates from Swedish broilers with the coincidental finding of transferrable decreased susceptibility to narasin. J. Appl. Microbiol. 112, 716–722. doi: 10.1111/j.1365-2672.2012.05254.x
Nilsson, O., Myrenås, M., and Ågren, J. (2016). Transferable genes putatively conferring elevated minimum inhibitory concentrations of narasin in Enterococcus faecium from Swedish broilers. Vet. Microbiol. 184, 80–83. doi: 10.1016/j.vetmic.2016.01.012
Noble, W. C., Virani, Z., and Cree, R. G. A. (1992). Co-transfer of vancomycin and other resistance genes from Enterococcus faecalis NCTC 12201 to Staphylococcus aureus. FEMS Microbiol. Lett. 93, 195–198. doi: 10.1016/0378-1097(92)90528-V
NORM/NORM-VET (2023). Usage of antimicrobial agents and Occurence of antimicrobial resistance in Norway. Available at: https://www.vetinst.no/en/surveillance-programmes/norm-norm-vet-report (Accessed November 15, 2023).
NORM/NORM-VET 2004 (2005). Usage of antimicrobial agents and occurrence of antimicrobial resistance in Norway. Tromsø/Oslo 2005. ISSN: 1502–2307 (print)/1890–9965 (electronic).
NORM/NORM-VET 2007 (2008). Usage of antimicrobial agents and occurrence of antimicrobial resistance in Norway. Tromsø/Oslo 2008. ISSN: 1502–2307 (print)/1890–9965 (electronic).
NORM/NORM-VET 2008 (2009). “Usage of antimicrobial agents and occurrence of antimicrobial resistance in Norway” in Tromsø / Oslo 2009. ISSN:1502–2307 (print) / 1890–9965 (electronic).
NORM/NORM-VET 2009 (2010). Usage of antimicrobial agents and occurrence of antimicrobial resistance in Norway. Tromsø/Oslo 2010. ISSN: 1502–2307 (print)/1890–9965 (electronic).
NORM/NORM-VET 2013 (2014). Usage of antimicrobial agents and occurrence of antimicrobial resistance in Norway. Tromsø/Oslo 2014. ISSN: 1502–2307 (print)/1890–9965 (electronic).
NORM/NORM-VET 2018 (2019). Usage of antimicrobial agents and occurrence of antimicrobial resistance in Norway. Tromsø/Oslo 2019. ISSN:1502–2307 (print)/1890–9965 (electronic), 1–141.
NORM/NORM-VET 2020 (2021). Usage of antimicrobial agents and occurrence of antimicrobial resistance in Norway. Tromsø/Oslo 2021. ISSN: 1502–2307 (print)/1890–9965 (electronic), 1–153
Olivares, J., Bernardini, A., Garcia-Leon, G., Corona, F., Sanchez, M. B., and Martinez, J. L. (2013). The intrinsic resistome of bacterial pathogens. Front. Microbiol. 4:103. doi: 10.3389/fmicb.2013.00103
PHAC (2016). Canadian antimicrobial resistance surveillance system—report 2016. Available at: https://www.canada.ca/fr/sante-publique/services/publications/medicaments-et-produits-sante/systeme-canadien-surveillance-resistance-antimicrobiens-rapport-2022.html (Accessed January 6, 2024).
Pikkemaat, M. G., Rapallini, M. L. B. A., Stassen, J. H. M., Alewijn, M., and Wullings, B. A. (2022). Ionophore resistance and potential risk of ionophore driven co-selection of clinically relevant antimicrobial resistance in poultry (Report / Wageningen Food Safety Research; No. WFSR 2022.005). Wageningen Food Safety Res. 1–34. doi: 10.18174/565488
Plata, G., Baxter, N. T., Susanti, D., Volland-Munson, A., Gangaiah, D., Nagireddy, A., et al. (2022). Growth promotion and antibiotic induced metabolic shifts in the chicken gut microbiome. Commun. Biol. 5:293. doi: 10.1038/s42003-022-03239-6
Pöntinen, A. K., Top, J., Arredondo-Alonso, S., Tonkin-Hill, G., Freitas, A. R., Novais, C., et al. (2021). Apparent nosocomial adaptation of Enterococcus faecalis predates the modern hospital era. Nat. Commun. 12:1523. doi: 10.1038/s41467-021-21749-5
Pressman, B. C. (1968). Ionophorous antibiotics as models for biological transport. Fed. Proc. 27, 1283–1288.
Rokitskaya, T. I., Firsov, A. M., Khailova, L. S., Kotova, E. A., and Antonenko, Y. N. (2023). Selectivity of cation transport across lipid membranes by the antibiotic salinomycin. Biochim. Biophys. Acta Biomembr. 1865:184182. doi: 10.1016/j.bbamem.2023.184182
Russell, J. B. (1987). A proposed mechanism of monensin action in inhibiting ruminal bacterial growth: effects on ion flux and protonmotive force. J. Anim. Sci. 64, 1519–1525. doi: 10.2527/jas1987.6451519x
Russell, J. B., and Strobel, H. J. (1989). Effect of Ionophores on ruminal fermentation. Appl. Environ. Microbiol. 55, 1–6. doi: 10.1128/aem.55.1.1-6.1989
Russell, J. B., Strobel, H. J., and Chen, G. J. (1988). Enrichment and isolation of a ruminal bacterium with a very high specific activity of ammonia production. Appl. Environ. Microbiol. 54, 872–877. doi: 10.1128/aem.54.4.872-877.1988
Russell, J. B., Strobel, H. J., and Martin, S. A. (1990). Strategies of nutrient transport by ruminal bacteria. J. Dairy Sci. 73, 2996–3012. doi: 10.3168/jds.S0022-0302(90)78987-4
Rutkowski, J., and Brzezinski, B. (2013). Structures and properties of naturally occurring polyether antibiotics. Biomed. Res. Int. 2013:162513. doi: 10.1155/2013/162513
Rychlik, J. L., and Russell, J. B. (2002). The adaptation and resistance of Clostridium aminophilum F to the butyrivibriocin-like substance of Butyrivibrio fibrisolvens JL5 and monensin. FEMS Microbiol. Lett. 209, 93–98. doi: 10.1111/j.1574-6968.2002.tb11115.x
Scharen, M., Drong, C., Kiri, K., Riede, S., Gardener, M., Meyer, U., et al. (2017). Differential effects of monensin and a blend of essential oils on rumen microbiota composition of transition dairy cows. J. Dairy Sci. 100, 2765–2783. doi: 10.3168/jds.2016-11994
Simjee, S., Heffron, A. L., Pridmore, A., and Shryock, T. R. (2012). Reversible monensin adaptation in Enterococcus faecium, Enterococcus faecalis and Clostridium perfringens of cattle origin: potential impact on human food safety. J. Antimicrob. Chemother. 67, 2388–2395. doi: 10.1093/jac/dks236
Simm, R., Slettemeås, J. S., Norström, M., Dean, K. R., Kaldhusdal, M., and Urdahl, A. M. (2019). Significant reduction of vancomycin resistant E. faecium in the Norwegian broiler population coincided with measures taken by the broiler industry to reduce antimicrobial resistant bacteria. PLoS One 14:e0226101. doi: 10.1371/journal.pone.0226101
Sletvold, H., Johnsen, P. J., Hamre, I., Simonsen, G. S., Sundsfjord, A., and Nielsen, K. M. (2008). Complete sequence of Enterococcus faecium pVEF3 and the detection of an ω-ε-ζ toxin-antitoxin module and an ABC transporter. Plasmid 60, 75–85. doi: 10.1016/j.plasmid.2008.04.002
Sletvold, H., Johnsen, P. J., Simonsen, G. S., Aasnæs, B., Sundsfjord, A., and Nielsen, K. M. (2007). Comparative DNA analysis of two vanA plasmids from Enterococcus faecium strains isolated from poultry and a poultry farmer in Norway. Antimicrob. Agents Chemother. 51, 736–739. doi: 10.1128/AAC.00557-06
Sletvold, H., Johnsen, P. J., Wikmark, O.-G., Simonsen, G. S., Sundsfjord, A., and Nielsen, K. M. (2010). Tn1546 is part of a larger plasmid-encoded genetic unit horizontally disseminated among clonal Enterococcus faecium lineages. J. Antimicrob. Chemother. 65, 1894–1906. doi: 10.1093/jac/dkq219
Smith, C. K., and Galloway, R. B. (1983). Influence of monensin on cation influx and glycolysis of Eimeria tenella sporozoites in vitro. J. Parasitol. 69, 666–670. doi: 10.2307/3281137
Smith, C. K., Galloway, R. B., and White, S. L. (1981). Effect of ionophores on survival, penetration, and development of Eimeria tenella sporozoites in vitro. J. Parasitol. 67, 511–516. doi: 10.2307/3280482
Sørum, M., Johnsen, P. J., Aasnes, B., Rosvoll, T., Kruse, H., Sundsfjord, A., et al. (2006). Prevalence, persistence, and molecular characterization of glycopeptide-resistant enterococci in Norwegian poultry and poultry farmers 3 to 8 years after the ban on avoparcin. Appl. Environ. Microbiol. 72, 516–521. doi: 10.1128/AEM.72.1.516-521.2006
SWEDRES/SVARM 2013 (2014). Use of antimicrobials and occurrence of antimicrobial resistance in Sweden. Solna/Uppsala ISSN 1650-6332, 1–115.
SWEDRES/SVARM 2016 (2017). Consumption of antibiotics and occurrence of antibiotic resistance in Sweden. Solna/Uppsala ISSN 1650-6332, 1–124.
SWEDRES/SVARM 2020 (2021). Sales of antibiotics and occurrence of antibiotic resistance in Sweden. Solna/Uppsala ISSN 1650-6332, 1–115.
SWEDRES-SVARM (2023). Sales of antibiotics and occurrence of antibiotic resistance in Sweden. Available at: https://www.sva.se/en/what-we-do/antibiotics/svarm-resistance-monitoring/swedres-svarm-reports/ (Accessed November 15, 2023).
Van Kessel, J. S., and Russell, J. B. (1992). Energetics of arginine and lysine transport by whole cells and membrane vesicles of strain SR, a monensin-sensitive ruminal bacterium. Appl. Environ. Microbiol. 58, 969–975. doi: 10.1128/aem.58.3.969-975.1992
Wang, H., Zhang, H., Zhu, Y., Wu, Z., Cui, C., and Cai, F. (2021). Anticancer mechanisms of Salinomycin in breast Cancer and its clinical applications. Front. Oncol. 11:654428. doi: 10.3389/fonc.2021.654428
Weaver, K. E., Kwong, S. M., Firth, N., and Francia, M. V. (2009). The RepA_N replicons of gram-positive bacteria: a family of broadly distributed but narrow host range plasmids. Plasmid 61, 94–109. doi: 10.1016/j.plasmid.2008.11.004
Keywords: antimicrobial resistance, polyether ionophore, one health, vancomycin resistance, anticoccidials, coccidiostats, growth promoters
Citation: Frederiksen RF, Slettemeås JS, Granstad S, Lagesen K, Pikkemaat MG, Urdahl AM and Simm R (2024) Polyether ionophore resistance in a one health perspective. Front. Microbiol. 15:1347490. doi: 10.3389/fmicb.2024.1347490
Received: 30 November 2023; Accepted: 09 January 2024;
Published: 29 January 2024.
Edited by:
Benoit Doublet, Institut National de recherche pour l’agriculture, l’alimentation et l’environnement (INRAE), FranceReviewed by:
Vittoria Mattioni Marchetti, University of Pavia, ItalyCopyright © 2024 Frederiksen, Slettemeås, Granstad, Lagesen, Pikkemaat, Urdahl and Simm. This is an open-access article distributed under the terms of the Creative Commons Attribution License (CC BY). The use, distribution or reproduction in other forums is permitted, provided the original author(s) and the copyright owner(s) are credited and that the original publication in this journal is cited, in accordance with accepted academic practice. No use, distribution or reproduction is permitted which does not comply with these terms.
*Correspondence: Roger Simm, cm9nZXIuc2ltbUBpYnYudWlvLm5v
Disclaimer: All claims expressed in this article are solely those of the authors and do not necessarily represent those of their affiliated organizations, or those of the publisher, the editors and the reviewers. Any product that may be evaluated in this article or claim that may be made by its manufacturer is not guaranteed or endorsed by the publisher.
Research integrity at Frontiers
Learn more about the work of our research integrity team to safeguard the quality of each article we publish.